- 1Department of Rheumatology, Affiliated Hospital of Nantong University, Medical School of Nantong University, Nantong, China
- 2Department of Rheumatology, Affiliated Hospital of Nantong University, Nantong, China
- 3Information Center, The First People’s Hospital of Nantong City, Nantong, China
- 4Department of Geriatrics, Affiliated Hospital of Nantong University, Nantong, China
Aging induces a series of immune related changes, which is called immunosenescence, playing important roles in many age-related diseases, especially neurodegenerative diseases, tumors, cardiovascular diseases, autoimmune diseases and coronavirus disease 2019(COVID-19). However, the mechanism of immunosenescence, the association with aging and successful aging, and the effects on diseases are not revealed obviously. In order to provide theoretical basis for preventing or controlling diseases effectively and achieve successful aging, we conducted the review and found that changes of aging-related phenotypes, deterioration of immune organ function and alterations of immune cell subsets participated in the process of immunosenescence, which had great effects on the occurrence and development of age-related diseases.
1 Introduction
Aging, as a universal biological phenomenon, is an inevitable trend during lifespan and shows close effect on the immune system. The immune system is one of the most ubiquitous systems of the organism which can protect the human body from internal or external pathogens and interacts with neural, circulatory and other systems (1–3). Aging induces declining functions of the immune system, a process called immunosenescence, affecting the composition, quantity and function of immune organs, immune cells and cytokines (4). As a result of immunosenescence, the incidence of many age-related diseases is increased, including neurodegenerative diseases, cancers, cardiovascular diseases, autoimmune diseases and the COVID-19, ultimately resulting in organ failure and death (5–7). For a long time, immunosenescence has been considered harmful. However, later scientists revised the negative meaning because derogatory descriptions did not seize its essence. Immunosenescence is a multifactorial and dynamic complex phenomenon, which is shown as a lengthy adjusting and remodeled process existing in immune system during lifespan (8, 9). This review will compile the most recent researches of immunosenescence, including its relation with aging and its role in age-related diseases, thereby, providing scientists with theoretical rationales for intervention targets to aging.
2 The role of aging-related phenotypes in immunosenescence
The molecular and cellular mechanisms of immunosenescence are mostly unclear. Many aging related phenotypes contribute to or are attributed to immunosenescence, including senescence-associated secretory phenotype (SASP), chronic inflammation, shortened telomere and decreased telomerase activity, and metabolic alternations, which are risk factors of age-related diseases (Figure 1).
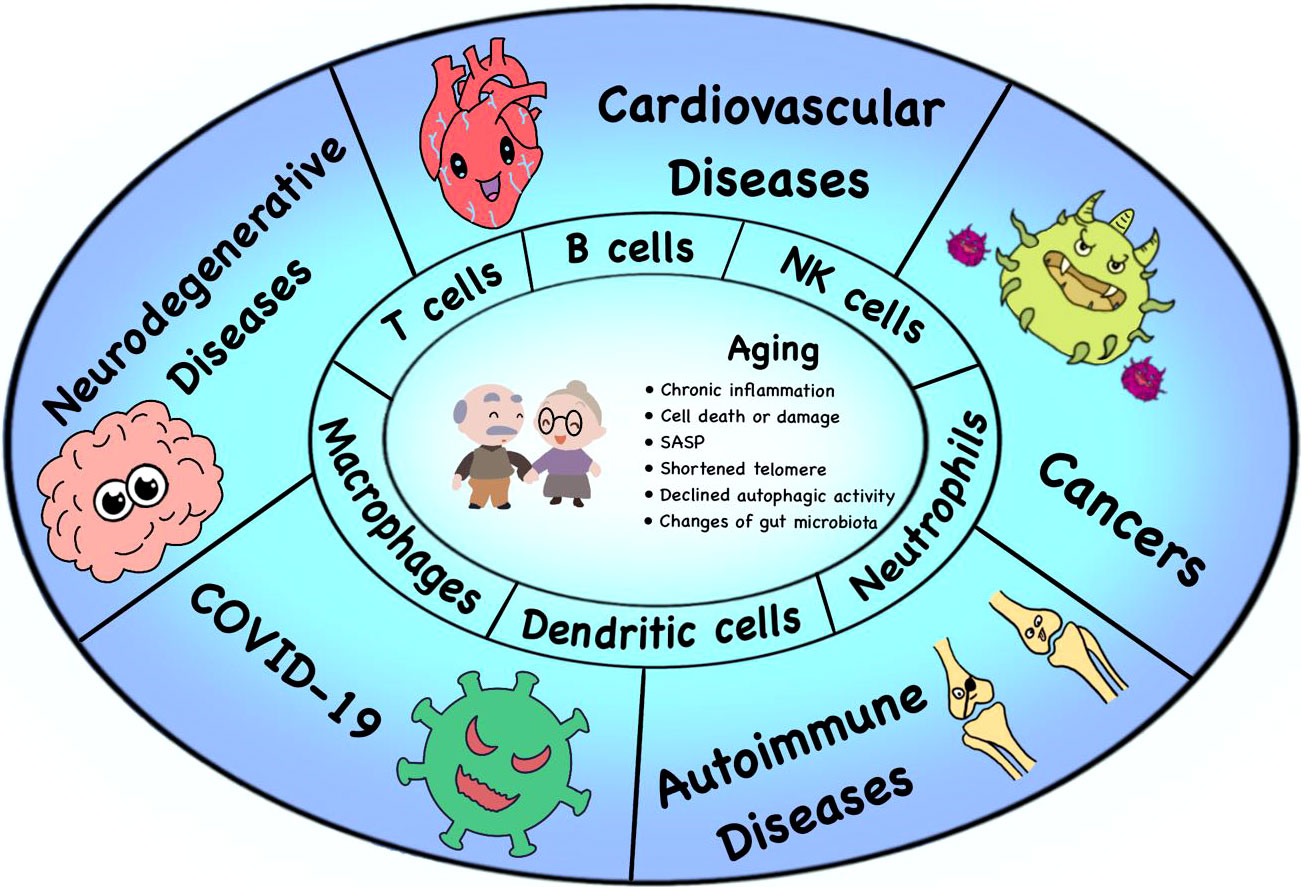
Figure 1 Immunosenescence and age-related diseases. Many factors lead to immunosenescence, including the accumulation of SASP, chronic inflammation, changes of gut microbiota, shortened telomere and declined autophagic activity. The process of immunosenescence causes a series of changes in immune cell subsets (especially T cells, B cells, NK cells, DCs, neutrophils and macrophages), thus leading to the occurrence of various age-related diseases, such as neurodegenerative diseases, tumors, cardiovascular diseases, autoimmune diseases and the COVID-19.
2.1 SASP and immunosenescence
SASP is a pro-inflammatory phenotype including inflammatory factors, chemokines (CXCL, CCL), growth factors and extracellular matrix proteases, and accumulates persistently with the increase of senescent cells in various organs (10, 11). SASP is a universal characteristic of cell senescence. It spreads senescence in autocrine or paracrine manner and activates signal pathways (such as NF-kB, mTOR or p38MAPK) to affect cell microenvironment (11, 12). SASP induces inflammation, recruits immune cells and affects adjacent and distant cells or tissues (13). Actually, SASP has a close relationship with the immune system, for example, macrophage chemokines (MCP-1) are the main components of SASP (14). Certain SASP components are recognized by receptors on natural killer (NK) cells, T cells and monocytes/macrophages, and then affect other immune cells, which further release more proinflammatory cytokines and aggravate age-related pathology (13). SASP is a double-edged sword and different components induce different biological activities. It is beneficial that temporary secretion of SASP may be a danger warning to nearby cells and promote immune clearance of impaired cells (11). For example, SASP may attract innate and adaptive immune cells near tumor cells and precancerous lesions to resist cancer invasion (15). However, persistent secretion of SASP may cause chronic systemic inflammation and tissue damage and inhibit immune cell function in the elderly (11, 16). For instance, the SASP produced by precancerous hepatocytes may attract immature myeloid cells to inhibit NK cells and promote hepatocellular carcinoma progression, which seems to contradict the previous research (17). Why the SASP exhibits multiple and sometimes opposite effects, which remains to be explained.
2.2 Inflammation and immunosenescence
During aging, a state of chronic, low-grade, sterile inflammation has been known as inflammaging, which is essential to the aging process (18, 19). Many components (nucleic acids, mitochondrial DNA, cardiolipin, mitochondria and heat shock proteins) from cell death or damage increase with age, which may be recognized by innate immune receptors like toll-like receptors (TLRs), NOD-Like Receptors (NLR) and cGMP-AMP synthase (cGAS) and produce pro-inflammatory cytokines (20). SASP secreted from senescent adaptive immune cells, including T/B cells, may contribute to inflammation (21). Moreover, changes in the gut microbiota of the elderly may activate macrophages to a pro-inflammatory state and induce multiple inflammatory pathways, which is an important source of inflammation (22, 23). Macrophages initiate the inflammatory response and activate other immune cells by secreting inflammatory factors such as tumor necrosis factor (TNF)-α and interferon (IFN)-γ (7).
There is an imbalance between inflammatory and immune reactions in the process of aging, which reduces the efficiency of immune responses and creates an immunosuppressive microenvironment (24). Inflammatory mediators promote myelopoiesis and increase the immunosuppressive cells compensably, especially regulatory T (Treg) cells and M2 macrophages that secrete immunosuppressive factors, such as transforming growth factor-β (TGF-β), ROS and interleukin-10 (IL-10). IL-10 further support the proliferation and activation of Treg cells and M2 macrophages (25, 26). Interestingly, these factors also inhibit some immune cells and promote their immunosenescence, for example, TGF-β can inhibit the differentiation of helper T(Th) cells, reduce the cytotoxicity of CD8 T cells and NK cells and weaken the immune response of B cells (27). Once the balance is broken, a persistent increase of inflammatory response influences the activation of T/B lymphocytes, which is called immune paralysis and considered as one of the clinical features of immunosenescence (28). High levels of proinflammatory cytokines such as TNF-α damage human B cells and reduce the production of protective antibodies significantly (29, 30). Other cells such as macrophages and NK cells develop immune paralysis after long-term inflammation (31). Remarkably, inflammation is closely related to immunosenescence, but the debate continues about whether inflammation is a cause or result of immunosenescence.
2.3 Telomere and immunosenescence
The telomere biology system containing telomeres (DNA–protein complexes at the ends of chromosomes) and telomerases (reverse transcriptases that add DNA repeats to the ends of telomeres), is essential to maintaining the integrity of the genes and cells (32). In the immune system, the average telomere length and telomerase activity in lymphocytes decline with age (33, 34). The progressive telomere attrition from naive to effector memory cells shortens the telomere length, which may be resulted from mitochondrial stress. Moreover, shortened telomere could lead to DNA damage and cell cycle arrest, ultimately resulting in damaged cell function and inefficient pathogen clearance (35, 36).
Telomerase plays a vital role in immune activation, differentiation and immunosenescence through acting on key immunomodulatory factors such as NF-kB and β-catenin (37). The downregulation of telomerase activity is detrimental to the immune response and activates aging cells in the cloning process (37). The decrease of telomerase activity is usually accompanied by the increased intracellular ROS and the reduced CD28 expression. Senescent CD28-T cells with the shortest telomere length and the lowest telomerase activity produce decreased antiviral cytokines and increased pro-inflammatory cytokines (38, 39). CD28 costimulatory signal is necessary for upregulation of telomerase activity. A study measuring telomerase activity of T cells suggests that only telomerase activity of CD28+T cells is increased significantly under immune stimulation (40).
2.4 Metabolism and immunosenescence
It is clear that immune function is highly dependent on nutritional metabolism. The interaction between immune and metabolic process is termed as immunometabolism (41). The metabolic disorders of main nutrients (such as glucose, lipids and amino acids) in immune cells during aging lead to the dysregulation of nicotinamide adenine dinucleotide (NAD+) metabolism, activating inflammatory pathways and accelerating immunosenescence (42). With the increase of age, the level of glycolytic metabolism decreases and mitochondria energy metabolism is abnormal, which impairs T and B cell activation (43, 44). The NAD+ is a coenzyme which catalyzes cellular metabolic functions and converts to NADH. NAD+ decreases with age, which is resulted from reduced NAD+ biosynthesis, caused by chronic inflammation with increased oxidative stress and inflammatory cytokines, and increased NAD+ consumption, caused by DNA damage (45, 46). The reduction of NAD+ metabolism activates NLRP3 inflammatory bodies during age, which may be the key to inflammatory diseases (47). Proteostasis, an importance process to maintain protein structure and function, is compromised with age (48). Proteins are composed of a variety of amino acids that have a great impact on immune response, especially T cells (49). During aging, multiple proteins cannot be degraded and accumulate in tissues, contributing to the occurrence of age-related pathologies (50).
3 The contribution of immune organs on immunosenescence
3.1 Bone marrow involution with aging
Bone marrow contains haematopoietic stem cells (HSCs) and non-HSCs. HSCs are multifunctional immature cell populations that possess self-renewing capacity and give rise to all blood cells of immune system (51, 52). HSCs are decreased with aging and the senescent HSCs acquire increased DNA damage, dysfunctional function and myeloid bias, affecting the generation of naive T cells severely (53, 54).
HSCs show a more shift toward myeloid biased HSCs with age. The lymphoid-biased HSCs loss and the ability of common lymphoid progenitors (CLPs) to differentiate into the progenitor B cells is compromised so that the progenitor B cells decrease, which may be caused by changes in the different microRNAs (such as miR-29a, miR125b, and miR-150) and transcription factors (55–58). Other studies have shown that lymphoid-biased HSCs could be inhibited by TGF-β (59). Besides, the capacity of bone marrow stromal cells to release IL-7 (an important cytokine for survival and proliferation of B-lineage precursors) declines gradually, which is another mechanism for the development of progenitor B cells (60). However, senescence has no apparent effect on pro-B, pre-B and immature B cells (61).
Although age usually leads to decreased bone marrow cell density, the numbers of bone marrow resident NK cells (62) and macrophages (63) tend to increase in the elderly. NK cells are derived from bone marrow, which are characterized by high expression of specific markers CD16, CD56 or CD57. The HSCs are more likely to differentiate into NK cells, therefore, the frequency and absolute value of NK cells increase in the elderly (62). However, NK cells also display the loss of telomeres and the decrease of telomerase activity with age, which may lead to reduction of NK cell growth and proliferation (64). The aged macrophages have decreased ability to secrete inflammatory cytokines (63). Together, the composition of bone marrow and the ability to differentiate into functional immune cells are significantly impaired with age.
3.2 Thymic involution with aging
The thymus is a central lymphoid organ and responsible to produce naive T cells, playing an essential role in cellular and humoral immunity. Resulting from the loss of trophic cytokines such as IL-7 and decreased stem cell activity of medullary thymic epithelial cells, which are the main thymic stromal cells producing T cells, the thymus gradually degenerate that accompanies senescence (65, 66). T cells undergo T cell receptor (TCR) genes rearranging, positive and negative selection in the thymic cortex and medulla and become single positive naive T cells (CD4 or CD8) that are exported to the periphery (67–69). Thymic involution reduces naive T cells and TCR repertoire (70–72). CD8 T cells (especially cytotoxic CD8+ T cells) tend to loss much more severe than CD4 T cells which could be maintained by homeostasis and proliferation (73, 74). Thymic involution interferes with the negative selection resulting in the release of autoreactive T cells that become activated in the periphery and produce low-level proinflammatory cytokines (including TNF-α and IL-6) which lead to chronic low-grade inflammation and self-tissue damage (75). However, atrophic thymus balances the defective negative selection by enhancing thymic Treg (tTreg) cell production relatively in the elderly (76). The elderly suffer from high risk of cytomegalovirus (CMV) infection, which can accelerate immunosenescence by decreasing naive T cell diversity and exaggerating the cytokine storms (77). Interestingly, it is reported that well-preserved naive T cells can be found in centenarians (8).
3.3 Impaired peripheral lymphoid organs with aging
The peripheral lymphoid organs, predominantly referring to spleen and lymph nodes, provide the settled site for immune cells to be proliferation, maturation and differentiation, and participant in immune response. The peripheral lymphoid organs are also of vital importance in the interaction between T cells, B cells and antigen presenting cells (APC) (69). The aged spleen upregulates IL-6 expression, impairs the recruitment of T cells and inhibits phagocytosis of macrophages in the marginal zone (78–80). The key function of lymph nodes is to coordinate immune response. The lymphocytes in lymph nodes change significantly with age, including increased B cells and memory CD4 T cells, decreased γδ T cells, CD8 T cells, naive CD4 T cells, IgM-expressing B cells and follicular dendritic cells (FDCs) (81, 82). The lymph nodes show signs of aging, including permeability changes, senescent cell aggregation and inflammation, which may be disadvantageous for immune cell migration and recruitment (83), leading to decreased humoral immunity (84) and increased susceptibility to infections in the elderly (85).
Mucosa-associated lymphoid tissue (MALT) is also a part of peripheral immune organs, which is located on the surface of mucosal tissue and plays an important role in immune protection. Naive T/B cells and DCs in intestinal lymphoid tissue are reduced with age, which may explain the increased gastrointestinal cancers in the elderly (86). Therefore, age-related structural disorders of peripheral lymphoid organs and the changes of immune cells seem to be the main reasons for immunosenescence.
4 The alterations of immune cell subsets related to immunosenescence
Immunosenescence reflects the regulation of innate and acquired immune system, in which cell subsets, surface markers, quantity and function of immune cells, such as T cells, B cells, NK cells, DCs, neutrophils and macrophages, undergo a series of changes (Figure 2).
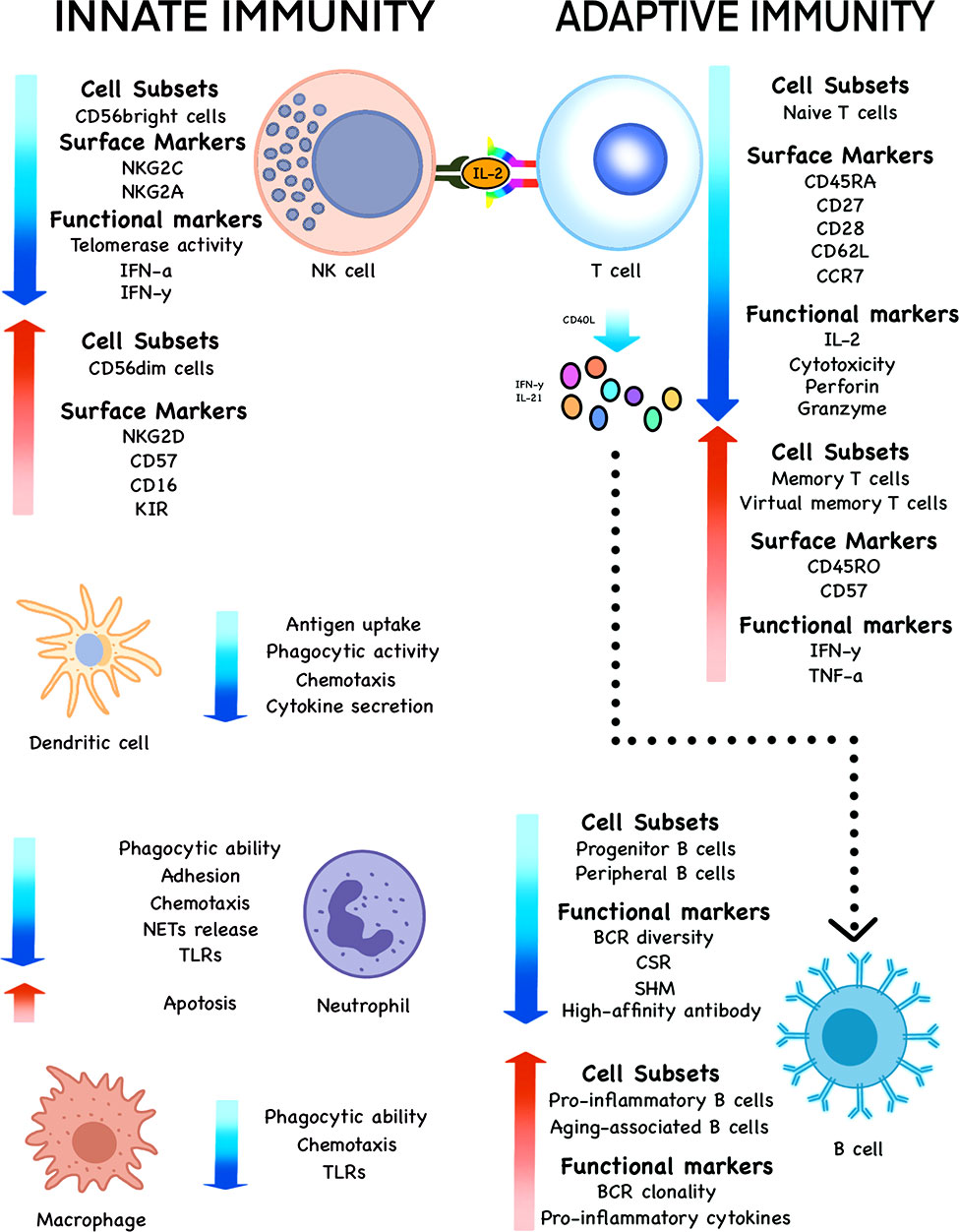
Figure 2 Changes of various cell subsets, surface markers and functional markers during immunosenescence. The subsets, phenotypes and functions of innate immune cells, such as NK cells, DCs, neutrophils and macrophages undergo significant changes with the increase of age in the elderly. The same is true of adaptive immune cells such as T and B cells.
4.1 Adaptive immune cells
4.1.1 T Cells
T cells, deriving from HSCs, mature in the thymus and migrate to peripheral lymphoid organs to expand and differentiate into memory and effector T cells under the antigenic stimulus, exerting a profound effect on immune system functions (87). T cells have specificity in recognition of foreign antigens and can be divided into several subsets including Th cells, cytotoxic T(Tc) cells and Treg cells, according to their different functions of immune response (88, 89). T cells undergo senescence with the loss of costimulatory molecules CD27 and CD28, the decreased growth factor IL-2 and the increased pro-inflammatory cytokine production (90–92). Senescent T cells can activate the inflammatory processes by contacting other immune cells, secreting pro-inflammatory cytokines or acting directly on the target tissues, eventually resulting in tissue damages and participation in the pathogenesis of aging (93). The genes related to leukocyte activation and immunity in aged memory T cells increase, which reduces the ability to recognize new pathogens and the response to vaccination, and increases risks for infection in the elderly (94).
4.1.1.1 Helper T cells
Th cells, expressing the CD4 surface marker, coordinate the activities of the immune system by secreting cytokines or assisting other lymphocytes. Th cells are subdivided into Th1, Th2, Th9, Th17, Th22 and follicular helper T cells (Tfh). In aged humans, naive CD4 T cells tend to proliferate and differentiate into effector memory Th9 cells that secrete increased cytokines IL-9 due to the upregulation of the TGFβR3 receptor, leading to higher PU.1, BATF and IRF4 expression (95). The single-cell RNA sequencing uncovers that aging promotes T cells from naive to effector subtypes, among which Th1 and Th17 cell subsets are dominant. These subgroups are highly correlated with IL-6, IL-27 and IFN, which promotes chronic inflammation and declines immunity partly (96).
Tfh cells, presenting in lymphoid organs and peripheral blood, provide help for B cells that activate, differentiate and produce high-affinity antibodies by signals (such as ICOS, IL-12 and CD40L) (97). Reduced ICOS expression with aging could limit the number of Tfh cells (98). Increased pro-inflammatory cytokines IL‐12 with aging contribute to the formation of Tfh cells (99) and support differentiation of other Th cells, such as Th1 and Th17 cells (100). CD40L, highly expressed in Tfh cells, interacts with CD40 on B cells, which is vital for B cell immune response (101). Tfh cells express decreased CD40L in aged people, which reduces the assistance to B cells and contributes to decreased antibody titers after immunization (102).
4.1.1.2 Cytotoxic T cells
Tc cells (also known as killer T cells), expressing the CD8 surface marker, are crucial in immune defense against harmful pathogens by secreting cytotoxic substances such as granzyme and perforin (103). As people get older, Tc cell proliferation is impaired along with the decreased naive cell marker (CD45RA and CD27), the lymphocyte adhesion molecule SELL (CD62L) and the lymphoid tissue homing chemokine receptor (CCR7), while the expression of memory cell marker CD45RO and the senescent marker CD57 increase (104). Cytotoxicity of Tc cells is reduced with aging, which decreases the killing effect on virus and increases disease risk in the elderly (61). Furthermore, cellular senescence is usually considered as the main mechanism of aging-related T-cell dysfunction (105). Tc cells also show cellular senescence characteristics, such as high levels of SA-βGal activity, p16INK4a, macroH2A and dysfunctional telomeres (106). Interestingly, aging might endow Tc cells with apoptosis resistance, for example, the antiapoptotic genes such as Serpina3g Id2 and S1pr5 are upregulated (94).
Some studies have confirmed a special kind of cells that express CD8 molecules and acquire memory phenotypes in the absence of antigen-specific immune responses, and are often termed virtual memory CD8 T (TVM) cells (107–110). These cells could patrol and monitor at the early stage, and disposal of pathogens during the effect-period, so they have been a bridge between innate and acquired immunity. TVM cells accumulate with age by cytokine stimulation (such as IL-4 and IL-15) but not by antigenic stimulation and exhibit characteristics consistent with senescence (111).
4.1.1.3 Regulatory T cells
Treg cells, expressing inhibitory receptors such as programmed death 1 (PD-1) and cytotoxic T lymphocyte-associated antigen-4 (CTLA-4), make much difference to maintaining immune balance and limiting immunopathology by negatively regulating immune responses and secreting immunosuppressive cytokines TGF-β and IL-10 (112, 113). Treg cells are separated into natural Treg cells (nTregs) and induced Treg cells (iTregs or aTregs) (114). Despite thymic involution, the number and proportion of Treg cells increase in the elderly (115, 116), because Treg cells are derived from not only the thymus but also the differentiation of peripheral CD4+T cells and the proliferation of CD45RO+Treg cells (117), but their clonal diversity is reduced (118). A few studies have shown that Treg cell function decreases in the elderly, however, the overall data suggest that Treg function remains the same or even increases during aging, which is consistent with the fact that older individuals are more susceptible to infection and malignant tumors, while they are likely to develop autoimmune diseases due to Treg cell dysfunction (119).
The enhanced Treg cell function is related to increased expression level of forkhead box protein 3 (Foxp3) and hypomethylation of Foxp3 that is a master regulator of Treg cell function (120). Treg cells are more likely to be influenced by age-dependent autophagy inhibition due to more dependence on oxidative phosphorylation (121). Treg and Th17 cells are the key regulators of immune homeostasis. In the process of aging, the Th17/Treg imbalance that is driven driven by antigens or cytokines may result in abnormal immune response and the occurrence of various diseases (122, 123). The accumulation of CD8+Treg cells that mainly come from CD8+CD28-T cells, contributes to immune deficiency and declined adaptive responses with increasing age (124). Although a great progress has been made in the role of Treg cells related to immunosenescence, there are still many problems remaining to be resolved.
4.1.2 B cells
B cells, a subset of adaptive immune cells, are crucially important in both cellular immunity and humoral immunity through secretion of antibodies, presentation of antigens and regulation of T cell functions (125). In addition to alterations in HSCs, intermediate and mature stages of B cell development also show a series of aging-associated changes. With increasing age, the proportion of peripheral B cells decreases (126). However, the number and frequency of pro-inflammatory B cells are expanded, which is largely because of increased pro-inflammatory signals CD40L, IFN-γ and IL-21 (127). Older adults display decreased repertoire diversity and increased BCR clonality (128). Aging also downregulates the expression of molecules with regard to immunoglobulin class-switch recombination (CSR) and somatic hypermutation (SHM) and reduces high-affinity antibody production (61). Neutralizing antibody responses and secretion of switched IgG play an important role during infection and vaccine efficacy. These shifts might increase the risk of bacteria and viruses in the elderly, such as the high hospitalization and mortality resulted from COVID-19 (129).
It is reported that there is a novel B cell subset in human peripheral blood that accumulates with age, which is called the aging-associated B cells (ABCs) (130, 131). ABCs are generated by Follicular (FO) B cells via interactions between MHC class II and CD40/CD40L and distinguished from other B cells by their markers such as CD11b, CD11c and T-bet and signal transduction pathway such as TLR7 (132). ABCs displaying significant SHM and secreting autoantibodies, are closely related to autoimmune diseases (58, 131, 133). ABCs are reported to increase in elderly humans, especially in senile women with autoimmune diseases of lupus and rheumatoid arthritis (RA) (130) (Figure 3).
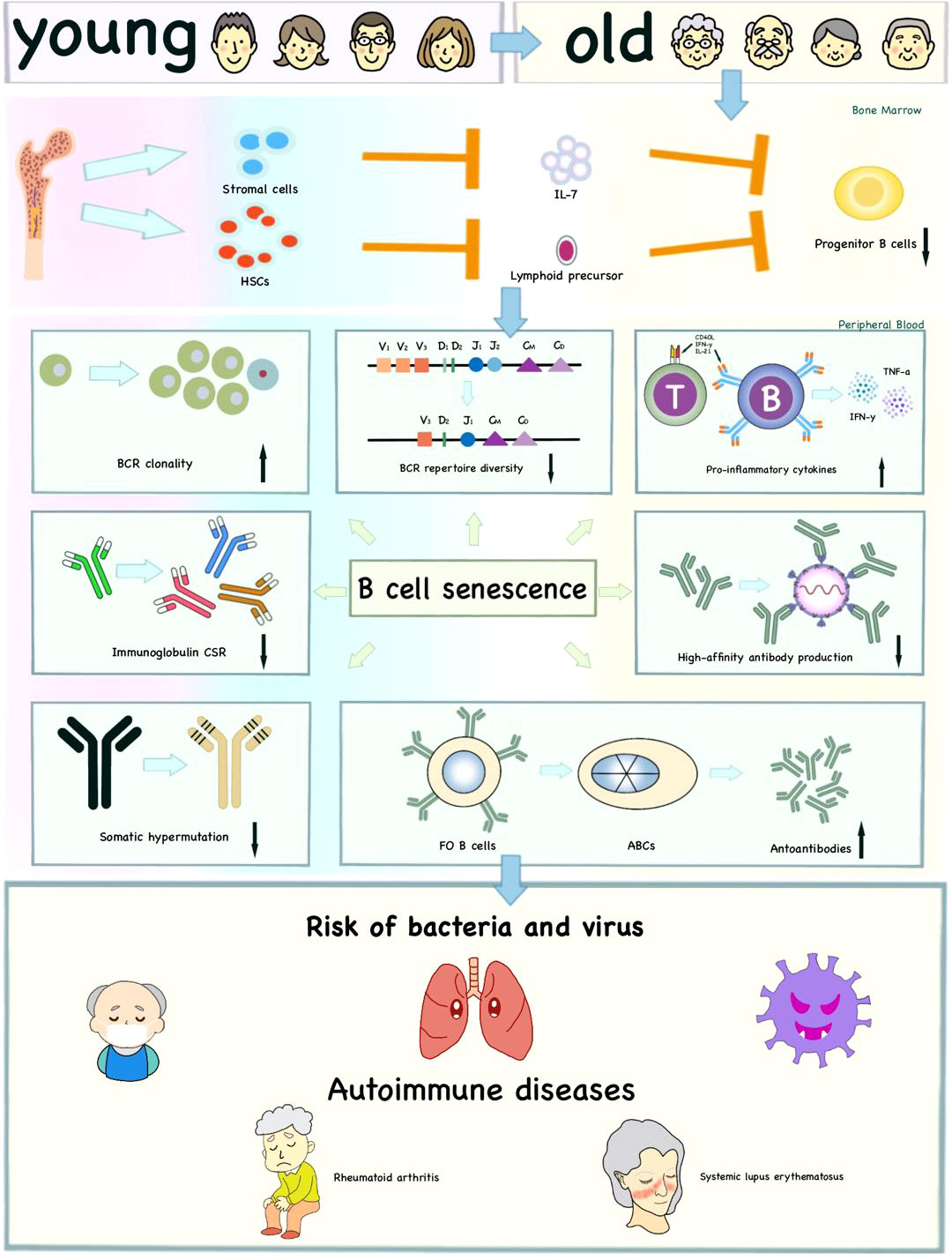
Figure 3 Effects of immunosenescence on B cells and age-related diseases. The reduced lymphoid output and impaired ability of bone marrow stromal cells to release IL-7 influence the production of progenitor B cells with age to some extent. Moreover, immunosenescence affects the intrinsic defects of B cells, including decreased BCR repertoire diversity, CSR, SHM, high-affinity antibody production and increased BCR clonality. The ABCs increase autoantibodies and pro-inflammatory cytokines. These age-related changes together increase the risk of infection and autoimmune diseases in the elderly.
4.2 Innate immune cells
4.2.1 NK cells
NK cells, as important components of human immunity, are a population of large granular lymphocytes with cytotoxic and immunomodulatory functions. Aging redistributes NK cells in numbers, phenotypes and functions (134).
NK cells contain two subpopulations, CD56bright immunoregulatory cells and CD56dim cytotoxic cells, which are differentially influenced by aging (135). While CD56bright cells are decreased in old individuals, CD56dim cells are expanded, suggesting the increase of NK cells with age results mainly from the expansion of CD56dim cells. Therefore, aging induces NK cells from immature but robust cytokine producers CD56bright NK cells to experienced and terminally differentiated CD56dimCD57+NK cells (136, 137). CD56dimCD57+cells are highly differentiated NK cell subpopulations, which have higher cytotoxic capacity, lower cytokine responsiveness and proliferation ability (138). During the aging process, CD56dimNK cells continue to differentiate and the expression of activated receptors natural-killer group 2 member D (NKG2D), immunoglobulin-like killer receptors (KIR), CD57 and CD16 increases, while the expression of activated receptors NKG2C and inhibitory receptors NKG2A decreases (139). Therefore, the cytotoxic function of NK cells is determined by the balance of activatory and inhibitory membrane receptor signals.
It has been shown that NK cell-activating cytokines (such as IL-2, IFN-α and IFN-γ) in old individuals are reduced, especially aged 75 to 85. IL-2 is an intermediary between innate and adaptive immunity and contributes to T cell and NK cell proliferation. Decreased cytokines (especially IL-2) may damage the immune response, leading to an increased incidence of infections among the elderly (140, 141). Moreover, aging may amplify sex difference in NK cells. Immature CD56brightNK cells and mature CD56dimNK cells in old women account for higher ratio. NK cells in female show stronger cytotoxicity, IFN-α responses to NKp46 crosslinking and MIP-1β production against external threats (142).
4.2.2 Other cells
DCs, as central orchestrators of the immune response, are a bridge between innate and adaptive immunity. There is no significant effect on DC numbers and phenotypes in old humans, nevertheless, NK cells in skin and plasma cells (also called langerhans cells) are found to decrease. Besides, senescence could compromise the functions of DCs with regard to antigen uptake, phagocytic activity, chemotaxis and migration and cytokine secretion (143–145).
Neutrophils are a critical component of innate immunity (146). In aged individuals, a low-grade inflammatory state could lead to epigenetic changes in neutrophils which causes specific abnormalities in metabolism and function, such as diminished phagocytic ability (147), abnormal adhesion and chemotaxis (148), increased apoptosis (149), reduced NETs release (150) and TLR dysfunction (151).
Macrophages, as potent immunoregulatory innate immune cells, have a crucial effect on immune defense and regulation of inflammation (152). It has been highlighted that aging could disrupt circadian gene regulation and function of macrophages (153). Meanwhile, aging could be reversed by reprogramming glucose metabolism of macrophages and re-establishing youthful immune homeostasis (154).
5 The impact of immunosenescence on age-related diseases
5.1 Immunosenescence and neurodegenerative diseases
Alzheimer’s disease (AD), one of the most severe neurodegenerative diseases in the elderly, is characterized by elevated amyloid-β (Aβ) plaque deposition, neuroinflammation and brain-resident immune cells (microglia) (155–157). Elevated Aβ deposition can be captured by local APCs in the brain, which causes the activation and expansion of Aβ-reactive T cells, ultimately resulting in brain inflammation (158). AD patients have lower naive cells, higher memory cells and a significant telomere shortening of T cells (159, 160). The analysis of flow cytometry on peripheral blood of AD patients shows that CD8+T effector memory CD45RA+ (TEMRA) cells increase and TCR signaling is enhanced (161). Growing evidence indicates immunosurveillant CD8 T cells in the human brain, which represents central nervous system aging. Senescent T cells participate in enhancing proinflammatory effects of microglia, such as elevated proinflammatory cytokines, increased reactive oxygen species, dysfunctional lysosomal deposits, and eventually promote neuroinflammation (162, 163). Accumulated Treg cells in the peripheral immune system can impair the inflammation resolving immune cells’ infiltration into the central nervous system to suppress AD neuroinflammation, so targeting Treg cells has been an effective strategy to alleviate AD (157). These findings suggest immunity is involved in the development of neurodegenerative diseases, but further researches are necessary to study the interaction between senescent cell subsets and AD.
5.2 Immunosenescence and cancers
The declined immunity in older humans may increase the risk of cancers, which may be mediated by multiple cells (164, 165). In elderly people, immune function is obviously suppressed, which leads to increased tumor-infiltrating Treg cells, promoting tumor growth and metastasis (166, 167). For example, patients with breast cancer are found to exhibit immunosenescence, especially CD8+T cells (168). Macrophages contain two basic polarized states, proinflammatory classical activated (M1) and anti-inflammatory alternatively activated macrophages (M2). Tumor-associated macrophages (TAMs) usually display M2-like phenotypes to inhibit T cell activation and promote tumor metastasis, but macrophages can be polarized to kill tumor cells. Therefore, regulating the polarization of macrophages has been the potential effective strategies for anti-tumor therapy (169, 170). However, NK and B cells are less documented compared to T cells and macrophages of the tumor microenvironment, so extensive elucidations are expected in future.
5.3 Immunosenescence and cardiovascular diseases
Cardiovascular disease, which is associated with immunosenescence, has a high prevalence in the elderly population and is the leading cause of death among the elderly. T cells accelerate aging in patients with coronary heart disease and acute myocardial infarction, including telomere shortening and decreased expression of CD28 (171). Senescent T cells secrete pro-inflammatory cytokines, which activate macrophages and release metalloproteinases to degrade extracellular matrix (172). Senescent T cells also release cytotoxic components, such as perforin and granzymes, which damage endothelial cells and vascular smooth muscle cells directly (173). These results suggest senescent T cells may be involved in the pathophysiological process of cardiovascular diseases through inflammatory response and cytotoxicity.
Uncontrolled activation of the immune system has been resulted from the pathogenesis of hypertension, especially increased cytotoxic T cells (CD28- and CD57+) (174) and senescent NK cells that promote vascular remodeling and angiogenesis (175), which amplify the hypertensive action by releasing proinflammatory cytokines and cytotoxic mediators. With the increase of age, the accumulation of proinflammatory cytokines might increase monocyte specific TLR signaling, which is associated with the development of chronic heart failure (176, 177). Furthermore, senescent T cells are related to cardiovascular disease-related risk factors. For example, CD8+CD28-T cells accumulate in individuals with CMV infections that increase vascular inflammation and arterial blood pressure, promoting the occurrence of cardiovascular diseases (6, 178). In future, it needs to explore the impact of T cell senescence on cardiovascular diseases and determine whether senescent T cells are drivers or results of cardiovascular diseases.
5.4 Immunosenescence and autoimmune diseases
Immunosenescence is also associated with autoimmune diseases, especially RA. Immunosenescence is often accompanied by increased level of inflammation and both of them are the major contributors to age-related diseases. High levels of the pro-inflammatory cytokines, such as TNF and IL-6, lead to chronic inflammatory states for long time, which cause Th17/Treg imbalance and amplified immune response in the development of RA (179, 180). Nowadays, many studies have confirmed that RA patients exhibit premature immunosenescence, including thymus degeneration, clonal expansion of peripheral T cells and the loss of costimulatory receptor CD28 (181). Immunesenescence also deteriorates both articular and extra-articular manifestations, for example, CD4+CD28-T cells are especially marked in RA patients who have extra-articular inflammations or atherosclerotic diseases, and CD28-T cells are associated with poor cognitive functions of RA patients (181, 182). At present, the effects of T cell senescence on the occurrence and development of RA have been widely concerned, and other immune cells (such as Treg cells on RA, T/B cells on lupus or Sjogren Syndrome) have been reported, suggesting immunosenescence has an adverse effect on autoimmune diseases.
5.5 Immunosenescence and COVID-19
Due to aging-related immune changes, the pulmonary and systemic inflammatory responses are intensified, causing an increased risk of respiratory bacterial and viral infection such as influenza and the COVID-19 in the elderly (183, 184). The numbers of monocytes increase in the elderly, especially CD14 monocytes, which have high inflammatory gene expression and activate inflammatory signaling pathways, leading to the reduced ability of immune response (185). During the new crown epidemic, the relationship between immunosenescence and infections has received unprecedented attention. The COVID-19 has resulted in many deaths in the globe, which is characterized by hyperinflammation and cytokine storm severely involved in the lung, heart, kidneys and other multiple organs and systems (186). The elderly with COVID-19 show rapid clinical progress, high incidence and mortality (187–189), accompanying with heavy systemic inflammation and tissue damages, which would be related to immunosenescence, such as the decrease of plasmacytoid DCs (pDCs), alveolar macrophages and NK cells (183) and the increase of IGSF21+ DCs (187), neutrophils and CD14 monocytes (185). The immune cell sequencing shows that SARS-CoV-2 promotes immune cell polarization, mainly from naive T cells to memory/effector T cells, and gene expression associated with inflammation and cell aging (128). The COVID-19 can activate CD4+T lymphocytes to differentiate into pathogenic Th1 cells and produce cytokines (GM-CSF, etc), triggering cytokine storm (190). Severe SARS-CoV-2 patients show lessened number of CD4+ and CD8+T cells that express higher inhibitory receptors such as PD-1 and Tim-3, suggesting an exhausted status in T cells (185). Besides, the SARS-CoV-2 virus causes CD8+T cell senescence via TCR signaling and expressing CTLA-4 and TIGIT, and makes senescent CD8+T cells unable to release perforin and granzymes, which may explain susceptibility among the elderly (191). Actually, the decrease of adaptive immune response plays little role in COVID-19 mortality. Multiple organ failure and death are more associated with hyperfunctional natural immunity, high inflammation level and cytokine storm (186). To sum up, compromised immune function of the elderly is easier for the virus to spread and damage the tissues, which reinforces the necessity to resist immunosenescence and improve the immune function (such as the vaccine) to protect the body from the COVID-19.
Older people have a reduced response to vaccination because of immunosenescence, so it is important to strengthen the research on the safety and effectiveness of COVID-19 vaccine among the elderly population. However, the current studies on immune response in the elderly after COVID-19 vaccination have drawn different conclusions. The mRNA-1273 vaccine induces similar neutralizing antibody levels in different age groups (192). BNT162b1 and BNT162b2 vaccine induce similar neutralizing antibody titers between young and old people (193). ChAdOx1 nCoV-19 vaccine induces strong neutralizing antibody responses and cellular immune response against the spike glycoprotein at all ages, and the vaccine causes fewer side effects in the elderly (194). Despite the low neutralizing antibody level of the elderly, the mRNA vaccine produces similar immune response rates between old and young adults, which is opposite to other traditional vaccines. The mRNA vaccine, as a new type of vaccine, plays a much larger role in germinal center response, neutralizing antibody production, Tfh cell response and specific memory B cell response than traditional vaccine (195). Remarkably, mRNA vaccine enhances neutralizing antibody production after the second immunization, while traditional vaccine enhances non-specific antibodies (195). Furthermore, other studies have shown that the serum neutralizing antibody level of the elderly is still low after the first dose of BNT162b2 vaccine, but antibody immune response is improved significantly against variants of concern (VOC) after the second dose (196). The new crown inactivated vaccine booster makes SARS-CoV-2-specific memory B lymphocytes (about 7%) carry broad-spectrum neutralizing antibodies to provide effective protection against Omicron variant (197). Therefore, the elderly should not only be vaccinated actively, but also vaccinated with booster shots to resist diminished vaccine potency. Nowadays, mRNA vaccine has gradually replaced the traditional vaccine as the most popular vaccine for vaccinators of different ages (especially the elderly). However, vaccine induced protective mechanism of old vaccinators are still unclear, and more researches remain to be done.
6 Immunosenescence and successful aging
For a long time, immunosenescence has been considered harmful. However, it is noteworthy that immunosenescence is a remodeling and retuning process with increase in some new functions rather than complete decline of immune function (9). Serum levels of lgG and lgA are increased with age, which is conducive to protecting against viral and bacterial infections effectively in older people (198). Although the generation of naive T/B cells continues to decline, the adaptive immune system adjusts to age-related changes and protects the body from most pathogens. Only later in life does the immune function decline gradually, which increases morbidity and mortality in the elderly (199). But not all older people suffer from age-related diseases, centenarians can delay the aging process and live up to the limits of human life. Centenarians have a large quantity of anti-inflammatory molecules, such as TGF-β1, IL-10 and IL-1 receptor antagonist (IL-1RA), to counterbalance increased inflammatory molecules, such as IL-1β, IL-6, TNF-α, IL-8, C-reactive protein (CRP) and CXCL9, achieving a dynamic balance between pro-inflammatory and anti-inflammatory levels (8). In addition, telomere length and telomerase activity are higher in centenarians (200).
In centenarians, the degradation of immune function is not obvious. Interestingly, the expansion of cytotoxic CD4+ T cells has been found in supercentenarians and makes them resistant to diseases (201). CD8+T cells of centenarians are highly differentiated with decreased CD28 expression (which are also called CD8+CD28−, CD8+KIR+, NK-like CD8+ or innate CD8+T cells) (202) and higher CD45RA expression (203). In centenarian offspring, the number of B cells decreases significantly, but naive B cells and IgM increase, which might be one of the reasons for resisting infection and prolonging the lives (204). The cytotoxic capability of NK cells in centenarians (up to about 55%), which is very similar to the young groups (about 63%), is higher than that in middle-aged groups (about 33%) (205). NK T cells bearing γδ TCR show higher cytotoxicity and IFN-γ production in centenarians, which is beneficial to fighting diseases and successful aging (141). Moreover, neutrophil chemotaxis and microbicidal capacity and lymphoproliferation are higher in centenarians, while neutrophil and lymphocyte adherence are lower (206). Therefore, anti-inflammatory molecules, cytotoxic CD4+ T cells, naive B cells and well-preserved NK cells would be the hallmark of successful aging (Figure 4).
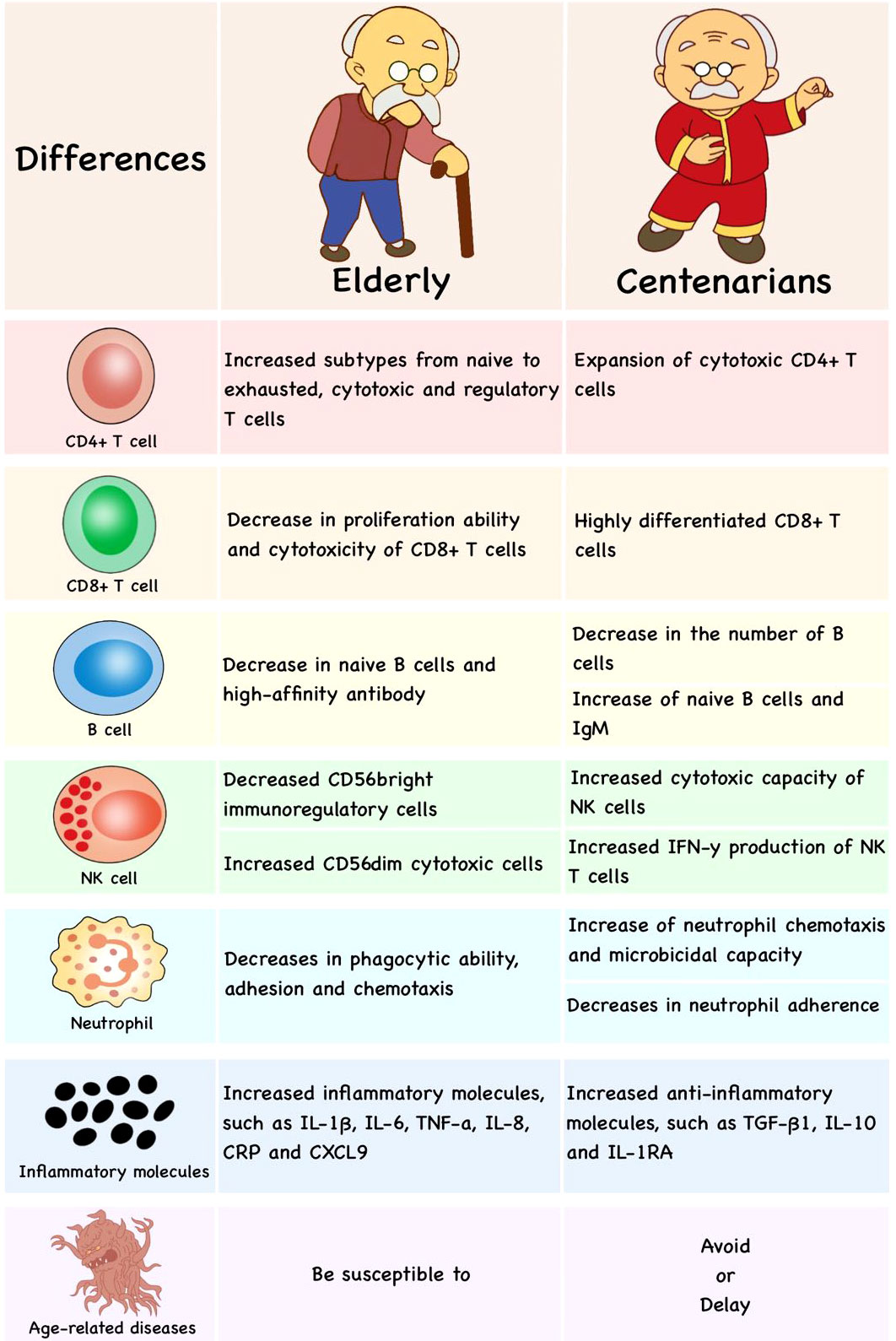
Figure 4 The differences in immune cells of published researches between the elderly and centenarians. Compared with the elderly, centenarians have more anti-inflammatory molecules, cytotoxic CD4+T cells, highly differentiated CD8+T cells, naive B cells and well-preserved NK cells, which would be the hallmark of successful aging.
7 Therapeutic strategies for successful aging
There are currently several strategies to deal with senescence and senescent cells. First of all, rejuvenation of old HSCs may be an effective therapeutic strategy to restore the balance between myeloid and lymphatic systems and the numbers of T and B cells (207). The involution of the thymus is one of the main features of aging, which might lead to the decrease of T cells, so restoring the structure and function of the aging thymus could reverse immunosenescence (208). Thymo-stimulatory property of IL-10, leptin, keratinocyte growth factor (KGF) and thymic stromal lymphopoietin (TSLP) may contribute to immune reconstitution of the elderly (69). IL-7 is a crucial cytokine for T cell development, so IL-7 treatment promotes the expansion of peripheral T cells and the diversity of TCR (209, 210). Telomerase is a significant component for T cell development, so upregulation of telomerase expression enhances T cell immune response and prolongs lifespan (211, 212).
Senescent cells cause immune cells dysfunction by recruiting SASP, which is connected with many chronic diseases, so clearing senescent cells is of great importance. Senotherapeutic strategies contain two types: senolytics (removing senescent cells selectively) and senomophics (changing senescence phenotypes) (213). A novel senolytic agent ABT-263 causes apoptosis in senescent cells by targeting Bcl-2 family members (a negative regulator of apoptosis) (214). A FOXO4 peptide induces apoptosis of senescent cells by interfering the FOXO4-p53 interaction (215). A component of grape seed extract procyanidin C1 (PCC1) is a natural senolytic agent and extends lifespan in mice (216). Nowadays immunotherapy is a promising therapeutic strategy against senescent cells (217). Modified T cells that express a chimeric antigen receptor (CAR) have been applied in cancer treatments successfully (218), based on which, engineering CAR T-cells with NKG2D receptors contributes to recognizing and eliminating senescent cells with NKG2D ligands (219). Chemotherapeutic agents (doxorubicin, melphalan and bortezomib) enhance the killing effect of NK cells to clear senescent cells by upregulating the expression of NKG2D receptors on tumor cells (220). The main culprit of senescent cells is the SASP, therefore, the way to prevent cell senescence is to control or neutralize SASP by blocking main upstream regulators (such as GATA4, NF-κB and BRD4) or using targeting drugs (221). Rapamycin, a common inhibitor of mTOR, prevents senescence through decreasing markers of senescence in peripheral T cells and inhibiting SASP regulators (222–224). Metformin has been known to reduce SASP by modulating NF-κB signaling and delay the aging process (213).
Immune checkpoint blockade (ICB) therapy has been applied in cancers, for example, PD-L1 and IDO may restrain T cells immunity (225, 226). However, data on the safety and toxicity of ICB therapy are limited, so further researches are required to evaluate the therapeutic effects of ICB especially on the elderly. Treg targeted therapy is vital for cancer therapy and the treatment of autoimmunity, but sometimes has some risks. For example, Treg targeted therapy treats autoimmune diseases through inhibiting autoreactive immune components, but increases tumor immune escape and the risk of cancer, which results in the complexity of Treg targeted therapy (227). Growth differentiation factor 15 (GDF15) is a stress response gene caused by mitochondrial dysfunction and maintains the immunosuppressive function of Treg cells, so the intervention of GDF15 may improve the immune function of the elderly (228). Notably, rituximab is an anti-CD20 monoclonal antibody, which may inhibit pro-inflammatory B cell subsets such as ABCs, combating age-related autoimmune diseases. Fruit and vegetables, richen in carotenoids, increase the number of NK cells and the function of Th cells, ultimately enhancing the immune function (229). Vitamin E supplementation strengthens the function of T cells by reducing PGE2 production in macrophages, having a beneficial effect on healthy elderly (230, 231). It has also been shown that exercise decreases the number of Th17 cells and inflammatory markers and increases the level of IL-7, thymic function and autophagy activity (36, 232), suggesting the contribution of diet and exercise for the plasticity of aging.
The more we understand the cellular and molecular mechanisms of aging, the more opportunities we create to intervene aging and age-related diseases. In conclusion, the evidence suggests that targeting drugs and a good lifestyle together help to boost the immune system and enable the elderly to live longer and heathier.
8 Conclusion
Immunosenescence is a complex and varied process of immune system, which participates in many age-related diseases, with the alteration of immune cell subsets, cytokine secretion and the defect in cell function and quantity (176, 233, 234). It is of significant importance to explore the molecular and cellular mechanisms of immunosenescence with single-cell techniques to dissect some phenomena deeply and systematically (235). Moreover, it is necessary to distinguish markers of immunosenescence, quantify the immunity and establish normal reference range of immune cells among individuals at different age, which contributes to screening, preventing and intervening diseases even at subclinical stage. Therefore, it is obviously necessary to find novel targets and therapy for immunosenescence, for example, vaccines and microbiome regulation, to decline the negative effects of immunosenescence and promote successful aging.
Author contributions
ZG and CS contributed to conception and design of the study. YW and CD complete the review of literature and wrote the first draft of the manuscript. YH contribute to the graphic visualization. All authors contributed to manuscript revision, read, and approved the submitted version.
Funding
This work was supported by National Natural Science Foundation of China (grant number 82071838 and 82101891), Jiangsu province senile health scientific research project (grant number LR2021035) and Science and technology Project of Nantong City (grant number JC2021119) and Postgraduate Research & Practice Innovation Program of Jiangsu Province (grant number SJCX21_1459 and KYCX21_3106).
Conflict of interest
The authors declare that the research was conducted in the absence of any commercial or financial relationships that could be construed as a potential conflict of interest.
Publisher’s note
All claims expressed in this article are solely those of the authors and do not necessarily represent those of their affiliated organizations, or those of the publisher, the editors and the reviewers. Any product that may be evaluated in this article, or claim that may be made by its manufacturer, is not guaranteed or endorsed by the publisher.
References
1. McComb S, Thiriot A, Akache B, Krishnan L, Stark F. Introduction to the immune system. Methods Mol Biol (2019) 2024:1–24. doi: 10.1007/978-1-4939-9597-4_1
2. Parkin J, Cohen B. An overview of the immune system. Lancet (9270) 2001:1777–89:357. doi: 10.1016/S0140-6736(00)04904-7
3. Fulop T, Larbi A, Dupuis G, Le Page A, Frost EH, Cohen AA, et al. Immunosenescence and inflamm-aging as two sides of the same coin: Friends or foes? Front Immunol (2017) 8:1960. doi: 10.3389/fimmu.2017.01960
4. Pawelec G. Age and immunity: What is "immunosenescence"? Exp Gerontol (2018) 105:4–9. doi: 10.1016/j.exger.2017.10.024
5. Fulop T, Larbi A, Hirokawa K, Cohen AA, Witkowski JM. Immunosenescence is both functional/adaptive and dysfunctional/maladaptive. Semin Immunopathol (2020) 42(5):521–36. doi: 10.1007/s00281-020-00818-9
6. Barbé-Tuana F, Funchal G, Schmitz CRR, Maurmann RM, Bauer ME. The interplay between immunosenescence and age-related diseases. Semin Immunopathol (2020) 42(5):545–57. doi: 10.1007/s00281-020-00806-z
7. Müller L, Di Benedetto S, Pawelec G. The immune system and its dysregulation with aging. Subcell Biochem (2019) 91:21–43. doi: 10.1007/978-981-13-3681-2_2
8. Santoro A, Bientinesi E, Monti D. Immunosenescence and inflammaging in the aging process: age-related diseases or longevity? Ageing Res Rev (2021) 71:101422. doi: 10.1016/j.arr.2021.101422
9. Franceschi C, Monti D, Barbieri D, Grassilli E, Troiano L, Salvioli S, et al. Immunosenescence in humans: deterioration or remodelling? Int Rev Immunol (1995) 12(1):57–74. doi: 10.3109/08830189509056702
10. Zhu Y, Tchkonia T, Pirtskhalava T, Gower AC, Ding H, Giorgadze N, et al. The achilles' heel of senescent cells: from transcriptome to senolytic drugs. Aging Cell (2015) 14(4):644–58. doi: 10.1111/acel.12344
11. Guan Y, Zhang C, Lyu G, Huang X, Zhang X, Zhuang T, et al. Senescence-activated enhancer landscape orchestrates the senescence-associated secretory phenotype in murine fibroblasts. Nucleic Acids Res (2020) 48(19):10909–23. doi: 10.1093/nar/gkaa858
12. Chambers CR, Ritchie S, Pereira BA, Timpson P. Overcoming the senescence-associated secretory phenotype (SASP): a complex mechanism of resistance in the treatment of cancer. Mol Oncol (2021) 15(12):3242–55. doi: 10.1002/1878-0261.13042
13. Prata L, Ovsyannikova IG, Tchkonia T, Kirkland JL. Senescent cell clearance by the immune system: Emerging therapeutic opportunities. Semin Immunol (2018) 40:101275. doi: 10.1016/j.smim.2019.04.003
14. Tchkonia T, Zhu Y, van Deursen J, Campisi J, Kirkland JL. Cellular senescence and the senescent secretory phenotype: therapeutic opportunities. J Clin Invest (2013) 123(3):966–72. doi: 10.1172/JCI64098
15. Cuollo L, Antonangeli F, Santoni A, Soriani A. The senescence-associated secretory phenotype (SASP) in the challenging future of cancer therapy and age-related diseases. Biol (Basel) (2020) 9(12):485. doi: 10.3390/biology9120485
16. Rodier F, Campisi J. Four faces of cellular senescence. J Cell Biol (2011) 192(4):547–56. doi: 10.1083/jcb.201009094
17. Birch J, Gil J. Senescence and the SASP: many therapeutic avenues. Genes Dev (2020) 34(23-24):1565–76. doi: 10.1101/gad.343129.120
18. Zhu Y, Armstrong JL, Tchkonia T, Kirkland JL. Cellular senescence and the senescent secretory phenotype in age-related chronic diseases. Curr Opin Clin Nutr Metab Care (2014) 17(4):324–8. doi: 10.1097/MCO.0000000000000065
19. Ray D, Yung R. Immune senescence, epigenetics and autoimmunity. Clin Immunol (2018) 196:59–63. doi: 10.1016/j.clim.2018.04.002
20. Biagi E, Nylund L, Candela M, Ostan R, Bucci L, Pini E, et al. Through ageing, and beyond: gut microbiota and inflammatory status in seniors and centenarians. PLoS One (2010) 5(5):e10667. doi: 10.1371/journal.pone.0010667
21. Sanada F, Taniyama Y, Muratsu J, Otsu R, Shimizu H, Rakugi H, et al. Source of chronic inflammation in aging. Front Cardiovasc Med (2018) 5:12. doi: 10.3389/fcvm.2018.00012
22. Mangiola F, Nicoletti A, Gasbarrini A, Ponziani FR. Gut microbiota and aging. Eur Rev Med Pharmacol Sci (2018) 22(21):7404–13. doi: 10.26355/eurrev_201811_16280
23. Franceschi C, Garagnani P, Parini P, Giuliani C, Santoro A. Inflammaging: a new immune-metabolic viewpoint for age-related diseases. Nat Rev Endocrinol (2018) 14(10):576–90. doi: 10.1038/s41574-018-0059-4
24. Conte M, Martucci M, Chiariello A, Franceschi C, Salvioli S. Mitochondria, immunosenescence and inflammaging: a role for mitokines? Semin Immunopathol (2020) 42(5):607–17. doi: 10.1007/s00281-020-00813-0
25. Salminen A. Immunosuppressive network promotes immunosenescence associated with aging and chronic inflammatory conditions. J Mol Med (Berl) (2021) 99(11):1553–69. doi: 10.1007/s00109-021-02123-w
26. De Maeyer RPH, Chambers ES. The impact of ageing on monocytes and macrophages. Immunol Lett (2021) 230:1–10. doi: 10.1016/j.imlet.2020.12.003
27. Li MO, Wan YY, Sanjabi S, Robertson AK, Flavell RA. Transforming growth factor-beta regulation of immune responses. Annu Rev Immunol (2006) 24:99–146. doi: 10.1146/annurev.immunol.24.021605.090737
28. Fulop T, Dupuis G, Baehl S, Le Page A, Bourgade K, Frost E, et al. From inflamm-aging to immune-paralysis: a slippery slope during aging for immune-adaptation. Biogerontology (2016) 17(1):147–57. doi: 10.1007/s10522-015-9615-7
29. Frasca D, Romero M, Diaz A, Alter-Wolf S, Ratliff M, Landin AM, et al. A molecular mechanism for TNF-α-mediated downregulation of b cell responses. J Immunol (2012) 188(1):279–86. doi: 10.4049/jimmunol.1003964
30. Frasca D, Diaz A, Romero M, Landin AM, Blomberg BB. High TNF-α levels in resting b cells negatively correlate with their response. Exp Gerontol (2014) 54:116–22. doi: 10.1016/j.exger.2014.01.004
31. Roquilly A, Jacqueline C, Davieau M, Mollé A, Sadek A, Fourgeux C, et al. Alveolar macrophages are epigenetically altered after inflammation, leading to long-term lung immunoparalysis. Nat Immunol (2020) 21(6):636–48. doi: 10.1038/s41590-020-0673-x
32. Blackburn EH, Epel ES, Lin J. Human telomere biology: A contributory and interactive factor in aging, disease risks, and protection. Science (2015) 350(6265):1193–8. doi: 10.1126/science.aab3389
33. Aubert G, Baerlocher GM, Vulto I, Poon SS, Lansdorp PM. Collapse of telomere homeostasis in hematopoietic cells caused by heterozygous mutations in telomerase genes. PloS Genet (2012) 8(5):e1002696. doi: 10.1371/journal.pgen.1002696
34. Lin Y, Damjanovic A, Metter EJ, Nguyen H, Truong T, Najarro K, et al. Age-associated telomere attrition of lymphocytes in vivo is co-ordinated with changes in telomerase activity, composition of lymphocyte subsets and health conditions. Clin Sci (Lond) (2015) 128(6):367–77. doi: 10.1042/CS20140481
35. Fali T, Papagno L, Bayard C, Mouloud Y, Boddaert J, Sauce D, et al. New insights into lymphocyte differentiation and aging from telomere length and telomerase activity measurements. J Immunol (2019) 202(7):1962–9. doi: 10.4049/jimmunol.1801475
36. Zhou D, Borsa M, Simon AK. Hallmarks and detection techniques of cellular senescence and cellular ageing in immune cells. Aging Cell (2021) 20(2):e13316. doi: 10.1111/acel.13316
37. de Punder K, Heim C, Wadhwa PD, Entringer S. Stress and immunosenescence: The role of telomerase. Psychoneuroendocrinology (2019) 101:87–100. doi: 10.1016/j.psyneuen.2018.10.019
38. Lin J, Epel E, Cheon J, Kroenke C, Sinclair E, Bigos M, et al. Analyses and comparisons of telomerase activity and telomere length in human T and b cells: insights for epidemiology of telomere maintenance. J Immunol Methods (2010) 352(1-2):71–80. doi: 10.1016/j.jim.2009.09.012
39. Effros RB. Kleemeier award lecture 2008–the canary in the coal mine: telomeres and human healthspan. J Gerontol A Biol Sci Med Sci (2009) 64(5):511–5. doi: 10.1093/gerona/glp001
40. Huang EE, Tedone E, O'Hara R, Cornelius C, Lai TP, Ludlow A, et al. The maintenance of telomere length in CD28+ T cells during T lymphocyte stimulation. Sci Rep (2017) 7(1):6785. doi: 10.1038/s41598-017-05174-7
41. Mathis D, Shoelson SE. Immunometabolism: an emerging frontier. Nat Rev Immunol (2011) 11(2):81. doi: 10.1038/nri2922
42. Li PH, Zhang R, Cheng LQ, Liu JJ, Chen HZ. Metabolic regulation of immune cells in proinflammatory microenvironments and diseases during ageing. Ageing Res Rev (2020) 64:101165. doi: 10.1016/j.arr.2020.101165
43. Waters LR, Ahsan FM, Ten Hoeve J, Hong JS, Kim DNH, Minasyan A, et al. Ampk regulates IgD expression but not energy stress with b cell activation. Sci Rep (2019) 9(1):8176. doi: 10.1038/s41598-019-43985-y
44. Kurupati RK, Haut LH, Schmader KE, Ertl HC. Age-related changes in b cell metabolism. Aging (Albany NY) (2019) 11(13):4367–81. doi: 10.18632/aging.102058
45. Imai SI. The NAD world 2.0: the importance of the inter-tissue communication mediated by NAMPT/NAD(+)/SIRT1 in mammalian aging and longevity control. NPJ Syst Biol Appl (2016) 2:16018. doi: 10.1038/npjsba.2016.18
46. Imai S, Guarente L. NAD+ and sirtuins in aging and disease. Trends Cell Biol (2014) 24(8):464–71. doi: 10.1016/j.tcb.2014.04.002
47. Lee KA, Robbins PD, Camell CD. Intersection of immunometabolism and immunosenescence during aging. Curr Opin Pharmacol (2021) 57:107–16. doi: 10.1016/j.coph.2021.01.003
48. Campisi J, Kapahi P, Lithgow GJ, Melov S, Newman JC, Verdin E. From discoveries in ageing research to therapeutics for healthy ageing. Nature (2019) 571(7764):183–92. doi: 10.1038/s41586-019-1365-2
49. Han C, Ge M, Ho PC, Zhang L. Fueling T-cell antitumor immunity: Amino acid metabolism revisited. Cancer Immunol Res (2021) 9(12):1373–82. doi: 10.1158/2326-6066.CIR-21-0459
50. López-Otín C, Blasco MA, Partridge L, Serrano M, Kroemer G. The hallmarks of aging. Cell (2013) 153(6):1194–217. doi: 10.1016/j.cell.2013.05.039
51. Yamashita M, Iwama A. Aging and clonal behavior of hematopoietic stem cells. Int J Mol Sci (2022) 23(4):1948. doi: 10.3390/ijms23041948
52. Kohn DB, Bauer G, Rice CR, Rothschild JC, Carbonaro DA, Valdez P, et al. A clinical trial of retroviral-mediated transfer of a rev-responsive element decoy gene into CD34(+) cells from the bone marrow of human immunodeficiency virus-1-infected children. Blood (1999) 94(1):368–71. doi: 10.1182/blood.V94.1.368.413a47_368_371
53. Pang WW, Price EA, Sahoo D, Beerman I, Maloney WJ, Rossi DJ, et al. Human bone marrow hematopoietic stem cells are increased in frequency and myeloid-biased with age. Proc Natl Acad Sci U S A (2011) 108(50):20012–7. doi: 10.1073/pnas.1116110108
54. Snoeck HW. Aging of the hematopoietic system. Curr Opin Hematol (2013) 20(4):355–61. doi: 10.1097/MOH.0b013e3283623c77
55. Cho RH, Sieburg HB, Muller-Sieburg CE. A new mechanism for the aging of hematopoietic stem cells: aging changes the clonal composition of the stem cell compartment but not individual stem cells. Blood (2008) 111(12):5553–61. doi: 10.1182/blood-2007-11-123547
56. Min H, Montecino-Rodriguez E, Dorshkind K. Effects of aging on the common lymphoid progenitor to pro-b cell transition. J Immunol (2006) 176(2):1007–12. doi: 10.4049/jimmunol.176.2.1007
57. Miller JP, Allman D. The decline in b lymphopoiesis in aged mice reflects loss of very early b-lineage precursors. J Immunol (2003) 171(5):2326–30. doi: 10.4049/jimmunol.171.5.2326
58. Kogut I, Scholz JL, Cancro MP, Cambier JC. B cell maintenance and function in aging. Semin Immunol (2012) 24(5):342–9. doi: 10.1016/j.smim.2012.04.004
59. Challen GA, Boles NC, Chambers SM, Goodell MA. Distinct hematopoietic stem cell subtypes are differentially regulated by TGF-beta1. Cell Stem Cell (2010) 6(3):265–78. doi: 10.1016/j.stem.2010.02.002
60. Stephan RP, Reilly CR, Witte PL. Impaired ability of bone marrow stromal cells to support b-lymphopoiesis with age. Blood (1998) 91(1):75–88. doi: 10.1182/blood.V91.1.75
61. Frasca D, Diaz A, Romero M, Garcia D. Blomberg BB. b cell immunosenescence. Annu Rev Cell Dev Biol (2020) 36:551–74. doi: 10.1146/annurev-cellbio-011620-034148
62. Kyoizumi S, Kubo Y, Kajimura J, Yoshida K, Imai K, Hayashi T, et al. Age-associated changes in the differentiation potentials of human circulating hematopoietic progenitors to T- or NK-lineage cells. J Immunol (2013) 190(12):6164–72. doi: 10.4049/jimmunol.1203189
63. Wang CQ, Udupa KB, Xiao H, Lipschitz DA. Effect of age on marrow macrophage number and function. Aging (Milano) (1995) 7(5):379–84. doi: 10.1007/BF03324349
64. Gayoso I, Sanchez-Correa B, Campos C, Alonso C, Pera A, Casado JG, et al. Immunosenescence of human natural killer cells. J Innate Immun (2011) 3(4):337–43. doi: 10.1159/000328005
65. Shirakawa K, Sano MT. Cell immunosenescence in aging, obesity, and cardiovascular disease. Cells (2021) 10(9):2435. doi: 10.3390/cells10092435
66. Andrew D, Aspinall R. Age-associated thymic atrophy is linked to a decline in IL-7 production. Exp Gerontol (2002) 37(2-3):455–63. doi: 10.1016/S0531-5565(01)00213-3
67. Hamazaki Y. Adult thymic epithelial cell (TEC) progenitors and TEC stem cells: Models and mechanisms for TEC development and maintenance. Eur J Immunol (2015) 45(11):2985–93. doi: 10.1002/eji.201545844
68. Hamazaki Y, Fujita H, Kobayashi T, Choi Y, Scott HS, Matsumoto M, et al. Medullary thymic epithelial cells expressing aire represent a unique lineage derived from cells expressing claudin. Nat Immunol (2007) 8(3):304–11. doi: 10.1038/ni1438
69. Gruver AL, Hudson LL, Sempowski GD. Immunosenescence of ageing. J Pathol (2007) 211(2):144–56. doi: 10.1002/path.2104
70. Rezzani R, Nardo L, Favero G, Peroni M, Rodella LF. Thymus and aging: morphological, radiological, and functional overview. Age (Dordr) (2014) 36(1):313–51. doi: 10.1007/s11357-013-9564-5
71. JH J, DG M. The immune response against human cytomegalovirus links cellular to systemic senescence. Cells (2020) 9(3):766. doi: 10.3390/cells9030766
72. Britanova OV, Shugay M, Merzlyak EM, Staroverov DB, Putintseva EV, Turchaninova MA, et al. Dynamics of individual T cell repertoires: From cord blood to centenarians. J Immunol (2016) 196(12):5005–13. doi: 10.4049/jimmunol.1600005
73. Czesnikiewicz-Guzik M, Lee WW, Cui D, Hiruma Y, Lamar DL, Yang ZZ, et al. T Cell subset-specific susceptibility to aging. Clin Immunol (2008) 127(1):107–18. doi: 10.1016/j.clim.2007.12.002
74. Le Page A, Dupuis G, Larbi A, Witkowski JM, Fülöp T. Signal transduction changes in CD4(+) and CD8(+) T cell subpopulations with aging. Exp Gerontol (2018) 105:128–39. doi: 10.1016/j.exger.2018.01.005
75. Coder BD, Wang H, Ruan L, Su DM. Thymic involution perturbs negative selection leading to autoreactive T cells that induce chronic inflammation. J Immunol (2015) 194(12):5825–37. doi: 10.4049/jimmunol.1500082
76. Oh J, Wang W, Thomas R, Su DM. Capacity of tTreg generation is not impaired in the atrophied thymus. PloS Biol (2017) 15(11):e2003352. doi: 10.1371/journal.pbio.2003352
77. Kadambari S, Klenerman P, Pollard AJ. Why the elderly appear to be more severely affected by COVID-19: The potential role of immunosenescence and CMV. Rev Med Virol (2020) 30(5):e2144. doi: 10.1002/rmv.2144
78. Park J, Miyakawa T, Shiokawa A, Nakajima-Adachi H, Tanokura M, Hachimura S. Splenic stromal cells from aged mice produce higher levels of IL-6 compared to young mice. Mediators Inflamm (2014) 2014:826987. doi: 10.1155/2014/826987
79. Lefebvre JS, Maue AC, Eaton SM, Lanthier PA, Tighe M, Haynes L. The aged microenvironment contributes to the age-related functional defects of CD4 T cells in mice. Aging Cell (2012) 11(5):732–40. doi: 10.1111/j.1474-9726.2012.00836.x
80. Li G, Smithey MJ, Rudd BD, Nikolich-Žugich J. Age-associated alterations in CD8α+ dendritic cells impair CD8 T-cell expansion in response to an intracellular bacterium. Aging Cell (2012) 11(6):968–77. doi: 10.1111/j.1474-9726.2012.00867.x
81. Connoy AC, Trader M, High KP. Age-related changes in cell surface and senescence markers in the spleen of DBA/2 mice: a flow cytometric analysis. Exp Gerontol (2006) 41(2):225–9. doi: 10.1016/j.exger.2005.11.003
82. Lazuardi L, Jenewein B, Wolf AM, Pfister G, Tzankov A, Grubeck-Loebenstein B. Age-related loss of naïve T cells and dysregulation of T-cell/B-cell interactions in human lymph nodes. Immunology (2005) 114(1):37–43. doi: 10.1111/j.1365-2567.2004.02006.x
83. Thompson HL, Smithey MJ, Surh CD, Nikolich-Žugich J. Functional and homeostatic impact of age-related changes in lymph node stroma. Front Immunol (2017) 8:706. doi: 10.3389/fimmu.2017.00706
84. Turner VM, Mabbott NA. Structural and functional changes to lymph nodes in ageing mice. Immunology (2017) 151(2):239–47. doi: 10.1111/imm.12727
85. Briceño O, Lissina A, Wanke K, Afonso G, von Braun A, Ragon K, et al. Reduced naïve CD8(+) T-cell priming efficacy in elderly adults. Aging Cell (2016) 15(1):14–21. doi: 10.1111/acel.12384
86. Budamagunta V, Foster TC, Zhou D. Cellular senescence in lymphoid organs and immunosenescence. Aging (Albany NY) (2021) 13(15):19920–41. doi: 10.18632/aging.203405
87. Zhu J, Yamane H, Paul WE. Differentiation of effector CD4 T cell populations (*). Annu Rev Immunol (2010) 28:445–89. doi: 10.1146/annurev-immunol-030409-101212
88. Dutta A, Venkataganesh H, Love PE. Epigenetic regulation of T cell development. Int Rev Immunol (2021), 1–9. doi: 10.1080/08830185.2021.2022661
89. White HD, Crassi KM, Givan AL, Stern JE, Gonzalez JL, Memoli VA, et al. CD3+ CD8+ CTL activity within the human female reproductive tract: influence of stage of the menstrual cycle and menopause. J Immunol (1997) 158(6):3017–27.
90. Larbi A, Fulop T. From "truly naïve" to "exhausted senescent" T cells: when markers predict functionality. Cytom A (2014) 85(1):25–35. doi: 10.1002/cyto.a.22351
91. Pawelec G. Immunosenenescence: role of cytomegalovirus. Exp Gerontol (2014) 54:1–5. doi: 10.1016/j.exger.2013.11.010
92. Li M, Yao D, Zeng X, Kasakovski D, Zhang Y, Chen S, et al. Age related human T cell subset evolution and senescence. Immun Ageing (2019) 16:24. doi: 10.1186/s12979-019-0165-8
93. Sharabi A, Tsokos GC. T Cell metabolism: new insights in systemic lupus erythematosus pathogenesis and therapy. Nat Rev Rheumatol (2020) 16(2):100–12. doi: 10.1038/s41584-019-0356-x
94. Yang X, Wang X, Lei L, Sun L, Jiao A, Zhu K, et al. Age-related gene alteration in naïve and memory T cells using precise age-tracking model. Front Cell Dev Biol (2020) 8:624380. doi: 10.3389/fcell.2020.624380
95. Hu B, Li G, Ye Z, Gustafson CE, Tian L, Weyand CM, et al. Transcription factor networks in aged naïve CD4 T cells bias lineage differentiation. Aging Cell (2019) 18(4):e12957. doi: 10.1111/acel.12957
96. Elyahu Y, Hekselman I, Eizenberg-Magar I, Berner O, Strominger I, Schiller M, et al. Aging promotes reorganization of the CD4 T cell landscape toward extreme regulatory and effector phenotypes. Sci Adv (2019) 5(8):eaaw8330. doi: 10.1126/sciadv.aaw8330
97. Varricchi G, Bencivenga L, Poto R, Pecoraro A, Shamji MH, Rengo G. The emerging role of T follicular helper (T(FH)) cells in aging: Influence on the immune frailty. Ageing Res Rev (2020) 61:101071. doi: 10.1016/j.arr.2020.101071
98. Choi YS, Kageyama R, Eto D, Escobar TC, Johnston RJ, Monticelli L, et al. ICOS receptor instructs T follicular helper cell versus effector cell differentiation via induction of the transcriptional repressor Bcl6. Immunity (2011) 34(6):932–46. doi: 10.1016/j.immuni.2011.03.023
99. Schmitt N, Bustamante J, Bourdery L, Bentebibel SE, Boisson-Dupuis S, Hamlin F, et al. IL-12 receptor β1 deficiency alters in vivo T follicular helper cell response in humans. Blood (2013) 121(17):3375–85. doi: 10.1182/blood-2012-08-448902
100. Ouyang X, Yang Z, Zhang R, Arnaboldi P, Lu G, Li Q, et al. Potentiation of Th17 cytokines in aging process contributes to the development of colitis. Cell Immunol (2011) 266(2):208–17. doi: 10.1016/j.cellimm.2010.10.007
101. Eaton SM, Burns EM, Kusser K, Randall TD, Haynes L. Age-related defects in CD4 T cell cognate helper function lead to reductions in humoral responses. J Exp Med (2004) 200(12):1613–22. doi: 10.1084/jem.20041395
102. Lefebvre JS, Lorenzo EC, Masters AR, Hopkins JW, Eaton SM, Smiley ST, et al. Vaccine efficacy and T helper cell differentiation change with aging. Oncotarget (2016) 7(23):33581–94. doi: 10.18632/oncotarget.9254
103. Zahran AM, Saad K, Elsayh KI, Abdou MAA, Abo-Elgheet AM, Eloseily EM, et al. Upregulation of cytotoxic T-cells in pediatric patients with gaucher disease. Sci Rep (2022) 12(1):4977. doi: 10.1038/s41598-022-08843-4
104. Shin MS, Yim K, Moon K, Park HJ, Mohanty S, Kim JW, et al. Dissecting alterations in human CD8+ T cells with aging by high-dimensional single cell mass cytometry. Clin Immunol (2019) 200:24–30. doi: 10.1016/j.clim.2019.01.005
105. Vermes I, Haanen C, Steffens-Nakken H, Reutelingsperger C. A novel assay for apoptosis. flow cytometric detection of phosphatidylserine expression on early apoptotic cells using fluorescein labelled annexin v. J. Immunol Methods (1995) 184(1):39–51. doi: 10.1016/0022-1759(95)00072-i
106. Martínez-Zamudio RI, Dewald HK, Vasilopoulos T, Gittens-Williams L, Fitzgerald-Bocarsly P, Herbig U. Senescence-associated β-galactosidase reveals the abundance of senescent CD8+ T cells in aging humans. Aging Cell (2021) 20(5):e13344. doi: 10.1111/acel.13344
107. Hussain T, Quinn KM. Similar but different: virtual memory CD8 T cells as a memory-like cell population. Immunol Cell Biol (2019) 97(7):675–84. doi: 10.1111/imcb.12277
108. White JT, Cross EW, Kedl RM. Antigen-inexperienced memory CD8(+) T cells: where they come from and why we need them. Nat Rev Immunol (2017) 17(6):391–400. doi: 10.1038/nri.2017.34
109. Quinn KM, Fox A, Harland KL, Russ BE, Li J, Nguyen THO, et al. Age-related decline in primary CD8(+) T cell responses is associated with the development of senescence in virtual memory CD8(+) T cells. Cell Rep (2018) 23(12):3512–24. doi: 10.1016/j.celrep.2018.05.057
110. Pribikova M, Moudra A, Stepanek O. Opinion: Virtual memory CD8 T cells and lymphopenia-induced memory CD8 T cells represent a single subset: Homeostatic memory T cells. Immunol Lett (2018) 203:57–61. doi: 10.1016/j.imlet.2018.09.003
111. Daniels MA, Teixeiro E. Forget 'ME' not virtual memory T cells. Nat Immunol (2020) 21(5):499–500. doi: 10.1038/s41590-020-0668-7
112. Wang H, Wang Z, Cao W, Wu Q, Yuan Y, Zhang X. Regulatory T cells in COVID-19. Aging Dis (2021) 12(7):1545–53. doi: 10.14336/AD.2021.0709
113. Sakaguchi S, Miyara M, Costantino CM, Hafler DA. FOXP3+ regulatory T cells in the human immune system. Nat Rev Immunol (2010) 10(7):490–500. doi: 10.1038/nri2785
114. McHugh J. Induced t(reg) cells stay on course. Nat Rev Rheumatol (2021) 17(1):2. doi: 10.1038/s41584-020-00558-y
115. Gregg R, Smith CM, Clark FJ, Dunnion D, Khan N, Chakraverty R, et al. The number of human peripheral blood CD4+ CD25high regulatory T cells increases with age. Clin Exp Immunol (2005) 140(3):540–6. doi: 10.1111/j.1365-2249.2005.02798.x
116. Lages CS, Suffia I, Velilla PA, Huang B, Warshaw G, Hildeman DA, et al. Functional regulatory T cells accumulate in aged hosts and promote chronic infectious disease reactivation. J Immunol (2008) 181(3):1835–48. doi: 10.4049/jimmunol.181.3.1835
117. Vukmanovic-Stejic M, Zhang Y, Cook JE, Fletcher JM, McQuaid A, Masters JE, et al. Human CD4+ CD25hi Foxp3+ regulatory T cells are derived by rapid turnover of memory populations in vivo. J Clin Invest (2006) 116(9):2423–33. doi: 10.1172/JCI28941
118. Churov AV, Mamashov KY, Novitskaia AV. Homeostasis and the functional roles of CD4(+) treg cells in aging. Immunol Lett (2020) 226:83–9. doi: 10.1016/j.imlet.2020.07.004
119. Jagger A, Shimojima Y, Goronzy JJ, Weyand CM. Regulatory T cells and the immune aging process: a mini-review. Gerontology (2014) 60(2):130–7. doi: 10.1159/000355303
120. Garg SK, Delaney C, Toubai T, Ghosh A, Reddy P, Banerjee R, et al. Aging is associated with increased regulatory T-cell function. Aging Cell (2014) 13(3):441–8. doi: 10.1111/acel.12191
121. Clarke AJ, Simon AK. Autophagy in the renewal, differentiation and homeostasis of immune cells. Nat Rev Immunol (2019) 19(3):170–83. doi: 10.1038/s41577-018-0095-2
122. Schmitt V, Rink L, Uciechowski P. The Th17/Treg balance is disturbed during aging. Exp Gerontol (2013) 48(12):1379–86. doi: 10.1016/j.exger.2013.09.003
123. Gao S, Hao B, Yang XF, Chen WQ. Decreased CD200R expression on monocyte-derived macrophages correlates with Th17/Treg imbalance and disease activity in rheumatoid arthritis patients. Inflammation Res (2014) 63(6):441–50. doi: 10.1007/s00011-014-0716-6
124. Simone R, Zicca A, Saverino D. The frequency of regulatory CD3+CD8+CD28- CD25+ T lymphocytes in human peripheral blood increases with age. J Leukoc Biol (2008) 84(6):1454–61. doi: 10.1189/jlb.0907627
125. de Mol J, Kuiper J, Tsiantoulas D, Foks AC. The dynamics of b cell aging in health and disease. Front Immunol (2021) 12:733566. doi: 10.3389/fimmu.2021.733566
126. Frasca D, Landin AM, Lechner SC, Ryan JG, Schwartz R, Riley RL, et al. Aging down-regulates the transcription factor E2A, activation-induced cytidine deaminase, and ig class switch in human b cells. J Immunol (2008) 180(8):5283–90. doi: 10.4049/jimmunol.180.8.5283
127. Nickerson KM, Christensen SR, Cullen JL, Meng W, Luning Prak ET, Shlomchik MJ. TLR9 promotes tolerance by restricting survival of anergic anti-DNA b cells, yet is also required for their activation. J Immunol (2013) 190(4):1447–56. doi: 10.4049/jimmunol.1202115
128. Zheng Y, Liu X, Le W, Xie L, Li H, Wen W, et al. A human circulating immune cell landscape in aging and COVID-19. Protein Cell (2020) 11(10):740–70. doi: 10.1007/s13238-020-00762-2
129. Kline KA, Bowdish DM. Infection in an aging population. Curr Opin Microbiol (2016) 29:63–7. doi: 10.1016/j.mib.2015.11.003
130. Rubtsov AV, Rubtsova K, Fischer A, Meehan RT, Gillis JZ, Kappler JW, et al. Toll-like receptor 7 (TLR7)-driven accumulation of a novel CD11c+ b-cell population is important for the development of autoimmunity. Blood (2011) 118(5):1305–15. doi: 10.1182/blood-2011-01-331462
131. Hao Y, O'Neill P, Naradikian MS, Scholz JL, Cancro MP. A b-cell subset uniquely responsive to innate stimuli accumulates in aged mice. Blood (2011) 118(5):1294–304. doi: 10.1182/blood-2011-01-330530
132. Du SW, Arkatkar T, Al Qureshah F, Jacobs HM, Thouvenel CD, Chiang K, et al. Functional characterization of CD11c(+) age-associated b cells as memory b cells. J Immunol (2019) 203(11):2817–26. doi: 10.4049/jimmunol.1900404
133. Russell Knode LM, Naradikian MS, Myles A, Scholz JL, Hao Y, Liu D, et al. Age-associated b cells express a diverse repertoire of V(H) and vκ genes with somatic hypermutation. J Immunol (2017) 198(5):1921–7. doi: 10.4049/jimmunol.1601106
134. Naumova E, Pawelec G, Mihaylova A. Natural killer cells, ageing and cancer. Cancer Immunol Immunother (2016) 65(4):367–70. doi: 10.1007/s00262-016-1817-6
135. Solana R, Campos C, Pera A, Tarazona R. Shaping of NK cell subsets by aging. Curr Opin Immunol (2014) 29:56–61. doi: 10.1016/j.coi.2014.04.002
136. Manser AR, Uhrberg M. Age-related changes in natural killer cell repertoires: impact on NK cell function and immune surveillance. Cancer Immunol Immunother (2016) 65(4):417–26. doi: 10.1007/s00262-015-1750-0
137. Solana R, Tarazona R, Gayoso I, Lesur O, Dupuis G, Fulop T. Innate immunosenescence: effect of aging on cells and receptors of the innate immune system in humans. Semin Immunol (2012) 24(5):331–41. doi: 10.1016/j.smim.2012.04.008
138. Lopez-Vergès S, Milush JM, Pandey S, York VA, Arakawa-Hoyt J, Pircher H, et al. CD57 defines a functionally distinct population of mature NK cells in the human CD56dimCD16+ NK-cell subset. Blood (2010) 116(19):3865–74. doi: 10.1182/blood-2010-04-282301
139. Björkström NK, Riese P, Heuts F, Andersson S, Fauriat C, Ivarsson MA, et al. Expression patterns of NKG2A, KIR, and CD57 define a process of CD56dim NK-cell differentiation uncoupled from NK-cell education. Blood (2010) 116(19):3853–64. doi: 10.1182/blood-2010-04-281675
140. Rink L, Cakman I, Kirchner H. Altered cytokine production in the elderly. Mech Ageing Dev (1998) 102(2-3):199–209. doi: 10.1016/S0047-6374(97)00153-X
141. Mocchegiani E, Malavolta M. NK and NKT cell functions in immunosenescence. Aging Cell (2004) 3(4):177–84. doi: 10.1111/j.1474-9728.2004.00107.x
142. Al-Attar A, Presnell SR, Peterson CA, Thomas DT, Lutz CT. The effect of sex on immune cells in healthy aging: Elderly women have more robust natural killer lymphocytes than do elderly men. Mech Ageing Dev (2016) 156:25–33. doi: 10.1016/j.mad.2016.04.001
143. Gupta S. Role of dendritic cells in innate and adaptive immune response in human aging. Exp Gerontol (2014) 54:47–52. doi: 10.1016/j.exger.2013.12.009
144. Agrawal A, Gupta S. Impact of aging on dendritic cell functions in humans. Ageing Res Rev (2011) 10(3):336–45. doi: 10.1016/j.arr.2010.06.004
145. Fuentes E, Fuentes M, Alarcón M, Palomo I. Immune system dysfunction in the elderly. Acad Bras Cienc (2017) 89(1):285–99. doi: 10.1590/0001-3765201720160487
146. Verschoor CP, Loukov D, Naidoo A, Puchta A, Johnstone J, Millar J, et al. Circulating TNF and mitochondrial DNA are major determinants of neutrophil phenotype in the advanced-age, frail elderly. Mol Immunol (2015) 65(1):148–56. doi: 10.1016/j.molimm.2015.01.015
147. Butcher S, Chahel H, Lord JM. Review article: ageing and the neutrophil: no appetite for killing? Immunology (2000) 100(4):411–6. doi: 10.1046/j.1365-2567.2000.00079.x
148. Niwa Y, Kasama T, Miyachi Y, Kanoh T. Neutrophil chemotaxis, phagocytosis and parameters of reactive oxygen species in human aging: cross-sectional and longitudinal studies. Life Sci (1989) 44(22):1655–64. doi: 10.1016/0024-3205(89)90482-7
149. Dubey M, Nagarkoti S, Awasthi D, Singh AK, Chandra T, Kumaravelu J, et al. Nitric oxide-mediated apoptosis of neutrophils through caspase-8 and caspase-3-dependent mechanism. Cell Death Dis (2016) 7(9):e2348. doi: 10.1038/cddis.2016.248
150. Cunha LL, Perazzio SF, Azzi J, Cravedi P, Riella LV. Remodeling of the immune response with aging: Immunosenescence and its potential impact on COVID-19 immune response. Front Immunol (2020) 11:1748. doi: 10.3389/fimmu.2020.01748
151. Qian F, Guo X, Wang X, Yuan X, Chen S, Malawista SE, et al. Reduced bioenergetics and toll-like receptor 1 function in human polymorphonuclear leukocytes in aging. Aging (Albany NY) (2014) 6(2):131–9. doi: 10.18632/aging.100642
152. Weiskopf K, Weissman IL. Macrophages are critical effectors of antibody therapies for cancer. MAbs (2015) 7(2):303–10. doi: 10.1080/19420862.2015.1011450
153. Blacher E, Tsai C, Litichevskiy L, Shipony Z, Iweka CA, Schneider KM, et al. Aging disrupts circadian gene regulation and function in macrophages. Nat Immunol (2021) 23(2):229–36. doi: 10.1038/s41590-021-01083-0
154. Minhas PS, Latif-Hernandez A, McReynolds MR, Durairaj AS, Wang Q, Rubin A, et al. Restoring metabolism of myeloid cells reverses cognitive decline in ageing. Nature (2021) 590(7844):122–8. doi: 10.1038/s41586-020-03160-0
155. Durur DY, Tastan B, Ugur Tufekci K, Olcum M, Uzuner H, Karakülah G, et al. Alteration of miRNAs in small neuron-derived extracellular vesicles of alzheimer's disease patients and the effect of extracellular vesicles on microglial immune responses. J Mol Neurosci (2022) 72(6):1182–94. doi: 10.1007/s12031-022-02012-y
156. Lu Y, Li K, Hu Y, Wang X. Expression of immune related genes and possible regulatory mechanisms in alzheimer's disease. Front Immunol (2021) 12:768966. doi: 10.3389/fimmu.2021.768966
157. Baruch K, Rosenzweig N, Kertser A, Deczkowska A, Sharif AM, Spinrad A, et al. Breaking immune tolerance by targeting Foxp3(+) regulatory T cells mitigates alzheimer's disease pathology. Nat Commun (2015) 6:7967. doi: 10.1038/ncomms8967
158. Monsonego A, Zota V, Karni A, Krieger JI, Bar-Or A, Bitan G, et al. Increased T cell reactivity to amyloid beta protein in older humans and patients with Alzheimer disease. J Clin Invest (2003) 112(3):415–22. doi: 10.1172/JCI200318104
159. Tan J, Town T, Abdullah L, Wu Y, Placzek A, Small B, et al. CD45 isoform alteration in CD4+ T cells as a potential diagnostic marker of alzheimer's disease. J Neuroimmunol (2002) 132(1-2):164–72. doi: 10.1016/S0165-5728(02)00309-0
160. Panossian LA, Porter VR, Valenzuela HF, Zhu X, Reback E, Masterman D, et al. Telomere shortening in T cells correlates with alzheimer's disease status. Neurobiol Aging (2003) 24(1):77–84. doi: 10.1016/S0197-4580(02)00043-X
161. Gate D, Saligrama N, Leventhal O, Yang AC, Unger MS, Middeldorp J, et al. Clonally expanded CD8 T cells patrol the cerebrospinal fluid in alzheimer's disease. Nature (2020) 577(7790):399–404. doi: 10.1038/s41586-019-1895-7
162. Ritzel RM, Crapser J, Patel AR, Verma R, Grenier JM, Chauhan A, et al. Age-associated resident memory CD8 T cells in the central nervous system are primed to potentiate inflammation after ischemic brain injury. J Immunol (2016) 196(8):3318–30. doi: 10.4049/jimmunol.1502021
163. Mosher KI, Wyss-Coray T. Microglial dysfunction in brain aging and alzheimer's disease. Biochem Pharmacol (2014) 88(4):594–604. doi: 10.1016/j.bcp.2014.01.008
164. Palmer S, Albergante L, Blackburn CC, Newman TJ. Thymic involution and rising disease incidence with age. Proc Natl Acad Sci U S A (2018) 115(8):1883–8. doi: 10.1073/pnas.1714478115
165. Swann JB, Smyth MJ. Immune surveillance of tumors. J Clin Invest (2007) 117(5):1137–46. doi: 10.1172/JCI31405
166. Singer K, Cheng WC, Kreutz M, Ho PC, Siska PJ. Immunometabolism in cancer at a glance. Dis Model Mech (2018) 11(8):dmm034272. doi: 10.1242/dmm.034272
167. Ye J, Ma C, Hsueh EC, Eickhoff CS, Zhang Y, Varvares MA, et al. Tumor-derived γδ regulatory T cells suppress innate and adaptive immunity through the induction of immunosenescence. J Immunol (2013) 190(5):2403–14. doi: 10.4049/jimmunol.1202369
168. Onyema OO, Decoster L, Njemini R, Forti LN, Bautmans I, De Waele M, et al. Chemotherapy-induced changes and immunosenescence of CD8+ T-cells in patients with breast cancer. Anticancer Res (2015) 35(3):1481–9.
169. Su Z, Zhang P, Yu Y, Lu H, Liu Y, Ni P, et al. HMGB1 facilitated macrophage reprogramming towards a proinflammatory M1-like phenotype in experimental autoimmune myocarditis development. Sci Rep (2016) 6:21884. doi: 10.1038/srep21884
170. Sun L, Kees T, Almeida AS, Liu B, He XY, Ng D, et al. Activating a collaborative innate-adaptive immune response to control metastasis. Cancer Cell (2021) 39(10):1361–74.e9. doi: 10.1016/j.ccell.2021.08.005
171. Yu HT, Park S, Shin EC, Lee WW. T Cell senescence and cardiovascular diseases. Clin Exp Med (2016) 16(3):257–63. doi: 10.1007/s10238-015-0376-z
172. Leon ML, Zuckerman SH. Gamma interferon: a central mediator in atherosclerosis. Inflammation Res (2005) 54(10):395–411. doi: 10.1007/s00011-005-1377-2
173. Johnson JL. Matrix metalloproteinases: influence on smooth muscle cells and atherosclerotic plaque stability. Expert Rev Cardiovasc Ther (2007) 5(2):265–82. doi: 10.1586/14779072.5.2.265
174. Youn JC, Yu HT, Lim BJ, Koh MJ, Lee J, Chang DY, et al. Immunosenescent CD8+ T cells and c-X-C chemokine receptor type 3 chemokines are increased in human hypertension. Hypertension (2013) 62(1):126–33. doi: 10.1161/HYPERTENSIONAHA.113.00689
175. Rajagopalan S, Long EO. Cellular senescence induced by CD158d reprograms natural killer cells to promote vascular remodeling. Proc Natl Acad Sci U S A (2012) 109(50):20596–601. doi: 10.1073/pnas.1208248109
176. Fülöp T, Dupuis G, Witkowski JM, Larbi A. The role of immunosenescence in the development of age-related diseases. Rev Invest Clin (2016) 68(2):84–91.
177. Birks EJ, Felkin LE, Banner NR, Khaghani A, Barton PJ, Yacoub MH. Increased toll-like receptor 4 in the myocardium of patients requiring left ventricular assist devices. J Heart Lung Transpl (2004) 23(2):228–35. doi: 10.1016/S1053-2498(03)00106-2
178. Bentz GL, Yurochko AD. Human CMV infection of endothelial cells induces an angiogenic response through viral binding to EGF receptor and beta1 and beta3 integrins. Proc Natl Acad Sci U S A (2008) 105(14):5531–6. doi: 10.1073/pnas.0800037105
179. Niu Q, Cai B, Huang ZC, Shi YY, Wang LL. Disturbed Th17/Treg balance in patients with rheumatoid arthritis. Rheumatol Int (2012) 32(9):2731–6. doi: 10.1007/s00296-011-1984-x
180. Franceschi C, Bonafè M, Valensin S, Olivieri F, De Luca M, Ottaviani E, et al. Inflamm-aging. an evolutionary perspective on immunosenescence. Ann N Y Acad Sci (2000) 908:244–54. doi: 10.1111/j.1749-6632.2000.tb06651.x
181. Petersen LE, Grassi-Oliveira R, Siara T, dos Santos SG, Ilha M, de Nardi T, et al. Premature immunosenescence is associated with memory dysfunction in rheumatoid arthritis. Neuroimmunomodulation (2015) 22(3):130–7. doi: 10.1159/000358437
182. Chalan P, van den Berg A, Kroesen BJ, Brouwer L, Boots A. Rheumatoid arthritis, immunosenescence and the hallmarks of aging. Curr Aging Sci (2015) 8(2):131–46. doi: 10.2174/1874609808666150727110744
183. Pietrobon AJ, Teixeira FME, Sato MN. I Mmunosenescence and inflammaging: Risk factors of severe COVID-19 in older people. Front Immunol (2020) 11:579220. doi: 10.3389/fimmu.2020.579220
184. Chen Y, Klein SL, Garibaldi BT, Li H, Wu C, Osevala NM, et al. Aging in COVID-19: Vulnerability, immunity and intervention. Ageing Res Rev (2021) 65:101205. doi: 10.1016/j.arr.2020.101205
185. Zhou Y, Fu B, Zheng X, Wang D, Zhao C, Qi Y, et al. Pathogenic T-cells and inflammatory monocytes incite inflammatory storms in severe COVID-19 patients. Natl Sci Rev (2020) 7(6):998–1002. doi: 10.1093/nsr/nwaa041
186. Blagosklonny MV. From causes of aging to death from COVID-19. Aging (Albany NY) (2020) 12(11):10004–21. doi: 10.18632/aging.103493
187. Chow RD, Majety M, Chen S. The aging transcriptome and cellular landscape of the human lung in relation to SARS-CoV-2. Nat Commun (2021) 12(1):4. doi: 10.1038/s41467-020-20323-9
188. Marcon G, Tettamanti M, Capacci G, Fontanel G, Spanò M, Nobili A, et al. COVID-19 mortality in Lombardy: the vulnerability of the oldest old and the resilience of male centenarians. Aging (Albany NY) (2020) 12(15):15186–95. doi: 10.18632/aging.103872
189. Perrotta F, Corbi G, Mazzeo G, Boccia M, Aronne L, D'Agnano V, et al. COVID-19 and the elderly: insights into pathogenesis and clinical decision-making. Aging Clin Exp Res (2020) 32(8):1599–608. doi: 10.1007/s40520-020-01631-y
190. Abdulamir AS, Hafidh RR. The possible immunological pathways for the variable immunopathogenesis of COVID-19 infections among healthy adults, elderly and children. (2020) 17(4):em202. doi: 10.29333/ejgm/7850
191. Omarjee L, Perrot F, Meilhac O, Mahe G, Bousquet G, Janin A. Immunometabolism at the cornerstone of inflammaging, immunosenescence, and autoimmunity in COVID-19. Aging (Albany NY) (2020) 12(24):26263–78. doi: 10.18632/aging.202422
192. Anderson EJ, Rouphael NG, Widge AT, Jackson LA, Roberts PC, Makhene M, et al. Safety and immunogenicity of SARS-CoV-2 mRNA-1273 vaccine in older adults. N Engl J Med (2020) 383(25):2427–38. doi: 10.1056/NEJMoa2028436
193. Walsh EE, Frenck RW Jr., Falsey AR, Kitchin N, Absalon J, Gurtman A, et al. Safety and immunogenicity of two RNA-based covid-19 vaccine candidates. N Engl J Med (2020) 383(25):2439–50. doi: 10.1056/NEJMoa2027906
194. Ramasamy MN, Minassian AM, Ewer KJ, Flaxman AL, Folegatti PM, Owens DR, et al. Safety and immunogenicity of ChAdOx1 nCoV-19 vaccine administered in a prime-boost regimen in young and old adults (COV002): a single-blind, randomised, controlled, phase 2/3 trial. Lancet (2021) 396(10267):1979–93. doi: 10.1016/S0140-6736(20)32466-1
195. Lederer K, Castaño D, Gómez Atria D, Oguin TH 3rd, Wang S, Manzoni TB, et al. SARS-CoV-2 mRNA vaccines foster potent antigen-specific germinal center responses associated with neutralizing antibody generation. Immunity (2020) 53(6):1281–95.e5. doi: 10.1016/j.immuni.2020.11.009
196. Collier DA, Ferreira I, Kotagiri P, Datir RP, Lim EY, Touizer E, et al. Age-related immune response heterogeneity to SARS-CoV-2 vaccine BNT162b2. Nature (2021) 596(7872):417–22. doi: 10.1038/s41586-021-03739-1
197. Wang K, Jia Z, Bao L, Wang L, Cao L, Chi H, et al. Memory b cell repertoire from triple vaccinees against diverse SARS-CoV-2 variants. Nature (2022) 603(7903):919–25. doi: 10.1038/s41586-022-04466-x
198. Paganelli R, Quinti I, Fagiolo U, Cossarizza A, Ortolani C, Guerra E, et al. Changes in circulating b cells and immunoglobulin classes and subclasses in a healthy aged population. Clin Exp Immunol (1992) 90(2):351–4. doi: 10.1111/j.1365-2249.1992.tb07954.x
199. Weng NP. Aging of the immune system: how much can the adaptive immune system adapt? Immunity (2006) 24(5):495–9. doi: 10.1016/j.immuni.2006.05.001
200. Tedone E, Huang E, O'Hara R, Batten K, Ludlow AT, Lai TP, et al. Telomere length and telomerase activity in T cells are biomarkers of high-performing centenarians. Aging Cell (2019) 18(1):e12859. doi: 10.1111/acel.12859
201. Hashimoto K, Kouno T, Ikawa T, Hayatsu N, Miyajima Y, Yabukami H, et al. Single-cell transcriptomics reveals expansion of cytotoxic CD4 T cells in supercentenarians. Proc Natl Acad Sci U S A (2019) 116(48):24242–51. doi: 10.1073/pnas.1907883116
202. Arosa FA, Esgalhado AJ, Padrão CA, Cardoso EM. Divide, conquer, and sense: CD8(+)CD28(-) T cells in perspective. Front Immunol (2016) 7:665. doi: 10.3389/fimmu.2016.00665
203. Cossarizza A, Ortolani C, Paganelli R, Barbieri D, Monti D, Sansoni P, et al. CD45 isoforms expression on CD4+ and CD8+ T cells throughout life, from newborns to centenarians: implications for T cell memory. Mech Ageing Dev (1996) 86(3):173–95. doi: 10.1016/0047-6374(95)01691-0
204. Colonna-Romano G, Buffa S, Bulati M, Candore G, Lio D, Pellicanò M, et al. B cells compartment in centenarian offspring and old people. Curr Pharm Des (2010) 16(6):604–8. doi: 10.2174/138161210790883750
205. Sansoni P, Cossarizza A, Brianti V, Fagnoni F, Snelli G, Monti D, et al. Lymphocyte subsets and natural killer cell activity in healthy old people and centenarians. Blood (1993) 82(9):2767–73. doi: 10.1182/blood.V82.9.2767.2767
206. Martínez De Toda I, Vida C, García-Salmones M, Alonso-Fernández P, de la Fuente M. Immune function, oxidative, and inflammatory markers in centenarians as potential predictors of survival and indicators of recovery after hospital admission. J Gerontol A Biol Sci Med Sci (2020) 75(10):1827–33. doi: 10.1093/gerona/glz250
207. Chen C, Liu Y, Liu Y, Zheng P. mTOR regulation and therapeutic rejuvenation of aging hematopoietic stem cells. Sci Signal (2009) 2(98):ra75. doi: 10.1126/scisignal.2000559
208. Kim MJ, Miller CM, Shadrach JL, Wagers AJ, Serwold T. Young, proliferative thymic epithelial cells engraft and function in aging thymuses. J Immunol (2015) 194(10):4784–95. doi: 10.4049/jimmunol.1403158
209. Janot-Sardet C, Assouline B, Cheynier R, Morre M, Beq S. A validated assay to measure soluble IL-7 receptor shows minimal impact of IL-7 treatment. J Immunol Methods (2010) 353(1-2):115–23. doi: 10.1016/j.jim.2009.12.003
210. Okoye AA, Rohankhedkar M, Konfe AL, Abana CO, Reyes MD, Clock JA, et al. Effect of IL-7 therapy on naive and memory T cell homeostasis in aged rhesus macaques. J Immunol (2015) 195(9):4292–305. doi: 10.4049/jimmunol.1500609
211. Hathcock KS, Jeffrey Chiang Y, Hodes RJ. In vivo regulation of telomerase activity and telomere length. Immunol Rev (2005) 205:104–13. doi: 10.1111/j.0105-2896.2005.00267.x
212. Muñoz-Lorente MA, Cano-Martin AC, Blasco MA. Mice with hyper-long telomeres show less metabolic aging and longer lifespans. Nat Commun (2019) 10(1):4723. doi: 10.1038/s41467-019-12664-x
213. Di Micco R, Krizhanovsky V, Baker D, d'Adda di Fagagna F. Cellular senescence in ageing: from mechanisms to therapeutic opportunities. Nat Rev Mol Cell Biol (2021) 22(2):75–95. doi: 10.1038/s41580-020-00314-w
214. Zhu Y, Tchkonia T, Fuhrmann-Stroissnigg H, Dai HM, Ling YY, Stout MB, et al. Identification of a novel senolytic agent, navitoclax, targeting the bcl-2 family of anti-apoptotic factors. Aging Cell (2016) 15(3):428–35. doi: 10.1111/acel.12445
215. Baar MP, Brandt RMC, Putavet DA, Klein JDD, Derks KWJ, Bourgeois BRM, et al. Targeted apoptosis of senescent cells restores tissue homeostasis in response to chemotoxicity and aging. Cell (2017) 169(1):132–47.e16. doi: 10.1016/j.cell.2017.02.031
216. Xu Q, Fu Q, Li Z, Liu H, Wang Y, Lin X, et al. The flavonoid procyanidin C1 has senotherapeutic activity and increases lifespan in mice. Nat Metab (2021) 3(12):1706–26. doi: 10.1038/s42255-021-00491-8
217. Song P, An J, Zou MH. Immune clearance of senescent cells to combat ageing and chronic diseases. Cells (2020) 9(3):671. doi: 10.3390/cells9030671
218. June CH, O'Connor RS, Kawalekar OU, Ghassemi S, Milone MC. CAR T cell immunotherapy for human cancer. Science (2018) 359(6382):1361–5. doi: 10.1126/science.aar6711
219. Baumeister SH, Murad J, Werner L, Daley H, Trebeden-Negre H, Gicobi JK, et al. Phase I trial of autologous CAR T cells targeting NKG2D ligands in patients with AML/MDS and multiple myeloma. Cancer Immunol Res (2019) 7(1):100–12. doi: 10.1158/2326-6066.CIR-18-0307
220. Soriani A, Zingoni A, Cerboni C, Iannitto ML, Ricciardi MR, Di Gialleonardo V, et al. ATM-ATR-dependent up-regulation of DNAM-1 and NKG2D ligands on multiple myeloma cells by therapeutic agents results in enhanced NK-cell susceptibility and is associated with a senescent phenotype. Blood (2009) 113(15):3503–11. doi: 10.1182/blood-2008-08-173914
221. Fafián-Labora JA, O'Loghlen A. Classical and nonclassical intercellular communication in senescence and ageing. Trends Cell Biol (2020) 30(8):628–39. doi: 10.1016/j.tcb.2020.05.003
222. Yousefzadeh MJ, Flores RR, Zhu Y, Schmiechen ZC, Brooks RW, Trussoni CE, et al. An aged immune system drives senescence and ageing of solid organs. Nature (2021) 594(7861):100–5. doi: 10.1038/s41586-021-03547-7
223. Li J, Kim SG, Blenis J. Rapamycin: one drug, many effects. Cell Metab (2014) 19(3):373–9. doi: 10.1016/j.cmet.2014.01.001
224. Laberge RM, Sun Y, Orjalo AV, Patil CK, Freund A, Zhou L, et al. MTOR regulates the pro-tumorigenic senescence-associated secretory phenotype by promoting IL1A translation. Nat Cell Biol (2015) 17(8):1049–61. doi: 10.1038/ncb3195
225. Munn DH, Mellor AL. IDO in the tumor microenvironment: Inflammation, counter-regulation, and tolerance. Trends Immunol (2016) 37(3):193–207. doi: 10.1016/j.it.2016.01.002
226. Dammeijer F, van Gulijk M, Mulder EE, Lukkes M, Klaase L, van den Bosch T, et al. The PD-1/PD-L1-Checkpoint restrains T cell immunity in tumor-draining lymph nodes. Cancer Cell (2020) 38(5):685–700.e8. doi: 10.1016/j.ccell.2020.09.001
227. Rocamora-Reverte L, Melzer FL, Würzner R, Weinberger B. The complex role of regulatory T cells in immunity and aging. Front Immunol (2020) 11:616949. doi: 10.3389/fimmu.2020.616949
228. Moon JS, Goeminne LJE, Kim JT, Tian JW, Kim SH, Nga HT, et al. Growth differentiation factor 15 protects against the aging-mediated systemic inflammatory response in humans and mice. Aging Cell (2020) 19(8):e13195. doi: 10.1111/acel.13195
229. Maijó M, Clements SJ, Ivory K, Nicoletti C, Carding SR. Nutrition, diet and immunosenescence. Mech Ageing Dev (2014) 136–137:116–28. doi: 10.1016/j.mad.2013.12.003
230. Meydani SN, Han SN, Wu D. Vitamin e and immune response in the aged: molecular mechanisms and clinical implications. Immunol Rev (2005) 205(1):269–84. doi: 10.1111/j.0105-2896.2005.00274.x
231. Wu D, Meydani SN. Age-associated changes in immune and inflammatory responses: impact of vitamin e intervention. J Leukoc Biol (2008) 84(4):900–14. doi: 10.1189/jlb.0108023
232. Duggal NA, Pollock RD, Lazarus NR, Harridge S, Lord JM. Major features of immunesenescence, including reduced thymic output, are ameliorated by high levels of physical activity in adulthood. Aging Cell (2018) 17(2):e12750. doi: 10.1111/acel.12750
233. Salminen A, Kaarniranta K, Kauppinen A. Immunosenescence: the potential role of myeloid-derived suppressor cells (MDSC) in age-related immune deficiency. Cell Mol Life Sci (2019) 76(10):1901–18. doi: 10.1007/s00018-019-03048-x
234. Simon AK, Hollander GA, McMichael A. Evolution of the immune system in humans from infancy to old age. Proc Biol Sci (2015) 282(1821):20143085. doi: 10.1098/rspb.2014.3085
Keywords: immunosenescence, age-related diseases, successful aging, inflammation, centenarian
Citation: Wang Y, Dong C, Han Y, Gu Z and Sun C (2022) Immunosenescence, aging and successful aging. Front. Immunol. 13:942796. doi: 10.3389/fimmu.2022.942796
Received: 13 May 2022; Accepted: 08 July 2022;
Published: 02 August 2022.
Edited by:
Ke Rui, Affiliated Hospital of Jiangsu University, ChinaReviewed by:
Yolanda María Pacheco, Institute of Biomedicine of Seville, Spanish National Research Council (CSIC), SpainWeikan Wang, University of North Texas Health Science Center, United States
Copyright © 2022 Wang, Dong, Han, Gu and Sun. This is an open-access article distributed under the terms of the Creative Commons Attribution License (CC BY). The use, distribution or reproduction in other forums is permitted, provided the original author(s) and the copyright owner(s) are credited and that the original publication in this journal is cited, in accordance with accepted academic practice. No use, distribution or reproduction is permitted which does not comply with these terms.
*Correspondence: Zhifeng Gu, guzf@ntu.edu.cn; Chi Sun, tdfy_sc@126.com
†These authors have contributed equally to this work and share first authorship