- 1Faculty of Medicine, Wroclaw Medical University, Wroclaw, Poland
- 2Faculty of Pharmacy, Wroclaw Medical University, Wroclaw, Poland
- 3Department of Molecular and Cellular Biology, Faculty of Pharmacy, Wroclaw Medical University, Wroclaw, Poland
Cancer cells possess specific properties, such as multidrug resistance or unlimited proliferation potential, due to the presence of specific proteins on their cell membranes. The release of proliferation-related proteins from the membrane can evoke a loss of adaptive ability in cancer cells and thus enhance the effects of anticancer therapy. The upregulation of cancer-specific membrane antigens results in a better outcome of immunotherapy. Moreover, cytotoxic T-cells may also become more effective when stimulated ex-vivo toward the anticancer response. Therefore, the modulation of membrane proteins may serve as an interesting attempt in anticancer therapy. The presence of membrane antigens relies on various physical factors such as temperature, exposure to radiation, or drugs. Therefore, changing the tumor microenvironment conditions may lead to cancer cells becoming sensitized to subsequent therapy. This paper focuses on the therapeutic approaches modulating membrane antigens and enzymes in anticancer therapy. It aims to analyze the possible methods for modulating the antigens, such as pharmacological treatment, electric field treatment, photodynamic reaction, treatment with magnetic field or X-ray radiation. Besides, an overview of the effects of chemotherapy and immunotherapy on the immunophenotype of cancer cells is presented. Finally, the authors review the clinical trials that involved the modulation of cell immunophenotype in anticancer therapy.
Introduction
‘Membrane antigens’ is a term used by researchers to refer to the molecules present on the cell membrane that may be recognized by immune system cells. Modulation of membrane components remains a unique approach in anticancer therapy (1). By the sensitization of cancer, scientists aim to overexpress tumor-specific molecules found on cell membranes (2–6). This method attempts to overcome the immune escape of cancer cells and aims to make single cancer cells visible to the immune system. The controlled regulation of the immunophenotype of cells can overexpress or downregulate the expression of specific plasma membrane-associated antigens (7, 8). The method opens a new perspective for sensitizing the cells to the subsequent standard therapy and expecting a better clinical outcome (5).
Immunotherapy focuses on targeting cancer-related antigens on the cell surface (4). The more an antigen is specific to cancer, the fewer side effects occur. For instance, when targeting CD20 in leukaemia therapy, it is blood and bone marrow that are most affected (9). However, when MAGE receptors are targeted in melanoma therapy, testes and blood are affected as well (10). The efficacy of the treatment also depends on the abundance of the targeted antigen on cancer cells. Specifically, the more targets, the higher the probability of recognizing cancer cells by antibodies or T-cell receptors (TCR) (11).
Tumor immunotherapy may be based on cellular therapeutics or typical immunotherapy agents such as antibodies (12, 13). Both options rely on targeting tumor cells by binding to a specific epitope. When a specific antigen is targeted with an antibody, the cell with the attached antibody might become an ideal target for immune cells (14). Moreover, the antibody-antigen interaction alone may reduce the activity of the targeted protein or even decrease its enzymatic activity (15). Sometimes the antibody remains conjugated with the radioisotope or chemotherapy agent (16, 17). In such cases, the cytotoxic effect is stronger and more specific than in systemic therapy. Conversely, when the reaction with the antigen depends on the interaction of TCR or membrane-bound immunoglobulin with the antigen, then the whole T-cell is involved in the interaction with the target (18). The abundance of the target of immunotherapy affects the immune recognition of the alien cell (19).
There are at least three mechanisms that affect the targeting of cancer cells. The first one involves the loss of human leukocyte antigen (HLA) in the least differentiated cancers. Here, the alien cell should be eliminated by NK cells due to the lack of the major histocompatibility complex (MHC) I molecules in the missing-self mechanism (20). However, in some cases, NK cells might not kill a cell lacking MHC (21). The second factor is the presence of cancer-specific molecules that may become targets of the therapy (22). An ideal target should be specific to cancer and highly abundant on cancer cells. However, tumors differ in the expression of cancer-specific molecules (23–27). In general, high expression of cancer antigen is required for effective therapy and may be effectively treated with targeted immunotherapy (19). The third option – the PD-1 and CTLA-4 molecule pathways show that the effects of the expression of specific target molecules might be depleted by the immune escape of the cancer cell (28). The cell induces the expression of molecules that prevent/inhibit the activation of the lymphocyte and thus anticancer activity. Interestingly, some studies showed an attempt to target the tumor microenvironment, even presenting the advantages of this method over the blockade of immune checkpoints in the tumor (29, 30).
The paper reviews various methods for the regulation of membrane antigens. First, it presents an overview of the effects of chemotherapy on immunotherapy-related antigens on cancer cells. In the next paragraphs, electroporation, photodynamic therapy, x-ray radiation, and the application of ultrasounds or magnetic fields are reviewed in case of the modulatory effects on membrane antigens. Finally, the authors present the clinical trials involving the modulation of membrane antigens in anticancer therapy.
Methods for Modulation of Membrane Antigens
Chemotherapy and Pharmacotherapy
Chemotherapy may modulate the expression of membrane antigens via several distinct pathways. First, some drugs can interact with transcription factors, directly affecting the expression of the protein (31). Second, chemotherapy agents might interact with the already synthesized protein. The interaction can inhibit or stimulate the protein’s function (32, 33). When a chemotherapy drug enhances the activity of the membrane protein, no additional units of the protein are required. Conversely, when the interaction remains inhibitory or the ligand blocks the receptor’s binding pocket and when units of a new protein are required for cell functioning, the new protein may be biosynthesized, and thus the overall expression of the protein may increase (34). However, when a negative interaction between ligand and the receptor occurs, sometimes no additional protein is synthesized. For instance, when the chemotherapy agent blocks the receptor, which transmits the biological signal, the cell might shift toward the alternative signaling pathway and then the ligand-receptor interaction does not affect the expression of the protein (35).
SERMs
The first group of chemotherapy drugs that modulate the expression of hormone receptors on cells is hormone agonists or antagonists. Hormone agonists, via binding to the receptor, may stimulate the receptor and transduce the hormonal signal to the nucleus (36). On the other hand, the binding of the agonist might lead to the blockage of the receptor, and therefore, the hormones cannot act at the cellular level. Overexpression of the receptor may overcome the blockage of the receptor and result in the cell becoming sensitive to hormonal stimulation. Such tendencies are mostly observed in prostate and breast cancers (37–39). The latter is connected with the expression of estrogen receptor which is a predictive and prognostic marker in hormone-dependent cancers.
Selective estrogen receptor modulators (SERMs) are commonly used in breast tumors expressing estrogen receptor-α (ERα) (40). One of them, tamoxifen, is a prodrug metabolized by the cytochrome P450 system to 4-hydroxytamoxifen – a pharmacologically active molecule. The estrogen receptor consists of two subunits: α (ERα) and β (ERβ) and their proportions depend on the tissue. ERα plays a significant role in the development of the mammary gland, bone mineral density and the hypothalamic-pituitary axis, while ERβ, among others, regulates ovulation. The ligand (estrogen) binds to the ER and leads to receptor activation, next step is the recruitment of coactivators or corepressors involved in the transcription process. The ligand-receptor complex ultimately activates or inhibits the production of a specific protein by the cell (41). In breast cancer, estrogens stimulating ERα increase proliferation, while activating ERβ inhibits the ability to reproduce. Reduced proliferation is associated with the activation or inhibition of the expression of genes regulated by estrogen receptors and the modulation of cell signaling pathways (42, 43). A study by Saji et al. suggests that the presence of ERβ is beneficial in the hormonal treatment of ER-positive breast cancer (44). Long-term blockade of the estrogen receptor by SERMs (especially tamoxifen) results in the development of resistance that may also engage cross-talk pathways. It leads to increased ER expression in breast cancer cells associated with receptor activation and hypersensitivity to low estrogen levels (45). Overexpression of membrane antigens such as ERBB2 (HER2/neu) and/or epidermal growth factor receptor (EGFR/HER1) is associated with a significant reduction of response to treatment with tamoxifen but greater sensitivity to letrozole (46). Long-term stimulation of estrogen receptors in the endometrium may induce proliferation and increase the risk of uterine cancer (41).
The expression of ERβ in cancer may lead to the inhibition of tumor growth (47). For this reason, ERβ agonists may find application in the prevention or treatment of colorectal cancer in which the levels of these receptors are reduced compared to normal expression in the colorectal epithelium (42, 48). Prinaberel (ERB-041, strong and selective ERβ stimulator) inhibits proliferation, increases tumor cell death by modulating the expression of specific genes (49). Studies show that CXC motif chemokine receptor 4 (CXCR4) expression is decreased during treatment with two selective ERβ agonists: ERB-041 and liquiritigenin (47). CXCR4 is a transmembrane protein and, together with ligand stromal cell-derived factor-1 (SDF-1) is relevant in the invasion of cancer cells (50). Stimulation of the β subunit of the estrogen receptor has the same effect in prostate cancer (51). Therefore, tamoxifen and raloxifene reduce cell migration, prevent the development of prostate cancer and the formation of metastases (52). It is worth noting that cell death in prostate cancer occurs as a result of androgen-independent apoptosis connected with the activation of ERβ (51). The activation of ERβ by a selective ERβ agonist diarylpropionitrile (DPN) increases the adhesion of breast cancer cells by enhancing the surface expression of integrin α1 and integrin β1 (53). The effect also reduces the migration of neoplastic cells (53). ERb1 affects the up-regulation of E-cadherin expression by inhibiting its transcriptional repressors ZEB1/2 and up-regulating miR-200a, miR-200b and miR-429 in basal-like breast cancer (54). Cadherins are adhesion proteins that are important for maintaining tight cell connections. Stimulation of the β subunit of the estrogen receptor in androgen-independent prostate cancer cells leads to the modulation of the expression of adhesion proteins. DPN downregulates N-cadherin expression in PC-3 cells and increases the expression of E-cadherin and β-catenin in the cell membrane of the DU-145 line (55). This promising result prompted a search for more ERβ agonists which may have important therapeutic significance. Besides, ERβ downregulates EGFR transcription (56).
Estrogen receptor antagonists are effective drugs in hormone-dependent breast cancer. One of them, fulvestrant (ICI 182,780) is a pure antagonist with a high binding affinity, devoid of agonist activity in other tissues as compared to SERMs. As a result of impaired dimerization, the receptor cannot stimulate or inhibit the transcription of target genes (57). It inhibits the nuclear transport of ER and contributes to proteasome-mediated ER destruction, therefore it is classified as a selective estrogen receptor degrader (SERD) (58, 59). The advantage of fulvestrant over tamoxifen is that it reduces the level of estrogen receptors (ER) and progesterone receptors in cancer cells (57). Fulvestrant is indicated especially in locally advanced or metastatic hormone receptor-positive (HR+) and human epidermal growth factor 2 negative (HER2-) breast cancer in postmenopausal women (60, 61).
Aromatase Inhibitors
Aromatase inhibitors (AI) are essential in treating ER-positive breast cancer in women after menopause. Aromatase is an enzyme that is involved in the final step in steroid biosynthesis, which is the conversion of androgens into estrogens (62). Third-generation AI are divided into steroidal or type I (structural similarity to androstenedione – a natural ligand) and non-steroidal (type II) aromatase inhibitors. Type I steroidal drugs include formestane and exemestane. Exemestane binds directly to the androstenedione binding site and consequently has an irreversible inhibitory effect. It competes with androstenedione and testosterone (63), reducing estrogen levels, which inhibits hormone-stimulated breast cancer cells. Exemestane exhibits modest androgenic activity, which may prevent increased bone loss compared to non-steroidal aromatase inhibitors (64). Anastrozole and letrozole are classified as nonsteroidal type II inhibitors. They effectively and reversibly inhibit the activity of aromatase. Meta-analyses of phase 3 randomized controlled trials prove that with aromatase inhibitors, progression-free survival (PFS) is longer, although no significant change in overall survival in postmenopausal women with HR+ advanced breast cancer has been noted (65).
Aromatase inhibitors are considered effective second-line agents following the occurrence of tamoxifen-induced drug resistance (66). However, breast cancer cells may also exhibit resistance to aromatase inhibitors. Drug-induced estrogen deprivation results in hypersensitivity of estrogen receptors to residual estrogen levels. One of the reasons for the decreased response to treatment is the adaptive increase in the expression of estrogen receptors. Stimulation of ER is also connected with the intensified expression of ERBB2 (HER2/neu)/ERBB3, mitogen-activated protein kinases (MAPKs) and insulin-like growth factor IGF-receptor signaling caused by long-term estrogen deprivation (LTED) (45). ERs are mainly nuclear receptors, but it is worth noting that they are also present in the cytoplasm and cell membrane, because they originate from the same transcript (67). Extranuclear ER after binding estrogen interacts with transmembrane EGFR and insulin-like growth factor-I receptor (IGF-IR) to initiate signaling for cell proliferation (68). As a result of activation of the membrane receptor, neoplastic cells will proliferate and increase the tumor mass (68).
Androgen Deprivation Therapy
Androgen deprivation therapy (ADT) can lead to the modulation of prostate-specific membrane antigen (PSMA) levels in prostate cancer patients. The reason is the use of drugs that reduce the concentration of androgens, including luteinizing hormone-releasing hormone (LHRH) agonists, such as leuprolide or goserelin LHRH antagonists, for example, degarelix. Androgen receptor (AR) inhibitors such as bicalutamide, flutamide or enzalutamide, and androgen synthesis inhibitors – abiraterone, also cause androgen deprivation. Previous studies suggest that short-term ADT increases PSMA expression, while long-term ADT has the opposite effect (7, 8, 69). The up-regulation of prostate-specific membrane antigen expression may be an attractive target of therapy in the future. Phase 1 of the clinical trial proves that an antibody-drug conjugate (ADC) targeting PSMA has specific anti-tumor activity not only in the preclinical model. PSMA ADC consists of a fully human monoclonal IgG1 antibody conjugated to monomethyl auristatin E (MMAE) through a dipeptide linker (valine-citrulline) that disintegrates inside the tumor cell. MMAE selectively binds to PSMA-positive cells, it inhibits microtubule polymerization, resulting in cell cycle arrest and cell death (70). The quick internalization of membrane antigen improves the transport of the conjugate into the cell (71).
Standard Chemotherapy Agents and Natural Compounds
Docetaxel increases surface expression of the carcinoembryonic antigen (CEA), calreticulin (CRT), mucin-1 (MUC-1) and Fas in cancer cells. CRT is expressed on the surface of dying cells, which allows dendritic cells to present antigens. A higher concentration of CRT enables a faster and more effective response of the immune system (72). Docetaxel-resistant cells also showed elevated levels of Fas or CEA and were lysed by cytotoxic T lymphocytes (CTL) after exposure to docetaxel. This proves that cancer cells, despite not responding to this drug, are immunogenically modulated. In the case of prostate cancer in humans, the presence of docetaxel resulted in increased levels of prostate-specific antigen (PSA), prostate stem cell antigen (PSCA) and PSMA. This drug leads to high sensitivity to antigen-specific CTL killing (1).
The effects of chemotherapeutics on the expression of membrane proteins are summarized in Figure 1.
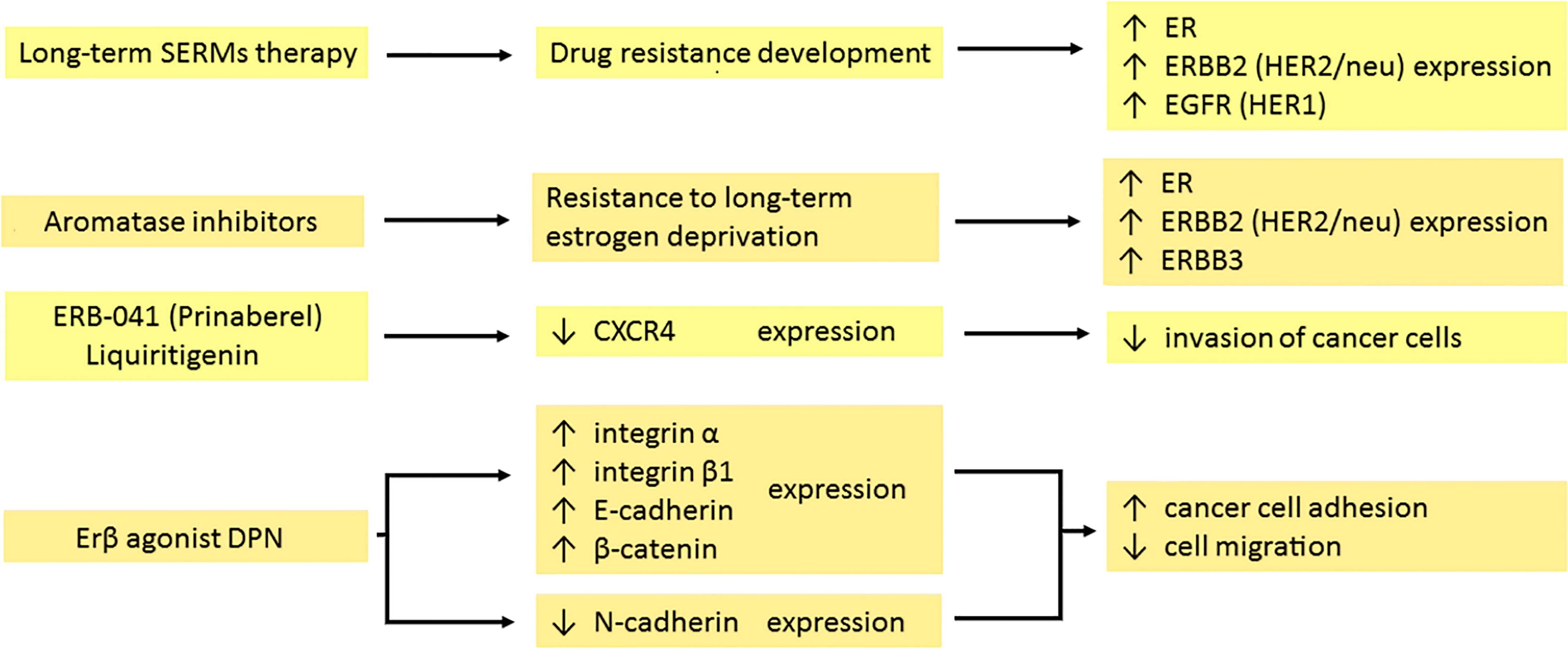
Figure 1 Effects of hormonal therapy of the tumour on the expression of membrane antigens. Long-term therapy with both selective estrogen receptor modulators (SERMs) and aromatase inhibitors (AI) leads to the development of drug resistance. In response, cancer cells increase the expression of several receptors: ER, ERBB2 (HER2/neu), ERBB3 and EGFR (HER1). Prinaberel and liquiritigenin downregulate CXCR4 expression, reducing tumour growth, cell proliferation and invasion. DPN as a selective agonist of the β subunit of the estrogen receptor enhances integrin α and β1, E-cadherin and β-catenin expression, while suppressing the expression of N-cadherin. It leads to intensified adhesion and impaired migration of cancer cells. ER, estrogen receptor; ERBB2, erb-b2 receptor tyrosine kinase 2; ERBB3, erb-b3 receptor tyrosine kinase 3; EGFR, epidermal growth factor receptor; CXCR4, CXC motif chemokine receptor 4; DPN, diarylpropionitrile.
Anthracyclines can regulate the expression of antigens on the cell membrane surface. Doxorubicin reduces the surface expression of B7-H1 in breast cancer cells to a minimum while upregulating nuclear expression (73). B7-H1 has an anti-apoptotic effect, correlates with tumor invasion and predicts patient survival (74, 75). Therefore, its downregulation and transport of the molecule to the nucleus plays an important role in initiating cancer cell death (73).
Cell death in head and neck squamous cell carcinoma (HNSCC) is mediated by the perforin/granzyme pathway associated with the activity of the membrane pro-apoptotic protein Bcl-2. Tumor resistance correlates with the expression of this protein, which is confirmed experimentally (76). The concentration of the anti-apoptotic protein Bcl-2 was reduced using the combination of cisplatin with 5-fluorouracil (5-FU) indicating the intensification of apoptosis of neoplastic cells (77).
The expression of CEA and MUC-1 increased sharply after treatment with 5-FU (78). CEA is a member of a family of highly related cell surface glycoproteins. It is involved in the adhesion of cancer cells and leads to increased metastasis (79, 80). Upregulation of MUC-2 synthesis is also observed in human colon cancer cells after 5-fluorouracil treatment (81). The use of 5-FU, mitomycin-C or oxaliplatin leads to higher expression of epithelial cell adhesion molecule (EpCAM) and LeY antigen, which is a blood group antigen with a potent expression on the surface of epithelial tumors, including small cell lung cancer (13, 82).
Both betulinic acid (BA) and curcumin inhibit specificity protein (Sp) transcription factors that modulate EGFR expression. The effect reduces the amount of EGFR mRNA produced in bladder cancer cells (83). Downregulation of receptor levels induces cell death through autophagy (84).
Nonsteroidal Anti-Inflammatory Drugs
Nonsteroidal anti-inflammatory drugs (NSAIDs) can modulate the expression of membrane molecules. E-cadherin, a transmembrane protein is responsible for the sequestration of β-catenin to the cell membrane and cell adhesion. Its absence leads to the spread of cancer cells as a result of epithelial-mesenchymal transition (EMT) (85). In colon cancer, sulindac protects against the loss of E-cadherin and the accumulation of nuclear β-catenin (86). In contrast, indomethacin and celecoxib reduce E-cadherin expression. This is associated with the invasion and chemoresistance of non–small cell lung cancer (NSCLC) cells following treatment with celecoxib. Results are not optimistic as to the use of celecoxib and indomethacin in lung cancer (87–89).
NSAIDs reduce the expression of the membrane protein EGFR. This results in the inhibition of cell proliferation (90). Sulindac metabolites – sulindac sulfide (SS) and sulindac sulfone (SF) – also downregulate EGFR activation and/or expression in cancer cells, leading to attenuation of EGFR signaling in colon cancer cells (91). Sulindac and celecoxib are involved in the modulation of the physicochemical properties of the cell membrane, which may be associated with anti-cancer properties (89). Licofelone (a novel dual 5-LOX/COX inhibitor) leads to changes in the proportions of saturated, monounsaturated and polyunsaturated fatty acids and increases the cholesterol content of the cell membrane in HCA-7 colon cancer cells. The compound acts via the inhibition of EGFR kinase activity, p44-42 MAPK and AKT cascades, which results in a transition to the apoptotic cell-death pathway (92). Indomethacin causes increased E-cadherin expression in colon cancer and up-regulation in pancreatic cancer. It suppresses proliferation and intensifies cell adhesion (93, 94). In summary, regular use of nonsteroidal anti-inflammatory drugs (NSAIDs) is associated with a decreased mortality from colorectal cancer (95).
The effects of chemotherapeutics on the expression of membrane proteins are summarized in Figure 2.
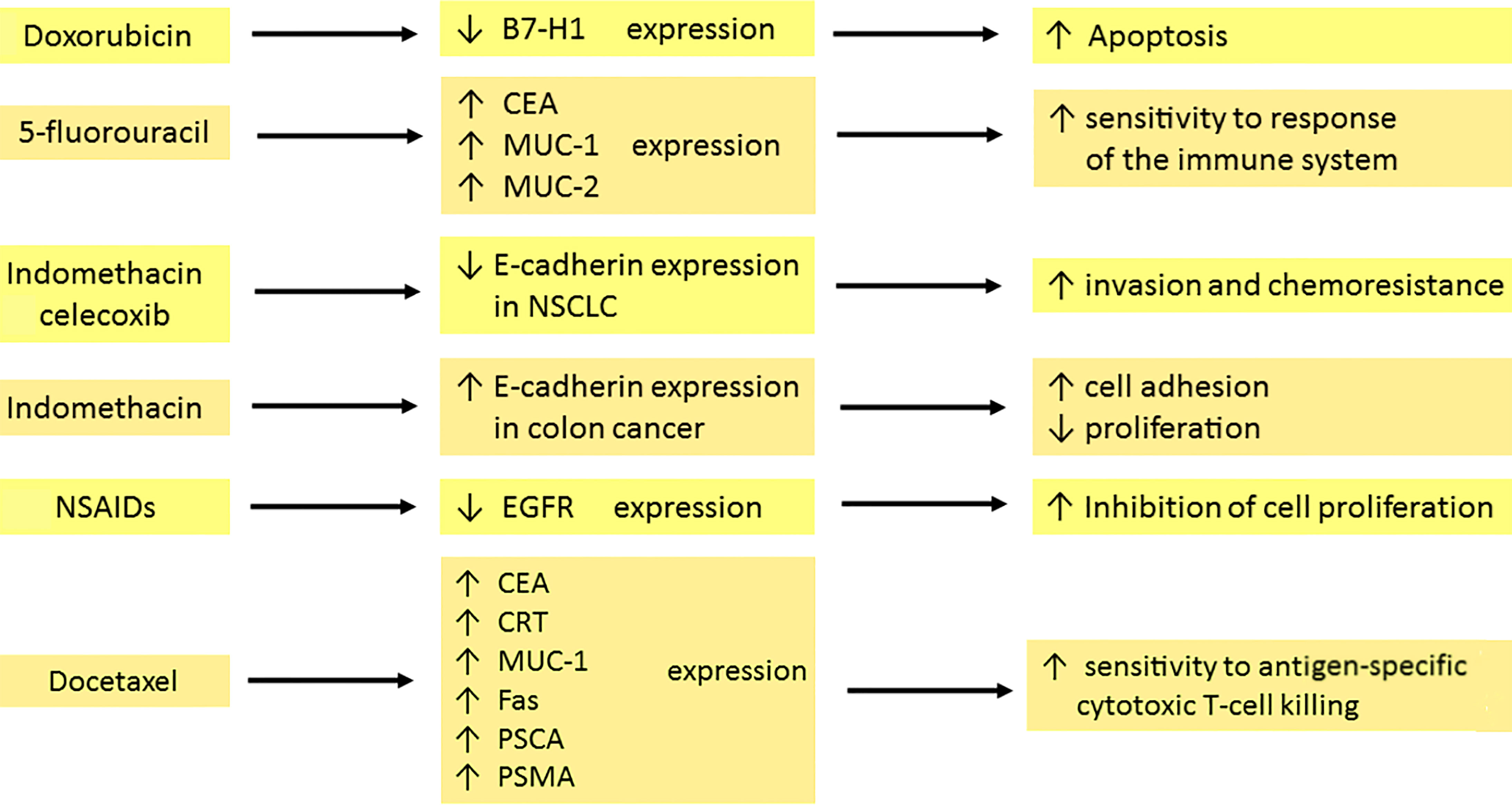
Figure 2 Effects of chemo- and pharmacotherapy of the tumour on the expression of membrane antigens. Doxorubicin, through the inhibition of surface anti-apoptotic B7-H1 expression in breast cancer, is involved in the initiation of cell death. 5–fluorouracil enhances CEA, MUC-1 and MUC-2 expression, which is associated with sensitization of the immune system. Indomethacin and celecoxib decrease the expression of E-cadherin in NSCLC, which leads to amplified invasion and drug resistance development. In contrast, indomethacin in colon cancer increases E-cadherin expression. Therefore, tumour cells have a stronger capacity for adhesion but diminished proliferation. The reduction of EGFR expression by NSAIDs contributes to the inhibition of cell proliferation. Docetaxel upregulates the expression of CEA, CRT, MUC-1, Fas, PSCA and PSMA. The result is a high sensitivity to killing by cytotoxic T cells. carcinoembryonic antigen (CEA), mucin-1 (MUC-1), mucin-2 (MUC-2), non–small cell lung cancer (NSCLC), nonsteroidal anti-inflammatory drugs (NSAIDs), calreticulin (CRT), prostate stem cell antigen (PSCA), prostate-specific membrane antigen (PSMA).
Regulation of Multidrug Resistance Proteins by Pharmacotherapy
Multidrug resistance (MDR) is a serious problem that hampers the effective treatment of cancer. It may also increase mortality among cancer patients. MDR is associated with the insensitivity of cancer cells to several groups of drugs. The members of the superfamily of ABC transporters called ATP-binding cassette transporters pump drugs out of the cell. The best-described efflux protein is P-glycoprotein (P-GP) encoded by the gene ABCB1 (MDR1 – multidrug resistance 1). Multidrug resistance-associated protein 1 (MRP1/ABCC1) or ABCG2 also plays a significant role in multidrug resistance (96). Drugs related to ABC transporters include taxanes (e.g., docetaxel and paclitaxel) and vinca alkaloids (vinblastine and vincristine), anthracyclines (daunorubicin and doxorubicin), topoisomerase inhibitors (etoposide and topotecan), and tyrosine kinase inhibitors (dasatinib and gefitinib) (97).
As an integral membrane protein, P-GP is overexpressed on the surface of cancer cells. This is one of the major barriers to achieving a therapeutic drug concentration. Overcoming this barrier would reduce the doses of the chemotherapeutic drug, the number of side effects and shorten the treatment time. Blockers should act selectively on ABC transporters in the tumor. Their efficiency depends on the expression of the transporters. There are several mechanisms of P-GP inhibition. One of them is competitive inhibition that blocks drug binding. Other mechanisms are connected with noncompetitive inhibitors, disturbing the membrane lipid bilayer and downregulating the expression of P-GP (98). Three generations of P-GP inhibitors that inhibit the efflux of drugs have been distinguished. The first generation is not used in cancer therapy but rather in treating high blood pressure, bacterial, fungal and viral infections or allergies (99). Unfortunately, these drugs have to be taken in high doses, which leads to toxicity. The second-generation drugs are more potent and less toxic but interact with the CYP3A4 enzyme and other ABC transporters (100, 101). The third generation of inhibitors is the most specific for P-GP. These compounds have been examined in clinical studies, but with no spectacular results both in reducing toxicity and effectiveness in increasing the overall survival of the patients (102, 103). Investigators are currently researching the fourth generation which involves natural sources/derivatives, peptidomimetics and dual-activity ligands (104, 105).
The limited success of ABC transporter inhibitors in clinical trials is related to the multitude of elements that contribute to multidrug resistance. The use of modulators in clinical trials leads to a slight improvement in the therapy of patients. Importantly, many serious side effects have been reported, making their safety questionable (99). For this reason, the high toxicity of the generation of P-gp inhibitors encourages the study of natural products that can be used as MDR modulators. Recent studies indicate that natural products, such as vegetables, fruits and their components (especially polyphenols or flavonoids), can be used as MDR modulators (106, 107).
Some anti-cancer drugs can regulate the expression of proteins from the MRP family. Under the influence of doxorubicin, the expression of MRP1 transporter increases in small lung cancer. This drug also leads to MRP2 overexpression, which is not detected before treatment (108). In breast cancer resistance protein/ATP-binding cassette sub-family G member 2 (BCRP/ABCG2), expression is upregulated with an increase in the concentration of mitoxantrone used. This correlates with the degree of drug resistance of cancer cells (109). Reduced response to methotrexate treatment is also associated with BCRP protein expression (110). Vincristine resistance results from increased expression of ABCB1 (MDR1), ABCC1 (MRP1), ABCC2 (MRP2) and ABCC3 (MRP3) genes, which may depend on cell type, exposure time, and drug concentration (111).
P-glycoprotein overexpression can be observed as a result of paclitaxel and docetaxel treatment (112). Interestingly, in the cisplatin-resistant ovarian cancer cell line, the expression of P-GP is increased even though the drug is not a substrate of P-GP. Generalized stress response to long-term cisplatin treatment and reactive oxygen species (ROS) production results in P-GP overexpression that induces paclitaxel resistance (113). Lee et al. found that selective cyclooxygenase inhibitors inhibit MDR1 expression and P-glycoprotein in taxane-resistant ovarian cancer, thus sensitizing cells to paclitaxel (114). The effects of chemotherapeutics on the expression of MDR-related proteins are summarized in Figure 3.
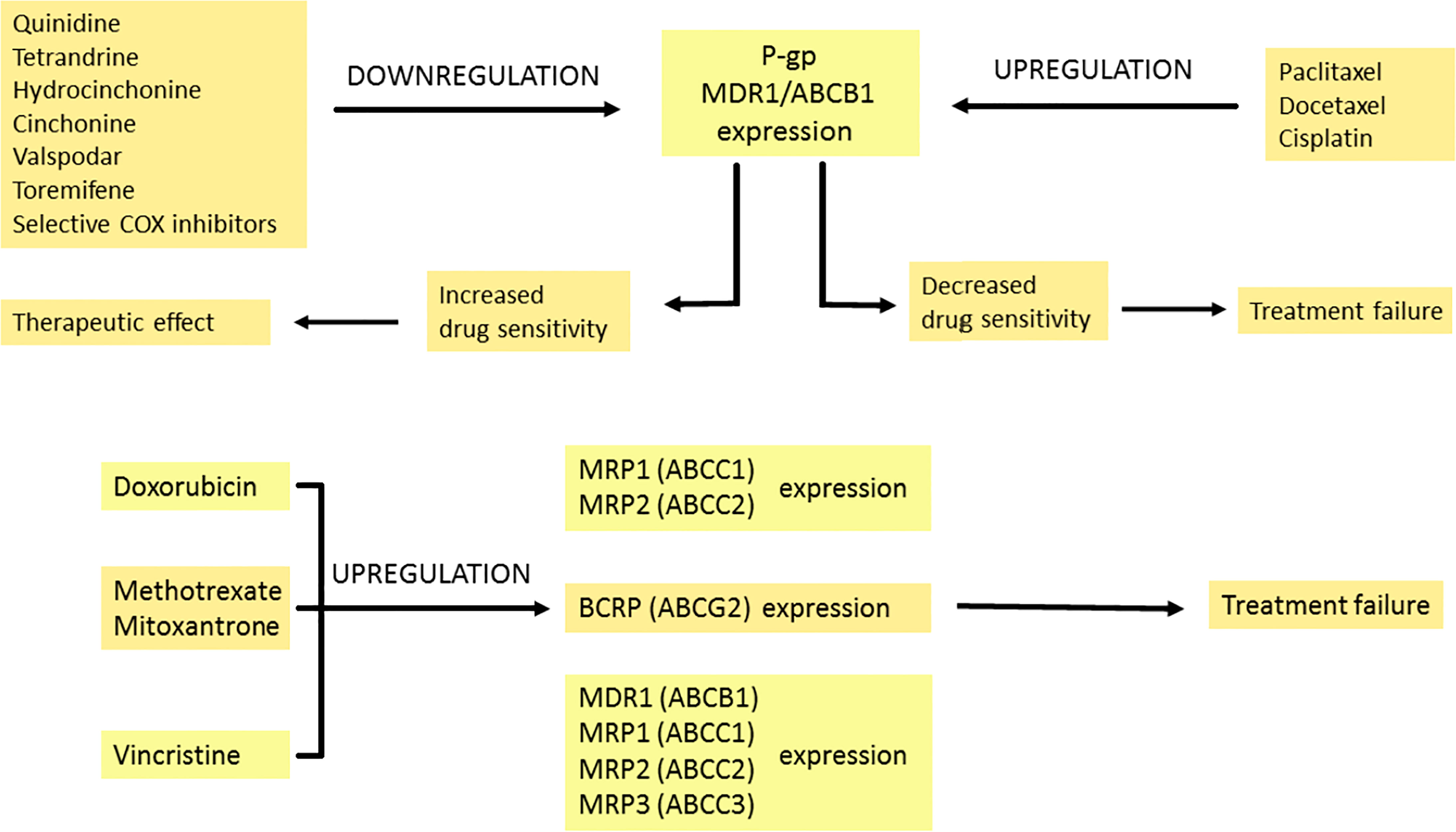
Figure 3 The modulation of multidrug resistance proteins by pharmacotherapy. Expression of P-GP can be increased or decreased by many drugs. Quinidine, tetrandrine, hydrocinchonine, cinchonine, valspodar, toremifene and selective COX inhibitors downregulate P-GP expression, which leads to enhanced transport of the drug into tumour cells and therapeutic effect. In contrast, when the expression of P-GP is downregulated by paclitaxel, docetaxel and cisplatin, it is connected with decreased drug sensitivity and treatment failure. Doxorubicin amplifies MRP1 and MRP2 expression. Methotrexate and mitoxantrone intensify BCRP expression. Vincristine upregulates MDR1, MRP1, MRP2 and MRP3 expression. The modulation effects described above are associated with reduced treatment response. Breast Cancer Resistance Protein (BCRP), P-glycoprotein (P-GP), cyclooxygenase (COX), multidrug resistance 1 (MDR1), multidrug resistance-associated protein 1-3 (MRP1-3).
Physical Methods and Their Mechanisms
Treatment With Electric Field
Treatment of the cells with a pulsed electric field (PEF) leads to various biological effects. These include electroporation – both reversible and irreversible (IRE), electrostimulation and changes in membrane properties (115).
Electroporation is the formation of water pores in biological membranes. Depending on cell integrity after PEF treatment, two types can be distinguished: reversible and irreversible electroporation (115). The electric field has to exceed the electroporation threshold to electroporate the membrane. For most human cell lines, 1300 V/cm PEF leads to high-level cell permeabilization (116). In the case of smaller cells, such as human erythrocytes, the EP threshold is much higher (117). In vitro, plasmalemma electroporation is analyzed by fluorochrome uptake studies. Depending on the size of the fluorochrome, different pore sizes are analyzed. For instance, Yo-Pro-1 may be used to analyze wider pores than fluo-8 aided calcium ion detection (118). During reversible electroporation, the electropore becomes smaller in time after the end of pulse delivery (119). Therefore, cells are permeable even for a short time after PEF treatment. Flow cytometry makes it possible to analyze the time dependence of the electropore annihilation. On the other hand, irreversible electroporation includes instant induction of necrosis after PEF treatment (115). The reversibility of the process might be analyzed by the long-term assessment of cell permeability. For instance, a previous work by the present authors showed the potency of trypan blue kinetic staining studies in the differentiation between reversible and irreversible electroporation protocols (115).
Treatment with electric field may be used to modulate membrane antigens in two distinct attempts. The first includes the electroporation of the cells with the plasmid encoding a specific molecule (for instance, a cytokine such as IL-12), followed by cytokine expression and release (120–122). In this case, electroporation does not directly affect the membrane, but via the stimulation with an exogenous molecule, it modulates the systemic response to cancer antigens and sensitivity of the immune cells to the paracrine signal (123). Similarly, Pen et al. showed that electroporation with mRNA encoding constitutively active TLR4 may be used to activate dendritic cells (124). Besides, the immune response of the cell might also be modulated by electroporation with siRNA. Dannull et al. showed that it is possible to modify the antigen processing system in dendritic cells by electroporation with immunoproteasome-targeting siRNA (125). DNA encoding a variety of other molecules may also be electroporated to induce specific modifications to the immune response. For instance, when Tanning et al. electroporated HIV-1 antigen with the ectodomain of PD-L1, anti-HIV-1 response was enhanced in comparison to control samples (126). There is an entirely new concept in which electroporation supports the action of DNA vaccines via an additional stimulation of the immune system (127). All the abovementioned effects arise from the changes in the composition of membrane antigens.
Interestingly, aside from the cellular effects of PEF treatment, irreversible electroporation is involved in the increased concentration of cancer-specific molecules in the tumor microenvironment. This allows the immune cells infiltrating the tumor to process cancer molecules and extensively work as antigen-presenting cells, therefore increasing the immune recognition of the tumor. The method is currently widely examined due to its simplicity and possible use as an adjuvant cancer therapy. The mechanism of IRE is slightly different from reversible electroporation. Here, the release of cancer-specific molecules to the tumor microenvironment induces the immune response against cancer. In this case, the immunophenotype of immune cells changes to one set to kill the alien cells (128). Hester et al. proved that IRE may transiently alleviate the immune suppression and enables the activation of T-cells in the tumor site (129). Curiously enough, Zihao Dai et al. proved that IRE of post-ablation hepatocellular carcinoma induces CD8+ cell response against cancer via the release of danger-associated molecular patterns (DAMPs) and the downregulation of Treg and PD-1+ cells in the tumor tissue (130). However, the release of damage-associated molecular patterns to the extracellular compartment activates cytotoxic T-cells, thus modifying their cell membrane antigens toward the cytotoxic phenotype of the membrane. There are several types of DAMPs, including histones, genomic DNA, HMGB1, IL1a, IL33, ATP, F-actin, Cyclophilin A, HSP, uric acid, mitochondrial DNA and calreticulin (131). Molecules originate from different compartments of the cell, like nucleus, cytosol or mitochondria (132). These flow out from the cells after cell damage to the extracellular space. Afterward, mentioned DAMPs are recognized mostly by Toll-like receptors (TLR) as well as RAGE, TIM3, P2Y2, P2X7, DNGF1, CD147, CD91, SERC1, FEEL1 and NLRP3 molecules (131). Interestingly, Yimingjiang et al. claim that the effects of nsPEF are similar to PD-1 blockade in the treatment of hepatocellular carcinoma (133).
When EP is combined with the administration of chemotherapeutics, the process is called electrochemotherapy (ECT) (134). The therapeutic agent might be administered intravenously or locally to the tumor site. Some of the most commonly used chemotherapeutics include bleomycin for melanoma treatment or calcium chloride for clinical trials in pancreatic cancer management (135, 136). The effects of ECT on the modulation of membrane antigens in the anticancer response are similar to those achieved in standalone electrochemotherapy. Gerlini et al. proved the activation of dendritic cells following electrochemotherapy of melanoma (137). However, the difference between ECT and EP in terms of modulation of membrane antigens is the higher cytotoxicity induced by ECT related to the administration of the chemotherapeutic agent (118). The cytotoxicity may also affect the composition of membrane antigens.
Intracellular effects of PEF treatment include the permeabilization of intracellular organelles, stimulation of the biosynthesis of specific proteins and modulation of antigens. The first effect was widely described in the Jurkat cell line, in which the nanosecond pulses induced the permeabilization of the mitochondrial outer membrane and, therefore, the release of cytochrome c and reactive oxygen species to the cytoplasm (138). After the disruption of cell membrane integrity, the cell increases the biosynthesis of the components of its cytoskeleton (139). However, in short-term response, Kiełbik et al. proved that reorganization of actin fibers occurs after electroporation (118). The effect might be a response of the cell to unfavorable conditions. Besides, nanosecond pulsed electric field may also affect the formation of enhanced speckle (140). A similar effect relates to the permeabilization of the endoplasmic reticulum. Conversely, protein biosynthesis requires high amounts of energy, thus the PEF stress-induced inhibition of translation serves as a mechanism for cell survival (141). Moreover, several biological effects also arise from the application of electric fields, for instance, eIF2α phosphorylation and 4E-BP1 dephosphorylation (142). Moreover, other studies suggest that nsPEFs activate the major MAPK kinase downstream signaling pathways via p38, JNK and ERK (143, 144). From the mechanistic point of view, however, the mechanisms through which nsPEF modulates the activity of membrane kinases remain unknown. Some authors hypothesize that the effect occurs via the induction of cell stress and thus the activation of kinases. Also, studies by Vernier et al. prove that the biological changes may arise from the increased concentration of cytoplasmic calcium (145). A similar tendency was observed by Morotomi-Yano et al. who proved the activation of AMPK with the increased content of cytoplasmic Ca2+ (146). The study introduces an entirely new mechanism in which the stimulation with nsPEFs may modulate the immunophenotype of the cells. AMPK is one of the critical regulators of vesicle trafficking, which is why its inhibition disallows the proper membrane-vesicle interaction and thus in the long term affects the composition of membrane antigens (147). Moreover, nsPEFs may regulate the phenotype of the membrane and presumably the content of antigens in chondrocytes via the Wnt/β-catenin pathway (148). Interestingly, an example of direct modulation of membrane antigen by nsPEF is the CD95 death receptor which could be modulated in Jurkat and U937 cell lines (149). Significant effects and high expectations based on the aforementioned studies prove that much research has yet to be done in this field.
nsPEF also exerts an effect on protein antigens and changes membrane lipid composition via the interaction with scramblase (150), an enzyme responsible for the transport of negatively charged phospholipids between the inner and outer leaflet of the membrane. As a consequence, the lipid profile of the plasmalemma alters.
All the mentioned mechanisms in which the electric field modulates the immunophenotype of the cells are summarized in Figure 4.
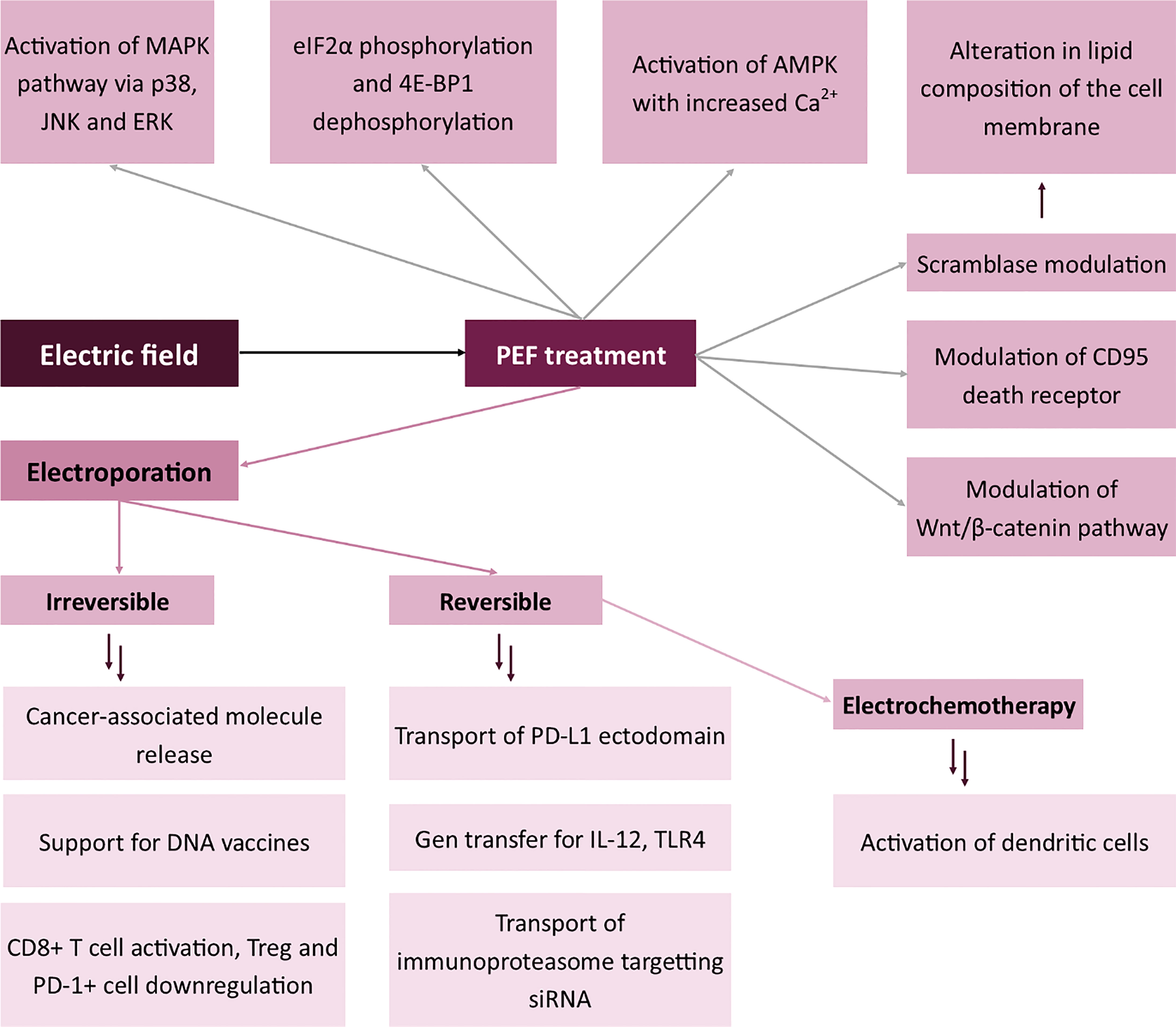
Figure 4 Effects of electric field on the expression of membrane antigens. Mitogen-activated protein kinase (MAPK), c-Jun N-terminal kinase (JNK), Extracellular signal-regulated kinase (ERK), Eukaryotic Translation Initiation Factor 2A (eIF2α), Eukaryotic translation initiation factor 4E-binding protein 1 (4E-BP1), 5’ AMP-activated protein kinase (AMPK), Programmed death-ligand 1 (PD-L1), Toll-like receptor 4 (TLR-4).
Photodynamic Reaction
Photodynamic reaction (PDT/PDR) includes the irradiation of the photosensitizer with an adequate wavelength light. The physical mechanism of PDR includes the shift of electrons to the high-energy state forwarded by the emission of excess energy to biomolecules (151). The process is responsible for the irreversible oxidation of intracellular components and even redox changes in the DNA. Without proper genome editing and cell repair system, cancer cells die from the accumulation of invalid proteins and genetic material (152). In the case of the cellular changes induced by the photodynamic reaction, various membrane proteins undergo alternations. For instance, actin bound to the membrane by zyxin was proved to change its structure dramatically after the irradiation of melanoma cells with curcumin (153, 154). Moreover, PDT/PDR induces the stress response of the irradiated cells and therefore changes in the cytoskeleton which prevents the cells from physical disruption of their integrity (153).
In PDT, an inactive anticancer agent is administered systemically or locally. Even in systemic administration, PDT is a highly selective treatment as the local light irradiation goes in parallel with the accumulation of photosensitizer within a tumor. Still, if the local administration of the photosensitizer to the tumor site is possible, it decreases the systemic side effects (151).
PDT influences the immunostimulatory attributes of antigen-presenting cells, thus alleviating autoimmune diseases (155). Mroz et al. described the effects of PDT on cancer cells and the immune system in vivo (156). The authors demonstrated that PDT induces the increased killing of cancer and the production of TNFα and IFNγ in the tumor tissue. Moreover, PDT elicited the development of epitope-specific CD8+ cells followed by the destruction of distant untreated antigen-positive tumors. The much lower CTLs-related effects on antigen-negative cells prove that PDT induces changes in immune cell membranes thus allowing for the higher cytotoxicity toward the tumor. High selectivity and specificity of PDT toward the antigen was demonstrated also by other authors, for example, Kabingu et al. The group showed that PDT enhances the systemic recognition of Hip1, a tumor antigen associated with basal cell carcinoma (157). In another study, Mroz showed that PDT induces a potent epitope-specific response against testis antigen P1A and simultaneously toward cancer (158). Reginato et al. presented a method of combining Treg depletion by cyclophosphamide with PDT to potentiate PDT-mediated immunity (159). Wang et al. also presented an interesting attempt to enhance the immunomodulatory effects of PDT. The authors engineered an antigen-photosensitizer nanocarrier to facilitate ROS-triggered immune response to PDT (160). Remarkably, the antitumor effect of antigen-specific immunization may be enhanced by the use of antigen-specific conjugates – as demonstrated by Wang et al. in their successful attempts of targeting PSMA on prostate cancer cells (161).
Concerning the mechanism of PDT-aided immunity and therefore the activation of immune cell cytotoxicity (also membrane cytotoxicity-related antigen expression), Korbelik suggested that the vigorous innate immune reaction arises from the intensified phagocytosis of dead tumor cells (162). Zhang et al. provided evidence that PDT also induces presentation of surface MHC I related antigens and enhances antigen processing through the restoration of TAP1 protein expression in glioma cells (163). TAP1 can potentiate the transport of new antigen peptides and therefore is involved in PDT-related immunomodulation (163). The generation of ROS plays a significant role in PDT-induced immunity (160). Interestingly, some photosensitizers, such as protoporphyrin IX, may be selectively accumulated only in activated lymphocytes. The same authors proved the functional alternations in antigen-specific and nonspecific immune components (164).
Aside from cancer-related changes in the cell membrane, PDT may also be used to induce the immunosuppression of contact hypersensitivity (165). Light radiation was proved to induce genomic changes, leading to the induction of POMC expression (166). When keratinocytes are irradiated with UVB light, POMC is extensively biosynthesized via a p53-related pathway and converted to αMSH or ACTH afterward (167, 168). Therefore, aside from the local effects, the light might induce systemic effects – such as the increased biosynthesis of corticosteroids (169).
The mechanisms in which light affects the immunophenotype of the cells are summarized in Figure 5.
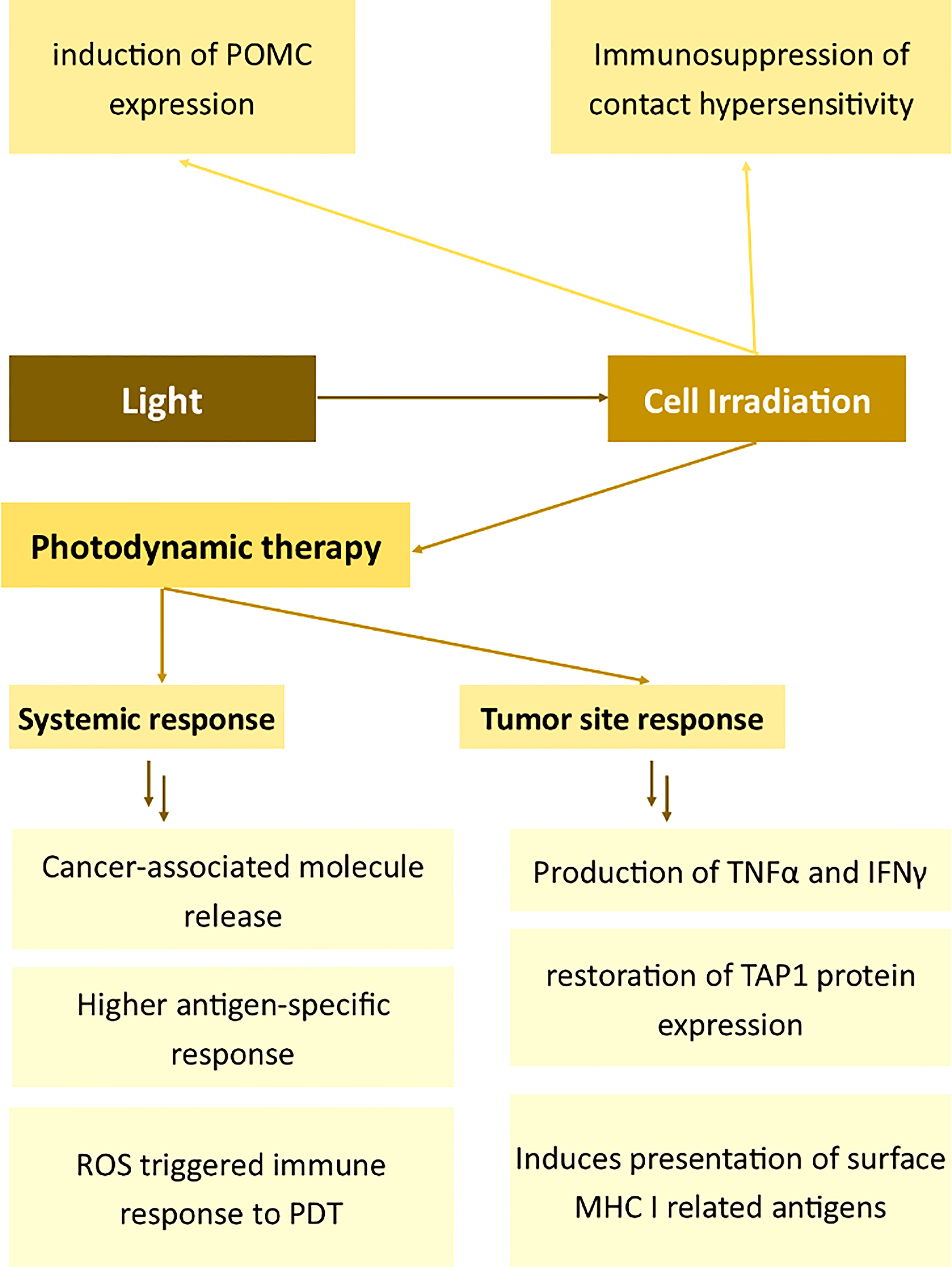
Figure 5 Effects of light on the expression of membrane antigens. Proopiomelanocortin (POMC), Tumour Necrosis Factor α (TNFα), Interferon γ (IFNγ), Transporter associated with antigen processing 1 (TAP1), Major Histocompatibility Complex I (MHC I), Photodynamic therapy (PDT).
Radiation Therapy
Radiation therapy involves the application of high-energy waves (like X-ray or γ-waves) or particles (like electrons, α particles, β particles or protons) against cancer. Early clinical attempts showed the advantages of X-rays and alpha particles over the beta particles, thus more research studies concerned the more effective techniques.
X-Ray Radiation
X-ray radiation rises from the emission from the atomic nuclei. Roentgen radiation involves the emission of X-ray waves (wavelengths ranging from 0.01 to 10 nm) and the administration of the radiation dose to the tissue (170, 171). There is no evidence that X-ray induces the poration of the membrane – instead, it exerts effects on the genetic material of the cell and the redox potential in the cytoplasm (172, 173).
In non-cancerous cells, the genetic material repair system is valid and radiation effects are reversible (174–176). The only side effects of X-ray treatment include skin burns and the induction of neoplasms in susceptible patients (177, 178). Conversely, in cancer cells, DNA-repair systems are often impaired, therefore X-ray treatment induces irreversible damage to the genetic material (179, 180). Neoplasms develop and the abnormal cells accumulate in the irradiated site. The changes in the genetic material induce the biosynthesis of redox-related proteins as well as free radical scavengers (181). The composition of surface antigens is also changing. Irreparable changes occur in the cell, and the neoplasm induces the expression of death-related molecules (182).
Studies on a murine model demonstrated that the immune response following irradiation relates to the locus of the antigen affinity (183). Namely, antigens associated with the H-2 locus were much more resistant to irradiation than those related to H-1 and H-3 loci. The author shows the modulatory role of X-ray on membrane antigens in relation to transplantation immunity (183). Other papers also prove decreased rejection rates (ratio of unsuccessful transplantations) in patients after Total Body Irradiation (TBI) (184). The mechanism of the effect of X-rays on cell immunophenotype may also be related to changes in proliferating cell nuclear antigen (PCNA) and thus the proliferation phenotype in V79 hamster fibroblasts (185). Also, Miura et al. showed that PCNA is involved in the repair mechanisms after X-ray radiation and thus in the proliferation properties of human fibroblasts (186). Since cells change membrane antigens as they proliferate, all the mentioned processes have to relate to changes in the composition of membrane antigens. On the other hand, Shreder et al. described the lack of the effects of X-rays on transcription factors such as PPARγ, C/EBPα and C/EBPβ in human pre-adipocytes (187). However, the authors claim the modulation of cells by inflammatory mediators rather than genomic factors (187). Interestingly, studies on osteoblasts demonstrated that X-ray irradiation induces the differentiation of cells via the RhoA pathway and the change in the cytoskeleton (188).
X-ray radiation also had a direct effect on cancer-related molecules on the cell membrane of human gastric cancer cells causing an increase in CEA membrane content and elevating the level of MHC I (189). Wittenborn et al. showed an autoimmune phenotype achieved in mixed chimaera models after ionizing X-ray radiation and Cesium-137 treatment (190). Finally, Tandl et al. show that X-ray elicited calcium ion signaling cascade and, in consequence, activated human T-lymphocytes (191).
The mechanisms in which X-rays modulate membrane antigens of cells are summarized in Figure 6.
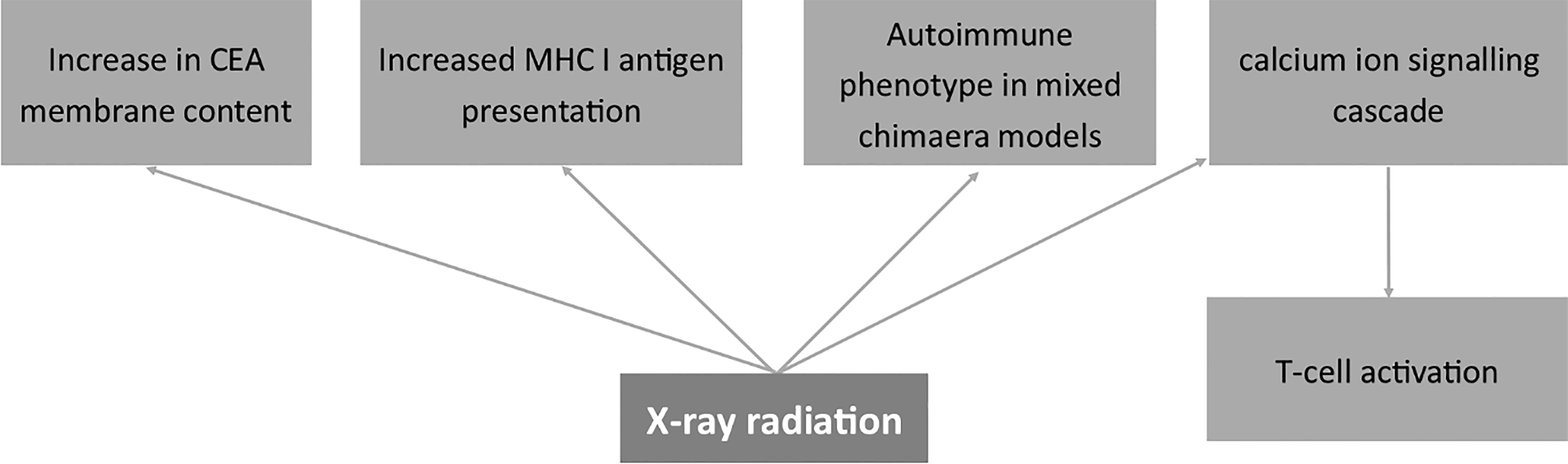
Figure 6 Effects of X-ray radiation on the expression of membrane antigens. Carcinoembryonic antigen (CEA), Major Histocompatibility Complex I (MHC I).
Gamma Radiation
Gamma radiation may be generated with isotope-based generators, like Cobalt 60 (192). γ radiation exerts dualistic effects on human cells, depending on the dose of the radiation and the expression of REG4, BIRC5 and NEIL2 genes (193). Long term low doses of γ waves exposure induces carcinogenesis in the healthy organism. Daniels et al. showed that the protracted exposure to low-dose gamma radiation is significantly associated with leukemia (194). Conversely, annual outdoor ambient gamma dose rate is not associated with childhood leukemia in German population nor increased the risk in United Kingdom children (195, 196). However, when the dose significantly increases and is administered to the tumor site, it induces apoptosis in cancer cells and is being used in the anticancer therapy. Interestingly, both effects are observed during the therapy and not only cancer is affected by the radiation, but the non-cancerous cells as well (192). Cancer cells gain susceptibility to the γ radiation therapy, when it is combined with other modalities of treatment. For instance, studies by Hofmanova et al. proved the increased susceptibility to γ radiation when the cells were simultaneously treated with lipoxygenase inhibitors (197). Besides, curcumin enhances the cytotoxic effect of radiation in MCF-7 cells (198). According to the molecular changes induced by gamma radiation, CuZnSOD and MnSOD proteins expression increases in the time-dependent manner (199).
Nuclear Medicine
Like X-ray radiation, nuclear medicine involves the irradiation of cancer cells, however, in this case with α particles instead of X-ray waves (200, 201). In the past, β radiation was used for studies on therapeutic applications (200). In nuclear medicine therapy, a radioactive isotope emits radiation, driving cancer cells to apoptosis and causing damage to the genetic material of the tumor.
Depending on the stage of tumor differentiation, the isotope may be administered locally or systemically. In well-differentiated cancers, such as hyperthyroidism and differentiated thyroid cancer, the most commonly applied isotope – I-131 – is captured nearly fully in the thyroid cells (202). Radioisotope might be conjugated with a molecule specifically captured by the organ. For instance, adrenal cortex cells uptake 131I-6-β-iodomethyl-19-norcholesterol or 6-methyl-75Se-methyl-19-norcholesterol which is used in the diagnostic scintigraphy of the adrenal cortex (203). The method allows for the synthesis of more specific therapeutics and decreases the number of systemic side effects of the therapy. Moreover, highly effective iodine conjugates, such as I-Metaiodobenzylguanidine (131I-MIBG) are applied in targeted radiotherapy for children with neuroblastoma (204). Aside from tumors, radioiodine might be used for the therapy of Graves’ Disease (205).
Hyperthermia and Hypothermia
Hyperthermia is a state of the body where the temperature is elevated to induce a specific response. In pathology, it occurs systemically in fever (206–208). However, studies by Muckle et al. prove that hyperthermia may also be artificially induced as part of anticancer therapy (209). Several methods may be used to elevate the body’s temperature – electric field, light radiation and heat convection. When the temperature remains exceptionally high, the cancer tissue undergoes necrotic death and immunization is similar to that obtained in IRE or other ablation techniques (210–214). The parameters of the applied heat suppliers have to be carefully set (215). Depending on the applied temperature, heating methods may be divided into lethal and sub-lethal hyperthermia. Both processes drastically differ in biological response of the cancer cells. Sublethal temperatures induce the epithelial to mesenchymal transition in breast cancer cells, simultaneously increasing the chemosensitivity of the cells (216). Henle et al. proved that sub-lethal radiation or heat damage may become lethal when treated with 40oC after the damage or may be repaired when treated with lower temperatures (217). The tendency that sub-lethal hyperthermia increases the cytotoxic effect of radiation damage was also proved by the other authors (218). The control of quiescent cells by sublethal hyperthermia is useful for suppressing the repair of both potentially lethal and sublethal damage (219). Like in IRE, lethal hyperthermia-aided ablation induces systemic response to tumor antigens and may be potentially used in the treatment of metastatic disease (220). The effects of hyperthermia may be enhanced by dendritic cell immunotherapy following hyperthermia (221). Moreover, the release of DAMPs to the extracellular space induces an enhanced cytotoxic phenotype of immune cells and an increased infiltration of the tumor microenvironment (222). Moreover, photothermal ablation modulates the intratumoral myeloid population toward tumor immunogenicity (223). On the other hand, multiple exposures to radiofrequency radiation hyperthermia suppressed cell-mediated immunocompetence (224). The immune system response is not evoked exclusively by extremely high temperatures. Also, studies by Umar et al. proved that febrile temperature induces CD4 T-cell differentiation regulated by the TRPV channel and the Notch signaling pathway (225). Hyperthermia also increases the presentation of HLA-DR in NK and NKT cells (226). The mechanism of action of hyperthermia also relies on Heat Shock Proteins (HSP) and thus indirectly on MHC I (227). Extracellular HSP70 serves as a link between NK and dendritic cells via the induction of NKG2D ligand and MHC class I chain-related protein A overexpression and the augmentation of IFN-γ release (228).
On the other hand, hypothermia is known to inhibit the classical complement pathway. Besides, it is associated with decreased expression of pro- and anti-inflammatory effectors (229).
All the abovementioned mechanisms in which temperature affects the membrane antigens are summarized in Figure 7.
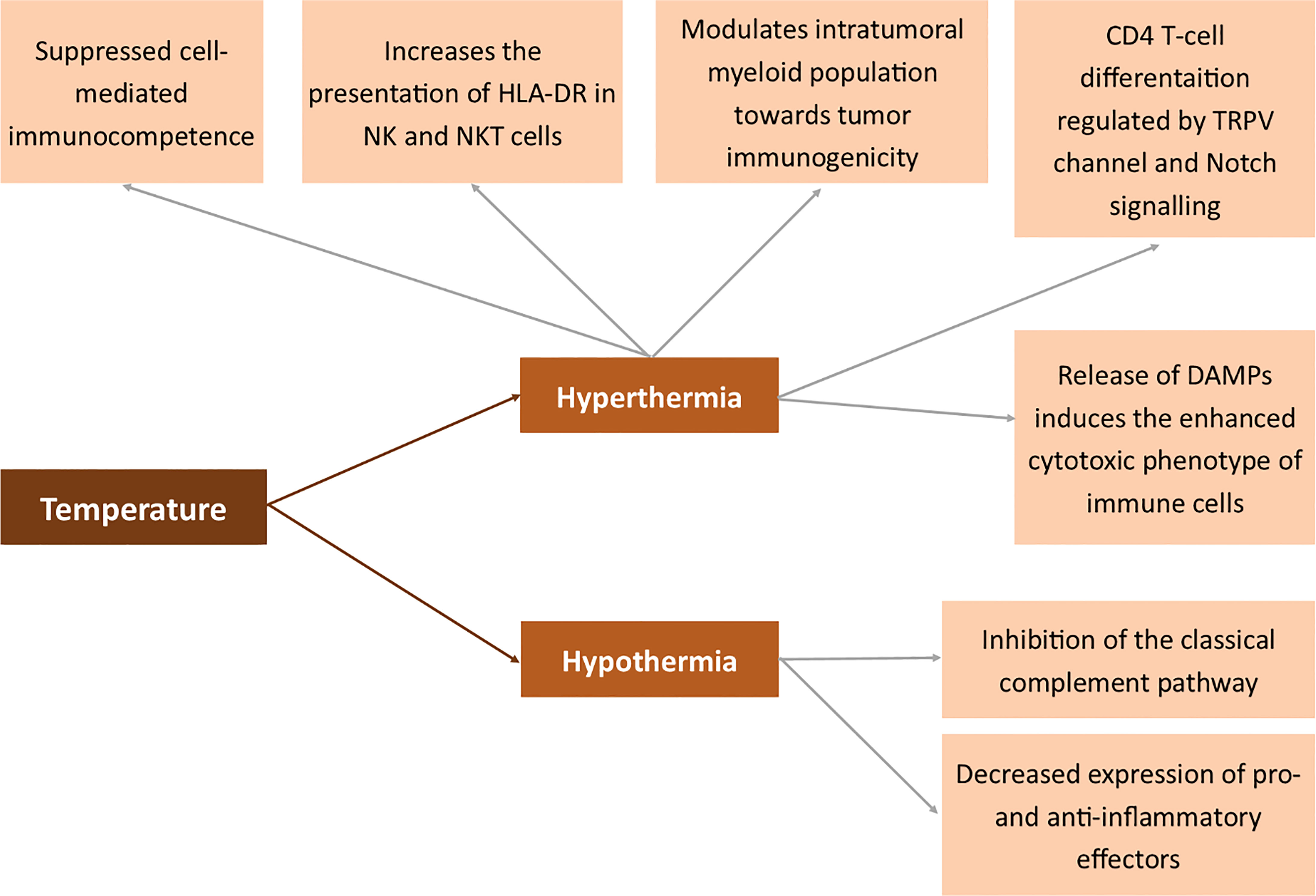
Figure 7 Effects of temperature on the expression of membrane antigens. Human leukocyte antigen DR (HLA-DR), transient receptor potential cation channel (TRPV), Damage-associated molecular pattern (DAMP).
Magnetic Field
Treatment of cells with magnetic field (MF) is not fully covered in the literature yet. There are three types of MF – alternating (AMF), pulsating (PMF) or static magnetic field (SMF). In the case of PMF, the effects are nearly identical to the ones in the electroporation technique (230). On the other hand, AMF and static MF have not been analyzed in anticancer therapy yet. Only a few papers show the potential of magnetic field in the induction of cell death (231, 232).
Several authors combined MF treatment with other anticancer modalities, such as electroporation, and obtained favorable results in permeabilizing cells (233). Besides, specific MF-dependent nanovesicles are synthesized to enhance the anticancer effect of the therapy severely targeting melanoma (234). Aside from the effects on ferromagnetic metal-containing enzymes, MF also induces local hyperthermia and results in the ablation of the tissue (231). All the cytotoxic effects relate to the change of membrane antigen composition to the one connected with cell death.
Ultrasound Treatment
Sonodynamic reaction (SDR) takes place when ultrasounds act on the cells in a medium containing ultrasound-activated compounds – sonosensitizers (235, 236). The effects of ultrasounds on human tissues are much more complex than in PDR. Ultrasounds act both on the sonosensitizer (in SDR) and systemically (237, 238). When the sono-active compound gets to the cell, ultrasound treatment induces implosion and sonoluminescent light emission (239–241). Moreover, the collection of diffused gases leads to forming a high-volume gas bubble in a process called cavitation (242).
Aside from specific antigen response, the cell prevents itself from future disruption, and therefore the increased biosynthesis and organization of the cytoskeleton takes place. Mechanisms in which ultrasounds modulate membrane antigens may relate to a ROS burst after SDR or the activation of autophagy (243, 244). Li et al. proved that autophagy in the case of SDR may relate to the translocation of TFEB induced by a ROS burst following SDR (245). Also, hypoxia may relate to the modulatory effects of SDR (246). Several attempts were made to stimulate immunity after SDR. One of them was the generation of a two-dimensional coordination nanosheet which additionally activated the immune response via the utilization of the TLR9 agonist in its structure (247).
Boiling histotripsy of the tumor tissue via the application of ultrasounds is similar to the effects of IRE (248). The mechanism is similar and the methods seem complimentary and both modulate the immunophenotype of CD8+ cells to a phenotype which is more cytotoxic toward cancer cells. Also, high-intensity focused ultrasound therapy seems to modulate the activation of CD8+ and CD4+ cells infiltrating the tumor tissue (249). Moreover, HIFU induced the increased expression of Nlrp3, Jun, Mefv, Il6 and Il1β and alterations in macrophage polarization in studies by Fite et al. The authors also described the upregulation of several immune pathways involving Nod1, Nlrp3, Aim2, Ctsb, Tlr1/2/4/7/8/9, Oas2, and RhoA (250). Studies by Ji et al. showed a shift from polarized M2 macrophages into M1 phenotype and depletion of myeloid-derived suppressors in the tumor microenvironment following the application of ultrasounds (251). In the case of immune cells, nitric oxide (NO) is responsible for the shift in the functioning of immune cells (251).
At the other end of the spectrum of ultrasound use is sonoporation (252). The process involves the formation of a pore in the cell membrane with the use of ultrasounds. Mechanisms involved in sonoporation include cavitation, shear stress to the membrane, endocytosis and finally mechanical stress to the membrane (253). The method seems very promising; however, due to its novelty, only several studies concern the application of ultrasounds in the modulation of membrane antigens. The first attempt includes the transport of antigen mRNA to dendritic cells, which afterward, via the expression of antigenic peptide, can act as DC vaccines against tumor cells in vivo (254). Research also concerned the transport of siRNA molecules to the primary T cells which resulted in a decreased methylation-controlled J protein expression (255). The Golgi-associated transmembrane protein downregulation is involved in increased resistance to specific drugs by inducing expression of the ABCB1 drug transporter via the c-Jun-related pathway. Therefore, sonoporation may be used to modulate drug-resistant proteins (256).
All the mentioned mechanisms in which ultrasounds modulate the membrane antigens of the cells are summarized in Figure 8.
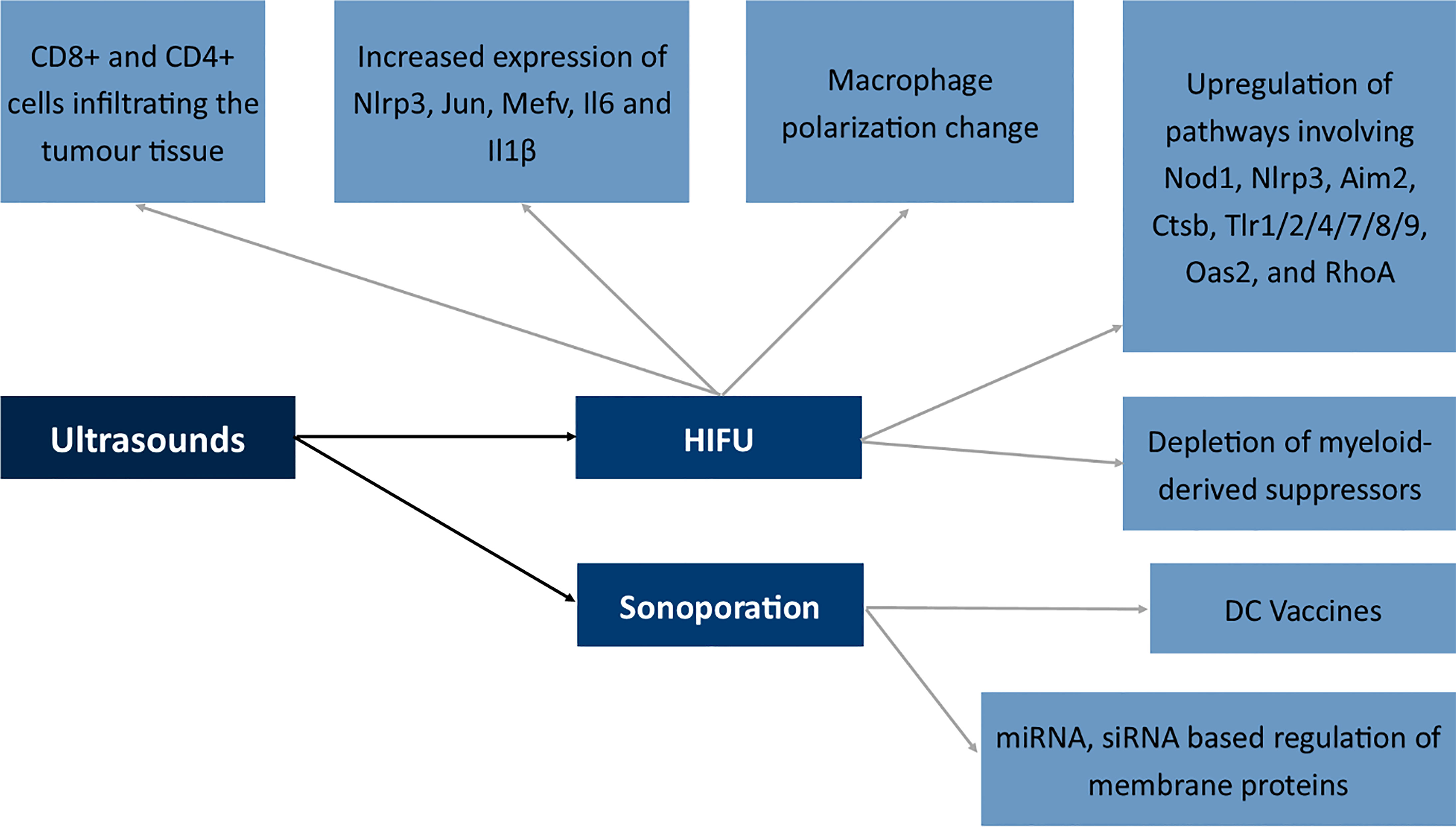
Figure 8 Effects of ultrasounds on the expression of membrane antigens. NLR Family Pyrin Domain Containing 3 (Nlrp3), Jun Proto-Oncogene (Jun), Mediterranean fever gene for marenostrin (MEFV), Dendritic cell (DC), Nucleotide-binding oligomerization domain-containing protein 1 (Nod1), Absent In Melanoma 2 (Aim2), Cathepsin B (Ctsb), Toll-like receptor 1/2/4/7/8/9 (Tlr1/2/4/7/8/9), 2’-5’-Oligoadenylate Synthetase 2 (Oas2), Ras homolog family member A (RhoA), High-intensity focused ultrasound (HIFU).
Modulation of Membrane Antigen in Clinical Practice
Modulation of membrane antigens has found a variety of clinical applications. Affected membrane proteins and receptors might be used in the production of lymphocytes used in cell-based therapies or sensitization of the tumor toward chemotherapy. In general, the modulatory effect is, in most cases, the side effect of the used therapy. Sometimes the result is profitable – for instance after PDT or PEF treatment and in some cases, the effect is negative and leads to the increase in aggressiveness of cancer – like sometimes after hormonal therapy.
CAR-T or CAR-NK cells therapies target cancer-specific antigens with the use of T- or NK-cells with a newly introduced CAR receptor. In the process of CAR design, the new receptor has to be specific to the targeted cells and not self-destroy the CAR-T/NK cells. The therapeutic cells easily find their application in leukaemia or in the treatment of other haematological malignancies. For instance, in B-ALL, cytotoxic cells often target CD20 or CD19 antigens (12). Most T-ALL CAR target the CD7 molecule which is also present on CAR-T cells (257). Therefore, to avoid fratricide during the production of anti-CD7 CAR-T cells, self-reactive CD7 molecules are depleted by gene silencing methods or deleted with the CRISPR-Cas system. A similar approach may be implemented in the production of anti-CD5 or anti-CD2 CAR-T cells. Cell-based immunotherapy presents several limitations in terms of antigen expression. First, the efficacy of immunotherapy depends on the number of targeted receptors. Second, the therapy has to be personalized – the cells should be compatible with the HLA system of the patient, and thus most of CAR-T cells are produced from patients’ own T-cells. The third problem is the low efficacy of targeting solid tumors. There are a few factors that contribute to it – a low abundance of solid-tumor specific antigens and low infiltration of CAR-T cells to the tumor site. To overcome the problems, researchers investigated the methods for increasing cancer-specific molecules and their immunogenicity. The attempt was applied in clinical practice, and several clinical trials involved the modulation of membrane antigens via the application of pharmacotherapy and biological therapeutics. Table 1 summarizes completed clinical trials in the modulation of membrane antigens.
Sensitization of the tumor to chemotherapy is mostly connected with mitosis inhibition or induction of additional mutations in the tumor site using radiotherapy or irradiation. In this case, the tumor is less aggressive and viable, therefore the effects of additional chemotherapy are achieved more easily and fewer tumors are resistant to the therapy. However, the modulation of membrane antigens as a supportive method before chemo- or immunotherapy remains a novel idea. The first attempts at the therapy came with the use of photodynamic therapy as an adjuvant method for cancer treatment. The tumor site is irradiated with a photosensitizer after the excision of the vast majority of the tumor. The radiation involves increased infiltration of the tumor site by immune cells and thus prevents the rebuilding of the tumor from the local metastatic cells. The process is related to the increased abundance of DAMPs in the tumor site and the effect of PDT on tumor-associated immune cells. Similarly, treatment with a pulsed electric field in the irreversible electroporation protocol is associated with an increased release of DAMPs to the tumor microenvironment and the sensitization of APCs to tumor-associated molecules. However, in this case, PEF treatment also directly affects the T-cells, making them more cytotoxic toward the tumor. Table 2 presents the currently ongoing clinical trials in this area.
Summary
Modulation of membrane antigens is a promising attempt in anticancer therapy. Modern oncology should not only focus on the induction of cell death in tumor cells but also aim to sensitize the body toward cancer cells and prepare the targeted tumor for anticancer therapy to increase its efficiency. The various methods that could be used to modify tumor antigens include pharmacotherapy or treatment with ultrasounds, X-rays, electric and magnetic field. Not only changes in the tumor membrane may be beneficial in the therapy – also alternations in immune system cells may result in a better therapeutic outcome.
Author Contributions
WS, NJ, NS, and OM contributed to conception and design of the study. BN organized the database. WS, NJ, and NS wrote the first draft of the manuscript. All authors contributed to manuscript revision, read, and approved the submitted version.
Funding
The authors would like to acknowledge the funding by the Polish Ministry of Education and Science under project number SKN/SP/496717/2021 and partial funding by the Department of Molecular and Cellular Biology under project No SUB.D260. 22.016 and the National Science Centre (Poland) project No. 2017/27/N/NZ3/01110 (to OM). The publication was prepared under the project financed from the funds granted by the Ministry of Education and Science under the “Regional Initiative of Excellence” programme for the years 2019-2022, project number 016/RID/2018/19, the amount of funding PLN 9,354,023.74.
Conflict of Interest
The authors declare that the research was conducted in the absence of any commercial or financial relationships that could be construed as a potential conflict of interest.
Publisher’s Note
All claims expressed in this article are solely those of the authors and do not necessarily represent those of their affiliated organizations, or those of the publisher, the editors and the reviewers. Any product that may be evaluated in this article, or claim that may be made by its manufacturer, is not guaranteed or endorsed by the publisher.
References
1. Hodge JW, Garnett CT, Farsaci B, Palena C, Tsang KY, Ferrone S, et al. Chemotherapy-Induced Immunogenic Modulation of Tumor Cells Enhances Killing by Cytotoxic T Lymphocytes and is Distinct From Immunogenic Cell Death. Int J Cancer (2013) 133:624–36. doi: 10.1002/IJC.28070
2. Fabian KP, Wolfson B, Hodge JW. From Immunogenic Cell Death to Immunogenic Modulation: Select Chemotherapy Regimens Induce a Spectrum of Immune-Enhancing Activities in the Tumor Microenvironment. Front Oncol (2021) 11:728018/BIBTEX. doi: 10.3389/FONC.2021.728018/BIBTEX
3. Zitvogel L, Apetoh L, Ghiringhelli F, Kroemer G. Immunological Aspects of Cancer Chemotherapy. Nat Rev Immunol (2008) 8:59–73. doi: 10.1038/nri2216
4. Bracci L, Schiavoni G, Sistigu A, Belardelli F. Immune-Based Mechanisms of Cytotoxic Chemotherapy: Implications for the Design of Novel and Rationale-Based Combined Treatments Against Cancer. Cell Death Differ (2014) 21:15. doi: 10.1038/CDD.2013.67
5. Emens LA, Middleton G. The Interplay of Immunotherapy and Chemotherapy: Harnessing Potential Synergies. Cancer Immunol Res (2015) 3:436. doi: 10.1158/2326-6066.CIR-15-0064
6. Coleman CN, Turrisi AT. Radiation and Chemotherapy Sensitizers and Protectors. Crit Rev Oncol Hematol (1990) 10:225–52. doi: 10.1016/1040-8428(90)90033-O
7. Liu T, Wu LY, Fulton MD, Johnson JM, Berkman CE. Prolonged Androgen Deprivation Leads to Downregulation of Androgen Receptor and Prostate-Specific Membrane Antigen in Prostate Cancer Cells. Int J Oncol (2012) 41:2087–92. doi: 10.3892/IJO.2012.1649/HTML
8. Wright GL, Grob BM, Haley C, Grossman K, Newhall K, Petrylak D, et al. Upregulation of Prostate-Specific Membrane Antigen After Androgen-Deprivation Therapy. Urology (1996) 48:326–34. doi: 10.1016/S0090-4295(96)00184-7
9. Cragg MS, Walshe CA, Ivanov AO, Glennie MJ. The Biology of CD20 and its Potential as a Target for mAb Therapy. Curr Dir Autoimmun (2005) 8:140–74. doi: 10.1159/000082102
10. Bodey B, Siegel SE, Kaiser HE. MAGE-1, a Cancer/Testis-Antigen, Expression in Childhood Astrocytomas as an Indicator of Tumor Progression. In Vivo (2002) 16:583–8.
11. Zalba S, ten Hagen TLM. Cell Membrane Modulation as Adjuvant in Cancer Therapy. Cancer Treat Rev (2017) 52:48–57. doi: 10.1016/j.ctrv.2016.10.008
12. Zhao Z, Chen Y, Francisco NM, Zhang Y, Wu M. The Application of CAR-T Cell Therapy in Hematological Malignancies: Advantages and Challenges. Acta Pharm Sin B (2018) 8:539. doi: 10.1016/J.APSB.2018.03.001
13. Flieger D, Hoff AS, Sauerbruch T, Schmidt-Wolf IGH. Influence of Cytokines, Monoclonal Antibodies and Chemotherapeutic Drugs on Epithelial Cell Adhesion Molecule (EpCAM) and LewisY Antigen Expression. Clin Exp Immunol (2001) 123:9–14. doi: 10.1046/J.1365-2249.2001.01435.X
14. Gómez Román VR, Murray JC, Weiner LM. Antibody-Dependent Cellular Cytotoxicity (ADCC). Antib Fc Link Adapt Innate Immun (2014), 1–27. doi: 10.1016/B978-0-12-394802-1.00001-7
15. Uda NR, Stenner F, Seibert V, Herzig P, Markuly N, van Dijk M, et al. Humanized Monoclonal Antibody Blocking Carbonic Anhydrase 12 Enzymatic Activity Leads to Reduced Tumor Growth In Vitro. Anticancer Res (2019) 39:4117–28. doi: 10.21873/ANTICANRES.13570
16. Kawashima H. Radioimmunotherapy: A Specific Treatment Protocol for Cancer by Cytotoxic Radioisotopes Conjugated to Antibodies. Sci World J (2014) 2014: 492061. doi: 10.1155/2014/492061
18. Zhao Q, Jiang Y, Xiang S, Kaboli PJ, Shen J, Zhao Y, et al. Engineered TCR-T Cell Immunotherapy in Anticancer Precision Medicine: Pros and Cons. Front Immunol (2021) 12:658753/BIBTEX. doi: 10.3389/FIMMU.2021.658753/BIBTEX
19. Stopfer LE, Gajadhar AS, Patel B, Gallien S, Frederick DT, Boland GM, et al. Absolute Quantification of Tumor Antigens Using Embedded Mhc-I Isotopologue Calibrants. Proc Natl Acad Sci U S A (2021) 118:2111173118. doi: 10.1073/PNAS.2111173118/-/DCSUPPLEMENTAL
20. Borrego F. The First Molecular Basis of the “Missing Self” Hypothesis. J Immunol (2006) 177:5759–60. doi: 10.4049/JIMMUNOL.177.9.5759
21. Kumar V, McNerney ME. A New Self: MHC-Class-I-Independent Natural-Killer-Cell Self-Tolerance. Nat Rev Immunol (2005) 5:363–74. doi: 10.1038/nri1603
22. Marshall HT, Djamgoz MBA. Immuno-Oncology: Emerging Targets and Combination Therapies. Front Oncol (2018) 8:315/BIBTEX. doi: 10.3389/FONC.2018.00315/BIBTEX
23. Barrow C, Browning J, MacGregor D, Davis ID, Sturrock S, Jungbluth AA, et al. Tumor Antigen Expression in Melanoma Varies According to Antigen and Stage. Clin Cancer Res (2006) 12:764–71. doi: 10.1158/1078-0432.CCR-05-1544
24. Mulcahy KA, Rimoldi D, Brasseur F, Rodgers S, Liénard D, Marchand M, et al. Infrequent Expression of the MAGE Gene Family in Uveal Melanomas. Int J Cancer (1996) 66:738–42. doi: 10.1002/(SICI)1097-0215(19960611)66:6<738::AID-IJC5>3.0.CO;2-0
25. Martin AD, Wang X, Sandberg ML, Negri KR, Wu ML, Toledo Warshaviak D, et al. Re-Examination of MAGE-A3 as a T-Cell Therapeutic Target. J Immunother (2021) 44:95–105. doi: 10.1097/CJI.0000000000000348
26. Chen X, Wang L, Liu J, Huang L, Li Y, Gao Q, et al. Expression and Prognostic Relevance of MAGE-A3 and MAGE-C2 in non-Small Cell Lung Cancer. Oncol Lett (2017) 13:1609–18. doi: 10.3892/OL.2017.5665/HTML
27. Ulloa-Montoya F, Louahed J, Dizier B, Gruselle O, Spiessens B, Lehmann FF, et al. Predictive Gene Signature in MAGE-A3 Antigen-Specific Cancer Immunotherapy. J Clin Oncol (2013) 31:2388–95. doi: 10.1200/JCO.2012.44.3762
28. Daud AI. Negative But Not Futile: MAGE-A3 Immunotherapeutic for Melanoma. Lancet Oncol (2018) 19:852–3. doi: 10.1016/S1470-2045(18)30353-X
29. Tang T, Huang X, Zhang G, Hong Z, Bai X, Liang T. Advantages of Targeting the Tumor Immune Microenvironment Over Blocking Immune Checkpoint in Cancer Immunotherapy. Signal Transduction Targeting Ther (2021) 6:1–13. doi: 10.1038/s41392-020-00449-4
30. Murciano-Goroff YR, Warner AB, Wolchok JD. The Future of Cancer Immunotherapy: Microenvironment-Targeting Combinations. Cell Res (2020) 30:507–19. doi: 10.1038/s41422-020-0337-2
31. Gniazdowski M, Denny W, Nelson S, Czyz M. Transcription Factors as Targets for DNA-Interacting Drugs. Curr Med Chem (2003) 10:909–24. doi: 10.2174/0929867033457683
32. Ramsay RR, Tipton KF. Assessment of Enzyme Inhibition: A Review With Examples From the Development of Monoamine Oxidase and Cholinesterase Inhibitory Drugs. Mol A J Synth Chem Nat Prod Chem (2017) 22(7), 1192. doi: 10.3390/MOLECULES22071192
33. Anderson KW, Mast N, Hudgens JW, Lin JB, Turko IV, Pikuleva IA. Mapping of the Allosteric Site in Cholesterol Hydroxylase CYP46A1 for Efavirenz, a Drug That Stimulates Enzyme Activity. J Biol Chem (2016) 291:11876–86. doi: 10.1074/JBC.M116.723577/ATTACHMENT/F2404062-090E-4F3D-84C1-3282196AA2E1/MMC1.ZIP
34. Feldman M. Adaptive Response and Enzyme Induction. In: M Feldman, LS Friedman and LJ Brandt, editors. Pithawa AK. Sleisenger and Fordtran's Gastrointestinal and Liver Disease: Med J Armed Forces India (2007) 63(2):205. doi: 10.1016/S0377-1237(07)80085-2
35. Krishnamurty R, Maly DJ. Biochemical Mechanisms of Resistance to Small-Molecule Protein Kinase Inhibitors. ACS Chem Biol (2010) 5:121. doi: 10.1021/CB9002656
36. Sever R, Glass CK. Signaling by Nuclear Receptors. Cold Spring Harb Perspect Biol (2013) 5. doi: 10.1101/CSHPERSPECT.A016709
37. Qamar S, Khokhar MA, Farooq S, Ashraf S, Humayon WA, Rehman A. Association of P53 Overexpression With Hormone Receptor Status and Triple Negative Breast Carcinoma. J Coll Physicians Surg Pak (2019) 29:164–7. doi: 10.29271/JCPSP.2019.02.164
38. Urbanucci A, Sahu B, Seppälä J, Larjo A, Latonen LM, Waltering KK, et al. Overexpression of Androgen Receptor Enhances the Binding of the Receptor to the Chromatin in Prostate Cancer. Oncogene (2011) 31:2153–63. doi: 10.1038/onc.2011.401
39. Linja MJ, Savinainen KJ, Saramäki OR, Tammela TL, Vessella RL, Visakorpi T. Amplification and Overexpression of Androgen Receptor Gene in Hormone-Refractory Prostate Cancer. Cancer Res (2001) 61:3550–5.
40. Sharma D, Saxena NK, Davidson NE, Vertino PM. Restoration of Tamoxifen Sensitivity in Estrogen Receptor–Negative Breast Cancer Cells: Tamoxifen-Bound Reactivated ER Recruits Distinctive Corepressor Complexes. Cancer Res (2006) 66:6370–8. doi: 10.1158/0008-5472.CAN-06-0402
41. Archer DF. The Gynecologic Effects of Lasofoxifene, an Estrogen Agonist/Antagonist, in Postmenopausal Women. Menopause (2011) 18:6–7. doi: 10.1097/GME.0B013E318203A46B
42. Paterni I, Granchi C, Katzenellenbogen JA, Minutolo F. Estrogen Receptors Alpha (Erα) and Beta (Erβ): Subtype-Selective Ligands and Clinical Potential. Steroids (2014) 0:13. doi: 10.1016/J.STEROIDS.2014.06.012
43. Chang EC, Frasor J, Komm B, Katzenellenbogen BS. Impact of Estrogen Receptor Beta on Gene Networks Regulated by Estrogen Receptor Alpha in Breast Cancer Cells. Endocrinology (2006) 147:4831–42. doi: 10.1210/EN.2006-0563
44. Saji S, Hirose M, Toi M. Clinical Significance of Estrogen Receptor Beta in Breast Cancer. Cancer Chemother Pharmacol (2005) 56 Suppl 1: 21–26. doi: 10.1007/S00280-005-0107-3
45. Johnston SRD, Dowsett M. Aromatase Inhibitors for Breast Cancer: Lessons From the Laboratory. Nat Rev Cancer (2003) 3:821–31. doi: 10.1038/nrc1211
46. Ellis MJ, Coop A, Singh B, Mauriac L, Llombert-Cussac A, Jänicke F, et al. Letrozole is More Effective Neoadjuvant Endocrine Therapy Than Tamoxifen for ErbB-1- and/or ErbB-2-Positive, Estrogen Receptor-Positive Primary Breast Cancer: Evidence From a Phase III Randomized Trial. J Clin Oncol (2001) 19:3808–16. doi: 10.1200/JCO.2001.19.18.3808
47. Hinsche O, Girgert R, Emons G, Gründker C. Estrogen Receptor β Selective Agonists Reduce Invasiveness of Triple-Negative Breast Cancer Cells. Int J Oncol (2015) 46:878–84. doi: 10.3892/IJO.2014.2778/HTML
48. Hartman J, Edvardsson K, Lindberg K, Zhao C, Williams C, Ström A, et al. Tumor Repressive Functions of Estrogen Receptor Beta in SW480 Colon Cancer Cells. Cancer Res (2009) 69:6100–6. doi: 10.1158/0008-5472.CAN-09-0506
49. Topi G, Satapathy SR, Dash P, Fred Mehrabi S, Ehrnström R, Olsson R, et al. Tumour-Suppressive Effect of Oestrogen Receptor β in Colorectal Cancer Patients, Colon Cancer Cells, and a Zebrafish Model. J Pathol (2020) 251:297–309. doi: 10.1002/PATH.5453
50. Olbrich T, Ziegler E, Türk G, Schubert A, Emons G, Gründker C. Kisspeptin-10 Inhibits Bone-Directed Migration of GPR54-Positive Breast Cancer Cells: Evidence for a Dose-Window Effect. Gynecol Oncol (2010) 119:571–8. doi: 10.1016/J.YGYNO.2010.08.018
51. McPherson SJ, Hussain S, Balanathan P, Hedwards SL, Niranjan B, Grant M, et al. Estrogen Receptor-Beta Activated Apoptosis in Benign Hyperplasia and Cancer of the Prostate is Androgen Independent and TNFalpha Mediated. Proc Natl Acad Sci U S A (2010) 107:3123–8. doi: 10.1073/PNAS.0905524107
52. Piccolella M, Crippa V, Messi E, Tetel MJ, Poletti A. Modulators of Estrogen Receptor Inhibit Proliferation and Migration of Prostate Cancer Cells. Pharmacol Res (2014) 79:13–20. doi: 10.1016/J.PHRS.2013.10.002
53. Lindberg K, Ström A, Lock JG, Gustafsson J.Å., Haldosén LA, Helguero LA. Expression of Estrogen Receptor β Increases Integrin α1 and Integrin β1 Levels and Enhances Adhesion of Breast Cancer Cells. J Cell Physiol (2010) 222:156–67. doi: 10.1002/JCP.21932
54. Thomas C, Rajapaksa G, Nikolos F, Hao R, Katchy A, McCollum CW, et al. ERbeta1 Represses Basal Breast Cancer Epithelial to Mesenchymal Transition by Destabilizing EGFR. Breast Cancer Res (2012) 14: R148 . doi: 10.1186/BCR3358
55. Silva RdeS, Lombardi APG, de Souza DS, Vicente CM, Porto CS. Activation of Estrogen Receptor Beta (Erβ) Regulates the Expression of N-Cadherin, E-Cadherin and β-Catenin in Androgen-Independent Prostate Cancer Cells. Int J Biochem Cell Biol (2018) 96:40–50. doi: 10.1016/J.BIOCEL.2018.01.008
56. Samanta S, Sharma VM, Khan A, Mercurio AM. Regulation of IMP3 by EGFR Signaling and Repression by Erβ: Implications for Triple-Negative Breast Cancer. Oncogene (2012) 31:4689–97. doi: 10.1038/ONC.2011.620
57. Boér K. Fulvestrant in Advanced Breast Cancer: Evidence to Date and Place in Therapy. Ther Adv Med Oncol (2017) 9:465–79. doi: 10.1177/1758834017711097
58. Chung SH, Lambert PF. Prevention and Treatment of Cervical Cancer in Mice Using Estrogen Receptor Antagonists. Proc Natl Acad Sci (2009) 106:19467–72. doi: 10.1073/PNAS.0911436106
59. Fan M, Rickert EL, Chen L, Aftab SA, Nephew KP, Weatherman RV. Characterization of Molecular and Structural Determinants of Selective Estrogen Receptor Downregulators. Breast Cancer Res Treat (2007) 103:37–44. doi: 10.1007/S10549-006-9353-2
60. Flemming J, Madarnas Y, Franek JA. Fulvestrant for Systemic Therapy of Locally Advanced or Metastatic Breast Cancer in Postmenopausal Women: A Systematic Review. Breast Cancer Res Treat (2009) 115:255–68. doi: 10.1007/S10549-008-0137-8/FIGURES/A
61. Li J, Wang Z, Shao Z. Fulvestrant in the Treatment of Hormone Receptor-Positive/Human Epidermal Growth Factor Receptor 2-Negative Advanced Breast Cancer: A Review. Cancer Med (2019) 8:1943–57. doi: 10.1002/CAM4.2095
62. Carreau S, Lambard S, Delalande C, Denis-Galeraud I, Bilinska B, Bourguiba S. Aromatase Expression and Role of Estrogens in Male Gonad: A Review. Reprod Biol Endocrinol (2003) 1:1–6. doi: 10.1186/1477-7827-1-35/FIGURES/2
63. Hamadeh IS, Patel JN, Rusin S, Tan AR. Personalizing Aromatase Inhibitor Therapy in Patients With Breast Cancer. Cancer Treat Rev (2018) 70:47–55. doi: 10.1016/J.CTRV.2018.07.014
64. Bertelli G, Gangadhara S. Exemestane in Postmenopausal Women With Early or Advanced Breast Cancer: A Review. (2010) 11(11):1933–1942. doi: 10.1517/14656566.2010.495945
65. Robertson JFR, Paridaens RJ, Lichfield J, Bradbury I, Campbell C. Meta-Analyses of Phase 3 Randomised Controlled Trials of Third Generation Aromatase Inhibitors Versus Tamoxifen as First-Line Endocrine Therapy in Postmenopausal Women With Hormone Receptor-Positive Advanced Breast Cancer. Eur J Cancer (2021) 145:19–28. doi: 10.1016/J.EJCA.2020.11.038
66. Kvinnsland S, Anker G, Dirix LY, Bonneterre J, Prove AM, Wilking N, et al. High Activity and Tolerability Demonstrated for Exemestane in Postmenopausal Women With Metastatic Breast Cancer Who had Previously Failed on Tamoxifen Treatment. Eur J Cancer (2000) 36:976–82. doi: 10.1016/S0959-8049(00)00041-1
67. Razandi M, Pedram A, Greene GL, Levin ER. Cell Membrane and Nuclear Estrogen Receptors (ERs) Originate From a Single Transcript: Studies of ERalpha and ERbeta Expressed in Chinese Hamster Ovary Cells. Mol Endocrinol (1999) 13:307–19. doi: 10.1210/MEND.13.2.0239
68. Levin ER, Pietras RJ. Estrogen Receptors Outside the Nucleus in Breast Cancer. Breast Cancer Res Treat (2008) 108:351–61. doi: 10.1007/S10549-007-9618-4/FIGURES/3
69. Vaz S, Hadaschik B, Gabriel M, Herrmann K, Eiber M, Costa D. Influence of Androgen Deprivation Therapy on PSMA Expression and PSMA-Ligand PET Imaging of Prostate Cancer Patients. Eur J Nucl Med Mol Imaging (2020) 47:9–15. doi: 10.1007/S00259-019-04529-8/TABLES/1
70. Petrylak DP, Kantoff P, Vogelzang NJ, Mega A, Fleming MT, Stephenson JJ, et al. Phase 1 Study of PSMA ADC, an Antibody-Drug Conjugate Targeting Prostate-Specific Membrane Antigen, in Chemotherapy-Refractory Prostate Cancer. Prostate (2019) 79:604–13. doi: 10.1002/PROS.23765
71. Ma D, Hopf CE, Malewicz AD, Donovan GP, Senter PD, Goeckeler WF, et al. Potent Antitumor Activity of an Auristatin-Conjugated, Fully Human Monoclonal Antibody to Prostate-Specific Membrane Antigen. Clin Cancer Res (2006) 12:2591–6. doi: 10.1158/1078-0432.CCR-05-2107
72. Zhou J, Wang G, Chen Y, Wang H, Hua Y, Cai Z. Immunogenic Cell Death in Cancer Therapy: Present and Emerging Inducers. J Cell Mol Med (2019) 23:4854. doi: 10.1111/JCMM.14356
73. Ghebeh H, Lehe C, Barhoush E, Al-Romaih K, Tulbah A, Al-Alwan M, et al. Doxorubicin Downregulates Cell Surface B7-H1 Expression and Upregulates its Nuclear Expression in Breast Cancer Cells: Role of B7-H1 as an Anti-Apoptotic Molecule. Breast Cancer Res (2010) 12:1–12. doi: 10.1186/BCR2605/FIGURES/5
74. Azuma T, Yao S, Zhu G, Flies AS, Flies SJ, Chen L. B7-H1 is a Ubiquitous Antiapoptotic Receptor on Cancer Cells. Blood (2008) 111:3635–43. doi: 10.1182/BLOOD-2007-11-123141
75. Chen L, Deng H, Lu M, Xu B, Wang Q, Jiang J, et al. B7-H1 Expression Associates With Tumor Invasion and Predicts Patient’s Survival in Human Esophageal Cancer. Int J Clin Exp Pathol (2014) 7:6015.
76. Jans DA, Sutton VR, Jans P, Froelich CJ, Trapani JA. BCL-2 Blocks Perforin-Induced Nuclear Translocation of Granzymes Concomitant With Protection Against the Nuclear Events of Apoptosis. J Biol Chem (1999) 274:3953–61. doi: 10.1074/JBC.274.7.3953
77. Gelbard A, Garnett CT, Abrams SI, Patel V, Gutkind JS, Palena C, et al. Combination Chemotherapy and Radiation of Human Squamous Cell Carcinoma of the Head and Neck Augments CTL-Mediated Lysis. Clin Cancer Res (2006) 12:1897–905. doi: 10.1158/1078-0432.CCR-05-1761
78. Modrak DE, Gold DV, Goldenberg DM, Blumenthal RD. Colonic Tumor CEA, CSAp and MUC-1 Expression Following Radioimmunotherapy or Chemotherapy. Tumor Biol (2003) 24:32–9. doi: 10.1159/000070658
79. Tibbetts LM, Doremus CM, Tzanakakis GN, Vezeridis MP. Liver Metastases With 10 Human Colon Carcinoma Cell Lines in Nude Mice and Association With Carcinoembryonic Antigen Production 71:2. doi: 10.1002/1097-0142(19930115)71:2
80. Yoshioka T, Masuko T, Kotanagi H, Aizawa O, Saito Y, Nakazato H, et al. Homotypic Adhesion Through Carcinoembryonic Antigen Plays a Role in Hepatic Metastasis Development. Jpn J Cancer Res (1998) 89:177–85. doi: 10.1111/J.1349-7006.1998.TB00546.X
81. Choi SR, Cho M, Kim HR, Ahn DH, Sleisenger MH, Kim YS. Biological Properties and Expression of Mucins in 5-Fluorouracil Resistant HT29 Human Colon Cancer Cells. Int J Oncol (2000) 17:141–7. doi: 10.3892/IJO.17.1.141
82. Krug LM, Milton DT, Jungbluth AA, Chen LC, Quaia E, Pandit-Taskar N, et al. Targeting Lewis Y (Ley) in Small Cell Lung Cancer With a Humanized Monoclonal Antibody, Hu3s193: A Pilot Trial Testing Two Dose Levels. J Thorac Oncol (2007) 2:947–52. doi: 10.1097/JTO.0B013E3181560DCC
83. Chadalapaka G, Jutooru I, Burghardt R, Safe S. Drugs That Target Specificity Proteins Downregulate Epidermal Growth Factor Receptor in Bladder Cancer Cells. Mol Cancer Res (2010) 8:739–50. doi: 10.1158/1541-7786.MCR-09-0493
84. Weihua Z, Tsan R, Huang WC, Wu Q, Chiu CH, Fidler IJ, et al. Survival of Cancer Cells is Maintained by EGFR Independent of its Kinase Activity. Cancer Cell (2008) 13:385–93. doi: 10.1016/J.CCR.2008.03.015
85. Moreno-Bueno G, Cubillo E, Sarrió D, Peinado H, Rodríguez-Pinilla SM, Villa S, et al. Genetic Profiling of Epithelial Cells Expressing E-Cadherin Repressors Reveals a Distinct Role for Snail, Slug, and E47 Factors in Epithelial-Mesenchymal Transition. Cancer Res (2006) 66:9543–56. doi: 10.1158/0008-5472.CAN-06-0479
86. Greenspan EJ, Nichols FC, Rosenberg DW. Molecular Alterations Associated With Sulindac-Resistant Colon Tumors in ApcMin/+ Mice. Cancer Prev Res (2010) 3:1187–97. doi: 10.1158/1940-6207.CAPR-09-0270
87. Kato T, Fujino H, Oyama S, Kawashima T, Murayama T. Indomethacin Induces Cellular Morphological Change and Migration via Epithelial-Mesenchymal Transition in A549 Human Lung Cancer Cells: A Novel Cyclooxygenase-Inhibition-Independent Effect. Biochem Pharmacol (2011) 82:1781–91. doi: 10.1016/J.BCP.2011.07.096
88. Wang ZL, Fan ZQ, Jiang HD, Qu JM. Selective Cox-2 Inhibitor Celecoxib Induces Epithelial-Mesenchymal Transition in Human Lung Cancer Cells via Activating MEK-ERK Signaling. Carcinogenesis (2013) 34:638–46. doi: 10.1093/CARCIN/BGS367
89. Vaish V, Sanyal SN. Non Steroidal Anti-Inflammatory Drugs Modulate the Physicochemical Properties of Plasma Membrane in Experimental Colorectal Cancer: A Fluorescence Spectroscopic Study. Mol Cell Biochem (2011) 358:161–71. doi: 10.1007/S11010-011-0931-1/FIGURES/7
90. Liggett JL, Zhang X, Eling TE, Baek SJ. Anti-Tumor Activity of non-Steroidal Anti-Inflammatory Drugs: Cyclooxygenase-Independent Targets. Cancer Lett (2014) 346:217–24. doi: 10.1016/J.CANLET.2014.01.021
91. Pangburn HA, Kraus H, Ahnen DJ, Rice PL. Sulindac Metabolites Inhibit Epidermal Growth Factor Receptor Activation and Expression. J Carcinog (2005) 4:16. doi: 10.1186/1477-3163-4-16
92. Tavolari S, Munarini A, Storci G, Laufer S, Chieco P, Guarnieri T. The Decrease of Cell Membrane Fluidity by the non-Steroidal Anti-Inflammatory Drug Licofelone Inhibits Epidermal Growth Factor Receptor Signalling and Triggers Apoptosis in HCA-7 Colon Cancer Cells. Cancer Lett (2012) 321:187–94. doi: 10.1016/J.CANLET.2012.02.003
93. Kapitanović S, Čačev T, Antica M, Kralj M, Cavrić G, Pavelić K, et al. Effect of Indomethacin on E-Cadherin and β-Catenin Expression in HT-29 Colon Cancer Cells. Exp Mol Pathol (2006) 80:91–6. doi: 10.1016/J.YEXMP.2005.04.008
94. Han L, Peng B, Ma Q, Ma J, Li J, Li W, et al. Indometacin Ameliorates High Glucose-Induced Proliferation and Invasion Via Modulation of E-Cadherin in Pancreatic Cancer Cells. Curr Med Chem (2013) 20:4142–52. doi: 10.2174/09298673113209990249
95. Hua X, Phipps AI, Burnett-Hartman AN, Adams SV, Hardikar S, Cohen SA, et al. Timing of Aspirin and Other Nonsteroidal Anti-Inflammatory Drug Use Among Patients With Colorectal Cancer in Relation to Tumor Markers and Survival. J Clin Oncol (2017) 35:2806. doi: 10.1200/JCO.2017.72.3569
96. Szakács G, Paterson JK, Ludwig JA, Booth-Genthe C, Gottesman MM. Targeting Multidrug Resistance in Cancer. Nat Rev Drug Discovery (2006) 5:219–34. doi: 10.1038/nrd1984
97. Cho Y, Kim YK. Cancer Stem Cells as a Potential Target to Overcome Multidrug Resistance. Front Oncol (2020) 10:764/BIBTEX. doi: 10.3389/FONC.2020.00764/BIBTEX
98. Dong J, Qin Z, Zhang WD, Cheng G, Yehuda AG, Ashby CR, et al. Medicinal Chemistry Strategies to Discover P-Glycoprotein Inhibitors: An Update. Drug Resist Updat (2020) 49:100681. doi: 10.1016/J.DRUP.2020.100681
99. Palmeira A, Sousa E, Vasconcelos MH, Pinto MM. Three Decades of P-Gp Inhibitors: Skimming Through Several Generations and Scaffolds. Curr Med Chem (2012) 19:1946–2025. doi: 10.2174/092986712800167392
100. Krishna R, Mayer LD. Multidrug Resistance (MDR) in Cancer: Mechanisms, Reversal Using Modulators of MDR and the Role of MDR Modulators in Influencing the Pharmacokinetics of Anticancer Drugs. Eur J Pharm Sci (2000) 11:265–83. doi: 10.1016/S0928-0987(00)00114-7
101. Amin ML. P-Glycoprotein Inhibition for Optimal Drug Delivery. Drug Target Insights (2013) 7:27. doi: 10.4137/DTI.S12519
102. Tamaki A, Ierano C, Szakacs G, Robey RW, Bates SE. The Controversial Role of ABC Transporters in Clinical Oncology. Essays Biochem (2011) 50:209. doi: 10.1042/BSE0500209
103. Choi YH, Yu A-M. ABC Transporters in Multidrug Resistance and Pharmacokinetics, and Strategies for Drug Development. Curr Pharm Des (2014) 20:793. doi: 10.2174/138161282005140214165212
104. Lee J, Kang J, Kwon N-Y, Sivaraman A, Naik R, Jin S-Y, et al. Dual Inhibition of P-Gp and BCRP Improves Oral Topotecan Bioavailability in Rodents. (2021) 13(4):559. doi: 10.3390/pharmaceutics13040559
105. Zakeri-Milani P, Valizadeh H. Intestinal Transporters: Enhanced Absorption Through P-Glycoprotein-Related Drug Interactions. Expert Opin Drug Metab Toxicol (2014) 10:859–71. doi: 10.1517/17425255.2014.905543
106. Ullah MF. Cancer Multidrug Resistance (MDR): A Major Impediment to Effective Chemotherapy. Asian Pacific J Cancer Prev (2008) 9:1–16.
107. Przystupski D, Niemczura MJ, Górska A, Supplitt S, Kotowski K, Wawryka P, et al. In Search of Panacea—review of Recent Studies Concerning Nature-Derived Anticancer Agents. Nutrients (2019) 11(6):1426. doi: 10.3390/nu11061426
108. Minko T, Pakunlu RI, Wang Y, Khandare JJ, Saad M. New Generation of Liposomal Drugs for Cancer. Anticancer Agents Med Chem (2008) 6:537–52. doi: 10.2174/187152006778699095
109. Ji N, Yuan J, Liu J, Tian S. Developing Multidrug-Resistant Cells and Exploring Correlation Between BCRP/ABCG2 Over-Expression and DNA Methyltransferase. Acta Biochim Biophys Sin (Shanghai) (2010) 42:854–62. doi: 10.1093/ABBS/GMQ097
110. Volk EL, Farley KM, Wu Y, Li F, Robey RW, Schneider E. Overexpression of Wild-Type Breast Cancer Resistance Protein Mediates Methotrexate Resistance. Cancer Res (2002) 62:5035 LP–5040.
111. Huang R, Murry DJ, Kolwankar D, Hall SD, Foster DR. Vincristine Transcriptional Regulation of Efflux Drug Transporters in Carcinoma Cell Lines. Biochem Pharmacol (2006) 71:1695–704. doi: 10.1016/J.BCP.2006.03.009
112. Shionoya M, Jimbo T, Kitagawa M, Soga T, Tohgo A. DJ-927, a Novel Oral Taxane, Overcomes P-Glycoprotein-Mediated Multidrug Resistance In Vitro and In Vivo. Cancer Sci (2003) 94:459–66. doi: 10.1111/J.1349-7006.2003.TB01465.X
113. Stordal B, Hamon M, McEneaney V, Roche S, Gillet JP, O’Leary JJ, et al. Resistance to Paclitaxel in a Cisplatin-Resistant Ovarian Cancer Cell Line Is Mediated by P-Glycoprotein. PloS One (2012) 7:e40717. doi: 10.1371/JOURNAL.PONE.0040717
114. Lee JP, Hahn HS, Hwang SJ, Choi JY, Park JS, Lee IH, et al. Selective Cyclooxygenase Inhibitors Increase Paclitaxel Sensitivity in Taxane-Resistant Ovarian Cancer by Suppressing P-Glycoprotein Expression. J Gynecol Oncol (2013) 24:273–9. doi: 10.3802/JGO.2013.24.3.273
115. Szlasa W, Kiełbik A, Szewczyk A, Rembiałkowska N, Novickij V, Tarek M, et al. Oxidative Effects During Irreversible Electroporation of Melanoma Cells-In Vitro Study. Molecules (2020) 26:154. doi: 10.3390/molecules26010154
116. Campana LG, Edhemovic I, Soden D, Perrone AM, Scarpa M, Campanacci L, et al. Electrochemotherapy – Emerging Applications Technical Advances, New Indications, Combined Approaches, and Multi-Institutional Collaboration. Eur J Surg Oncol (2019) 45:92–102. doi: 10.1016/j.ejso.2018.11.023
117. Saulis G, Saul R. Comparison of Electroporation Threshold for Different Cell Lines In Vitro. (2009) 115:. doi: 10.12693/APhysPolA.115.1056
118. Kiełbik A, Szlasa W, Michel O, Szewczyk A, Tarek M, Saczko J, et al. In Vitro Study of Calcium Microsecond Electroporation of Prostate Adenocarcinoma Cells. Molecules (2020) 25:5406. doi: 10.3390/molecules25225406
119. Saulis G, Venslauskas MS, Naktinis J. Kinetics of Pore Resealing in Cell Membranes After Electroporation. J Electroanal Chem Interfacial Electrochem (1991) 321:1–13. doi: 10.1016/0022-0728(91)85564-6
120. Lucas ML, Heller L, Coppola D, Heller R. IL-12 Plasmid Delivery by in Vivo Electroporation for the Successful Treatment of Established Subcutaneous B16.F10 Melanoma. Mol Ther (2002) 5:668–75. doi: 10.1006/mthe.2002.0601
121. Daud AI, DeConti RC, Andrews S, Urbas P, Riker AI, Sondak VK, et al. Phase I Trial of Interleukin-12 Plasmid Electroporation in Patients With Metastatic Melanoma. J Clin Oncol (2008) 26:5896–903. doi: 10.1200/JCO.2007.15.6794
122. Heller L, Merkler K, Westover J, Cruz Y, Coppola D, Benson K, et al. Evaluation of Toxicity Following Electrically Mediated Interleukin-12 Gene Delivery in a B16 Mouse Melanoma Model. Clin Cancer Res (2006) 12:3177–83. doi: 10.1158/1078-0432.CCR-05-2727
123. Cha E, Daud A. Plasmid IL-2 Electroporation in Melanoma. Hum Vaccines Immunother (2012) 8:1734–8. doi: 10.4161/hv.22573
124. Pen JJ, Keersmaecker B, Maenhout SK, Van Nuffel AMT, Heirman C, Corthals J, et al. Modulation of Regulatory T Cell Function by Monocyte-Derived Dendritic Cells Matured Through Electroporation With mRNA Encoding CD40 Ligand, Constitutively Active TLR4, and CD70. J Immunol (2013) 191:1976–83. doi: 10.4049/JIMMUNOL.1201008
125. Dannull J, Lesher DT, Holzknecht R, Qi W, Hanna G, Seigler H, et al. Immunoproteasome Down-Modulation Enhances the Ability of Dendritic Cells to Stimulate Antitumor Immunity. Blood (2007) 110:4341–50. doi: 10.1182/BLOOD-2007-04-083188
126. Tannig P, Peter AS, Lapuente D, Klessing S, Damm D, Tenbusch M, et al. Modulation of Vaccine-Induced HIV-1-Specific Immune Responses by Co-Electroporation of PD-L1 Encoding DNA. Vaccines (2020) 8:27. doi: 10.3390/VACCINES8010027
127. Ginsberg AA, Shen X, Hutnick NA, Weiner DB. Improvement of DNA Vaccines by Electroporation. Gene Vaccines (2012) 9783709104392:145–62. doi: 10.1007/978-3-7091-0439-2_7
128. Beitel-White N, Martin RCGG, Li Y, Brock RM, Allen IC, Davalos RV. Real-Time Prediction of Patient Immune Cell Modulation During Irreversible Electroporation Therapy. (2019) 9:17739. doi: 10.1038/s41598-019-53974-w
129. Scheffer HJ, Stam AGM, Geboers B, Vroomen LGPH, Ruarus A, de Bruijn B, et al. Irreversible Electroporation of Locally Advanced Pancreatic Cancer Transiently Alleviates Immune Suppression and Creates a Window for Antitumor T Cell Activation. Oncoimmunology (2019) 8:11. doi: 10.1080/2162402X.2019.1652532
130. Dai Z, Wang Z, Lei K, Liao J, Peng Z, Lin M, et al. Irreversible Electroporation Induces CD8+ T Cell Immune Response Against Post-Ablation Hepatocellular Carcinoma Growth. Cancer Lett (2021) 503:1–10. doi: 10.1016/J.CANLET.2021.01.001
131. Vénéreau E, Ceriotti C, Bianchi ME. DAMPs From Cell Death to New Life. Front Immunol (2015) 6:422/BIBTEX. doi: 10.3389/FIMMU.2015.00422/BIBTEX
132. Roh JS, Sohn DH. Damage-Associated Molecular Patterns in Inflammatory Diseases. Immune Netw (2018) 18(4):e27. doi: 10.4110/IN.2018.18.E27
133. Yimingjiang M, Tuergan T, Chen X, Wen H, Shao Y, Zhang R, et al. Comparative Analysis of Immunoactivation by Nanosecond Pulsed Electric Fields and PD-1 Blockade in Murine Hepatocellular Carcinoma. Anal Cell Pathol (2020) 2020:9582731. doi: 10.1155/2020/9582731
134. Novickij V, Malyško V, Želvys A, Balevičiūte A, Zinkevičiene A, Novickij J, et al. Electrochemotherapy Using Doxorubicin and Nanosecond Electric Field Pulses: A Pilot In Vivo Study. Molecules (2020) 25(20), 4601. doi: 10.3390/molecules25204601
135. Gallagher M, Chin KY, MacKenzie-Ross A. Bleomycin Electrochemotherapy for the Management of Locally Advanced Metastatic Melanoma: Two Notable Clinical Cases Potentially Indicating a Greater Therapeutic Role in the Era of Targeted and Immuno-Therapy. JPRAS Open (2020) 26:43–8. doi: 10.1016/J.JPRA.2020.09.007
136. Rudno-rudzinska J, Kielan W, Guziński M, Płochocki M, Kulbacka J. The First Study of Irreversible Electroporation With Calcium Ions and Chemotherapy in Patients With Locally Advanced Pancreatic Adenocarcinoma. Appl Sci (2020) 10:1–12. doi: 10.3390/app10155163
137. Gerlini G, Di Gennaro P, Borgognoni L. Enhancing Anti-Melanoma Immunity by Electrochemotherapy and In Vivo Dendritic-Cell Activation. (2012) 1:1655–1657. doi: 10.4161/ONCI.21991
138. Napotnik TB, Wu YH, Gundersen MA, Miklavčič D, Vernier PT. Nanosecond Electric Pulses Cause Mitochondrial Membrane Permeabilization in Jurkat Cells. Bioelectromagnetics (2012) 33:257–64. doi: 10.1002/BEM.20707
139. Szlasa W, Kiełbik A, Szewczyk A, Novickij V, Tarek M, Łapińska Z, et al. Atorvastatin Modulates the Efficacy of Electroporation and Calcium Electrochemotherapy. Int J Mol Sci (2021) 22:11245. doi: 10.3390/IJMS222011245
140. Chen N, Garner AL, Chen G, Jing Y, Deng Y, Swanson RJ, et al. Nanosecond Electric Pulses Penetrate the Nucleus and Enhance Speckle Formation. Biochem Biophys Res Commun (2007) 364:220–5. doi: 10.1016/J.BBRC.2007.09.125
141. Yano K, Morotomi-Yano K. Cell Stress Responses to Pulsed Electric Fields. Handb Electroporation (2016), 1–17. doi: 10.1007/978-3-319-26779-1_17-1
142. Morotomi-Yano K, Oyadomari S, Akiyama H, Yano K. Ichi Nanosecond Pulsed Electric Fields Act as a Novel Cellular Stress That Induces Translational Suppression Accompanied by Eif2α Phosphorylation and 4E-BP1 Dephosphorylation. Exp Cell Res (2012) 318:1733–44. doi: 10.1016/J.YEXCR.2012.04.016
143. Morotomi-Yano K, Akiyama H, Yano KI. Nanosecond Pulsed Electric Fields Activate MAPK Pathways in Human Cells. Arch Biochem Biophys (2011) 515:99–106. doi: 10.1016/J.ABB.2011.09.002
144. Morotomi-Yano K, Uemura Y, Katsuki S, Akiyama H, Yano Ki. Activation of the JNK Pathway by Nanosecond Pulsed Electric Fields. Biochem Biophys Res Commun (2011) 408:471–6. doi: 10.1016/J.BBRC.2011.04.056
145. Vernier PT, Sun Y, Marcu L, Salemi S, Craft CM, Gundersen MA. Calcium Bursts Induced by Nanosecond Electric Pulses. Biochem Biophys Res Commun (2003) 310:286–95. doi: 10.1016/j.bbrc.2003.08.140
146. Morotomi-Yano K, Akiyama H, Yano Ki. Nanosecond Pulsed Electric Fields Activate AMP-Activated Protein Kinase: Implications for Calcium-Mediated Activation of Cellular Signaling. Biochem Biophys Res Commun (2012) 428:371–5. doi: 10.1016/J.BBRC.2012.10.061
147. Samovski D, Su X, Xu Y, Abumrad NA, Stahl PD. Insulin and AMPK Regulate FA Translocase/CD36 Plasma Membrane Recruitment in Cardiomyocytes via Rab GAP AS160 and Rab8a Rab GTPase. J Lipid Res (2012) 53:709–17. doi: 10.1194/JLR.M023424
148. Zhang K, Guo J, Ge Z, Zhang J. Nanosecond Pulsed Electric Fields (nsPEFs) Regulate Phenotypes of Chondrocytes Through Wnt/β-Catenin Signaling Pathway. Sci Rep (2014) 4:1–8. doi: 10.1038/srep05836
149. Estlack LE, Roth CC, Thompson GL, Lambert WA, Ibey BL. Nanosecond Pulsed Electric Fields Modulate the Expression of Fas/CD95 Death Receptor Pathway Regulators in U937 and Jurkat Cells. Apoptosis (2014) 19:1755–68. doi: 10.1007/S10495-014-1041-9/FIGURES/8
150. Muratori C, Pakhomov AG, Gianulis E, Meads J, Casciola M, Mollica PA, et al. Activation of the Phospholipid Scramblase TMEM16F by Nanosecond Pulsed Electric Fields (nsPEF) Facilitates its Diverse Cytophysiological Effects. J Biol Chem (2017) 292:19381–91. doi: 10.1074/JBC.M117.803049
151. Kwiatkowski S, Knap B, Przystupski D, Saczko J, Kędzierska E, Knap-Czop K, et al. Photodynamic Therapy – Mechanisms, Photosensitizers and Combinations. Biomed Pharmacother (2018) 106:1098–107. doi: 10.1016/j.biopha.2018.07.049
152. El-Hussein A, Harith M, Abrahamse H. Assessment of DNA Damage After Photodynamic Therapy Using a Metallophthalocyanine Photosensitizer. Int J Photoenergy (2012) 2012, 281068:10. doi: 10.1155/2012/281068
153. Szlasa W, Supplitt S, Drąg-Zalesińska M, Przystupski D, Kotowski K, Szewczyk A, et al. Effects of Curcumin Based PDT on the Viability and the Organization of Actin in Melanotic (A375) and Amelanotic Melanoma (C32)– In Vitro Studies. Biomed Pharmacother (2020) 132:110883. doi: 10.1016/j.biopha.2020.110883
154. Nix DA, Fradelizi J, Bockholt S, Menichi B, Louvard D, Friederich E, et al. Targeting of Zyxin to Sites of Actin Membrane Interaction and to the Nucleus *. J Biol Chem (2001) 276:34759–67. doi: 10.1074/JBC.M102820200
155. Hunt DWC, Levy JG. Immunomodulatory Aspects of Photodynamic Therapy. Expert Opinion on Investigational Drugs (1998) 7:57–64. doi: 10.1517/13543784.7.1.57
156. Mroz P, Szokalska A, Wu MX, Hamblin MR. Photodynamic Therapy of Tumors Can Lead to Development of Systemic Antigen-Specific Immune Response. PloS One (2010) 5:e15194. doi: 10.1371/JOURNAL.PONE.0015194
157. Kabingu E, Oseroff AR, Wilding GE, Gollnick SO. Enhanced Systemic Immune Reactivity to a Basal Cell Carcinoma Associated Antigen Following Photodynamic Therapy. Clin Cancer Res (2009) 15:4460–6. doi: 10.1158/1078-0432.CCR-09-0400
158. Mroz P, Vatansever F, Muchowicz A, Hamblin MR. Photodynamic Therapy of Murine Mastocytoma Induces Specific Immune Responses Against the Cancer/Testis Antigen P1A. Cancer Res (2013) 73:6462–70. doi: 10.1158/0008-5472.CAN-11-2572
159. Reginato E, Mroz P, Chung H, Kawakubo M, Wolf P, Hamblin MR. Photodynamic Therapy Plus Regulatory T-Cell Depletion Produces Immunity Against a Mouse Tumour That Expresses a Self-Antigen. Br J Cancer (2013) 109:2167–74. doi: 10.1038/bjc.2013.580
160. Wang H, Wang K, He L, Liu Y, Dong H, Li Y. Engineering Antigen as Photosensitiser Nanocarrier to Facilitate ROS Triggered Immune Cascade for Photodynamic Immunotherapy. Biomaterials (2020) 244:119964. doi: 10.1016/J.BIOMATERIALS.2020.119964
161. Wang X, Tsui B, Ramamurthy G, Zhang P, Meyers J, Kenney ME, et al. Theranostic Agents for Photodynamic Therapy of Prostate Cancer by Targeting Prostate-Specific Membrane Antigen. Mol Cancer Ther (2016) 15:1834–44. doi: 10.1158/1535-7163.MCT-15-0722
162. Korbelik M. PDT-Associated Host Response and its Role in the Therapy Outcome. Lasers Surg Med (2006) 38:500–8. doi: 10.1002/LSM.20337
163. Zhang SY, Li JL, Xu XK, Zheng MG, Wen CC, Li FC. HMME-Based PDT Restores Expression and Function of Transporter Associated With Antigen Processing 1 (TAP1) and Surface Presentation of MHC Class I Antigen in Human Glioma. J Neurooncol (2011) 105:199–210. doi: 10.1007/S11060-011-0584-7/FIGURES/4
164. Hryhorenko EA, Oseroff AR, Morgan J, Rittenhouse-Diakun K. Antigen Specific and Nonspecific Modulation of the Immune Response by Aminolevulinic Acid Based Photodynamic Therapy. Immunopharmacology (1998) 40:231–40. doi: 10.1016/S0162-3109(98)00047-2
165. Mroz P, Hamblin MR. The Immunosuppressive Side of PDT. Photochem Photobiol Sci (2011) 10:751–8. doi: 10.1039/C0PP00345J
166. Harno E, White A. Adrenocorticotropic Hormone. Endocrinol Adult Pediatr (2016) 1–2:129–146.e5. doi: 10.1016/B978-0-323-18907-1.00008-1
167. Regazzetti C, Sormani L, Debayle D, Bernerd F, Tulic MK, De Donatis GM, et al. Melanocytes Sense Blue Light and Regulate Pigmentation Through Opsin-3. J Invest Dermatol (2018) 138:171–8. doi: 10.1016/J.JID.2017.07.833
168. Yamamoto H, Yamane T, Iguchi K, Tanaka K, Iddamalgoda A, Unno K, et al. Melanin Production Through Novel Processing of Proopiomelanocortin in the Extracellular Compartment of the Auricular Skin of C57BL/6 Mice After UV-Irradiation. Sci Rep (2015) 5:1–11. doi: 10.1038/srep14579
169. Akalestou E, Genser L, Rutter GA. Glucocorticoid Metabolism in Obesity and Following Weight Loss. Front Endocrinol (Lausanne) (2020) 11:59/BIBTEX. doi: 10.3389/FENDO.2020.00059/BIBTEX
170. Chen H, Rogalski MM, Anker JN. Advances in Functional X-Ray Imaging Techniques and Contrast Agents. Phys Chem Chem Phys (2012) 14:13469. doi: 10.1039/C2CP41858D
171. Lusic H, Grinstaff MW. X-Ray-Computed Tomography Contrast Agents. Chem Rev (2012) 113:1641–66. doi: 10.1021/CR200358S
172. Bradley MO, Erickson LC. Comparison of the Effects of Hydrogen Peroxide and X-Ray Irradiation on Toxicity, Mutation, and DNA Damage/Repair in Mammalian Cells (V-79). Biochim Biophys Acta - Nucleic Acids Protein Synth (1981) 654:135–41. doi: 10.1016/0005-2787(81)90146-5
173. Wozny AS, Vares G, Alphonse G, Lauret A, Monini C, Magné N, et al. ROS Production and Distribution: A New Paradigm to Explain the Differential Effects of X-Ray and Carbon Ion Irradiation on Cancer Stem Cell Migration and Invasion. Cancers (2019) 11:468. doi: 10.3390/CANCERS11040468
174. Alhmoud JF, Woolley JF, Al Moustafa AE, Malki MI. DNA Damage/Repair Management in Cancers. Cancers (Basel) (2020) 12(4), 1050. doi: 10.3390/CANCERS12041050
175. Willers H, Xia F, Powell SN. Recombinational DNA Repair in Cancer and Normal Cells: The Challenge of Functional Analysis. J Biomed Biotechnol (2002) 2002:86–93. doi: 10.1155/S1110724302204027
176. Li LY, Guan Y, Chen XS, Yang JM, Cheng Y. DNA Repair Pathways in Cancer Therapy and Resistance. Front Pharmacol (2021) 11:629266/BIBTEX. doi: 10.3389/FPHAR.2020.629266/BIBTEX
177. Borek C. X-Ray-Induced In Vitro Neoplastic Transformation of Human Diploid Cells. Nat (1980) 283:776–8. doi: 10.1038/283776a0
178. Pack GT, Davis J. Radiation Cancer of the Skin1. Radiology (1965) 84:436–42. doi: 10.1148/84.3.436
179. Charames GS, Bapat B. Genomic Instability and Cancer. Curr Mol Med (2005) 3:589–96. doi: 10.2174/1566524033479456
180. Nordling CO. A New Theory on the Cancer-Inducing Mechanism. Br J Cancer (1953) 7:68. doi: 10.1038/BJC.1953.8
181. Miller IP, Pavlović I, Poljšak B, Šuput D, Milisav I. Beneficial Role of ROS in Cell Survival: Moderate Increases in H2O2 Production Induced by Hepatocyte Isolation Mediate Stress Adaptation and Enhanced Survival. Antioxidants (2019) 8(10): 434. doi: 10.3390/ANTIOX8100434
182. Yang CR, Leskov K, Hosley-Eberlein K, Criswell T, Pink JJ, Kinsella TJ, et al. Nuclear Clusterin/XIP8, an X-Ray-Induced Ku70-Binding Protein That Signals Cell Death. Proc Natl Acad Sci (2000) 97:5907–12. doi: 10.1073/PNAS.97.11.5907
183. McKhann CF. The Effect of X-Ray on the Antigenicity of Donor Cells in Transplantation Immunity Charles F. McKhann. J Immunol (1964) 92 (5):811–815. doi: 10.1097/00007890-196411000-00026
184. Murray JE, Merrill JP, Dammin GJ, Dealy JB, Walter CW, Brooke MS, et al. Study on Transplantation Immunity After Total Body Irradiation: Clinical and Experimental Investigation. Surgery (1960) 48:272–84. doi: 10.5555/URI:PII:0039606060902294
185. Lohr F, Hof H, Weber KJ, Latz D, Wenz F. X-Ray Induced Changes in Immunostaining of Proliferating Cell Nuclear Antigen (PCNA) in V79 Hamster Fibroblasts. Strahlenther Onkol (1998) 174:575–9. doi: 10.1007/BF03038295
186. Miura M, Sasaki T, Takasaki Y. Characterization of X-Ray-Induced Immunostaining of Proliferating Cell Nuclear Antigen in Human Diploid Fibroblasts. Radiat Res (1996) 145:75–80. doi: 10.2307/3579198
187. Shreder K, Rapp F, Tsoukala I, Rzeznik V, Wabitsch M, Fischer-Posovszky P, et al. Impact of X-Ray Exposure on the Proliferation and Differentiation of Human Pre-Adipocytes. Int J Mol Sci (2018) 19:2717. doi: 10.3390/IJMS19092717
188. Huang Q, Chai H, Wang S, Sun Y, Xu W. 0.5-Gy X-Ray Irradiation Induces Reorganization of Cytoskeleton and Differentiation of Osteoblasts. Mol Med Rep (2021) 23:1–11. doi: 10.3892/MMR.2021.12018/HTML
189. Hareyama M, Imai K, Kubo K, Takahashi H, Koshiba H, Hinoda Y, et al. Effect of Radiation on the Expression of Carcinoembryonic Antigen of Human Gastric Adenocarcinoma Cells. Cancer (1991) 67:2269–74. doi: 10.1002/1097-0142(19910501)67:9<2269::aid-cncr2820670910>3.0.co;2-z
190. Wittenborn TR, Hagert CF, Ferapontov A, Fonager S, Jensen L, Winther G, et al. Comparison of Gamma and X-Ray Irradiation for Myeloablation and Establishment of Normal and Autoimmune Syngeneic Bone Marrow Chimeras. PloS One (2021) 16:e0247501. doi: 10.1371/JOURNAL.PONE.0247501
191. Tandl D, Sponagel T, Fuck S, Smit T, Hehlgans S, Jakob B, et al. X-Ray Irradiation Activates Immune Response in Human T-Lymphocytes by Eliciting a Ca2+ Signaling Cascade. bioRxiv (2020) 2020.11.13.379982. doi: 10.1101/2020.11.13.379982
192. Kadam SB, Shyama SK, Almeida VG. Evaluation of the In Vivo Genotoxic Effects of Gamma Radiation on the Peripheral Blood Leukocytes of Head and Neck Cancer Patients Undergoing Radiotherapy. Mutat Res Toxicol Environ Mutagen (2013) 752:42–6. doi: 10.1016/J.MRGENTOX.2013.01.003
193. Kobunai T, Watanabe T, Fukusato T. REG4, NEIL2, and BIRC5 Gene Expression Correlates With Gamma-Radiation Sensitivity in Patients With Rectal Cancer Receiving Radiotherapy. Anticancer Res (2011) 31:4147–53.
194. Daniels RD, Schubauer-Berigan MK. A Meta-Analysis of Leukaemia Risk From Protracted Exposure to Low-Dose Gamma Radiation. Occup Environ Med (2011) 68:457–64. doi: 10.1136/OEM.2009.054684
195. Cartwright RA, Law G, Roman E, Gilman E, Eden OB, Mott M, et al. The United Kingdom Childhood Cancer Study of Exposure to Domestic Sources of Ionising Radiation: 2: Gamma Radiation. Br J Cancer (2002) 86:1727. doi: 10.1038/SJ.BJC.6600277
196. Spix C, Grosche B, Bleher M, Kaatsch P, Scholz-Kreisel P, Blettner M. Background Gamma Radiation and Childhood Cancer in Germany: An Ecological Study. Radiat Environ Biophys (2017) 56:127–38. doi: 10.1007/S00411-017-0689-2/FIGURES/4
197. Hofmanová J, Musilová E, Kozubík A. Suppression of Human Cancer Cell Proliferation by Lipoxygenase Inhibitors and Gamma-Radiation In Vitro. Gen Physiol Biophys (1996) 15:317–31.
198. Girdhani S, Ahmed MM, Mishra KP. Enhancement of Gamma Radiation-Induced Cytotoxicity of Breast Cancer Cells by Curcumin. Mol Cell Pharmacol (2009) 1:208–17. doi: 10.4255/MCPHARMACOL.09.25
199. Vučić V, Isenović ER, Adžić M, Ruždijić S, Radojčić MB. Effects of Gamma-Radiation on Cell Growth, Cycle Arrest, Death, and Superoxide Dismutase Expression by DU 145 Human Prostate Cancer Cells. Braz J Med Biol Res (2006) 39:227–36. doi: 10.1590/S0100-879X2006000200009
200. Kassis AI. Therapeutic Radionuclides: Biophysical and Radiobiologic Principles. Semin Nucl Med (2008) 38:358. doi: 10.1053/J.SEMNUCLMED.2008.05.002
201. Lassmann M, Eberlein U. Targeted Alpha-Particle Therapy: Imaging, Dosimetry, and Radiation Protection. Annals of the ICRP (2018) 47(3–4): 187–195. doi: 10.1177/0146645318756253
202. Wyszomirska A. Iodine-131 for Therapy of Thyroid Diseases. Physical and Biological Basis. Nucl Med Rev Cent East Eur (2012) 15:120–3. doi: 10.5603/NMR.2011.00022
203. Gregianin M, Bui F, Varotto L, Zucchetta P, Macri C. [Nuclear Medicine Methods for the Diagnosis of Adrenal Tumors]. Minerva Endocrinol (1995) 20:27–38.
204. Quach A, Ji L, Mishra V, Sznewajs A, Veatch J, Huberty J, et al. Thyroid and Hepatic Function After High Dose 131i-Metaiodobenzylguanidine (131i-MIBG) Therapy for Neuroblastoma. Pediatr Blood Cancer (2011) 56:191. doi: 10.1002/PBC.22767
205. Mumtaz M, Lin LS, Hui KC, Khir ASM. Radioiodine I-131 For The Therapy Of Graves’ Disease. Malays J Med Sci (2009) 16:25.
206. Kiyatkin EA. Brain Hyperthermia During Physiological and Pathological Conditions: Causes, Mechanisms, and Functional Implications. Curr Neurovasc Res (2005) 1:77–90. doi: 10.2174/1567202043480233
207. Kiyatkin EA. Brain Hyperthermia as Physiological and Pathological Phenomena. Brain Res Rev (2005) 50:27–56. doi: 10.1016/J.BRAINRESREV.2005.04.001
208. Kiyatkin EA. Physiological and Pathological Brain Hyperthermia. Prog Brain Res (2007) 162:219–43. doi: 10.1016/S0079-6123(06)62012-8
209. Muckle DS, Dickson JA. The Selective Inhibitory Effect of Hyperthermia on the Metabolism and Growth of Malignant Cells. Br J Cancer (1971) 25:771–8. doi: 10.1038/bjc.1971.91
210. Ito A, Honda H, Kobayashi T. Cancer Immunotherapy Based on Intracellular Hyperthermia Using Magnetite Nanoparticles: A Novel Concept of “Heat-Controlled Necrosis” With Heat Shock Protein Expression. Cancer Immunol Immunother (2006) 55:320–8. doi: 10.1007/S00262-005-0049-Y/FIGURES/6
211. Poon IKH, Hulett MD, Parish CR. Molecular Mechanisms of Late Apoptotic/Necrotic Cell Clearance. Cell Death Differ (2009) 17:381–97. doi: 10.1038/cdd.2009.195
212. Westman J, Grinstein S, Marques PE. Phagocytosis of Necrotic Debris at Sites of Injury and Inflammation. Front Immunol (2020) 10:3030/BIBTEX. doi: 10.3389/FIMMU.2019.03030/BIBTEX
213. Basu S, Binder RJ, Suto R, Anderson KM, Srivastava PK. Necrotic But Not Apoptotic Cell Death Releases Heat Shock Proteins, Which Deliver a Partial Maturation Signal to Dendritic Cells and Activate the NF-Kappa B Pathway. Int Immunol (2000) 12:1539–46. doi: 10.1093/INTIMM/12.11.1539
214. Guo X, Du F, Liu Q, Guo Y, Wang Q, Huang W, et al. Immunological Effect of Irreversible Electroporation on Hepatocellular Carcinoma. BMC Cancer (2021) 21:443. doi: 10.1186/S12885-021-08176-X
215. Lee SY, Szigeti GP, Szasz AM. Oncological Hyperthermia: The Correct Dosing in Clinical Applications. Int J Oncol (2019) 54:627–43. doi: 10.3892/IJO.2018.4645/HTML
216. Lee TH, Bu J, Kim BH, Poellmann MJ, Hong S, Hyun SH. Sub-Lethal Hyperthermia Promotes Epithelial-to-Mesenchymal-Like Transition of Breast Cancer Cells: Implication of the Synergy Between Hyperthermia and Chemotherapy. RSC Adv (2018) 9:52–7. doi: 10.1039/C8RA08472F
217. Henle KJ, Leeper DB. Interaction of Sublethal and Potentially Lethal 45°-Hyperthermia and Radiation Damage at 0, 20, 37 or 40°C. Eur J Cancer (1979) 15:1387–94. doi: 10.1016/0014-2964(79)90116-6
218. Ben Hur E, Elkind MM, Bronk BV. Thermally Enhanced Radioresponse of Cultured Chinese Hamster Cells: Inhibition of Repair of Sublethal Damage and Enhancement of Lethal Damage. Radiat Res (1974) 58:38–51. doi: 10.2307/3573947
219. Masunaga SI, Nagata K, Suzuki M, Kashino G, Kinashi Y, Ono K. Inhibition of Repair of Radiation-Induced Damage by Mild Temperature Hyperthermia, Referring to the Effect on Quiescent Cell Populations. Radiat Med (2007) 25:417–25. doi: 10.1007/S11604-007-0160-4
220. Adnan A, Muñoz NM, Prakash P, Habibollahi P, Cressman ENK, Sheth RA. Hyperthermia and Tumor Immunity. Cancers (2021) 13:2507. doi: 10.3390/CANCERS13112507
221. Tsang YW, Huang CC, Yang KL, Chi MS, Chiang HC, Wang YS, et al. Improving Immunological Tumor Microenvironment Using Electro-Hyperthermia Followed by Dendritic Cell Immunotherapy. BMC Cancer (2015) 15:1–11. doi: 10.1186/S12885-015-1690-2/FIGURES/6
222. Vancsik T, Kovago C, Kiss E, Papp E, Forika G, Benyo Z, et al. Modulated Electro-Hyperthermia Induced Loco-Regional and Systemic Tumor Destruction in Colorectal Cancer Allografts. J Cancer (2018) 9:41–53. doi: 10.7150/JCA.21520
223. Muñoz NM, Dupuis C, Williams M, Dixon K, McWatters A, Avritscher R, et al. Molecularly Targeted Photothermal Ablation Improves Tumor Specificity and Immune Modulation in a Rat Model of Hepatocellular Carcinoma. Commun Biol (2020) 3:1–10. doi: 10.1038/s42003-020-01522-y
224. Liburdy RP. Radiofrequency Radiation Alters the Immune System: Modulation of T- and B-Lymphocyte Levels and Cell-Mediated Immunocompetence by Hyperthermic Radiation. Radiat Res (1979) 77:34–46. doi: 10.2307/3575075
225. Umar D, Das A, Gupta S, Chattopadhyay S, Sarkar D, Mirji G, et al. Febrile Temperature Change Modulates CD4 T Cell Differentiation via a TRPV Channel-Regulated Notch-Dependent Pathway. Proc Natl Acad Sci USA (2020) 117:22357–66. doi: 10.1073/PNAS.1922683117/-/DCSUPPLEMENTAL
226. Tomiyama-Miyaji C, Watanabe M, Ohishi T, Kanda Y, Kainuma E, Bakir HY, et al. Modulation of the Endocrine and Immune Systems by Well-Controlled Hyperthermia Equipment. Biomed Res (2007) 28:119–25. doi: 10.2220/BIOMEDRES.28.119
227. Torigoe T, Tamura Y, Sato N. Heat Shock Proteins and Immunity: Application of Hyperthermia for Immunomodulation. Int J Hyperthermia (2009) 25:610–6. doi: 10.3109/02656730903315831
228. Qiao Y, Liu B, Li Z. Activation of NK Cells by Extracellularheat Shock Protein 70 Through Induction of NKG2D Ligands on Dendriticcells. Cancer Immun J Acad Cancer Immunol (2008) 8:12.
229. Shah TA, Pallera HK, Kaszowski CL, Bass WT, Lattanzio FA. Therapeutic Hypothermia Inhibits the Classical Complement Pathway in a Rat Model of Neonatal Hypoxic-Ischemic Encephalopathy. Front Neurosci (2021) 15:616734/BIBTEX. doi: 10.3389/FNINS.2021.616734/BIBTEX
230. Miklavcic D, Novickij V, Kranjc M, Polajzer T, Haberl Meglic S, Batista Napotnik T, et al. Contactless Electroporation Induced by High Intensity Pulsed Electromagnetic Fields via Distributed Nanoelectrodes. Bioelectrochemistry (2020) 132:107440. doi: 10.1016/J.BIOELECHEM.2019.107440
231. Rachakatla RS, Balivada S, Seo GM, Myers CB, Wang H, Samarakoon TN, et al. Attenuation of Mouse Melanoma by a/C Magnetic Field After Delivery of Bi-Magnetic Nanoparticles by Neural Progenitor Cells. ACS Nano (2010) 4:7093–104. doi: 10.1021/NN100870Z/SUPPL_FILE/NN100870Z_SI_001.PDF
232. Kimsa-Dudek M, Krawczyk A, Synowiec-Wojtarowicz A, Dudek S, Pawłowska-Góral K. The Impact of the Co-Exposure of Melanoma Cells to Chlorogenic Acid and a Moderate-Strength Static Magnetic Field. J Food Biochem (2020) 44:e13512. doi: 10.1111/JFBC.13512
233. Novickij V, Grainys A, Lastauskiene E, Kananavieiùte R, Pamedytyte D, Kalediene L, et al. Pulsed Electromagnetic Field Assisted In Vitro Electroporation: A Pilot Study. Sci Rep (2016) 6:1–10. doi: 10.1038/srep33537
234. García-Hevia L, Casafont Í., Oliveira J, Terán N, Fanarraga ML, Gallo J, et al. Magnetic Lipid Nanovehicles Synergize the Controlled Thermal Release of Chemotherapeutics With Magnetic Ablation While Enabling non-Invasive Monitoring by MRI for Melanoma Theranostics. Bioact Mater (2022) 8:153–64. doi: 10.1016/J.BIOACTMAT.2021.06.009
235. Liang C, Xie J, Luo S, Huang C, Zhang Q, Huang H, et al. A Highly Potent Ruthenium(II)-Sonosensitizer and Sonocatalyst for In Vivo Sonotherapy. Nat Commun (2021) 12:1–9. doi: 10.1038/s41467-021-25303-1
236. Li Y, Zhou Q, Deng Z, Pan M, Liu X, Wu J, et al. IR-780 Dye as a Sonosensitizer for Sonodynamic Therapy of Breast Tumor. Sci Rep (2016) 6:1–10. doi: 10.1038/srep25968
237. Zhang C, Xie Y, Luo X, Ji Q, Lu C, He C, et al. Effects of Therapeutic Ultrasound on Pain, Physical Functions and Safety Outcomes in Patients With Knee Osteoarthritis: A Systematic Review and Meta-Analysis. Clin Rehabil (2016) 30:960–71. doi: 10.1177/0269215515609415
238. Wan GY, Liu Y, Chen BW, Liu YY, Wang YS, Zhang N. Recent Advances of Sonodynamic Therapy in Cancer Treatment. Cancer Biol Med (2016) 13:325. doi: 10.20892/J.ISSN.2095-3941.2016.0068
239. Suslick KS, Flannigan DJ. Inside a Collapsing Bubble: Sonoluminescence and the Conditions During Cavitation. Annual Review Physical Chem (2007) 59(1): 659–683. doi: 10.1146/annurev.physchem.59.032607.093739
240. Beguin E, Shrivastava S, Dezhkunov NV, Mchale AP, Callan JF, Stride E. Direct Evidence of Multibubble Sonoluminescence Using Therapeutic Ultrasound and Microbubbles. ACS Appl Mater Interfaces (2019) 11:19913–9. doi: 10.1021/ACSAMI.9B07084/SUPPL_FILE/AM9B07084_SI_001.PDF
241. PENG CT. CHEMILUMINESCENCE. Liq. Scintill (1976), 313–29. doi: 10.1016/B978-0-12-522350-8.50033-7
242. Warnez M, Vlaisavljevich E, Xu Z. Damage Mechanisms for Ultrasound-Induced Cavitation in Tissue ARTICLES YOU MAY BE INTERESTED in. AIP Conference Proceedings (2017) 1821:80004. doi: 10.1063/1.4977634
243. Fu J, Li T, Zhu Y, Hao Y. Ultrasound-Activated Oxygen and ROS Generation Nanosystem Systematically Modulates Tumor Microenvironment and Sensitizes Sonodynamic Therapy for Hypoxic Solid Tumors. Adv Funct Mater (2019) 29:1906195. doi: 10.1002/ADFM.201906195
244. Su X, Wang P, Yang S, Zhang K, Liu Q, Wang X. Sonodynamic Therapy Induces the Interplay Between Apoptosis and Autophagy in K562 Cells Through ROS. Int J Biochem Cell Biol (2015) 60:82–92. doi: 10.1016/J.BIOCEL.2014.12.023
245. Li X, Zhang X, Zheng L, Kou J, Zhong Z, Jiang Y, et al. Hypericin-Mediated Sonodynamic Therapy Induces Autophagy and Decreases Lipids in THP-1 Macrophage by Promoting ROS-Dependent Nuclear Translocation of TFEB. Cell Death Dis (2016) 7:e2527–7. doi: 10.1038/cddis.2016.433
246. Zhou H, Sun J, Wu J, Wei H, Zhou X. Biodegradable Nanosonosensitizers With the Multiple Modulation of Tumor Microenvironment for Enhanced Sonodynamic Therapy. Int J Nanomedicine (2021) 16:2633–46. doi: 10.2147/IJN.S297571
247. Zhu W, Chen Q, Jin Q, Chao Y, Sun L, Han X, et al. Sonodynamic Therapy With Immune Modulatable Two-Dimensional Coordination Nanosheets for Enhanced Anti-Tumor Immunotherapy. Nano Res (2020) 14:212–21. doi: 10.1007/S12274-020-3070-8
248. Nam GH, Pahk KJ, Jeon S, Park HJ, Kim GB, Oh SJ, et al. Investigation of the Potential Immunological Effects of Boiling Histotripsy for Cancer Treatment. Adv Ther (2020) 3:1900214. doi: 10.1002/ADTP.201900214
249. van den Bijgaart RJE, Eikelenboom DC, Hoogenboom M, Fütterer JJ, den Brok MH, Adema GJ. Thermal and Mechanical High-Intensity Focused Ultrasound: Perspectives on Tumor Ablation, Immune Effects and Combination Strategies. Cancer Immunol Immunother (2017) 66:247–58. doi: 10.1007/S00262-016-1891-9
250. Fite BZ, Wang J, Kare AJ, Ilovitsh A, Chavez M, Ilovitsh T, et al. Immune Modulation Resulting From MR-Guided High Intensity Focused Ultrasound in a Model of Murine Breast Cancer. Sci Rep (2021) 11:927. doi: 10.1038/S41598-020-80135-1
251. Ji C, Si J, Xu Y, Zhang W, Yang Y, He X, et al. Mitochondria-Targeted and Ultrasound-Responsive Nanoparticles for Oxygen and Nitric Oxide Codelivery to Reverse Immunosuppression and Enhance Sonodynamic Therapy for Immune Activation. Theranostics (2021) 11(17): 8587–8604. doi: 10.7150/THNO.62572
252. Wawryka P, Kiełbik A, Iwanek G. Microbubble Based Sonoporation — From the Basics Into Clinical Implications. Med Res J (2019) 4:178–83. doi: 10.5603/MRJ.A2019.0032
253. Bernardes N, Fialho AM. Perturbing the Dynamics and Organization of Cell Membrane Components: A New Paradigm for Cancer-Targeted Therapies. Int J Mol Sci (2018) 19:3871. doi: 10.3390/ijms19123871
254. Dewitte H, Van Lint S, Heirman C, Thielemans K, De Smedt SC, Breckpot K, et al. The Potential of Antigen and TriMix Sonoporation Using mRNA-Loaded Microbubbles for Ultrasound-Triggered Cancer Immunotherapy. J Control Release (2014) 194:28–36. doi: 10.1016/J.JCONREL.2014.08.011
255. Karki A, Giddings E, Carreras A, Champagne D, Fortner K, Rincon M, et al. Sonoporation as an Approach for siRNA Delivery Into T Cells. Ultrasound Med Biol (2019) 45:3222–31. doi: 10.1016/J.ULTRASMEDBIO.2019.06.406
256. Hatle KM, Neveu W, Dienz O, Rymarchyk S, Barrantes R, Hale S, et al. Methylation-Controlled J Protein Promotes C-Jun Degradation To Prevent ABCB1 Transporter Expression. Mol Cell Biol (2007) 27:2952. doi: 10.1128/MCB.01804-06
257. Png YT, Vinanica N, Kamiya T, Shimasaki N, Coustan-Smith E, Campana D. Blockade of CD7 Expression in T Cells for Effective Chimeric Antigen Receptor Targeting of T-Cell Malignancies. Blood Adv (2017) 1:2348–60. doi: 10.1182/bloodadvances.2017009928
258. Bagarazzi ML, Yan J, Morrow MP, Shen X, Parker RL, Lee JC, et al. Immunotherapy Against HPV16/18 Generates Potent TH1 and Cytotoxic Cellular Immune Responses. Sci Transl Med (2012) 4(155):155ra138. doi: 10.1126/SCITRANSLMED.3004414
259. Yao X, Ahmadzadeh M, Lu YC, Liewehr DJ, Dudley ME, Liu F, et al. Levels of Peripheral CD4(+)FoxP3(+) Regulatory T Cells are Negatively Associated With Clinical Response to Adoptive Immunotherapy of Human Cancer. Blood (2012) 119:5688–96. doi: 10.1182/BLOOD-2011-10-386482
260. Kowolik CM, Topp MS, Gonzalez S, Pfeiffer T, Olivares S, Gonzalez N, et al. CD28 Costimulation Provided Through a CD19-Specific Chimeric Antigen Receptor Enhances In Vivo Persistence and Antitumor Efficacy of Adoptively Transferred T Cells. Cancer Res (2006) 66:10995–1004. doi: 10.1158/0008-5472.CAN-06-0160
261. Treon SP, Pilarski LM, Belch AR, Kelliher A, Preffer FI, Shima Y, et al. CD20-Directed Serotherapy in Patients With Multiple Myeloma: Biologic Considerations and Therapeutic Applications. J Immunother (2002) 25:72–81. doi: 10.1097/00002371-200201000-00008
262. Gutierrez R, Shah PD, Hamid O, Garfall AL, Posey A, Bishop MR, et al. Phase I Experience With First in Class TnMUC1 Targeted Chimeric Antigen Receptor T-Cells in Patients With Advanced TnMUC1 Positive Solid Tumors. J Clin Oncol (2021) 39:e14513–3. doi: 10.1200/JCO.2021.39.15_SUPPL.E14513
263. Pang N, Shi J, Qin L, Chen A, Tang Y, Yang H, et al. IL-7 and CCL19-Secreting CAR-T Cell Therapy for Tumors With Positive Glypican-3 or Mesothelin. J Hematol Oncol (2021) 14:1–6. doi: 10.1186/S13045-021-01128-9/FIGURES/2
264. Patnaik A, Kang SP, Rasco D, Papadopoulos KP, Elassaiss-Schaap J, Beeram M, et al. Phase I Study of Pembrolizumab (MK-3475; Anti–PD-1 Monoclonal Antibody) in Patients With Advanced Solid Tumors. Clin Cancer Res (2015) 21:4286–93. doi: 10.1158/1078-0432.CCR-14-2607
265. Ali SA, Shi V, Maric I, Wang M, Stroncek DF, Rose JJ, et al. T Cells Expressing an Anti-B-Cell Maturation Antigen Chimeric Antigen Receptor Cause Remissions of Multiple Myeloma. Blood (2016) 128:1688–700. doi: 10.1182/BLOOD-2016-04-711903
266. Maude SL, Frey N, Shaw PA, Aplenc R, Barrett DM, Bunin NJ, et al. Chimeric Antigen Receptor T Cells for Sustained Remissions in Leukemia. N Engl J Med (2014) 371:1507–17. doi: 10.1056/NEJMOA1407222
267. Frey NV, Shaw PA, Hexner EO, Pequignot E, Gill S, Luger SM, et al. Optimizing Chimeric Antigen Receptor T-Cell Therapy for Adults With Acute Lymphoblastic Leukemia. J Clin Oncol (2020) 38:415–22. doi: 10.1200/JCO.19.01892
268. Fry TJ, Shah NN, Orentas RJ, Stetler-Stevenson M, Yuan CM, Ramakrishna S, et al. CD22-Targeted CAR T Cells Induce Remission in B-ALL That is Naive or Resistant to CD19-Targeted CAR Immunotherapy. Nat Med (2018) 24:20–8. doi: 10.1038/NM.4441
269. Park JH, Rivière I, Gonen M, Wang X, Sénéchal B, Curran KJ, et al. Long-Term Follow-Up of CD19 CAR Therapy in Acute Lymphoblastic Leukemia. N Engl J Med (2018) 378:449–59. doi: 10.1056/NEJMOA1709919/SUPPL_FILE/NEJMOA1709919_DISCLOSURES.PDF
270. Lu Y-C, Parker L, Lu T, Zheng Z, Yao X, Robbins PF, et al. A Phase I Study of an HLA-DPB1*0401-Restricted T Cell Receptor Targeting MAGE-A3 for Patients With Metastatic Cancers. J Immunother Cancer (2015) 3:P158. doi: 10.1186/2051-1426-3-S2-P158
271. Gatti-Mays ME, Redman JM, Donahue RN, Palena C, Madan RA, Karzai F, et al. A Phase I Trial Using a Multitargeted Recombinant Adenovirus 5 (CEA/MUC1/Brachyury)-Based Immunotherapy Vaccine Regimen in Patients With Advanced Cancer. Oncologist (2020) 25:479. doi: 10.1634/THEONCOLOGIST.2019-0608
272. Rapoport AP, Aqui NA, Stadtmauer EA, Vogl DT, Xu YY, Kalos M, et al. Combination Immunotherapy After ASCT for Multiple Myeloma Using MAGE-A3/Poly-ICLC Immunizations Followed by Adoptive Transfer of Vaccine-Primed and Costimulated Autologous T Cells. Clin Cancer Res (2014) 20:1355. doi: 10.1158/1078-0432.CCR-13-2817
273. Beatty GL, Chiorean EG, Fishman MP, Saboury B, Teitelbaum UR, Sun W, et al. CD40 Agonists Alter Tumor Stroma and Show Efficacy Against Pancreatic Carcinoma in Mice and Humans. Science (2011) 331:1612–6. doi: 10.1126/SCIENCE.1198443
274. Straathof K, Flutter B, Wallace R, Jain N, Loka T, Depani S, et al. Antitumor Activity Without on-Target Off-Tumor Toxicity of GD2-Chimeric Antigen Receptor T Cells in Patients With Neuroblastoma. Sci Transl Med (2020) 12(571): eabd6169. doi: 10.1126/SCITRANSLMED.ABD6169
275. Hinrichs CS, Doran SL, Stevanovic S, Adhikary S, Mojadidi M, Kwong ML, et al. A Phase I/II Clinical Trial of E6 T-Cell Receptor Gene Therapy for Human Papillomavirus (HPV)-Associated Epithelial Cancers. (2017) 35(15_suppl):3009–3009. doi: 10.1200/JCO.2017.35.15_SUPPL.3009
276. Legat A, Maby-El Hajjami H, Baumgaertner P, Cagnon L, Maillard SA, Geldhof C, et al. Vaccination With LAG-3ig (IMP321) and Peptides Induces Specific CD4 and CD8 T-Cell Responses in Metastatic Melanoma Patients–Report of a Phase I/IIa Clinical Trial. Clin Cancer Res (2016) 22:1330–40. doi: 10.1158/1078-0432.CCR-15-1212
Keywords: antigens, membrane, modulation, anticancer therapy, cancer
Citation: Szlasa W, Janicka N, Sauer N, Michel O, Nowak B, Saczko J and Kulbacka J (2022) Chemotherapy and Physical Therapeutics Modulate Antigens on Cancer Cells. Front. Immunol. 13:889950. doi: 10.3389/fimmu.2022.889950
Received: 16 March 2022; Accepted: 06 June 2022;
Published: 06 July 2022.
Edited by:
Catherine Sautes-Fridman, U1138 Centre de Recherche des Cordeliers (CRC) (INSERM), FranceReviewed by:
Gabriele Multhoff, Technical University of Munich, GermanyPramod Darvin, Rajiv Gandhi Centre for Biotechnology, India
Copyright © 2022 Szlasa, Janicka, Sauer, Michel, Nowak, Saczko and Kulbacka. This is an open-access article distributed under the terms of the Creative Commons Attribution License (CC BY). The use, distribution or reproduction in other forums is permitted, provided the original author(s) and the copyright owner(s) are credited and that the original publication in this journal is cited, in accordance with accepted academic practice. No use, distribution or reproduction is permitted which does not comply with these terms.
*Correspondence: Wojciech Szlasa, wojciech.szlasa@outlook.com