- 1Departments of Neurology and Immunobiology, Yale School of Medicine, New Haven, CT, United States
- 2Faculty of Medicine, Imperial College London, London, United Kingdom
Cellular metabolic remodeling is intrinsically linked to the development, activation, differentiation, function, and survival of T cells. T cells transition from a catabolic, naïve state to an anabolic effector state upon T cell activation. Subsequently, specialization of T cells into T helper (Th) subsets, including regulatory T cells (Treg), requires fine-tuning of metabolic programs that better support and optimize T cell functions for that particular environment. Increasingly, studies have shown that changes in nutrient availability at both the cellular and organismal level during disease states can alter T cell function, highlighting the importance of better characterizing metabolic-immune axes in both physiological and disease settings. In support of these data, a growing body of evidence is emerging that shows specific lipid species are capable of altering the inflammatory functional phenotypes of T cells. In this review we summarize the metabolic programs shown to support naïve and effector T cells, and those driving Th subsets. We then discuss changes to lipid profiles in patients with multiple sclerosis, and focus on how the presence of specific lipid species can alter cellular metabolism and function of T cells.
Introduction
During the course of an immune response, CD4+ T cells transition from a naïve state, participating in immune surveillance, to a highly specialized subset of effector T cells (Teff) equipped with the capacity to rid hosts of invading pathogens and regulate immune responses. As this transition occurs, there is concurrent cellular metabolic remodeling that is intrinsically linked to the development, activation, differentiation, function, and survival of T cells. In addition to the different metabolic requirements of naïve and effector T cells, there are additional metabolic dependencies for CD4+ T helper (Th) subsets and regulatory T cells (Tregs), the latter relying on a different metabolic program than their effector counterparts, displaying metabolic flexibility while maintaining suppressive functions. For example, memory CD8+ T cells use fatty acids derived from extracellular glucose or those mobilized from lysosomes to support their fatty acid oxidation (FAO), whereas Tregs acquire fatty acids from the extracellular environment, although the mechanism is not well understood (1).
At the cellular and organismal level, previous studies have shown that nutrient availability in physiological health and disease states can alter T cell function. For example, fluctuations in salt intake and lipid composition in the diet have been shown to change the inflammatory profile of T cells (2–4), and feeding mice diets containing different fatty acid species alter the ratios of T cell subsets and outcome of experimental autoimmune encephalomyelitis (EAE) (5). Additionally, at the cellular level, manipulating metabolic pathways in T cells skews their differentiation towards specific Th cell subsets. Depending on the specific context this can be harmful or beneficial, as metabolic manipulation often determines Th17 versus Treg cell fates (6, 7). Together, the importance of the metabolic-immune axis is critical. However, while the link between metabolism and T cell function is appreciated, specifically how fluctuations in lipid availability, lipid species, and lipid metabolism dictates T cell functions and phenotypes is not well investigated. Studies have also shown that there are changes to lipid profiles in inflammatory settings such as obesity and autoimmune diseases like multiple sclerosis (MS) (8–10), further highlighting the importance of teasing apart the intricate links between metabolic and transcriptional changes within T cells.
In this review we discuss the changes in metabolic states as T cells change from naïve to effector cells and discuss the metabolic requirements driving CD4+ T cell subsets, including Treg cells. We briefly highlight the key roles of T cells in the pathogenesis of multiple sclerosis, and detail findings of altered lipid profiles in MS and murine models of EAE. We highlight data showing that altering lipid metabolism in T cells can alter T cell function in MS and EAE. Finally, we summarize how lipids are trafficked in the body and provide evidence supporting the idea that specific lipid species are capable of altering T cell phenotypes and functions.
Immunometabolism of T Cells
Metabolic Remodeling of T Cells Upon Activation
Upon activation, quiescent, naïve T cells have an increased need for cellular energy in the form of adenosine triphosphate (ATP) and biomass to meet proliferative demands and production of effector molecules. As such, the rapidly dividing cells utilize aerobic glycolysis, by which pyruvate is used to produce lactate in the presence of oxygen by cells with a great enough mitochondrial capacity to perform oxidative phosphorylation (OXPHOS), known as the Warburg effect (11, 12). T cell receptor (TCR) signals induce c-Myc, which initiates the expression of metabolic genes to induce glycolysis and mitochondrial metabolism necessary for T cell activation (13, 14). Both c-Myc and mammalian target of rapamycin (mTOR) are positive regulators of glycolysis in T cells (13, 15). mTOR exists as two complexes, mTORC1 and mTORC2. mTORC1 enhances glycolytic metabolism and can regulate other metabolic programs such as lipid synthesis and amino acid metabolism (16). mTOR can partially mediate TCR sensitivity (17), and co-stimulatory signals from CD28 have been shown to upregulate glucose utilization in T cells by recruitment of the phosphatidylinositol 3-kinase (PI3K) catalytic subunit p110γ which activates AKT and mTORC1 (18, 19).
AMP-activated protein kinase (AMPK) is another central node controlling metabolic remodeling during T cell activation. It is activated by TCR signaling via two different pathways. The first pathway is LKB1-dependent: AMPK can sense the cellular AMP-to-ATP ratio and control the progression of cells to complete activation (20). The second pathway of AMPK activation is calcium-dependent, mediated by activation of calcineurin and downstream kinase CAMKK (21) (Figure 1). T cells that lack AMPK are completely dependent on glycolysis and do not re-engage mitochondrial respiration upon glucose depletion (22). This suggests that activation of AMPK in early T cell activation limits mTOR signaling and prevents premature engagement of glycolysis that is associated with the high proliferative demands of clonal expansion (23). In the mitochondria, the tricarboxylic acid (TCA) cycle creates reducing equivalents that are fed into the electron transport chain (ETC) to generate ATP via OXPHOS. Substrates are derived from multiple cytosolic and mitochondrial metabolic pathways including glycolysis and the oxidation of amino acids and fatty acids. FAO translocates free fatty acids (FFA) into the mitochondrial matrix to be broken down and produce acetyl-CoA, which enters the TCA. Acetyl-CoA can also act as a substrate for epigenetic modifications, thereby illustrating how metabolism can directly influence gene expression (24). During the first 24-48 hours after T cell activation, T cells are dependent on ATP production from ATP synthase to become fully activated and meet proliferative demands (25). This reliance on ATP is demonstrated by limited T cell activation after knockdown of the ETC complex IV subunit COX10 (26), and by the inhibition of antigen-specific T cell activation upon loss of ETC complex III subunit RISP (27).
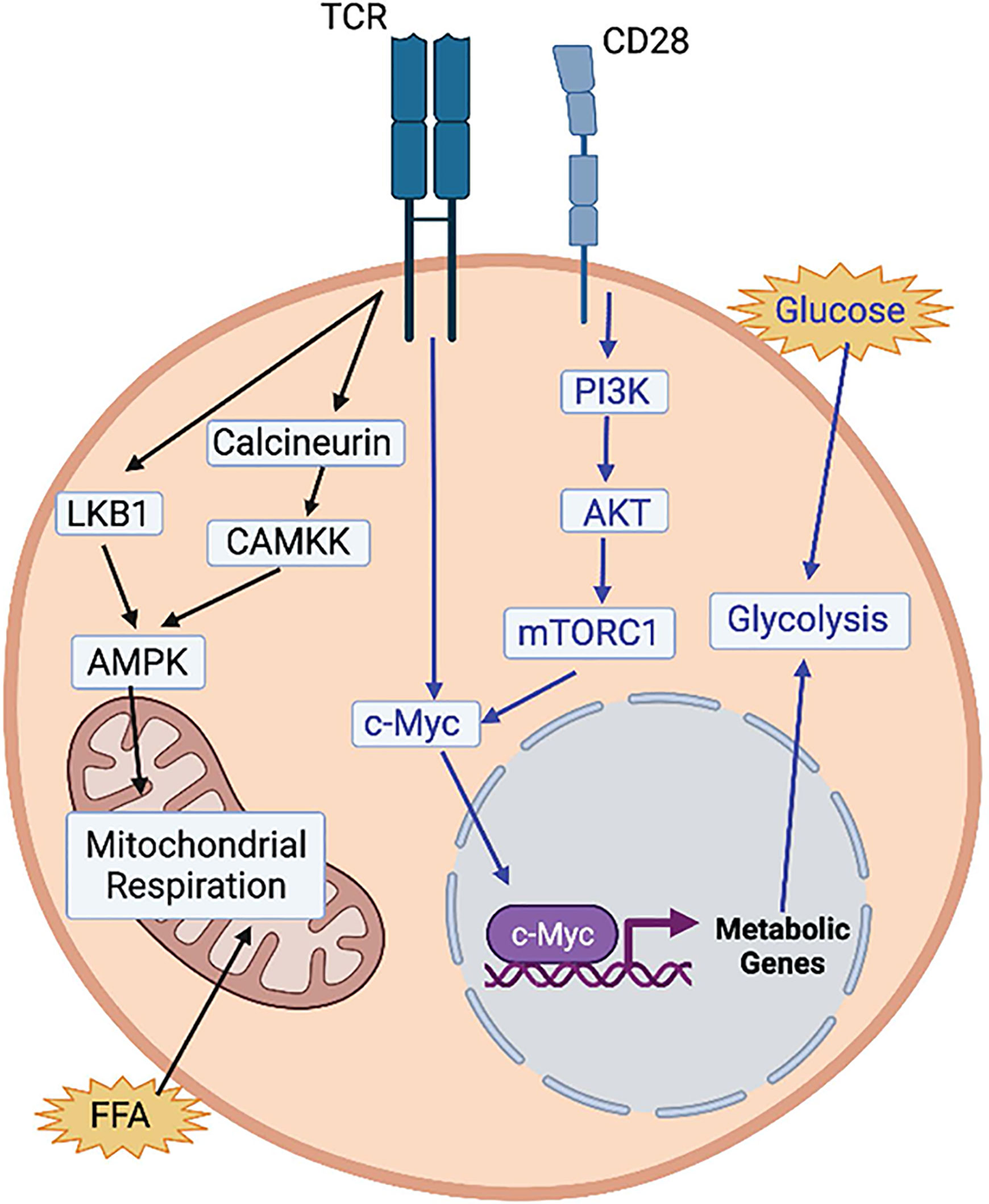
Figure 1 Regulation of T cell activation by metabolic pathways. T cell receptor (TCR) activation activates AMPK via 2 pathways (black arrows). Via an LKB1-dependent pathway and via and LKB1-independent pathway, through calcineurin and CAMKK. Both AMPK and uptake of free fatty acids (FFA) activate mitochondrial respiration, which has been shown to be necessary for T cell activation. Activation of glycolysis is also essential for T cell activation (blue arrows). TCR activation activates c-Myc which turns on the expression of glycolytic metabolic genes. CD28 activation also activates c-Myc via the PI3K-AKT-mTORC1 pathway. Image created with BioRender.com
Metabolic Requirements of CD4+ T Cell Subsets
After the initial metabolic reprogramming that occurs with T cell activation, Teff cells use glycolysis to proliferate and maintain their effector functions (28–30). However, Th cell subsets are supported by unique metabolic needs. For example, Th1 cells rely on glycolysis and glutaminolysis to support their growth and proliferation (31), and activating naïve CD4+ T cells in a glutamine deficient environment generates FOXP3+ Tregs even in the presence of Th1 polarizing cytokines (32). Further, the by-product of glutaminolysis, α-ketoglutarate, might serve as the metabolic determinant in Th1 differentiation by promoting expression of TBET and mTORC1 signaling (32). mTORC1 has been shown to phosphorylate TBET, and inhibition of mTORC1 signaling reduces production of interferon gamma (IFN)-γ (33), highlighting the importance of glucose uptake and aerobic glycolysis in the production of IFN-γ (25, 34, 35). Th1 expression of IFN-γ production is regulated transcriptionally and post-transcriptionally (22, 25), and when activated under glucose-depleted conditions, production of IFN-γ is inhibited (25, 35–38). IFN-γ production is limited by the binding of GAPDH to the 3’ UTR of ifng mRNA, which is reversed when GAPDH is engaged in glycolysis (25). Epigenetically, glycolysis has been shown to promote IFN-γ production in Th1 cells via lactate dehydrogenase (LDHA) (35). LDHA is required to sustain aerobic glycolysis that supports Th1 differentiation, and loss of LDHA leads to reduced H3K9 acetylation at the ifng locus that is associated with active transcription and reduced glycolytic flux (35) (Figure 2). Moreover, a recent study has demonstrated a role for mitochondrial metabolism in Th1 effector function by showing that mitochondrial metabolism uncouples Th1 proliferation and effector functions. The TCA cycle, and specifically, succinate dehydrogenase activity (ETC complex II), is required for Th1 effector functions including expression of IFN-γ, but suppresses proliferation and histone acetylation. Conversely, complex I of the ETC, the malate-aspartate shuttle, and mitochondrial citrate export maintain Th1 proliferation and promote histone acetylation to regulate T cell activation genes (39).
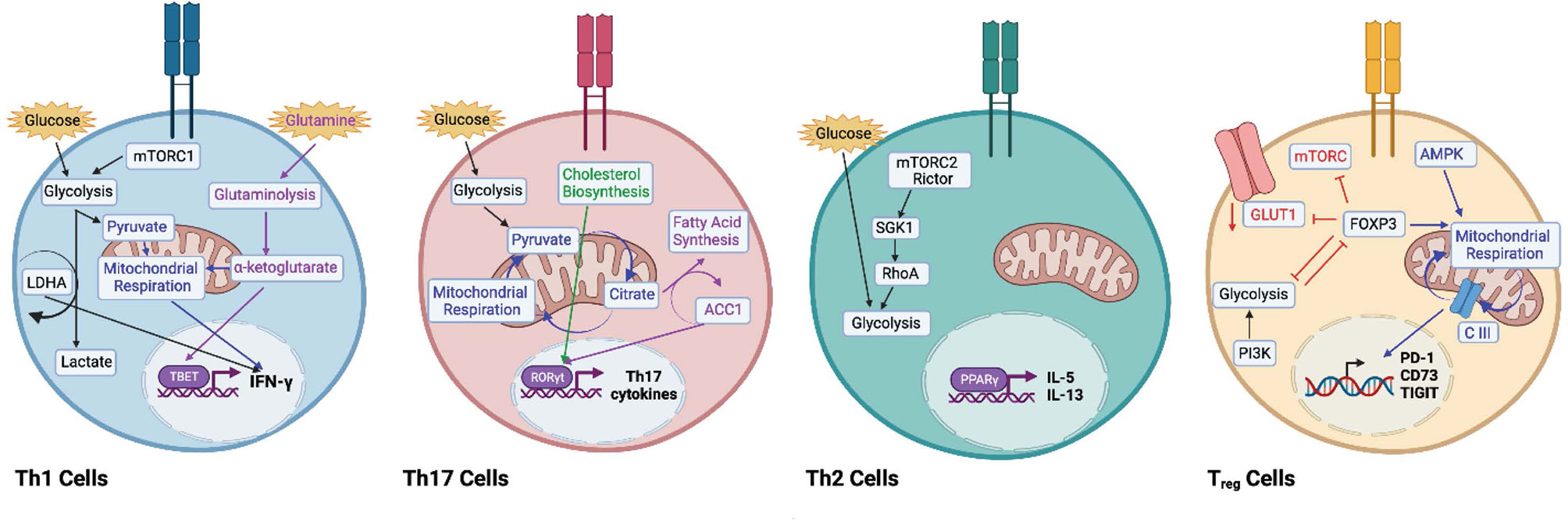
Figure 2 Metabolic remodelling to promote Th helper T cell subsets. Th1 cells engage in glycolysis (black arrows) and aerobic respiration (blue arrows) to support interferon (IFN)-γ expression. Lactate dehydrogenase (LDHA) has been shown to promote IFN-γ expression. Inhibition of mTORC1 decreases IFN-γ expression. Glutaminolysis (purple arrows) via ⍺-ketoglutarate promotes TBET expression. Th17 cells use both glycolysis (black arrows) and oxidative phosphorylation (blue arrows) to secrete Th17-associated cytokines. Acetyl-CoA carboxylase 1 (ACC1) promotes RORγt expression (purple arrows), and intermediates of cholesterol biosynthesis (green arrows) support the RORγt program. Th2 cells are supported by high levels of glycolysis. The mTORC2-Rictor complex supports the Th2 lineage via SGK1 and RhoA (black arrows). Peroxisome proliferator- activated receptor (PPAR)-γ has been shown to promote IL-5 and IL-13. Regulatory T (Treg) cells are supported by mitochondrial respiration (blue arrows), as AMPK expression drives fatty acid oxidation and oxidative phosphorylation. Complex III of the electron transport chain supports the expression of immunosuppressive genes like PD-1, CD73, and TIGIT. Expression of FOXP3 promotes AMPK expression and inhibits expression of mTORC1, GLUT1, and glycolysis (red arrows). Activation of PI3K drives glycolysis and decreases Treg suppression (black arrows). Glycolysis and FOXP3 reciprocally regulate each other. Image created with BioRender.com
In correlation with enhanced glycolytic metabolism, in vitro-generated Th17 cells express higher levels of pyruvate dehydrogenase kinase 1 (PDK1), which is essential for Th17 differentiation in vitro (40, 41). Th17 cells differentiated in vitro utilize both OXPHOS and glycolysis, and Th17 cells from steady state and inflamed tissues rely on OXPHOS for cytokine production (40). In support of these data, the OXPHOS inhibitor oligomycin reduces Th17 pro-inflammatory cytokine production and improves pathology in mouse models of colitis (40). Evidence suggests that lipid metabolism plays an important role in the function of Th17 cells under normal and stressed conditions (42, 43), and Th17 cells rely on de novo fatty acid synthesis, rather than acquisition of extracellular fatty acids (42). While the reasons for this are unclear, it has been shown that Th17 cells are particularly dependent on cholesterol synthesis, as intermediates of the pathway can bind to the RORγt promoter and promote interleukin (IL)-17 expression, whereas inhibition of cholesterol biosynthesis blocks Th17 development (44, 45). The ratio of intracellular saturated to polyunsaturated fatty acids has been shown to control CD5L expression, which can determine whether or not Th17 cells will adopt a pro- or anti-inflammatory phenotype (46). Saturated to polyunsaturated FFA ratios also affect the expression of metabolic genes cyp51 and sc4mol, which synthesize ligands for RORγt (43, 46). Given the intricacies of Th17 and Treg development, studies are unveiling a role for metabolic intermediates in skewing T cell lineages alongside cytokine polarization. For example, acetyl-CoA carboxylase-1 (ACC1)-deficient T cells default to the Treg lineage (6), and mice fed a high fat diet increases the expression of ACC1 in CD4+ T cells, which upregulates the expression of the Th17 master regulator, RORγt (7) (Figure 2). The increase of ACC1 expression in CD4+ T cells is mimicked in obese individuals and proposed to contribute to associated Th17 pathologies (7).
Th2 cells have been reported to display high glycolytic activity (31, 47). Ras homologue enriched in brain (Rheb) is a small GTPase that activates mTORC1. Rheb-deficient mice have impaired mTORC1 function and fail to generate Th1 and Th17 cells without alterations in Th2 differentiation. Conversely, rapamycin-insensitive companion of TOR (Rictor) is a component of the mTORC2 complex, and Rictor-deficient mice have impaired mTORC2 signaling, with differentiation into Th2 cells, but intact Th1 and Th17 generation (48). Further, downstream targets of mTORC2, SGK1 and the GTPase RhoA, have been shown to play important roles in the commitment to, and function of Th2 cells, respectively (49, 50). These data suggest mTORC2 is the signaling determinant of the Th2 lineage, not mTORC1, and that glycolytic engagement differs between Th2 and Th1 and Th17 lineages. Genome-wide transcriptional profiling of human Th2 cells from allergic asthma patients demonstrate a positive correlation between the expression of c-Myc and disease state further supporting glycolysis as a marker of Th2 pathogenicity (51). Lipids might also play a role in Th2 metabolism, as expression of peroxisome proliferator-activated receptor (PPAR)-γ is critical for Th2 responses against house dust mite antigens, and PPARγ-deficient mice display Th2 cells unable to produce IL-5 and IL-13 (51, 52) (Figure 2).
Metabolic Adaptations of Regulatory T Cells
In contrast to Teff cells, Tregs appear to exhibit a greater capacity for metabolic flexibility. Murine Tregs have been described to preferentially rely on OXPHOS rather than aerobic glycolysis for ATP production (31). However, metabolic adaptations of Treg cells are context-dependent and influenced by Treg origin and anatomical distribution (53). It remains unclear how thymic-derived Tregs (tTregs) and Tregs differentiated in the periphery from conventional Teff cells, termed peripheral Tregs (pTregs) differ metabolically. However, it appears that Treg proliferation and effector functions are maintained through different metabolic programs. Proteomic analysis of human Treg and Teff cells suggests that freshly isolated Tregs are highly glycolytic and proliferative, while in vitro proliferating Tregs engage in both glycolysis and FAO (53). Conversely, Teff cells switch from OXPHOS to aerobic glycolysis upon in vitro activation, and FAO appears to be indispensable for proliferation and effector functions (53). One explanation for the discrepancy between human and murine models is that mice are typically housed in pathogen-free environments, whereas human immune cells are constantly engaging with pathogens that requires Tregs to meet higher proliferative and functional demands (54).
AMPK and mTORC1 signals are important determinants of Treg fitness. Treg-specific mTOR deletion impairs Treg fitness and homeostasis resulting in a reduction of Tregs in the tissues and Teff cell activation and heightened inflammation at barrier sites (55). Inhibiting mTORC1 by rapamycin along with IL-2 signaling greatly expands Treg numbers (56). Conversely, AMPK, which activates FAO and OXPHOS, provides critical signals required for Treg differentiation (57). Activation of AMPK by metformin induces Treg differentiation and, in murine models, was sufficient to limit progression of EAE and inflammatory bowel disease (31, 58, 59).
FOXP3 is involved in the fine tuning of metabolic cues to maintain Treg function. Inflammatory stimuli and FOXP3 oppose each other through regulation of mTORC1 and glucose metabolism as Treg treatment with TLR1/TLR2 agonists enhances mTORC1 activity and Treg proliferation but reduces their suppressive capacity (60). In contrast, FOXP3 inhibits mTORC1 signaling and glycolysis while promoting OXPHOS and reduces the rate of proliferation (60), suggesting that mTORC1 activity and glycolysis are adequate for Treg proliferation in inflammatory environments. However, excessive mTORC1 activity impairs Treg function and indeed Tregs suppress mTORC1 activity via PP2A (48, 61, 62). Murine Tregs induced via transforming growth factor (TGF)-β express low levels of GLUT1 compared to Teff cells (31), and in human Tregs, GLUT1 expression is also thought to be limited by FOXP3 via AKT inhibition (63). Tregs that overexpress GLUT1 exhibit reduced CD25 and FOXP3 expression and fail to suppress colitis (60). Expression of FOXP3 allows Tregs to survive in lactate rich environments by increasing the ratio of NAD to NADH (64). Treg proliferation and suppressive functions can be uncoupled metabolically, which is likely advantageous in an inflammatory setting. For example, it might be advantageous for Tregs to engage in glycolysis to rapidly proliferate and deprive proliferating Teff cells of an essential nutrients, then switch to an OXPHOS program for optimal suppressor functions. Of note, PI3K-mTORC2 mediated activation of glucokinase, which activates glycolysis and mediates actin cytoskeletal rearrangements, was found to be important for proper migration of Tregs in skin allograft models (65). Therefore, although glycolysis seems to play a role in Treg migration and proliferation, utilization of OXPHOS appears to be the main metabolic program utilized for Treg function, which is dependent on FAO (31).
Cytokines that promote Treg differentiation such as TGF-β (66), activate AMPK (67) and promote FAO to skew naïve T cells into a Treg phenotype instead of pro-inflammatory Th17 cells (31, 68). In support of this observation, Treg differentiation and suppressive functions are reduced by the FAO inhibitor etomoxir (31), and suppression of PI3K activity by phosphatase and tensin homolog (PTEN) drives Treg differentiation (69). PTEN-deficient Tregs have elevated glycolytic activity and lose FOXP3 expression and suppressive function (70). These data suggest that the balance between PTEN and AKT functions can regulate Treg differentiation. Data also suggest a reciprocal relationship between FOXP3 and glycolysis as FOXP3 has been shown to interfere with c-Myc expression to dampen glycolytic activity (64) and forced expression of FOXP3 suppresses glycolysis-related genes while inducing lipid and oxidative metabolic genes required for maximum suppression (60). In murine models, mitochondrial complex III is required for Treg suppression through the active metabolism of 2-HG and succinate, resulting in de-repressed transcription of Treg suppressive genes, including PD-1, CD73, and TIGIT (Figure 2). Moreover, loss of mitochondrial complex III function accumulates 2-HG and succinate, which inhibits demethylases and represses transcription of Treg suppressive genes PD-1, CD73, Nrp1, and TIGIT. In addition, mice containing a RISP knockout, an essential component of mitochondrial complex III, die after one month exhibiting lethal inflammation as characterized by enlargement of lymph nodes and spleens along with lymphocytic infiltration into multiple organs (71).
Interestingly, in human Tregs, glycolytic activity has been linked to suppressive function via enolase-1, which mediates expression of a specific splice variant of FOXP3 that is necessary for suppression (72). However, this study also showed that the relationship between FOXP3 and metabolic intermediates changes depending on which metabolic program the cells are engaging. Upon inhibition of glycolysis with 2-DG, the non-glycolytic activity of enolase-1 represses FOXP3 expression, whereas the generation of Tregs under suboptimal TCR stimulation promotes FOXP3 expression via the glycolytic activity of enolase-1 (72) (Figure 2). Lack of mTOR activity does not decrease FOXP3 expression, but it does diminish cholesterol biosynthesis that has been shown to be required for suppressive function in vivo (73). Therefore, Tregs seem to be partially dependent on mTOR and glycolytic signals that are more oscillatory in nature (74), or perhaps for maintenance of metabolic intermediates that feed into lipid related pathways, as seen with cholesterol.
Fatty acids can induce cell death in T cells (75–78). It is suggested that Tregs have specifically evolved FAO dependence to combat fatty-acid-induced cell death via FOXP3 (79). For example, long chain fatty acids like palmitate, are pro-apoptotic through various mechanisms, including depolarization of mitochondrial action potential and reactive oxygen species generation (80). This shows that FOXP3 uniquely enables Tregs to utilize fatty acids as fuel by upregulating enzymes to engage in FAO and become resistant to apoptosis (79). This evolved anti-apoptotic strategy is likely even more useful during steady state or inflammation as Tregs are important in the maintenance of homeostasis in lipid-rich tissue environments. Undoubtedly, the metabolic-functional axis in these cells is incredibly complex, and is essential to our complete understanding of disease pathology and resolution.
Overall, it is clear that T cell metabolic programs are flexible and highly dynamic. T cells can utilize different substrates based on metabolic needs, and tailor metabolic programs to support effector functions. Studies investigating Th-subset differentiation show that specific metabolic engagements promote and suppress specific Th-lineage differentiation and functions.
The Role of Lipids in T Cell Function
Introduction to Lipids and Lipid Trafficking
Lipids provide the foundation of both cellular and organelle membranes, serve as fuel for cells, and are the precursors of bioactive lipid mediators. Lipids are incredibly diverse, just like proteins; however, because of limitations in technologies to study lipids, we are just beginning to understand the diverse effects lipids exert on biological processes such as gene expression and cellular function. It is understood that the storage and secretion of lipids from the adipose tissue is one mechanism by which the body can communicate hunger and the need for energy (81, 82). However, during lipolysis and even inflammation, FFAs are exposed to immune cells that reside in the tissues and in circulation. How immune cells sense and interpret these signals remains an active area of research, as evidence is emerging that not all lipids are created equal and perform the same functions on specific cell types.
Cells obtain fatty acids from lipid species in the diet, fats stored in cells as lipid droplets, and lipids synthesized in one organ for export to another. Lipid species from the diet are ingested as triglycerides, which travel into the small intestine where they are emulsified by bile salts, and form hydrophobic structures called micelles. Together, bile salts and pancreatic lipases breakdown triglycerides into FFAs. FFAs in the intestine are transported across intestinal cell membranes, where they are converted back into triglycerides, and packaged with cholesterol and other apolipoproteins into vesicles called chylomicrons. Chylomicrons move freely from the intestines, through the lymphatic system, and into the bloodstream where they either enter the liver, or the adipose tissue. In the adipose, they will either be oxidized for fuel, or stored until they are needed. When hormones in the body signal the need for metabolic fuel, triglycerides that are stored in adipose tissue are mobilized and transported to other tissues throughout the body where the fatty acids may be oxidized for energy (81).
Similar to amino acids, there are both essential and non-essential fatty acids. Essential fatty acids comprise groups of lipids that must be acquired from the diet, as they cannot be synthesized in the body. These include omega-3 and omega-6 fatty acids. Omega-3 fats are derived from fish, eggs, and other plant based sources and are typically associated with anti-inflammatory effects (82). In contrast, omega-6 fatty acids are found various oils and animal meats and are associated with the production of inflammatory bioactive mediators (83). Of interest, these two groups of fatty acids can compete with each other for insertion into cellular membranes, where they are cleaved and converted into lipid mediators and other signaling molecules (84). Further, studies have shown due to this competition, fats in the diet reflect the overall lipid composition in cellular membranes (84–87), highlighting an important link between diet and inflammatory status. Therefore, fat sources enriched in the Westernized diet may partially explain rises in some inflammatory associated diseases.
FFAs are further characterized according to length and saturation. SCFA are typically classified as having fewer than six carbons, whereas medium chain fatty acids (MCFA) contain between six to twelve carbons, and LCFA are those with more than twelve carbons. Saturated fatty acids contain no double bonds in their carbon tail, whereas monounsaturated and polyunsaturated fatty acids contain one double bond, and more than one double bond, respectively. SCFAs are able to freely move into the mitochondria for β-oxidation, whereas LCFAs must first enter the carnitine shuttle. Of note, the majority of FFAs obtained from the diet or released from the adipose tissue are MCFA or LCFAs. On the other hand, most of the SCFA studied are those derived from bacterial metabolism and associated byproducts.
Lipids Altering Immune Cell Phenotypes
There is great focus on understanding factors that drive and regulate immune cell phenotypes and functions in tissues. Recent studies have described contributions from cytokines and hormones secreted from tissue-resident cells and other neighboring immune cells (88–91), the presence of local antigens driving immune cell persistence in the tissues (88, 92, 93), and the changes in nutrients that prompt metabolic reprogramming in specific microenvironments, such as tumors (94, 95). Evidence has shown that CD4+ T cell fate is driven by availability of metabolic substrates and cell-intrinsic programs that determine metabolic requirements (96). Metabolic reprogramming is critical for T cell proliferation, differentiation, and function, however, how specific substrates such as lipids, affect T cells remains unanswered. Tregs rely mainly on FAO-driven OXPHOS for survival and function (31), so lipid species in the tissues likely provide a critical signal for maintenance of Treg survival and homeostatic functions within tissues as well. It is likely that altered lipid profiles in disease settings can impact T cell function and is of great interest to fully understand how tissue microenvironments shape T cell metabolism.
Recent data have shown that the length of fatty acids can differentially affect immune cell phenotypes. In the colon, resident gut bacteria produce SCFAs as a byproduct of fermentation (97, 98), which shapes the colonic Treg population (99). The addition of the SCFA propionate to the drinking water of germ-free mice increases Treg numbers in the colon, but not spleen or thymic Treg numbers. Further, antibiotic-mediated depletion of gut bacteria reduces Treg numbers that can be rescued with the addition of propionate to the drinking water (99). SCFAs promote FOXP3 expression and are dependent on fatty acid receptor, GPR43 (100, 101). In support of these data, the addition of SCFAs to naïve CD4+ T cells increases the proportion of FOXP3+ cells upon activation. However, the addition of LCFAs results in CD4+ T cells that produce more pro-inflammatory cytokines including IFN-γ and IL-17a that exacerbate EAE (5).
Unsaturated fatty acids like oleic acid and linoleic acid have been shown to modulate cytokine production in T cells (102, 103), but only saturated fatty acids induce cytokine secretion in the absence of T cell activation in a dose-dependent manner (102). In T cells, oleic acid has been shown to induce proliferation in the spleen and lymph nodes, but to inhibit Jurkat cell proliferation and IL-2 and IFN-γ production (104). In CD4+ T cells, fatty acid synthesis blockade by the inhibitor TOFA, reduces proliferation, which can be rescued by the addition of oleic acid specifically (105). Moreover, oleic acid can also rescue proliferation of CD4+ T cells cultured in fatty acid-free media (105). Interestingly, oleic acid has also been shown to decrease the content of arachidonic acid in cell membranes that result in decreased arachidonic acid-derived pro-inflammatory lipid mediators (86, 87), and to inhibit palmitic-induced inflammation in type 2 diabetes (106). Mechanistically, attenuation of palmitic acid-induced inflammation by oleic acid is linked to increases in CPT1a expression and FAO driven by AMPK activation. In adipocytes, oleic acid induces IL-10 and adiponectin expression which can activate AMPK (106). We have also shown that oleic acid is one of the most prevalent fatty acids in human adipose tissue, and is significantly decreased in the adipose tissue from MS patients (4).
In EAE models, docosahexaenoic acid (DHA) downregulates Th1 and Th17-related cytokines (107). Both DHA and eicosapentaenoic acid (EPA) have been shown to modulate the JAK-STAT pathway and IL-2 signaling in T cells (108). In Jurkat cell lines DHA exerts immunosuppressive effects by increasing calcium concentrations in cells (109–111). Similarly in vivo, mice fed a DHA- and EPA-enriched diet exhibited reduced T cell proliferation and IL-2 signaling (77, 112, 113). DHA has been also shown to induce dose-dependent reductions in the ability of Tregs to inhibit effector T cell proliferation. In contrast, DHA can upregulate FOXP3 mRNA and other immunosuppressive cytokines such as IL-10 (114).
Saturated fatty acids like palmitic acid have been shown to drive T cell activation via the PI3K/AKT pathway (5, 115, 116). Palmitic acid treatment also induces the expression of cytokines related to T cell activation such as IL-2, IL-6, IL-17A, TNF-α, and IFN-γ (102, 116). Similarly, lauric acid has been shown to have some pro-inflammatory properties, as it increases IL-2 and can promote Th17 differentiation in models of EAE (117).
The PPAR family of lipid receptors has been implicated in T cell biology, through regulation of IL-2 production (118–120), influencing Th17 and Treg differentiation (121), and regulation of inflammation (121–126). Similarly, SREBPs are transcription factors that regulate gene expression related to lipogenesis and cholesterol synthesis and uptake (127). SREBPs are important for lipid membrane synthesis that allows rapid expansion of proliferating T cells (128). However, in the absence of fatty acids or upon blockade of FAO, Treg development is inhibited in vitro. Tregs rely on exogenous fatty acids as their metabolic substrate (31, 42), which seems to impart a survival advantage for Tregs as FOXP3 alone upregulates enzymes associated with FAO and OXPHOS (79). Interestingly, triglyceride storage in Tregs limits protein kinase C activity to promote FOXP3 expression, potentially highlighting a feedback mechanism between exogenous fatty acid uptake in Tregs (79). Much of these data support the idea that fatty acids can differentially effect Teff cells and Treg subsets, but further, that specific fatty acid species have the potential to differentially affect Treg biology. However, the mechanism driving these changes remains unknown.
T Cells in Multiple Sclerosis
MS is an autoimmune disorder characterized by aberrant immune responses and immune cell infiltration in the central nervous system (CNS). Both relapsing-remitting MS and progressive MS experience demyelination and neurodegeneration because of ongoing inflammation in the CNS (129–131). Multiple immune cell types are found in the MS brain; however, autoreactive T cells are considered the main mediators of inflammation (132–137). In particular, the CD4+ T cell subsets, Th1 and Th17 cells promote inflammation via interactions with other immune cells types, such as B cells, follicular T helper cells (Tfh) cells, and resident CNS microglia. Tfh cells support B cell survival, differentiation, and expansion via interactions in the germinal center and through secretion of cytokines like IL-21 (138, 139). Tfh cells have been reported in MS lesions (140). In addition, numerous markers of Tfh cells, such as IL-21, are upregulated in MS (141), and altered ratios of Tfh cells have been reported (142). For instance, MS patients have elevated ratios of Th17-like Tfh cells, which are considered pathogenic and support B cell antibody production (142). Thus, Tfh cells likely play an important role in MS pathogenesis by supporting autoreactive B cell antibody production.
Th1 CD4+ T cells that are associated with MS express the transcription factor TBET and secrete pro-inflammatory cytokines IFN-γ and TNF-α, whereas Th17 associated CD4+ T cells express RORC2 and secrete IL-17, IL-21, and IL-22 cytokines. Both IFN-γ and IL-17 are thought to enhance immune activation and have been strongly associated in human disease, as the frequencies of Th1 and Th17 cells are increased in MS lesions and the cerebrospinal fluid (CSF) (130, 143). However, there is a dichotomous nature to the roles of Th1 and Th17 cells in MS. Increases in Th17 cells have been measured during clinical relapse in the CSF of MS patients (143); whereas other studies have found increases in IFN-γ upon relapse (144). Despite these differences, evidence suggests that both IFN-γ and IL-17 are pathogenic in MS, however, different cytokines might play different roles depending on the stage of the disease (for example initiating events of the disease versus exacerbation of the disease). Furthermore, with advances in single cell technologies, there is growing evidence of subsets within Th cell subsets, so it is likely that not all IFN-γ or IL-17-producing cells are pathogenic, but perhaps only a subset of these cells, making the signals and environmental cues that drive these pathogenic subsets of great interest to define.
Of importance are the pathogenic Th17 cells that secrete both IFN-γ and IL-17 and express both TBET and RORγt, termed Th1-like Th17 cells, which are found in multiple autoimmune disorders like multiple sclerosis (145), insulin-dependent diabetes mellitus, autoimmune arthritis and inflammatory colitis (146, 147). In EAE, Th1-like Th17 cells have been found to be the main drivers of disease pathology (148), and in humans, IFN-γ+;IL-17+ T cells are found in the blood and brain tissue of MS patients (149). These Th1-like Th17 cells are capable of crossing the blood brain barrier and have been correlated with inflammation in both MS patients and EAE models (148–150). Th1-like Th17 cells can also secrete granulocyte macrophage colony-stimulating factor (GM-CSF) which augments inflammation by activation of innate immune cell populations (151, 152). In contrast, subsets of Th17 cells can produce IL-10 and thought to play a more suppressive, protective role in inflammatory settings (153–156).
Another consequence of the ongoing inflammation in MS are the functional changes that occur in Tregs. Under homeostatic conditions Tregs are responsible for the maintenance of immune tolerance by preventing aberrant or excessive immune responses. However, during chronic inflammation, as with MS, Tregs acquire effector like properties, and express IFN-γ, termed, Th1-like Tregs (157). Th1-like Tregs upregulate other Th1 markers, such as TBET and CXCR3, and are less suppressive than their control counterparts in vitro (157). Increased frequencies of Th1-like Tregs have been found in patients with MS, type 1 diabetes, and in mouse models of Sjögren Syndrome, and are thought to contribute to the loss of tolerance in autoimmune diseases (158). Th1-like Tregs secrete IFN-γ via activation of the PI3K-AKT-FOXO1/3 pathway, and using an in vitro approach, activation of PI3K has been shown to be sufficient to induce a Th1-like phenotype in human Tregs (159, 160). Given that mTORC1 is downstream of the PI3K pathway it is possible that alterations in cellular metabolism also accompany such examples of Treg plasticity.
Development of MS is thought to arise from unfavorable interactions between genetic risk loci and environmental conditions and/or cues. Numerous environmental triggers have been proposed, including smoking, vitamin D, EBV infection, salt, and gut microbiota (161, 162). However, none of these factors reconcile the rise in MS incidence in the western world, except the changes in diet and consequential rise in obesity. The Western diet contains greater concentrations of salt, refined sugars, and unsaturated fats (163). We have reported that the physiologic sodium concentrations induce an inflammatory signature in Teff cells and drives a Th1-like phenotype in Tregs (2). Further, obesity has been identified as another risk factor for the development of MS, and adipose tissue of obese patients has been shown to host a chronic, pro-inflammatory environment, indicating that diet can influence immune cell activation and phenotypes within tissues, which might play an important role in MS (164, 165).
Altered Lipid Profiles in Multiple Sclerosis
Lipids play many roles in the CNS including signaling, structural support, mediating inflammation, and membrane biogenesis (9). Therefore, during inflammation changes in the availability and profiles of FFA species might alter disease pathogenesis by influencing immune cell function. In multiple sclerosis, immune cells attack the myelin sheath around nerves that are rich in lipids, containing approximately 700 different lipid species (11, 15, 16), and as a result many studies have found altered lipid profiles in the MS patients and EAE models (8, 166), that could potentially serve as biomarkers of the disease. At the time of diagnosis, the CSF of MS patients harbor differences in many lipid species, including pro-inflammatory arachidonic acid, compared to the non-MS control group (167). In support of these data, lipid profiling of mouse EAE brains showed that during acute inflammation, lipid metabolism is shifted from the pathway that creates common lipid substrates into the pathway producing the pro-inflammatory arachidonic acid (168). Studies profiling the phospholipids of serum samples from patients with MS have also demonstrated that patients with MS have a phospholipidomic signature different from that of healthy controls (169).
Altered T Cell Metabolic Profiles in Multiple Sclerosis
As with infections and metabolic syndromes, the inflammation induced in autoimmunity triggers metabolic alterations locally and systemically (166). In the blood of patients with MS and other autoimmune disorders such as rheumatoid arthritis and systemic lupus erythematosus, there are elevated levels of IL-1β, IL-6, TGF-β, and IL-23, which promote pathogenic Th17 cell differentiation and block Treg development in vivo and in vitro (170–176), proving that there is a disturbance in the balance between the induction and regulation of pro-inflammatory Teff cells. Although the metabolic cues that shift this balance are still being defined, there is evidence that glycolysis and OXPHOS are impaired during T cell activation in relapsing-remitting MS patients (177).
As mentioned above, glycolysis is a common feature of Th1 and Th17 CD4+ Teff cells (13, 47). Inhibiting glucose metabolism can inhibit differentiation of Th1 and Th17 lineages even in the presence of polarizing cytokine (31, 47). Further, blocking glycolysis using 2-deoxyglucose (2-DG) improves clinical outcomes in EAE models (47) (Figure 3). A direct link between dysregulated glucose metabolism in lymphocytes and autoimmunity was shown in mice that over-express GLUT1 in T cells (178). Increased glucose uptake drives IFN-γ and IL-2 expression in T cells upon in vitro stimulation, and deletion of GLUT1 reduces glycolysis in CD4+ T cells, and effector differentiation. These cells are also unable to provide protection in a model of colitis (34). Further, CD4+ T cells in lupus-prone mice show increases in glycolytic engagement compared to healthy control cells (179).
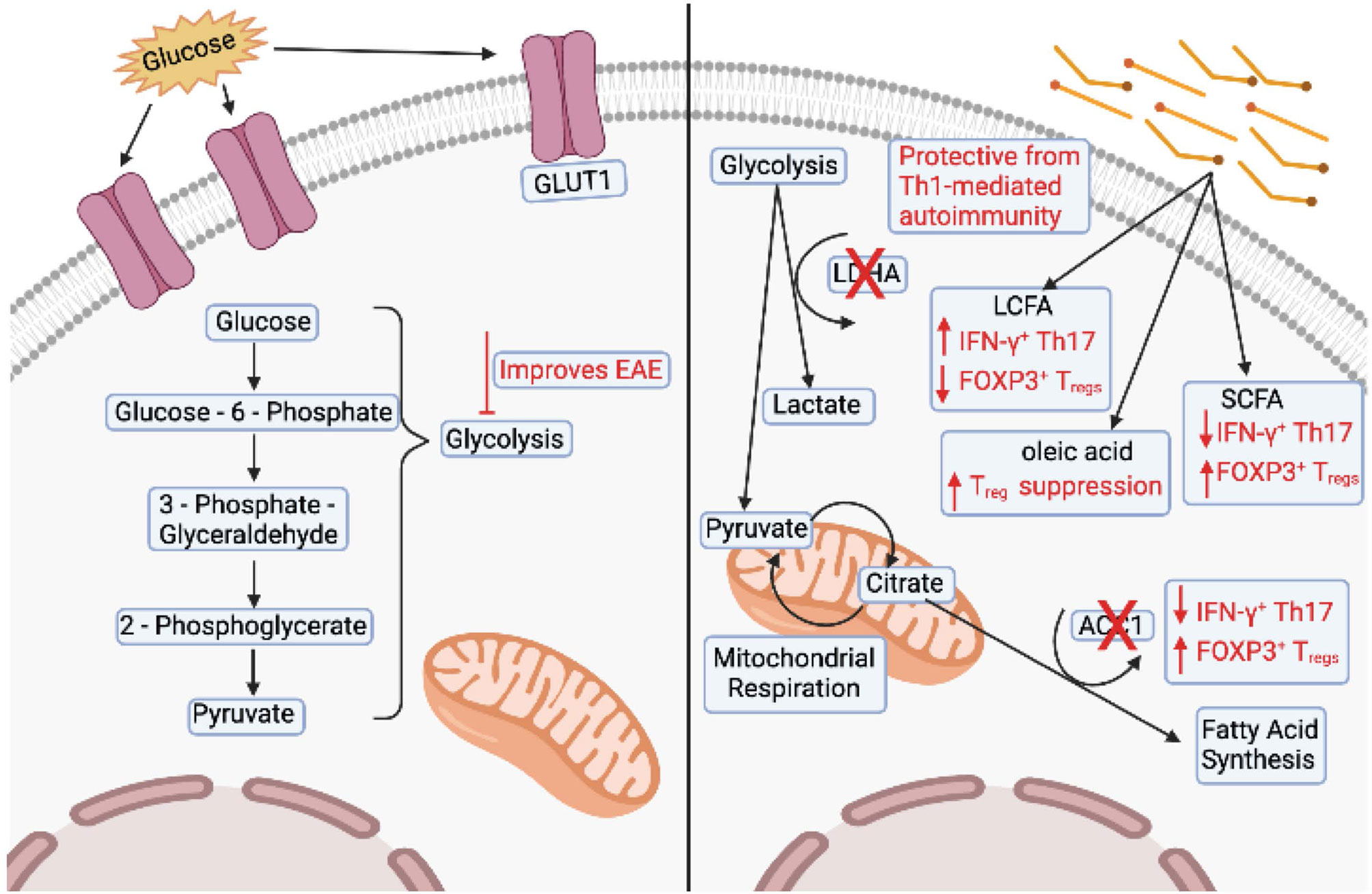
Figure 3 Changes in T cells associated with multiple sclerosis. The left panel shows overexpression of GLUT1 and dysregulation of glycolysis associated with T cells in experimental autoimmune encephalomyelitis (EAE) models. Inhibition of glycolysis also improves clinical outcomes of EAE. The right panel shows that deletion of lactate dehydrogenase (LDHA) is protective in Th1-mediated autoimmunity. Deletion of acetyl-CoA carboxylase 1 (ACC1) in T cells decreases the number of interferon (IFN)-γ+ Th17 cells and increases the number of FOXP3+ Treg cells. Mice fed long chain fatty acids (LCFAs) have an increased number of IFN-γ+ and IL-17+ T cells and a decreased number of FOXP3+ cells. Conversely, mice fed short chain fatty acids (SCFAs) have an decreased number of IFN-γ+ and IL-17+ T cells and an increased number of FOXP3+ cells. Finally, T cells cultured in the presence of LCFA, oleic acid, can partially restore Treg suppression. Image created with BioRender.com
Some studies have reported alterations in T cell lipid metabolism as a contributor to MS pathogenesis (180–183). Activated T cells have higher cholesterol and fatty acid concentrations in their membrane (184, 185), therefore altered lipid-mediated signaling could contribute to MS pathogenesis (186). Specific deletion of ACC1 in T cells protects mice from EAE by reducing the number of IFN-γ+ Th17 cells and increases the number of FOXP3+ Tregs in the spinal cord (42). Conditional deletion of LDHA in T cells protects susceptible mice from Th1-mediated autoimmunity (35). Simvastatin, an inhibitor of cholesterol biosynthesis, was used in a clinical trial to treat MS and was able to attenuate brain atrophy and disease progression (187). In support, during the chronic phase of EAE, linoleic and cholesterol metabolism have been found to be altered (9, 10), and inhibition of cholesterol synthesis via statins, prevented EAE progression by blocking Th17 differentiation (188). However, statins broadly affect the immune system, including promotion of Th2 cells and inhibition of the cytokines that induce Th1 and Th17 differentiation, and therefore their effect on MS might not be directly related to their effect on cholesterol (187–189).
Long chain fatty acids (LCFAs) and short chain fatty acids (SCFAs) have been shown to exert opposing effects on T cell differentiation. LCFAs promote IFN-γ and IL-17 production and exacerbate EAE, whereas SCFAs induce FOXP3 expression and provide protection (5). We have recently shown that Tregs isolated from the adipose tissue of MS patients exhibit a pro-inflammatory transcriptome that resembles the transcriptome of Tregs treated in vitro with the pro-inflammatory LCFA arachidonic acid (4). Tregs isolated from the adipose of healthy subjects and Tregs exposed to oleic acid in vitro do not show an inflammatory transcriptome, but rather exhibit increased FAO-driven OXPHOS metabolism, creating a positive feedback mechanism that induces the expression of FOXP3 and enhances phosphorylation of STAT5, which acts to stabilize the Treg lineage and increase suppressive function (4). Finally, treating dysfunctional MS Tregs with oleic acid can partially restore their suppressive function, highlighting a role for dietary lipids in shaping Treg function and identity in MS (4, 92, 190, 191) (Figure 3). Further studies must address how metabolic perturbations generate and sustain pathogenic T cells in the context of MS and other autoimmune diseases. Metabolic signatures of T cells are intricately linked to their differentiation and activation status. However, under inflammatory conditions such as infection or during metabolic syndromes, autoimmunity, and cancer, the microenvironment drastically changes in regard to nutritional availability, forcing T cells to adapt to these changing environments.
Future studies focusing on how nutrient depletion or metabolite availability shapes metabolic utilization and differentiation of Teff and Treg cells will be critical to our understanding and implementation of targeted therapies. For example, Shi et al. have shown that blocking glycolysis improves clinical outcomes of EAE (47), and disease-modulatory effects of feeding mice both LCFAs and SCFAs have been observed (5). However, to realistically translate these data to human therapies we must first develop more reliable metabolic profiles of T cells and other cells involved in disease pathogenesis and progression during active disease states. Once established, we can couple these data to cytokine profiles or other functional readouts, e.g. suppressive capacity of Tregs, in order to more precisely define relevant metabolic targets. One current limitation of metabolic therapies is that the target molecules are often the regulators of central metabolic nodes that integrate many signals and crosstalk between multiple pathways, such as mTOR. However, the ability to fine tune T cell functions and phenotypes that are more favorable for disease outcomes will depend on specifically targeting certain metabolic intermediates.
Conclusions
Lipids are a critical component of all cellular and organelle membranes. They provide structural support to the cell, serve as fuel, and signaling platforms. Lipid species are as diverse as proteins, however, limitations in technologies to study lipids have left major gaps in our understanding of their range of impacting biological functions, especially in lymphocytes. Despite these gaps, we know that different T cell subsets differentially utilize lipids for fuel and function (192). Upon activation of an Teff cell, there is a switch from a predominantly OXPHOS metabolic program to aerobic glycolysis, a more anabolic process critical for growth and proliferation during an immune response (47, 192). Conversely, OXPHOS mainly supports survival of Tregs and memory T cells (31). Although these cell types rely on the same metabolic program, they acquire and utilize lipid species differently (15). Given the diversity of FFAs, profiling lipid composition in tissues is crucial to understand which FFA species might be important in regulating T cell function in specific microenvironments and physiological states. Current data show the pleiotropic effects lipid species exert on T cells, depending on fatty acid length, degree of saturation, and nutrient availability in the environment. There are clear correlations and fluctuations in lipid profiles with diet and disease states, so understanding these differences will be critical to discern how lipid signaling and lipid-driven metabolism affects the function and phenotypes of T cells in disease states. There are many remaining questions regarding fatty acid-specific effects on T cell function. For example, it is not well defined which FFA are preferentially metabolized, which can serve as signaling molecules, or which FFA exert their effects by increasing intermediates generated by FAO or other downstream metabolic processes. It is also not fully understood if lipid receptors display degrees of specificity beyond FFA length. Better characterization of these receptor families could potentially provide insight as to which FFA are being utilized by T cell subsets and how the receptor expression profile might shift in specific disease states. Regardless, given the constant exposure of T cells to lipid species in the tissues, it is certain these molecules have important implications in T cell biology.
Author Contributions
SLP wrote the manuscript under the supervision of MD-V and DAH. Correspondence should be addressed to MD-V. All authors contributed to the article and approved the submitted version.
Funding
This work was supported by grants to DAH from the NIH (U19 AI089992, R25 NS079193, P01 AI073748, U24 AI11867, R01 AI22220, UM 1HG009390, P01 AI039671, P50 CA121974, and R01 CA227473); the National Multiple Sclerosis Society (NMSS) (CA 1061- A-18, RG-1802-30153); the Nancy Taylor Foundation for Chronic Diseases; and Race to Erase MS.
Conflict of Interest
DH has received funding for his laboratory from Bristol Myers Squibb and Genentech. A child of DAH is an employee of Sanofi. Further information regarding funding is available at: https://openpaymentsdata.cms.gov/physician/166753.
The remaining authors declare that the research was conducted in the absence of any commercial or financial relationships that could be construed as a potential conflict of interest.
Publisher’s Note
All claims expressed in this article are solely those of the authors and do not necessarily represent those of their affiliated organizations, or those of the publisher, the editors and the reviewers. Any product that may be evaluated in this article, or claim that may be made by its manufacturer, is not guaranteed or endorsed by the publisher.
Abbreviations
ACC1, Acetyl-CoA carboxylase-1; ATP, Adenosine triphosphate; AMPK, AMP activated protein kinase; CNS, Central nervous system; CSF, Cerebrospinal fluid; DHA, Docosahexaenoic acid; Teff, Effector T cells; EPA, Eicosapentaenoic acid; ETC, Electron transport chain; EAE, Experimental autoimmune encephalomyelitis; FAO, Fatty acid oxidation; Tfh, Follicular T helper cells; FFA, Free fatty acid; GM-CSF, Granulocyte macrophage colony-stimulating factor; LCFA, Long chain fatty acids; IFN-γ, Interferon gamma; IL, Interleukin; LDHA, Lactate dehydrogenase; mTOR, Mammalian target of rapamycin; MCFA, Medium chain fatty acids; MS, Multiple sclerosis; OXPHOS, Oxidative phosphorylation; pTreg, Peripheral Treg; PPAR, Peroxisome proliferator-activated receptor; PTEN, Phosphatase and tensin homolog; PI3K, Phosphatidylinositol 3-kinase; PDK1, Pyruvate dehydrogenase kinase 1; Rheb, Ras homologue enriched in brain; Rictor, Rapamycin-insensitive companion of TOR; Treg, Regulatory T cells; SCFA, Short chain fatty acids; TCR, T cell receptor; Th, T helper; tTreg, Thymic Treg; TGF, Transforming growth factor; TCA, Tricarboxylic acid.
References
1. Klocperk A, Grecová J, Šišmová K, Kayserová J, Froňková E, Šedivá A. Helios Expression in T-Regulatory Cells in Patients With Di George Syndrome. J Clin Immunol (2014) 34:864–70. doi: 10.1007/s10875-014-0071-y
2. Hernandez AL, Kitz A, Wu C, Lowther DE, Rodriguez DM, Vudattu N, et al. Sodiu Chlorde Inhibits the Suppressive Function of FOXP3+ Regulatory T Cells. J Clin Invest (2015) 125:4212–22. doi: 10.1172/JCI81151
3. Kleinewietfeld M, Manzel A, Titze J, Kvakan H, Yosef N, Linker R, et al. Sodium Chloride Drives Autoimmune Disease by the Induction of Pathogenic T(H)17 Cells. Nature (2013) 496:518. doi: 10.1038/nature11868
4. Pompura SL, Wagner A, Kitz A, LaPerche J, Yosef N, Dominguez-Villar M, et al. Oleic Acid Restores Suppressive Defects in Tissue-Resident FOXP3 Tregs From Patients With Multiple Sclerosis. J Clin Invest (2021) 131(2):e138519 doi: 10.1172/JCI138519
5. Haghikia A, Jörg S, Duscha A, Berg J, Manzel A, Waschbisch A, et al. Dietary Fatty Acids Directly Impact Central Nervous System Autoimmunity via the Small Intestine. Immunity (2015) 43:817–29. doi: 10.1016/j.immuni.2015.09.007
6. Mendoza A, Fang V, Chen C, Serasinghe M, Verma A, Mulle J, et al. Lymphatic Endothelial S1P Promotes Mitochondrial Function and Survival in Naive T Cells. Nature (2017) 546:158. doi: 10.1038/nature22352
7. Endo Y, Asou HK, Matsugae N, Hirahara K, Shinoda K, Tumes DJ, et al. Obesity Drives Th17 Cell Differentiation by Inducing the Lipid Metabolic Kinase, Acc1. Cell Rep (2015) 12:1042–55. doi: 10.1016/j.celrep.2015.07.014
8. Bhargava P, Calabresi PA. Metabolomics in Multiple Sclerosis. Multiple Sclerosis J (2016) 22:451–60. doi: 10.1177/1352458515622827
9. Giri S, Laila P, Singh J, Suhail H, Deshpande M, Datta I, et al. Profile of Circulatory Metabolites in Chronic Mouse Model of Multiple Sclerosis Using Untargeted Global Metabolomics. J Immunol (2014) 192:10.
10. Poisson LM, Suhail H, Singh J, Datta I, Denic A, Labuzek K, et al. Untargeted Plasma Metabolomics Identifies Endogenous Metabolite With Drug-Like Properties in Chronic Animal Model of Multiple Sclerosis. J Biol Chem (2015) 290(52):30697–712. doi: 10.1074/jbc.M115.679068
11. Warburg O, Wind F, Negelein E. The Metabolism of Tumors in the Body. J Gen Physiol (1927) 8:519–30. doi: 10.1085/jgp.8.6.519
12. Heiden MGV, Cantley LC, Thompson CB. Understanding the Warburg Effect: The Metabolic Requirements of Cell Proliferation. Science (2009) 324:1029–33. doi: 10.1126/science.1160809
13. Wang R, Dillon C, Shi LZ, Milasta S, Carter R, Finkelstein D, et al. The Transcription Factor Myc Controls Metabolic Reprogramming Upon T Lymphocyte Activation. Immunity (2011) 35:871–82. doi: 10.1016/j.immuni.2011.09.021
14. Sena LA, Li S, Jairaman A, Prakriya M, Ezponda T, Hildeman DA, et al. Mitochondria Are Required for Antigen-Specific T Cell Activation Through Reactive Oxygen Species Signaling. Immunity (2013) 38:225–36. doi: 10.1016/j.immuni.2012.10.020
15. Pollizzi KN, Patel CH, Sun IH, Oh MH, Waickman AT, Wen J, et al. Mtorc1 and Mtorc2 Selectively Regulate CD8(+) T Cell Differentiation. J Clin Invest (2015) 125:2090–108. doi: 10.1172/JCI77746
16. Yang K, Neale G, Green DR, He W, Chi H. The Tumor Suppressor Tsc1 Enforces Quiescence of Naive T Cells to Promote Immune Homeostasis and Function. Nat Immunol (2011) 12:888–U811. doi: 10.1038/ni.2068
17. Saxton RA, Sabatini DM. mTOR Signaling in Growth, Metabolism, and Disease. Cell (2017) 168:960–76. doi: 10.1016/j.cell.2017.02.004
18. Inoki K, Zhu TQ, Guan KL. TSC2 Mediates Cellular Energy Response to Control Cell Growth and Survival. Cell (2003) 115:577–90. doi: 10.1016/S0092-8674(03)00929-2
19. Frauwirth KA, Riley JL, Harris MH, Parry RV, Rathmell JC, Plas DR, et al. The CD28 Signaling Pathway Regulates Glucose Metabolism. Immunity (2002) 16:769–77. doi: 10.1016/S1074-7613(02)00323-0
20. MacIver NJ, Blagih J, Saucillo DC, Tonelli L, Griss T, Rathmell JC, et al. The Liver Kinase B1 Is a Central Regulator of T Cell Development, Activation, and Metabolism. J Immunol (2011) 187:4187–98. doi: 10.4049/jimmunol.1100367
21. Tamás P, Hawley SA, Clarke RG, Mustard KJ, Green K, Hardie DG, et al. Regulation of the Energy Sensor AMP-Activated Protein Kinase by Antigen Receptor and Ca2+ in T Lymphocytes. J Exp Med (2006) 203:1665–70. doi: 10.1084/jem.20052469
22. Blagih J, Coulombe F, Vincent EE, Dupuy F, Vázquez GC, Yurchenko Ekaterina, et al. The Energy Sensor AMPK Regulates T Cell Metabolic Adaptation and Effector Responses In Vivo. Immunity (2015) 42:41–54. doi: 10.1016/j.immuni.2014.12.030
23. Adams WC, Chen YH, Kratchmarov R, Yen B, Nish SA, Lin WHW, et al. Anabolism-Associated Mitochondrial Stasis Driving Lymphocyte Differentiation Over Self-Renewal. Cell Rep (2016) 17:3142–52. doi: 10.1016/j.celrep.2016.11.065
24. Kinnaird A, Zhao S, Wellen KE, Michelakis ED. Metabolic Control of Epigenetics in Cancer. Nat Rev Cancer (2016) 16:694–707. doi: 10.1038/nrc.2016.82
25. Chang C-H, Curtis JD, Maggi LB Jr, Faubert B, Villarino AV, O’Sullivan D, et al. Posttranscriptional Control of T Cell Effector Function by Aerobic Glycolysis. Cell (2013) 153:1239–51. doi: 10.1016/j.cell.2013.05.016
26. Tarasenko TN, Pacheco SE, Koenig MK, Gomez-Rodriguez J, Kapnick SM, Diaz F, et al. Cytochrome C Oxidase Activity Is a Metabolic Checkpoint That Regulates Cell Fate Decisions During T Cell Activation and Differentiation. Cell Metab (2017) 25:1254. doi: 10.1016/j.cmet.2017.05.007
27. Jackson SH, Devadas S, Kwon J, Pinto LA, Williams MS. T Cells Express a Phagocyte-Type NADPH Oxidase That Is Activated After T Cell Receptor Stimulation. Nat Immunol (2004) 5:818–27. doi: 10.1038/ni1096
28. Hosios AM, Hecht VC, Danai LV, Johnson MO, Rathmell JC, Steinhauser ML, et al. Amino Acids Rather Than Glucose Account for the Majority of Cell Mass in Proliferating Mammalian Cells. Dev Cell (2016) 36:540–9. doi: 10.1016/j.devcel.2016.02.012
29. Liberti MV, Locasale JW. The Warburg Effect: How Does it Benefit Cancer Cells? Trends Biochem Sci (2016) 41:211–8. doi: 10.1016/j.tibs.2015.12.001
30. Fox CJ, Hammerman PS, Thompson CB. Fuel Feeds Function: Energy Metabolism and the T-Cell Response. Nat Rev Immunol (2005) 5:844–52. doi: 10.1038/nri1710
31. Michalek RD, Gerriets VA, Jacobs SR, Macintyre AN, MacIver NJ, Mason EF, et al. Cutting Edge: Distinct Glycolytic and Lipid Oxidative Metabolic Programs Are Essential for Effector and Regulatory CD4(+) T Cell Subsets. J Immunol (2011) 186:3299–303. doi: 10.4049/jimmunol.1003613
32. Klysz D, Tai X, Robert PA, Craveiro M, Cretenet G, Oburoglu L, et al. Glutamine-Dependent Alpha-Ketoglutarate Production Regulates the Balance Between T Helper 1 Cell and Regulatory T Cell Generation. Sci Signaling (2015) 8(396):ra97. doi: 10.1126/scisignal.aab2610
33. Chornoguz O, Hagan RS, Haile A, Arwood ML, Gamper CJ, Banerjee A, et al. Mtorc1 Promotes T-Bet Phosphorylation To Regulate Th1 Differentiation. J Immunol (2017) 198:3939–48. doi: 10.4049/jimmunol.1601078
34. Macintyre AN, Gerriets VA, Nichols AG, Michalek RD, Rudolph MC, Deoliveira D, et al. The Glucose Transporter Glut1 Is Selectively Essential for CD4 T Cell Activation and Effector Function. Cell Metab (2014) 20:61–72. doi: 10.1016/j.cmet.2014.05.004
35. Peng M, Yin N, Chhangawala S, Xu K, Leslie CS, Li MO. Aerobic Glycolysis Promotes T Helper 1 Cell Differentiation Through an Epigenetic Mechanism. Science (2016) 354:481–4. doi: 10.1126/science.aaf6284
36. Cham CM, Driessens G, O’Keefe JP, Gajewski TF. Glucose Deprivation Inhibits Multiple Key Gene Expression Events and Effector Functions in CD8(+) T Cells. Eur J Immunol (2008) 38:2438–50. doi: 10.1002/eji.200838289
37. Cham CM, Gajewski TF. Glucose Availability Regulates IFN-Gamma Production and P70s6 Kinase Activation in CD8(+) Effector T Cells. J Immunol (2005) 174:4670–7. doi: 10.4049/jimmunol.174.8.4670
38. Gubser PM, Bantug GR, Razik L, Fischer M, Dimeloe S, Hoenger G, et al. Rapid Effector Function of Memory CD8(+) T Cells Requires an Immediate-Early Glycolytic Switch. Nat Immunol (2013) 14:1064. doi: 10.1038/ni.2687
39. Bailis W, Shyer JA, Zhao J, Canaveras JCG, Al Khazal FJ, Qu R, et al. Distinct Modes of Mitochondrial Metabolism Uncouple T Cell Differentiation and Function. Nature (2019) 571:403. doi: 10.1038/s41586-019-1311-3
40. Franchi L, Monteleone I, Hao L-Y, Spahr MA, Zhao W, Liu X, et al. Inhibiting Oxidative Phosphorylation In Vivo Restrains Th17 Effector Responses and Ameliorates Murine Colitis. J Immunol (2017) 198:2735–46. doi: 10.4049/jimmunol.1600810
41. Gerriets VA, Kishton RJ, Nichols AG, Macintyre AN, Inoue M, Ilkayeva O, et al. Metabolic Programming and PDHK1 Control CD4(+) T Cell Subsets and Inflammation. J Clin Invest (2015) 125:194–207. doi: 10.1172/JCI76012
42. Berod L, Friedrich C, Nandan A, Freitag J, Hagemann S, Harmrolfs K, et al. De Novo Fatty Acid Synthesis Controls the Fate Between Regulatory T and T Helper 17 Cells. Nat Med (2014) 20:1327–33. doi: 10.1038/nm.3704
43. Gaublomme JT, Yosef N, Lee Y, Gertner RS, Yang LV, Wu C, et al. Single-Cell Genomics Unveils Critical Regulators of Th17 Cell Pathogenicity. Cell (2015) 163(6):1400–12. doi: 10.1016/j.cell.2015.11.009
44. Hu X, Wang Y, Hao L-Y, Liu X, Lesch CA, Sanchez BM, et al. Sterol Metabolism Controls T(H)17 Differentiation by Generating Endogenous ROR Gamma Agonists. Nat Chem Biol (2015) 11(2):141–U184. doi: 10.1038/nchembio.1714
45. Santori FR, Huang P, van dePavert SA, Douglass EF Jr, Leaver DJ, Haubrich BA, et al. Identification of Natural ROR Gamma Ligands That Regulate the Development of Lymphoid Cells. Cell Metab (2015) 21:286–97. doi: 10.1016/j.cmet.2015.01.004
46. Wang C, Yosef N, Gaublomme J, Wu C, Lee Y, Clish CB, et al. CD5L/AIM Regulates Lipid Biosynthesis and Restrains Th17 Cell Pathogenicity. Cell (2015) 163(6):1413–27. doi: 10.1016/j.cell.2015.10.068
47. Shi LZ, Wang R, Huang G, Vogel P, Neale G, Green DR, et al. HIF1 Alpha-Dependent Glycolytic Pathway Orchestrates a Metabolic Checkpoint for the Differentiation of T(H)17 and T-Reg Cells. J Exp Med (2011) 208:1367–76. doi: 10.1084/jem.20110278
48. Delgoffe GM, Kole TP, Zheng Y, Zarek PE, Matthews KL, Xiao B, et al. The mTOR Kinase Differentially Regulates Effector and Regulatory T Cell Lineage Commitment. Immunity (2009) 30:832–44. doi: 10.1016/j.immuni.2009.04.014
49. Heikamp EB, Patel CH, Collins S, Waickman A, Oh M-H, Sun I-H, et al. The AGC Kinase SGK1 Regulates T(H)1 and T(H)2 Differentiation Downstream of the Mtorc2 Complex. Nat Immunol (2014) 15:457. doi: 10.1038/ni.2867
50. Yang J-Q, Kalim KW, Li Y, Zhang S, Hinge A, Filippi M-D, et al. RhoA Orchestrates Glycolysis for T(H)2 Cell Differentiation and Allergic Airway Inflammation. J Allergy Clin Immunol (2016) 137:231. doi: 10.1016/j.jaci.2015.05.004
51. Seumois G, Zapardiel-Gonzalo J, White B, Singh D, Schulten V, Dillon M, et al. Transcriptional Profiling of Th2 Cells Identifies Pathogenic Features Associated With Asthma. J Immunol (2016) 197:655–64. doi: 10.4049/jimmunol.1600397
52. Chen T, Tibbitt CA, Feng X, Stark JM, Rohrbeck L, Rausch L, et al. PPAR-Gamma Promotes Type 2 Immune Responses in Allergy and Nematode Infection. Sci Immunol (2017) 2(9):eaal5196. doi: 10.1126/sciimmunol.aal5196
53. Procaccini C, Carbone F, Di Silvestre D, Brambilla F, De Rosa V, Galgani M, et al. The Proteomic Landscape of Human Ex Vivo Regulatory and Conventional T Cells Reveals Specific Metabolic Requirements. Immunity (2016) 44:712–2. doi: 10.1016/j.immuni.2016.02.022
54. Beura LK, Hamilton SE, Bi K, Schenkel JM, Odumade OA, Casey KA, et al. Normalizing the Environment Recapitulates Adult Human Immune Traits in Laboratory Mice. Nature (2016) 532:512. doi: 10.1038/nature17655
55. Chapman NM, Zeng H, Nguyen T-LM, Wang Y, Vogel P, Dhungana Y, et al. mTOR Coordinates Transcriptional Programs and Mitochondrial Metabolism of Activated T-Reg Subsets to Protect Tissue Homeostasis. Nat Commun (2018) 9(1):2095. doi: 10.1038/s41467-018-04392-5
56. Asanuma S, Tanaka J, Sugita J, Kosugi M, Shiratori S, Wakasa K, et al. Expansion of CD4(+)CD25(+) Regulatory T Cells From Cord Blood CD4(+) Cells Using the Common Gamma-Chain Cytokines (IL-2 and IL-15) and Rapamycin. Ann Hematol (2011) 90:. doi: 10.1007/s00277-010-1121-z
57. Raud B, Roy DG, Divakaruni AS, Tarasenko TN, Franke R, Ma EH, et al. Etomoxir Actions on Regulatory and Memory T Cells Are Independent of Cpt1a-Mediated Fatty Acid Oxidation. Cell Metab (2018) 28:504. doi: 10.1016/j.cmet.2018.06.002
58. Sun Y, Tian T, Gao J, Liu X, Hou H, Cao R, et al. Metformin Ameliorates the Development of Experimental Autoimmune Encephalomyelitis by Regulating T Helper 17 and Regulatory T Cells in Mice. J Neuroimmunol (2016) 292:58–67. doi: 10.1016/j.jneuroim.2016.01.014
59. Lee S-Y, Lee SH, Yang E-J, Kim E-K, Kim J-K, Shin D-Y, et al. Metformin Ameliorates Inflammatory Bowel Disease by Suppression of the STAT3 Signaling Pathway and Regulation of the Between Th17/Treg Balance. PloS One (2015) 10(9):e0135858. doi: 10.1371/journal.pone.0135858
60. Gerriets VA, et al. Foxp3 and Toll-Like Receptor Signaling Balance T-Reg Cell Anabolic Metabolism for Suppression. Nat Immunol (2016) 17:1459–66. doi: 10.1038/ni.3577
61. Kopf H, de la Rosa GM, Howard OMZ, Chen X. Rapamycin Inhibits Differentiation of Th17 Cells and Promotes Generation of Foxp3+T Regulatory Cells. Int Immunopharmacol (2007) 7:1819–24. doi: 10.1016/j.intimp.2007.08.027
62. Apostolidis SA, Rodríguez-Rodríguez N, Suárez-Fueyo A, Dioufa N, Ozcan E, Crispín JC, et al. Phosphatase PP2A is Requisite for the Function of Regulatory T Cells. Nat Immunol (2016) 17:556. doi: 10.1038/ni.3390
63. Basu S, Hubbard B, Shevach EM. Foxp3-Mediated Inhibition of Akt Inhibits Glut1 (Glucose Transporter 1) Expression in Human T Regulatory Cells. J Leukocyte Biol (2015) 97:279–83. doi: 10.1189/jlb.2AB0514-273RR
64. Angelin A, Gil-de-Gómez L, Dahiya S, Jiao J, Guo L, Levine MH, et al. Foxp3 Reprograms T Cell Metabolism to Function in Low-Glucose, High-Lactate Environments. Cell Metab (2017) 25:1282. doi: 10.1016/j.cmet.2016.12.018
65. Kishore M, Cheung KCP, Fu H, Bonacina F, Wang G, Coe D, et al. Regulatory T Cell Migration Is Dependent on Glucokinase-Mediated Glycolysis. Immunity (2017) 47(5):875–889.e10. doi: 10.1016/j.immuni.2017.10.017
66. Travis MA, Sheppard D. TGF-β Activation and Function in Immunity Annu Rev Immunol (2014). 32:51-82. doi: 10.1146/annurev-immunol-032713-120257
67. Xie M, Zhang D, Dyck JRB, Li Y, Zhang H, Morishima M, et al. A Pivotal Role for Endogenous TGF-Beta-Activated Kinase-1 in the LKB1/AMP-Activated Protein Kinase Energy-Sensor Pathway. Proc Natl Acad Sci USA (2006) 103:17378–83. doi: 10.1073/pnas.0604708103
68. Gualdoni GA, Mayer K, Göschl L, Boucheron N, Ellmeier W, Zlabinge GJ. The AMP Analog AICAR Modulates the T-Reg/T(h)17 Axis Through Enhancement of Fatty Acid Oxidation. FASEB J (2016) 30:3800–9. doi: 10.1096/fj.201600522R
69. Huynh A, DuPage M, Priyadharshini B, Sage PT, Quiros J, Borges CM, et al. Control of PI(3) Kinase in T-Reg Cells Maintains Homeostasis and Lineage Stability. Nat Immunol (2015) 16:188. doi: 10.1038/ni.3077
70. Sharmahul MD, Shinde R, Mcgaha L, Huang L, Holmgaard RB, Wolchok JD, et al. The PTEN Pathway in T-Regs is a Critical Driver of the Suppressive Tumor Microenvironment. Sci Adv (2015) 1(10):e1500845. doi: 10.1126/sciadv.1500845
71. Weinberg SE, Singer BD, Steinert EM, Martinez CA, Mehta MM, Martínez-Reyes I, et al. Mitochondrial Complex III is Essential for Suppressive Function of Regulatory T Cells. Nature (2019) 565:495. doi: 10.1038/s41586-018-0846-z
72. De Rosa V, Galgani M, Porcellini A, Colamatteo A, Santopaolo M, Zuchegna C, et al. Glycolysis Controls the Induction of Human Regulatory T Cells by Modulating the Expression of FOXP3 Exon 2 Splicing Variants. Nat Immunol (2015) 16:1174–84. doi: 10.1038/ni.3269
73. Zeng H, Yang K, Cloer C, Neale G, Vogel P, Chi H. Mtorc1 Couples Immune Signals and Metabolic Programming to Establish T-Reg-Cell Function. Nature (2013) 499:485. doi: 10.1038/nature12297
74. Procaccini C, De Rosa V, Galgani M, Abanni L, Calì G, Porcellini A, et al. An Oscillatory Switch in mTOR Kinase Activity Sets Regulatory T Cell Responsiveness. Immunity (2010) 33:929–41. doi: 10.1016/j.immuni.2010.11.024
75. Rueda CM, et al, Rodríguez-Perea AL, Moreno-Fernandez M, Jackson CM, Melchior JT, Davidson WS, et al. High Density Lipoproteins Selectively Promote the Survival of Human Regulatory T Cells. J Lipid Res (2017) 58:1514–23. doi: 10.1194/jlr.M072835
76. de Jong AJ, Kloppenburg M, Toes REM, Ioan-Facsinay A. Fatty Acids, Lipid Mediators, and T-Cell Function. Front Immunol (2014) 5. doi: 10.3389/fimmu.2014.00483
77. Cury-Boaventura MF, Gorjao R, de Lima TM, Newsholme P, Curi R. Comparative Toxicity of Oleic and Linoleic Acid on Human Lymphocytes (Vol 78, Pg 1448, 2006). Life Sci (2014) 112:97–7. doi: 10.1016/j.lfs.2013.01.014
78. Takahashi HK, Cambiaghi TD, Luchessi AD, Hirabara SM, Vinolo MAR, Newsholme P, et al. Activation of Survival and Apoptotic Signaling Pathways in Lymphocytes Exposed to Palmitic Acid. J Cell Physiol (2012) 227(1):339–50. doi: 10.1002/jcp.22740
79. Howie D, Cobbold SP, Adams E, Bokum AT, Necula AS, Zhang W, et al. Foxp3 Drives Oxidative Phosphorylation and Protection From Lipotoxicity. JCI Insight (2017) 2(3):e89160. doi: 10.1172/jci.insight.89160
80. Xu S, Nam SM, Kim J-H, Das R, Choi S-K, Nguyen TT, et al. Palmitate Induces ER Calcium Depletion and Apoptosis in Mouse Podocytes Subsequent to Mitochondrial Oxidative Stress. Cell Death Dis (2015) 6(11):e1976. doi: 10.1038/cddis.2015.331
82. Calder PC. Omega-3 Fatty Acids and Inflammatory Processes. Nutrients (2010) 2(3):355–74. doi: 10.3390/nu2030355
83. Innes JK, Calder PC. Omega-6 Fatty Acids and Inflammation. Prostaglandins Leukotrienes Essential Fatty Acids (2018) 132:41–8. doi: 10.1016/j.plefa.2018.03.004
84. Field CJ, Clandinin MT. Modulation Of Adipose-Tissue Fat Composition By Diet - A Review. Nutr Res (1984) 4:743–55. doi: 10.1016/S0271-5317(84)80050-0
85. Body DR. The Lipid-Composition Of Adipose-Tissue. Prog Lipid Res (1988) 27:39–60. doi: 10.1016/0163-7827(88)90004-5
86. Corsetto PA, Montorfano G, Zava S, Jovenitti IE, Cremona A, Berra B, et al. Effects of N-3 PUFAs on Breast Cancer Cells Through Their Incorporation in Plasma Membrane. Lipids Health Dis (2011) 10:73. doi: 10.1186/1476-511X-10-73
87. Kambe T, Murakami M, Kudo I. Polyunsaturated Fatty Acids Potentiate Interleukin-1-Stimulated Arachidonic Acid Release by Cells Overexpressing Type IIA Secretory Phospholipase a(2). FEBS Lett (1999) 453:81–4. doi: 10.1016/S0014-5793(99)00702-4
88. Kolodin D, van Panhuys N, Li C, Magnuson AM, Cipolletta D, Miller C M, et al. Antigen- and Cytokine-Driven Accumulation of Regulatory T Cells in Visceral Adipose Tissue of Lean Mice. Cell Metab (2015) 21:543–57. doi: 10.1016/j.cmet.2015.03.005
89. Vasanthakumar A, Moro K, Xin A, Liao Y, Gloury R, Kawamoto S, et al. The Transcriptional Regulators IRF4, BATF and IL-33 Orchestrate Development and Maintenance of Adipose Tissue-Resident Regulatory T Cells. Nat Immunol (2015) 16:544–4. doi: 10.1038/ni0515-544d
90. Han JM, Wu D, Denroche HC, Yao Y, Verchere B, Levings MK. IL-33 Reverses an Obesity-Induced Deficit in Visceral Adipose Tissue ST2(+) T Regulatory Cells and Ameliorates Adipose Tissue Inflammation and Insulin Resistance. J Immunol (2015) 194:4777–83. doi: 10.4049/jimmunol.1500020
91. Schiering C, Krausgruber T, Chomka A, Fröhlich A, Adelmann K, Wohlfert EA, et al. The Alarmin IL-33 Promotes Regulatory T-Cell Function in the Intestine. Nature (2014) 513:564. doi: 10.1038/nature13577
92. Feuerer M, Herrero L, Cipolletta D, Naaz A, Wong J, Nayer A, et al. Lean, But Not Obese, Fat is Enriched for a Unique Population of Regulatory T Cells That Affect Metabolic Parameters. Nat Med (2009) 15:930–U137. doi: 10.1038/nm.2002
93. Farini A, Meregalli M, Belicchi M, Battistelli M, Parolini D, D’Antona G, et al. T and B Lymphocyte Depletion has a Marked Effect on the Fibrosis of Dystronhic Skeletal Muscles in the Scid/Mdx Mouse. J Pathol (2007) 213:229–38. doi: 10.1002/path.2213
94. Scharping NE, Menk AV, Moreci RS, Whetstone RD, Dadey RE, Watkins SC, et al. The Tumor Microenvironment Represses T Cell Mitochondrial Biogenesis to Drive Intratumoral T Cell Metabolic Insufficiency and Dysfunction. Immunity (2016) 45(2):374–88. doi: 10.1016/j.immuni.2016.07.009
95. Siska PJ, Rathmell JC. T Cell Metabolic Fitness in Antitumor Immunity. Trends Immunol (2015) 36:257–64. doi: 10.1016/j.it.2015.02.007
96. Howie D, Ten Bokum A, Necula AS, Cobbold SP, Waldmann H. The Role of Lipid Metabolism in T Lymphocyte Differentiation and Survival. Front Immunol (2018) 8. doi: 10.3389/fimmu.2017.01949
97. Round JL, Mazmanian SK. Inducible Foxp(3+) Regulatory T-Cell Development by a Commensal Bacterium of the Intestinal Microbiota. Proc Natl Acad Sci USA (2010) 107:12204–9. doi: 10.1073/pnas.0909122107
98. Atarashi K, Tanoue T, Shima T, Imaoka A, Kuwahara T, Momose Y, et al. Induction of Colonic Regulatory T Cells by Indigenous Clostridium Species. Science (2011) 331:337–41. doi: 10.1126/science.1198469
99. Smith PM, Howitt MR, Panikov N, Michaud M, Gallini CA, Bohlooly-Y M, et al. The Microbial Metabolites, Short-Chain Fatty Acids, Regulate Colonic T-Reg Cell Homeostasis. Science (2013) 341:569–73. doi: 10.1126/science.1241165
100. Tan J, McKenzie C, Vuillermin PJ, Goverse G, Vinuesa CG, Mebius RE, et al. Dietary Fiber and Bacterial SCFA Enhance Oral Tolerance and Protect Against Food Allergy Through Diverse Cellular Pathways. Cell Rep (2016) 15:2809–24. doi: 10.1016/j.celrep.2016.05.047
101. Zheng Y, Josefowicz S, Chaudhry A, Peng XP, Forbush K, Rudensky AY. Role of Conserved non-Coding DNA Elements in the Foxp3 Gene in Regulatory T-Cell Fate. Nature (2010) 463:808–U120. doi: 10.1038/nature08750
102. Stentz FB, Kitabchi AE. Palmitic Acid-Induced Activation of Human T-Lymphocytes and Aortic Endothelial Cells With Production of Insulin Receptors, Reactive Oxygen Species, Cytokines, and Lipid Peroxidation. Biochem Biophys Res Commun (2006) 346:721–6. doi: 10.1016/j.bbrc.2006.05.159
103. Szamel M, Rehermann B, Krebs B, Kurrle R, Resch K. Activation Signals In Human-Lymphocytes - Incorporation Of Poly-Unsaturated Fatty-Acids Into Plasma-Membrane Phospholipids Regulates Il-2 Synthesis Via Sustained Activation Of Protein Kinase-C. J Immunol (1989) 143(9):2806–13.
104. Carrillo C, Cavia MD, Alonso-Torre S. Role of Oleic Acid in Immune System; Mechanism of Action; A Review. Nutricion Hospitalaria (2012) 27(4):978–90. https://doi.org/10.3305/nh.2012.27.4.5783
105. Angela M, Endo Y, Asou HK, Yamamoto T, Tumes DJ, Tokuyama H, et al. Fatty Acid Metabolic Reprogramming via mTOR-Mediated Inductions of PPAR Gamma Directs Early Activation of T Cells. Nat Commun (2016) 7:13683. doi: 10.1038/ncomms13683
106. Palomer X, Barroso E, Zarei M, Botteri G, Vazquez-Carrera M. PPAR Beta/Delta and Lipid Metabolism in the Heart. Biochim Et Biophys Acta-Molecular Cell Biol Lipids (2016) 1861:1569–78. doi: 10.1016/j.bbalip.2016.01.019
107. Kim W, Barhoumi R, McMurray DN, Chapkin RS. Dietary Fish Oil and DHA Down-Regulate Antigen-Activated CD4+ T-Cells While Promoting the Formation of Liquid-Ordered Mesodomains. Br J Nutr (2014) 111(2):254–60. doi: 10.1017/S0007114513002444
108. Denys A, Hichami A, Khan NA. Eicosapentaenoic Acid and Docosahexaenoic Acid Modulate MAP Kinase Enzyme Activity in Human T-Cells. Mol Cell Biochem (2002) 232(1–2):143–8. doi: 10.1023/a:1014806122510
109. Bonin A, Khan NA. Regulation of Calcium Signalling by Docosahexaenoic Acid in Human T-Cells. Implication of CRAC Channels. J Lipid Res (2000) 41(2):277–4.
110. Chow SC, Jondal M. Polyunsaturated Free Fatty Acids Stimulate an Increase in Cytosolic Ca2+ by Mobilizing the Inositol 1,4,5-Trisphosphate-Sensitive Ca2+ Pool in T Cells Through a Mechanism Independent of Phosphoinositide Turnover. J Biol Chem (1990) 265(2):902–7.
111. Aires V, Hichami A, Moutairou K, Khan NA. Docosahexaenoic Acid and Other Fatty Acids Induce a Decrease in pHi in Jurkat T-Cells. Br J Pharmacol (2003) 140(7):1217–26. doi: 10.1038/sj.bjp.0705563
112. Terada S, Takizawa M, Yamamoto S, Ezaki O, Hiroshige I, Akagawa KS. Suppressive Mechanisms of EPA on Human T Cell Proliferation. Microbiol Immunol (2001) 45(6):473–81. doi: 10.1111/j.1348-0421.2001.tb02647.x
113. Zurier RB, Rosetti RG, Laposata M. Human Peripheral Blood T Lymphocyte Proliferation After Activation of the T Cell Receptor: Effects of Unsaturated Fatty Acids. Prostaglandins Leukotrienes Essential Fatty Acids (1999) 60(5–6):371–5. doi: 10.1016/s0952-3278(99)80015-5
114. Radzikowska U, Rinaldi AO, Sözener ZC, Karaguzel D, Wojcik M, Cypryk K, et al. The Influence of Dietary Fatty Acids on Immune Responses. Nutrients (2019) 11(12):2990. doi: 10.3390/nu11122990
115. Mauro C, Smith J, Cucchi D, Coe D, Fu H, Bonacina F, et al. Obesity-Induced Metabolic Stress Leads to Biased Effector Memory CD4(+) T Cell Differentiation via PI3K p110 delta-Akt-Mediated Signals. Cell Metab (2017) 25:593–609. doi: 10.1016/j.cmet.2017.01.008
116. Zhou T, Wang G, Lyu Y, Wang L, Zuo S, Zuo J, et al. Upregulation of SLAMF3 on Human T Cells is Induced by Palmitic Acid Through the STAT5-PI3K/Akt Pathway and Features the Chronic Inflammatory Profiles of Type 2 Diabetes. Cell Death Dis (2019) 10(8):559. doi: 10.1038/s41419-019-1791-y
117. Hammer A, Schliep A, Jörg S, Haghikia A, Gold R, Kleinewietfeld M, et al. Impact of Combined Sodium Chloride and Saturated Long-Chain Fatty Acid Challenge on the Differentiation of T Helper Cells in Neuroinflammation. J Neuroinflamm (2017) 14:1–9. doi: 10.1186/s12974-017-0954-y
118. Choi J-M, Bothwell ALM. The Nuclear Receptor PPARs as Important Regulators of T-Cell Functions and Autoimmune Diseases. Mol Cells (2012) 33:217–22. doi: 10.1007/s10059-012-2297-y
119. Yang XY, Wang LH, Chen T, Hodge DR, Resau JH, DaSilva L, et al. Activation of Human T Lymphocytes is Inhibited by Peroxisome Proliferator-Activated Receptor Gamma (PPAR Gamma) Agonists - PPAR Gamma Co-Association With Transcription Factor NFAT. J Biol Chem (2000) 275:4541–4. doi: 10.1074/jbc.275.7.4541
120. Clark RB, Bishop-Bailey D, Estrada-Hernandez T, Hla T, Puddington L, Padula SJ. The Nuclear Receptor PPAR Gamma and Immunoregulation: PPAR Gamma Mediates Inhibition of Helper T Cell Responses. J Immunol (2000) 164:1364–71. doi: 10.4049/jimmunol.164.3.1364
121. Wohlfert EA, Nichols FC, Nevins E, Clark RB. Peroxisome Proliferator-Activated Receptor Gamma (PPAR Gamma) and Immunoregulation: Enhancement of Regulatory T Cells Through PPAR Gamma-Dependent and -Independent Mechanisms. J Immunol (2007) 178:4129–35. doi: 10.4049/jimmunol.178.7.4129
122. Su CG, Wen X, Bailey ST, Jiang W, Rangwala SM, Keilbaugh SA, et al. A Novel Therapy for Colitis Utilizing PPAR-Gamma Ligands to Inhibit the Epithelial Inflammatory Response. J Clin Invest (1999) 104:383–9. doi: 10.1172/JCI7145
123. Desreumaux P, Dubuquoy L, Nutten S, Peuchmaur M, Englaro W, Schoonjans K, et al. Attenuation of Colon Inflammation Through Activators of the Retinoid X Receptor (RXR)/peroxisome Proliferator-Activated Receptor Gamma (BPAR Gamma) Heterodimer: A Basis for New Therapeutic Strategies. J Exp Med (2001) 193:827–38. doi: 10.1084/jem.193.7.827
124. Klotz L, Burgdorf S, Dani I, Saijo K, Flossdorf J, Hucke S, et al. The Nuclear Receptor PPAR Gamma Selectively Inhibits Th17 Differentiation in a T Cell-Intrinsic Fashion and Suppresses CNS Autoimmunity. J Exp Med (2009) 206:2079–89. doi: 10.1084/jem.20082771
125. Gocke AR, Hussain RZ, Yang Y, Peng H, Weiner J, Ben L-H, et al. Transcriptional Modulation of the Immune Response by Peroxisome Proliferator-Activated Receptor-Alpha Agonists in Autoimmune Disease. J Immunol (2009) 182:4479–87. doi: 10.4049/jimmunol.0713927
126. Polak PE, Kalinin S, Russo CD, Gavrilyuk V, Sharp A, Peters JM, et al. Protective Effects of a Peroxisome Proliferator-Activated Receptor-Beta/Delta Agonist in Experimental Autoimmune Encephalomyelitis. J Neuroimmunol (2005) 168:65–75. doi: 10.1016/j.jneuroim.2005.07.006
127. Hua XX, Wu J, Goldstein JL, Brown MS, Hobbs HH. Structure Of The Human Gene Encoding Sterol Regulatory Element-Binding Protein-1 (Srebf1) And Localization Of Srebf1 And Srebf2 To Chromosomes 17p11.2 And 22q13. Genomics (1995) 25:667–73. doi: 10.1016/0888-7543(95)80009-B
128. Kidani Y, Elsaesser H, Hock MB, Vergnes L, Williams KJ, Argus JP, et al. Sterol Regulatory Element-Binding Proteins are Essential for the Metabolic Programming of Effector T Cells and Adaptive Immunity. Nat Immunol (2013) 14:489. doi: 10.1038/ni.2570
129. Tauhid S, Neema M, Healy BC, Weiner HL, Bakshi R. MRI Phenotypes Based on Cerebral Lesions and Atrophy in Patients With Multiple Sclerosis. J Neurol Sci (2014) 346(1–2):250–4. doi: 10.1016/j.jns.2014.08.047
130. Fletcher JM, Lalor SJ, Sweeney CM, Tubridy N, Mills KHG. T Cells in Multiple Sclerosis and Experimental Autoimmune Encephalomyelitis. Clin Exp Immunol (2010) 162(1):1–11. doi: 10.1111/j.1365-2249.2010.04143.x
131. Frischer JM, Bramow S, Dal-Bianco A, Lucchinetti CF, Rauschka H, Schmidbauer M, et al. The Relation Between Inflammation and Neurodegeneration in Multiple Sclerosis Brains. Brain (2021) 132:1175–89. doi: 10.1093/brain/awp070
132. Zhang J, Weiner HL, Hafler DA. Autoreactive T Cells in Multiple Sclerosis. Int Rev Immunol (1992) 9(3):183–201. doi: 10.3109/08830189209061790
133. Zhang J, Markovic-Plese S, Lacet B, Raus J, Weiner HL, Hafler DA. Increased Frequency of Interleukin 2-Responsive T Cells Specific for Myelin Basic Protein and Proteolipid Protein in Peripheral Blood and Cerebrospinal Fluid of Patients With Multiple Sclerosis. J Exp Med (1994) 179(3):973–84. doi: 10.1084/jem.179.3.973
134. Raine CS. The Dale E. McFarlin Memorial Lecture: The Immunology of the Multiple Sclerosis Lesion. Ann Neurol (1994) 36 Suppl:S61–72. doi: 10.1002/ana.410360716
135. Peeters LM, Vanheusden M, Somers V, Wijmeersch BV, Stinissen P, Broux Bieke, et al. Cytotoxic CD4+ T Cells Drive Multiple Sclerosis Progression. Front Immunol (2017) 8. doi: 10.3389/fimmu.2017.01160
136. Fletcher JM, Lalor SJ, Sweeney CM, Tubridy N, Mills KHG. Roles of CD4 and CD8 T Lymphocytes in Multiple Sclerosis and Experimental Autoimmune Encephalomyelitis - Neuroinflammation - Wiley Online Library. Clin Exp Immunol (2010) 162(1):1-11.
137. Kaskow BJ, Baecher-Allan C. Effector T Cells in Multiple Sclerosis. Cold Spring Harbor Perspect Med (2018) 8(4):a029025. doi: 10.1101/cshperspect.a029025
138. Linterman MA, Beaton L, Yu D, Ramiscal RR, Srivastava M, Hogan JJ, et al. IL-21 Acts Directly on B Cells to Regulate Bcl-6 Expression and Germinal Center Responses. J Exp Med (2010) 207(2):353–63. doi: 10.1084/jem.20091738
139. Batten M, Ramamoorthi N, Kljavin NM, Ma CS, Cox JH, Dengler HS, et al. IL-27 Supports Germinal Center Function by Enhancing IL-21 Production and the Function of T Follicular Helper Cells. J Exp Med (2010) 207(13):2895–906. doi: 10.1084/jem.20100064
140. Tzartos JS, Craner MJ, Friese MA, Jakobsen KB, Newcombe J, Esiri MM, et al. IL-21 and IL-21 Receptor Expression in Lymphocytes and Neurons in Multiple Sclerosis Brain. Am J Pathol (2011) 178(2):794–802. doi: 10.1016/j.ajpath.2010.10.043
141. Romme CJ, Börnsen L, Ratzer R, Piehl F, Khademi M, Olsson T, et al. Systemic Inflammation in Progressive Multiple Sclerosis Involves Follicular T-Helper, Th17- and Activated B-Cells and Correlates With Progression. PloS One (2013) 8(3):e57820. doi: 10.1371/journal.pone.0057820
142. Morita R, Schmitt N, Bentebibel SA, Ranganathan R, Bourdery L, Zurawski G, et al. Human Blood CXCR5(+)CD4(+) T Cells are Counterparts of T Follicular Cells and Contain Specific Subsets That Differentially Support Antibody Secretion. Immunity (2011) 34(1):108–21. doi: 10.1016/j.immuni.2010.12.012
143. Brucklacher-Waldert V, Stuerner K, Kolster M, Wolthausen J, Tolosa E. Phenotypical and Functional Characterization of T Helper 17 Cells in Multiple Sclerosis. Brain J Neurol (2009) 132(Pt 12):3329–41. doi: 10.1093/brain/awp289
144. Frisullo G, Nociti N, Iorio R, Patanella AK, Marti A, Caggiula M, et al. IL17 and IFNgamma Production by Peripheral Blood Mononuclear Cells From Clinically Isolated Syndrome to Secondary Progressive Multiple Sclerosis. Cytokine (2008) 44(1):22–. doi: 10.1016/j.cyto.2008.08.007
145. Leung S, Liu X, Fang L, Chen X, Guo T, Zhang J. The Cytokine Milieu in the Interplay of Pathogenic Th1/Th17 Cells and Regulatory T Cells in Autoimmune Disease. Cell Mol Immunol (2010) 7(3):182–9. doi: 10.1038/cmi.2010.22
146. Harbour SN, Maynard CL, Zindl CL, Schoeb TR, Weaver CT. Th17 Cells Give Rise to Th1 Cells That are Required for the Pathogenesis of Colitis. Proc Natl Acad Sci USA (2015) 112(22):7061–6. 10.1073/pnas.1415675112
147. Uchiyama R, Yonehara S, Taniguchi S, Ishido S, Ishii KJ, Tsutsui H, et al. Inflammasome and Fas-Mediated IL-1β Contributes to Th17/Th1 Cell Induction in Pathogenic Bacterial Infection In Vivo. J Immunol (2017) 199(3):1122–30. doi: 10.4049/jimmunol.1601373
148. Verstappen GM, Corneth OBJ, Bootsma H, Kroese FGM. Th17 Cells in Primary Sjogren ‘ s Syndrome: Pathogenicity and Plasticity. J Autoimmun (2018) 87:16–25. doi: 10.1016/j.jaut.2017.11.003
149. Kebir H, Ifergan I, Alvarez JI, Bernard M, Poirier J, Arbour N, et al. Preferential Recruitment of Interferon-Gamma-Expressing TH17 Cells in Multiple Sclerosis. Ann Neurol (2009) 66(3):390–402. doi: 10.1002/ana.21748.
150. Domingues HS, Mues M, Lassmann H, Wekerle H, Krishnamoorthy G. Functional and Pathogenic Differences of Th1 and Th17 Cells in Experimental Autoimmune Encephalomyelitis. PloS One (2010) 5(11):e15531. doi: 10.1371/journal.pone.0015531
151. Dos Passos GR, Sato DK, Becker J, Fujihara K. Th17 Cells Pathways in Multiple Sclerosis and Neuromyelitis Optica Spectrum Disorders: Pathophysiological and Therapeutic Implications. Mediators Inflammation (2016) 2016:5314541. doi: 10.1155/2016/5314541
152. Sie C, Korn T, Mitsdoerffer M. Th17 Cells in Central Nervous System Autoimmunity. Exp Neurol (2014) 262 Pt A. doi: 10.1016/j.expneurol.2014.03.009
153. Martin F, Apetoh L, Ghiringhelli F. Controversies on the Role of Th17 in Cancer: A TGF-β-Dependent Immunosuppressive Activity? Trends Mol Med (2012) 18. doi: 10.1016/j.molmed.2012.09.007
154. Zielinski CE, Mele F, Aschenbrenner D, Jarrossay D, Ronchi F, Gattorno M, et al. Pathogen-Induced Human T(H)17 Cells Produce IFN-Gamma or IL-10 and are Regulated by IL-1 Beta. Nature (2012) 484(7395):514–518. https://doi.org/10.1038/nature10957.
155. Ustun C, Miller JS, Munn DH, Weisdorf DJ, Blazar BR. Regulatory T Cells in Acute Myelogenous Leukemia: Is it Time for Immunomodulation? Blood (2011) 118. doi: 10.1182/blood-2011-07-365817
156. Ye J, Livergood RS, Peng G. The Role and Regulation of Human Th17 Cells in Tumor Immunity. Am J Pathol (2013) 182(1):10–20. doi: 10.1016/j.ajpath.2012.08.041
157. Dominguez-Villar M, Baecher-Allan CM, Hafler DA. Identification of T Helper Type 1-Like, Foxp3+ Regulatory T Cells in Human Autoimmune Disease. Nat Med (2011) 17:673–5. doi: 10.1038/nm.2389
158. McClymont SA, Putnam AL, Lee MR, Esensten JH, Liu W, Hulme MA, et al. Plasticity of Human Regulatory T Cells in Healthy Subjects and Patients With Type 1 Diabetes. J Immunol (2011) 186:3918–26. doi: 10.4049/jimmunol.1003099
159. Kitz A, de Marcken M, Gautron AS, Mitrovic MM, Hafler DA, Dominguez-Villar M. AKT Isoforms Modulate Th1-Like Treg Generation and Function in Human Autoimmune Disease. EMBO Rep (2016) 17:1169–83. doi: 10.15252/embr.201541905
160. Kitz A, Dominguez-Villar M. Molecular Mechanisms Underlying Th1-Like Treg Generation and Function. Cell Mol Life Sci (2017) 74:4059–75. doi: 10.1007/s00018-017-2569-y
161. de Carvalho JF, Pereira RM, Shoenfeld Y. The Mosiac of Autoimmunity: The Role of Environmental Factors. Front Biosci (2009) 1(2):501–9. https://doi.org/10.2741/e46
162. Munz C, Lunemann JD, Getts MT, Miller SD. Antiviral Immune Responses: Triggers of or Triggered by Autoimmunity? Nat Rev Immunol (2009) 9:246–58. doi: 10.1038/nri2527
163. Wells , Hodans F, Jean C, Buzby. Dietary Assessment of Major Trends in U.S. Food Consumption, 1970-2005. Economic Information Bulletin No. 33. Economic Research Service, U.S. Dept. of Agriculture. March 2008.
164. Hedstrom AK, Olsson T, Alfredsson L. High Body Mass Index Before Age 20 is Associated With Increased Risk for Multiple Sclerosis in Both Men and Women. Mult Scler (2012) 18:1334–6. doi: 10.1177/1352458512436596
165. Munger KL, Chitnis T, Ascherio A. Body Size and Risk of MS in Two Cohorts of US Women. Neurology (2009) 73:1543–50. doi: 10.1212/WNL.0b013e3181c0d6e0
166. Guma M, Tiziani S, Firestein GS. Metabolomics in Rheumatic Diseases: Desperately Seeking Biomarkers. Nat Rev Rheumatol (2016) 12:269–81. doi: 10.1038/nrrheum.2016.1
167. Nogueras L, Gonzalo H, Jové́ M, Sol J, Gil-Sanchez A, Hervá́s JV, et al. Lipid Profile of Cerebrospinal Fluid in Multiple Sclerosis Patients: A Potential Tool for Diagnosis. Sci Rep (2019) 9:1–9. doi: 10.1038/s41598-019-47906-x
168. Lewkowicz N, Piątek P, Namiecińska M, Domowicz M, Bonikowski R, Szemraji J, et al. Naturally Occurring Nervonic Acid Ester Improves Myelin Synthesis by Human Oligodendrocytes. Cells (2019) 8. doi: 10.3390/cells8080786
169. Ferreira HB, Melo T, Monteiro A, Paiva A, Domingues P, Domingues MR. Serum Phospholipidomics Reveals Altered Lipid Profile and Promising Biomarkers in Multiple Sclerosis. Arch Biochem Biophys (2021) 697:108672. doi: 10.1016/j.abb.2020.108672
170. Chung Y, Chang SH, Martinez GJ, Yang XO, Nurieva R, Kang HS, et al. Critical Regulation of Early Th17 Cell Differentiation by Interleukin-1 Signaling. Immunity (2009) 30:576–87. doi: 10.1016/j.immuni.2009.02.007
171. Ghoreschi K, Laurence A, Yang X-P, Tato CM, McGeachy MJ, Konkel JE, et al. Generation of Pathogenic T(H)17 Cells in the Absence of TGF-Beta Signalling. Nature (2010) 467(7318):967–971. https://doi.org/10.1038/nature09447
172. Heink S, Yogev N, Garbers C, Herwerth M, Aly L, Gasperi C, et al. Trans-Presentation of IL-6 by Dendritic Cells is Required for the Priming of Pathogenic T(H)17 Cells. Nat Immunol (2017) 18:74–85. doi: 10.1038/ni.3632
173. Jain R, Chen Y, Kanno Y, Joyce-Shaikh B, Vahedi G, Hirahara K, et al. Interleukin-23-Induced Transcription Factor Blimp-1 Promotes Pathogenicity of T Helper 17 Cells. Immunity (2016) 44:131–42. doi: 10.1016/j.immuni.2015.11.009
174. Lee Y, Awasthi A, Yosef N, Quintana FJ, Xiao S, Peters A, et al. Induction and Molecular Signature of Pathogenic T(H)17 Cells. Nat Immunol (2012) 13:991–9. doi: 10.1038/ni.2416
175. Mailer RKW, Joly AL, Liu S, Elias S, Tegner J, Andersson J. IL-1 Beta Promotes Th17 Differentiation by Inducing Alternative Splicing of FOXP3. Sci Rep (2015) 5. doi: 10.1038/srep14674
176. Pfeifle R, Rothe T, Ipseiz N, Scherer HU, Culemann S, Harre U, et al. Regulation of Autoantibody Activity by the IL-23-T(H)17 Axis Determines the Onset of Autoimmune Disease. Nat Immunol (2017) 18:104–13. doi: 10.1038/ni.3579
177. La Rocca C, Carbone F, De Rosa V, Colamatteo A, Galgani M, Perna F, et al. Immunometabolic Profiling of T Cells From Patients With Relapsing-Remitting Multiple Sclerosis Reveals an Impairment in Glycolysis and Mitochondrial Respiration. Metabol: Clin Exp (2017) 77:39–46. doi: 10.1016/j.metabol.2017.08.011
178. Jacobs SR, Herman CE, Maciver NJ, Wofford JA, Wieman HL, Hammen JJ, et al. Glucose Uptake is Limiting in T Cell Activation and Requires CD28-Mediated Akt-Dependent and Independent Pathways. J Immunol (2008) 180:4476–86. doi: 10.4049/jimmunol.180.7.4476
179. Yin Y, Choi SC, Xu Z, Perry DJ, Seay H, Crocker BP, et al. Normalization of CD4(+) T Cell Metabolism Reverses Lupus. Sci Trans Med (2015) 7. doi: 10.1126/scitranslmed.aaa0835
180. Van de KC, Killestein J, Popescu V, Rijkers E, Vrenken H, Lutjohann D, et al. Oxysterols and Cholesterol Precursors Correlate to Magnetic Resonance Imaging Measures of Neurodegeneration in Multiple Sclerosis. Multiple Sclerosis (Houndmills Basingstoke England) (2014) 20(4):412–7. doi: 10.1177/1352458513499421
181. Uher T, Fellows K, Horakova D, Zivadinov R, Vaneckova M, Sobisek L, et al. Serum Lipid Profile Changes Predict Neurodegeneration in Interferon-β1a-Treated Multiple Sclerosis Patients. J Lipid Res (2017) 58(2):403–411. doi: 10.1194/jlr.M072751
182. Cantuti-Castelvetri L, Fitzner D, Bosch-Queralt M, Weil MT, Su M, Sen P, et al. Defective Cholesterol Clearance Limits Remyelination in the Aged Central Nervous System. Sci (New York NY) (2018) 359(6376):684–688. doi: 10.1126/science.aan4183
183. Hubler Z, Allimuthu D, Bederman I, Elitt MS, Madhavan M, Allan KC, et al. Accumulation of 8,9-Unsaturated Sterols Drives Oligodendrocyte Formation and Remyelination. Nature (2018) 560(7718):372–376. doi: 10.1038/s41586-018-0360-3
184. Spann NJ, Glass CK. Sterols and Oxysterols in Immune Cell Function. Nat Immunol (2013) 14(9):893–900. doi: 10.1038/ni.2681
185. Lochner M, Berod L, Sparwasser T. Fatty Acid Metabolism in the Regulation of T Cell Function. Trends Immunol (2015) 36:81–91. doi: 10.1016/j.it.2014.12.005
186. Grassi S, Giussani P, Mauri L, Prioni S, Sonnino S, Prinetti A. Lipid Rafts and Neurodegeneration: Structural and Functional Roles in Physiologic Aging and Neurodegenerative Diseases. J Lipid Res (2020) 61(5):636–654. doi: 10.1194/jlr.TR119000427
187. Youssef S, Stüve O, Patarroyo JC, Ruiz PJ, Radosevich JL, Hur EM, et al. The HMG-CoA Reductase Inhibitor, Atorvastatin, Promotes a Th2 Bias and Reverses Paralysis in Central Nervous System Autoimmune Disease. Nature (2002) 420:78–84. doi: 10.1038/nature01158
188. Zhang X, Tao Y, Troiani L, Markovic-Plese S. Simvastatin Inhibits IFN Regulatory Factor 4 Expression and Th17 Cell Differentiation in CD4(+) T Cells Derived From Patients With Multiple Sclerosis. J Immunol (2011) 187:3431–7. doi: 10.4049/jimmunol.1100580
189. Zhang X, Tao Y, Wang J, Garcia-Mata R, Markovic-Plese S. Simvastatin Inhibits Secretion of Th17-Polarizing Cytokines and Antigen Presentation by DCs in Patients With Relapsing Remitting Multiple Sclerosis. Eur J Immunol (2013) 43(1):281–9. doi: 10.1002/eji.201242566
190. Xia M, Hu S, Fu Y, Jin W, Yi Q, Matsui Y, et al. CCR10 Regulates Balanced Maintenance and Function of Resident Regulatory and Effector T Cells to Promote Immune Homeostasis in Skin. J Allergy Clin Immunol (2014) 134:634–44. doi: 10.1016/j.jaci.2014.03.010
191. Burzyn D, Kuswanto W, Kolodin D, Shadrach J, Cerletti M, Jang Y, et al. A Special Population of Regulatory T Cells Potentiates Muscle Repair. Cell (2013) 155:1282–95. doi: 10.1016/j.cell.2013.10.054
Keywords: T cell, multiple sclerosis, immunometabolism, fatty acids, T regulatory (Treg) cell
Citation: Pompura SL, Hafler DA and Dominguez-Villar M (2022) Fatty Acid Metabolism and T Cells in Multiple Sclerosis. Front. Immunol. 13:869197. doi: 10.3389/fimmu.2022.869197
Received: 04 February 2022; Accepted: 30 March 2022;
Published: 04 May 2022.
Edited by:
Manolo Sambucci, Laboratory of Neuroimmunology, Santa Lucia Foundation (IRCCS), ItalyReviewed by:
Veit Rothhammer, University Hospital FAU Erlangen Neurnberg, GermanyJoseph Barbi, University at Buffalo, United States
Copyright © 2022 Pompura, Hafler and Dominguez-Villar. This is an open-access article distributed under the terms of the Creative Commons Attribution License (CC BY). The use, distribution or reproduction in other forums is permitted, provided the original author(s) and the copyright owner(s) are credited and that the original publication in this journal is cited, in accordance with accepted academic practice. No use, distribution or reproduction is permitted which does not comply with these terms.
*Correspondence: Margarita Dominguez-Villar, bS5kb21pbmd1ZXotdmlsbGFyQGltcGVyaWFsLmFjLnVr