- 1Department of Oncology, Tongji Hospital of Tongji Medical College, Huazhong University of Science and Technology, Wuhan, China
- 2Department of Breast Surgery, The First Affiliated Hospital, College of Medicine, Zhejiang University, Hangzhou, China
- 3Department of Gynecology, The Second Affiliated Hospital of Zhejiang University School of Medicine, Hangzhou, China
- 4Department of Urology, Institute of Urology, Tongji Hospital, Tongji Medical College, Huazhong University of Science and Technology, Wuhan, China
Transforming growth factor-β (TGF-β) signaling regulates multiple physiological processes, such as cell proliferation, differentiation, immune homeostasis, and wound healing. Besides, TGF-β plays a vital role in diseases, including cancer. Accumulating evidence indicates that TGF-β controls the composition and behavior of immune components in the tumor microenvironment (TME). Advanced cancers leverage TGF-β to reshape the TME and escape immune surveillance. TGF-β-mediated immune evasion is an unfavorable factor for cancer immunotherapy, especially immune checkpoint inhibitors (ICI). Numerous preclinical and clinical studies have demonstrated that hyperactive TGF-β signaling is closely associated with ICI resistance. It has been validated that TGF-β blockade synergizes with ICI and overcomes treatment resistance. TGF-β-targeted therapies, including trap and bispecific antibodies, have shown immense potential for cancer immunotherapy. In this review, we summarized the predictive value of TGF-β signaling and the prospects of TGF-β-targeted therapies for cancer immunotherapy.
1 Background
Transforming growth factor-β (TGF-β) exists in the extracellular matrix as latent precursors with prodomain, and the transformation from latent pro-TGF-β molecule to active TGF-β is a multiple-step process (1). Firstly, pro-TGF-β contains a long signal sequence, a long N-terminal sequence named latency-associated peptide (LAP), and a short C-terminal, which is the mature cytokine (2). Then, dimerized pro-TGF-β is cleaved by Furin (a protease) in Golgi complex. As a result, the bioactive TGF-β moieties are linked with LAP homodimer through disulfide bonds. The LAP encircles bioactive TGF-β moiety and hampers the binding of TGF-β with its receptor. After secretion, The LAP homodimer could anchor to Glycoprotein A repetitions predominant (GARP) on the cell surface or crosslink with the extracellular matrix by latent TGF-β binding proteins (LTBPs). Then, active TGF-β is released by integrin-transmitted forces when cell contraction (Figure 1) (4).
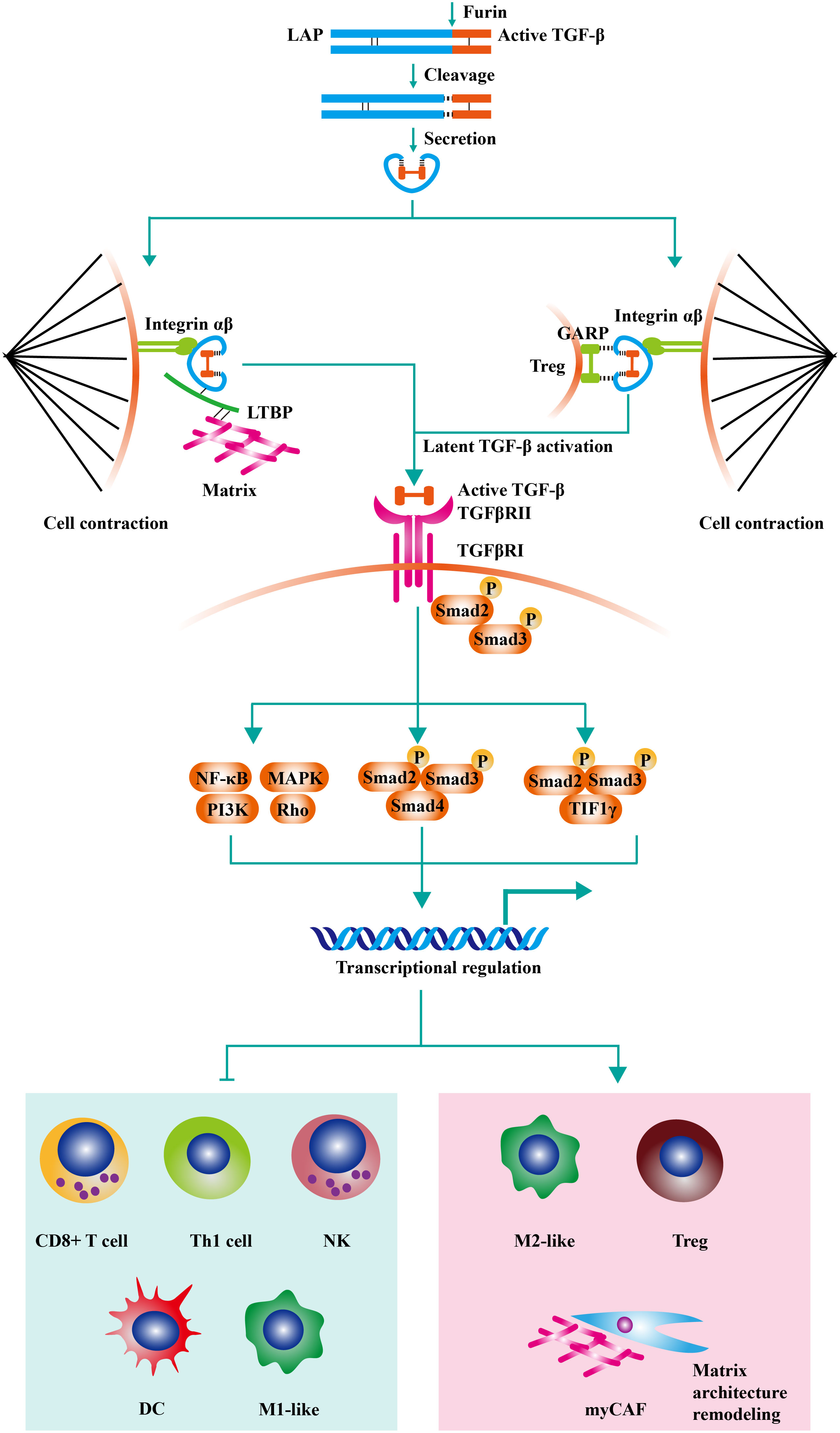
Figure 1 The negative effects of TGF-β signaling on anti-tumor immunity. Pro-TGF-β contains a long signal sequence, a long N-terminal sequence named latency-associated peptide (LAP), and a short C-terminal, which is the mature cytokine. Then, dimerized pro-TGF-β is cleaved by Furin (a protease) in Golgi complex. As a result, the bioactive TGF-β moieties are linked with LAP homodimer through disulfide bonds. The LAP encircles bioactive TGF-β moiety and hampers the binding of TGF-β with its receptor. After secretion, The LAP homodimer could anchor to Glycoprotein A repetitions predominant (GARP) on Treg or crosslink with extracellular matrix by latent TGF-β binding proteins (LTBPs). Then, active TGF-β is released by integrin-transmitted forces when cell contraction. TGF-β signaling is triggered by the interaction of TGF-β ligands with TGF-β type II receptors (TGFβRII). Following the recruitment and phosphorylation of TGF-β type I receptors (TGFβRI) by TGFβRII, SMAD2 and SMAD3 are phosphorylated and further assembled into trimeric complexes with SMAD4. The SMAD complexes could translocate into cell nucleus and regulate the expression of TGF-β-targeted genes. TGF-β acts on various immune cells in the tumor microenvironment, inducing the generation of a suppressive immune microenvironment. On the one hand, TGF-β inhibits the cytotoxic activity of CD8+ T cells, CD4+ T cells, and NK cells. On the other hand, TGF-β increases the proportion of regulatory T cells (Treg) and M2-like macrophage. Moreover, recent studies have found that TGF-β modulates the activity of tumor-associated fibroblast (CAF) and increases the content of collagen fibers in the tumor stroma (contributed mainly by myCAF). The thickened collagen fibers surrounding the tumor tissue are detrimental to lymphocyte infiltration, resulting in an immune-excluded tumor type. Adapted from Bai et al, 2019 (3).
TGF-β signaling is triggered by the interaction of TGF-β ligands with TGF-β type II receptors (TGFβRII) (5). Following the recruitment and phosphorylation of TGF-β type I receptors (TGFβRI) by TGFβRII, SMAD2 and SMAD3 are phosphorylated and further assembled into trimeric complexes with SMAD4 (6). The SMAD complexes could translocate into cell nucleus and regulate the expression of TGF-β-targeted genes, including TWIST1, SNAI1, and SNAI2 (7). Besides canonical SMAD signaling, TGF-β can initiate non-SMAD signalings, such as PI3K-AKT, MAPK, and RHO-ROCK pathways (8–10). TGF-β signaling plays a vital role in embryonic development and homeostasis by controlling cell proliferation, apoptosis, survival, differentiation, and stem-cell self-renewal (11).
TGF-β is a bifunctional cytokine in cancer, acting as tumor promoter and suppressor (12). For healthy cells and early-stage cancer cells, TGF-β inhibits tumorigenesis by inducing cell-cycle arrest (13). However, for late-stage cancers, cancer cells could bypass TGF-β-mediated apoptosis by mutating core components of TGF-β pathway (14). Contrarily, TGF-β promotes tumorigenesis by inducing epithelial-to-mesenchymal transition (EMT), eventually contributing to enhanced metastasis and chemoresistance (15–17). Besides, TGF-β also supports tumor progression by improving angiogenesis and immune evasion (4, 18). This transformation of TGF-β from tumor suppressor to tumor promoter is an important biological characteristic for advanced cancers (19).
The discovery of immune checkpoints and the development of drugs represented by programmed cell death protein 1/programmed cell death ligand 1 (PD-1/PD-L1) monoclonal antibodies are landmark events in cancer immunotherapy (20–24). Anti-PD-1/PD-L1 treatments have shown potent and sustained antitumor effects in patients across multiple cancer types (25–32). However, the low response rate is a crucial drawback of anti-PD-1/PD-L1 therapies, and ideal molecular markers are unavailable to select patients (33–35). The classical cancer-immunity cycle model describes antitumor immunity as a cascade of multistep cascade responses (36). PD-1/PD-L1 axis in the tumor is not the only immunosuppressive pathway (37). It has been shown that hyperactive TGF-β signaling in the tumor microenvironment (TME) can broadly modulate multiple immune cell activities, reshape the TME, and collectively participate in tumor cell immune escape (3). The TGF-β and PD-1/PD-L1 pathways are independent of and complementary to each other. Recent studies have shown that TGF-β is a determinant for anti-PD-1/PD-L1 therapies, which could effectively predict treatment efficacy (38–40). Therefore, constructing TGF-β-involved predictive biomarkers and exploring TGF-β-targeted therapies are valuable to cancer immunotherapy.
2 TGF-β signaling-targeted antitumor agents
Given that TGF-β contributes to cancer immune evasion and immunotherapy resistance, blocking TGF-β could overcome immunotherapy resistance by reprogramming the TME. At present, TGF-β signaling has been a hot therapeutic target for cancer investigators, and enormous efforts have been expended on the development of TGF-β-targeted agents (41). TGF-β blockade strategies, including monoclonal antibodies (containing bispecific antibodies), ligand traps (containing bi-functional proteins), receptor kinase inhibitors, vaccines, and antisense oligonucleotides, are under clinical evaluation (Table 1andFigure 2) (42).
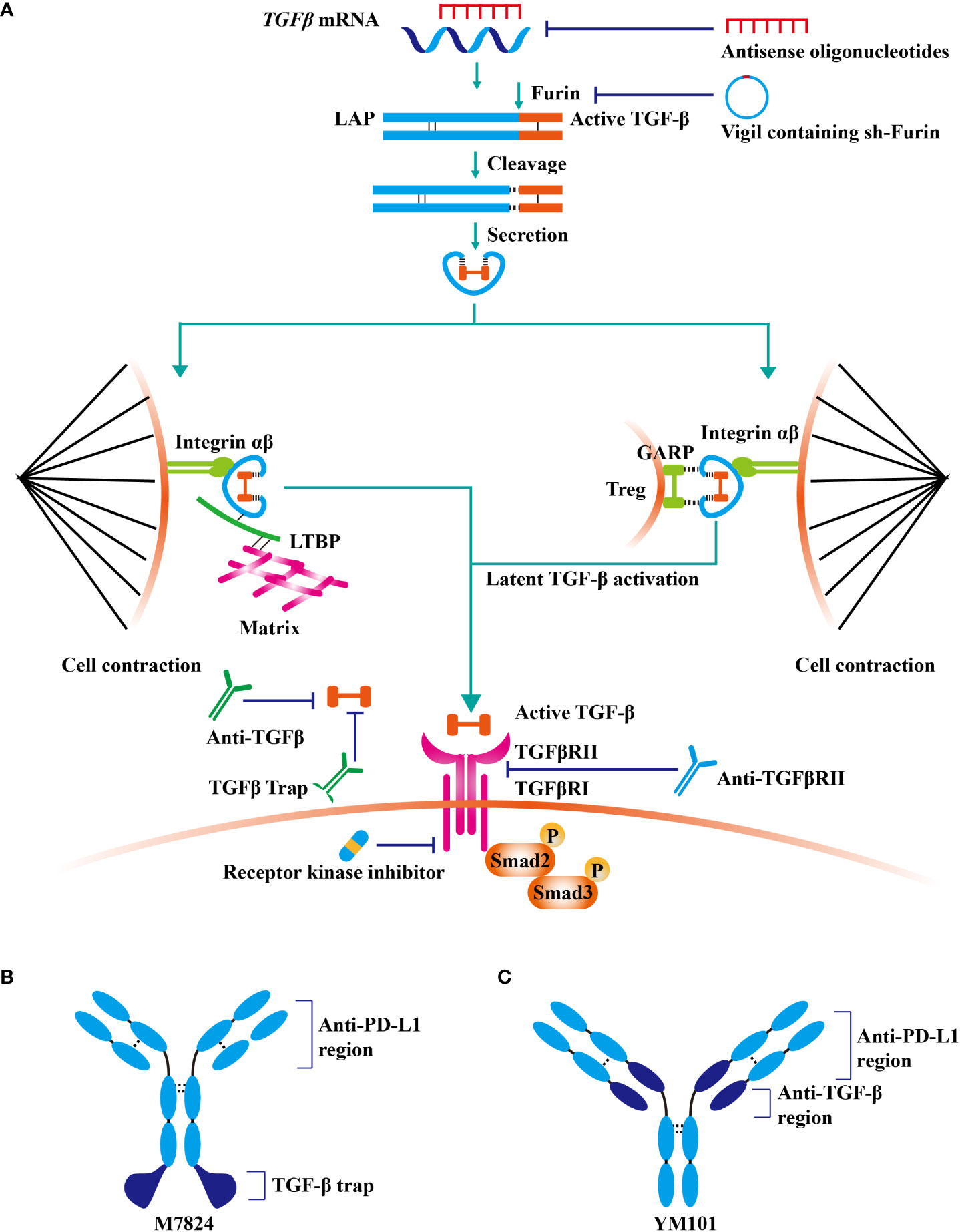
Figure 2 TGF-β signaling-targeted antitumor agents. At present, TGF-β signaling has been a hot therapeutic target for cancer investigators, and enormous efforts have been expended on the development of TGF-β-targeted agents. (A) TGF-β blockade strategies, including monoclonal antibodies (containing bispecific antibodies), ligand traps (containing bi-functional proteins), receptor kinase inhibitors, vaccines, and antisense oligonucleotides, are under clinical evaluation. (B) The structure of fusion protein M7824. (C) The structure of bispecific antibody YM101.
2.1 Antibodies targeting TGF-β or its receptor
Fresolimumab (also termed GC1008) is a pan-TGF-β blockade antibody developed by Genzyme for fibrotic diseases and cancers (43). Fresolimumab exhibited antitumor activity in renal cell carcinoma and melanoma with acceptable safety (43). Besides, in metastatic breast cancer, 10 mg/kg fresolimumab combined with irradiation outperformed 1 mg/kg fresolimumab plus irradiation in overall survival (Hazard ratio =2.73, P = 0.039) (44). The higher dose of fresolimumab was correlated with increased peripheral blood mononuclear cell and expanded CD8 memory T cell pool (44). Additionally, pan-TGF-β blockade antibodies 1D11 (developed by Genzyme) and 2G7 (developed by Genentech) exhibited antitumor activity in preclinical studies (45, 46). Notably, selective anti-TGF-β1 antibody SRK181 was sufficient to relieve the resistance to immune checkpoint inhibitors in murine models (47).
Y3022859 is an IgG1 antibody targeting TGFβRII (developed by Eli Lilly). In the phase 1 study of advanced solid tumors, the dose of more than 25 mg was unsafe in consideration of cytokine storm (48). Besides, anti-αvβ6 integrin antibody 264RAD (developed by AstraZeneca) could suppress TGF-β signaling by inhibiting latent TGF-β activation. The antitumor effect of 264RAD has been validated in multiple murine tumor models (49–51). Moreover, GARP, a protein mainly expressed on Treg surface, acts as the docking receptor to concentrate latent TGF-β (52). Selectively inhibiting GARP on Treg by antibody targeting GARP-TGF-β1 complexes effectively retarded tumor growth and relieved resistance to anti-PD-1/PD-L1 resistance (53). Notably, YM101 is an anti-PD-L1/TGF-β bispecific antibody (developed by Yi et al), which could simultaneously suppress PD-L1 and TGF-β signaling pathways (54). The preclinical data demonstrated YM101 effectively reprogrammed the TME and reserved immunotherapy resistance (54–56).
2.2 TGF-β receptor kinase inhibitor
TGF-β receptor kinase inhibitors block TGF-β signaling by occupying the ATP-binding domain of receptor (57). Vactosertib (developed by MedPacto) is a small-molecule inhibitor of TGFβRI (58). Vactosertib retarded tumor growth and prolonged survival in murine models by inhibiting EMT, cancer stemness, and metastasis (59–61). Also, galunisertib is a TGFβRI inhibitor developed by Eli Lilly (62, 63). Galunisertib showed potent antitumor activity in murine breast cancer, hepatocellular carcinoma, colon cancer, and lung cancer models (62). In clinical studies, galunisertib plus gemcitabine improved the overall survival of pancreatic cancer, relative to gemcitabine monotherapy (64). Besides, in the single-arm phase 2 trial of advanced rectal cancer, galunisertib combined with neoadjuvant chemoradiotherapy was tolerated, with an improved response rate (32%) (65). However, in the phase 2 study of recurrent glioblastoma, patients who received lomustine did not benefit from additional galunisertib treatment (66). Similarly, in a phase 1b study, galunisertib could not enhance the efficacy of ramucirumab in advanced hepatocellular carcinoma (67). LY573636 is a TGF-βRI inhibitor developed by Eli Lilly as well (68). Although several clinical trials showed that LY573636 had tolerable toxicity (69, 70), the results of the phase 2 study indicated that the autitumor effect of LY573636 was modest in NSCLC patients (71). At present, more than ten TGF-β receptor kinase inhibitors are in clinical or preclinical evaluations, including but not limited to LY2109761 (developed by Eli Lilly) (72), SB-431542 (developed by GlaxoSmithKline) (73), SB-505124 (developed by GlaxoSmithKline) (74), and IN-1130 (developed by In2Gen) (75, 76).
2.3 TGF-β trap
AVID200 (developed by Forbius/Bristol-Myers Squibb) is a computationally-designed trap that could effectively neutralize TGF-β1 and TGF-β3, with weak activity against TGF-β2 (77). The data of animal and human showed AVID200 enhanced antitumor immune response and reduced protumor and cardiotoxic effects caused by TGF-β2 blockade (77). Additionally, luspatercept (developed by Acceleron Pharma and Celgene) is a fusion protein containing the extracellular domain of human activin type 2B receptor and IgG, which has been approved as an erythroid maturation agent for β-thalassemia (78–80). Furthermore, soluble betaglycan (reported Bandyopadhyay et al.) inhibited angiogenesis, tumor growth, and metastasis in mice by antagonizing TGF-β (81).
M7824 (developed by Merck KGaA) is a bifunctional fusion protein consisting of anti-PD-L1 antibody and extracellular domain of the TGFβRII (82). M7824 showed potent antitumor activity in preclinical and phase 1 clinical studies by restoring antitumor immunity (82, 83). Similarly, anti-PD-L1/TGFβR fusion protein SHR-1701 (developed by Hengrui) overcame anti-PD-1/PD-L1 resistance in lung cancer (84).
2.4 Antisense oligonucleotides
Antisense oligonucleotides could directly silence genes participating in cancer progression. AP 12009 (developed by Antisense Pharma) is an antisense oligodeoxynucleotide targeting TGF-β2 (85). The data from phase IIb study of high-grade glioma demonstrated that 10 µM AP 12009 improved patients’ overall survival (86). Besides, other antisense oligonucleotides targeting TGF-β, such as AP 11014 and AP 15012, were still in preclinical tests (87, 88).
2.5 Cancer vaccine
Some cancer vaccines contain components suppressing TGF-β signaling pathway. Vigil (also termed gemogenovatucel-T, developed by Gradalis) is an autologous cancer vaccine that expresses granulocyte-macrophage colony-stimulating factor and decreases the expression of furin and its downstream TGF-β1 and TGF-β2 (89). In the phase 2b trial of advanced ovarian cancer, although vigil was well tolerated in patients, the primary endpoint was not met (90). Further investigations in other types of cancers are still undergoing (89). Moreover, Lucanix (also known as belagenpumatucel-L, developed by NovaRx) consists of allogeneic NSCLC cells transfected with the plasmid encoding TGF-β2 antisense gene (91, 92). In the phase III study NCT00676507, Lucanix improved the overall survival of NSCLC patients, especially these received prior chemotherapy or radiation (93).
3 Immune checkpoint inhibitor and its predictive biomarkers
PD-1/PD-L1 is an important signaling pathway to suppress immune responses and maintain autoimmune homeostasis (94, 95). However, in the TME, the hyperactive PD-1/PD-L1 pathway inhibits immune surveillance. It is traditionally believed that PD-L1, which is highly expressed on tumor cells, binds to PD-1 on the surface of T cells and suppresses the activity of T cells (96). PD-1/PD-L1 monoclonal antibody rescues T cells and restores antitumor immunity by blocking this negative immunomodulatory signal (97, 98). Recent studies have found that anti-PD-L1 antibodies also activate dendritic cells (DC) (99) and natural killer (NK) cells (100). Although PD-1/PD-L1 monoclonal antibodies are approved for the treatment of various cancers and have shown promising results in some patients, the problem of low objective response rates has not been effectively addressed (82, 101, 102). Therefore, screening for molecular biomarkers adapted to PD-1/PD-L1 therapy is an urgent issue at the present stage.
In terms of clinical efficacy, PD-L1 expression could not predict patient outcomes well, and even some patients whose tumors do not express PD-L1 can benefit from anti-PD-1/PD-L1 treatment (103–105). Apart from PD-L1 level, other predictive biomarkers have been identified, including tumor mutational burden (TMB) (106), mismatch repair (MMR) deficiency (107), the status of tumor-infiltrating lymphocyte (TIL) (108), immunosuppressive cell populations (109), oncogenic driver mutations (110–112), neoantigen repertoire (113), gut microbiota (114–116), inflammation-related genes (117, 118), extracellular vesicles (119), and patient’s clinical characteristics (120).
4 The role of TGF-β in cancer immunology and immunotherapy
High TGF-β in tumor tissues is mainly produced by tumor cells and mesenchymal cells. TGF-β promotes EMT of tumor cells and acts on various immune cells in the TME, inducing the generation of a suppressive immune microenvironment (121). On the one hand, TGF-β inhibits the cytotoxic activity of CD8+ T cells, CD4+ T cells, and NK cells. On the other hand, TGF-β increases the proportion of regulatory T cells (Treg) and myeloid-derived suppressor cells (MDSC) (122–125). Moreover, recent studies have found that TGF-β modulates the activity of tumor-associated fibroblast (CAF) and increases the content of collagen fibers in the tumor stroma (126). The thickened collagen fibers surrounding the tumor tissue are detrimental to lymphocyte infiltration, resulting in an immune-excluded tumor type (126). It is generally believed that this type of tumor does not respond to anti-PD-1/PD-L1 therapy, while antagonizing the TGF-β signaling pathway significantly improves anti-PD-1/PD-L1 therapeutic resistance and enhances the effect of antitumor immunotherapy (53, 127). Actually, although CAF was broadly classified into myofibroblastic (myCAF) and inflammatory and growth factor-enriched subgroups, some specific phenotypes are validated to participate in tumor progression as well (128). Besides, Grauel et al. found that TGF-β blockade induced the differentiation of IFN-licensed CAF, enhanced T cell recruitment and infiltration, and improved the effect of anti-PD-1 (129). Moreover, Krishnamurty identified a TGF-β-dependent CAF cluster with highly expressed LRRC15, which could support tumor progression by limiting T cell activity. Abrogating LRRC15+ CAF also significantly enhanced the efficacy of anti-PD-1 in mouse models (130).
Microsatellite-stable (MSS) colorectal cancer (CRC) is generally regarded as the cold tumor with poor immunogenicity and scare immune cell infiltration, which is unlikely to benefit from anti-PD-1/PD-L1 (131). However, this type of CRC could be conquered by the combination of anti-TGF-β and anti-PD-1/PD-L1 (132). Tauriello et al. established a metastatic CRC model by genetically engineering Apc, Kras, Tgfbr2, and Trp53 quadruple mutant mice (132). Metastatic cancer tissues display characteristics of human MSS CRC: low mutation burden, T cell depletion, and TGF-β activation (132). Normal intestinal mucosa and adenoma had T cell infiltration in the mesenchyme, but not in adjacent cancer tissue (132). Anti-PD-1/PD-L1 treatment had limited effects on these tumors, while TGF-β inhibitors increased the sensitivity of anti-PD-1/PD-L1 treatment (132). Further investigations showed that combination therapy upregulated T-bet and IFN-γ levels in CD4+ Th1 cells and increased GZMB generation in CTLs, eventually eradicating metastases and prolonging survival (132). The results support that the TME with hyperactive TGF-β signaling caused T cell depletion and a decrease in Th1 effector cells, leading to cancer immune escape (132).
Besides, Mariathasan et al. analyzed cancer tissues from patients with metastatic urothelial carcinoma receiving anti-PD-L1 treatment (126). The responders were characterized by high PD-L1 expression, high tumor mutation burden/neoantigen, and CD8+ effector T cells (126). The non-responders had tumor tissue containing dense mesenchymal stroma, CAF with high TGF-β activity, and T cell deficiency (126). The mouse breast cancer EMT-6 model mimicked the phenotype of epithelial carcinoma, where blocking either PD-L1 or TGF-β alone was ineffective (126). Combined inhibition of TGF-β and PD-1 signaling reduces TGF-β activity in stromal cells, promotes T cell infiltration into the tumor, stimulates a robust immune response, and leads to tumor regression (126). In conclusion, several studies have shown that TGF-β pathway activity is hyperactivated in anti-PD-1/PD-L1-resistant tumor tissues (3). The high expression of TGF-β in the TME suppresses the antitumor immune response (3). The immunosuppressive mechanisms of TGF-β and PD-1/PD-L1 pathways on tumors are independent and complementary, promoting the escape from immune surveillance (36).
5 The predictive value of TGF-β signaling for anti-PD-1/PD-L1 treatment
In parallel with the immunosuppressive role of TGF-β in cancer immunology, the predictive value of TGF-β signaling in anti-PD-1/PD-L1 therapies has been well documented in multiple clinical studies. In the single-arm phase 2 study NCT02662309, 95 muscle-invasive urothelial cancer patients were recruited and received anti-PD-L1 treatment before cystectomy (38). In this study, the presence of preexisting activated CD8+ T cells (dual CD8 and GZMB positive staining) in the tumor was closely correlated with patient outcomes. Moreover, FAP, the surrogate biomarker of CAF, was upregulated in relapsing tumor tissues but was downregulated in responders (38). Notably, the signatures of cytotoxic T cell and TGF-β signaling could also effectively predict treatment response to atezolizumab (38). In addition, in the single-institutional phase 2 trial NCT02658019 for advanced hepatocellular carcinoma (HCC), patients with low plasma TGF-β (< 200 pg/ml) at baseline had improved OS and PFS after anti-PD-1 treatment (39). Also, in non-small cell lung cancer (NSCLC), TGF-β concentration in the plasma collected seven days after anti-PD-1 treatment effectively predicted patient outcomes (133).
Transcriptomic data of microsatellite instability-high/mismatch repair-deficient gastrointestinal tumors showed TGF-β, EMT, Wnt/β-catenin, angiogenesis, hypoxia, KRAS, mTORC1, and metabolism-associated pathways were enriched in non-responders after PD-1 treatment (40). Similarly, the transcriptomic profile of metastatic bone and soft tissue sarcomas demonstrated that TGF-β signaling enrichment was negatively correlated with the efficacy of anti-PD-1 (134). Furthermore, the TGF-β signature (based on mRNA levels of BMPR2, FKBP1A, SLC20A1, SKIL, TGFBR1, and XIAP) predicted anti-PD-1/PD-L1 resistance in gynecologic cancer (135). The high TGF-β score was associated with shorter progression-free survival after immunotherapy (8.1 vs. 2.8 months, P < 0.05) (135). Additionally, for triple-negative breast cancer receiving Durvalumab with Nab-Paclitaxel, RNA-seq data showed that EMT, TGF-β, and extracellular matrix pathways were enriched in patients with residual disease (136).
6 TGF-β blockade enhancing the efficacy of anti-PD-1/PD-L1 therapy
Given the negative role of TGF-β signaling in cancer immunology and immunotherapy, it is rational to enhance ICI efficacy by blocking TGF-β. In preclinical explorations and clinical practice, combination therapies of TGF-β inhibitor and anti-PD-1/PD-L1, as well as anti-PD-L1/TGF-β bispecific antibodies/fusion proteins, have made rapid progress (137).
6.1 TGF-β inhibitor combined with anti-PD-1/PD-L1
The synergistic effect between TGF-β inhibitor (e.g. anti-TGF-β, receptor kinase inhibitor, cancer vaccine) and anti-PD-1/PD-L1 has been validated in multiple murine tumor models, including but not limited to CT26 (mouse colon cancer), MC38 (mouse colon cancer), 3LL (mouse Lewis lung cancer), and EMT-6 (mouse breast cancer) (47, 54, 138, 139). Mechanistically, the combination therapy reverses TGF-β-mediated immune exclusion, enhances immune infiltration, improves the activities of effectors, and alters the polarization of macrophages (140).
In the advanced NSCLC patients, the interim results of NCT03732274 showed that galunisertib (TGFβRI kinase inhibitor) combined with durvalumab (anti-PD-L1) had potent antitumor activity with a manageable safety profile (response rate: 30.8% for PD-L1≥1% tumors; response rate: 40.0% for PDL1≥25%) (141). However, in the single-arm, multicenter, phase Ib study NCT02734160, galunisertib plus durvalumab was tolerable in metastatic pancreatic cancer, in spite of the limited antitumor activity (142).
6.2 Anti-PD-L1/TGF-β bispecific antibody or bi-functional protein
Actually, most PD-1/PD-L1 and TGF-β dual blockade strategies in clinical practice are fulfilled by anti-PD-L1/TGF-β bispecific antibody or bi-functional protein, which has strategic advantages over the conventional two-agent combination. More importantly, due to the unique structure, bispecific antibodies or bi-functional proteins might have better tumor specificity and therapeutic effects (54, 82, 143). M7824 (fusion protein containing anti-PD-L1 and TGF-β trap) outperformed anti-PD-L1 and TGF-β trap in preclinical studies by mobilizing antitumor immunity (82, 144). Notably, in the phase 1 study NCT02517398, the response rate in NSCLC patients with high PD-L1 expression was high as 85.7% (83). Besides, the results of other early-stage clinical trials were encouraging as well (145). At present, the efficacy of M7824 is under evaluation in more than ten types of cancers, including NSCLC, triple-negative breast cancer, urothelial carcinoma, biliary tract cancer, gastric cancer, HPV-associated malignancies, and thymic carcinoma. Similarly, SHR-1701 (fusion protein of anti-PD-L1 antibody and TGF-β trap) exhibited encouraging antitumor activity in advanced tumors in the phase 1 study NCT03710265 (response rate: 17.8%) (146). Moreover, multiple phase 1/2 studies demonstrated the powerful antitumor activity of SHR-1701 in cervical cancer, EGFR-mutated NSCLC, biliary tract cancer, and pancreatic cancer (147–150)
YM101 is the first publicly reported anti-PD-L1/TGF-β bispecific antibody in the world (54). In the preclinical studies, YM101 overcame anti-PD-L1 resistance in 3LL, CT26, and EMT-6 tumor models (54). Investigations in the TME showed that YM101 expanded the numbers of TIL, M1-like macrophage, and DC, but decreased M2-like macrophage (54). The surrogate of YM101, Y101D is under evaluation in advanced solid tumors (NCT05028556).
7 Conclusions
TGF-β is a paradoxical regulator in cancer progression, which acts as a suppressor in early-stage cancer but as a promoter in advanced cancer. The negative effects of TGF-β on cancer immune surveillance have been well studied, including impairing immune infiltration, inducing the differentiation toward MDSC/M2-like macrophage/Treg, limiting the cytotoxicity of T cell and NK cell, and undermining the antigen presentation capability of DC. Accumulating evidence shows that TGF-β not only promotes cancer immune evasion but also predicts the efficacy of immune checkpoint inhibitors. Increased TGF-β level at baseline is commonly associated with a poor response to anti-PD-1/PD-L1 therapy. Blocking TGF-β could improve response to anti-PD-1/PD-L1 and patient outcomes. At present, dual PD-1/PD-L1 and TGF-β blockade have made a breakthrough, especially by anti-PD-L1/TGF-β bispecific antibody or bi-functional protein. This updated immune checkpoint inhibitor might alter the therapeutic paradigm for cancer in the future.
Author contributions
MY and TL performed the selection of literature, drafted the manuscript and prepared the figures. MN and YW collected the related references and participated in discussion. KW and ZZ designed this review and revised the manuscript. All authors contributed to the article and approved the submitted version.
Funding
This work was supported by the National Natural Science Foundation of China (Nos. 82073370, 82272794, and 81874120) and China Postdoctoral Science Foundation (No. 2022M722766).
Conflict of interest
The authors declare that the research was conducted in the absence of any commercial or financial relationships that could be construed as a potential conflict of interest.
Publisher’s note
All claims expressed in this article are solely those of the authors and do not necessarily represent those of their affiliated organizations, or those of the publisher, the editors and the reviewers. Any product that may be evaluated in this article, or claim that may be made by its manufacturer, is not guaranteed or endorsed by the publisher.
Abbreviations
CAF, carcinoma-associated fibroblast; CRC, colorectal cancer; DC, dendritic cell; EMT, epithelial-mesenchymal transition; GARP, Glycoprotein A repetitions predominant; HCC, hepatocellular carcinoma; ICI, immune checkpoint inhibitor; LAP, latency-associated peptide; LTBP, latent TGF-β binding protein; MDSC: myeloid-derived suppressor cell; MSS: microsatellite-stable; MMR, mismatch repair; NK, natural killer; NSCLC, non-small cell lung cancer; PD-1, programmed cell death protein 1; TGF-β, transforming growth factor-beta; TGFβRI, TGF-β type I receptor; TGFβRII, TGF-β type II receptor; TMB, tumor mutational burden; TME, tumor microenvironment; TIL, tumor-infiltrating lymphocyte; Treg, regulatory T cell.
References
1. Aubert A, Mercier-Gouy P, Aguero S, Berthier L, Liot S, Prigent L, et al. Latent TGF-β activation is a hallmark of the tenascin family. Front Immunol (2021) 12:613438. doi: 10.3389/fimmu.2021.613438
2. Travis MA, Sheppard D. TGF-β activation and function in immunity. Annu Rev Immunol (2014) 32:51–82. doi: 10.1146/annurev-immunol-032713-120257
3. Bai X, Yi M, Jiao Y, Chu Q, Wu K. Blocking TGF-β signaling to enhance the efficacy of immune checkpoint inhibitor. Onco Targets Ther (2019) 12:9527–38. doi: 10.2147/OTT.S224013
4. Batlle E, Massagué J. Transforming growth factor-β signaling in immunity and cancer. Immunity. (2019) 50:924–40. doi: 10.1016/j.immuni.2019.03.024
5. Hata A, Chen YG. TGF-β signaling from receptors to smads. Cold Spring Harb Perspect Biol (2016) 8:a022061. doi: 10.1101/cshperspect.a022061
6. Derynck R, Budi EH. Specificity, versatility, and control of TGF-β family signaling. Sci Signal (2019) 12:eaav5183. doi: 10.1126/scisignal.aav5183
7. Meng XM, Nikolic-Paterson DJ, Lan HY. TGF-β: the master regulator of fibrosis. Nat Rev Nephrol. (2016) 12:325–38. doi: 10.1038/nrneph.2016.48
8. Colak S, Ten Dijke P. Targeting TGF-β signaling in cancer. Trends Cancer. (2017) 3:56–71. doi: 10.1016/j.trecan.2016.11.008
9. Zhang YE. Non-smad signaling pathways of the TGF-β family. Cold Spring Harb Perspect Biol (2017) 9:a022129. doi: 10.1101/cshperspect.a022129
10. Derynck R, Zhang YE. Smad-dependent and smad-independent pathways in TGF-beta family signalling. Nature. (2003) 425:577–84. doi: 10.1038/nature02006
11. Zi Z. Molecular engineering of the TGF-β signaling pathway. J Mol Biol (2019) 431:2644–54. doi: 10.1016/j.jmb.2019.05.022
12. Seoane J, Gomis RR. TGF-β family signaling in tumor suppression and cancer progression. Cold Spring Harb Perspect Biol (2017) 9:a022277. doi: 10.1101/cshperspect.a022277
13. Yu Y, Feng XH. TGF-β signaling in cell fate control and cancer. Curr Opin Cell Biol (2019) 61:56–63. doi: 10.1016/j.ceb.2019.07.007
14. Zhao M, Mishra L, Deng CX. The role of TGF-β/SMAD4 signaling in cancer. Int J Biol Sci (2018) 14:111–23. doi: 10.7150/ijbs.23230
15. Lamouille S, Xu J, Derynck R. Molecular mechanisms of epithelial-mesenchymal transition. Nat Rev Mol Cell Biol (2014) 15:178–96. doi: 10.1038/nrm3758
16. Chandra Jena B, Sarkar S, Rout L, Mandal M. The transformation of cancer-associated fibroblasts: Current perspectives on the role of TGF-β in CAF mediated tumor progression and therapeutic resistance. Cancer Lett (2021) 520:222–32. doi: 10.1016/j.canlet.2021.08.002
17. Wang X, Thiery JP. Harnessing carcinoma cell plasticity mediated by TGF-β signaling. Cancers (Basel). (2021) 13:3397. doi: 10.3390/cancers13143397
18. Miyazono K, Katsuno Y, Koinuma D, Ehata S, Morikawa M. Intracellular and extracellular TGF-β signaling in cancer: some recent topics. Front Med (2018) 12:387–411. doi: 10.1007/s11684-018-0646-8
19. Fleming NI, Jorissen RN, Mouradov D, Christie M, Sakthianandeswaren A, Palmieri M, et al. SMAD2, SMAD3 and SMAD4 mutations in colorectal cancer. Cancer Res (2013) 73:725–35. doi: 10.1158/0008-5472.CAN-12-2706
20. Dong H, Strome SE, Salomao DR, Tamura H, Hirano F, Flies DB, et al. Tumor-associated B7-H1 promotes T-cell apoptosis: a potential mechanism of immune evasion. Nat Med (2002) 8:793–800. doi: 10.1038/nm730
21. Chen L, Han X. Anti-PD-1/PD-L1 therapy of human cancer: past, present, and future. J Clin Invest. (2015) 125:3384–91. doi: 10.1172/JCI80011
22. Marin-Acevedo JA, Kimbrough EO, Manochakian R, Zhao Y, Lou Y. Immunotherapies targeting stimulatory pathways and beyond. J Hematol Oncol (2021) 14:78. doi: 10.1186/s13045-021-01085-3
23. Qin S, Xu L, Yi M, Yu S, Wu K, Luo S. Novel immune checkpoint targets: moving beyond PD-1 and CTLA-4. Mol Cancer. (2019) 18:155. doi: 10.1186/s12943-019-1091-2
24. Salik B, Smyth MJ, Nakamura K. Targeting immune checkpoints in hematological malignancies. J Hematol Oncol (2020) 13:111. doi: 10.1186/s13045-020-00947-6
25. Topalian SL, Hodi FS, Brahmer JR, Gettinger SN, Smith DC, McDermott DF, et al. Safety, activity, and immune correlates of anti-PD-1 antibody in cancer. N Engl J Med (2012) 366:2443–54. doi: 10.1056/NEJMoa1200690
26. Cercek A, Lumish M, Sinopoli J, Weiss J, Shia J, Lamendola-Essel M, et al. PD-1 blockade in mismatch repair-deficient, locally advanced rectal cancer. N Engl J Med (2022) 386:2363–76. doi: 10.1056/NEJMoa2201445
27. Ansell SM, Lesokhin AM, Borrello I, Halwani A, Scott EC, Gutierrez M, et al. PD-1 blockade with nivolumab in relapsed or refractory hodgkin's lymphoma. N Engl J Med (2015) 372:311–9. doi: 10.1056/NEJMoa1411087
28. Nghiem PT, Bhatia S, Lipson EJ, Kudchadkar RR, Miller NJ, Annamalai L, et al. PD-1 blockade with pembrolizumab in advanced merkel-cell carcinoma. N Engl J Med (2016) 374:2542–52. doi: 10.1056/NEJMoa1603702
29. Tang B, Yan X, Sheng X, Si L, Cui C, Kong Y, et al. Safety and clinical activity with an anti-PD-1 antibody JS001 in advanced melanoma or urologic cancer patients. J Hematol Oncol (2019) 12:7. doi: 10.1186/s13045-018-0693-2
30. Borghaei H, Paz-Ares L, Horn L, Spigel DR, Steins M, Ready NE, et al. Nivolumab versus docetaxel in advanced nonsquamous non-Small-Cell lung cancer. N Engl J Med (2015) 373:1627–39. doi: 10.1056/NEJMoa1507643
31. Hamanishi J, Mandai M, Ikeda T, Minami M, Kawaguchi A, Murayama T, et al. Safety and antitumor activity of anti-PD-1 antibody, nivolumab, in patients with platinum-resistant ovarian cancer. J Clin Oncol (2015) 33:4015–22. doi: 10.1200/JCO.2015.62.3397
32. Yang Y, Li Y, Gu H, Dong M, Cai Z. Emerging agents and regimens for multiple myeloma. J Hematol Oncol (2020) 13:150. doi: 10.1186/s13045-020-00980-5
33. Yi M, Jiao D, Xu H, Liu Q, Zhao W, Han X, et al. Biomarkers for predicting efficacy of PD-1/PD-L1 inhibitors. Mol Cancer. (2018) 17:129. doi: 10.1186/s12943-018-0864-3
34. Yi M, Zheng X, Niu M, Zhu S, Ge H, Wu K. Combination strategies with PD-1/PD-L1 blockade: current advances and future directions. Mol Cancer. (2022) 21:28. doi: 10.1186/s12943-021-01489-2
35. Darvin P, Toor SM, Sasidharan Nair V, Elkord E. Immune checkpoint inhibitors: recent progress and potential biomarkers. Exp Mol Med (2018) 50:1–11. doi: 10.1038/s12276-018-0191-1
36. Chen DS, Mellman I. Oncology meets immunology: the cancer-immunity cycle. Immunity. (2013) 39:1–10. doi: 10.1016/j.immuni.2013.07.012
37. Chen DS, Mellman I. Elements of cancer immunity and the cancer-immune set point. Nature. (2017) 541:321–30. doi: 10.1038/nature21349
38. Powles T, Kockx M, Rodriguez-Vida A, Duran I, Crabb SJ, van der Heijden MS, et al. Clinical efficacy and biomarker analysis of neoadjuvant atezolizumab in operable urothelial carcinoma in the ABACUS trial. Nat Med (2019) 25:1706–14. doi: 10.1038/s41591-019-0628-7
39. Feun LG, Li YY, Wu C, Wangpaichitr M, Jones PD, Richman SP, et al. Phase 2 study of pembrolizumab and circulating biomarkers to predict anticancer response in advanced, unresectable hepatocellular carcinoma. Cancer. (2019) 125:3603–14. doi: 10.1002/cncr.32339
40. Chida K, Kawazoe A, Suzuki T, Kawazu M, Ueno T, Takenouchi K, et al. Transcriptomic profiling of MSI-H/dMMR gastrointestinal tumors to identify determinants of responsiveness to anti-PD-1 therapy. Clin Cancer Res (2022) 28:2110–7. doi: 10.1158/1078-0432.CCR-22-0041
41. Huang CY, Chung CL, Hu TH, Chen JJ, Liu PF, Chen CL. Recent progress in TGF-β inhibitors for cancer therapy. BioMed Pharmacother. (2021) 134:111046. doi: 10.1016/j.biopha.2020.111046
42. Kim BG, Malek E, Choi SH, Ignatz-Hoover JJ, Driscoll JJ. Novel therapies emerging in oncology to target the TGF-β pathway. J Hematol Oncol (2021) 14:55. doi: 10.1186/s13045-021-01053-x
43. Morris JC, Tan AR, Olencki TE, Shapiro GI, Dezube BJ, Reiss M, et al. Phase I study of GC1008 (fresolimumab): a human anti-transforming growth factor-beta (TGFβ) monoclonal antibody in patients with advanced malignant melanoma or renal cell carcinoma. PloS One (2014) 9:e90353. doi: 10.1371/journal.pone.0090353
44. Formenti SC, Lee P, Adams S, Goldberg JD, Li X, Xie MW, et al. Focal irradiation and systemic TGFβ blockade in metastatic breast cancer. Clin Cancer Res (2018) 24:2493–504. doi: 10.1158/1078-0432.CCR-17-3322
45. Qin F, Liu X, Chen J, Huang S, Wei W, Zou Y, et al. Anti-TGF-β attenuates tumor growth via polarization of tumor associated neutrophils towards an anti-tumor phenotype in colorectal cancer. J Cancer. (2020) 11:2580–92. doi: 10.7150/jca.38179
46. Arteaga CL, Hurd SD, Winnier AR, Johnson MD, Fendly BM, Forbes JT. Anti-transforming growth factor (TGF)-beta antibodies inhibit breast cancer cell tumorigenicity and increase mouse spleen natural killer cell activity. implications for a possible role of tumor cell/host TGF-beta interactions in human breast cancer progression. J Clin Invest. (1993) 92:2569–76. doi: 10.1172/JCI116871
47. Martin CJ, Datta A, Littlefield C, Kalra A, Chapron C, Wawersik S, et al. Selective inhibition of TGFβ1 activation overcomes primary resistance to checkpoint blockade therapy by altering tumor immune landscape. Sci Transl Med (2020) 12:eaay8456. doi: 10.1126/scitranslmed.aay8456
48. Tolcher AW, Berlin JD, Cosaert J, Kauh J, Chan E, Piha-Paul SA, et al. A phase 1 study of anti-TGFβ receptor type-II monoclonal antibody LY3022859 in patients with advanced solid tumors. Cancer Chemother Pharmacol (2017) 79:673–80. doi: 10.1007/s00280-017-3245-5
49. Eberlein C, Kendrew J, McDaid K, Alfred A, Kang JS, Jacobs VN, et al. A human monoclonal antibody 264RAD targeting αvβ6 integrin reduces tumour growth and metastasis, and modulates key biomarkers in vivo. Oncogene (2013) 32:4406–16. doi: 10.1038/onc.2012.460
50. Reader CS, Vallath S, Steele CW, Haider S, Brentnall A, Desai A, et al. The integrin αvβ6 drives pancreatic cancer through diverse mechanisms and represents an effective target for therapy. J Pathol (2019) 249:332–42. doi: 10.1002/path.5320
51. Moore KM, Thomas GJ, Duffy SW, Warwick J, Gabe R, Chou P, et al. Therapeutic targeting of integrin αvβ6 in breast cancer. J Natl Cancer Inst (2014) 106:dju169. doi: 10.1093/jnci/dju169
52. Zimmer N, Trzeciak ER, Graefen B, Satoh K, Tuettenberg A. GARP as a therapeutic target for the modulation of regulatory T cells in cancer and autoimmunity. Front Immunol (2022) 13:928450. doi: 10.3389/fimmu.2022.928450
53. de Streel G, Bertrand C, Chalon N, Liénart S, Bricard O, Lecomte S, et al. Selective inhibition of TGF-β1 produced by GARP-expressing tregs overcomes resistance to PD-1/PD-L1 blockade in cancer. Nat Commun (2020) 11:4545. doi: 10.1038/s41467-020-17811-3
54. Yi M, Zhang J, Li A, Niu M, Yan Y, Jiao Y, et al. The construction, expression, and enhanced anti-tumor activity of YM101: a bispecific antibody simultaneously targeting TGF-β and PD-L1. J Hematol Oncol (2021) 14:27. doi: 10.1186/s13045-021-01045-x
55. Yi M, Niu M, Zhang J, Li S, Zhu S, Yan Y, et al. Combine and conquer: manganese synergizing anti-TGF-β/PD-L1 bispecific antibody YM101 to overcome immunotherapy resistance in non-inflamed cancers. J Hematol Oncol (2021) 14:146. doi: 10.1186/s13045-021-01155-6
56. Yi M, Niu M, Wu Y, Ge H, Jiao D, Zhu S, et al. Combination of oral STING agonist MSA-2 and anti-TGF-β/PD-L1 bispecific antibody YM101: a novel immune cocktail therapy for non-inflamed tumors. J Hematol Oncol (2022) 15:142. doi: 10.1186/s13045-022-01363-8
57. Huynh LK, Hipolito CJ, Ten Dijke P. A perspective on the development of TGF-β inhibitors for cancer treatment. Biomolecules (2019) 9:743. doi: 10.3390/biom9110743
58. Song KM, Chung DY, Choi MJ, Ghatak K, Minh NN, Limanjaya A, et al. Vactosertib, a novel, orally bioavailable activin receptor-like kinase 5 inhibitor, promotes regression of fibrotic plaques in a rat model of peyronie's disease. World J Mens Health (2020) 38:552–63. doi: 10.5534/wjmh.190071
59. Choi J, Park J, Cho I, Sheen Y. Co-Treatment with vactosertib, a novel, orally bioavailable activin receptor-like kinase 5 inhibitor, suppresses radiotherapy-induced epithelial-to-mesenchymal transition, cancer cell stemness, and lung metastasis of breast cancer. Radiol Oncol (2022) 56:185–97. doi: 10.2478/raon-2022-0012
60. Hong E, Park S, Ooshima A, Hong CP, Park J, Heo JS, et al. Inhibition of TGF-β signalling in combination with nal-IRI plus 5-Fluorouracil/Leucovorin suppresses invasion and prolongs survival in pancreatic tumour mouse models. Sci Rep (2020) 10:2935. doi: 10.1038/s41598-020-59893-5
61. Park J, Choi J, Cho I, Sheen YY. Radiotherapy-induced oxidative stress and fibrosis in breast cancer are suppressed by vactosertib, a novel, orally bioavailable TGF-β/ALK5 inhibitor. Sci Rep (2022) 12:16104. doi: 10.1038/s41598-022-20050-9
62. Herbertz S, Sawyer JS, Stauber AJ, Gueorguieva I, Driscoll KE, Estrem ST, et al. Clinical development of galunisertib (LY2157299 monohydrate), a small molecule inhibitor of transforming growth factor-beta signaling pathway. Drug Des Devel Ther (2015) 9:4479–99. doi: 10.2147/DDDT.S86621
63. Rodon J, Carducci MA, Sepulveda-Sánchez JM, Azaro A, Calvo E, Seoane J, et al. First-in-human dose study of the novel transforming growth factor-β receptor I kinase inhibitor LY2157299 monohydrate in patients with advanced cancer and glioma. Clin Cancer Res (2015) 21:553–60. doi: 10.1158/1078-0432.CCR-14-1380
64. Melisi D, Garcia-Carbonero R, Macarulla T, Pezet D, Deplanque G, Fuchs M, et al. Galunisertib plus gemcitabine vs. gemcitabine for first-line treatment of patients with unresectable pancreatic cancer. Br J Cancer. (2018) 119:1208–14. doi: 10.1038/s41416-018-0246-z
65. Yamazaki T, Gunderson AJ, Gilchrist M, Whiteford M, Kiely MX, Hayman A, et al. Galunisertib plus neoadjuvant chemoradiotherapy in patients with locally advanced rectal cancer: a single-arm, phase 2 trial. Lancet Oncol (2022) 23:1189–200. doi: 10.1016/S1470-2045(22)00446-6
66. Brandes AA, Carpentier AF, Kesari S, Sepulveda-Sanchez JM, Wheeler HR, Chinot O, et al. A phase II randomized study of galunisertib monotherapy or galunisertib plus lomustine compared with lomustine monotherapy in patients with recurrent glioblastoma. Neuro Oncol (2016) 18:1146–56. doi: 10.1093/neuonc/now009
67. Harding JJ, Do RK, Yaqubie A, Cleverly A, Zhao Y, Gueorguieva I, et al. Phase 1b study of galunisertib and ramucirumab in patients with advanced hepatocellular carcinoma. Cancer Med (2021) 10:3059–67. doi: 10.1002/cam4.3880
68. Meier T, Uhlik M, Chintharlapalli S, Dowless M, Van Horn R, Stewart J, et al. Tasisulam sodium, an antitumor agent that inhibits mitotic progression and induces vascular normalization. Mol Cancer Ther (2011) 10:2168–78. doi: 10.1158/1535-7163.MCT-11-0323
69. Kirkwood JM, Gonzalez R, Reintgen D, Clingan PR, McWilliams RR, de Alwis DP, et al. A phase 2 study of tasisulam sodium (LY573636 sodium) as second-line treatment for patients with unresectable or metastatic melanoma. Cancer. (2011) 117:4732–9. doi: 10.1002/cncr.26068
70. Fujiwara Y, Ando Y, Mukohara T, Kiyota N, Chayahara N, Mitsuma A, et al. A phase I study of tasisulam sodium using an albumin-tailored dose in Japanese patients with advanced solid tumors. Cancer Chemother Pharmacol (2013) 71:991–8. doi: 10.1007/s00280-013-2092-2
71. Scagliotti GV, Ilaria R Jr., Novello S, von Pawel J, Fischer JR, Ermisch S, et al. Tasisulam sodium (LY573636 sodium) as third-line treatment in patients with unresectable, metastatic non-small-cell lung cancer: a phase-II study. J Thorac Oncol (2012) 7:1053–7. doi: 10.1097/JTO.0b013e3182519d79
72. Flechsig P, Dadrich M, Bickelhaupt S, Jenne J, Hauser K, Timke C, et al. LY2109761 attenuates radiation-induced pulmonary murine fibrosis via reversal of TGF-β and BMP-associated proinflammatory and proangiogenic signals. Clin Cancer Res (2012) 18:3616–27. doi: 10.1158/1078-0432.CCR-11-2855
73. Lee AJ, Mahoney CM, Cai CC, Ichinose R, Stefani RM, Marra KG, et al. Sustained delivery of SB-431542, a type I transforming growth factor beta-1 receptor inhibitor, to prevent arthrofibrosis. Tissue Eng Part A. (2021) 27:1411–21. doi: 10.1089/ten.tea.2021.0029
74. DaCosta Byfield S, Major C, Laping NJ, Roberts AB. SB-505124 is a selective inhibitor of transforming growth factor-beta type I receptors ALK4, ALK5, and ALK7. Mol Pharmacol (2004) 65:744–52. doi: 10.1124/mol.65.3.744
75. Moon JA, Kim HT, Cho IS, Sheen YY, Kim DK. IN-1130, a novel transforming growth factor-beta type I receptor kinase (ALK5) inhibitor, suppresses renal fibrosis in obstructive nephropathy. Kidney Int (2006) 70:1234–43. doi: 10.1038/sj.ki.5001775
76. Lee GT, Hong JH, Mueller TJ, Watson JA, Kwak C, Sheen YY, et al. Effect of IN-1130, a small molecule inhibitor of transforming growth factor-beta type I receptor/activin receptor-like kinase-5, on prostate cancer cells. J Urol. (2008) 180:2660–7. doi: 10.1016/j.juro.2008.08.008
77. O'Connor-McCourt MD, Tremblay G, Lenferink A, Sulea T, Zwaagstra J, Koropatnick J. Abstract 1759: AVID200, a highly potent TGF-beta trap, exhibits optimal isoform selectivity for enhancing anti-tumor T-cell activity, without promoting metastasis or cardiotoxicity. Cancer Res (2018) 78:1759. doi: 10.1158/1538-7445.AM2018-1759
78. Cappellini MD, Taher AT. The use of luspatercept for thalassemia in adults. Blood Adv (2021) 5:326–33. doi: 10.1182/bloodadvances.2020002725
79. Markham A. Luspatercept: First approval. Drugs. (2020) 80:85–90. doi: 10.1007/s40265-019-01251-5
80. Cappellini MD, Viprakasit V, Taher AT, Georgiev P, Kuo KHM, Coates T, et al. A phase 3 trial of luspatercept in patients with transfusion-dependent β-thalassemia. N Engl J Med (2020) 382:1219–31. doi: 10.1056/NEJMoa1910182
81. Bandyopadhyay A, López-Casillas F, Malik SN, Montiel JL, Mendoza V, Yang J, et al. Antitumor activity of a recombinant soluble betaglycan in human breast cancer xenograft. Cancer Res (2002) 62:4690–5. doi: 10.1002/cncr.10753
82. Lan Y, Zhang D, Xu C, Hance KW, Marelli B, Qi J, et al. Enhanced preclinical antitumor activity of M7824, a bifunctional fusion protein simultaneously targeting PD-L1 and TGF-β. Sci Transl Med (2018) 10:eaan5488. doi: 10.1126/scitranslmed.aan5488
83. Paz-Ares L, Kim TM, Vicente D, Felip E, Lee DH, Lee KH, et al. Bintrafusp Alfa, a bifunctional fusion protein targeting TGF-β and PD-L1, in second-line treatment of patients with NSCLC: Results from an expansion cohort of a phase 1 trial. J Thorac Oncol (2020) 15:1210–22. doi: 10.1016/j.jtho.2020.03.003
84. Cheng B, Ding K, Chen P, Ji J, Luo T, Guo X, et al. Anti-PD-L1/TGF-βR fusion protein (SHR-1701) overcomes disrupted lymphocyte recovery-induced resistance to PD-1/PD-L1 inhibitors in lung cancer. Cancer Commun (Lond). (2022) 42:17–36. doi: 10.1002/cac2.12244
85. Schlingensiepen KH, Schlingensiepen R, Steinbrecher A, Hau P, Bogdahn U, Fischer-Blass B, et al. Targeted tumor therapy with the TGF-beta 2 antisense compound AP 12009. Cytokine Growth Factor Rev (2006) 17:129–39. doi: 10.1016/j.cytogfr.2005.09.002
86. Bogdahn U, Hau P, Stockhammer G, Venkataramana NK, Mahapatra AK, Suri A, et al. Targeted therapy for high-grade glioma with the TGF-β2 inhibitor trabedersen: results of a randomized and controlled phase IIb study. Neuro Oncol (2011) 13:132–42. doi: 10.1093/neuonc/noq142
87. Schlingensiepen K-H, Bischof A, Egger T, Hafner M, Herrmuth H, Jachimczak P, et al. The TGF-beta1 antisense oligonucleotide AP 11014 for the treatment of non-small cell lung, colorectal and prostate cancer: Preclinical studies. J Clin Oncol (2004) 22:3132. doi: 10.1200/jco.2004.22.90140.3132
88. Lampropoulos P, Zizi-Sermpetzoglou A, Rizos S, Kostakis A, Nikiteas N, Papavassiliou AG. TGF-beta signalling in colon carcinogenesis. Cancer Lett (2012) 314:1–7. doi: 10.1016/j.canlet.2011.09.041
89. Barve M, Aaron P, Manning L, Bognar E, Wallraven G, Horvath S, et al. Pilot study of combination gemogenovatucel-T (Vigil) and durvalumab in women with relapsed BRCA-wt triple-negative breast or ovarian cancer. Clin Med Insights Oncol (2022) 16:11795549221110501. doi: 10.1177/11795549221110501
90. Rocconi RP, Grosen EA, Ghamande SA, Chan JK, Barve MA, Oh J, et al. Gemogenovatucel-T (Vigil) immunotherapy as maintenance in frontline stage III/IV ovarian cancer (VITAL): a randomised, double-blind, placebo-controlled, phase 2b trial. Lancet Oncol (2020) 21:1661–72. doi: 10.1016/S1470-2045(20)30533-7
91. Rijavec E, Biello F, Genova C, Barletta G, Maggioni C, Dal Bello MG, et al. Belagenpumatucel-l for the treatment of non-small cell lung cancer. Expert Opin Biol Ther (2015) 15:1371–9. doi: 10.1517/14712598.2015.1073709
92. Nemunaitis J, Nemunaitis M, Senzer N, Snitz P, Bedell C, Kumar P, et al. Phase II trial of belagenpumatucel-l, a TGF-beta2 antisense gene modified allogeneic tumor vaccine in advanced non small cell lung cancer (NSCLC) patients. Cancer Gene Ther (2009) 16:620–4. doi: 10.1038/cgt.2009.15
93. Giaccone G, Bazhenova LA, Nemunaitis J, Tan M, Juhász E, Ramlau R, et al. A phase III study of belagenpumatucel-l, an allogeneic tumour cell vaccine, as maintenance therapy for non-small cell lung cancer. Eur J Cancer. (2015) 51:2321–9. doi: 10.1016/j.ejca.2015.07.035
94. Qin W, Hu L, Zhang X, Jiang S, Li J, Zhang Z, et al. The diverse function of PD-1/PD-L pathway beyond cancer. Front Immunol (2019) 10:2298. doi: 10.3389/fimmu.2019.02298
95. Niu M, Liu Y, Yi M, Jiao D, Wu K. Biological characteristics and clinical significance of soluble PD-1/PD-L1 and exosomal PD-L1 in cancer. Front Immunol (2022) 13:827921. doi: 10.3389/fimmu.2022.827921
96. Sun C, Mezzadra R, Schumacher TN. Regulation and function of the PD-L1 checkpoint. Immunity. (2018) 48:434–52. doi: 10.1016/j.immuni.2018.03.014
97. Ohaegbulam KC, Assal A, Lazar-Molnar E, Yao Y, Zang X. Human cancer immunotherapy with antibodies to the PD-1 and PD-L1 pathway. Trends Mol Med (2015) 21:24–33. doi: 10.1016/j.molmed.2014.10.009
98. Yi M, Niu M, Xu L, Luo S, Wu K. Regulation of PD-L1 expression in the tumor microenvironment. J Hematol Oncol (2021) 14:10. doi: 10.1186/s13045-020-01027-5
99. Mayoux M, Roller A, Pulko V, Sammicheli S, Chen S, Sum E, et al. Dendritic cells dictate responses to PD-L1 blockade cancer immunotherapy. Sci Transl Med (2020) 12:eaav7431. doi: 10.1126/scitranslmed.aav7431
100. Dong W, Wu X, Ma S, Wang Y, Nalin AP, Zhu Z, et al. The mechanism of anti-PD-L1 antibody efficacy against PD-L1-Negative tumors identifies NK cells expressing PD-L1 as a cytolytic effector. Cancer Discovery (2019) 9:1422–37. doi: 10.1158/2159-8290.CD-18-1259
101. Zhu S, Zhang T, Zheng L, Liu H, Song W, Liu D, et al. Combination strategies to maximize the benefits of cancer immunotherapy. J Hematol Oncol (2021) 14:156. doi: 10.1186/s13045-021-01164-5
102. Yin Z, Yu M, Ma T, Zhang C, Huang S, Karimzadeh MR, et al. Mechanisms underlying low-clinical responses to PD-1/PD-L1 blocking antibodies in immunotherapy of cancer: a key role of exosomal PD-L1. J Immunother Cancer. (2021) 9:e001698. doi: 10.1136/jitc-2020-001698
103. Sharma P, Callahan MK, Bono P, Kim J, Spiliopoulou P, Calvo E, et al. Nivolumab monotherapy in recurrent metastatic urothelial carcinoma (CheckMate 032): a multicentre, open-label, two-stage, multi-arm, phase 1/2 trial. Lancet Oncol (2016) 17:1590–8. doi: 10.1016/S1470-2045(16)30496-X
104. Horn L, Spigel DR, Vokes EE, Holgado E, Ready N, Steins M, et al. Nivolumab versus docetaxel in previously treated patients with advanced non-Small-Cell lung cancer: Two-year outcomes from two randomized, open-label, phase III trials (CheckMate 017 and CheckMate 057). J Clin Oncol (2017) 35:3924–33. doi: 10.1200/JCO.2017.74.3062
105. Yamazaki N, Takenouchi T, Fujimoto M, Ihn H, Uchi H, Inozume T, et al. Phase 1b study of pembrolizumab (MK-3475; anti-PD-1 monoclonal antibody) in Japanese patients with advanced melanoma (KEYNOTE-041). Cancer Chemother Pharmacol (2017) 79:651–60. doi: 10.1007/s00280-016-3237-x
106. Hellmann MD, Ciuleanu TE, Pluzanski A, Lee JS, Otterson GA, Audigier-Valette C, et al. Nivolumab plus ipilimumab in lung cancer with a high tumor mutational burden. N Engl J Med (2018) 378:2093–104. doi: 10.1056/NEJMoa1801946
107. Le DT, Durham JN, Smith KN, Wang H, Bartlett BR, Aulakh LK, et al. Mismatch repair deficiency predicts response of solid tumors to PD-1 blockade. Science. (2017) 357:409–13. doi: 10.1126/science.aan6733
108. Solomon B, Young RJ, Bressel M, Urban D, Hendry S, Thai A, et al. Prognostic significance of PD-L1(+) and CD8(+) immune cells in HPV(+) oropharyngeal squamous cell carcinoma. Cancer Immunol Res (2018) 6:295–304. doi: 10.1158/2326-6066.CIR-17-0299
109. Briere D, Sudhakar N, Woods DM, Hallin J, Engstrom LD, Aranda R, et al. The class I/IV HDAC inhibitor mocetinostat increases tumor antigen presentation, decreases immune suppressive cell types and augments checkpoint inhibitor therapy. Cancer Immunol Immunother. (2018) 67:381–92. doi: 10.1007/s00262-017-2091-y
110. Biton J, Mansuet-Lupo A, Pécuchet N, Alifano M, Ouakrim H, Arrondeau J, et al. TP53, STK11, and EGFR mutations predict tumor immune profile and the response to anti-PD-1 in lung adenocarcinoma. Clin Cancer Res (2018) 24:5710–23. doi: 10.1158/1078-0432.CCR-18-0163
111. Liu C, Zheng S, Jin R, Wang X, Wang F, Zang R, et al. The superior efficacy of anti-PD-1/PD-L1 immunotherapy in KRAS-mutant non-small cell lung cancer that correlates with an inflammatory phenotype and increased immunogenicity. Cancer Lett (2020) 470:95–105. doi: 10.1016/j.canlet.2019.10.027
112. Hastings K, Yu HA, Wei W, Sanchez-Vega F, DeVeaux M, Choi J, et al. EGFR mutation subtypes and response to immune checkpoint blockade treatment in non-small-cell lung cancer. Ann Oncol (2019) 30:1311–20. doi: 10.1093/annonc/mdz141
113. Yi M, Qin S, Zhao W, Yu S, Chu Q, Wu K. The role of neoantigen in immune checkpoint blockade therapy. Exp Hematol Oncol (2018) 7:28. doi: 10.1186/s40164-018-0120-y
114. Yi M, Yu S, Qin S, Liu Q, Xu H, Zhao W, et al. Gut microbiome modulates efficacy of immune checkpoint inhibitors. J Hematol Oncol (2018) 11:47. doi: 10.1186/s13045-018-0592-6
115. Gopalakrishnan V, Spencer CN, Nezi L, Reuben A, Andrews MC, Karpinets TV, et al. Gut microbiome modulates response to anti-PD-1 immunotherapy in melanoma patients. Science. (2018) 359:97–103. doi: 10.1126/science.aan4236
116. Routy B, Le Chatelier E, Derosa L, Duong CPM, Alou MT, Daillère R, et al. Gut microbiome influences efficacy of PD-1-based immunotherapy against epithelial tumors. Science. (2018) 359:91–7. doi: 10.1126/science.aan3706
117. Yi M, Li A, Zhou L, Chu Q, Luo S, Wu K. Immune signature-based risk stratification and prediction of immune checkpoint inhibitor's efficacy for lung adenocarcinoma. Cancer Immunol Immunother. (2021) 70:1705–19. doi: 10.1007/s00262-020-02817-z
118. Thompson JC, Hwang WT, Davis C, Deshpande C, Jeffries S, Rajpurohit Y, et al. Gene signatures of tumor inflammation and epithelial-to-mesenchymal transition (EMT) predict responses to immune checkpoint blockade in lung cancer with high accuracy. Lung Cancer. (2020) 139:1–8. doi: 10.1016/j.lungcan.2019.10.012
119. Mathew M, Zade M, Mezghani N, Patel R, Wang Y, Momen-Heravi F. Extracellular vesicles as biomarkers in cancer immunotherapy. Cancers (Basel). (2020) 12:2825. doi: 10.3390/cancers12102825
120. Biton J, Ouakrim H, Dechartres A, Alifano M, Mansuet-Lupo A, Si H, et al. Impaired tumor-infiltrating T cells in patients with chronic obstructive pulmonary disease impact lung cancer response to PD-1 blockade. Am J Respir Crit Care Med (2018) 198:928–40. doi: 10.1164/rccm.201706-1110OC
121. Larson C, Oronsky B, Carter CA, Oronsky A, Knox SJ, Sher D, et al. TGF-beta: a master immune regulator. Expert Opin Ther Targets. (2020) 24:427–38. doi: 10.1080/14728222.2020.1744568
122. Thomas DA, Massagué J. TGF-beta directly targets cytotoxic T cell functions during tumor evasion of immune surveillance. Cancer Cell (2005) 8:369–80. doi: 10.1016/j.ccr.2005.10.012
123. Kanamori M, Nakatsukasa H, Okada M, Lu Q, Yoshimura A. Induced regulatory T cells: Their development, stability, and applications. Trends Immunol (2016) 37:803–11. doi: 10.1016/j.it.2016.08.012
124. Park BV, Freeman ZT, Ghasemzadeh A, Chattergoon MA, Rutebemberwa A, Steigner J, et al. TGFβ1-mediated SMAD3 enhances PD-1 expression on antigen-specific T cells in cancer. Cancer Discovery (2016) 6:1366–81. doi: 10.1158/2159-8290.CD-15-1347
125. Tauriello DVF, Sancho E, Batlle E. Overcoming TGFβ-mediated immune evasion in cancer. Nat Rev Cancer. (2022) 22:25–44. doi: 10.1038/s41568-021-00413-6
126. Mariathasan S, Turley SJ, Nickles D, Castiglioni A, Yuen K, Wang Y, et al. TGFβ attenuates tumour response to PD-L1 blockade by contributing to exclusion of T cells. Nature. (2018) 554:544–8. doi: 10.1038/nature25501
127. Li L, Wei JR, Dong J, Lin QG, Tang H, Jia YX, et al. Laminin γ2-mediating T cell exclusion attenuates response to anti-PD-1 therapy. Sci Adv (2021) 7:eabc8346. doi: 10.1126/sciadv.abc8346
128. Affo S, Nair A, Brundu F, Ravichandra A, Bhattacharjee S, Matsuda M, et al. Promotion of cholangiocarcinoma growth by diverse cancer-associated fibroblast subpopulations. Cancer Cell (2021) 39:866–82.e11. doi: 10.1016/j.ccell.2021.03.012.
129. Grauel AL, Nguyen B, Ruddy D, Laszewski T, Schwartz S, Chang J, et al. TGFβ-blockade uncovers stromal plasticity in tumors by revealing the existence of a subset of interferon-licensed fibroblasts. Nat Commun (2020) 11:6315. doi: 10.1038/s41467-020-19920-5
130. Krishnamurty AT, Shyer JA, Thai M, Gandham V, Buechler MB, Yang YA, et al. LRRC15(+) myofibroblasts dictate the stromal setpoint to suppress tumour immunity. Nature. (2022) 611:148–54. doi: 10.1038/s41586-022-05272-1
131. Le DT, Uram JN, Wang H, Bartlett BR, Kemberling H, Eyring AD, et al. PD-1 blockade in tumors with mismatch-repair deficiency. N Engl J Med (2015) 372:2509–20. doi: 10.1056/NEJMoa1500596
132. Tauriello DVF, Palomo-Ponce S, Stork D, Berenguer-Llergo A, Badia-Ramentol J, Iglesias M, et al. TGFβ drives immune evasion in genetically reconstituted colon cancer metastasis. Nature. (2018) 554:538–43. doi: 10.1038/nature25492
133. Koh J, Hur JY, Lee KY, Kim MS, Heo JY, Ku BM, et al. Regulatory (FoxP3(+)) T cells and TGF-β predict the response to anti-PD-1 immunotherapy in patients with non-small cell lung cancer. Sci Rep (2020) 10:18994. doi: 10.1038/s41598-020-76130-1
134. Lu J, Li T, Liao Z, Yu H, Zhao Y, Wu H, et al. The efficacies and biomarker investigations of antiprogrammed death-1 (anti-PD-1)-based therapies for metastatic bone and soft tissue sarcoma. Cancer Biol Med (2021) 19:910–30. doi: 10.20892/j.issn.2095-3941.2021.0270.
135. Ni Y, Soliman A, Joehlin-Price A, Rose PG, Vlad A, Edwards RP, et al. High TGF-β signature predicts immunotherapy resistance in gynecologic cancer patients treated with immune checkpoint inhibition. NPJ Precis Oncol (2021) 5:101. doi: 10.1038/s41698-021-00242-8
136. Blenman KRM, Marczyk M, Karn T, Qing T, Li X, Gunasekharan V, et al. Predictive markers of response to neoadjuvant durvalumab with nab-paclitaxel and dose-dense Doxorubicin/Cyclophosphamide in basal-like triple-negative breast cancer. Clin Cancer Res (2022) 28:2587–97. doi: 10.1158/1078-0432.CCR-21-3215
137. Shi X, Yang J, Deng S, Xu H, Wu D, Zeng Q, et al. TGF-β signaling in the tumor metabolic microenvironment and targeted therapies. J Hematol Oncol (2022) 15:135. doi: 10.1186/s13045-022-01349-6
138. Terabe M, Robertson FC, Clark K, De Ravin E, Bloom A, Venzon DJ, et al. Blockade of only TGF-β 1 and 2 is sufficient to enhance the efficacy of vaccine and PD-1 checkpoint blockade immunotherapy. Oncoimmunology. (2017) 6:e1308616. doi: 10.1080/2162402X.2017.1308616
139. Lim YW, Coles GL, Sandhu SK, Johnson DS, Adler AS, Stone EL. Single-cell transcriptomics reveals the effect of PD-L1/TGF-β blockade on the tumor microenvironment. BMC Biol (2021) 19:107. doi: 10.1186/s12915-021-01034-z
140. Gulley JL, Schlom J, Barcellos-Hoff MH, Wang XJ, Seoane J, Audhuy F, et al. Dual inhibition of TGF-β and PD-L1: a novel approach to cancer treatment. Mol Oncol (2022) 16:2117–34. doi: 10.1002/1878-0261.13146
141. Cho BC, Lee KH, Han J-Y, Yong Shim B, Kim HR, Pyo K-H, et al. 363 vactosertib and durvalumab as second or later line treatment for PD-L1 positive non-small cell lung cancer: interim result. J ImmunoTherapy Cancer. (2020) 8:A222. doi: 10.1136/jitc-2020-SITC2020.0363
142. Melisi D, Oh DY, Hollebecque A, Calvo E, Varghese A, Borazanci E, et al. Safety and activity of the TGFβ receptor I kinase inhibitor galunisertib plus the anti-PD-L1 antibody durvalumab in metastatic pancreatic cancer. J Immunother Cancer. (2021) 9:e002068. doi: 10.1136/jitc-2020-002068.
143. Lan Y, Yeung TL, Huang H, Wegener AA, Saha S, Toister-Achituv M, et al. Colocalized targeting of TGF-β and PD-L1 by bintrafusp alfa elicits distinct antitumor responses. J Immunother Cancer. (2022) 10:e004122. doi: 10.1136/jitc-2021-004122
144. Lind H, Gameiro SR, Jochems C, Donahue RN, Strauss J, Gulley JM, et al. Dual targeting of TGF-β and PD-L1 via a bifunctional anti-PD-L1/TGF-βRII agent: status of preclinical and clinical advances. J Immunother Cancer. (2020) 8:e000433. doi: 10.1136/jitc-2019-000433.
145. Strauss J, Heery CR, Schlom J, Madan RA, Cao L, Kang Z, et al. Phase I trial of M7824 (MSB0011359C), a bifunctional fusion protein targeting PD-L1 and TGFβ, in advanced solid tumors. Clin Cancer Res (2018) 24:1287–95. doi: 10.1158/1078-0432.CCR-17-2653
146. Liu D, Gong J, Liu T, Li K, Yin X, Liu Y, et al. Phase 1 study of SHR-1701, a bifunctional fusion protein targeting PD-L1 and TGF-β, in patients with advanced solid tumors. J Clin Oncol (2021) 39:2503. doi: 10.1200/JCO.2021.39.15_suppl.2503
147. Feng J, Tang D, Wang J, Zhou Q, Peng J, Lou H, et al. SHR-1701, a bifunctional fusion protein targeting PD-L1 and TGF-β, for recurrent or metastatic cervical cancer: a clinical expansion cohort of phase 1 study. Clin Cancer Res (2022). doi: 10.1158/1078-0432.CCR-22-0346
148. Shi M, Chen J, Li K, Fang Y, Wen G, Li X, et al. SHR-1701, a bifunctional fusion protein targeting PD-L1 and TGF-β, for advanced NSCLC with EGFR mutations: Data from a multicenter phase 1 study. J Clin Oncol (2021) 39:9055. doi: 10.1200/JCO.2021.39.15_suppl.9055
149. Xie J, Meng Z, Chen L, Hua Y, Li H. Phase II study of SHR-1701 combined with famitinib in the treatment of advanced pancreatic cancer or biliary tract cancer. J Clin Oncol (2022) 40:568. doi: 10.1200/JCO.2022.40.4_suppl.568
Keywords: cancer biotherapy, cancer immunotherapy, tumor microenvironment, TGF-β, PD-1, PD-L1, bispecific antibody
Citation: Yi M, Li T, Niu M, Wu Y, Zhao Z and Wu K (2022) TGF-β: A novel predictor and target for anti-PD-1/PD-L1 therapy. Front. Immunol. 13:1061394. doi: 10.3389/fimmu.2022.1061394
Received: 04 October 2022; Accepted: 06 December 2022;
Published: 19 December 2022.
Edited by:
Fu Wang, Xi’an Jiaotong University, ChinaReviewed by:
Jean-René Pallandre, INSERM U1098 Interactions, Hôte-Greffon-Tumeur & Ingénierie Cellulaire et Génique, FranceChristina Stuelten, National Cancer Institute (NIH), United States
Copyright © 2022 Yi, Li, Niu, Wu, Zhao and Wu. This is an open-access article distributed under the terms of the Creative Commons Attribution License (CC BY). The use, distribution or reproduction in other forums is permitted, provided the original author(s) and the copyright owner(s) are credited and that the original publication in this journal is cited, in accordance with accepted academic practice. No use, distribution or reproduction is permitted which does not comply with these terms.
*Correspondence: Kongming Wu, kmwu@tjh.tjmu.edu.cn; Zhenyu Zhao, zhaozhenyutjh@hust.edu.cn
†These authors have contributed equally to this work