- 1Department of General Surgery, The First Affiliated Hospital of Dalian Medical University, Dalian, China
- 2Institute (College) of Integrative Medicine, Dalian Medical University, Dalian, China
- 3Laboratory of Integrative Medicine, The First Affiliated Hospital of Dalian Medical University, Dalian, China
- 4Department of Traditional Chinese Medicine, The Second Affiliated Hospital of Dalian Medical University, Dalian, China
- 5Department of Molecular Diagnostics and Experimental Therapeutics, Beckman Research Institute of City of Hope Comprehensive Cancer Center, Duarte, CA, United States
Severe acute pancreatitis (SAP), one of the most serious abdominal emergencies in general surgery, is characterized by acute and rapid onset as well as high mortality, which often leads to multiple organ failure (MOF). Acute lung injury (ALI), the earliest accompanied organ dysfunction, is the most common cause of death in patients following the SAP onset. The exact pathogenesis of ALI during SAP, however, remains unclear. In recent years, advances in the microbiota-gut-lung axis have led to a better understanding of SAP-associated lung injury (PALI). In addition, the bidirectional communications between intestinal microbes and the lung are becoming more apparent. This paper aims to review the mechanisms of an imbalanced intestinal microbiota contributing to the development of PALI, which is mediated by the disruption of physical, chemical, and immune barriers in the intestine, promotes bacterial translocation, and results in the activation of abnormal immune responses in severe pancreatitis. The pathogen-associated molecular patterns (PAMPs) mediated immunol mechanisms in the occurrence of PALI via binding with pattern recognition receptors (PRRs) through the microbiota-gut-lung axis are focused in this study. Moreover, the potential therapeutic strategies for alleviating PALI by regulating the composition or the function of the intestinal microbiota are discussed in this review. The aim of this study is to provide new ideas and therapeutic tools for PALI patients.
Introduction
Severe acute pancreatitis (SAP) is a serious abdominal disease with multiple complications and high mortality rates with an average annual incidence of about 6.75 cases per 100,000 people worldwide. Systemic inflammatory response syndrome (SIRS), multiple organ dysfunction syndromes (MODS), and sepsis are frequently associated with it (1, 2). Acute lung injury (ALI), the most common and earliest organ dysfunction during SAP, is the leading cause of death in SAP patients, particularly in elderly SAP patients (60%-70%) (3–5). Multi-mechanisms mediated crosstalk among the pancreatic necrosis, bacteremia, intestinal barrier failure, etc. are the reported reasons for the pathological process of SAP-associated lung injury (PALI) (6). However, the pathogenesis of PALI has not been fully elucidated to date, partly attributed to the lack of effective treatment strategies as well as a longer treatment cycle, unsatisfying prognosis, and economic burden of the disease (7, 8).
In recent years, with the in-depth research in terms of the gut microbiota, their critical roles in holding the intestinal immune barrier, and the defense functions are increasingly revealed. In PALI, the molecular mechanisms by which the gut microbiota mediate PALI have become a hot topic (9–11). Trillions of microorganisms (including bacteria, fungi, and viruses) are present in the host intestine, and they perform a variety of regulatory effects on the physiological activities through their metabolites, or the signaling pathways (axis), symbiotically co-existing with the host (12). A microecological balance is achieved between host and microorganisms under a healthy state, which is referred to as homeostasis, and normal physiological functions are maintained in the host. However, external factors (such as infection, toxins, dietary changes, disease, etc.) might alter or disrupt the balance between them and the disturbance of intestinal microbiota will in turn induce or accelerate disease progression (13).
Studies on the microbiota-gut-lung axis have enhanced our understanding of PALI, and current research has pointed out that bidirectional communication exists between the lung and gut microbiota, with intestinal microorganisms and their metabolites playing key roles (14). Moreover, opportunistic pathogenic microbes in the intestine and their PAMPs are metronomes in the pathogenesis of ALI (15). Intestinal microbes are prospective targets in the treatment and control of PALI.
However, to the best of our knowledge, reviews on the involvement of intestinal microbiota in the pathogenies of PALI are lacking. In this paper, we first describe the structural and species alterations of the intestinal microbiota during SAP, which causes intestinal barrier injury, including physical, chemical, and immune barrier disruptions. Then, the possible pathways of barrier injury-induced bacterial translocation under SAP, and the mechanisms of bacterial translocation-associated ALI, are discussed. Specifically, the pathogen-associated molecular patterns (PAMPs) mediated immunol mechanisms in the occurrence of PALI by binding with pattern recognition receptors (PRRs) via the microbiota-gut-lung axis are focused. The diagram of summary contents of this paper is illustrated in Figure 1. Finally, this paper summarizes the prospective intervention strategies for the treatment of PALI mediated by the regulation of intestinal microbiota as well, which is expected to provide references in clinical practice in the future.
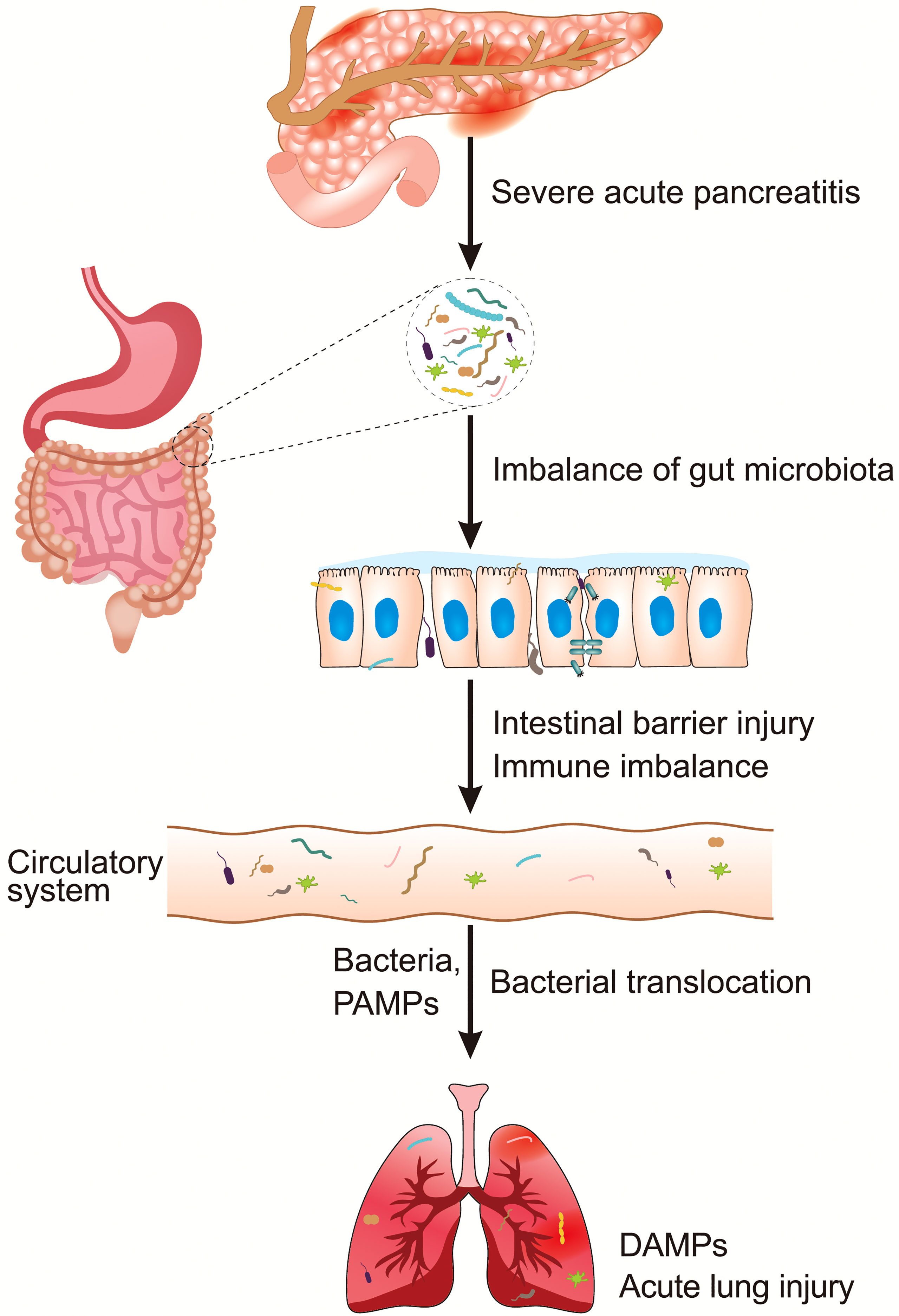
Figure 1 An overview of intestinal microbiota dysbiosis in PALI. DAMPs, damage-associated molecular patterns; PALI, severe acute pancreatitis-associated acute lung injury; PAMPs, pathogen-associated molecular patterns.
Dysbiosis of Intestinal Microbiota in SAP
With the development of high-throughput sequencing techniques, several studies have proven that the abundance, as well as the diversity, of the intestinal microbiota are significantly altered during SAP (Table 1). The diversity of intestinal microbiota is reduced in SAP patients in comparison to the healthy controls, indicating a tendency of obligate anaerobic bacteria to be replaced by facultative anaerobic bacteria as the dominant bacteria in the intestine. At the phylum level, the abundance of Proteobacteria and Actinobacteria is elevated in the intestine of SAP patients, whereas the abundance of Firmicutes and Bacteroides is decreased. At the genus level, SAP patients show a significant increase in the abundance of facultative anaerobic bacteria in the intestine, such as Escherichia, Enterococcus, Enterobacter, Streptococcus, etc., with a decrease in Bacteroides, Bifidobacterium, and Blautia, etc., and the SAP animal models show similar results (2, 16–18, 20, 21). Furthermore, analyses of the bacterial community reveal that the most noticeable differences in species abundance are located in the cecum and colon (24).
Dysbiosis of Gut Microbiota Damages the Intestinal Barrier
The 100 trillion microorganisms in the gut are separated from the host by physical, chemical, and immune barriers of the intestine (25). Current studies have pointed out that the damage to the intestinal barrier integrity caused by the dysfunction of intestinal microecology during SAP primarily contributes to the development of PALI (26–30). The diagram depicting the mechanism is summarized in Figure 2.
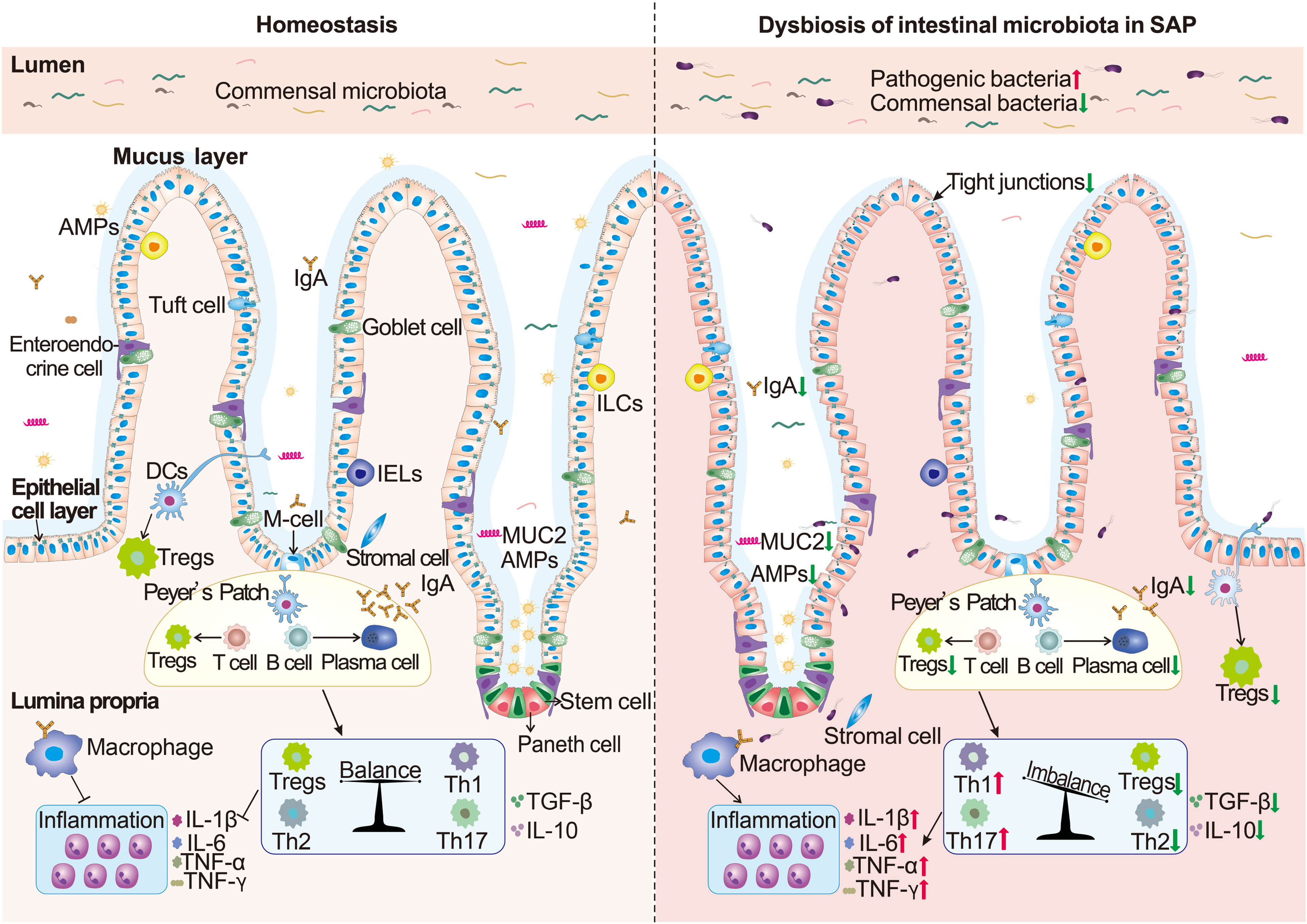
Figure 2 The mechanisms of intestinal barrier disruption mediated by intestinal bacterial dysbiosis. The increase of intestinal pathogenic bacteria and the decrease of commensal metabolites in severe acute pancreatitis (SAP) jointly lead to the thinning of the intestinal mucus layer and the decrease of antimicrobial peptides (AMPs), mucin2 (MUC2), and IgA (SIgA) secretion, resulting in direct contact of pathogenic bacteria with the intestinal epithelial cells (IECs) and an “inflammatory storm”. The abnormal immune responses further lead to IEC damage, slow renewal, and disruption of the tight junctions (TJs) in epithelial cells, further stimulating excessive pro-inflammatory factors on the intestinal mucosal immune system. Finally, the integrity of the intestinal barrier is destroyed, bacteria and products translocate to the lungs, and then PALI ensues.
Intestinal Chemical Barrier Integrity
The chemical barrier in the intestine is mainly composed of the mucus layer on the surface of the intestinal epithelium, which contains digestive fluids (gastric acid, intestinal fluid, bile, etc.) and mucus secreted from goblet cells (GCs), and the antimicrobial peptides (AMPs) that are secreted from paneth cells (PCs) in intestinal epithelial cells (IECs) (31, 32). The intestinal mucus layer develops the first line defense of the intestine against pathogens (33–35). The secretion of mucus is regulated by microbes as well. As a case in point, the obligate anaerobic bacteria could induce the secretion of AMPs and raise the density of GCs and the production of mucin 2 through their metabolites (acetic acid) in order to strengthen the solidity of the barrier (36).
In the early stages of SAP, the mucus layer begins to disrupt, and it is observed that the mRNA levels of lysozyme and α-defensin are reduced (37–39). Increased colonization of opportunistic pathogenic bacteria in the ileum, such as Escherichia coli, Streptococcus, Proteus, and Enterococcus, could lead to a reduction in AMPs and the possibility of pathogenic bacteria directly penetrating the mucus layer. Then, the bacterial translocation (BT) in the intestine (especially the ileum) will lead to injury of the intestinal epithelial cells (IECs) (17). With the progress of SAP, large amounts of Ca2+ and fatty acids (butyric acid) are consumed, resulting in a higher pH environment in the intestine, which inhibits the survival of probiotics, leading to a further reduction in the repairment of chemical barrier integrity (31, 40, 41).
Intestinal Physical Barrier Integrity
The physical barrier of the intestine is made up of a layer of IECs that includes the columnar epithelium and cell gap junction (31). The major IECs, namely enterocytes, GCs, PCs, and enteroendocrine cells, are differentiated from the progenitor cells derived from the Lgr5+ intestinal stem cells located in the Lieberkühn crypt, and IECs could be updated every 3-5 days (42). The physical barrier separates the bacteria from the lamina propria immune cells and performs sensory/defense functions or immune tolerance through PRRs mediated by PAMPs on the microbial surface (43, 44). Cell junctions include tight junctions (TJs), adhesion junctions, and desmosomes, with TJs being the main mode, including claudin, occludin, tricellulin, zonula occludens-1 (ZO-1), etc., and they form an osmotic barrier, close the gap between IECs, and maintain the polarity of cells (45).
The disruption of the intestinal physical barrier during SAP is primarily attributed to an imbalance in the anaerobic bacteria with pathogenic bacteria as the dominant bacteria. Lactobacillus reuteri, the dominant anaerobic bacterium in the gut, can stimulate the proliferation and differentiation of Lgr5+ cells, reducing the levels of proinflammatory cytokines, serum lipopolysaccharide (LPS), and peptidoglycan, and restoring damaged intestinal epithelia. In addition, the abundance of Bifidobacterium in the intestine is positively correlated with the expression of occludin, claudin-1, and ZO-1 in TJs (46, 47). When the dysbiosis of microorganisms occurs, reduction in the dominant anaerobic bacteria causes TJs injury and increased permeability in the intestine. Meanwhile, the increased pathogenic bacteria will activate PAMPs, leading to a persistent and overwhelming “inflammatory storm” on the intestinal cell surface through the activation of mitogen-activated protein kinase (MAPK) or nuclear factor-kappa B (NF-κB) pathways, resulting in the necrosis of epithelial cells and the shedding of TJs. Then, the intestinal pathogens in the lamina propria could activate the damage-associated molecular patterns (DAMPs), which cause abnormal immune responses in the intestine (48–50).
Intestinal Immune Barrier Integrity
IECs, intestinal intraepithelial lymphocytes (IELs), lamina propria lymphocytes (LPLs), Peyer patch (PP), mesenteric lymph nodes (MLNs), as well as secretory IgA (SIgA) from plasma cells make up the intestinal immune barrier (25, 51). As the last line of defense against pathogenic bacteria, the intestinal immune system maintains a delicate dynamic balance with the commensal bacteria (52).
The intestinal microbiota has been found to be a central hub for the development and regulation of the host’s innate and adaptive immune system (53). Germ-free (GF) mice have severe immunodeficiencies, manifested mainly in the reduction of gut-associated lymphoid tissues (GALTs), Th17 cells, regulatory T cells (Tregs), plasma cells, and epithelial CD8+ T cells (54). Bacterooides fragilis, Lactobacilli, and Bacteroides thetaiotaomicron, etc., could directly modulate Tregs, Th1 cells, Th17 cells, plasma cells, and intervene in the intestinal immune homeostasis (55–57). During SAP, an increase in Salmonella, Escherichia coli, Enterococcus, and Proteus could promote the secretion of pro-inflammatory cytokines (IL-1, IL-6, TNF-α, IFN-γ, etc.) by dendritic cells (DCs) and macrophages, mediated by PAMPs, resulting in the differentiation of immature T cells into Th1/Th17 cells and the subsequent immune responses (58). Moreover, the proliferation of pathogenic bacteria exerts an inhibitory effect on Tregs, Th2 cells, and B cells, which causes a decrease in the secretion of SIgA and anti-inflammatory cytokines (IL-10/TGF-β), and results in a continuous “pathological” activation of immune responses and a “suicidal” attack in the intestine (58–60).
Injury of Barrier Functions by Changes in Bacterial Metabolites
Numerous studies have demonstrated that bacterial metabolites are involved in the functions of the intestinal barrier (61). For instance, short-chain fatty acids (SCFAs), generated by Bifidobacterium, Bacteroides, Brucella, and Clostridium, can enhance the physical and immune barriers of the gut in a variety of ways, such as inhibiting the proliferation of harmful bacteria, promoting the recovery of IECs, enhancing the protein expression of TJs, and maintaining host immune homeostasis, etc., thereby making them the “loyal guardians” (62, 63). Furthermore, deoxycholic acid (DCA), a secondary bile acid metabolite produced by Bacteroides, enhances the gut physical barrier by inhibiting the renewal and repair of IECs by activating Farnesoid X receptor (FXR) (64). Indole-3-ethanol, a metabolite of tryptophan produced by Lactobacillus, boosts the function of TJs by binding the arylhydrocarbon receptor (AhR) (65). Trimethylamine N-oxide (TMAO) formed from dietary choline promotes the maturation and secretion of IL-1β and IL-18 mediated by nucleotide-binding oligomerization domain-like receptors (NLRs), consequently regulating intestinal inflammation (66).
Pathways of Bacterial Translocation to the Lung
It is observed that certain bacteria from the lower gastrointestinal tract are enriched in SAP lungs and this phenomenon is referred to as bacterial translocation (BT) (67–69). BT is described as the process by which bacteria and/or their products pass through the intestinal barrier and enter the systemic circulation, tissues, or organs (such as lungs) by MLNs and the portal system, and is thought to be the primary reason for PALI, systemic sepsis, and MODS in patients with SAP (70). Also, lower levels of barrier proteins including occludin, ZO-1, and activation of the platelet-activating receptor (PAFr) are observed to be accompanied by BT (71, 72).
In recent years, high-throughput sequencing techniques have deepened our understanding of BT, the incidence of which is closely linked to the severity of SAP and the degree of bacterial imbalance (19, 73–75). Current studies have suggested that intestinal BT to the lung is primarily mediated by lymphatic and hematogenous dissemination, as summarized in Figure 3, and BT is proven to be a facilitator, rather than a trigger for PALI (76). The thoracic duct allows the mesenteric lymphatic fluid to enter the circulatory system, and the lungs are the first exposure points to those pathogens in the pulmonary circulation. Injecting mesenteric lymphatic fluid (from SAP rats) into the veins of healthy rats could trigger PALI, and mesenteric lymphatics ligation or accelerating its excretion can help alleviate inflammation symptoms in rat models (77–80). Moreover, danger-associated molecular patterns (DAMPs) released from lymphatics play a regulatory role in eliciting the ALI (81).
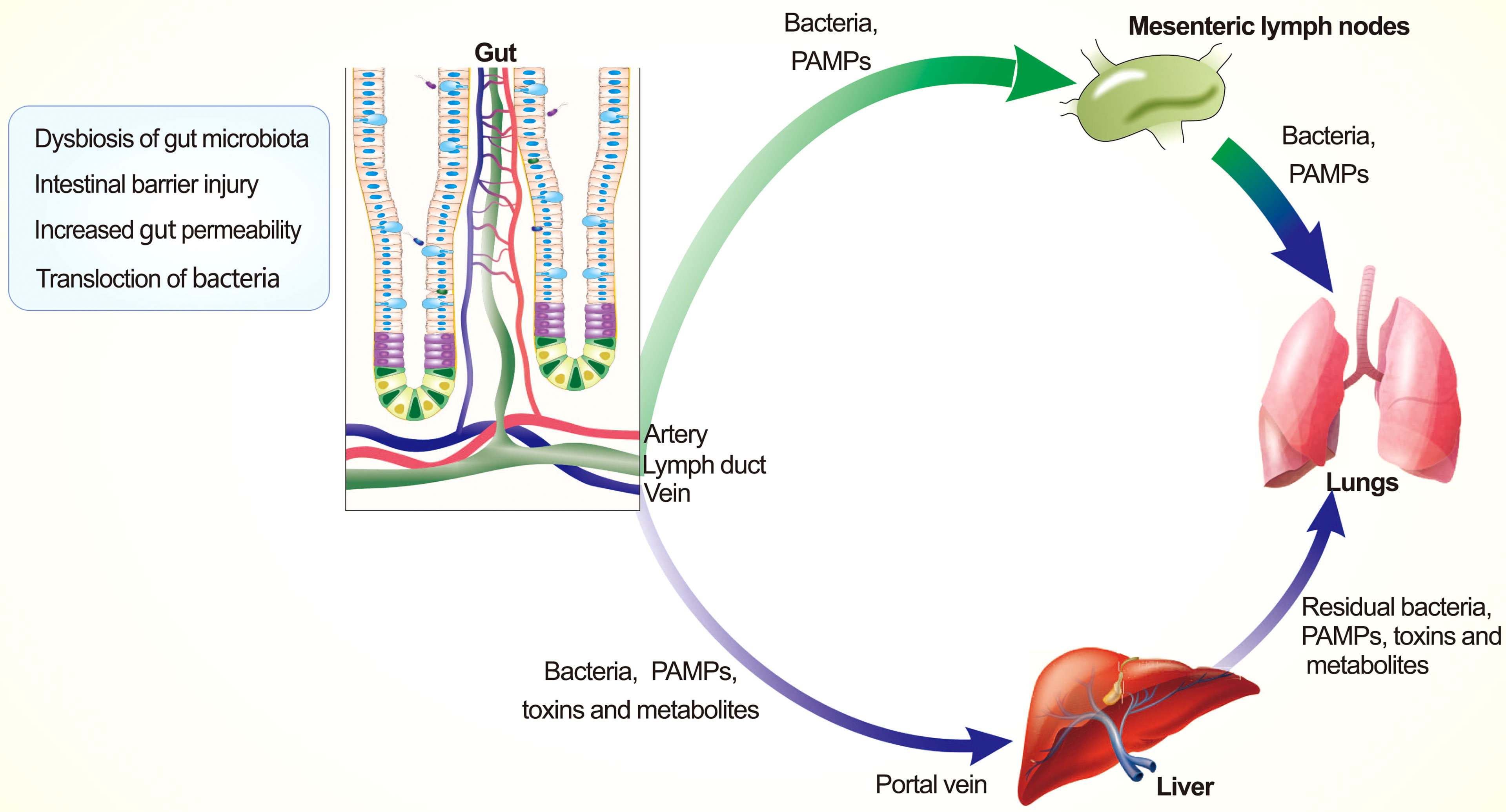
Figure 3 Pathways of intestinal bacterial translocation. Intestinal pathogenic bacteria, PAMPs, and metabolites translocate to the lung via lymphatic route and blood circulatory system.
In the early stage of SAP, the kupffer cell forms a “firewall” in the liver that is able to remove the majority of pathogens and PAMPs from the bloodstream. With the increase in pathogenic bacteria, the intestinal inflammatory storm is initiated, leading to the disordered immune system and impaired intestinal barrier integrity, which results in the accelerated shift of microbes and their PAMPs, and then the intestinal toxins enter the vena cava and induce PALI (82, 83). In the late stage of SAP, a substantial amount of ascites is produced, and the pathogenic bacteria and PAMPs will cause bacterial peritonitis following the “fermentation” in the peritoneal cavity and then enter the circulatory system via the mesenteric lymphatic system or visceral capillaries to cause ALI (84). However, the choice and timing of abdominal puncture drainage (APD) in SAP patients is controversial. Early APD is recommended as an effective adjuvant in SAP patients by the current American Gastroenterological Association (AGA) guidelines for the management of necrotizing pancreatitis (85). While some scholars claim that APD does not decrease the overall risks of mortality in SAP patients, the mechanisms of APD in PALI still need to be further investigated (86, 87).
Mechanisms of ALI Caused by Intestinal Bacterial Dysbiosis
From a phylogenetic viewpoint, both the gut and lungs develop from the endoderm and connect to the outside environment, where the epithelial barrier and microbiota synergistically work to protect against pathogens (88). The crosstalk between the gut microbiota and the lung is referred to as the gut-lung axis, and its bidirectional regulation is critical for maintaining the host immune homeostasis as well as dynamic balance. Soluble microbial components and their metabolites, which are transported along with the circulatory system, are key mediators by which the intestinal microbiota and the lungs communicate with each other (89). Disturbed intestinal microbiota during SAP could cause PALI through a variety of mechanisms, as shown in Figure 4.
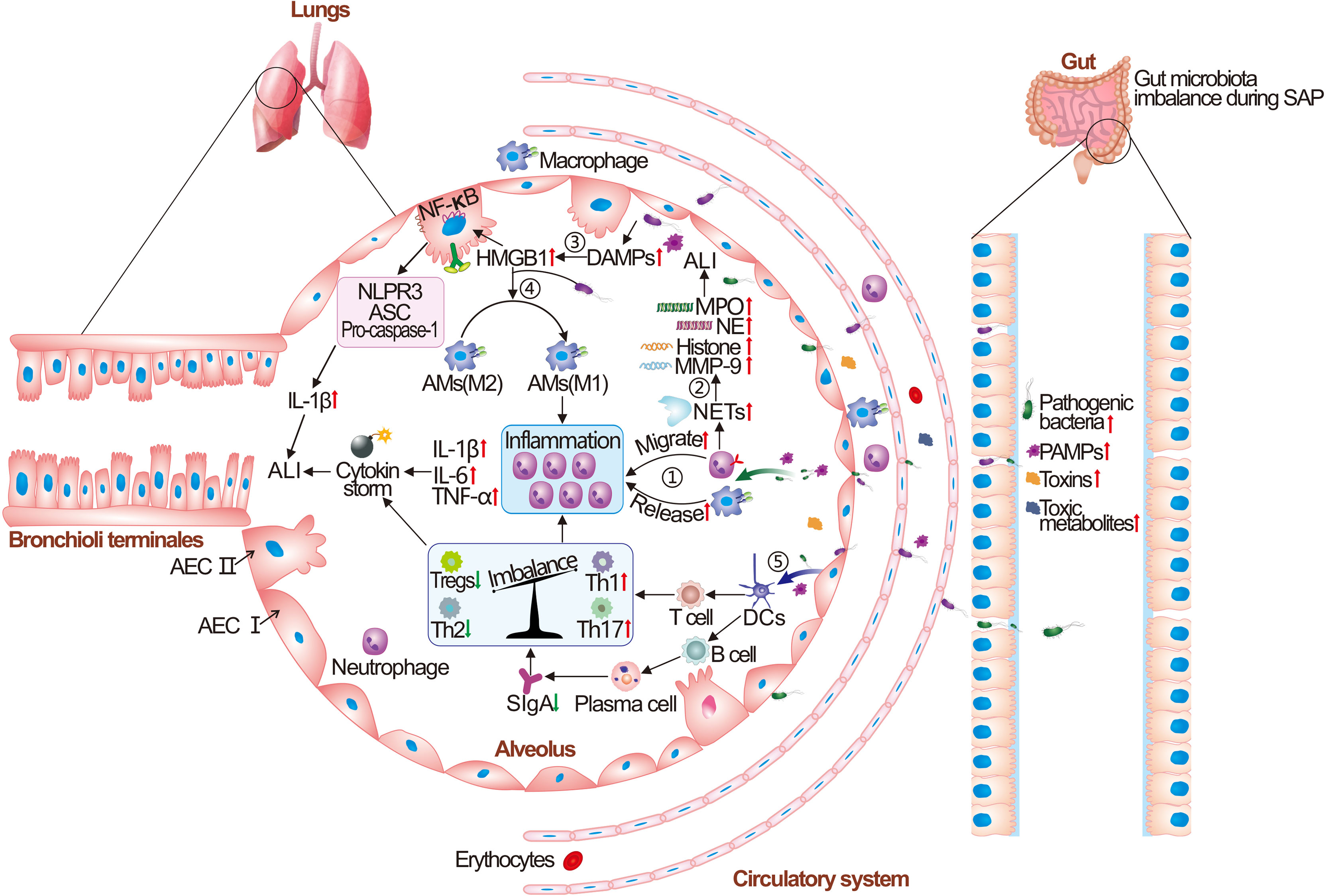
Figure 4 The pathogenesis of intestinal microbiota dysbiosis caused acute lung injury (ALI). Briefly, the intestinal pathogenic bacteria and harmful metabolites are translocated to the lung and recognized by pulmonary innate immune cells (AECs, AMs, DCs, and NK cells). Then, the release of persistent pro-inflammatory cytokines is induced by binding to their pattern recognition receptors (PRRs) on the cell surface, causing an “inflammatory storm”. A large number of neutrophil cells aggregate in the alveoli and neutrophil extracellular trap network (NETs) lead to increased release of neutrophil elastase (NE), myeloperoxidase (MPO), and histone. The inflammatory factors, granulins, and infiltrated red blood cells from vascular cause the damage and hemorrhage of lung tissue. Subsequently, the lung tissue initiates the immune responses of damage-associated molecular patterns (DAMPs), causing increased release of high mobility group protein B1 (HMGB1), the abnormal polarization of M1-type macrophages, and release of NOD-like receptor thermal protein domain associated protein 3 (NLRP3). Finally, lung tissue initiates an adaptive immune response, and increased production of pro-inflammatory Th1 and Th17 immune cells and suppression of Tregs cells could be observed, resulting in the paralysis of the immune system and irreversible ALI. AECs, alveolar epithelial cells; AMs, alveolar macrophages; DCs, dendritic cells; ASC, Apoptosis-associated speck-like protein containing a caspase recruit domain; SIgA, secretory IgA.
Intestinal Bacterial Translocation-Mediated PALI
The disruption of gut barrier integrity during SAP leads to the translocation of pathogenic bacteria and their PAMPs to lung tissue, which can directly cause the release of inflammatory cytokines (TNF-α, IL-1β, and IL-6) from lung monocytes and macrophages, and subsequently act on the lung capillaries, alveolar epithelial cells (AECs), and the fluid layer on the alveolar surface to cause extensive ALI. Then, the DAMPs could be initiated in the lung tissue that causes a cascade reaction, including the extracellular migration of pro-inflammatory factors such as high mobility group box-1 (HMGB1), heat shock proteins (HSPs), and their bindings to the receptor for advanced glycation end products (RAGE), Toll-like receptor2 (TLR-2), and Toll-like receptor4 (TLR-4) on the surface of AECs. It will create a positive feedback loop that further leads to the upregulation of necrosis, apoptosis, and edema in the lungs (90–92). Elevated HMGB1 levels in lung tissue and bronchoalveolar lavage fluid (BALF) are demonstrated to be positively correlated with the level of pro-inflammatory cytokines using the PALI mice model. The PALI mice model was established by giving them 4g/kg intraperitoneal injection of L-arginine every hour for 2 hours, and the underlying mechanisms are linked to the activation of the TLR4/MyD88/NF-κB pathway and release of NLRP3 inflammasomes (93–96).
Neutrophil extracellular traps (NETs) are additional reported mediators correlated with the severity of decease and microbial diversity in ALI patients (97). NETs are decompressed chromatin and encapsulated granule proteins containing net-like structures that are released outside the cell from neutrophils stimulated by bacteria, PAMPs, etc. (98). NETs have the capacity to trap bacteria and other pathogens, but excessive activation could lead to the PALI and MODS by disrupting the endothelial/epithelial cell integrity, the intercellular junctions, and the functions of macrophages in the lungs. Pulmonary capillary microthrombosis could be promoted as well, mediated by the influence on intercellular communication, upregulation of matrix metalloproteinase-9 (MMP-9) expression, and release of inflammatory factors (15, 99–103). Furthermore, the administration of HMGB1 could stimulate the endoplasmic reticulum stress (ERS) and induce PALI by stimulating the formation of NETs (104).
Alterations in the Functions of Alveolar Macrophages
AMs, which are widely distributed in the pulmonary mesenchyme, perform the functions of phagocytosis, chemotaxis, immunity, and secretion. They play important roles in the defense against pathogens that cause PALI. Macrophages are presented in alveoli and part of macrophages in the lung interstitium are differentiated from monocytes; both of them constitute the innate immune functions in the lung. In a healthy state, macrophages in the alveoli maintain normal immunological function, while in the case of an immune imbalance (such as SAP/PALI), some macrophages tend to polarize toward M1-type macrophages which leads to fewer M2-type macrophages and excessive inflammatory responses (in Figure 5). M1-type AMs may be able to regulate the inflammatory responses and tissue damage by recruiting and activating the neutrophils and mononuclear phagocytes through secreting pro-inflammatory cytokines. M2-type AMs could release inhibitory cytokines that mediate the anti-inflammatory effects and tissue repair. Abnormal differentiation of AMs to the M1-type might induce severe inflammatory responses as the “catalyst” in the pathogenesis of PALI (105–107). Gram-negative bacteria are regarded as M1-type AM inducers, and the translocation of intestinal bacteria (such as Escherichia coli and Shigella) during SAP could result in the polarization of AMs to M1 type (108, 109). M1-type AMs polarization is linked to abnormal activation of the TLR4/NF-κB pathway, and M2-type AMs polarization is regulated by the IL-4/peroxisome proliferators-activated receptor-γ (PPAR-γ) pathway (105). Apart from this, HMGB1 could trigger PALI by inducing the polarization of AMs to M1 type, the activation of inflammatory vesicles in AMs, and the regulation of the RAGE/NF-κB signaling pathway (110).
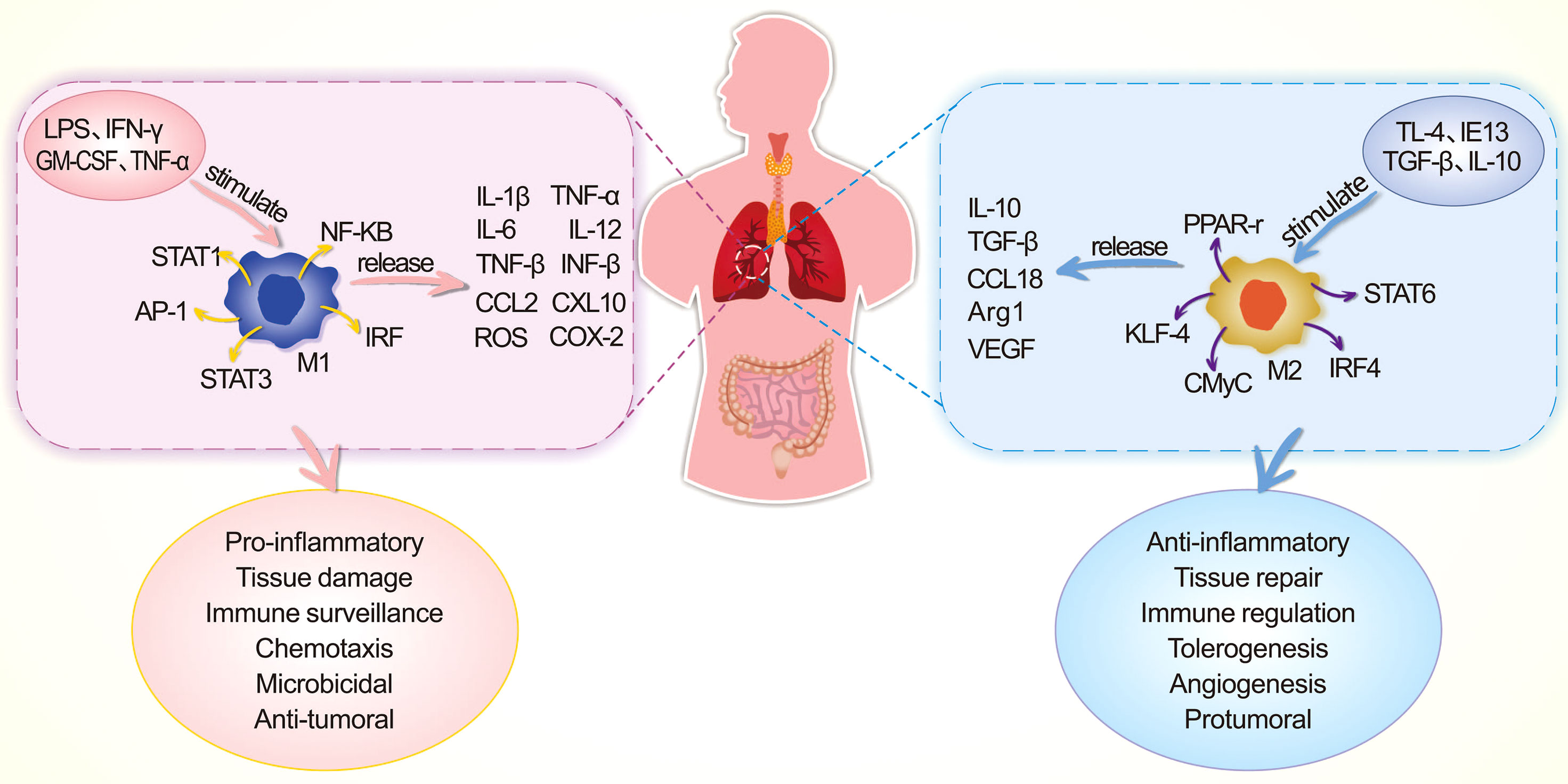
Figure 5 Comparison of M1-type macrophages and M2-type macrophages, in terms of stimulatory factors, transcription factors, released mediators, and functional properties during the process of PALI. AP-1, activating protein-1; ARG1, arginase 1; CMyC, transcription factor CMyC; GM-CSF, granulocyte-macrophage colony-stimulating factor; IFN-γ, interferon γ; IRF, interferon regulatory factor; KLF-4, krüppel-like factor 4; LPS, lipopolysaccharide; NF-κB, nuclear transcription factor κB; ROS, reactive oxygen species; STAT, signal transduction and activation of transcription factor; TGF-β, transforming growth factor ß; VEGF, vascular endothelial growth factor.
Pyroptosis is programmed cell death mediated by cysteinyl aspartate-specific proteinase-1 (caspase-1), which is associated with the progression of PALI, as well as a reduction in SCFAs producing bacteria in the intestine (111). The “waterfall” inflammatory responses during PALI stimulate the swelling, rupture, and perforation of AMs. Then, a large amount of pro-inflammatory factors could be released, and the pyroptosis program is initiated by the abnormal activation of MAPK (112). Besides, the severity of PALI is linked to the exosomes generated by lung cells. Neutrophil-derived exosome miR-30d-5p could induce the polarization of AMs to M1-type, leading to the pyroptosis of AMs via the NF-κB signaling pathway (113). In addition, extracellular vesicles (EVs) released by macrophages can promote the polarization of M1-type AMs, causing the injury to the lungs (114). In contrast, the AECs-derived exosome miR-27b-3p can impact AMs functions by targeting RGS1-mediated calcium signaling-dependent immune responses (115).
Activation of Abnormal Innate Immune Responses
Abnormal activation of the innate immune system in the lungs plays a decisive role in the pathogenesis of PALI (116). AECs, AMs, innate lymphoid cells (ILCs), natural killer cells (NKs), DCs, etc., of the pulmonary innate immune system can identify PAMPs and defend against the foreign pathogenic bacteria. Multiple targets are involved in the recognition of PAMPs (TLRs, NLRs, ALRs, etc.), which can result in the production of inflammatory factors, chemokines, interferons, reactive oxygen species (ROS), and others, thereby inducing the pro-inflammatory immunity (117). The increase in pathogen load or the release of “waterfall” inflammatory factors, in turn, stimulates the pulmonary adaptive immune system, in which T and B cells are activated and work together with the innate immune response in order to release SIgA, defensins, lysozyme, and complement (C3a, C5a), which then clear the pathogenic bacteria. Irreversible PALI is caused by the immune system paralysis for the imbalance between Th17 and Tregs, the increased antibody dependence-enhancement, the abnormal increase in complement, and the inactivation of innate immune cell function (116, 118, 119).
In addition, pathogenic bacteria-induced abnormal activation of DCs can increase the expression of intercellular cell adhesion molecule-1 (ICAM-1), and endothelial activation and abnormal immune responses are promoted by neutrophil infiltration, resulting in PALI (120). Facultative anaerobes can cause single amino acid nitration of Ras homolog gene family member A (RhoA) in PALI, resulting in the increased migration of immune cells along with the endothelial cell permeability (121). It is also reported that HMGB1 may exacerbate the bacterial endotoxin-induced PALI by regulating the cellular autophagy in DCs via Tregs inhibition and activating the PI3K/Akt/mammalian target of the rapamycin (mTOR) pathway (122, 123). Certain immune cells (ILC2s, ILC3s, Th17, etc.) migrating to the lungs (from the intestine) may play a direct role in the mediation of the abnormal immune responses during PALI (124).
Metabolites From Intestinal Bacteria Mediate PALI
Several metabolites produced by the intestinal bacteria have been found to play a role in the mediation of the pathological process of PALI. Firstly, bacteria-derived LPS can induce PALI by activating NF-κB and MAPK pathways, and reported changes in metabolomics are also associated with abnormal fatty acid, phospholipid, and bile acid pathways (125). Free fatty acids (FFA) levels, a metabolite of triglycerides (TG), are found to be considerably higher in the serum of PALI mice, and FFAs are shown to induce the formation of NETs as well as activate DCs and T cells in the lungs (126, 127). Besides, the bacterial-derived metabolites p-cresol sulfate (PCS) and trimethylamine N-oxide (TMAO) are potential molecular markers for ERS in the lungs (128). Yumeng Huang et al. claimed that increased bacteria-derived 5-hydroxytryptophan (5-HT) in the peripheral circulation activates the formation of pulmonary NETs as well (129). While, several metabolites from intestinal bacteria, such as bile acids (ocaliva and ursodeoxycholic acid), butyric acid, etc., are proven to have protective effects on PALI (130–132).
In summary, the crosstalk between the intestinal microorganisms/bacterial metabolites and the inflammatory storm of the immune system in the lungs jointly lead to the development of PALI. The better understanding of the microbiota-gut-lung axis will aid in the elucidation of pathogenesis in PALI and provide theoretical foundations for the treatment of PALI by regulating intestinal microecology.
Interventions by Regulating the Intestinal Microbiota
Due to the roles of intestinal microbiota in SAP, it has become a prospective therapeutic target for the treatment of PALI. In recent years, several strategies mediated by modulating the intestinal microecology or corresponding functions have been applied by clinicians, which not only reduce the in-hospital mortality of SAP patients but also minimize the risks of MODS to a certain extent (133–135). Herein, clinical and basic studies on the supplementation of probiotics/prebiotics, antibiotics treatment, fecal microbiota transplantation (FMT), the intervention of medication, and early enteral nutrition (EN) are discussed.
Probiotics and Prebiotics
Probiotics and prebiotics have been shown in recent years to have potential benefits in a variety of clinical conditions. However, the use of probiotics in the treatment of SAP remains debatable (136–140). It has been reported that probiotics and prebiotics can enhance intestinal barrier functions and strengthen the mucosal immune system. The underlying mechanisms are linked to the upregulation of TJ proteins, inhibition of pathogenic bacteria, and the induction of mucin2 (MUC2), AMPs, and SCFAs, as well as the reconstruction of the intestinal microenvironment (mediated by PRRs, etc.), as illustrated in Figure 6 (141–145). Pujo et al. pointed out that probiotics produced 3-hydroxyoctadecenoic acid (C18-3OH), which could regulate the inflammatory response by binding to the PPAR-γ in intestinal epithelial cells, mediated by AP-1 (active protein-1), NF-κB, STAT3 (signal transducer and activator of transcription 3), and macrophage differentiation during PALI (146). Additionally, Lactobacillus royale, Saccharomyces boulardii, and Bifidobacterium have been reported to inhibit BT and decrease the incidence of PALI (46, 147–150). A double-blind clinical trial demonstrated that the time of hospitalization and abdominal pain is shortened in SAP patients following Bacillus subtilis and Enterococcus faecalis supplement (151). Similar to probiotics, prebiotics such as galactooligosaccharides, lactosucrose, and inulin-type fructan can substantially repair the functions of the intestinal barrier in SAP rats and have shown therapeutic potential in PALI (152–155).
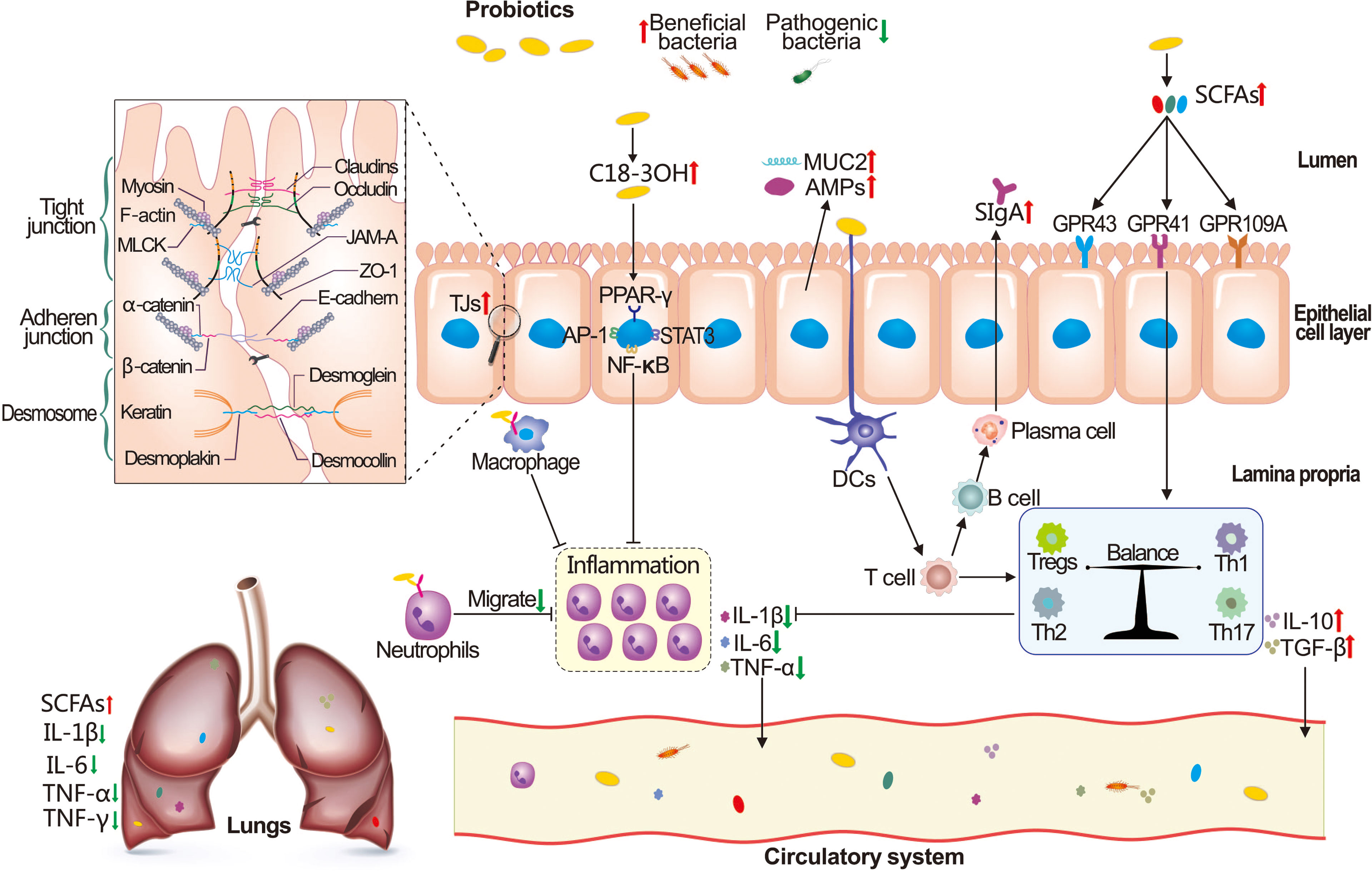
Figure 6 Possible mechanisms of probiotics supplementation on PALI recovery. Probiotics can restore the function of the intestinal barrier by inhibiting the proliferation of pathogenic bacteria, increasing the production of 3-hydroxyoctadecenoic acid (C18-3OH), short-chain fatty acids (SCFAs), antimicrobial peptides (AMPs), mucin2 (MUC2), and secretory IgA (SIgA), and regulating the expression of tight junction (TJ) proteins. In addition, probiotics have the capacity to reduce the neutrophil infiltration and release of inflammatory cytokines, maintain the balance of the intestinal immune system, and reduce the translocation of bacteria, inflammatory factors, etc., resulting in the alleviation of PALI.
Some studies, on the other hand, propose the opposite results. For instance, Gou et al. conducted a randomized controlled trial and concluded that probiotics have no beneficial impact on the 536 SAP patients (156). In addition, a double-blind trial showed that treatment with probiotics for 7 days did not present an advantage on the intestinal permeability as well as endotoxemia in SAP patients (157). The authors conclude that the heterogeneity of probiotics observed during SAP attributes to multiple factors such as the pancreatitis etiology, type of probiotics, intervention time of treatment, and the individuation in gut microbiota (158–160).
Antibiotics
Antibiotics are commonly used by clinicians for prophylactically inhibiting the growth of pathogenic bacteria in SAP patients and reducing secondary SAP infection complications. Previous studies have found that clearance of intestinal bacteria with a combination of broad-spectrum antibiotics can reduce the severity of PALI in mice. However, the lethal effect on specific intestinal bacteria might result in the risk of fungal infections along with the rapid proliferation of drug-resistant bacteria, fueling heated debates regarding antibiotic usage in pancreatic surgery (22, 161).
A study by Soares et al. revealed that the use of meropenem accelerates the progress of SAP and it causes the multi-drug resistant BT (162). Jacobs et al. observed the sustaining high levels of IL-6 in alveolar lavage fluid of PALI mice after 2 weeks of therapy with advanced antibiotics (163). The latest World Society of Emergency Surgery (WSES) guidelines for the management of SAP report that the prophylactic use of antibiotics in patients with AP is not associated with a reduction in mortality or morbidity (164). Hence, antibiotics are no longer recommended as a priority for SAP patients with the exception of obvious infections. And the choice of broad-spectrum antibiotics should take the bacteriological changes in SAP and their pharmacokinetics into account, and further studies must be conducted (165, 166).
FMT
In clinical practice, FMT has been developed as a second-line treatment option for recurrent infection of Clostridium Difficile, and it has now become a treatment option for a variety of diseases including IBD, irritable bowel syndrome (IBS), autism, hepatic encephalopathy, etc. (167–170). Even though evident changes have been observed in SAP, the identification of beneficial strains, antibiotic-resistant strains, and detrimental strains remains a challenge, which creates a gap in the protection against PALI by FMT. Furthermore, the lack of standard in FMT is another question that limits its use. A randomized controlled clinical trial by Ding et al. pointed out that FMT could not reduce the complications in SAP patients and that there is a risk of disruption in the intestinal barrier (171). Yin et al., on the other hand, found that FMT attenuates the LPS-induced PALI in rats by inhibiting the PI3K/AKT/NF-κB signaling pathway, the mechanisms of which are associated with the expression of ICAM-1 (172, 173). Since the current findings are primarily limited to animal studies, more evidence is needed to further assess the benefits of FMT in clinical usage.
Traditional Chinese Medicine
TCM has its unique advantages in the treatment of SAP, and herbal formulas such as Da Cheng Qi Decoction, Chaiqin Chengqi Decoction, Da Chaihu Decoction, etc., have been extensively utilized in SAP patients (174). Our previous studies showed that emodin, the main active component in Rheum officinale, could alleviate PALI by targeting the cold-inducible RNA-binding protein (CIRP)/NLRP3/IL-1β/CXCL1 pathway and the inhibition of neutrophil protease (5, 175). Because the majority of the herbal drugs are taken orally, the components inevitably pass through the digestive tract and reach the intestine, allowing for interactions with the intestinal microbiota. Piao et al. argued that picroside II increases the abundance of Lactobacillus, Prevotella, and restores the intestinal barrier by inhibiting the TLR4-dependent PI3K/AKT/NF-κB pathway, which thereby alleviates SAP (11) (176). Moreover, emodin has been reported to repair intestinal barrier functions and alleviate PALI by upregulating SCFA-producing bacteria (such as Akkermansia Muciniphila and Bacteroides) (177).
Enteral Nutrition
In clinical treatment, patients with SAP are frequently advised to fast and decompress the gastrointestinal tract to lower the burden on the pancreas. Long-term enteral nutrition deprivation in SAP patients, on the one hand, will lead to the reduction of digestive fluid (bile, intestinal fluid, lysozyme, etc.) and accelerate the injury of the intestinal barrier; on the other hand, it will cause intestinal mucosal epithelial necrosis and atrophy of intestinal villi, which further increases the risk of PALI (178). However, in order to maintain the function of the intestine, large amounts of fluids (including crystalloids, colloids, electrolytes, etc.) neglect the possible secondary ischemia/reperfusion (I/R) injury. And I/R injury in SAP leads to the increase of intestinal permeability as well as the disruption of intestinal microecological balance, all of which can contribute to BT risks and inflammatory responses (179). As a result, the current guidelines for the management of SAP recommend that patients should be given enteral nutrition (by intranasal tube or oral administration) at the early stage rather than total parenteral nutrition (TPN), so as to preserve the intestinal functions and prevent the secondary infectious complications (164). Early nutrition, according to Zhao et al., shortens the length of stay in hospital along with the occurrence of PALI in patients (180). Sun et al. found that early enteral nutrition affects the functions of the intestinal immune system, thereby reducing pancreatic necrosis and systemic inflammatory responses (181). Furthermore, glutamine supplementation is reported to be related to improved intestinal permeability and oxidative stress in SAP patients (182).
Summary and Prospections
Given the critical role of the microbiota-gut-lung axis in the pathogenesis of PALI, gut microbiota, regarded as a “bridge” in the pathogenesis of PALI, has been increasingly emphasized. In this paper, we summarized the dysbiosis process in the abundance of intestinal microbiota under SAP as well as the possible mechanisms by which intestinal microecological disorders accelerate bacterial translocation through disruption of the intestinal physical, chemical, and immune barriers to cause PALI. In particular, the PAMPs-mediated immunol mechanisms in the occurrence of PALI by binding with pattern recognition receptors (PRRs) via the microbiota-gut-lung axis are taken into account. Finally, we analyze several potential effective interventions that may alleviate PALI by regulating the intestinal microbiota, and it is expected to provide further references for clinical applications. Many questions still need to be addressed and further studies on the molecular mechanisms of the bidirectional communications between the intestinal microbiota and the lungs remain to be investigated. Moreover, inhibition of bacterial translocation by restoring the homeostasis of the intestinal microbiota may be a promising therapeutic target for protecting against PALI. The exploration of bacterial markers for early diagnosis of PALI is another promising subsequent topic in this field. More cohort studies and optimally designed clinical trials regarding intestinal microbiota interventions are necessary for the purpose of evaluating the safety and therapeutic efficacy of PALI treatment. Furthermore, the findings on the microbiota-gut-lung axis are expected to be useful for the diagnosis and prognosis of PALI.
Author Contributions
ZW and FL wrote the manuscript; ZW, YL, and SM made the figures; JL, HG, QY, SM, and CX revised the manuscript; HC designed the manuscript. All authors have read and approved the final manuscript.
Funding
This study was supported by the National Key R & D Programmes of China (No. 2019YFE0119300), the National Natural Science Foundation of China (No. 82074158 and No.82104594).
Conflict of Interest
The authors declare that the research was conducted in the absence of any commercial or financial relationships that could be construed as a potential conflict of interest.
Publisher’s Note
All claims expressed in this article are solely those of the authors and do not necessarily represent those of their affiliated organizations, or those of the publisher, the editors and the reviewers. Any product that may be evaluated in this article, or claim that may be made by its manufacturer, is not guaranteed or endorsed by the publisher.
Glossary
References
1. Kong L, Deng J, Zhou X, Cai B, Zhang B, Chen X, et al. Sitagliptin Activates the P62-Keap1-Nrf2 Signalling Pathway to Alleviate Oxidative Stress and Excessive Autophagy in Severe Acute Pancreatitis-Related Acute Lung Injury. Cell Death Dis (2021) 12(10):928. doi: 10.1038/s41419-021-04227-0
2. Jin M, Zhang H, Wu M, Wang Z, Chen X, Guo M, et al. Colonic Interleukin-22 Protects Intestinal Mucosal Barrier and Microbiota Abundance in Severe Acute Pancreatitis. FASEB J (2022) 36(3):e22174. doi: 10.1096/fj.202101371R
3. Wu J, Zhang J, Zhao J, Chen S, Zhou T, Xu J. Treatment of Severe Acute Pancreatitis and Related Lung Injury by Targeting Gasdermin D-Mediated Pyroptosis. Front Cell Dev Biol (2021) 9:780142. doi: 10.3389/fcell.2021.780142
4. Zhou J, Zhou P, Zhang Y, Wang G, Fan Z. Signal Pathways and Markers Involved in Acute Lung Injury Induced by Acute Pancreatitis. Dis Markers (2021) 2021:9947047. doi: 10.1155/2021/9947047
5. Xu C, Zhang J, Liu J, Li Z, Liu Z, Luo Y, et al. Proteomic Analysis Reveals the Protective Effects of Emodin on Severe Acute Pancreatitis Induced Lung Injury by Inhibiting Neutrophil Proteases Activity. J Proteomics (2020) 220:103760. doi: 10.1016/j.jprot.2020.103760
6. Ye W, Zheng C, Yu D, Zhang F, Pan R, Ni X, et al. Lipoxin A4 Ameliorates Acute Pancreatitis-Associated Acute Lung Injury Through the Antioxidative and Anti-Inflammatory Effects of the Nrf2 Pathway. Oxid Med Cell Longev (2019) 2019:2197017. doi: 10.1155/2019/2197017
7. Liang XY, Jia TX, Zhang M. Intestinal Bacterial Overgrowth in the Early Stage of Severe Acute Pancreatitis Is Associated With Acute Respiratory Distress Syndrome. World J Gastroenterol (2021) 27(15):1643–54. doi: 10.3748/wjg.v27.i15.1643
8. Ge P, Luo Y, Okoye CS, Chen H, Liu J, Zhang G, et al. Intestinal Barrier Damage, Systemic Inflammatory Response Syndrome, and Acute Lung Injury: A Troublesome Trio for Acute Pancreatitis. BioMed Pharmacother (2020) 132:110770. doi: 10.1016/j.biopha.2020.110770
9. Yang J, Wei H, Zhou Y, Szeto CH, Li C, Lin Y, et al. High-Fat Diet Promotes Colorectal Tumorigenesis Through Modulating Gut Microbiota and Metabolites. Gastroenterology (2022) 162(1):135–49.e2. doi: 10.1053/j.gastro.2021.08.041
10. Chakrabarti A, Geurts L, Hoyles L, Iozzo P, Kraneveld AD, La Fata G, et al. The Microbiota-Gut-Brain Axis: Pathways to Better Brain Health. Perspectives on What We Know, What We Need to Investigate and How to Put Knowledge Into Practice. Cell Mol Life Sci (2022) 79(2):80. doi: 10.1007/s00018-021-04060-w
11. Piao X, Liu B, Sui X, Li S, Niu W, Zhang Q, et al. Picroside II Improves Severe Acute Pancreatitis-Induced Intestinal Barrier Injury by Inactivating Oxidative and Inflammatory TLR4-Dependent PI3K/AKT/NF-κb Signaling and Improving Gut Microbiota. Oxid Med Cell Longev (2020) 2020:3589497. doi: 10.1155/2020/3589497
12. Dixit K, Chaudhari D, Dhotre D, Shouche Y, Saroj S. Restoration of Dysbiotic Human Gut Microbiome for Homeostasis. Life Sci (2021) 278:119622. doi: 10.1016/j.lfs.2021.119622
13. de Vos WM, Tilg H, Van Hul M, Cani PD. Gut Microbiome and Health: Mechanistic Insights. Gut (2022) 71(5):1020–32. doi: 10.1136/gutjnl-2021-326789
14. Ye S, Si C, Deng J, Chen X, Kong L, Zhou X, et al. Understanding the Effects of Metabolites on the Gut Microbiome and Severe Acute Pancreatitis. BioMed Res Int (2021) 2021:1516855. doi: 10.1155/2021/1516855
15. Zoulikha M, Xiao Q, Boafo GF, Sallam MA, Chen Z, He W. Pulmonary Delivery of siRNA Against Acute Lung Injury/Acute Respiratory Distress Syndrome. Acta Pharm Sin B (2021) 12(2):600–20. doi: 10.1016/j.apsb.2021.08.009
16. Hu X, Gong L, Zhou R, Han Z, Ji L, Zhang Y, et al. Variations in Gut Microbiome Are Associated With Prognosis of Hypertriglyceridemia-Associated Acute Pancreatitis. Biomolecules (2021) 11(5):695. doi: 10.3390/biom11050695
17. Yu S, Xiong Y, Fu Y, Chen G, Zhu H, Mo X, et al. Shotgun Metagenomics Reveals Significant Gut Microbiome Features in Different Grades of Acute Pancreatitis. Microb Pathog (2021) 154:104849. doi: 10.1016/j.micpath.2021.104849
18. Yu S, Xiong Y, Xu J, Liang X, Fu Y, Liu D, et al. Identification of Dysfunctional Gut Microbiota Through Rectal Swab in Patients With Different Severity of Acute Pancreatitis. Dig Dis Sci (2020) 65(11):3223–37. doi: 10.1007/s10620-020-06061-4
19. Li Q, Wang C, Tang C, Zhao X, He Q, Li J. Identification and Characterization of Blood and Neutrophil-Associated Microbiomes in Patients With Severe Acute Pancreatitis Using Next-Generation Sequencing. Front Cell Infect Microbiol (2018) 8:5. doi: 10.3389/fcimb.2018.00005
20. van den Berg FF, van Dalen D, Hyoju SK, van Santvoort HC, Besselink MG, Wiersinga WJ, et al. Western-Type Diet Influences Mortality From Necrotising Pancreatitis and Demonstrates a Central Role for Butyrate. Gut (2021) 70(5):915–27. doi: 10.1136/gutjnl-2019-320430
21. Mei QX, Hu JH, Huang ZH, Fan JJ, Huang CL, Lu YY, et al. Pretreatment With Chitosan Oligosaccharides Attenuate Experimental Severe Acute Pancreatitis via Inhibiting Oxidative Stress and Modulating Intestinal Homeostasis. Acta Pharmacol Sin (2021) 42(6):942–53. doi: 10.1038/s41401-020-00581-5
22. Zhu Y, He C, Li X, Cai Y, Hu J, Liao Y, et al. Gut Microbiota Dysbiosis Worsens the Severity of Acute Pancreatitis in Patients and Mice. J Gastroenterol (2019) 54(4):347–58. doi: 10.1007/s00535-018-1529-0
23. Su SY, Tang QQ. Altered Intestinal Microflora and Barrier Injury in Severe Acute Pancreatitis can be Changed by Zinc. Int J Med Sci (2021) 18(14):3050–8. doi: 10.7150/ijms.45980
24. van den Berg FF, Hugenholtz F, Boermeester MA, Zaborina O, Alverdy JC. Spatioregional Assessment of the Gut Microbiota in Experimental Necrotizing Pancreatitis. BJS Open (2021) 5(5):zrab061. doi: 10.1093/bjsopen/zrab061
25. Stolfi C, Maresca C, Monteleone G, Laudisi F. Implication of Intestinal Barrier Dysfunction in Gut Dysbiosis and Diseases. Biomedicines (2022) 10(2):289. doi: 10.3390/biomedicines10020289
26. Duan H, Yu L, Tian F, Zhai Q, Fan L, Chen W. Antibiotic-Induced Gut Dysbiosis and Barrier Disruption and the Potential Protective Strategies. Crit Rev Food Sci Nutr (2022) 62(6):1427–52. doi: 10.1080/10408398.2020.1843396
27. Tian Y, Shu R, Lei Y, Xu Y, Zhang X, Luo H. Somatostatin Attenuates Intestinal Epithelial Barrier Injury During Acute Intestinal Ischemia-Reperfusion Through Tollip/Myeloiddifferentiationfactor 88/Nuclear Factor Kappa-B Signaling. Bioengineered (2022) 13(3):5005–20. doi: 10.1080/21655979.2022.2038450
28. Du L, Hao YM, Yang YH, Zheng Y, Wu ZJ, Zhou MQ, et al. DHA-Enriched Phospholipids and EPA-Enriched Phospholipids Alleviate Lipopolysaccharide-Induced Intestinal Barrier Injury in Mice via a Sirtuin 1-Dependent Mechanism. J Agric Food Chem (2022) 70(9):2911–22. doi: 10.1021/acs.jafc.1c07761
29. Untersmayr E, Brandt A, Koidl L, Bergheim I. The Intestinal Barrier Dysfunction as Driving Factor of Inflammaging. Nutrients (2022) 14(5):949. doi: 10.3390/nu14050949
30. Li XY, He C, Zhu Y, Lu NH. Role of Gut Microbiota on Intestinal Barrier Function in Acute Pancreatitis. World J Gastroenterol (2020) 26(18):2187–93. doi: 10.3748/wjg.v26.i18.2187
31. Gieryńska M, Szulc-Dąbrowska L, Struzik J, Mielcarska MB, Gregorczyk-Zboroch KP. Integrity of the Intestinal Barrier: The Involvement of Epithelial Cells and Microbiota-a Mutual Relationship. Anim (Basel) (2022) 12(2):145. doi: 10.3390/ani12020145
32. Erdem Büyükkiraz M, Kesmen Z. Antimicrobial Peptides (AMPs): A Promising Class of Antimicrobial Compounds. J Appl Microbiol (2022) 132(3):1573–96. doi: 10.1111/jam.15314
33. Hansson GC. Mucins and the Microbiome. Annu Rev Biochem (2020) 89:769–93. doi: 10.1146/annurev-biochem-011520-105053
34. Schütte A, Ermund A, Becker-Pauly C, Johansson ME, Rodriguez-Pineiro AM, Bäckhed F, et al. Microbial-Induced Meprin β Cleavage in MUC2 Mucin and a Functional CFTR Channel are Required to Release Anchored Small Intestinal Mucus. Proc Natl Acad Sci U S A (2014) 111(34):12396–401. doi: 10.1073/pnas.1407597111
35. Johansson ME, Jakobsson HE, Holmén-Larsson J, Schütte A, Ermund A, Rodríguez-Piñeiro AM, et al. Normalization of Host Intestinal Mucus Layers Requires Long-Term Microbial Colonization. Cell Host Microbe (2015) 18(5):582–92. doi: 10.1016/j.chom.2015.10.007
36. Shimizu K, Ojima M, Ogura H. Gut Microbiota and Probiotics/Synbiotics for Modulation of Immunity in Critically Ill Patients. Nutrients (2021) 13(7):2439. doi: 10.3390/nu13072439
37. Fritz S, Hackert T, Hartwig W, Rossmanith F, Strobel O, Schneider L, et al. Bacterial Translocation and Infected Pancreatic Necrosis in Acute Necrotizing Pancreatitis Derives From Small Bowel Rather Than From Colon. Am J Surg (2010) 200(1):111–7. doi: 10.1016/j.amjsurg.2009.08.019
38. Fishman JE, Levy G, Alli V, Zheng X, Mole DJ, Deitch EA. The Intestinal Mucus Layer is a Critical Component of the Gut Barrier That Is Damaged During Acute Pancreatitis. Shock (2014) 42(3):264–70. doi: 10.1097/shk.0000000000000209
39. Chen J, Huang C, Wang J, Zhou H, Lu Y, Lou L, et al. Dysbiosis of Intestinal Microbiota and Decrease in Paneth Cell Antimicrobial Peptide Level During Acute Necrotizing Pancreatitis in Rats. PLoS One (2017) 12(4):e0176583. doi: 10.1371/journal.pone.0176583
40. Ahuja M, Schwartz DM, Tandon M, Son A, Zeng M, Swaim W, et al. Orai1-Mediated Antimicrobial Secretion From Pancreatic Acini Shapes the Gut Microbiome and Regulates Gut Innate Immunity. Cell Metab (2017) 25(3):635–46. doi: 10.1016/j.cmet.2017.02.007
41. Guo Y, Huang C, Liu L, Fu X, Lu Y, Zheng J, et al. Paneth Cell Ablation Aggravates Pancreatic and Intestinal Injuries in a Rat Model of Acute Necrotizing Pancreatitis After Normal and High-Fat Diet. Mediators Inflammation (2019) 2019:8474523. doi: 10.1155/2019/8474523
42. Zhu G, Hu J, Xi R. The Cellular Niche for Intestinal Stem Cells: A Team Effort. Cell Regener (2021) 10(1):1. doi: 10.1186/s13619-020-00061-5
43. Mehandru S, Colombel JF. The Intestinal Barrier, an Arbitrator Turned Provocateur in IBD. Nat Rev Gastroenterol Hepatol (2021) 18(2):83–4. doi: 10.1038/s41575-020-00399-w
44. Peterson LW, Artis D. Intestinal Epithelial Cells: Regulators of Barrier Function and Immune Homeostasis. Nat Rev Immunol (2014) 14(3):141–53. doi: 10.1038/nri3608
45. Suzuki T. Regulation of the Intestinal Barrier by Nutrients: The Role of Tight Junctions. Anim Sci J (2020) 91(1):e13357. doi: 10.1111/asj.13357
46. Wu H, Xie S, Miao J, Li Y, Wang Z, Wang M, et al. Lactobacillus Reuteri Maintains Intestinal Epithelial Regeneration and Repairs Damaged Intestinal Mucosa. Gut Microbes (2020) 11(4):997–1014. doi: 10.1080/19490976.2020.1734423
47. Zhao L, Xie Q, Etareri Evivie S, Liu D, Dong J, Ping L, et al. Bifidobacterium Dentium N8 With Potential Probiotic Characteristics Prevents LPS-Induced Intestinal Barrier Injury by Alleviating the Inflammatory Response and Regulating the Tight Junction in Caco-2 Cell Monolayers. Food Funct (2021) 12(16):7171–84. doi: 10.1039/d1fo01164b
48. Haussner F, Chakraborty S, Halbgebauer R, Huber-Lang M. Challenge to the Intestinal Mucosa During Sepsis. Front Immunol (2019) 10:891. doi: 10.3389/fimmu.2019.00891
49. Barichello T, Generoso JS, Singer M, Dal-Pizzol F. Biomarkers for Sepsis: More Than Just Fever and Leukocytosis-a Narrative Review. Crit Care (2022) 26(1):14. doi: 10.1186/s13054-021-03862-5
50. Zheng J, Lou L, Fan J, Huang C, Mei Q, Wu J, et al. Commensal Escherichia Coli Aggravates Acute Necrotizing Pancreatitis Through Targeting of Intestinal Epithelial Cells. Appl Environ Microbiol (2019) 85(12):e00059–19. doi: 10.1128/aem.00059-19
51. Hao W, Hao C, Wu C, Xu Y, Jin C. Aluminum Induced Intestinal Dysfunction via Mechanical, Immune, Chemical and Biological Barriers. Chemosphere (2022) 288(Pt 2):132556. doi: 10.1016/j.chemosphere.2021.132556
52. Yoo JY, Groer M, Dutra SVO, Sarkar A, McSkimming DI. Gut Microbiota and Immune System Interactions. Microorganisms (2020) 8(10):1587. doi: 10.3390/microorganisms8101587
53. Pagliari D, Saviano A, Newton EE, Serricchio ML, Dal Lago AA, Gasbarrini A, et al. Gut Microbiota-Immune System Crosstalk and Pancreatic Disorders. Mediators Inflamm (2018) 2018:7946431. doi: 10.1155/2018/7946431
54. Pickard JM, Zeng MY, Caruso R, Núñez G. Gut Microbiota: Role in Pathogen Colonization, Immune Responses, and Inflammatory Disease. Immunol Rev (2017) 279(1):70–89. doi: 10.1111/imr.12567
55. Zhu Y, Wang X, Zhu L, Tu Y, Chen W, Gong L, et al. Lactobacillus Rhamnosus GG Combined With Inosine Ameliorates Alcohol-Induced Liver Injury Through Regulation of Intestinal Barrier and Treg/Th1 Cells. Toxicol Appl Pharmacol (2022) 439:115923. doi: 10.1016/j.taap.2022.115923
56. Wei H, Wang JY. Role of Polymeric Immunoglobulin Receptor in IgA and IgM Transcytosis. Int J Mol Sci (2021) 22(5):2284. doi: 10.3390/ijms22052284
57. Nakamoto N, Amiya T, Aoki R, Taniki N, Koda Y, Miyamoto K, et al. Commensal Lactobacillus Controls Immune Tolerance During Acute Liver Injury in Mice. Cell Rep (2017) 21(5):1215–26. doi: 10.1016/j.celrep.2017.10.022
58. Barbara G, Barbaro MR, Fuschi D, Palombo M, Falangone F, Cremon C, et al. Inflammatory and Microbiota-Related Regulation of the Intestinal Epithelial Barrier. Front Nutr (2021) 8:718356. doi: 10.3389/fnut.2021.718356
59. Nishihara Y, Ogino H, Tanaka M, Ihara E, Fukaura K, Nishioka K, et al. Mucosa-Associated Gut Microbiota Reflects Clinical Course of Ulcerative Colitis. Sci Rep (2021) 11(1):13743. doi: 10.1038/s41598-021-92870-0
60. Atif SM, Uematsu S, Akira S, McSorley SJ. CD103-CD11b+ Dendritic Cells Regulate the Sensitivity of CD4 T-Cell Responses to Bacterial Flagellin. Mucosal Immunol (2014) 7(1):68–77. doi: 10.1038/mi.2013.25
61. Liu Q, Yu Z, Tian F, Zhao J, Zhang H, Zhai Q, et al. Surface Components and Metabolites of Probiotics for Regulation of Intestinal Epithelial Barrier. Microb Cell Fact (2020) 19(1):23. doi: 10.1186/s12934-020-1289-4
62. Ratajczak W, Rył A, Mizerski A, Walczakiewicz K, Sipak O, Laszczyńska M. Immunomodulatory Potential of Gut Microbiome-Derived Short-Chain Fatty Acids (SCFAs). Acta Biochim Pol (2019) 66(1):1–12. doi: 10.18388/abp.2018_2648
63. Gonçalves P, Araújo JR, Di Santo JP. A Cross-Talk Between Microbiota-Derived Short-Chain Fatty Acids and the Host Mucosal Immune System Regulates Intestinal Homeostasis and Inflammatory Bowel Disease. Inflammation Bowel Dis (2018) 24(3):558–72. doi: 10.1093/ibd/izx029
64. Mroz MS, Lajczak NK, Goggins BJ, Keely S, Keely SJ. The Bile Acids, Deoxycholic Acid and Ursodeoxycholic Acid, Regulate Colonic Epithelial Wound Healing. Am J Physiol Gastrointest Liver Physiol (2018) 314(3):G378–87. doi: 10.1152/ajpgi.00435.2016
65. Kahalehili HM, Newman NK, Pennington JM, Kolluri SK, Kerkvliet NI, Shulzhenko N, et al. Dietary Indole-3-Carbinol Activates AhR in the Gut, Alters Th17-Microbe Interactions, and Exacerbates Insulitis in NOD Mice. Front Immunol (2020) 11:606441. doi: 10.3389/fimmu.2020.606441
66. Yang S, Li X, Yang F, Zhao R, Pan X, Liang J, et al. Gut Microbiota-Dependent Marker TMAO in Promoting Cardiovascular Disease: Inflammation Mechanism, Clinical Prognostic, and Potential as a Therapeutic Target. Front Pharmacol (2019) 10:1360. doi: 10.3389/fphar.2019.01360
67. Dickson RP, Singer BH, Newstead MW, Falkowski NR, Erb-Downward JR, Standiford TJ, et al. Enrichment of the Lung Microbiome With Gut Bacteria in Sepsis and the Acute Respiratory Distress Syndrome. Nat Microbiol (2016) 1(10):16113. doi: 10.1038/nmicrobiol.2016.113
68. Frati F, Salvatori C, Incorvaia C, Bellucci A, Di Cara G, Marcucci F, et al. The Role of the Microbiome in Asthma: The Gut-Lung Axis. Int J Mol Sci (2018) 20(1):123. doi: 10.3390/ijms20010123
69. Fromentin M, Ricard JD, Roux D. Lung Microbiome in Critically Ill Patients. Life (Basel) (2021) 12(1):7. doi: 10.3390/life12010007
70. Wang C, Li Q, Ren J. Microbiota-Immune Interaction in the Pathogenesis of Gut-Derived Infection. Front Immunol (2019) 10:1873. doi: 10.3389/fimmu.2019.01873
71. Wen W, Zheng H, Jiang Y, Huang L, Li D, Zhang J, et al. Effect of Intestinal Epithelial Autophagy on Bacterial Translocation in Severe Acute Pancreatitis. Clin Res Hepatol Gastroenterol (2017) 41(6):703–10. doi: 10.1016/j.clinre.2017.03.007
72. Keely S, Glover LE, Weissmueller T, MacManus CF, Fillon S, Fennimore B, et al. Hypoxia-Inducible Factor-Dependent Regulation of Platelet-Activating Factor Receptor as a Route for Gram-Positive Bacterial Translocation Across Epithelia. Mol Biol Cell (2010) 21(4):538–46. doi: 10.1091/mbc.e09-07-0573
73. Li Q, Wang C, Tang C, He Q, Li N, Li J. Bacteremia in Patients With Acute Pancreatitis as Revealed by 16S Ribosomal RNA Gene-Based Techniques. Crit Care Med (2013) 41(8):1938–50. doi: 10.1097/CCM.0b013e31828a3dba
74. Yokoyama Y, Fukaya M, Mizuno T, Ebata T, Asahara T, Nagino M. Clinical Importance of "Occult-Bacterial Translocation" in Patients Undergoing Highly Invasive Gastrointestinal Surgery: A Review. Surg Today (2021) 51(4):485–92. doi: 10.1007/s00595-020-02126-z
75. Arab JP, Martin-Mateos RM, Shah VH. Gut-Liver Axis, Cirrhosis and Portal Hypertension: The Chicken and the Egg. Hepatol Int (2018) 12(Suppl 1):24–33. doi: 10.1007/s12072-017-9798-x
76. Vaishnavi C. Translocation of Gut Flora and its Role in Sepsis. Indian J Med Microbiol (2013) 31(4):334–42. doi: 10.4103/0255-0857.118870
77. Liu Y, Chen C, Sun Q, Sun H, Liu N, Liu Q, et al. Mesenteric Lymph Duct Drainage Attenuates Lung Inflammatory Injury and Inhibits Endothelial Cell Apoptosis in Septic Rats. BioMed Res Int (2020) 2020:3049302. doi: 10.1155/2020/3049302
78. Zhang YM, Zhang SK, Cui NQ. Intravenous Infusion of Mesenteric Lymph From Severe Intraperitoneal Infection Rats Causes Lung Injury in Healthy Rats. World J Gastroenterol (2014) 20(16):4771–7. doi: 10.3748/wjg.v20.i16.4771
79. González-Loyola A, Bovay E, Kim J, Lozano TW, Sabine A, Renevey F, et al. FOXC2 Controls Adult Lymphatic Endothelial Specialization, Function, and Gut Lymphatic Barrier Preventing Multiorgan Failure. Sci Adv (2021) 7(29):eabf4335. doi: 10.1126/sciadv.abf4335
80. Deitch EA. Gut Lymph and Lymphatics: A Source of Factors Leading to Organ Injury and Dysfunction. Ann N Y Acad Sci (2010) 1207(Suppl 1):E103–11. doi: 10.1111/j.1749-6632.2010.05713.x
81. Assimakopoulos SF, Triantos C, Thomopoulos K, Fligou F, Maroulis I, Marangos M, et al. Gut-Origin Sepsis in the Critically Ill Patient: Pathophysiology and Treatment. Infection (2018) 46(6):751–60. doi: 10.1007/s15010-018-1178-5
82. Zhao D, Yang F, Wang Y, Li S, Li Y, Hou F, et al. ALK1 Signaling is Required for the Homeostasis of Kupffer Cells and Prevention of Bacterial Infection. J Clin Invest (2022) 132(3):e150489. doi: 10.1172/jci150489
83. Llamas MA, Aller MA, Marquina D, Nava MP, Arias J. Bacterial Translocation to Mesenteric Lymph Nodes Increases in Chronic Portal Hypertensive Rats. Dig Dis Sci (2010) 55(8):2244–54. doi: 10.1007/s10620-009-1001-3
84. Zhang G, Jazwinski Faust A. Spontaneous Bacterial Peritonitis. Jama (2021) 325(11):1118. doi: 10.1001/jama.2020.10292
85. Baron TH, DiMaio CJ, Wang AY, Morgan KA. American Gastroenterological Association Clinical Practice Update: Management of Pancreatic Necrosis. Gastroenterology (2020) 158(1):67–75. doi: 10.1053/j.gastro.2019.07.064
86. Liu L, Liu W, Yan H, Cui J, Zhou J, Wang T, et al. Abdominal Paracentesis Drainage Does Not Bring Extra Risk to Patients With Severe Acute Pancreatitis. J Clin Gastroenterol (2016) 50(5):439. doi: 10.1097/mcg.0000000000000488
87. Huang SQ, Wen Y, Sun HY, Deng J, Zhang YL, Huang QL, et al. Abdominal Paracentesis Drainage Attenuates Intestinal Inflammation in Rats With Severe Acute Pancreatitis by Inhibiting the HMGB1-Mediated TLR4 Signaling Pathway. World J Gastroenterol (2021) 27(9):815–34. doi: 10.3748/wjg.v27.i9.815
88. Enaud R, Prevel R, Ciarlo E, Beaufils F, Wieërs G, Guery B, et al. The Gut-Lung Axis in Health and Respiratory Diseases: A Place for Inter-Organ and Inter-Kingdom Crosstalks. Front Cell Infect Microbiol (2020) 10:9. doi: 10.3389/fcimb.2020.00009
89. Calfee CS, Janz DR, Bernard GR, May AK, Kangelaris KN, Matthay MA, et al. Distinct Molecular Phenotypes of Direct vs Indirect ARDS in Single-Center and Multicenter Studies. Chest (2015) 147(6):1539–48. doi: 10.1378/chest.14-2454
90. Shirey KA, Blanco JCG, Vogel SN. Targeting TLR4 Signaling to Blunt Viral-Mediated Acute Lung Injury. Front Immunol (2021) 12:705080. doi: 10.3389/fimmu.2021.705080
91. Tan JY, Tang YC, Huang J. Gut Microbiota and Lung Injury. Adv Exp Med Biol (2020) 1238:55–72. doi: 10.1007/978-981-15-2385-4_5
92. Donovan C, Liu G, Shen S, Marshall JE, Kim RY, Alemao CA, et al. The Role of the Microbiome and the NLRP3 Inflammasome in the Gut and Lung. J Leukoc Biol (2020) 108(3):925–35. doi: 10.1002/jlb.3mr0720-472rr
93. Zhu CJ, Yang WG, Li DJ, Song YD, Chen SY, Wang QF, et al. Calycosin Attenuates Severe Acute Pancreatitis-Associated Acute Lung Injury by Curtailing High Mobility Group Box 1- Induced Inflammation. World J Gastroenterol (2021) 27(44):7669–86. doi: 10.3748/wjg.v27.i44.7669
94. Techarang T, Jariyapong P, Viriyavejakul P, Punsawad C. High Mobility Group Box-1 (HMGB-1) and its Receptors in the Pathogenesis of Malaria-Associated Acute Lung Injury/Acute Respiratory Distress Syndrome in a Mouse Model. Heliyon (2021) 7(12):e08589. doi: 10.1016/j.heliyon.2021.e08589
95. Wu Y, Huang D, Wang X, Pei C, Xiao W, Wang F, et al. Suppression of NLRP3 Inflammasome by Platycodin D via the TLR4/MyD88/NF-κb Pathway Contributes to Attenuation of Lipopolysaccharide Induced Acute Lung Injury in Rats. Int Immunopharmacol (2021) 96:107621. doi: 10.1016/j.intimp.2021.107621
96. Qu L, Chen C, Chen Y, Li Y, Tang F, Huang H, et al. High-Mobility Group Box 1 (HMGB1) and Autophagy in Acute Lung Injury (ALI): A Review. Med Sci Monit (2019) 25:1828–37. doi: 10.12659/msm.912867
97. Dicker AJ, Crichton ML, Pumphrey EG, Cassidy AJ, Suarez-Cuartin G, Sibila O, et al. Neutrophil Extracellular Traps are Associated With Disease Severity and Microbiota Diversity in Patients With Chronic Obstructive Pulmonary Disease. J Allergy Clin Immunol (2018) 141(1):117–27. doi: 10.1016/j.jaci.2017.04.022
98. Lefrançais E, Mallavia B, Zhuo H, Calfee CS, Looney MR. Maladaptive Role of Neutrophil Extracellular Traps in Pathogen-Induced Lung Injury. JCI Insight (2018) 3(3):e98178. doi: 10.1172/jci.insight.98178
99. Saffarzadeh M, Juenemann C, Queisser MA, Lochnit G, Barreto G, Galuska SP, et al. Neutrophil Extracellular Traps Directly Induce Epithelial and Endothelial Cell Death: A Predominant Role of Histones. PLoS One (2012) 7(2):e32366. doi: 10.1371/journal.pone.0032366
100. Zhao J, Zhang G, Cui W, Tian B. Progress of Neutrophil Extracellular Traps in Airway Inflammation of Acute Lung Injury/Acute Respiratory Distress Syndrome: Review. Xi Bao Yu Fen Zi Mian Yi Xue Za Zhi (2020) 36(7):664–70.
101. Conhaim RL, Mangino MJ, Dovi WF, Watson KE, Warner TF, Harms BA. Microthrombus Formation may Trigger Lung Injury After Acute Blood Loss. Shock (2010) 34(6):601–7. doi: 10.1097/SHK.0b013e3181e46e2a
102. Ojima M, Yamamoto N, Hirose T, Hamaguchi S, Tasaki O, Kojima T, et al. Serial Change of Neutrophil Extracellular Traps in Tracheal Aspirate of Patients With Acute Respiratory Distress Syndrome: Report of Three Cases. J Intensive Care (2020) 8:25. doi: 10.1186/s40560-020-00444-5
103. Mikacenic C, Moore R, Dmyterko V, West TE, Altemeier WA, Liles WC, et al. Neutrophil Extracellular Traps (NETs) Are Increased in the Alveolar Spaces of Patients With Ventilator-Associated Pneumonia. Crit Care (2018) 22(1):358. doi: 10.1186/s13054-018-2290-8
104. Zhan Y, Ling Y, Deng Q, Qiu Y, Shen J, Lai H, et al. HMGB1-Mediated Neutrophil Extracellular Trap Formation Exacerbates Intestinal Ischemia/Reperfusion-Induced Acute Lung Injury. J Immunol (2022) 208(4):968–78. doi: 10.4049/jimmunol.2100593
105. Chen X, Tang J, Shuai W, Meng J, Feng J, Han Z. Macrophage Polarization and its Role in the Pathogenesis of Acute Lung Injury/Acute Respiratory Distress Syndrome. Inflammation Res (2020) 69(9):883–95. doi: 10.1007/s00011-020-01378-2
106. Cheng P, Li S, Chen H. Macrophages in Lung Injury, Repair, and Fibrosis. Cells (2021) 10(2):436. doi: 10.3390/cells10020436
107. Bernasconi E, Pattaroni C, Koutsokera A, Pison C, Kessler R, Benden C, et al. Airway Microbiota Determines Innate Cell Inflammatory or Tissue Remodeling Profiles in Lung Transplantation. Am J Respir Crit Care Med (2016) 194(10):1252–63. doi: 10.1164/rccm.201512-2424OC
108. Christoffersen TE, Hult LT, Kuczkowska K, Moe KM, Skeie S, Lea T, et al. In Vitro Comparison of the Effects of Probiotic, Commensal and Pathogenic Strains on Macrophage Polarization. Probiotics Antimicrob Proteins (2014) 6(1):1–10. doi: 10.1007/s12602-013-9152-0
109. Dickson RP, Schultz MJ, van der Poll T, Schouten LR, Falkowski NR, Luth JE, et al. Lung Microbiota Predict Clinical Outcomes in Critically Ill Patients. Am J Respir Crit Care Med (2020) 201(5):555–63. doi: 10.1164/rccm.201907-1487OC
110. Wang J, Li R, Peng Z, Hu B, Rao X, Li J. HMGB1 Participates in LPS−induced Acute Lung Injury by Activating the AIM2 Inflammasome in Macrophages and Inducing Polarization of M1 Macrophages via TLR2, TLR4, and RAGE/Nf−κb Signaling Pathways. Int J Mol Med (2020) 45(1):61–80. doi: 10.3892/ijmm.2019.4402
111. Sencio V, Barthelemy A, Tavares LP, Machado MG, Soulard D, Cuinat C, et al. Gut Dysbiosis During Influenza Contributes to Pulmonary Pneumococcal Superinfection Through Altered Short-Chain Fatty Acid Production. Cell Rep (2020) 30(9):2934–47. doi: 10.1016/j.celrep.2020.02.013
112. Li D, Ren W, Jiang Z, Zhu L. Regulation of the NLRP3 Inflammasome and Macrophage Pyroptosis by the P38 MAPK Signaling Pathway in a Mouse Model of Acute Lung Injury. Mol Med Rep (2018) 18(5):4399–409. doi: 10.3892/mmr.2018.9427
113. Jiao Y, Zhang T, Zhang C, Ji H, Tong X, Xia R, et al. Exosomal miR-30d-5p of Neutrophils Induces M1 Macrophage Polarization and Primes Macrophage Pyroptosis in Sepsis-Related Acute Lung Injury. Crit Care (2021) 25(1):356. doi: 10.1186/s13054-021-03775-3
114. Hu Q, Lyon CJ, Fletcher JK, Tang W, Wan M, Hu TY. Extracellular Vesicle Activities Regulating Macrophage- and Tissue-Mediated Injury and Repair Responses. Acta Pharm Sin B (2021) 11(6):1493–512. doi: 10.1016/j.apsb.2020.12.014
115. Feng Z, Zhou J, Liu Y, Xia R, Li Q, Yan L, et al. Epithelium- and Endothelium-Derived Exosomes Regulate the Alveolar Macrophages by Targeting RGS1 Mediated Calcium Signaling-Dependent Immune Response. Cell Death Differ (2021) 28(7):2238–56. doi: 10.1038/s41418-021-00750-x
116. Kumar V. Pulmonary Innate Immune Response Determines the Outcome of Inflammation During Pneumonia and Sepsis-Associated Acute Lung Injury. Front Immunol (2020) 11:1722. doi: 10.3389/fimmu.2020.01722
117. Opitz B, van Laak V, Eitel J, Suttorp N. Innate Immune Recognition in Infectious and Noninfectious Diseases of the Lung. Am J Respir Crit Care Med (2010) 181(12):1294–309. doi: 10.1164/rccm.200909-1427SO
118. Root-Bernstein R. Innate Receptor Activation Patterns Involving TLR and NLR Synergisms in COVID-19, ALI/ARDS and Sepsis Cytokine Storms: A Review and Model Making Novel Predictions and Therapeutic Suggestions. Int J Mol Sci (2021) 22(4):2108. doi: 10.3390/ijms22042108
119. Zhao D, Wang C, Liu X, Liu N, Zhuang S, Zhang Q, et al. CircN4bp1 Facilitates Sepsis-Induced Acute Respiratory Distress Syndrome Through Mediating Macrophage Polarization via the miR-138-5p/EZH2 Axis. Mediators Inflammation (2021) 2021:7858746. doi: 10.1155/2021/7858746
120. Bui TM, Wiesolek HL, Sumagin R. ICAM-1: A Master Regulator of Cellular Responses in Inflammation, Injury Resolution, and Tumorigenesis. J Leukoc Biol (2020) 108(3):787–99. doi: 10.1002/jlb.2mr0220-549r
121. Abedi F, Hayes AW, Reiter R, Karimi G. Acute Lung Injury: The Therapeutic Role of Rho Kinase Inhibitors. Pharmacol Res (2020) 155:104736. doi: 10.1016/j.phrs.2020.104736
122. Li R, Zhang J, Pan S, Yuan Y, Qi H, Shu H, et al. HMGB1 Aggravates Lipopolysaccharide-Induced Acute Lung Injury Through Suppressing the Activity and Function of Tregs. Cell Immunol (2020) 356:104192. doi: 10.1016/j.cellimm.2020.104192
123. Li R, Zou X, Huang H, Yu Y, Zhang H, Liu P, et al. HMGB1/PI3K/Akt/mTOR Signaling Participates in the Pathological Process of Acute Lung Injury by Regulating the Maturation and Function of Dendritic Cells. Front Immunol (2020) 11:1104. doi: 10.3389/fimmu.2020.01104
124. Miskolci V, Klemm LC, Huttenlocher A. Cell Migration Guided by Cell-Cell Contacts in Innate Immunity. Trends Cell Biol (2021) 31(2):86–94. doi: 10.1016/j.tcb.2020.11.002
125. Wang T, Lin S, Liu R, Li H, Liu Z, Zhang X, et al. Metabolomic Profile Perturbations of Serum, Lung, Bronchoalveolar Lavage Fluid, Spleen and Feces in LPS-Induced Acute Lung Injury Rats Based on HPLC-ESI-QTOF-Ms. Anal Bioanal Chem (2020) 412(5):1215–34. doi: 10.1007/s00216-019-02357-1
126. Kokuho N, Terasaki Y, Kunugi S, Saito Y, Urushiyama H, Terasaki M, et al. Analyses of Alveolar Epithelial Injury via Lipid-Related Stress in Mammalian Target of Rapamycin Inhibitor-Induced Lung Disease. Lab Invest (2019) 99(6):853–65. doi: 10.1038/s41374-018-0158-9
127. Chen W, Chen H, Yang ZT, Mao EQ, Chen Y, Chen EZ. Free Fatty Acids-Induced Neutrophil Extracellular Traps Lead to Dendritic Cells Activation and T Cell Differentiation in Acute Lung Injury. Aging (Albany NY) (2021) 13(24):26148–60. doi: 10.18632/aging.203802
128. Wang Z, Ma P, Wang Y, Hou B, Zhou C, Tian H, et al. Untargeted Metabolomics and Transcriptomics Identified Glutathione Metabolism Disturbance and PCS and TMAO as Potential Biomarkers for ER Stress in Lung. Sci Rep (2021) 11(1):14680. doi: 10.1038/s41598-021-92779-8
129. Huang Y, Ji Q, Zhu Y, Fu S, Chen S, Chu L, et al. Activated Platelets Autocrine 5-Hydroxytryptophan Aggravates Sepsis-Induced Acute Lung Injury by Promoting Neutrophils Extracellular Traps Formation. Front Cell Dev Biol (2021) 9:777989. doi: 10.3389/fcell.2021.777989
130. Kim JY, Piao C, Kim G, Lee S, Lee MS, Jeong JH, et al. Combined Delivery of a Lipopolysaccharide-Binding Peptide and the Heme Oxygenase-1 Gene Using Deoxycholic Acid-Conjugated Polyethylenimine for the Treatment of Acute Lung Injury. Macromol Biosci (2017) 17(8):1600490. doi: 10.1002/mabi.201600490
131. Fei J, Fu L, Hu B, Chen YH, Zhao H, Xu DX, et al. Obeticholic Acid Alleviate Lipopolysaccharide-Induced Acute Lung Injury via Its Anti-Inflammatory Effects in Mice. Int Immunopharmacol (2019) 66:177–84. doi: 10.1016/j.intimp.2018.11.005
132. Ying XD, Wei G, An H. Sodium Butyrate Relieves Lung Ischemia-Reperfusion Injury by Inhibiting NF-κb and JAK2/STAT3 Signaling Pathways. Eur Rev Med Pharmacol Sci (2021) 25(1):413–22. doi: 10.26355/eurrev_202101_24409
133. Lee JG, Lee J, Lee AR, Jo SV, Park CH, Han DS, et al. Impact of Short-Chain Fatty Acid Supplementation on Gut Inflammation and Microbiota Composition in a Murine Colitis Model. J Nutr Biochem (2022) 101:108926. doi: 10.1016/j.jnutbio.2021.108926
134. Peterson CT, Perez Santiago J, Iablokov SN, Chopra D, Rodionov DA, Peterson SN. Short-Chain Fatty Acids Modulate Healthy Gut Microbiota Composition and Functional Potential. Curr Microbiol (2022) 79(5):128. doi: 10.1007/s00284-022-02825-5
135. Lei Y, Tang L, Liu S, Hu S, Wu L, Liu Y, et al. Parabacteroides Produces Acetate to Alleviate Heparanase-Exacerbated Acute Pancreatitis Through Reducing Neutrophil Infiltration. Microbiome (2021) 9(1):115. doi: 10.1186/s40168-021-01065-2
136. Snigdha S, Ha K, Tsai P, Dinan TG, Bartos JD, Shahid M. Probiotics: Potential Novel Therapeutics for Microbiota-Gut-Brain Axis Dysfunction Across Gender and Lifespan. Pharmacol Ther (2022) 231:107978. doi: 10.1016/j.pharmthera.2021.107978
137. Chaiyasut C, Sivamaruthi BS, Lailerd N, Sirilun S, Khongtan S, Fukngoen P, et al. Probiotics Supplementation Improves Intestinal Permeability, Obesity Index and Metabolic Biomarkers in Elderly Thai Subjects: A Randomized Controlled Trial. Foods (2022) 11(3):268. doi: 10.3390/foods11030268
138. Lee M, Chang EB. Inflammatory Bowel Diseases (IBD) and the Microbiome-Searching the Crime Scene for Clues. Gastroenterology (2021) 160(2):524–37. doi: 10.1053/j.gastro.2020.09.056
139. Hill C, Guarner F, Reid G, Gibson GR, Merenstein DJ, Pot B, et al. Expert Consensus Document. The International Scientific Association for Probiotics and Prebiotics Consensus Statement on the Scope and Appropriate Use of the Term Probiotic. Nat Rev Gastroenterol Hepatol (2014) 11(8):506–14. doi: 10.1038/nrgastro.2014.66
140. Gibson GR, Hutkins R, Sanders ME, Prescott SL, Reimer RA, Salminen SJ, et al. Expert Consensus Document: The International Scientific Association for Probiotics and Prebiotics (ISAPP) Consensus Statement on the Definition and Scope of Prebiotics. Nat Rev Gastroenterol Hepatol (2017) 14(8):491–502. doi: 10.1038/nrgastro.2017.75
141. Liu Y, Wang J, Wu C. Modulation of Gut Microbiota and Immune System by Probiotics, Pre-Biotics, and Post-Biotics. Front Nutr (2021) 8:634897. doi: 10.3389/fnut.2021.634897
142. Stavropoulou E, Bezirtzoglou E. Probiotics in Medicine: A Long Debate. Front Immunol (2020) 11:2192. doi: 10.3389/fimmu.2020.02192
143. Bottari B, Castellone V, Neviani E. Probiotics and COVID-19. Int J Food Sci Nutr (2021) 72(3):293–9. doi: 10.1080/09637486.2020.1807475
144. Camilleri M. Human Intestinal Barrier: Effects of Stressors, Diet, Prebiotics, and Probiotics. Clin Transl Gastroenterol (2021) 12(1):e00308. doi: 10.14309/ctg.0000000000000308
145. Parada Venegas D, de la Fuente MK, Landskron G, González MJ, Quera R, Dijkstra G, et al. Short Chain Fatty Acids (SCFAs)-Mediated Gut Epithelial and Immune Regulation and Its Relevance for Inflammatory Bowel Diseases. Front Immunol (2019) 10:277. doi: 10.3389/fimmu.2019.00277
146. Pujo J, Petitfils C, Le Faouder P, Eeckhaut V, Payros G, Maurel S, et al. Bacteria-Derived Long Chain Fatty Acid Exhibits Anti-Inflammatory Properties in Colitis. Gut (2021) 70(6):1088–97. doi: 10.1136/gutjnl-2020-321173
147. Durmaz S, Kurtoğlu T, Barbarus E, Çetin NK, Yılmaz M, Rahman ÖF, et al. Probiotic Saccharomyces Boulardii Alleviates Lung Injury by Reduction of Oxidative Stress and Cytokine Response Induced by Supraceliac Aortic Ischemia-Reperfusion Injury in Rats. Braz J Cardiovasc Surg (2021) 36(4):515–21. doi: 10.21470/1678-9741-2020-0153
148. Karen M, Yuksel O, Akyürek N, Ofluoğlu E, Cağlar K, Sahin TT, et al. Probiotic Agent Saccharomyces Boulardii Reduces the Incidence of Lung Injury in Acute Necrotizing Pancreatitis Induced Rats. J Surg Res (2010) 160(1):139–44. doi: 10.1016/j.jss.2009.02.008
149. Takauji S, Konishi H, Fujiya M, Ueno N, Tanaka H, Sato H, et al. Polyphosphate, Derived From Lactobacillus Brevis, Modulates the Intestinal Microbiome and Attenuates Acute Pancreatitis. Dig Dis Sci (2021) 66(11):3872–84. doi: 10.1007/s10620-020-06747-9
150. Mantis NJ, Rol N, Corthésy B. Secretory IgA's Complex Roles in Immunity and Mucosal Homeostasis in the Gut. Mucosal Immunol (2011) 4(6):603–11. doi: 10.1038/mi.2011.41
151. Wan YD, Zhu RX, Bian ZZ, Sun TW. Effect of Probiotics on Length of Hospitalization in Mild Acute Pancreatitis: A Randomized, Double-Blind, Placebo-Controlled Trial. World J Gastroenterol (2021) 27(2):224–32. doi: 10.3748/wjg.v27.i2.224
152. Rose EC, Odle J, Blikslager AT, Ziegler AL. Probiotics, Prebiotics and Epithelial Tight Junctions: A Promising Approach to Modulate Intestinal Barrier Function. Int J Mol Sci (2021) 22(13):6729. doi: 10.3390/ijms22136729
153. Zhong Y, Cai D, Cai W, Geng S, Chen L, Han T. Protective Effect of Galactooligosaccharide-Supplemented Enteral Nutrition on Intestinal Barrier Function in Rats With Severe Acute Pancreatitis. Clin Nutr (2009) 28(5):575–80. doi: 10.1016/j.clnu.2009.04.026
154. He Y, Wu C, Li J, Li H, Sun Z, Zhang H, et al. Inulin-Type Fructans Modulates Pancreatic-Gut Innate Immune Responses and Gut Barrier Integrity During Experimental Acute Pancreatitis in a Chain Length-Dependent Manner. Front Immunol (2017) 8:1209. doi: 10.3389/fimmu.2017.01209
155. Pham VT, Calatayud M, Rotsaert C, Seifert N, Richard N, Van den Abbeele P, et al. Antioxidant Vitamins and Prebiotic FOS and XOS Differentially Shift Microbiota Composition and Function and Improve Intestinal Epithelial Barrier In Vitro. Nutrients (2021) 13(4):1125. doi: 10.3390/nu13041125
156. Gou S, Yang Z, Liu T, Wu H, Wang C. Use of Probiotics in the Treatment of Severe Acute Pancreatitis: A Systematic Review and Meta-Analysis of Randomized Controlled Trials. Crit Care (2014) 18(2):R57. doi: 10.1186/cc13809
157. Sharma B, Srivastava S, Singh N, Sachdev V, Kapur S, Saraya A. Role of Probiotics on Gut Permeability and Endotoxemia in Patients With Acute Pancreatitis: A Double-Blind Randomized Controlled Trial. J Clin Gastroenterol (2011) 45(5):442–8. doi: 10.1097/MCG.0b013e318201f9e2
158. Zhu Y, Mei Q, Fu Y, Zeng Y. Alteration of Gut Microbiota in Acute Pancreatitis and Associated Therapeutic Strategies. BioMed Pharmacother (2021) 141:111850. doi: 10.1016/j.biopha.2021.111850
159. Kaku N, Matsumoto N, Sasaki D, Tsuda K, Kosai K, Uno N, et al. Effect of Probiotics on Gut Microbiome in Patients With Administration of Surgical Antibiotic Prophylaxis: A Randomized Controlled Study. J Infect Chemother (2020) 26(8):795–801. doi: 10.1016/j.jiac.2020.03.008
160. Sornsenee P, Singkhamanan K, Sangkhathat S, Saengsuwan P, Romyasamit C. Probiotic Properties of Lactobacillus Species Isolated From Fermented Palm SAP in Thailand. Probiotics Antimicrob Proteins (2021) 13(4):957–69. doi: 10.1007/s12602-021-09754-y
161. Li YS. Rational Use of Antibiotics in Severe Acute Pancreatitis: Controversy and Progress. Zhonghua Yi Xue Za Zhi (2021) 101(30):2346–8. doi: 10.3760/cma.j.cn112137-20210307-00580
162. Soares FS, Amaral FC, Silva NLC, Valente MR, Santos LKR, Yamashiro LH, et al. Antibiotic-Induced Pathobiont Dissemination Accelerates Mortality in Severe Experimental Pancreatitis. Front Immunol (2017) 8:1890. doi: 10.3389/fimmu.2017.01890
163. Jacobs MC, Lankelma JM, Wolff NS, Hugenholtz F, de Vos AF, van der Poll T, et al. Effect of Antibiotic Gut Microbiota Disruption on LPS-Induced Acute Lung Inflammation. PLoS One (2020) 15(11):e0241748. doi: 10.1371/journal.pone.0241748
164. Leppäniemi A, Tolonen M, Tarasconi A, Segovia-Lohse H, Gamberini E, Kirkpatrick AW, et al. 2019 WSES Guidelines for the Management of Severe Acute Pancreatitis. World J Emerg Surg (2019) 14:27. doi: 10.1186/s13017-019-0247-0
165. Wittekamp BHJ, Oostdijk EAN, Cuthbertson BH, Brun-Buisson C, Bonten MJM. Selective Decontamination of the Digestive Tract (SDD) in Critically Ill Patients: A Narrative Review. Intensive Care Med (2020) 46(2):343–9. doi: 10.1007/s00134-019-05883-9
166. Bauer M. The Liver-Gut-Axis: Initiator and Responder to Sepsis. Curr Opin Crit Care (2022) 28(2):216–20. doi: 10.1097/mcc.0000000000000921
167. Secombe KR, Al-Qadami GH, Subramaniam CB, Bowen JM, Scott J, Van Sebille YZA, et al. Guidelines for Reporting on Animal Fecal Transplantation (GRAFT) Studies: Recommendations From a Systematic Review of Murine Transplantation Protocols. Gut Microbes (2021) 13(1):1979878. doi: 10.1080/19490976.2021.1979878
168. Ng SC, Kamm MA, Yeoh YK, Chan PKS, Zuo T, Tang W, et al. Scientific Frontiers in Faecal Microbiota Transplantation: Joint Document of Asia-Pacific Association of Gastroenterology (APAGE) and Asia-Pacific Society for Digestive Endoscopy (APSDE). Gut (2020) 69(1):83–91. doi: 10.1136/gutjnl-2019-319407
169. Ooijevaar RE, Terveer EM, Verspaget HW, Kuijper EJ, Keller JJ. Clinical Application and Potential of Fecal Microbiota Transplantation. Annu Rev Med (2019) 70:335–51. doi: 10.1146/annurev-med-111717-122956
170. El-Salhy M, Hatlebakk JG, Gilja OH, Bråthen Kristoffersen A, Hausken T. Efficacy of Faecal Microbiota Transplantation for Patients With Irritable Bowel Syndrome in a Randomised, Double-Blind, Placebo-Controlled Study. Gut (2020) 69(5):859–67. doi: 10.1136/gutjnl-2019-319630
171. Ding L, He C, Li X, Huang X, Lei Y, Ke H, et al. Efficacy and Safety of Faecal Microbiota Transplantation for Acute Pancreatitis: A Randomised, Controlled Study. Front Med (Lausanne) (2021) 8:772454. doi: 10.3389/fmed.2021.772454
172. Yin GF, Li B, Fan XM. Effects and Mechanism of Fecal Transplantation on Acute Lung Injury Induced by Lipopolysaccharide in Rats. Zhonghua Yi Xue Za Zhi (2019) 99(20):1582–7. doi: 10.3760/cma.j.issn.0376-2491.2019.20.013
173. Tang J, Xu L, Zeng Y, Gong F. Effect of Gut Microbiota on LPS-Induced Acute Lung Injury by Regulating the TLR4/NF-kB Signaling Pathway. Int Immunopharmacol (2021) 91:107272. doi: 10.1016/j.intimp.2020.107272
174. Yang C, Wang T, Chen J, He J, Li Y, Chen C, et al. Traditional Chinese Medicine Formulas Alleviate Acute Pancreatitis: Pharmacological Activities and Mechanisms. Pancreas (2021) 50(10):1348–56. doi: 10.1097/mpa.0000000000001931
175. Xu Q, Wang M, Guo H, Liu H, Zhang G, Xu C, et al. Emodin Alleviates Severe Acute Pancreatitis-Associated Acute Lung Injury by Inhibiting the Cold-Inducible RNA-Binding Protein (CIRP)-Mediated Activation of the NLRP3/IL-1β/CXCL1 Signaling. Front Pharmacol (2021) 12:655372. doi: 10.3389/fphar.2021.655372
176. Li J, Han J, Lv J, Wang S, Qu L, Jiang Y. Saikosaponin A-Induced Gut Microbiota Changes Attenuate Severe Acute Pancreatitis Through the Activation of Keap1/Nrf2-ARE Antioxidant Signaling. Oxid Med Cell Longev (2020) 2020:9217219. doi: 10.1155/2020/9217219
177. Ji C, Lu F, Wu Y, Lu Z, Mo Y, Han L, et al. Rhubarb Enema Increasing Short-Chain Fatty Acids That Improves the Intestinal Barrier Disruption in CKD May be Related to the Regulation of Gut Dysbiosis. BioMed Res Int (2022) 2022:1896781. doi: 10.1155/2022/1896781
178. Zou XP, Chen M, Wei W, Cao J, Chen L, Tian M. Effects of Enteral Immunonutrition on the Maintenance of Gut Barrier Function and Immune Function in Pigs With Severe Acute Pancreatitis. JPEN J Parenter Enteral Nutr (2010) 34(5):554–66. doi: 10.1177/0148607110362691
179. McClave SA. Factors That Worsen Disease Severity in Acute Pancreatitis: Implications for More Innovative Nutrition Therapy. Nutr Clin Pract (2019) 34 Suppl 1:S43–8. doi: 10.1002/ncp.10371
180. Zhao XL, Zhu SF, Xue GJ, Li J, Liu YL, Wan MH, et al. Early Oral Refeeding Based on Hunger in Moderate and Severe Acute Pancreatitis: A Prospective Controlled, Randomized Clinical Trial. Nutrition (2015) 31(1):171–5. doi: 10.1016/j.nut.2014.07.002
181. Sun JK, Mu XW, Li WQ, Tong ZH, Li J, Zheng SY. Effects of Early Enteral Nutrition on Immune Function of Severe Acute Pancreatitis Patients. World J Gastroenterol (2013) 19(6):917–22. doi: 10.3748/wjg.v19.i6.917
Keywords: severe acute pancreatitis-associated lung injury, intestinal microbiota, microbiota-gut-lung axis, bacterial translocation, pathogen-associated molecular patterns, immune imbalance
Citation: Wang Z, Li F, Liu J, Luo Y, Guo H, Yang Q, Xu C, Ma S and Chen H (2022) Intestinal Microbiota - An Unmissable Bridge to Severe Acute Pancreatitis-Associated Acute Lung Injury. Front. Immunol. 13:913178. doi: 10.3389/fimmu.2022.913178
Received: 05 April 2022; Accepted: 11 May 2022;
Published: 14 June 2022.
Edited by:
Hongpeng Jia, The Johns Hopkins Hospital, United StatesReviewed by:
Chhinder Sodhi, Johns Hopkins University, United StatesGang Liu, University of Alabama at Birmingham, United States
Yukihiro Yamaguchi, University of North Carolina at Chapel Hill, United States
Copyright © 2022 Wang, Li, Liu, Luo, Guo, Yang, Xu, Ma and Chen. This is an open-access article distributed under the terms of the Creative Commons Attribution License (CC BY). The use, distribution or reproduction in other forums is permitted, provided the original author(s) and the copyright owner(s) are credited and that the original publication in this journal is cited, in accordance with accepted academic practice. No use, distribution or reproduction is permitted which does not comply with these terms.
*Correspondence: Shurong Ma, c2h1cm9uZ21hOTRAMTYzLmNvbQ==; Hailong Chen, Y2hlbmhhaWxvbmdAZG11LmVkdS5jbg==
†These authors contributed equally to this work and share the first authorship