- Ocugen Inc., Malvern, PA, United States
The world has responded to the COVID-19 pandemic with unprecedented speed and vigor in the mass vaccination campaigns, targeted to reduce COVID-19 severity and mortality, reduce the pressure on the healthcare system, re-open society, and reduction in disease mortality and morbidity. Here we review the preclinical and clinical development of BBV152, a whole virus inactivated vaccine and an important tool in the fight to control this pandemic. BBV152, formulated with a TLR7/8 agonist adjuvant generates a Th1-biased immune response that induces high neutralization efficacy against different SARS-CoV-2 variants of concern and robust long-term memory B- and T-cell responses. With seroconversion rates as high as 98.3% in vaccinated individuals, BBV152 shows 77.8% and 93.4% protection from symptomatic COVID-19 disease and severe symptomatic COVID-19 disease respectively. Studies in pediatric populations show superior immunogenicity (geometric mean titer ratio of 1.76 compared to an adult) with a seroconversion rate of >95%. The reactogenicity and safety profiles were comparable across all pediatric age groups between 2-18 yrs. as in adults. Like most approved vaccines, the BBV152 booster given 6 months after full vaccination, reverses a waning immunity, restores the neutralization efficacy, and shows synergy in a heterologous prime-boost study with about 3-fold or 300% increase in neutralization titers against multiple SARS-CoV-2 variants of concern. Based on the interim Phase III data, BBV152 received full authorization for adults and emergency use authorization for children from ages 6 to 18 years in India. It is also licensed for emergency use in 14 countries globally. Over 313 million vaccine doses have already been administered in India alone by April 18th, 2022.
Introduction
Severe acute respiratory syndrome coronavirus 2 (SARS-CoV-2) has created a global emergency due to the rapid worldwide spread of coronavirus disease 2019 (COVID-19) (1). As of July 26, 2022, SARS-CoV-2 and its variants have caused more than 571 million infections and 6.38 million deaths, with the US alone accounting for 89.6 million cases and 1,018,073 deaths (covid19.who.int). In earlier SARS-CoV-2 variants about 15% of infected patients developed pneumonia and around 5% of patients developed more critical symptoms such as acute respiratory distress syndrome and multiple organ failure (2, 3). The Omicron variant (B.1.1.529) while being much more (2-5-fold) infective than the Delta (B.1.617.2) variant shows 2-5-fold less fatality (4), and caused significantly less morbidity, hospital admissions and requirement for oxygen supplementation in adults (5). However, the hospitalization rates for children were higher during the Omicron peak than the Delta peak and might be explained by a shift in the COVID-19 symptoms due to Omicron. Severe forms of COVID-19 are associated with lymphocyte dysfunction, monocyte and granulocyte abnormalities leading to cytokine storm with increased levels of IL-1β, IL-6, IL-2, IL-8, IL-17, IP10, MCP1, MIP1α, G-CSF, GM-CSF, and TNF-α. High levels of C-reactive protein (CRP), D-dimer, immunoglobulin G (IgG), and total antibodies are also observed in severe COVID-19 (6–9). However, reports investigating the correlation between high viral loads and COVID-19 severity vary from high to no statistical correlation (10–19).
More than 6.5 million globally surveilled SARS-CoV-2 sequences shared by the Global Initiative on Sharing All Influenza Data (GISAID), allow real-time tracking of SARS-CoV-2 mutations and new variants (20). While many of the SARS-CoV-2 mutations are expected to be either neutral or deleterious for the virus, a small proportion of these mutations alter the virulence, transmissibility, and viral interactions with host immunity, which confer a survival advantage to the virus. Most of these beneficial mutations have occurred in key areas of the SARS- CoV-2 immunodominant spike (S) protein leading to the emergence of five main variants of concern (VOC): Alpha (B.1.1.7) in the United Kingdom, Beta (B.1.351) in South Africa, Gamma (B1.1.28-P.1) in Brazil, Delta (B.1.617.2) in India, and Omicron (B.1.1.529) in Botswana and South Africa (21–26). This has resulted in a catastrophic impact on global efforts against the SARS-CoV-2 pandemic, including vaccinations.
Vaccine lessons learned from natural SARS-CoV-2 infection
Dysregulation of macrophages, neutrophils, Th17 cells, monocytes, basophils, eosinophils, megakaryocytes, and erythroid progenitor cells plays a role in severe COVID-19 disease (27–32). On the other hand, properly functioning CD4+, CD8+ cells, NK cells, and dendritic cells reduce the disease severity (33–37). For instance, in the lungs, tissue-resident memory CD8+ T cells are important for protection against repeated infection with respiratory viruses (33) and severe COVID-19 cases are also associated with CD8+ lymphopenia and Th17 polarization of T cells (34). Long-lasting B and T memory cells can persist in recovered individuals long after neutralizing antibody titers have waned and a good T follicular helper cell activation indicates a matured humoral immune response with specific memory B cells to rapidly respond to possible reinfection (38, 39). While the currently approved vaccines are based on a single spike protein epitope, the immunological memory after natural infection captures a diverse repertoire of SARS-CoV-2 epitopes for both B and T cell responses (40–42). Therefore, it is imperative to understand immune response to natural infection to better identify ‘correlates of protection’ against COVID-19. For instance, the nucleocapsid (N) protein is more conserved than S-protein in the coronavirus genus and one of the most abundant structural proteins in infected cells (43). N-protein levels were reported to regulate the severity of immune response to SARS-CoV-2 (44). Most of the recovered COVID-19 patients presented a stronger specific immune response against the SARS-CoV-2 N-protein and its fragments than the Receptor Binding Domain (RBD) of the S-protein (45). Patient sera during both the acute and the convalescent phase of COVID-19, showed IgM and IgG specific reactivity to N- and C-termini of the membrane (M) protein of SARS-CoV-2 (46). Antibodies against the M-protein show similar levels of reactivity as antibodies against the immunodominant epitopes of S or N proteins, but none of the vaccines approved in the US target the M-protein. Since emerging Variants of Concern (VOCs) show several mutations in the S- proteins, an in-depth study of M-protein as a vaccine target is required to move the evolutionary pressure away from S-protein. Using both S and N protein as vaccines have shown better virus neutralization at distal tissues sites (away from the primary respiratory site of infection) (47). With the emergence of the Omicron variant, the efficacy of single target (S-protein) mRNA vaccines has been wanning (48). The mRNA vaccine specifically targeting the omicron S-protein (mRNA-Omicron) did not perform better than the mRNA-1273 vaccine targeting the ancestral SARS-CoV-2 S-protein (49–53). These data suggest that targeting multiple SARS-CoV-2 proteins (S, N, M) would better replicate the natural COVID-19 infection and might trigger better protection against current and future SARS-CoV-2 variants.
BBV152
Multiple vaccines for SARS-CoV-2 are highly effective in reducing the COVID-19 pandemic burden. However, to meet the global need for billions of COVID-19 vaccine doses, a collective effort to identify, evaluate, validate, and manufacture effective vaccines is paramount. BBV152 is a whole-virion inactivated SARS-CoV-2 vaccine generated using the Asp614Gly (B.1.1.7) variant. BBV152 is the first vaccine formulated with a toll-like receptor 7/8 (TLR7/8) agonist molecule, imidazoquinoline gallamide (IMDG), adsorbed to alum and approved for use in a large population (54) (Figure 1). This vaccine is administered as two intramuscular 6µg doses, 4 weeks apart. In Phase I/II clinical trial, 98.3% of the candidates administered BBV152 developed antibodies against SARS-CoV-2 (seroconverted) (55). In a corresponding Phase III trial, BBV152 protected up to 77.8% of vaccinated candidates against symptomatic infection, 93.4% from severe disease, and 63.6% from asymptomatic disease (56).
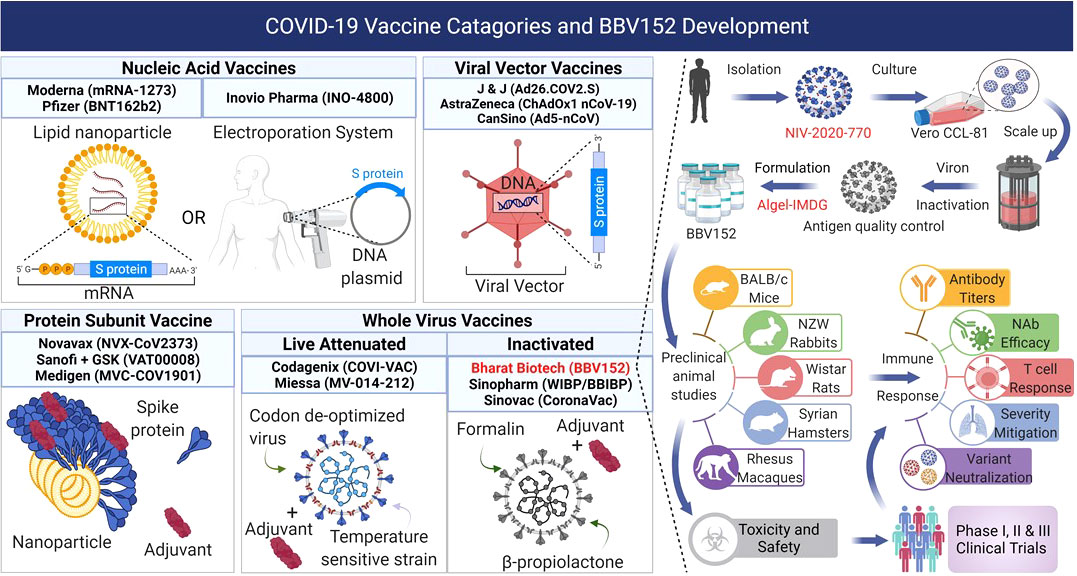
Figure 1 COVID-19 vaccines in development, clinical trials, or approved use, fall into four main categories: 1) Nucleic acid vaccines that use RNA or DNA to provide host cells with the instructions to make the viral antigens. 2) Viral vector vaccines use replicating or non-replicating, non-pathogenic viruses to deliver the genetic instructions to the host cells to produce antigens. 3) Protein subunit vaccines use fragments of antigens as proteins or assembled virus-like nanoparticles delivered directly into the host. 4) Whole virus vaccines use the complete virus that is either attenuated or completely inactivated. BBV152 is a whole virus inactivated vaccine produced from the NIV-2020-770 strain of SARS-CoV-2 strain by destroying the viral genetic material while leaving the antigens intact. The inactivated virus is formulated with the Algel-IMDG adjuvant (TLR7/8 agonist) and used for preclinical animal studies for safety and immunogenicity followed subsequently by Phase I, II, and III clinical trials to evaluate its safety and efficacy in human participants. Figure created with BioRender.com.
Preclinical development
Inactivated vaccines against viral diseases have well-established safety profiles and have been licensed and used for decades (57). The Vero cell vaccine manufacturing platform is well-characterized with proven safety in other licensed, live, and inactivated vaccines such as Ervebo (Ebola), Vepacel (Influenza), IMOVAX (Polio), VERORAB (Rabies), RotaTeq (Rotavirus) and ACAM2000 (Smallpox) (58–62). The BBV152 vaccine was developed from the SARS-CoV-2 strain (NIV-2020-770 - GISAID sequence EPI_ISL_420545 – G-clade) using the Vero CCL-81 system for sample propagation and virus isolation (Figure 1) (63–65). Inactivation of the β-propiolactone treated live virus was assessed by viral cytopathic activity and amplification while intact coronaviral morphology was verified by transmission electron microscopy, and the presence of intact S (S1, S2, and receptor binding (RBD) domains) and N-protein antigens was verified by Western blots and by immunoelectron microscopy (66).
Vaccine-induced disease enhancement due to Th2-like immunity is a concern for patients vaccinated against SARS-CoV-2 (Figure 2) (67). Several studies show that patients with higher antibody titers against SARS-CoV-2 were associated with higher antigen levels (viral loads) and more prolonged exposure leading to more severe disease symptoms (14, 19, 68). Patients with mild and asymptomatic COVID-19 infections show high levels of anti-SARS-CoV-2 T-cell (Th1) responses (69, 70). These data suggest that strong Th1 responses correlate with mild clinical presentations, whereas strong Th2 responses correlate with severe COVID-19. BBV152 circumvents this Th2 bias using a new IMDG (TLR7/8 agonist) class adjuvant that induces strong type I interferon responses from antigen-presenting cells such as dendritic cells and monocytes-macrophages leading to the development of a Th1 cell-based immunity instead of a pathogenic Th2 humoral immunity (Figure 2) (71). Like the mRNA COVID-19 vaccines, BBV152 also induces a strong CD8 T cell response in vaccinated individuals (72, 73). Preclinical BBV152 immunogenicity and safety studies done on multiple animal models are summarized in Table 1. The maximum tolerated dose of TLR7/8 agonist (IMDG) in Algel-IMDG formulation was 30 ug/animal in mice and rats (76). The BBV152-Algel-IMDG formulations were evaluated as safe in mouse, rat, and rabbit models (Figure 1) by repeated-dose toxicity, mutagenicity, histopathology, and by clinical pathology investigations such as hematology, clinical biochemistry, coagulation parameters, and urinalysis monitoring. Necropsy with organ histopathology revealed no significant difference between animals treated with antigen alone, adjuvants alone, or adjuvanted vaccine. Animals administered adjuvanted formulations showed local inflammatory changes characterized by mild infiltration of mononuclear cells and the presence of macrophages at the injection site (76). BBV152 immunogenicity was tested in BALB/c mice, Wistar rats, and in New Zealand white rabbits on days 0, 7, and 14. The respective sera were evaluated for antibody binding by ELISA and efficacy of live SARS-CoV-2 neutralization by plaque reduction assays. Animals administered BBV152 (adjuvanted vaccine) showed higher antibody binding (to S1, RBD, and N proteins) and higher neutralizing antibody (NAb) titers than those administered the antigen alone (76). High IgG2a/IgG1 in day 21 serum samples, higher levels of interferon-γ (IFNγ), IL-2, 4, 6, 17A, TNFα, and higher populations of CD4 Tfh-cells (follicular helper) suggest that Algel-IMDG (TLR7/8 agonist) adjuvant induces Th1 biased, T-cell mediated, protective immunity (66, 76).
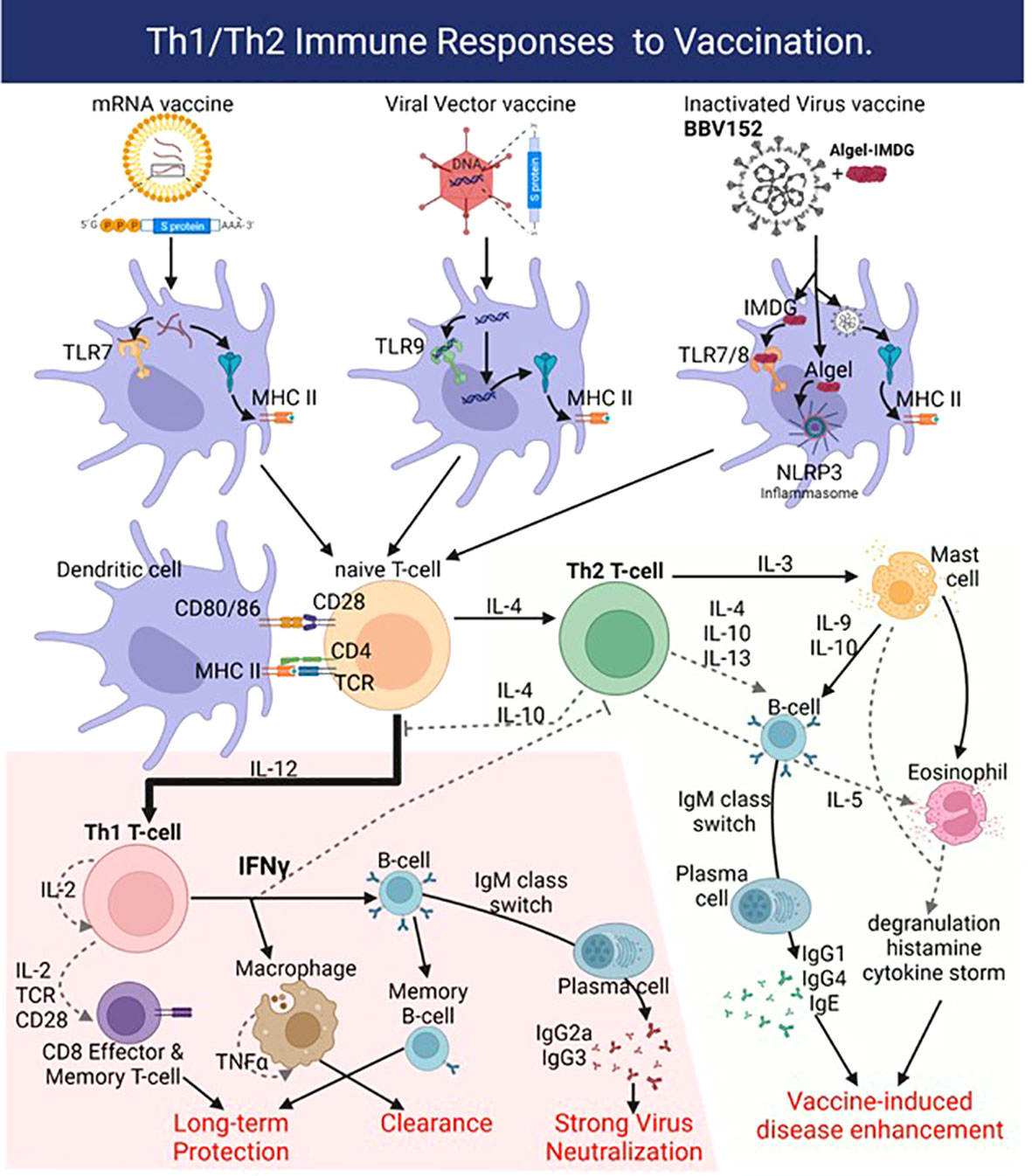
Figure 2 Vaccines deliver the SARS-CoV-2 antigen into dendritic cells (DC) using lipid nanoparticles (mRNA vaccines), adenoviral vectors, or in the case of BBV152 by using the inactivated SARS-CoV-2 virus. Adjuvant activity of the vaccines triggers innate sensors like the mRNA sensor Toll-like receptor 7 (TLR7) or double-strand DNA sensor TLR9 for mRNA and Viral Vector vaccines, respectively. The Algel-IMDG adjuvant in BBV152 activates TLR7/8 (IMDG) and the NLRP3 inflammasome (Algel). The resulting production of IL-12 drives naïve T-cells towards the pro-inflammatory Th1-biased response that generates strongly neutralizing antibodies (IgG1, IgG3 subtypes) and virus-specific CD8 effector T-cells to clear the infection. Generation of viral antigen-specific memory B- and CD8 T-cells affords long-term protection. Production of IL-4 however drives a Th2-biased response with high titers of IgG4 and IgE, along with recruitment and activation of mast cells and eosinophils, which causes vaccine-induced disease enhancement or antibody-dependent enhancement. Figure created with BioRender.com.
BBV152 immunogenicity and protection in Syrian hamsters and rhesus macaques
Among various small laboratory animal models, Syrian hamsters were found to be a suitable model for SARS-CoV-2 research, as the virus has been shown to replicate both in the upper and lower respiratory tract (77) (Table 1). Syrian hamsters (n=9/group) immunized with phosphate-buffered saline (PBS-control), BBV152-Algel or BBV152-Algel-IMDG (3 µg or 6 µg) on days 0, 14, and 35 and inoculated on day 50 with intranasal SARS-CoV-2 (NIV-2020-770) median tissue culture infectious dose (TCID50) of 105.5 (74). By 21 days post-immunization, hamsters receiving BBV152 with Algel-IMDG adjuvant produced significantly higher NAbs than the Algel alone group.
BBV152 vaccinated hamsters showed rapid clearance of SARS-CoV-2 from lungs, trachea, nasal turbinates, and extra-pulmonary tissues while also showing lower inflammation mediated alveolar damage, hemorrhages, inflammatory cell infiltration, hyaline membrane formation, and eosinophilic edematous exudate than the PBS-treated control group (Figure 1) (74). A similar assessment of immunogenicity of the three BBV152 formulations in rhesus macaques (75) (Table 1) showed protective efficacy against SARS-CoV-2 pneumonia and preserved the normal histology of the lungs. BBV152-treated macaques showed normal post-infection oxygen saturation (SpO2), lower levels of pro-inflammatory cytokine IL-6, and protection against post-infection CD8 T-cell lymphopenia. The lung histopathology of BBV152-treated animals showed no signs of eosinophilic infiltration, indicating no vaccine-enhanced disease (Figure 1) (75).
BBV152 safety phase I trial
BBV152 safety and immunogenicity in humans were first evaluated in a double-blind, multicenter, randomized, controlled Phase I trial (ClinicalTrials.gov: NCT04471519) (Table 2). Individuals with positive SARS-CoV-2 nucleic acid and/or serology tests were excluded. Among the 375 healthy adults aged 18 to 55 years at 11 hospitals across India, three groups of 100 were randomly assigned to receive either one of three BBV152 formulations (3 or 6 µg with Algel-IMDG or 6 µg with Algel), and 75 were assigned to an Algel only control vaccine group. Intramuscular vaccine/control doses were administered on the day of randomization and again on day 14. As the primary outcome, solicited local and systemic adverse reactions were reported by 17 (17%; 95% CI, 10.5–26.1) participants in the 3 µg BBV152-Algel-IMDG group, 21 (21%; 13.8–30.5) in the 6 µg BBV152- Algel-IMDG group, 14 (14%; 95% CI, 8.1–22.7) in the 6 µg BBV152-Algel group, and 10 (10%; 95% CI, 6.9–23.6) in the Algel-only group. The most common solicited adverse events were injection site pain (17 [5%] of 375 participants), headache (13 [3%]), fatigue (11 [3%]), fever (nine [2%]), and nausea or vomiting (seven [2%]) (Table 3). All solicited adverse events were mild (43 [69%] of 62) or moderate (19 [31%]) and were more frequent after the first dose. BBV152 immunogenicity was measured as the study’s secondary outcome using both IgG levels (Anti-S1, Anti-RBD, and Anti-N IgG1) and appearance of NAbs from baseline to days 14, 28, 42, 104, and 194. Seroconversion rates were 87.9, 91.9, and 82.8% in the 3 µg with Algel-IMDG, 6 µg with Algel-IMDG, and 6 µg with Algel groups, respectively. Analysis performed on randomly selected blood samples at one study site, showed enhanced CD3, CD4, and CD8 T-cell (Th1) activation measured by IFN-γ ELISpot responses against SARS-CoV-2 in both Algel-IMDG vaccinated groups on day 28 (54) (Table 2).
BBV152 immunogenicity phase II trial
Following the tolerable safety outcomes and enhanced immune responses for BBV152 reported in the Phase I trial, both Algel-IMDG formulations were selected for a double-blind, randomized, multicenter Phase II trial in healthy adults and adolescents (aged 12 to 65 years) at 9 hospitals in India. After excluding participants already positive for SARS-CoV-2 (by serology or RT-PCR), 380 candidates were randomly assigned (1:1) to receive either 3 µg or 6 µg BBV152 with Algel-IMDG. As the primary study outcome, the group receiving 6 µg BBV152 with Algel-IMDG showed significantly higher titers of anti-SARS-CoV-2 NAbs and 98.3% [95% CI, 95.1–99.6] seroconversion rates (defined as a post-vaccination titer that was at least four-fold higher than the baseline) (55). IgG antibodies to all epitopes (S1 glycoprotein, RBD, and N protein) were detected (Table 2). Secondary outcomes revealed a Th1-biased response induced in both groups, measured by cytokine response (high Th1 cytokines - IFNγ, IL-2, and TNFα and low Th2 cytokines - IL-5, IL-10, and IL-13) at 2 weeks after the second vaccine dose (day 42). Additionally, BBV152 induced CD4+ CD45RO+ memory T-cell responses with elevated co-stimulatory marker CD27 indicating the activation of the antigen recall memory T-cell response against SARS-CoV-2 (55). Candidates with an existing co-infection were excluded to avoid confounding the vaccine generated immune responses with Th2/Th17 immune responses to other pathogens. No serious adverse events were reported in the study. Most adverse events were mild and resolved within 24 hours of onset. No association between the dose of vaccine (3 µg or 6 µg BBV152) and the number of adverse events was observed. The most common solicited adverse event was injection site pain (Table 3).
BBV152 efficacy phase III trial
In the Phase II trial, BBV152 showed better immunogenicity, safety, and enhanced Th1 biased anti-SARS-CoV-2 immune responses compared to the Phase I trial. Therefore the 6 µg BBV152 with Algel-IMDG formulation was selected for the double-blind, randomized, controlled Phase III efficacy trial (Clinicaltrials.gov: NCT04641481). This study recruited 25798 participants between November 16, 2020, and January 7, 2021, of which 24419 were randomized to receive two doses of BBV152 (n = 12,221) or placebo (n = 12,198). The primary outcome was to assess the efficacy of BBV152 in preventing symptomatic COVID-19 (confirmed by RT-PCR) in a case-driven manner, along with sub-group analyses of asymptomatic and symptomatic efficacy. In a follow-up, at least two weeks after the second vaccination, 130 (0.77%) cases of symptomatic COVID-19 were reported among 16,973 participants; 24 in the BBV152 group and 106 in the placebo group, bringing the overall vaccine efficacy to 77.8% (95% CI: 65.2–86.4) (Table 2). One candidate from the vaccine group and 15 from the placebo group developed severe COVID-19 symptoms, giving BBV152 a protective efficacy of 93.4% against severe disease, while the efficacy against asymptomatic COVID-19 was 63.6% (95% CI, 29.0–82.4) (56). BBV152 protection in elderly (>60 yrs.) candidates was 67.8% (95% CI, 8.0–90.0) and in participants younger than 60 years was 79.4% (95% CI, 66.0–88.2). BBV152 was well tolerated with no differences in the distributions of solicited, unsolicited, or serious adverse events between vaccine and placebo groups. No cases of anaphylaxis or vaccine-related deaths were reported (Table 3).
BBV152 against variants of concern
The rapid surge of SARS-CoV-2 cases due to the five variants of concern has resulted in a catastrophic impact on global efforts against the SARS-CoV-2 pandemic including vaccinations. Alpha (B.1.1.7), Beta (B.1.351), Gamma (B.1.1.28-P.1), Delta (B.1.617.2), and Omicron (B.1.1.529) variants pose serious public health concerns due to higher transmissibility, immune escape, and disease severity (Figure 3). The root of such concerns is due to the structure of these variants compared with the other known SARS-CoV-2 lineages. The Alpha (B.1.1.7) variant carries 8 mutations in spike-RBD, specifically N501Y, which enhances viral attachment to angiotensin-converting enzyme 2 (ACE2) on human cells (78). The Beta (B.1.351) variant has additional E484K and K417N mutations on the RBD, the Gamma (B.1.1.28) variant has a new L452R spike mutation plus two new mutations A119S and M234I in the N-protein, while the D614G, T478K, and P681R mutations were of concern in the Delta (B.1.617.2) variant (21, 23, 79, 80). The heavily mutated Omicron (B.1.1.529) variant spike shows 30 amino acid changes, three deletions, one insertion in the spike-RBD protein, and 3 mutations at the furin cleavage site that increase its infectivity (Figure 3) (81–84). Recent studies suggest that since most of the vaccine candidates are either recombinant or specifically target a single epitope of the original spike sequence, their immune response might not be as efficient against the new variants (85–89). An open-label, clinical intervention trial study done on the 4th dose of mRNA vaccines (Pfizer - BNT162b2 or Moderna - mRNA1273) reported the vaccine efficacy against Omicron infection at 30% (95%CI, -9% to 55%) and 11% (95%CI, -43% to +43%) for BNT162b2 and mRNA1273, respectively while local and systemic adverse reactions were reported in 80% and 40% recipients, respectively (48).
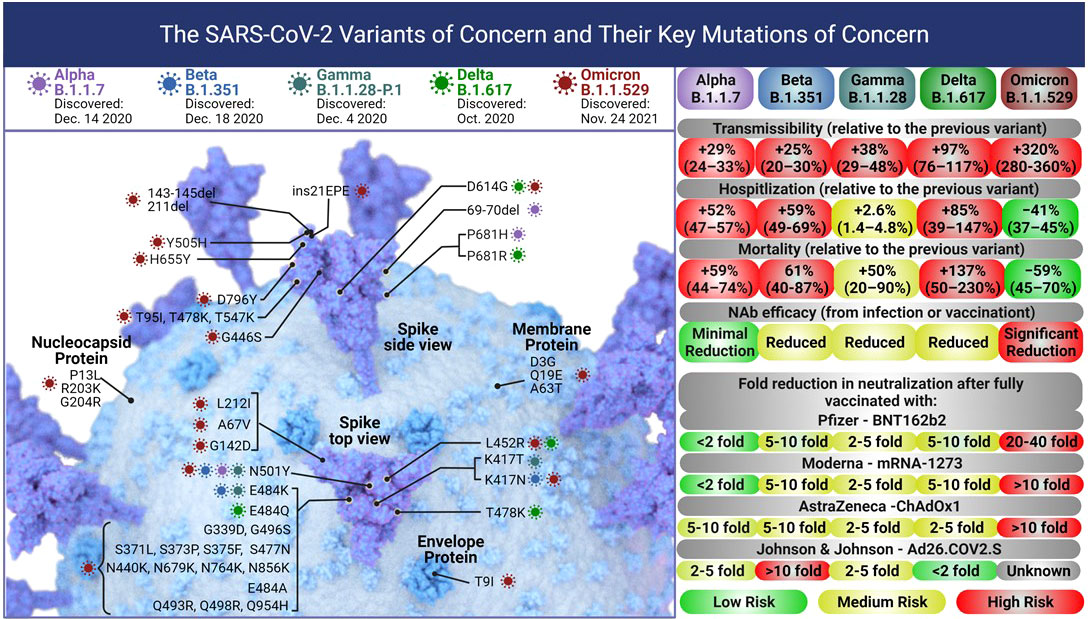
Figure 3 Each of the five SARS – CoV-2 variants of concern accumulated mutations in their Spike protein leading to changes in their transmissibility, and severity of infections leading to hospitalization or death. The current globally dominant variant, Omicron (B.1.1.529) has more than 50 mutations including 32 on the Spike protein and 15 on the RBD alone. These mutations have significantly increased the transmissibility of Omicron over the Delta variant. The severity (hospitalization and death) due to the Omicron variant is lower than Delta, however, the vaccine efficacy against Omicron drops significantly. Figure created with BioRender.com.
Sera from selected BBV152 vaccinated candidates of Phase I/II clinical trials (ClinicalTrials.gov: NCT04471519) were tested against the five VOC and the vaccine strain D614G, using plaque reduction neutralization test (PRNT50) assays, with the seroconversion rates of neutralizing antibodies being 98.6% (90). The sera from BBV152-vaccinated individuals could neutralize Alpha (B.1.1.7) (PRNT50-GMT: D614G = 700, Alpha = 670) (91) and Gamma (B.1.1.28.2) variants (PRNT50-GMT: D614G = 337.5, Gamma= 175.7) (92) with similar efficacy as D614G. For Beta (B.1.351) neutralization efficacy dropped 3-fold compared to D614 (PRNT50-GMT: D614G = 187.5, Beta= 61.57) (93). Delta (B.1.617.2) and Omicron (B.1.1.529) variants were neutralized at 1.5and 9.4-fold lower efficacy than D614G (PRNT50-GMT: D614G=706, Delta=480, Omicron=75) (94). All BBV152 boosted candidates showed neutralizing activity against the Delta variant, while over 90% showed neutralizing activity against the Omicron variant (94). Similar studies using higher drop in neutralization efficacy was observed with mRNA vaccines like BNT162b2 (Pfizer) (6.5-fold) and mRNA-1273 (Moderna) (8.6-fold) while the viral vector vaccine ChAdOx1 nCoV-19 (Astra Zeneca) showed an 86-fold reduction in efficacy against Beta (B.1.351) variant (21, 95, 96). Similarly, reduced Delta (B.1.617.2) variant neutralization was observed with the sera of BNT162b2 mRNA and single-dose ChAdOx1 nCoV-19 vaccinated individuals (79).
Protection with BBV152 homologous/heterologous prime-boost vaccination
BBV152 Phase I/II clinical trials reported an acceptable safety profile and enhanced Th1 (IFNγ producing CD4+ T-cells) and humoral responses (54, 55, 90). Based on the interim Phase III data, BBV152 was approved for use in India with over 313 million vaccine doses already administered by April 18th, 2022 (https://dashboard.cowin.gov.in/). Long-term immune responses can be enhanced by inducing trained immunity and down-regulating innate immune tolerance (97–99). To dissect the systemic immune responses induced by BBV152, the plasma levels of a panel of cytokines and chemokines were measured in 44 prime-boost vaccine recipients (100). While the first dose of the vaccine does not induce any significant changes in plasma cytokine levels, the second BBV152 dose enhanced plasma levels of Th1 (IFNγ, IL-2, TNFα), and Th2 (IL-4, IL-5, IL-10, and IL-13) cytokines as well as IL-17A and other pro-inflammatory cytokines (Figure 2). This was accompanied by elevated chemokines CCL4, CXCL1, CXCL2, and CX3CL1. A strong Th1 response correlates with milder COVID-19 symptoms (14, 19, 68). Although this study does not measure vaccine or ligand-specific immune responses, the induction of systemic cytokines indicates a robust systemic immune response following the BBV152 booster dose.
A retrospective cohort study at the All-India Institute of Medical Sciences measured the rate of reinfection (tested by RT-PCR or rapid antigen) in BBV152-vaccinated health care workers during the Delta variant surge in India (101). The 1917 health care workers were divided into 3 groups: unvaccinated (n = 472), partially vaccinated (n = 356), and fully vaccinated (n = 1089). Among the unvaccinated group, SARS-CoV-2 reinfection was observed in 60 of 472 (12.7%) with an incidence density (ID) of 18.05 per 100 person-years (95% CI, 14.02-23.25). Reinfection among partially vaccinated individuals was 39 of 356 (11.0%) with an ID of 15.62 per 100 person-years (95% CI, 11.42-21.38), and among fully vaccinated individuals was 17 of 1089 (1.6%) with an ID of 2.18 per 100 person-years (95% CI, 1.35-3.51). The estimated effectiveness of BBV152 against reinfection was 86% (95% CI, 77%-92%) in fully vaccinated individuals, with their IDs of 0.08 (95% CI, 0.01-0.55) and hospitalization rates of 0.13 (95% CI, 0.01-1.28) (101). A similar study was conducted in New York state to assess the effectiveness of the BNT162b2 (Pfizer), mRNA-1273 (Moderna), and Ad26.COV2.S (Johnson & Johnson) vaccines (102). During the week of August 28, 2021, when the Delta variant accounted for 99.6% of all COVID-19 infections in New York state, the median vaccine effectiveness against reinfections was 77.8% (95% CI, 70.1 - 86.8) for mRNA-1273 followed by 72.3% (95% CI, 63.7 - 77.5) for BNT162b2 and 69.4% (95% CI, 63.4 - 77.3) for Ad26.COV2.S. The combined median effectiveness against infection in fully vaccinated people during this Delta surge was 74.2% (95% CI, 63.4 - 86.8) and the effectiveness against hospitalizations was more than 90% (102). Therefore BBV152 showed equivalent protection levels as BNT162b2, mRNA-1273, and Ad26.COV2.S vaccines against breakthrough infections and hospitalizations in fully vaccinated individuals.
Heterologous prime-boost using different vaccine products allows for flexibility in cases of unpredictability or lack of supply of the same vaccine and may enhance vaccine effectiveness by reducing reactogenicity and enhancing the net vaccine effectiveness (103). A retrospective cohort study compared the virus neutralization (NAb) profiles of BBV152 and ChAdOx1-nCov-19 homologous prime-boosted individuals to those that were heterologous prime-boosted (first ChAdOx1-nCov-19 dose followed by second BBV152 dose) by PRNT50 assays (104). The geometric mean titer of NAbs against the B.1, Alpha, Beta and Delta variants for BBV152 were 162 (95% CI, 76.74-342), 122.7 (95% CI, 59.36-253.7), 48.43 (95% CI, 19.71-119) and 51.99 (95% CI 19.65-137.6) in the ChAdOx1-nCov-19 group and 156.6 (95% CI, 105.2-233.1), 112.4 (95% CI, 76.56-164.9), 52.09 (95% CI, 34.9-77.73) and 54.37 (95% CI, 27.26-108.4) in the BBV152 group. However, the sera of the heterologous group had roughly 3-fold higher NAb titers of 539.4 (95% CI, 263.9-1103), 396.1 (95% CI, 199.1-788), 151 (95% CI, 80.21-284.3), and 241.2 (95% CI, 74.99-775.9) respectively against B.1, Alpha, Beta and Delta variants (104). They also concluded that the heterologous group had reduced or similar reactogenicity and adverse events. A similar study by Atmar et al. concluded that the vaccination efficacies for Ad26.COV2.S followed by BNT162b2 or mRNA-1273 were 10 to 20-fold higher than Ad26.COV2.S homologous prime-boost vaccination with at par reactogenicity (105). It would be interesting to study if a dissimilar vaccine like BBV152 (the whole virus inactivated) would synergize the efficacies of mRNA vaccines like BNT162b2 or mRNA-1273 in the future heterologous prime-boost studies.
Impact of a third BBV152 booster dose on the persistence of anti-SARS-CoV-2 immunity
Multiple studies report the decline in anti-SARS-CoV-2 NAb titers within 2-6 months post-infection or immunization (106). The emergence of VOCs as the dominant infectious strains raises further concerns about the durability of vaccine responses since the level of NAb titers protective against the original strain might not be protective against VOCs (Figure 3) (107–110). With an increase in breakthrough infections by the highly transmissible Omicron (B.1.1.529) variant, it is vital to understand the role of NAb persistence in long-term vaccine efficacy (102, 111). The impact of a third BBV152 dose was tested on 184 randomly selected candidates of an ongoing BBV152 Phase II trial (ClinicalTrials.gov: NCT04471519). Candidates were divided into placebo (n= 93) or 6 µg BBV152-Algel-IMDG (n=91) groups and the dose was administered on day 215 (approximately 6 months) after the primary dose. Geometric mean NAb titers (PRNT50) induced 4 weeks after the second BBV152 dose were 197.0 (95% CI, 155.6–249.4) and reduced to 23.9 (95% CI, 14.0–40.6) by six months. Four weeks after the third (booster) BBV152 dose, the NAb titers increased to 746.6 (95% CI, 514.9–1081) compared with 100.7 (95% CI, 43.6–232.6) in the placebo group with seroconversion rates being 98.7% (95% CI, 92.8–99.9) and 79.8% (95% CI, 69.6–87.8), respectively. The third BBV152 dose led to increased NAb titers against VOCs such as Alpha (32.6-fold), Beta (161-fold), and Delta (264.7-fold). The BBV152 third dose also induced memory B-cells, central and effector memory CD4 T-cells, and cytotoxic effector memory CD8 TTEMRA cells (112). A more recent study compared the effect of the BBV152 booster (third) dose given 215 days after the second BBV152 dose in fully vaccinated individuals. The booster dose recipients had 19.11 (P < 0.0001), 14.70 (P = 0.0002), 16.51(P < 0.0001) and 18.53 (P = 0.0002) fold higher NAb titers against B.1, Beta, Delta and Omicron, respectively (113). Thus, the booster dose of Covaxin robustly triggered NAb responses and efficiently neutralized the multiple VOCs of SARS-CoV-2
BBV152 pediatric study
In 2020 at the onset of the pandemic, in adults, COVID-19 infections in children were not associated with severe symptoms requiring hospitalization or ventilation support, and pediatric deaths due to COVID-19 were limited to children with underlying chronic medical conditions (114, 115). Although asymptomatic during active COVID-19 infections, children have shown similar viral loads as adults and could be the source of ongoing infections (116–119). The new VOC have almost entirely replaced the original SARS-CoV-2 strain leading to higher rates of symptomatic and more severe COVID-19 in children (120). The failure to decrease infection rates in the ongoing pandemic has been associated with an increasing burden of infections in children (121). Therefore, several countries have now considered including children in their COVID-19 vaccination programs (122–124), making it a priority to develop new vaccines or establish the safety of current vaccines in children. A Phase II/III open-label, multi-center age de-escalation study was performed across six hospitals in India, in three pediatric age cohorts (n=526): ≤18->12years (group 1: n=176), ≤12->6 years (group 2: n=175) and ≤6->2 years (group 3: n=175) (125). No severe adverse events were reported with mild to moderate fever being the most frequent systemic events reported after the primary dose (group1- 5%, group2 – 10% & group3 – 13%), decreasing to 4% or less after the booster dose in all groups. Seroconversion rates in all 3 groups were equivalent to those seen in the adult population: group1 = 94.9% (95% CI, 90.5-97.5), group2 = 98.2% (95% CI, 94.9-99.6), and group 3 = 98.3% (95% CI, 95.0-99.6). All three pediatric groups showed 2-fold higher titers of NAbs (by PRNT50) than adults and IgG1:IgG4 ratios > 1 indicative of a Th1-biased robust anti-SARS-CoV-2 immune response (125). Both vaccine and placebo reactogenicity were similar and no severe adverse events were reported. Based on these findings BBV152 received emergency use listing in India, for children 11-18 years old in December 2021 and for children 6-11 years old in April 2022.
Conclusions
BBV152 is an exciting player in the Anti-SARS-CoV-2 vaccination landscape. It is a whole-virion inactivated SARS-CoV-2 vaccine generated against the B.1.1.7 variant and administered with the TLR7/8 agonist molecule – Algel-IMDG, to induce a Th1-biased immune response. In contrast to mRNA or viral vectored vaccines which express the Spike protein as the only antigen target, inactivated - purified BBV152 particles show intact coronaviral morphology and induce antibody responses against both the Spike and the Nucleocapsid proteins. BBV152 was safe in preclinical animal models such as BALB/c mice, Wistar rats, New Zealand rabbits, Syrian hamsters, and rhesus macaques, and generated protective NAbs plus CD4/CD8 T-cell responses (66, 74, 75). BBV152-vaccinated animals not only cleared the viral challenge but were also protected from COVID-19 pneumonia and associated irreversible damage to other organs. The BBV152 Phase I, II, and III trials established not only its safety in humans but also its efficacy at engaging CD4 and CD8 T-cell responses in addition to producing protective NAb titers. BBV152 protected up to 78% of vaccinated candidates against symptomatic infection, 93.4% from severe disease, and 63.6% from asymptomatic disease (56). Additionally, sera from BBV152 vaccinated candidates in Phase I/II clinical trials could neutralize all five VOCs as measured by PRNT50 assays. However, as reported with other vaccine candidates this neutralization efficacy was reduced with Delta and Omicron variants (91–94).
Studies show that SARS-CoV-2 vaccinations at $35 per dose even at 60% efficacy are considerably cost effective ($8,200 per quality adjusted life-year (126). As the highly transmissible Omicron (B.1.1.529) variant becomes dominant globally, the protective NAb titers from initial vaccination are proving to be inadequate in preventing breakthrough infections (102, 111). A third BBV152 booster, 6 months after the prime vaccine dose, not only reverses the decline in the NAb titers with time but also leads to a significant increase in NAb titers which might provide better protection against multiple VOC especially the Omicron variant (113). The BBV152 third dose also induced memory B-cells, central and effector memory CD4 T-cells, and cytotoxic effector memory CD8 TTEMRA cells (112). Perhaps one of the most interesting pieces of data to emerge from the coordinated global vaccination efforts is the possibility to enhance the immune response by heterologous prime-boost vaccination. The WHO Strategic Advisory Group of Experts on Immunization (SAGE) reviewed the evidence and issued the following interim guidance: Although the direct evidence is limited due to the multiplicity of different vaccine combinations, heterologous schedules have consistently shown enhanced immunogenicity when inactivated virus vaccines were administered either before or after vectored or mRNA vaccines (103, 104, 127). Certain key gaps in the evidence to be addressed include: 1) effectiveness and duration of protection of heterologous versus homologous vaccine schedules, especially for heterologous schedules involving inactivated vaccines; 2) long-term safety, immunogenicity, and effectiveness of heterologous vaccination, and surveillance for rare adverse events; 3) the ideal time interval between the primary series and booster dose; 4) correlates and duration of protection for homologous and heterologous schedules; and 5) immunogenicity and efficacy of heterologous and homologous vaccine schedules against VOC like the Omicron variant.
Challenges and the path forward
The global vaccine campaigns, a testament to human innovation and dedication have encountered and overcome several challenges ranging from scientific, logistical, societal, and marketing (Figure 4). Key implementation parameters like the manufacturing and distribution speed and the extent of vaccine delivery in a population plays just as critical a role in vaccination success as the efficacy of the vaccine itself. In the US, Operation Warp Speed was critical for early vaccination efforts by infusing investments in vaccine development, manufacturing, and distribution, however reaching vaccine-hesitant and underserved groups limited the success of the vaccination program. Globally in underserved areas, efforts to design and implement strategies on supply chain and distribution are still desperately needed (128).
Almost all SARS-CoV-2 vaccines being developed require prime-boost vaccination with protective efficacies declining by 6 months. The global vaccination endeavors face additional challenges from the emergence of VOCs that might require higher NAb titers for protection. To fulfill the global demand would therefore require pharma companies to supply billions of doses annually and the governments to develop effective prioritization strategies. Nucleic acid and viral vector vaccines that require storage at −70°C from manufacturing to vaccination run into ultra-cold chain issues for distribution in rural and underdeveloped areas that limit their use. BBV152 can be stored and distributed at 2 to 8°C using existing cold chain infrastructure to mitigate these issues (129). There is a lack of well-defined correlates of protection against SARS-CoV-2 infection such as the exact protective antibody type and titer levels, T-cell responses responsible for the range of symptoms, and the variation in these correlates concerning VOCs. Knowing this information would provide measurable aspects of immune responses required to prevent severe symptoms, decrease breakthrough infections, and help develop vaccines that confer sterilizing immunity and prevent infection rather than just prevent disease. Additional consideration must be given to safety and efficacy trials in children, pregnant women, and groups with severely compromised immunity such as HIV+ and cancer patients under treatment (130–132). Currently, all leading vaccines are administered through the intramuscular route. However, several studies report the importance of mucosal immunity against SARS-CoV-2 infections (133–136), and 12 intranasal vaccine candidates are currently in different clinical phases around the world (137). The Omicron variant, currently the most dominant global SARS-CoV-2 variant, shows enhanced viral replication in the human bronchus and upper respiratory tract than the lungs (138). Although Omicron shows reduced damage to the lungs and therefore reduced disease severity, its enhanced transmission capacity poses a major threat to public health worldwide. The increased Omicron-related breakthrough infections in the vaccinated population could in part be due to poor or no mucosal immunity generated by the intra-muscular vaccines. Therefore, the role of booster doses with intranasal vaccines needs to be investigated further. Real-world data on the efficacy and safety of multiple homologous booster vaccines are just emerging. Of particular concern is the lowered efficacy of the 4th mRNA vaccine booster dose against the Omicron variant (BNT162b2 -30% and mRNA1273 -11%) and the rise in vaccine-related adverse events (48). As recent history suggests, emerging VOCs have been more transmissible than their previous counterparts with Omicron being about 3-fold or 300% more transmissible than Delta. Next-gen vaccines may be needed to improve the neutralization efficacy against future VOC and will take time to develop. While some studies report improvements in vaccine efficacy by heterologous prime-boost regimens (103–105), a more robust clinical study on the immunogenicity, reactogenicity, and safety of multiple vaccine combinations and schedules is required. One such study underway in the US will evaluate the immunogenicity and safety of BBV152 booster dose in participants fully vaccinated (two doses) with mRNA vaccines (ClinicalTrials.gov: NCT05258669).
Author contributions
All authors made substantial contributions in the article preparation at the following stages: (1) the conception and design of the manuscript and interpretation of data, (2) drafting and revising the article for important intellectual content, (3) final approval of the version to be submitted.
Funding
This research did not receive specific funding but was performed as part of the employment of the authors, by Ocugen Inc.
Conflict of interest
The authors are employed by Ocugen Inc., which has commercialization rights for BBV152 in North America.
Publisher’s note
All claims expressed in this article are solely those of the authors and do not necessarily represent those of their affiliated organizations, or those of the publisher, the editors and the reviewers. Any product that may be evaluated in this article, or claim that may be made by its manufacturer, is not guaranteed or endorsed by the publisher.
References
1. Dong E, Du H, Gardner L. An interactive web-based dashboard to track COVID-19 in real time. Lancet Infect Dis (2020) 20(5):533–4. doi: 10.1016/S1473-3099(20)30120-1
2. Huang C, Wang Y, Li X, Ren L, Zhao J, Hu Y, et al. Clinical features of patients infected with 2019 novel coronavirus in wuhan, China. Lancet (2020) 395(10223):497–506. doi: 10.1016/S0140-6736(20)30183-5
3. Xu Z, Shi L, Wang Y, Zhang J, Huang L, Zhang C, et al. Pathological findings of COVID-19 associated with acute respiratory distress syndrome. Lancet Respir Med (2020) 8(4):420–2. doi: 10.1016/S2213-2600(20)30076-X
4. Sigal A, Milo R, Jassat W. Estimating disease severity of omicron and delta SARS-CoV-2 infections. Nat Rev Immunol (2022) 22:267–9. doi: 10.1038/s41577-022-00720-5
5. Wrenn JO, Pakala SB, Vestal G, Shilts MH, Brown HM, Bowen SM, et al. COVID-19 severity from omicron and delta SARS-CoV-2 variants. Influenza Other Respir Viruses (2022) 16:832–6. doi: 10.1111/irv.12982
6. Cao X. COVID-19: immunopathology and its implications for therapy. Nat Rev Immunol (2020) 20(5):269–70. doi: 10.1038/s41577-020-0308-3
7. Tan L, Kang X, Ji X, Li G, Wang Q, Li Y, et al. Validation of predictors of disease severity and outcomes in COVID-19 patients: A descriptive and retrospective study. Med (NY) (2020) 1(1):128–138.e3. doi: 10.1016/j.medj.2020.05.002
8. Yang L, Liu S, Liu J, Zhang Z, Wan X, Huang B, et al. COVID-19: immunopathogenesis and immunotherapeutics. Signal Transduct Target Ther (2020) 5(1):128. doi: 10.1038/s41392-020-00243-2
9. Zheng Z, Peng F, Xu B, Zhao J, Liu H, Peng J, et al. Risk factors of critical & mortal COVID-19 cases: A systematic literature review and meta-analysis. J Infect (2020) 81(2):e16–25. doi: 10.1016/j.jinf.2020.04.021
10. Abdulrahman A, Mallah SI, Alqahtani M. COVID-19 viral load not associated with disease severity: findings from a retrospective cohort study. BMC Infect Dis (2021) 21(1):688. doi: 10.1186/s12879-021-06376-1
11. El Zein S, Chehab O, Kanj A, Akrawe S, Alkassis S, Mishra T, et al. SARS-CoV-2 infection: Initial viral load (iVL) predicts severity of illness/outcome, and declining trend of iVL in hospitalized patients corresponds with slowing of the pandemic. PloS One (2021) 16(9):e0255981. doi: 10.1371/journal.pone.0255981
12. Knudtzen FC, Jensen TG, Lindvig SO, Rasmussen LD, Madsen LW, Hoegh SV, et al. SARS-CoV-2 viral load as a predictor for disease severity in outpatients and hospitalised patients with COVID-19: A prospective cohort study. PloS One (2021) 16(10):e0258421. doi: 10.1371/journal.pone.0258421
13. Lescure FX, Bouadma L, Nguyen D, Parisey M, Wicky PH, Behillil S, et al. Clinical and virological data of the first cases of COVID-19 in Europe: a case series. Lancet Infect Dis (2020) 20(6):697–706. doi: 10.1016/S1473-3099(20)30200-0
14. Liu Y, Yan LM, Wan L, Xiang TX, Le A, Liu JM, et al. Viral dynamics in mild and severe cases of COVID-19. Lancet Infect Dis (2020) 20(6):656–7. doi: 10.1016/S1473-3099(20)30232-2
15. Pan Y, Zhang D, Yang P, Poon LLM, Wang Q, et al. Viral load of SARS-CoV-2 in clinical samples. Lancet Infect Dis (2020) 20(4):411–2. doi: 10.1016/S1473-3099(20)30113-4
16. Shi F, Wu T, Zhu X, Ge Y, Zeng X, Chi Y, et al. Association of viral load with serum biomarkers among COVID-19 cases. Virology (2020) 546:122–6. doi: 10.1016/j.virol.2020.04.011
17. To KK, Tsang OT, Leung WS, Tam AR, Wu TC, Lung DC, et al. Temporal profiles of viral load in posterior oropharyngeal saliva samples and serum antibody responses during infection by SARS-CoV-2: an observational cohort study. Lancet Infect Dis (2020) 20(5):565–74. doi: 10.1016/S1473-3099(20)30196-1
18. Yu F, Yan L, Wang N, Yang S, Wang L, Tang Y, et al. Quantitative detection and viral load analysis of SARS-CoV-2 in infected patients. Clin Infect Dis (2020) 71(15):793–8. doi: 10.1093/cid/ciaa345
19. Zheng S, Fan J, Yu F, Feng B, Lou B, Zou Q, et al. Viral load dynamics and disease severity in patients infected with SARS-CoV-2 in zhejiang province, China, January-march 2020: retrospective cohort study. BMJ (2020) 369:m1443. doi: 10.1136/bmj.m1443
20. Meredith LW, Hamilton WL, Warne B, Houldcroft CJ, Hosmillo M, Jahun AS, et al. Rapid implementation of SARS-CoV-2 sequencing to investigate cases of health-care associated COVID-19: a prospective genomic surveillance study. Lancet Infect Dis (2020) 20(11):1263–72. doi: 10.1016/S1473-3099(20)30562-4
21. Abdool Karim SS, de Oliveira T. New SARS-CoV-2 variants - clinical, public health, and vaccine implications. N Engl J Med (2021) 384(19):1866–8. doi: 10.1056/NEJMc2100362
22. Callaway E. Heavily mutated omicron variant puts scientists on alert. Nature (2021) 600(7887):21. doi: 10.1038/d41586-021-03552-w
23. Harvey WT, Carabelli AM, Jackson B, Gupta RK, Thomson EC, Harrison EM, et al. SARS-CoV-2 variants, spike mutations and immune escape. Nat Rev Microbiol (2021) 19(7):409–24. doi: 10.1038/s41579-021-00573-0
24. Naveca FG, Nascimento V, de Souza VC, Corado AL, Nascimento F, Silva G, et al. COVID-19 in Amazonas, Brazil, was driven by the persistence of endemic lineages and P.1 emergence. Nat Med (2021) 27(7):1230–8. doi: 10.1038/s41591-021-01378-7
25. Silva S, Pena L. Collapse of the public health system and the emergence of new variants during the second wave of the COVID-19 pandemic in Brazil. One Health (2021) 13:100287. doi: 10.1016/j.onehlt.2021.100287
26. Tegally H, Wilkinson E, Giovanetti M, Iranzadeh A, Fonseca V, Giandhari J, et al. Detection of a SARS-CoV-2 variant of concern in south Africa. Nature (2021) 592(7854):438–43. doi: 10.1038/s41586-021-03402-9
27. Takahashi T, Ellingson MK, Wong P, Israelow B, Lucas C, Klein J, et al. Sex differences in immune responses that underlie COVID-19 disease outcomes. Nature (2020) 588(7837):315–20. doi: 10.1038/s41586-020-2700-3
28. Tan L, Wang Q, Zhang D, Ding J, Huang Q, Tang Y-Q, et al. Lymphopenia predicts disease severity of COVID-19: a descriptive and predictive study. Signal Transduct Target Ther (2020) 5(1):1–3. doi: 10.1038/s41392-020-0148-4
29. Didangelos A. COVID-19 hyperinflammation: what about neutrophils? MSphere (2020) 5(3):e00367–20. doi: 10.1128/mSphere.00367-20
30. Afrin LB, Weinstock LB, Molderings GJ. Covid-19 hyperinflammation and post-Covid-19 illness may be rooted in mast cell activation syndrome. Int J Infect Dis (2020) 100:327–32. doi: 10.1016/j.ijid.2020.09.016
31. Rodriguez L, Pekkarinen PT, Lakshmikanth T, Tan Z, Consiglio CR, Pou C, et al. Systems-level immunomonitoring from acute to recovery phase of severe COVID-19. Cell Rep Med (2020) 1(5):100078. doi: 10.1016/j.xcrm.2020.100078
32. Bernardes JP, Mishra N, Tran F, Bahmer T, Best L, Blase JI, et al. Longitudinal multi-omics analyses identify responses of megakaryocytes, erythroid cells, and plasmablasts as hallmarks of severe COVID-19. Immunity (2020) 53(6):1296–1314.e9. doi: 10.1016/j.immuni.2020.11.017
33. Uddbäck I, Cartwright EK, Schøller AS, Wein AN, Hayward SL, Lobby J, et al. Long-term maintenance of lung resident memory T cells is mediated by persistent antigen. Mucosal Immunol (2021) 14(1):92–9. doi: 10.1038/s41385-020-0309-3
34. Chen Z, John Wherry E. T Cell responses in patients with COVID-19. Nat Rev Immunol (2020) 20(9):529–36. doi: 10.1038/s41577-020-0402-6
35. Altmann DM, Boyton RJ. SARS-CoV-2 T cell immunity: Specificity, function, durability, and role in protection. Sci Immunol (2020) 5(49):eabd6160. doi: 10.1126/sciimmunol.abd6160
36. Ogega CO, Skinner NE, Blair PW, Park H-S, Littlefield K, Ganesan A, et al. Durable SARS-CoV-2 b cell immunity after mild or severe disease. J Clin Invest (2021) 131(7):1–12. doi: 10.1172/JCI145516
37. Sacchi A, Grassi G, Bordoni V, Lorenzini P, Cimini E, Casetti R, et al. Early expansion of myeloid-derived suppressor cells inhibits SARS-CoV-2 specific T-cell response and may predict fatal COVID-19 outcome. Cell Death Dis (2020) 11(10):1–9. doi: 10.1038/s41419-020-03125-1
38. Grifoni A, Weiskopf D, Ramirez SI, Mateus J, Dan JM, Moderbacher CR, et al. Targets of T cell responses to SARS-CoV-2 coronavirus in humans with COVID-19 disease and unexposed individuals. Cell (2020) 181(7):1489–501.e15. doi: 10.1016/j.cell.2020.05.015
39. Juno JA, Tan H-X, Lee WS, Reynaldi A, Kelly HG, Wragg K, et al. Humoral and circulating follicular helper T cell responses in recovered patients with COVID-19. Nat Med (2020) 26(9):1428–34. doi: 10.1038/s41591-020-0995-0
40. Ahluwalia P, Vaibhav K, Ahluwalia M, Mondal AK, Sahajpal N, Rojiani AM, et al. Infection and immune memory: Variables in robust protection by vaccines against SARS-CoV-2. Front Immunol (2021) 12:660019. doi: 10.3389/fimmu.2021.660019
41. Low JS, Vaqueirinho D, Mele F, Foglierini M, Jerak J, Perotti M, et al. Clonal analysis of immunodominance and cross-reactivity of the CD4 T cell response to SARS-CoV-2. Science (2021) 372(6548):1336–41. doi: 10.1126/science.abg8985
42. Boppana S, Qin K, Files JK, Russell RM, Stoltz R, Bibollet-Ruche F, et al. SARS-CoV-2-specific circulating T follicular helper cells correlate with neutralizing antibodies and increase during early convalescence. PloS Pathog (2021) 17(7):e1009761. doi: 10.1371/journal.ppat.1009761
43. He Y, Zhou Y, Wu H, Kou Z, Liu S, Jiang S, et al. Mapping of antigenic sites on the nucleocapsid protein of the severe acute respiratory syndrome coronavirus. J Clin Microbiol (2004) 42(11):5309–14. doi: 10.1128/JCM.42.11.5309-5314.2004
44. Zhao Y, Sui L, Wu P, Wang W, Wang Z, Yu Y, et al. A dual-role of SARS-CoV-2 nucleocapsid protein in regulating innate immune response. Signal Transduct Target Ther (2021) 6(1):331. doi: 10.1038/s41392-021-00742-w
45. Smits VAJ, Hernandez-Carralero E, Paz-Cabrera MC, Cabrera E, Hernandez-Reyes Y, Hernandez-Fernaud JR, et al. The nucleocapsid protein triggers the main humoral immune response in COVID-19 patients. Biochem Biophys Res Commun (2021) 543:45–9. doi: 10.1016/j.bbrc.2021.01.073
46. Jorrissen P, Schutz P, Weiand M, Vollenberg R, Schrempf IM, Ochs K, et al. Antibody response to SARS-CoV-2 membrane protein in patients of the acute and convalescent phase of COVID-19. Front Immunol (2021) 12:679841. doi: 10.3389/fimmu.2021.679841
47. Dangi T, Class J, Palacio N, Richner JM, Penaloza MacMaster P, et al. Combining spike- and nucleocapsid-based vaccines improves distal control of SARS-CoV-2. Cell Rep (2021) 36(10):109664. doi: 10.1016/j.celrep.2021.109664
48. Regev-Yochay G, Gonen T, Gilboa M, Mandelboim M, Indenbaum V, Amit S, et al. 4th dose COVID mRNA vaccines’ immunogenicity & efficacy against omicron VOC. medRxiv (2022):2022.02.15.22270948. doi: 10.1101/2022.02.15.22270948
49. Gagne M, Moliva JI, Foulds KE, Andrew SF, Flynn BJ, Werner AP, et al. mRNA-1273 or mRNA-omicron boost in vaccinated macaques elicits comparable b cell expansion, neutralizing antibodies and protection against omicron. bioRxiv (2022):2022.02.03.479037. doi: 10.1101/2022.02.03.479037
50. Ying B, Scheaffer SM, Whitener B, Liang C-Y, Dmytrenko O, Mackin S, et al. Boosting with omicron-matched or historical mRNA vaccines increases neutralizing antibody responses and protection against b. 1.1.529 Infect Mice bioRxiv (2022):2022.02.07.479419. doi: 10.1101/2022.02.07.479419
51. Lee I-J, Sun C-P, Wu P-Y, Lan Y-H, Wang I-H, Liu W-C, et al. Omicron-specific mRNA vaccine induced potent neutralizing antibody against omicron but not other SARS-CoV-2 variants. bioRxiv (2022):2022.01.31.478406. doi: 10.1101/2022.01.31.478406
52. Hawman DW, Meade-White K, Clancy C, Archer J, Hinkley T, Leventhal SS, et al. Replicating RNA platform enables rapid response to the SARS-CoV-2 omicron variant and elicits enhanced protection in naïve hamsters compared to ancestral vaccine. bioRxiv (2022):2022.01.31.478520. doi: 10.1101/2022.01.31.478520
53. Waltz E. Omicron-targeted vaccines do no better than original jabs in early tests. Nature (2022). doi: 10.1038/d41586-022-00003-y
54. Ella R, Vadrevu KM, Jogdand H, Prasad S, Reddy S, Sarangi V, et al. Safety and immunogenicity of an inactivated SARS-CoV-2 vaccine, BBV152: a double-blind, randomised, phase 1 trial. Lancet Infect Dis (2021) 21(5):637–46. doi: 10.1016/S1473-3099(20)30942-7
55. Ella R, Reddy S, Jogdand H, Sarangi V, Ganneru B, Prasad S, et al. Safety and immunogenicity of an inactivated SARS-CoV-2 vaccine, BBV152: interim results from a double-blind, randomised, multicentre, phase 2 trial, and 3-month follow-up of a double-blind, randomised phase 1 trial. Lancet Infect Dis (2021) 21(7):950–61. doi: 10.1016/S1473-3099(21)00070-0
56. Ella R, Reddy S, Blackwelder W, Potdar V, Yadav P, Sarangi V, et al. Efficacy, safety, and lot-to-lot immunogenicity of an inactivated SARS-CoV-2 vaccine (BBV152): interim results of a randomised, double-blind, controlled, phase 3 trial. Lancet (2021) 398(10317):2173–84. doi: 10.1016/S0140-6736(21)02000-6
57. Sanders B, Koldijk M, Schuitemaker H. Inactivated viral vaccines, in vaccine analysis: strategies, principles, and control. Springer (2015) p. 45–80.
58. Bhandari N, Rongsen-Chandola T, Bavdekar A, John J, Antony K, Taneja S, et al. Efficacy of a monovalent human-bovine (116E) rotavirus vaccine in Indian infants: a randomised, double-blind, placebo-controlled trial. Lancet (2014) 383(9935):2136–43. doi: 10.1016/S0140-6736(13)62630-6
59. Sampath G, Madhusudana SN, Sudarshan MK, Ashwathnarayana DH, Mahendra BJ, Ullas TP, et al. Immunogenicity and safety study of indirab: a vero cell based chromatographically purified human rabies vaccine. Vaccine (2010) 28(24):4086–90. doi: 10.1016/j.vaccine.2010.03.064
60. Singh A, Mitra M, Sampath G, Venugopal P, Rao JV, Krishnamurthy B, et al. A Japanese encephalitis vaccine from India induces durable and cross-protective immunity against temporally and spatially wide-ranging global field strains. J Infect Dis (2015) 212(5):715–25. doi: 10.1093/infdis/jiv023
61. Vadrevu KM, Potula V, Khalatkar V, Mahantshetty NS, Shah A, Ella R, et al. Persistence of immune responses with an inactivated Japanese encephalitis single-dose vaccine, JENVAC and interchangeability with a live-attenuated vaccine. J Infect Dis (2020) 222(9):1478–87. doi: 10.1093/infdis/jiz672
62. Kiesslich S, Kamen AA. Vero cell upstream bioprocess development for the production of viral vectors and vaccines. Biotechnol Adv (2020) 44:107608. doi: 10.1016/j.biotechadv.2020.107608
63. Sarkale P, Patil S, Yadav PD, Nyayanit DA, Sapkal G, Baradkar S, et al. First isolation of SARS-CoV-2 from clinical samples in India. Indian J Med Res (2020) 151(2 & 3):244–50. doi: 10.4103/ijmr.IJMR_1029_20
64. Yadav PD, Potdar VA, Choudhary ML, Nyayanit DA, Agrawal M, Jadhav SM, et al. Full-genome sequences of the first two SARS-CoV-2 viruses from India. Indian J Med Res (2020) 151(2 & 3):200–9. doi: 10.4103/ijmr.IJMR_663_20
65. Potdar V, Cherian SS, Deshpande GR, Ullas PT, Yadav PD, Choudhary ML, et al. Genomic analysis of SARS-CoV-2 strains among indians returning from Italy, Iran & China, & Italian tourists in India. Indian J Med Res (2020) 151(2-3):255. doi: 10.4103/ijmr.IJMR_1058_20
66. Ganneru B, Jogdand H, Dharam VK, Molugu NR, Prasad SD, Vellimudu S, et al. Evaluation of safety and immunogenicity of an adjuvanted, TH-1 skewed, whole virion InactivatedSARS-CoV-2 vaccine - BBV152. bioRxiv (2020):2020.09.09.285445. doi: 10.1101/2020.09.09.285445
67. Lee WS, Wheatley AK, Kent SJ, DeKosky BJ, et al. Antibody-dependent enhancement and SARS-CoV-2 vaccines and therapies. Nat Microbiol (2020) 5(10):1185–91. doi: 10.1038/s41564-020-00789-5
68. Zhao J, Yuan Q, Wang H, Liu W, Liao X, Su Y, et al. Antibody responses to SARS-CoV-2 in patients with novel coronavirus disease 2019. Clin Infect Dis (2020) 71(16):2027–34. doi: 10.1093/cid/ciaa344
69. Mathew D, Giles JR, Baxter AE, Oldridge DA, Greenplate AR, Wu JE, et al. Deep immune profiling of COVID-19 patients reveals distinct immunotypes with therapeutic implications. Science (2020) 369(6508):1–17. doi: 10.1126/science.369.6508.1203-l
70. Sekine T, Perez-Potti A, Rivera-Ballesteros O, Stralin K, Gorin JB, Olsson A, et al. Robust T cell immunity in convalescent individuals with asymptomatic or mild COVID-19. Cell (2020) 183(1):158–68.e14. doi: 10.1016/j.cell.2020.08.017
71. Shukla NM, Mutz CA, Malladi SS, Warshakoon HJ, Balakrishna R, David SA, et al. Toll-like receptor (TLR)-7 and -8 modulatory activities of dimeric imidazoquinolines. J Med Chem (2012) 55(3):1106–16. doi: 10.1021/jm2010207
72. Oberhardt V, Luxenburger H, Kemming J, Schulien I, Ciminski K, Giese S, et al. Rapid and stable mobilization of CD8+ T cells by SARS-CoV-2 mRNA vaccine. Nature (2021) 597(7875):268–73. doi: 10.1038/s41586-021-03841-4
73. Vikkurthi R, Ansari A, Pai AR, Jha SN, Sachan S, Pandit S, et al. Inactivated whole-virion vaccine BBV152/Covaxin elicits robust cellular immune memory to SARS-CoV-2 and variants of concern. Nat Microbiol (2022) 7:974–85. doi: 10.1101/2021.11.14.21266294
74. Mohandas S, Yadav PD, Shete-Aich A, Abraham P, Vadrevu KM, Sapkal G, et al. Immunogenicity and protective efficacy of BBV152, whole virion inactivated SARS- CoV-2 vaccine candidates in the Syrian hamster model. iScience (2021) 24(2):102054. doi: 10.1016/j.isci.2021.102054
75. Yadav PD, Ella R, Kumar S, Patil DR, Mohandas S, Shete AM, et al. Immunogenicity and protective efficacy of inactivated SARS-CoV-2 vaccine candidate, BBV152 in rhesus macaques. Nat Commun (2021) 12(1):1386. doi: 10.1038/s41467-021-21639-w
76. Ganneru B, Jogdand H, Daram VK, Das D, Molugu NR, Prasad SD, et al. Th1 skewed immune response of whole virion inactivated SARS CoV 2 vaccine and its safety evaluation. iScience (2021) 24(4):102298. doi: 10.1016/j.isci.2021.102298
77. Mohandas S, Jain R, Yadav PD, Shete-Aich A, Sarkale P, Kadam M, et al. Evaluation of the susceptibility of mice & hamsters to SARS-CoV-2 infection. Indian J Med Res (2020) 151(5):479–82. doi: 10.1016/j.isci.2021.102054
78. Starr TN, Greaney AJ, Hilton SK, Ellis D, Crawford KHD, Dingens AS, et al. Deep mutational scanning of SARS-CoV-2 receptor binding domain reveals constraints on folding and ACE2 binding. Cell (2020) 182(5):1295–1310.e20. doi: 10.1016/j.cell.2020.08.012
79. Planas D, Veyer D, Baidaliuk A, Staropoli I, Guivel-Benhassine F, Rajah MM, et al. Reduced sensitivity of infectious SARS-CoV-2 variant b. 1.617. 2 to monoclonal antibodies and sera from convalescent and vaccinated individuals. Biorxiv (2021). doi: 10.1038/s41586-021-03777-9
80. Naveca F, Nascimento V, Souza V, Corado A, Nascimento F, Silva G, et al. Phylogenetic relationship of SARS-CoV-2 sequences from Amazonas with emerging Brazilian variants harboring mutations E484K and N501Y in the spike protein. Virol Org (2021) 1:1–8. doi: 10.21203/rs.3.rs-275494/v1
81. Wang SY, Juthani PV, Borges KA, Shallow MK, Gupta A, Price C, et al. Severe breakthrough COVID-19 cases in the SARS-CoV-2 delta (B. 1.617. 2) variant era. Lancet Microbe (2022) 3(1):e4–5. doi: 10.1016/S2666-5247(21)00306-2
82. Goldberg Y, Mandel M, Bar-On YM, Bodenheimer O, Freedman L, Ash N, et al. Protection and waning of natural and hybrid COVID-19 immunity. MedRxiv (2021). doi: 10.1101/2021.12.04.21267114
83. ECDC. Implications of the emergence and spread of the SARSCoV-2 B.1.1. 529 variant of concern (Omicron), for the EU/EEA. European Centre for Disease Prevention and Control. (2021).
84. UKHSA. SARS-CoV-2 variants of concern and variants under investigation in england. technical briefing 29. UK Health Security Agency (2021).
85. Hastie KM, Li H, Bedinger D, Schendel SL, Dennison SM, Li K, et al. Defining variant-resistant epitopes targeted by SARS-CoV-2 antibodies: A global consortium study. Science (2021) 374(6566):472–8. doi: 10.1126/science.abh2315
86. Liu L, Iketani S, Guo Y, Chan JF, Wang M, Liu L, et al. Striking antibody evasion manifested by the omicron variant of SARS-CoV-2. Nature (2022) 602(7898):676–81. doi: 10.1038/s41586-021-04388-0
87. Gruell H, Vanshylla K, Tober-Lau P, Hillus D, Schommers P, Lehmann C, et al. mRNA booster immunization elicits potent neutralizing serum activity against the SARS-CoV-2 omicron variant. Nat Med (2022) 28:477–80. doi: 10.21203/rs.3.rs-1168453/v1
88. Schubert M, Bertoglio F, Steinke S, Heine PA, Ynga-Durand MA, Maass H, et al. Human serum from SARS-CoV-2-vaccinated and COVID-19 patients shows reduced binding to the RBD of SARS-CoV-2 omicron variant. BMC Med (2022) 20(1):102. doi: 10.1186/s12916-022-02312-5
89. Edara VV, Manning KE, Ellis M, Lai L, Moore KM, Foster SL, et al. mRNA-1273 and BNT162b2 mRNA vaccines have reduced neutralizing activity against the SARS-CoV-2 omicron variant. Cell Rep Med (2022) 3(2):100529. doi: 10.1016/j.xcrm.2022.100529
90. Ella R, Reddy S, Jogdand H, Sarangi V, Ganneru B, Prasad S, et al. Safety and immunogenicity clinical trial of an inactivated SARS-CoV-2 vaccine, BBV152 (a phase 2, double-blind, randomised controlled trial) and the persistence of immune responses from a phase 1 follow-up report. medRxiv (2020):2020.12.21.20248643. doi: 10.1101/2020.12.21.20248643
91. Sapkal GN, Yadav PD, Ella R, Deshpande GR, Sahay RR, Gupta N, et al. Inactivated COVID-19 vaccine BBV152/COVAXIN effectively neutralizes recently emerged B.1.1.7 variant of SARS-CoV-2. J Travel Med (2021) 28(4). doi: 10.1093/jtm/taab051
92. Sapkal G, Yadav PD, Ella R, Abraham P, Patil DY, Gupta N, et al. Neutralization of VUI B.1.1.28 P2 variant with sera of COVID-19 recovered cases and recipients of covaxin an inactivated COVID-19 vaccine. J Travel Med (2021) 28(7). doi: 10.1093/jtm/taab077
93. Yadav PD, Sapkal GN, Ella R, Sahay RR, Nyayanit DA, Patil DY, et al. Neutralization against B.1.351 and B.1.617.2 with sera of COVID-19 recovered cases and vaccinees of BBV152. bioRxiv (2021):2021.06.05.447177. doi: 10.1101/2021.06.05.447177
94. Edara V-V, Patel M, Suthar MS. Covaxin (BBV152) vaccine neutralizes SARS-CoV-2 delta and omicron variants. medRxiv (2022):2022.01.24.22269189. doi: 10.1101/2022.01.24.22269189
95. Wibmer CK, Ayres F, Hermanus T, Madzivhandila M, Kgagudi P, Oosthuysen B, et al. SARS-CoV-2 501Y.V2 escapes neutralization by south African COVID-19 donor plasma. Nat Med (2021) 27(4):622–5. doi: 10.1038/s41591-021-01285-x
96. Wu K, Werner AP, Koch M, Choi A, Narayanan E, Stewart-Jones GBE, et al. Serum neutralizing activity elicited by mRNA-1273 vaccine. N Engl J Med (2021) 384(15):1468–70. doi: 10.1056/NEJMc2102179
97. Giamarellos-Bourboulis EJ, Tsilika M, Moorlag S, Antonakos N, Kotsaki A, Dominguez-Andres J, et al. Activate: Randomized clinical trial of BCG vaccination against infection in the elderly. Cell (2020) 183(2):315–323.e9. doi: 10.1016/j.cell.2020.08.051
98. Netea MG, Dominguez-Andres J, Barreiro LB, Chavakis T, Divangahi M, Fuchs E, et al. Defining trained immunity and its role in health and disease. Nat Rev Immunol (2020) 20(6):375–88. doi: 10.1038/s41577-020-0285-6
99. Netea MG, Giamarellos-Bourboulis EJ, Dominguez-Andres J, Curtis N, van Crevel R, van de Veerdonk FL, et al. Trained immunity: a tool for reducing susceptibility to and the severity of SARS-CoV-2 infection. Cell (2020) 181(5):969–77. doi: 10.1016/j.cell.2020.04.042
100. Kumar NP, Banurekha VV, C PG, Nancy A, Padmapriyadarsini C, Mary AS, et al. Prime-boost vaccination with Covaxin/BBV152 induces heightened systemic cytokine and chemokine responses. Front Immunol (2021) 12:752397. doi: 10.3389/fimmu.2021.752397
101. Malhotra S, Mani K, Lodha R, Bakhshi S, Mathur VP, Gupta P, et al. SARS-CoV-2 reinfection rate and estimated effectiveness of the inactivated whole virion vaccine BBV152 against reinfection among health care workers in new Delhi, India. JAMA Netw Open (2022) 5(1):e2142210. doi: 10.1001/jamanetworkopen.2021.42210
102. Rosenberg ES, Dorabawila V, Easton D, Bauer UE, Kumar J, Hoen R, et al. Covid-19 vaccine effectiveness in new York state. N Engl J Med (2022) 386(2):116–27. doi: 10.1056/NEJMoa2116063
103. WHO. Interim recommendations for heterologous COVID-19 vaccine schedules. World Health Organization (2021).
104. Kant R, Dwivedi G, Zaman K, Sahay RR, Sapkal G, Kaushal H, et al. Immunogenicity and safety of a heterologous prime-boost COVID-19 vaccine schedule: ChAdOx1 vaccine covishield followed by BBV152 covaxin. J Travel Med (2021) 28(8). doi: 10.1093/jtm/taab166
105. Atmar RL, Lyke KE, Deming ME, Jackson LA, Branche AR, El Sahly HM, et al. Heterologous SARS-CoV-2 booster vaccinations - preliminary report. medRxiv (2021). doi: 10.1101/2021.10.10.21264827
106. Marot S, Malet I, Leducq V, Zafilaza K, Sterlin D, Planas D, et al. Rapid decline of neutralizing antibodies against SARS-CoV-2 among infected healthcare workers. Nat Commun (2021) 12(1):844. doi: 10.1038/s41467-021-21111-9
107. Bernal JL, Andrews N, Gower C, Gallagher E, Simmons R, Thelwall S, et al. Effectiveness of covid-19 vaccines against the b. 1.617. 2 (Delta) variant. New Engl J Med (2021). doi: 10.1056/NEJMoa2108891
108. Puranik A, Lenehan PJ, Silvert E, Niesen MJM, Corchado-Garcia J, O'Horo JC, et al. Comparison of two highly-effective mRNA vaccines for COVID-19 during periods of alpha and delta variant prevalence. medRxiv (2021). doi: 10.1101/2021.08.06.21261707
109. Tang P, Hasan MR, Chemaitelly H, Yassine HM, Benslimane FM, Al Khatib HA, et al. BNT162b2 and mRNA-1273 COVID-19 vaccine effectiveness against the SARS-CoV-2 delta variant in Qatar. Nat Med (2021) 27(12):2136–43. doi: 10.1038/s41591-021-01583-4
110. Madhi SA, Baillie V, Cutland CL, Voysey M, Koen AL, Fairlie L, et al. Efficacy of the ChAdOx1 nCoV-19 covid-19 vaccine against the B.1.351 variant. N Engl J Med (2021) 384(20):1885–98. doi: 10.1056/NEJMoa2102214
112. Vadrevu KM, Ganneru B, Reddy S, Jogdand H, Raju D, Praturi U, et al. Persistence of immunity and impact of a third (booster) dose of an inactivated SARS-CoV-2 vaccine, BBV152; a phase 2, double-blind, randomised controlled trial. medRxiv (2022):2022.01.05.22268777. doi: 10.1101/2022.01.05.22268777
113. Deshpande GR, Yadav PD, Abraham P, Nyayanit DA, Sapkal GN, Shete AM, et al. Booster dose of the inactivated COVID-19 vaccine BBV152 (Covaxin) enhances the neutralizing antibody response against alpha, beta, delta and omicron variants of concern. J Travel Med (2022) 29(3):1–3. doi: 10.1093/jtm/taac039
114. Ladhani SN, Amin-Chowdhury Z, Davies HG, Aiano F, Hayden I, Lacy J, et al. COVID-19 in children: analysis of the first pandemic peak in England. Arch Dis Child (2020) 105(12):1180–5. doi: 10.1136/archdischild-2020-320042
115. Ludvigsson JF. Systematic review of COVID-19 in children shows milder cases and a better prognosis than adults. Acta Paediatr (2020) 109(6):1088–95. doi: 10.1111/apa.15270
116. Bi Q, Wu Y, Mei S, Ye C, Zou X, Zhang Z, et al. Epidemiology and transmission of COVID-19 in 391 cases and 1286 of their close contacts in shenzhen, China: a retrospective cohort study. Lancet Infect Dis (2020) 20(8):911–9. doi: 10.1016/S1473-3099(20)30287-5
117. Aykac K, Cura Yayla BC, Ozsurekci Y, Evren K, Oygar PD, Gurlevik SL, et al. The association of viral load and disease severity in children with COVID-19. J Med Virol (2021) 93(5):3077–83. doi: 10.1002/jmv.26853
118. Baggio S, L'Huillier AG, Yerly S, Bellon M, Wagner N, Rohr M, et al. Severe acute respiratory syndrome coronavirus 2 (SARS-CoV-2) viral load in the upper respiratory tract of children and adults with early acute coronavirus disease 2019 (COVID-19). Clin Infect Dis (2021) 73(1):148–50. doi: 10.1093/cid/ciaa1157
119. Rudan I, Adeloye D, Katikireddi SV, Murray J, Simpson C, Shah SA, et al. The COVID-19 pandemic in children and young people during 2020-2021: Learning about clinical presentation, patterns of spread, viral load, diagnosis and treatment. J Glob Health (2021) 11:01010. doi: 10.7189/jogh.11.01010
120. Otto SP, Day T, Arino J, Colijn C, Dushoff J, Li M, et al. The origins and potential future of SARS-CoV-2 variants of concern in the evolving COVID-19 pandemic. Curr Biol (2021) 31(14):R918–29. doi: 10.1016/j.cub.2021.06.049
121. Mallapaty S. Will COVID become a disease of the young? Nature (2021) 595(7867):343–4. doi: 10.1038/d41586-021-01862-7
122. Wong BLH, Ramsay ME, Ladhani SN. Should children be vaccinated against COVID-19 now? Arch Dis Childhood (2021) 106(12):1147–8. doi: 10.1136/archdischild-2020-321225
123. Klass P, Ratner AJ. Vaccinating children against covid-19 - the lessons of measles. N Engl J Med (2021) 384(7):589–91. doi: 10.1056/NEJMp2034765
124. Rosenstrom E, Mele J, Ivy J, Mayorga M, Patel M, Lich KH, et al. Vaccinating children against COVID-19 is essential prior to the removal of non-pharmaceutical interventions. medRxiv (2021). doi: 10.1101/2021.12.08.21267496
125. Vadrevu KM, Reddy S, Jogdand H, Ganneru B, Mirza N, Tripathy VN, et al. Immunogenicity and safety of an inactivated SARS-CoV-2 vaccine (BBV152) in children from 2 to 18 years of age: an open-label, age-de-escalation phase 2/3 study. medRxiv (2021):2021.12.28.21268468. doi: 10.1101/2021.12.28.21268468
126. Kohli M, Maschio M, Becker D, Weinstein MC. The potential public health and economic value of a hypothetical COVID-19 vaccine in the united states: Use of cost-effectiveness modeling to inform vaccination prioritization. Vaccine (2021) 39(7):1157–64. doi: 10.1016/j.vaccine.2020.12.078
127. Wanlapakorn N, Suntronwong N, Phowatthanasathian H, Yorsaeng R, Vichaiwattana P, Thongmee T, et al. Safety and immunogenicity of heterologous and homologous inactivated and adenoviral-vectored COVID-19 vaccines in healthy adults. medRxiv (2021):2021.11.04.21265908. doi: 10.1101/2021.11.04.21265908
128. Paltiel AD, Schwartz JL, Zheng A, Walensky RP. Clinical outcomes of a COVID-19 vaccine: Implementation over efficacy. Health Aff (Millwood) (2021) 40(1):42–52. doi: 10.1377/hlthaff.2020.02054
129. WHO. Annexes to the interim recommendations for use of the bharat biotech BBV152 COVAXIN® vaccine against COVID-19. World Health Organization (2021).
131. Hall S. COVID vaccines safely protect pregnant people: the data are in. Nature (2022) 601(7893):308–9. doi: 10.1038/d41586-022-00031-8
132. Magnus MC, Gjessing HK, Eide HN, Wilcox AJ, Fell DB, Haberg SE. Covid-19 vaccination during pregnancy and first-trimester miscarriage. N Engl J Med (2021) 385(21):2008–10. doi: 10.1056/NEJMc2114466
133. Hassan AO, Kafai NM, Dmitriev IP, Fox JM, Smith BK, Harvey IB, et al. A single-dose intranasal ChAd vaccine protects upper and lower respiratory tracts against SARS-CoV-2. Cell (2020) 183(1):169–184 e13. doi: 10.1016/j.cell.2020.08.026
134. Isho B, Abe KT, Zuo M, Jamal AJ, Rathod B, Wang JH, et al. Persistence of serum and saliva antibody responses to SARS-CoV-2 spike antigens in COVID-19 patients. Sci Immunol (2020) 5(52):1–20. doi: 10.1126/sciimmunol.abe5511
135. Chan RWY, Liu S, Cheung JY, Tsun JGS, Chan KC, Chan KYY, et al. The mucosal and serological immune responses to the novel coronavirus (SARS-CoV-2) vaccines. Front Immunol (2021) 12:744887. doi: 10.3389/fimmu.2021.744887
136. Lapuente D, Fuchs J, Willar J, Vieira Antao A, Eberlein V, Uhlig N, et al. Protective mucosal immunity against SARS-CoV-2 after heterologous systemic prime-mucosal boost immunization. Nat Commun (2021) 12(1):6871. doi: 10.1038/s41467-021-27063-4
137. Alu A, Chen L, Lei H, Wei Y, Tian X, Wei X. Intranasal COVID-19 vaccines: From bench to bed. EBioMedicine (2022) 76:103841. doi: 10.1016/j.ebiom.2022.103841
Keywords: SARS – CoV – 2, vaccine, heterologous prime and boost vaccines, inactivated virus adjuvanted vaccine, variants of concern (VOCs), vaccine challenges, original antigenic sin
Citation: Dotiwala F and Upadhyay AK (2022) A comprehensive review of BBV152 vaccine development, effectiveness, safety, challenges, and prospects. Front. Immunol. 13:940715. doi: 10.3389/fimmu.2022.940715
Received: 10 May 2022; Accepted: 08 August 2022;
Published: 13 September 2022.
Edited by:
Sandip D. Kamath, Medical University of Vienna, AustriaReviewed by:
Sohinee Sarkar, Royal Children’s Hospital, AustraliaBin Zheng, Zhejiang Academy of Medical Sciences, China
Copyright © 2022 Dotiwala and Upadhyay. This is an open-access article distributed under the terms of the Creative Commons Attribution License (CC BY). The use, distribution or reproduction in other forums is permitted, provided the original author(s) and the copyright owner(s) are credited and that the original publication in this journal is cited, in accordance with accepted academic practice. No use, distribution or reproduction is permitted which does not comply with these terms.
*Correspondence: Farokh Dotiwala, RmFyb2toLmRvdGl3YWxhQG9jdWdlbi5jb20=; Arun K. Upadhyay, QXJ1bi5VcGFkaHlheUBvY3VnZW4uY29t