- 1Chongqing Academy of Animal Sciences, Chongqing, China
- 2Key Laboratory of Pig Industry Sciences, Ministry of Agriculture, Chongqing, China
- 3Chongqing Camab Biotech Ltd., Chongqing, China
- 4Farm Animal Genetic Resource Exploration and Innovation Key Laboratory of Sichuan Province, Sichuan Agricultural University, Chengdu, China
Hypoxia is a common hallmark of healthy tissues in physiological states or chronically inflamed tissues in pathological states. Mammalian cells sense and adapt to hypoxia mainly through hypoxia-inducible factor (HIF) signaling. Many studies have shown that hypoxia and HIF signaling play an important regulatory role in development and function of innate immune cells and T cells, but their role in B cell biology is still controversial. B cells experience a complex life cycle (including hematopoietic stem cells, pro-B cells, pre-B cells, immature B cells, mature naïve B cells, activated B cells, plasma cells, and memory B cells), and the partial pressure of oxygen (PO2) in the corresponding developmental niche of stage-specific B cells is highly dynamic, which suggests that hypoxia and HIF signaling may play an indispensable role in B cell biology. Based on the fact that hypoxia niches exist in the B cell life cycle, this review focuses on recent discoveries about how hypoxia and HIF signaling regulate the development, metabolism, and function of B cells, to facilitate a deep understanding of the role of hypoxia in B cell-mediated adaptive immunity and to provide novel strategies for vaccine adjuvant research and the treatment of immunity-related or infectious diseases.
Introduction
The two types of host defense systems are innate and adaptive immunity. They work together to resist the invasion of foreign pathogens and maintain the homeostasis of internal environments, and are crucial for the evolutionary fitness of mammals. Innate immunity can quickly block and eliminate nonspecific pathogens and provides the first line of defense against infectious challenges (1). Adaptive immunity develops more slowly and not only destroys specific pathogens through the production of high-affinity, class-switched antibodies, but also creates immunological memory for similar antigens (2). Effective immunity in healthy individuals occurs at focal sites of immune activity, known as physiological immune niches, including bone marrow (BM), spleen, lymph nodes, and the intestinal mucosa (3, 4). In contrast, pathological immunological niches are sites of tissue pathology associated with inflammation and tissue dysfunction (5, 6). Microenvironmental features of immunological niches play a key regulatory role in the development, metabolism, and function of resident immune cells (7).
The vast majority of immune cells originate in the BM and migrate to peripheral immune tissues through the circulation for further maturation and effector function development. Immunological niches are microenvironments with dynamic partial pressure of oxygen (PO2), which induce different degrees of hypoxia adaptation by activating cellular hypoxia-inducible factor (HIF) signaling (8). Hypoxia and HIF signaling play an important role in the development and function of innate immune cells and T cells, but their effects on B cell biology remains controversial (9–11). B cells have a complex life cycle that is driven by both intrinsic (genetic programs) and extrinsic (antigen and cytokines) cues (12). The variable PO2 at corresponding immunological niches of stage-specific B cells is likely to be closely interlinked with the functional requirements of stage-specific cell adaptation (13). Given that pathological immunological niches have been expertly reviewed elsewhere (7), this review summarizes recent advances concerning how hypoxia and HIF signaling regulate the development, metabolism, and function of B cells in physiological immunological niches.
B cell life cycle
B cells were first discovered by Cooper in 1965, and three lineages have been identified to date, including B1, B2, and regulatory B cells (Bregs) (14, 15). B1 cells (including B1a and B1b), which originate from the fetal liver and are located in the peritoneum, spleen, and mucosa (16). B2 cells are produced in the BM throughout life and represent the bulk of the B cell pool, and contain follicular B (FOB) and marginal zone B (MZB) cells (17). Bregs represent a class of B cells with immunosuppressive properties and play a key role in the establishment of B cell tolerance primarily through the production of the anti-inflammatory cytokine IL-10 (18). This review focuses principally on the B2 lineage unless otherwise stated.
B2 cells originate from multipotent hematopoietic stem cells (HSCs) in the BM, where common lymphoid progenitors (CLP) are committed to pro-B cells through the expression of the transcription factor Pax5 (19). Pro-B cells undergo V(D)J recombination at the immunoglobulin (Ig) heavy-chain locus to differentiate into pre-B cells, which rearrange Ig light-chain genes to further develop into IgM+ immature B cells. Immature B cells undergo B cell receptor (BCR) editing and produce a diverse BCR repertoire with limited self-antigen recognition (20, 21). Then, they enter the circulation as transitional B cells, and migrate into peripheral lymphoid tissues to complete maturation, finally differentiating into FOB or MZB (22–24). FOB recirculate and reside in the periphery lymphoid follicles, while MZB locate to the margins of follicles, and respond to T cell-dependent (Td) and T cell-independent (Ti) antigens, respectively (6). Mature naïve B cells undergo class-switch recombination (CSR) and develop into short-lived plasmablasts that secrete low-affinity antibodies. Activated B cells extensively proliferate with the help of T follicular helper (Tfh) cells in the germinal center (GC), where B cells undergo BCR diversification by introducing random mutations into the V(D)J fragments of the heavy- and light-chain genes (known as somatic hypermutation, SHM) (25). Then, B cells differentiate into high-affinity antibody-secreting plasma cells and long-lived memory B cells to provide specific and long-term protection (Figure 1). B cell life cycle are precisely regulated through several defining transcription factors that have been systematically reviewed elsewhere (17, 26).
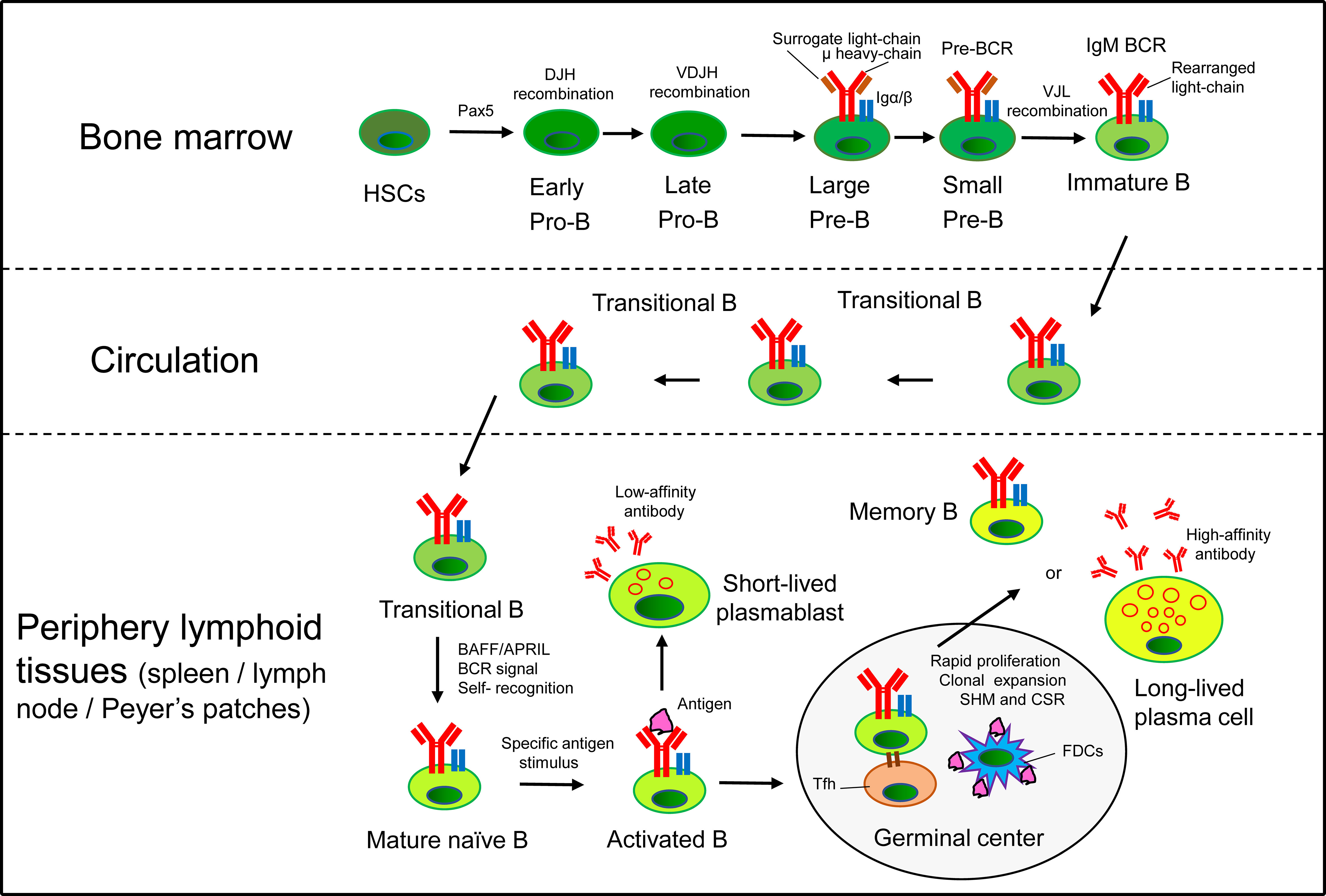
Figure 1 B cell life cycle. In the BM, HSCs commit to the B cell lineage producing pro-B cells. Pro-B cells undergo VDJ recombination at the Ig heavy-chain locus to differentiate into pre-B cells, which rearrange Ig light-chain genes to further develop into IgM+ immature B cells. Immature B cells egress from the BM and migrate into peripheral lymphoid tissues via the circulation (transitional B cells) to complete maturation. Mature naïve B cells respond to Td antigens and develop into short-lived plasmablasts that secrete low-affinity antibodies. Activated B cells extensively proliferate with the help of Tfh cells in the GC of periphery lymphoid tissues (such as spleen, lymph node and Peyer’s patches), where B cells undergo SHM and CSR. Finally, B cells differentiate into high-affinity antibody-secreting plasma cells and long-lived memory B cells to provide specific and long-term protection for the body.
HIF signaling
Hypoxic responses, whether physiological [embryogenesis (27)] or pathological [cancer (28)], external [organism level (29)] or internal [cellular level (30)], have been a research hotspot in recent decades. All metazoan cells have evolved an oxygen-sensing system that enables rapid adaptation to fluctuations in the PO2 of their resident niches. The master regulator of this system is the family of HIFs, a kind of heterodimeric transcription factors that comprise an O2-sensitive α subunit (HIF-1α/-2α/-3α) and a constitutively expressed β subunit [Aryl hydrocarbon receptor nuclear translocator 1/2 (ARNT1/2)] (30). HIF-1α is ubiquitously expressed and HIF-2α shows a more tissue-specific expression pattern (such as heart, lung, kidney, and placenta) and is also expressed in some immune cell subtypes (macrophages, neutrophils, and lymphocytes), whereas HIF-1α and HIF-2α have distinct but overlapping target gene sets (31, 32). Additionally, HIF-3α has been reported to be expressed only by epithelial cells in the lung and kidney and have both positive and negative effects on hypoxia-modulated gene expression depending on the specific context (33, 34).
HIF-α is composed of a Per-ARNT-Sim (PAS) domain and an N-terminal basic-helix-loop-helix (bHLH) domain that mediates dimerization and transcriptional activation, respectively. Under normoxia, two conserved proline residues (Pro402 and Pro564) in the O2-dependent degradation domain (ODD) of HIF-α are hydroxylated by one of three prolyl hydroxylase domain enzymes (PHD), which facilitates HIF-α binding to the von Hippel-Lindau (VHL) E3 ubiquitin ligase complex and leads to polyubiquitination and proteosomal degradation (35). Moreover, HIF-α is also hydroxylated on a specific asparagine residue (Asn803) in the C-terminal transactivation domain (CTAD) by factor-inhibiting HIF-1 (FIH-1), which hinders the transcriptional activation of HIF through inhibition of the binding of HIF to co-activator CREB-binding protein (CBP)/p300 (36). Hypoxia induces HIF-α protein stabilization by inactivating PHD and FIH. Activated HIF-α translocates from the cytoplasm to the nucleus, where it dimerizes with HIF-1β and recruits CBP/p300, and then binds to hypoxia response element (5’-RCGTG-3’) in the promoter or enhancer of HIF-regulated genes, of which more than 300 protein coding genes (PCGs) have been reported (37, 38). Moreover, hypoxia also regulates gene expression at the posttranscriptional level by modulating a specific subset of miRNAs [hypoxamiRs (39)] and lncRNAs [hypoxia responsive lncRNAs, HRLs (40)]. The consequence of the cellular hypoxia response activates multiple hypoxia-adaptive pathways involved in angiogenesis, anaerobic metabolism, and erythropoiesis, which triggers a cellular metabolism shift toward glycolysis and reestablishes O2 supply (8, 41) (Figure 2).
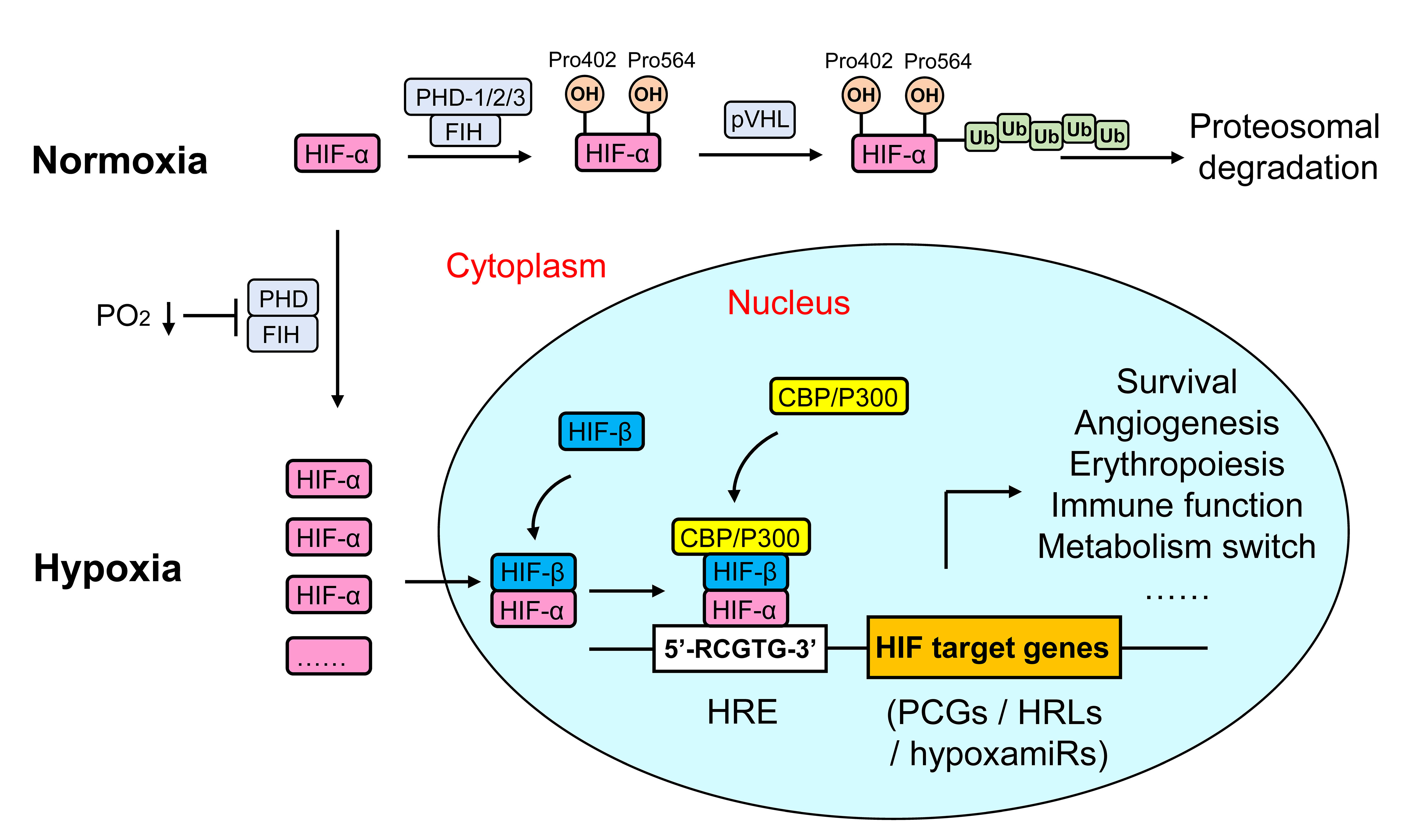
Figure 2 Regulation of HIF signaling in metazoan cells. HIFs are a kind of heterodimeric transcription factors that comprise an α subunit (O2-sensitive) and a β subunit (constitutive). Under normoxia, proline residues in the ODD of HIF-α are hydroxylated by PHDs or FIH, which facilitates HIF-α binding to the VHL E3 ubiquitin ligase complex, and leads to polyubiquitination and proteosomal degradation. Hypoxia induces HIF-α protein stabilization by inactivating PHD and FIH. Activated HIF-α translocates from the cytoplasm to the nucleus, where it dimerizes with HIF-1β and recruits CBP/p300, and then binds to HRE (5’-RCGTG-3’) in the promoter or enhancer of HIF-regulated genes [including PCGs, hypoxia-responsive lncRNAs (HRLs) and hypoxamiRs], finally regulate a variety of hypoxia-adaptive pathways such as angiogenesis, anaerobic metabolism, and erythropoiesis.
Hypoxia niches in the B cell life cycle
The vasculature supplies O2 and nutrients for all organs, tissues and cells of the body depending on their metabolic needs, meanwhile disposing of waste such as CO2 and metabolic products to establish a balance between supply and consumption (42, 43). Whether a tissue is hypoxic is determined by a combination of factors, including vessel size and density, distance from cells to the nearest vessel, cell density, and metabolic demands (6). The differences in these factors make the various tissues of the body form different hypoxic landscapes.
Under physiological conditions, PO2 varies throughout the body in a descending order from arterial blood (~13%), main organs (~6%; e.g., brain, liver, lung) to lymphoid tissues (~3%; e.g., spleen). The development process from hematopoietic progenitors to immature B cells occurs in the BM where the presence of hypoxic niches (average PO2 2.25%) and a PO2 gradient has been elucidated from direct [two-photon phosphorescence lifetime microscopy (44)] and indirect evidence [proteomic analysis (45), HIF-1α and its regulated genes (46) and surrogate hypoxic marker staining (47)]. Different degrees of hypoxic niches [e.g., severe hypoxia in perisinusoidal regions (1.3%) and lower hypoxia in endosteal regions (2.9%) (44)] in the BM have an important effect on cell fate and function, including self-renewal and quiescence maintenance in stem cells, B cell lineage recruitment, and V(D)J rearrangement in pro-B/pre-B cells (48). Nevertheless, the PO2 landscape of B cells at different developmental stages in BM needs to be accurately matched and further studied. Immature B cells enter into well-oxygenated circulation (average PO2 13.2%) and are in a relatively normoxic state before transport to various secondary lymphoid tissues. Then, B cells reencounter hypoxic niches within lymphoid tissues (average PO2: spleen 2.3% and lymph node 1.85%) and interact with specific antigens to differentiate into plasma cells and memory cells. Of note, the majority of GCs (PO2 approximately 1%) within secondary lymphoid tissues are poorly vascularized or are >40 μm away from blood vessels and highly express HIF protein, which is essential for the formation of antibody repertoire diversity and the development of an effective immune response (49–51). It is noteworthy that activated B cells mainly experience hypoxia in the light zone of GC, but the precise value of PO2 in the light/dark zone of GCs has not been directly reported (51). Overall, the B cell life cycle experiences hypoxia niches over a wide range of oxygen tensions (heterogeneity of local PO2) and HIF-α is activated to varying degrees, which will broadly shape B cell fate and profoundly affect B cell metabolism and function (Table 1).
Hypoxic regulation of B cell biology
The B cell life cycle frequently encounters hypoxia in physiological states, which leads to the variable activation of HIF in immunological niches. The temporal, spatial, and dynamic nature of hypoxic stimuli induces a series of precise regulatory events in B cells biology. We next discuss how hypoxia and HIF signaling regulate the development, metabolism, and function of B cells in physiological immunological niches.
Hypoxia regulates B cell development
Many studies have shown that hypoxia plays a vital role in regulating B cell development, mainly through the HIF signaling pathway. The most direct evidence is that HIF-1α gene deficiency causes abnormal B cell development and autoimmunity (autoantibodies accumulation) (59–61). From the perspective of different immunological niches, hypoxic niches in BM play an important role in the maintenance of HSCs homeostasis and in the regulation of cellular survival, proliferation, and differentiation (62). HIF signaling activation by PHD inhibitors promotes HSCs quiescence (reduces proliferation and enhances myeloid potential) in an HIF-dependent manner and facilitates hematopoiesis and improves blood recovery (including B cells) after severe irradiation (63). Hypoxia also plays an important synergistic effect on improving antibody-secreting plasma cell survival by regulating paracrine survival factors like fibronectin and YWHAZ (also known as 14-3-3zeta/delta) from BM stroma cells (64). Chabi et al. reported that hypoxia specifically favors in vitro human lymphoid development from early hematopoietic progenitor cells and enhances the in vivo B cell potential of lymphoid-primed multipotent progenitors (48). B cell lymphopoiesis occurs primarily in the BM and consists of multiple cellular stages. Florian et al. found that HIF activity is high in pro-B and pre-B cells and decreases in immature B cells. Stage-specific HIF suppression is required for normal B cell development because constitutive HIF-1α activation (VHL deficiency) leads to immature B cell developmental arrest and a reduction in peripheral B cell numbers (52).
In peripheral immunological niches, hypoxia causes an HIF-1α-dependent growth arrest in mouse splenic B cells through upregulation of the expression of the cyclin-dependent kinase inhibitors p21 and p27 (65). Hypoxia regulates WiL2-NS B cell (a human spleen-derived B lymphoblast cells) viability by mediating CXCR4 expression through HIF-1α/Nrf2 cooperatively binding to its promoter (66). Matthieu et al. demonstrated that hypoxia increases the generation of plasmablasts starting from peripheral blood-derived memory B cells by increasing cell cycle and division number via HIF-1α and HIF-2α (50).
The GC is a specialized micro-anatomy structure that develops in peripheral lymphoid tissues, where activated B cells undergo SHM and CSR to produce high-affinity, class-switched antibodies. The GC consists of a dark zone and light zone, where the dark zone contains actively dividing B cells known as centroblasts and the light zone contains nondividing B cells known as centrocytes (67). Sung et al. reported that activated B cells experience hypoxia predominantly in the GC light zone of the spleen, lymph node, and Peyer’s patches. B lymphoblasts rapidly proliferate in the dark zone, but they reduce proliferation and increase B cell death in the light zone (51). Therefore, hypoxia regulates B cell development in central and peripheral immunological niches from multiple aspects, mainly mediated by the HIF signaling pathway.
Hypoxic preconditioning is often used clinically as a protocol that induces a naturally protective immunophenotype in some diseases like strokes (68), this is at least partially effected via B cell function. Nancy et al. reported that repetitive hypoxic preconditioning (RHP) can enhance the protection from stroke-induced injury, mechanistically, as RHP increases in CD1dhiCD5+ regulatory B cells and inhibits the proliferation, development, and differentiation of B cells and B-T cell adaptive immune interactions in the spleen (69). Moreover, HIF activation or knockdown in other immune cells affect the development of B cells after vaccine immunization. Sung et al. found that the frequency of GC-phenotype B cells (GL7+ CD95+ IgDneg B220+) and antigen-specific B cells were substantially reduced in HIF knockdown mice after immunization with hapten-conjugated ovalbumin, which indicates that HIF plays a vital role in GC immune responses and the production of specific antibody-secreted plasma cells (70).
Hypoxia regulates B cell metabolism
From the antigen-independent B cell development in the BM to the antigen-driven production of antibody-secreted plasma cells in the peripheral GC, life for a B cell experiences dynamic metabolic processes to support the demands of cell differentiation and effector function (71).
B cells undergo multiple rounds of metabolic state changes during their development in the BM. Dynamic hypoxia in the BM activates HIF signaling, thereby affecting the metabolic activity of B cells at different developmental stages, which is necessary for the development and functional improvement of B cells (72). HSCs preferentially utilize glycolysis instead of mitochondrial oxidative phosphorylation for their energy needs (73). Early pro-B cells have a high metabolic demand to meet their own rapid proliferation needs, so both glycolysis and oxidative phosphorylation (OXPHOS) are increased because of the action of IL-7 signaling (74). After completing VDJ recombination in of heavy chains, late pro-B cells reduce their metabolic activity and gradually become quiescent state. Early-stage large pre-B cells express a surface pre-BCR formed from IgM pairs with a surrogate light chain, which triggers rapid proliferation and clonal expansion accompanied by increased glycolysis and OXPHOS (75). Large pre-B cells undergo a proliferative burst with the highest glycolysis activity occurring in B cell progenitors in the BM. Rapid proliferation at this stage increases cellular oxygen consumption to further enhance microenvironmental hypoxia and HIF-1α stability. Then, this activates the expression of glucose transporters and glycolytic enzymes to maintain high levels of glycolysis (76). As large pre-B cells differentiate into nonproliferating small pre-B cells, the metabolic activity of these cells gradually declines but rises again when these cells complete light-chain recombination (75). After differentiating into immature B cells, the metabolism levels of these cells decreases and may be dependent on fatty acids as their primary metabolic substrates (74). B cell development in the BM was shown to be highly dependent on glucose or glycolysis, and HIF-1α-deficient B cells in the BM are less capable of using glucose because glucose transporters and glycolytic-enzyme expression have been greatly decreased. HIF-1α-enabled glycolysis is required in a developmental stage-specific manner during B cell development in the BM (75). Additionally, some cytokines or growth factors, such as IGF-1 (77) and hematopoietic cell growth factors (78), are capable of regulating B cell glucose utilization and glycolysis to influence B cell fate. Studies have shown that the stromal cell-secreted cytokine IL-7 induces the PI3K-Akt pathway and promotes PLCγ-mediated mTOR activation, meaning these will contribute to promoting glucose utilization and B cell development (79, 80). Whether hypoxia directly affects these cytokines or growth factors to regulate B cell metabolism and fate remains to be further explored.
To date, relatively few studies have examined the metabolic state of transitional B cells in the circulation. Farmer et al. reported that transitional B cells had a relatively high metabolic activity, which adapted to the high oxygen levels in the circulation. The induction of metabolic quiescence was shown to be required for the switch from transitional B cells to FOB cells (22). In peripheral lymphoid tissues, naive mature B cells are in a quiescent state with low metabolic activity (81). When mature naive B cells respond to antigen, BCR stimulation mediates a rapid increase in glycolysis and OXPHOS (82). The BAFF-BAFF receptor signaling pathway induces glucose utilization through inhibiting glycogen synthase kinase 3 (Gsk3) through the PI3K-Akt pathway. Gsk3 is an important metabolic checkpoint regulator that promotes the survival of naive recirculating B cells and maintains metabolic quiescence (83). Activated B cells increase the expression of HIF-1α and cMyc to maintain glycolysis. Subsequently, B cells enter the GC cycle between the dark zone and the light zone. HIF-1α was shown to be dynamically regulated in GC B cells to ensure the different functions of HIF-1α at different stages (67). B cells in dark zones differentiate into centroblasts accompanied by BCL-6 upregulation and cMyc inhibition, and HIF-1α-mediated glycolytic activity under normoxia (aerobic glycolysis) provides energy for rapid proliferation of centroblasts (84). B cells then enter into the GC light zone and differentiation into centrocytes. Oxygen- and nutrient-poor microenvironments in the light zone maintain HIF-1α and AMPK expression and promote cell catabolism. cMyc is then upregulated in centrocytes and induces a glycolytic burst (anaerobic glycolysis) to meet the metabolic demands of B cells from the cells in the light zone reentering the dark zone. Centroblasts and centrocytes upregulate the expression of LDHB and MCT1 to utilize extracellular lactate (83). A recent study, however, demonstrated that highly proliferative B cells in the GC possess a special metabolic profile in which they mainly rely on fatty acid oxidation rather than on glycolysis. This view contrasts with previous reports that GC B cells rely on glycolysis for energy, which may be related to the different cell types used in the experiments (activated B cells in vitro and GC B cells ex vivo were used, respectively) (85). B cells differentiate into memory B cells or plasma cells after GC reaction. Memory B cells self-renew slowly and are relatively metabolically quiescent. Although plasma cells are highly terminally differentiated cells with no or weak proliferation abilities, they have high metabolic activity (high glucose uptake and glycolysis/OXPHOS) to supply energy for the production and secretion of specific antibodies (86). The effect of hypoxia on plasma cell metabolism remains to be further studied.
Hypoxia regulates B cell function
The ultimate mission of B cells in vertebrates is to produce high-affinity antibody-secreted plasma cells and long-lived memory B cells to eliminate specific pathogens and provide long-term protection (87). Many studies have demonstrated that hypoxia and HIF signaling can regulate B cell function through diverse and sophisticated manners, such as B cell migration, immune responses, SHM, and CSR. A nuanced understanding of the role of HIF in B cell function is very important, because the HIF signaling pathway is pharmacologically tractable and may serve as an important target for vaccine adjuvants and immunity-related treatment (6).
B cell migration between different immunological niches, such as BM, circulation, and peripheral immune tissues, is essential for their normal function. Hypoxia has been reported to have different effects on B cell migration depending on B cell location. Hypoxia upregulates the expression of CXCR4 at both the transcriptional and posttranslational levels (88), which induces the migration of peripheral blood B cells while inhibiting that of GC B cells, thus increasing mature B cell egress from Peyer’s patches and promoting the retention of immature B cells in the BM (89, 90). HIF also can modulate the B cell immune response, but the specific role of HIF in antibody formation is still controversial. Burrows et al. demonstrated that HIF-1α activation reduces BCR editing and repertoire diversity in murine B cells (52). Cho et al. found that hypoxia in the GC light zone has intricate effects on Td B cell response. Specifically, in this context, HIF activation reduces GC B cell proliferation, impairs affinity maturation, decreases the pro-inflammatory IgG2c isotype, and weaken secondary antibody responses. HIF hyperactivation is also detrimental to B cell function, but physiological hypoxia in normal GC may be time-specific and of functional benefit (51). In contrast, Abbott et al. reported that hypoxic conditions in vitro accelerate Ig CSR and plasma cell formation, while respiratory hyperoxia suppresses CSR and the GC reaction during immunization in vivo (49). Although these two studies appear to have opposite results, they imply that precise and appropriate HIF activation is necessary for an optimal immune response, and a targeted and timely manipulation of the HIF signaling pathway may provide an efficient approach for immune intervention.
SHM introduces high-frequency point mutations (10-4–10-3/base pair/cell division) in the variable regions of the Ig heavy and light chains higher than the rate of genome-wide spontaneous mutations (10–9), which facilitates iterative screening to obtain high-affinity antibodies (91). CSR events allow antibody-switching from IgM to IgG, IgA, or IgE isotypes by C-region exchange to achieve diverse effect functions. Hypoxia has been reported to affect affinity maturation and class-switching by modulating SHM and CSR, respectively, yet a detailed mechanism remains to be elucidated (6). Some DNA damage repair-related enzymes, such as activation-induced deaminase (AID), play an important role in the CSR and SHM (92). Hypoxia impairs antibody class-switching to the IgG2c isotype by decreasing AID expression at the mRNA and protein levels, but it does not reduce IgA switching (51). Reportedly, however, hypoxic conditions (1% O2) in vitro also can accelerate plasma cell formation and IgM+→IgG1+ class-switching (49). The contrasting effects of hypoxia in CSR may be associated with the specific differences in B cell subsets, as well as the extent and duration of hypoxia (6). Hypoxia has been reported to inhibit mismatch repair proteins (MSH2/MSH6) and base-excision repair proteins in the tumor-derived hypoxic niches, but promotes error-prone translation DNA synthesis (TLS) polymerase and induce mutagenesis, which suggested that hypoxia may affect SHM and CSR by mediating DNA-repair proteins (93). Besides SHM and CSR, RAG1/RAG2-mediated V(D)J gene rearrangement is a key factor in the formation of antibody repertoire diversity (94). The role of hypoxia in V(D)J gene rearrangement warrants further exploration in future studies, because this may provide an important target for the development of vaccine adjuvants in specific scenarios.
Noncoding RNAs, such as miRNA (95), lncRNA (96) and circRNA (97), play an important regulatory role in B cell development and function at the post-transcriptional level (98). For example, miR-181b impairs CSR by negatively regulating AID in B cells (99) and miR-155 promotes GC formation in vivo and the generation of IgG1-secreting plasma cells by downregulating the target gene Pu.1 (100). Hypoxia also has been reported to affect B cell function in part through regulating noncoding RNAs. A typical example is miR-210, a master hypoxamiR induced by HIF-1α and Oct-2 during B cell activation, which causes abnormalities in B cell subsets and functions, including B cell proliferation and CSR (101). Establishing the detailed regulatory network of hypoxamiR and hypoxia-responsive lncRNAs in B cell lymphogenesis is beneficial for systematically elucidating the effect of hypoxia on B cell biology.
Moreover, hypoxia has an effect on other immune cell interactions by modulating some cytokines (such as IL-2, IL-4, IL-10, and IFN-γ) to indirectly regulate B cell function (102). HIF-1α depletion from CD4+ T cells reduces the frequencies of antigen-specific GC B cells and overall antigen-specific antibodies after NP-ovalbumin immunization (70). Conversely, hypoxia also affects T cell immune responses (CD8+ T cell) by promoting the secretion of B cell-derived extracellular vesicles through HIF-1α-mediated Rab27a transcription (103). Intriguingly, HIF signaling is also activated in an oxygen-independent manner. The downstream activation of BCR, toll-like receptor (TLR) and cytokine receptor signaling cascades stabilizes HIF-α protein in B cells, which highlights the important role of oxygen-independent HIF signaling in B cell immune responses (6, 13).
Concluding remarks
B cells have a complex life cycle, and the oxygen tensions in corresponding developmental niches of stage-specific B cells are highly dynamic. PO2 fluctuation in different niches affects B cells lymphogenesis mainly through the HIF signaling pathway. Many studies from different perspectives have shown that hypoxia plays an important regulatory role in the development, metabolism, and function of B cells. It is necessary to recognize that hypoxia is a double-edged sword for B cells—that is, hypoxia in physiological states is required for B cell biology, whereas acute or severe hypoxia may induce or exacerbate B cell dysfunction. The regulation of hypoxia on B cells is sophisticated and multifaceted, such as HIF-dependent or HIF-independent pathways, regulation of protein-coding genes or through noncoding RNAs at the post-transcriptional level in a regulatory manner, and by direct regulation of B cells or indirect regulation of other immune cells as regulatory objects.
It will be interesting to elucidate when HIF is activated in the B cell life cycle, how hypoxia plays a regulatory role in different B cell resident immune niches, and what the regulatory outputs are in different scenarios. A comprehensive understanding of the complex regulatory mechanisms of hypoxia on B cell biology will (a) facilitate precise therapeutic manipulation of the HIF signaling pathway [B cell stage-specific or niche-specific HIF regulator (activator or inhibitor)] to modulate immune responses during vaccination as well as some immunity-related diseases (especially autoimmune diseases); (b) be beneficial to comprehensively evaluate the effect of drug treatment or vaccine immunity from the perspective of intrinsic hypoxia in the body’s internal environment; (c) help to compare the similarities and differences between physiological hypoxia and tumor’s microenvironmental hypoxia, and provide theoretical support for improving the effectiveness of antitumor therapy.
The following aspects deserve further research in future studies. (a) The direct detection method of PO2 in smaller micro-structure like light/dark zone of GCs is to be developed or optimized, based on this, the PO2 at corresponding niches of stage-specific B cells remains to be precisely decrypted. (b) Like HIF-1α, HIF-2α also plays a pivotal role in other immune cells, and the expression pattern and function of HIF-2α in B cells remains unclear (8). (c) Additionally, Bregs are immunosuppressive cells that support immunological tolerance, all while exerting immunosuppressive effects by secreting specific cytokines, such as IL-10, IL-35, and TGF-β (15). To our knowledge, the regulatory effects of hypoxia on Bregs have been rarely reported thus far. (d) Furthermore, the gastrointestinal mucosa is an important immunological niche, in which physiological hypoxia exists and can modulate innate immunity by promoting epithelial cell barrier functioning and regulating resident immune cells (104). How hypoxia affects B cell development and SIgA (a secretory antibody that play a critical role in mucosal immunity) production in intestinal mucosal immune tissues (such as Peyer’s patches) needs further study, which is beneficial to the development of therapeutic targets for intestinal-related diseases. (e) Last but not least, the SARS-CoV-2 virus, an important threat to global public health, has been reported to cause systemic hypoxia and a notable reduction in B cell numbers. Hypoxia contributes to the pronounced and persistent B cell pathology of acute SARS-CoV-2 (105). Evaluating the effect of early oxygen therapy on B cell immunodeficiency provides a reference for the treatment of similar major infectious diseases (106, 107).
Author contributions
Conceptualization, JZ and LG. Literature investigation, XW, JM, KL, and JS. Writing original draft: JZ and XW. Supervision: ML and LG. All authors have read and agreed to the published version of the manuscript.
Funding
This work was funded by the National Natural Science Foundation of China (32072687), the Natural Science Foundation of Chongqing (cstc2021jcyj-msxmX0630), the Performance Incentive and Guidance Project for Scientific Research Institutions in Chongqing (20524), the Special funded project of Chongqing Postdoctoral Fund (21310), Sichuan International Science and Technology Innovation Cooperation/Hong Kong, Macao and Taiwan Science, Technology Innovation Cooperation Project (2021YFH0033) and Science Foundation of the Sichuan Province (2022YFQ0022).
Conflict of interest
Authors JZ and LG were employed by the company Chongqing Camab Biotech Ltd.
The remaining authors declare that the research was conducted in the absence of any commercial or financial relationships that could be construed as a potential conflict of interest.
Publisher’s note
All claims expressed in this article are solely those of the authors and do not necessarily represent those of their affiliated organizations, or those of the publisher, the editors and the reviewers. Any product that may be evaluated in this article, or claim that may be made by its manufacturer, is not guaranteed or endorsed by the publisher.
References
1. Turvey SE, Broide DH. Innate immunity. J Allergy Clin Immun (2010) 125(2):S24–32. doi: 10.1016/j.jaci.2009.07.016
2. Boothby MR, Hodges E, Thomas JW. Molecular regulation of peripheral b cells and their progeny in immunity. Genes Dev (2019) 33(1-2):26–48. doi: 10.1101/gad.320192.118
3. Beerman I, Luis TC, Singbrant S, Celso CL, Méndez-Ferrer S. The evolving view of the hematopoietic stem cell niche. Exp Hematol (2017) 50:22–6. doi: 10.1016/j.exphem.2017.01.008
4. Campbell EL, Kao DJ, Colgan SP. Neutrophils and the inflammatory tissue microenvironment in the mucosa. Immunol Rev (2016) 273(1):112–20. doi: 10.1111/imr.12456
5. Biswas S, Davis H, Irshad S, Sandberg T, Worthley D, Leedham S. Microenvironmental control of stem cell fate in intestinal homeostasis and disease. J Pathol (2015) 237(2):135–45. doi: 10.1002/path.4563
6. Burrows N, Maxwell PH. Hypoxia and b cells. Exp Cell Res (2017) 356:197–203. doi: 10.1016/j.yexcr.2017.03.019
7. Taylor CT, Colgan SP. Regulation of immunity and inflammation by hypoxia in immunological niches. Nat Rev Immunol (2017) 17:774–85. doi: 10.1038/nri.2017.103
8. Palazon A, Goldrath A, Nizet V, Johnson R. HIF transcription factors, inflammation, and immunity. Immunity (2014) 41(4):518–28. doi: 10.1016/j.immuni.2014.09.008
9. McNamee EN, Johnson DK, Homann D, Clambey ET. Hypoxia and hypoxia-inducible factors as regulators of T cell development, differentiation, and function. Immunol Res (2013) 55(1):58–70. doi: 10.1007/s12026-012-8349-8
10. Bannoud N, Dalotto-Moreno T, Kindgard L, García P, Croci DO. Hypoxia supports differentiation of terminally exhausted cd8 t cells. Front Immunol (2021) 12:660944. doi: 10.3389/fimmu.2021.660944
11. Colgan SP, Furuta GT, Taylor CT. Hypoxia and innate immunity: keeping up with the hifsters. Annu Rev Immunol (2020) 38(1):341–63. doi: 10.1146/annurev-immunol-100819-121537
12. Pieper K, Grimbacher B, Eibel H. B-cell biology and development. J Allergy Clin Immun (2013) 131(4):959–71. doi: 10.1016/j.jaci.2013.01.046
13. Chen Y, Gaber T. Hypoxia/HIF modulates immune responses. Biomedicines (2021) 9(3):260. doi: 10.3390/biomedicines9030260
14. Cooper M, Peterson R, Good RA. Delineation of the thymic and bursal lymphoid systems in the chicken. Nature (1965) 205:143–6. doi: 10.1038/205143a0
15. Rosser E, Mauri C. Regulatory b cells: origin, phenotype, and function. Immunity (2015) 42(4):607–12. doi: 10.1016/j.immuni.2015.04.005
16. Kageyama Y, Katayama N. Ontogeny of human B1 cells. Int J Hematol (2019) 111(1):628–33. doi: 10.1007/s12185-019-02775-y
17. Wang JY. B cells in immunity and tolerance. Adv Exp Med Biol (2020) 1254:1–22. doi: 10.1007/978-981-15-3532-1
18. Catalán D, Mansilla MA, Ferrier A, Soto L, Aravena O. Immunosuppressive mechanisms of regulatory b cells. Front Immunol (2021) 12:611795. doi: 10.3389/fimmu.2021.611795
19. Ranzoni AM, Tangherloni A, Berest I, Riva SG, Myers B, Strzelecka PM, et al. Integrative single-cell rna-seq and atac-seq analysis of human developmental hematopoiesis. Cell Stem Cell (2021) 28(3):357–8. doi: 10.1016/j.stem.2020.11.015
20. David N. Mechanisms of central tolerance for b cells. Nat Rev Immunol (2017) 17:281–94. doi: 10.1038/nri.2017.19
21. Tussiwand R, Rauch M, Flück L, Rolink AG. BAFF-r expression correlates with positive selection of immature b cells. Eur J Immunol (2012) 42(1):206–16. doi: 10.1002/eji.201141957
22. Farmer JR, Allard-Chamard H, Sun N, Ahmad M, Pillai S. Induction of metabolic quiescence defines the transitional to follicular b cell switch. Sci Signal (2019) 12(604):eaaw5573. doi: 10.1126/scisignal.aaw5573
23. Zhao Y, Uduman M, Siu J, Tull TJ, Sanderson JD, Wu Y, et al. Spatiotemporal segregation of human marginal zone and memory b cell populations in lymphoid tissue. Nat Commun (2018) 9(1):1–15. doi: 10.1038/s41467-018-06089-1
24. Hammad H, Vanderkerken M, Pouliot P, Deswarte K, Toussaint W, Vergote K, et al. Transitional b cells commit to marginal zone b cell fate by Taok3-mediated surface expression of ADAM10. Nat Immunol (2017) 18(3):313–20. doi: 10.1038/ni.3657
25. Dawes JC, Uren AG. Forward and reverse genetics of b cell malignancies: from insertional mutagenesis to crispr-cas. Front Immunol (2021) 12:670280. doi: 10.3389/fimmu.2021.670280
26. Morgan D, Tergaonkar V. Unraveling b cell trajectories at single cell resolution. Trends Immunol (2022) 43(3):210–29. doi: 10.1016/j.it.2022.01.003
27. Vasilev DS, Dubrovskaya NM, Tumanova NL, Zhuravin IA. Prenatal hypoxia in different periods of embryogenesis differentially affects cell migration, neuronal plasticity, and rat behavior in postnatal ontogenesis. Front Neurosci (2016) 10:126. doi: 10.3389/fnins.2016.00126
28. Iacobazzi V. Cancer cell metabolism in hypoxia: role of hif-1 as key regulator and therapeutic target. Int J Mol Sci (2021) 22:5703. doi: 10.3390/ijms22115703
29. Yoann T, Jonathan FSM, Denis C, Arturo AV, Goncalo M, Laure P. Effects of hypoxia on metabolic functions in marine organisms: Observed patterns and modelling assumptions within the context of dynamic energy budget (DEB) theory. J Sea Res (2018) 143(1):231–42. doi: 10.1016/j.seares.2018.05.001
30. Kierans SJ, Taylor CT. Regulation of glycolysis by the hypoxia-inducible factor (HIF): implications for cellular physiology. J Physiol (2020) 599:23–37. doi: 10.1113/JP280572
31. Schdel J, Mole DR, Ratcliffe PJ. Pan-genomic binding of hypoxia-inducible transcription factors. Biol Chem (2013) 394(4):507–17. doi: 10.1515/hsz-2012-0351
32. Ewelina K, Christian S. Hypoxia, metabolism and immune cell function. Biomedicines (2018) 6(2):56. doi: 10.3390/biomedicines6020056
33. Yang SL, Wu C, Xiong ZF, Fang X. Progress on hypoxia-inducible factor-3: Its structure, gene regulation and biological function. Mol Med Rep (2015) 12:2411–6. doi: 10.3892/mmr.2015.3689
34. Duan C. Hypoxia-inducible factor 3 biology: complexities and emerging themes. Am J Physiol-Cell Ph (2016) 310:C260–9. doi: 10.1152/ajpcell.00315.2015
35. Semenza GL. Hypoxia-inducible factor 1 (HIF-1) pathway. Science’s STKE (2007) 407:cm8. doi: 10.1126/stke.4072007cm8
36. Maxwell PH, Wiesener MS, Chang GW, Clifford SC, Vaux EC, Cockman ME, et al. The tumour suppressor protein VHL targets hypoxia-inducible factors for oxygen-dependent proteolysis. Nature (1999) 399(6733):271–5. doi: 10.1038/20459
37. Semenza G. Hypoxia-inducible factors in physiology and medicine. Cell (2012) 148(3):399–408. doi: 10.1016/j.cell.2012.01.021
38. Schödel J, Oikonomopoulos S, Ragoussis J, Pugh CW, Ratcliffe PJ, Mole DR. High-resolution genome-wide mapping of HIF-binding sites by ChIP-seq. Blood (2011) 117(23):e207–17. doi: 10.1182/blood-2010-10-314427
39. Azzouzi HE, Leptidis S, Doevendans PA, Windt L. HypoxamiRs: regulators of cardiac hypoxia and energy metabolism. Trends Endocrin Met (2015) 26:502–8. doi: 10.1016/j.tem.2015.06.008
40. Shih JW, Kung HJ. Long non-coding RNA and tumor hypoxia: new players ushered toward an old arena. J BioMed Sci (2017) 24(1):1–19. doi: 10.1186/s12929-017-0358-4
41. Cimmino F, Avitabile M, Lasorsa VA, Montella A, Pezone L, Cantalupo S, et al. HIF-1 transcription activity: HIF1A driven response in normoxia and in hypoxia. BMC Med Genet (2019) 20(1):1–15. doi: 10.1186/s12881-019-0767-1
42. Ye J, Medzhitov R. Control strategies in systemic metabolism. Nat Met (2019) 1(10):947–57. doi: 10.1038/s42255-019-0118-8
43. Elia I, Haigis MC. Metabolites and the tumour microenvironment: from cellular mechanisms to systemic metabolism. Nat Met (2021) 3(1):21–32. doi: 10.1038/s42255-020-00317-z
44. Spencer JA, Ferraro F, Roussakis E, Klein A, Lin CP. Direct measurement of local oxygen concentration in the bone marrow of live animals. Nature (2014) 508(7495):269–73. doi: 10.1038/nature13034
45. Unwin RD, Smith DL, Blinco D, Wilson CL, Miller CJ, Evans CA, et al. Quantitative proteomics reveals posttranslational control as a regulatory factor in primary hematopoietic stem cells. Blood (2006) 107(12):4687–94. doi: 10.1182/blood-2005-12-4995
46. Takubo K, Goda N, Yamada W, Iriuchishima H, Ikeda E, Kubota Y, et al. Regulation of the hif-1α level is essential for hematopoietic stem cells. Cell Stem Cell (2010) 7:391–402. doi: 10.1016/j.stem.2010.06.020
47. Parmar K, Mauch P, Vergilio JA, Sackstein R, Down JD. Distribution of hematopoietic stem cells in the bone marrow according to regional hypoxia. P Nat Acad Sci USA (2007) 104(13):5431–6. doi: 10.1073/pnas.0701152104
48. Chabi S, Uzan B, Naguibneva I, Rucci J, Haddad R. Hypoxia regulates lymphoid development of human hematopoietic progenitors. Cell Rep (2019) 29(8):2307–20. doi: 10.1016/j.celrep.2019.10.050
49. Abbott RK, Thayer M, Labuda J, Silva M, Sitkovsky M. Germinal center hypoxia potentiates immunoglobulin class switch recombination. J Immunol (2016) 197(10):4014–20. doi: 10.4049/jimmunol.1601401
50. Schoenhals M, Jourdan M, Bruyer A, Kassambara A, Klein B, Moreaux J. Hypoxia favors the generation of human plasma cells. Cell Cycle (2017) 16(11):1104–17. doi: 10.1080/15384101.2017.1317408
51. Cho SH, Raybuck AL, Stengel K, Wei M, Beck TC, Volanakis E, et al. Germinal centre hypoxia and regulation of antibody qualities by a hypoxia response system. Nature (2016) 537(7619):234–8. doi: 10.1038/nature19334
52. Burrows N, Bashford-Rogers R, Bhute VJ, Pealver A, Maxwell PH. Dynamic regulation of hypoxia-inducible factor-1α activity is essential for normal b cell development. Nat Immunol (2020) 21(11):1–13. doi: 10.1038/s41590-020-0772-8
53. Baccin C, Al-Sabah J, Velten L, Helbling PM, Grünschlger F, Hernández-Malmierca P, et al. Combined single-cell and spatial transcriptomics reveal the molecular, cellular and spatial bone marrow niche organization. Nat Cell Biol (2020) 22:38–48. doi: 10.1038/s41556-019-0439-6
54. Ranzoni AM, Tangherloni A, Berest I, Riva SG, Myers B, Strzelecka PM, et al. Integrative single-cell rna-seq and atac-seq analysis of human developmental hematopoiesis - sciencedirect. Cell Stem Cell (2020) 3:472–87. doi: 10.1016/j.stem.2020.11.015
55. Brioschi S, Wang WL, Peng V, Wang M, Shchukina I, Greenberg ZJ, et al. Heterogeneity of meningeal b cells reveals a lymphopoietic niche at the CNS borders. Science (2021) 373:eabf9277. doi: 10.1126/science.abf9277
56. Ortiz-Prado E, Dunn JF, Vasconez J, Castillo D, Viscor G. Partial pressure of oxygen in the human body: a general review. Am J Blood Res (2019) 9(1):1–14.
57. Lr B, Sd B, Sm B, Lp D, Sbm D, Jp D, et al. Micro-needle implantable electrochemical oxygen sensor: ex-vivo and in-vivo studies. Biosens Bioelectron (2020) 153:112028. doi: 10.1016/j.bios.2020.112028
58. Sokal A, Chappert P, Barba-Spaeth G, Roeser A, Fourati S, Azzaoui I, et al. Maturation and persistence of the anti-SARS-CoV-2 memory b cell response. Cell (2021) 184(5):1201–13. doi: 10.1016/j.cell.2021.01.050
59. Kojima H, Gu H, Nomura S, Caldwell CC, Kobata T, Carmeliet P, et al. Abnormal b lymphocyte development and autoimmunity in hypoxia-inducible factor 1α-deficient chimeric mice. P Nat Acad Sci USA (2002) 99(4):2170–4. doi: 10.1073/pnas.052706699
60. Kojima H, Sitkovsky MV, Cascalho M. HIF-1α deficiency perturbs T and b cell functions. Curr Pharm Design (2003) 99:2170–4. doi: 10.2174/1381612033454388
61. Kojima H, Jones BT, Chen J, Cascalho M, Sitkovsky MV. Hypoxia-inducible factor 1α-deficient chimeric mice as a model to study abnormal b lymphocyte development and autoimmunity. Methods Enzymol (2004) 381(1):218–29. doi: 10.1016/S0076-6879(04)81016-4
62. Morikawa T, Takubo K. Hypoxia regulates the hematopoietic stem cell niche. Pflü Arch Eur J Phy (2015) 468(1):1–10. doi: 10.1007/s00424-015-1743-z
63. Forristal CE, Winkler IG, Nowlan B, Barbier V, Levesque JP. Pharmacologic stabilization of HIF-1α increases hematopoietic stem cell quiescence in vivo and accelerates blood recovery after severe irradiation. Blood (2013) 121(5):759–69. doi: 10.1182/blood-2012-02-408419
64. Nguyen DC, Garimalla S, Xiao H, Kyu S, Lee EH. Factors of the bone marrow microniche that support human plasma cell survival and immunoglobulin secretion. Nat Commun (2018) 9(1):1–12. doi: 10.1038/s41467-018-05853-7
65. Goda N, Ryan HE, Khadivi B, McNulty W, Rickert RC, Johnson RS. Hypoxia-inducible factor 1α is essential for cell cycle arrest during hypoxia. Mol Cell Bio (2003) 23:359–69. doi: 10.1128/MCB.23.1.359-369.2003
66. Jang JW, Thuy PX, Lee JW, Moon EY. CXCR4 promotes b cell viability by the cooperation of nuclear factor (erythroid-derived 2)-like 2 and hypoxia-inducible factor-1α under hypoxic conditions. Cell Death Dis (2021) 12:1–12. doi: 10.1038/s41419-021-03615-w
67. Mesin L, Ersching J, Victora GD. Germinal center b cell dynamics. Immunity (2016) 45:471–82. doi: 10.1016/j.immuni.2016.09.001
68. Stowe AM, Altay T, Freie AB, Gidday JM. Repetitive hypoxia extends endogenous neurovascular protection for stroke. Ann Neurol (2011) 69(6):975–85. doi: 10.1002/ana.22367
69. Monson NL, Ortega SB, Ireland SJ, Meeuwissen AJ, Chen D, Plautz EJ, et al. Repetitive hypoxic preconditioning induces an immunosuppressed b cell phenotype during endogenous protection from stroke. J Neuroinflamm (2014) 11(1):1–18. doi: 10.1186/1742-2094-11-22
70. Cho HS, Raybuck AL, Blagih J, Kemboi E, Haase VH, Jones RG, Boothby MR. Hypoxia-inducible factors in CD4+ T cells promote metabolism, switch cytokine secretion, and T cell help in humoral immunity. P Nat Acad Sci U S A (2019) 116:8975–84. doi: 10.1073/pnas.1811702116
71. Caro-Maldonado A, Wang R, Nichols AG, Kuraoka M, Milasta S, Sun LD, et al. Metabolic reprogramming is required for antibody production that is suppressed in anergic but exaggerated in chronically baff-exposed b cells. J Immunol (2014) 192(8):3626–36. doi: 10.4049/jimmunol.1302062
72. Sophia U, Merle S, Wolfgang S, Hans-Martin J, Dimitrios M, Dirk M. Regulation of energy metabolism during early b lymphocyte development. Int J Mol Sci (2018) 19(8):2192. doi: 10.3390/ijms19082192
73. Simsek T, Kocabas F, Zheng J, Deberardinis RJ, Mahmoud AI, Olson EN, et al. The distinct metabolic profile of hematopoietic stem cells reflects their location in a hypoxic niche. Cell Stem Cell (2010) 7(3):380–90. doi: 10.1016/j.stem.2010.07.011
74. Wilson CS, Moore DJ. B cell metabolism: an understudied opportunity to improve immune therapy in autoimmune type 1 diabetes. Immunometabolism (2020) 2(1):e200016. doi: 10.20900/immunometab20200016
75. Kojima H, Kobayashi A, Sakurai D, Kanno Y, Hase H, Takahashi R, et al. Differentiation stage-specific requirement in hypoxia-inducible factor-1alpha-regulated glycolytic pathway during murine b cell development in bone marrow. J Immunol (2010) 184(1):154–63. doi: 10.4049/jimmunol.0800167
76. Stein M, Dütting S, Mougiakakos D, Bösl M, Fritsch K, Reimer D, et al. A defined metabolic state in pre b cells governs b-cell development and is counterbalanced by swiprosin-2/EFhd1. Cell Death Differ (2017) 24:1239–52. doi: 10.1038/cdd.2017.52
77. Taguchi T, Takenouchi H, Matsui J, Tang WR, Itagaki M, Shiozawa Y, et al. Involvement of insulin-like growth factor-I and insulin-like growth factor binding proteins in pro-b-cell development. Exp Hematol (2006) 34(4):508–18. doi: 10.1016/j.exphem.2006.01.009
78. Bauer DE, Harris MH, Plas DR, Lum JJ, Hammerman PS, Rathmell JC, et al. Cytokine stimulation of aerobic glycolysis in hematopoietic cells exceeds proliferative demand. FASEB J (2004) 18:1303–5. doi: 10.1096/fj.03-1001fje
79. Clark MR, Mandal M, Ochiai K, Singh H. Orchestrating b cell lymphopoiesis through interplay of IL-7 receptor and pre-b cell receptor signalling. Nat Rev Immunol (2013) 14(2):69–80. doi: 10.1038/nri3570
80. Yu M, Chen Y, Zeng H, Zheng Y, Fu G, Zhu W, et al. PLCγ-dependent mTOR signalling controls IL-7-mediated early b cell development. Nat Commun (2017) 8(1):1–18. doi: 10.1038/s41467-017-01388-5
81. Akkaya M, Pierce S. From zero to sixty and back to zero again: the metabolic life of b cells. Curr Opin Immunol (2018) 57:1–7. doi: 10.1016/j.coi.2018.09.019
82. Munir A, Javier T, Roesler AS, Pietro M, Billur A, Theall BP, et al. Second signals rescue b cells from activation-induced mitochondrial dysfunction and death. Nat Immunol (2018) 19:871–84. doi: 10.1038/s41590-018-0156-5
83. Jellusova J, Cato MH, Apgar JR, Ramezani-Rad P, Leung CR, Chen C, et al. Gsk3 is a metabolic checkpoint regulator in b cells. Nat Immunol (2017) 18(3):303–12. doi: 10.1038/ni.3664
84. Li L, Feng C, Qin J, Li D, Zheng B. Regulation of humoral immune response by HIF-1α-dependent metabolic reprogramming of the germinal center reaction. Cell Immunol (2021) 367(5):104409. doi: 10.1016/j.cellimm.2021.104409
85. Weisel FJ, Mullett SJ, Elsner RA, Menk AV, Shlomchik MJ. Germinal center b cells selectively oxidize fatty acids for energy while conducting minimal glycolysis. Nat Immunol (2020) 21(3):331–42. doi: 10.1038/s41590-020-0598-4
86. Lam W, Becker A, Kennerly K, Wong R, Curtis J, Llufrio E, et al. Mitochondrial pyruvate import promotes long-term survival of antibody-secreting plasma cells. Immunity (2016) 5(1)60–73. doi: 10.1016/j.immuni.2016.06.011
87. Cyster JG, Allen C. B cell responses: cell interaction dynamics and decisions. Cell (2019) 177(3):524–40. doi: 10.1016/j.cell.2019.03.016
88. Piovan E, Tosello V, Indraccolo S, Masiero M, Persano L, Esposito G, et al. Differential regulation of hypoxia-induced cxcr4 triggering during b-cell development and lymphomagenesis. Cancer Res (2007) 67(18):8605–14. doi: 10.1158/0008-5472.CAN-06-4722
89. Schmidt TH, Bannard O, Gray EE, Cyster JG. CXCR4 promotes b cell egress from peyer’s patches. J Exp Med (2013) 210:1099–107. doi: 10.1084/jem.20122574
90. Beck TC, Gomes AC, Cyster JG, Pereira JP. CXCR4 and a cell-extrinsic mechanism control immature b lymphocyte egress from bone marrow. J Exp Med (2014) 211(13):2567–81. doi: 10.1084/jem.20140457
91. Opezzo P, Navarrete M, Chiorazzi N. AID in chronic lymphocytic leukemia: induction and action during disease progression. Front Oncol (2021) 11:753. doi: 10.3389/fonc.2021.634383
92. Hackney JA, Misaghi S, Senger K, Garris C, Sun Y, Lorenzo MN, et al. DNA Targets of AID evolutionary link between antibody somatic hypermutation and class switch recombination. Adv Immunol (2009) 101:163–89. doi: 10.1016/S0065-2776(08)01005-5
93. Scanlon SE, Glazer PM. Multifaceted control of DNA repair pathways by the hypoxic tumor microenvironment. DNA Repair (2015) 32:180–9. doi: 10.1016/j.dnarep.2015.04.030
94. Chi X, Li Y, Qiu X. V(D)J recombination, somatic hypermutation and class switch recombination of immunoglobulins: mechanism and regulation. Immunology (2020) 160(3):233–47. doi: 10.1111/imm.13176
95. Bing Z, Xi Z, Rong L, Yin W, Sui Z, Ren B, et al. The function of micrornas in b-cell development, lymphoma, and their potential in clinical practice. Front Immunol (2018) 9:936. doi: 10.3389/fimmu.2018.00936
96. Mette D, Sommer KL, Kirsten G. Long non-coding rnas guide the fine-tuning of gene regulation in b-cell development and malignancy. Int J Mol Sci (2019) 19:2475. doi: 10.3390/ijms19092475
97. Ji TT, Chen QN, Tao SD, Shi YY, Chen Y, Shen L, et al. The research progress of circular RNAs in hematological malignancies. Hematology (2019) 24(1):727–31. doi: 10.1080/16078454.2019.1669924
98. Kersy O, Salmon-Divon M, Shpilberg O, Hershkovitz-Rokah O. Non-coding rnas in normal b-cell development and in mantle cell lymphoma: from molecular mechanism to biomarker and therapeutic agent potential. Int J Mol Sci (2021) 22(17):9490. doi: 10.3390/ijms22179490
99. De Yebenes VG, Belver L, Pisano DG, Gonzalez S, Villasante A, Croce C, et al. miR-181b negatively regulates activation-induced cytidine deaminase in b cells. J Exp Med (2008) 205(10):2199–206. doi: 10.1084/jem.20080579
100. Vigorito E, Perks KL, Abreu-Goodger C, Bunting S, Xiang Z, Kohlhaas S, et al. microRNA-155 regulates the generation of immunoglobulin class-switched plasma cells. Immunity (2008) 27(6):847–59. doi: 10.1016/j.immuni.2007.10.009
101. Mok Y, Schwierzeck V, Thomas DC, Vigorito E, Rayner TF, Jarvis LB, et al. MiR-210 is induced by oct-2, regulates b cells, and inhibits autoantibody production. J Immunol (2013) 191(6):3037–48. doi: 10.4049/jimmunol.1301289
102. Christoph J, Cecile K. Cytokines in the germinal center niche. Antibodies (2016) 5(1):5. doi: 10.3390/antib5010005
103. Zhang F, Li R, Yang Y, Shi C, Shen Y, Lu C, et al. Specific decrease in b-cell-derived extracellular vesicles enhances post-chemotherapeutic cd8+ t cell responses. Immunity (2019) 50:738–50. doi: 10.1016/j.immuni.2019.01.010
104. Colgan SP, Campbell EL, Kominsky DJ. Hypoxia and mucosal inflammation. Ann Rev Pathol Mech (2016) 11(1):77–100. doi: 10.1146/annurev-pathol-012615-044231
105. Kotagiri P, Mescia F, Hanson A, Turner L, Smith K. The impact of hypoxia on b cells in COVID-19. EBioMedicine (2021) 77:103878. doi: 10.1016/j.ebiom.2022.103878
106. Serebrovska ZO, Chong EY, Serebrovska TV, Tumanovska LV, Xi L. Hypoxia, HIF-1α, and COVID-19: from pathogenic factors to potential therapeutic targets. Acta Pharmacol Sin (2020) 41(12):1539–46. doi: 10.1038/s41401-020-00554-8
Keywords: hypoxia, hypoxia-inducible factor signaling, B cell biology, development, metabolism, function
Citation: Zhang J, Wu X, Ma J, Long K, Sun J, Li M and Ge L (2022) Hypoxia and hypoxia-inducible factor signals regulate the development, metabolism, and function of B cells. Front. Immunol. 13:967576. doi: 10.3389/fimmu.2022.967576
Received: 13 June 2022; Accepted: 26 July 2022;
Published: 15 August 2022.
Edited by:
Hong Zan, The University of Texas Health Science Center at San Antonio, United StatesReviewed by:
Fredericus Van Eeden, The University of Sheffield, United KingdomPhilippe Auguste Robert, University of Oslo, Norway
Copyright © 2022 Zhang, Wu, Ma, Long, Sun, Li and Ge. This is an open-access article distributed under the terms of the Creative Commons Attribution License (CC BY). The use, distribution or reproduction in other forums is permitted, provided the original author(s) and the copyright owner(s) are credited and that the original publication in this journal is cited, in accordance with accepted academic practice. No use, distribution or reproduction is permitted which does not comply with these terms.
*Correspondence: Liangpeng Ge, Z2VsaWFuZ3BlbmcxOTgyQDE2My5jb20=
†These authors have contributed equally to this work