- 1School of Biotechnology, Jawaharlal Nehru University, New Delhi, India
- 2School of Life Sciences, Jawaharlal Nehru University, New Delhi, India
- 3Integrative Biosciences Center, Department of Ophthalmology, Visual and Anatomical Sciences, Wayne State University, School of Medicine, Detroit, MI, United States
- 4Microbiology Division, Defence Research and Development Establishment, Gwalior, India
- 5Glaxo Smith Kline (GSK) Vaccine, Siena, Italy
In the present scenario, immunization is of utmost importance as it keeps us safe and protects us from infectious agents. Despite the great success in the field of vaccinology, there is a need to not only develop safe and ideal vaccines to fight deadly infections but also improve the quality of existing vaccines in terms of partial or inconsistent protection. Generally, subunit vaccines are known to be safe in nature, but they are mostly found to be incapable of generating the optimum immune response. Hence, there is a great possibility of improving the potential of a vaccine in formulation with novel adjuvants, which can effectively impart superior immunity. The vaccine(s) in formulation with novel adjuvants may also be helpful in fighting pathogens of high antigenic diversity. However, due to the limitations of safety and toxicity, very few human-compatible adjuvants have been approved. In this review, we mainly focus on the need for new and improved vaccines; the definition of and the need for adjuvants; the characteristics and mechanisms of human-compatible adjuvants; the current status of vaccine adjuvants, mucosal vaccine adjuvants, and adjuvants in clinical development; and future directions.
Introduction
The invention of vaccines has been considered as one of the triumphs of medical research. Immunization not only stops the spread of infection during childhood but also provides a lifetime of protection against some diseases. However, the scientific community continues to face challenges in developing ideal vaccines against many infectious diseases, i.e., plague, tuberculosis, malaria, human immunodeficiency virus (HIV), and severe acute respiratory syndrome coronavirus 2 (SARS-CoV-2), due to immunological barriers such as inadequate immune response and weak immunological memory against vaccines (1–3). Apart from these obstacles, vaccine safety issues such as adverse effects in a population suffering from rare genetic disorders, systemic and local reactogenicity caused by diphtheria and tetanus toxoids along with whole cell pertussis (DTwP), and waning immunity shown by diphtheria and tetanus toxoids along with acellular pertussis (DTaP) (4) have been considered unacceptable, which further increases the impact of the challenge to solve the vaccine problem for emerging or reemerging disease threats. These challenges warrant new strategies that can help to understand immune responses for immunization and introduce new ways to induce robust immunity without sacrificing quality and safety (5).
The worldwide scientific community has recently witnessed significant disease outbreaks, i.e., SARS in 2003, the H1N1 influenza pandemic of 2009, Ebola virus in 2014 (6), the plague in Madagascar in 2017 (7), the Nipah outbreak in India in 2018 (8), and, the most notable thus far, the ongoing COVID-19 pandemic (9). In 2014, the Ebola epidemic created huge panic in developed countries as the mortality rate was found to be quite high in West African countries (10). These incidences force the scientific community not only to be alert but also to open up new avenues in pursuing new strategies to elucidate the mechanistic approach to develop an effective vaccine against these emerging pathogens (11). At present, the ongoing COVID-19 pandemic has greatly affected human lives worldwide and devastated the global economy; therefore, the scientific community at large is busy developing an effective and safe vaccine against SARS-CoV-2 (12).
Currently, inactivated, live-attenuated, subunit, and nucleic acid-based vaccines are the four types of vaccines available for the human population (13, 14). Live-attenuated vaccines comprise the whole pathogen that can replicate in the host body and induce strong immune responses. Live-attenuated vaccines have been observed to be the most effective against polio, Measles, Mumps and Rubella (MMR), chicken pox, influenza, rotavirus, and yellow fever. Killed whole-pathogen vaccines are inactivated by heat or chemicals [inactivated polio (Salk) and hepatitis A], are noninfectious, and are mostly safe. Inactivated (killed) vaccines have been observed to induce weak and short-term immunity, thus the need for boosters to achieve complete protection (15). It has been found that DTwP from India, which is a kind of licensed inactivated vaccine, bypasses waning immunity and shows long-term protective efficacy (4, 16).
Similar to inactivated whole-pathogen vaccines, purified or recombinant subunit vaccines do not contain live components of the pathogen, but they consist only of the antigenic parts of the pathogen, which makes them different from the former. Subunit vaccine antigens have been poorly immunogenic, hence the need to add components to enhance their protective immunity. Subunit vaccines sometimes use epitopes that are shown to identify and interact with T cells or immunoglobulins. Subunit vaccines have been generally documented safe in terms of toxicity and reactogenicity, as subunit vaccines comprise purified or recombinant antigens rather than the whole cell (17). There are a few very successful examples of subunit vaccines, such as hepatitis B virus (HBV), influenza virus (injection), and pertussis vaccines. The developed subunit vaccines have been found to be poorly immunogenic, and thus, multiple boosters and suitable adjuvantation are necessary to augment their protective potential. Recently, mRNA vaccines (nucleic acid-based vaccines) have been found to play an important role during the COVID-19 pandemic. These mRNA-based vaccines provide good immune response by directing the production of antibodies, thus preventing serious complications (18).
The need for adjuvants
Adjuvants were first discovered in 1920 by Gaston Ramon, a French scientist who observed in his findings that the inclusion of aluminum salts to vaccines enhanced their potential. The term “adjuvant” originated from the Latin word adjuvare, which means “to help.” Adjuvants are generally not immunogenic, but they modulate the immune responses in formulation with the given vaccines, thus not only reducing the required dose of vaccine but also extending immune memory. Typically, the vaccines are formulated with suitable adjuvants to augment the immune responses to the administered vaccine antigen to evaluate the potential to halt the contagion. Another important role of adjuvants is to direct humoral and cell-mediated immune responses to generate pathogen-specific immunity (19–22).
At present, adjuvants are exploited (a) to augment the immune response to the given vaccine and enhance the antibody response and the number of recipients that were vaccinated; (b) to enhance rates of seroconversion in individuals with diminished responsiveness due to age, illness, or therapeutic interventions, e.g., the use of the adjuvant MF59 with the influenza vaccine to improve/increase the response in aged individuals (23, 24); and (c) to reduce the dose and the number of boosters of vaccine antigens (25–27) as the ability of an adjuvant to allow comparable responses with considerably lower amounts of vaccine antigen might be crucial in regions where vaccine production facilities are limited and immunization is urgent in public in general. The demand for vaccines with multiple boosters poses noteworthy challenges worldwide. Adjuvants can decrease the number of required boosters to provide complete protection (26–28) (Figure 1).
Another reason for formulating a vaccine with an adjuvant is to attain qualitative modulation of the immune response. Adjuvants are used to a greater extent for underdeveloped vaccines to modulate types of immune responses that are not effectively stimulated by vaccine antigens without adjuvants. Adjuvants are used in preclinical and clinical studies (i) to provide functionally suitable immune responses (e.g., humoral or cellular, Th1, Th2, and Th17); furthermore, it has been observed that balanced Th1/Th2/Th17 responses increase the duration of T-cell responses and prolong mouse survival (29–33); (ii) to enhance long-term memory cells (e.g., T-cell memory) (34–36); (iii) to provide the initial rapid response that can be essential in a pandemic (37–39); and (iv) to modify the breadth, specificity, or affinity of the response (39, 40) (Figure 1). In this review, our main objective is to focus on the modulation of the immune response using various adjuvants.
Characteristics and mechanisms of action
Aluminum salts (alum)
Aluminum salts have been clinically approved, and they are the most widely used adjuvants in human vaccines. These adjuvants are made up of the precipitates of aluminum phosphate and aluminum hydroxide for the adsorption of vaccine antigens. Brenntag Biosector, Chemtrade, and SPI Pharma™ are some of the manufacturers that prepare these formulations. Alhydrogel®, Rehydragel™, and Adju-Phos® are tradenames of alum that are available in the market (41). Aluminum salts as adjuvants have been utilized for more than 80 years in vaccine research and usually stimulate the Th2 type of immune response (42, 43). Alum has been approved as a component of licensed human vaccines, i.e., Haemophilus influenzae type b (Hib), both hepatitis A and B viruses, tetanus, meningococcal virus, human papilloma virus (HPV), diphtheria, and the most recent SARS-CoV-2 (11, 41, 44–47). The mechanism of action of alum is almost known, and it is now clear that depot formation is not an essential step for the activity of alum as an adjuvant (48–50). Alum as an adjuvant primarily evokes innate immunity (50–52). Alum stimulates B-cell differentiation to augment antibody production (53). It is also well documented that alum stimulates the Th2 type of immune response in mice, but in humans, almost all the vaccine antigens in formulation with the alum adjuvant induce a mixed type of response, i.e., Th1 and Th2 (54, 55). Alum triggers the NLRP3 inflammasome to express interleukin (IL)-1β after in vitro priming of macrophages and dendritic cells (DCs) with lipopolysaccharide (LPS) (56). However, in vivo, the adjuvanticity of alum does not support the data (50, 51). It has been observed that alum stimulates the Th2 type of immune response and produces IL-4, IL-5, IgG1, and IgE (43, 57). Another functional activity performed by alum is the initiation of a signaling cascade by using DCs to carry out actin-mediated phagocytosis that leads to the activation of two kinase proteins (Src and Syk), which, in turn, mobilizes Ca2+ and finally activates the transcription factor NFAT (calcineurin-nuclear factor of activated T cells), resulting in the production of IL-2 (33, 58–60). In addition, alum mediates its adjuvanticity by activating the cascade of complement proteins (41, 61–63). Alum as an adjuvant is highly advantageous due to its safety, vaccine antigen stabilization, and the modulation of high-production and long-lasting antibody titers. Vaccines that have been formulated with an alum adjuvant cannot be filter sterilized, lyophilized, or frozen (64).
Adjuvants in emulsion forms (oil-in-water)
MF59 and AS03 adjuvants
MF59 is a highly safe and effective oil-in-water emulsion of squalene oil. Unlike Freund’s adjuvants, squalene is more readily metabolized and highly purified for vaccine development. Recently, MF59 has been licensed by Fluad™ (Seqirus, Melbourne, Australia) as an important component of flu vaccine for old people, and it has also been successfully used in infants and children later on (65, 66). Apart from this, it was also licensed to be used as a pandemic vaccine against H1N1 in children, infants, and pregnant women (67). It is evident from the findings that the MF59 adjuvant trivalent inactivated influenza vaccine (TIV) elicited a strong humoral and cellular immune response in infants in comparison to non-MF59 adjuvanted influenza vaccines (68, 69). The formulation of MF59 significantly modulated the weak efficacy of the influenza vaccine in infants. Subsequently, the HBV vaccine in formulation with the MF59 adjuvant was observed to induce a 100 times stronger immune response in comparison to alum (70). Similar to other adjuvants, the mechanism of action of MF59 is not yet fully elucidated. The effectiveness of the MF59 adjuvant does not depend on the formation of a depot at the site of vaccination because of the short half-life of MF59 (49, 71). However, the MF59 adjuvant has shown the potential to elicit a strong IgG and cell-mediated immune response (72). Moreover, MF59 can induce monocytes, macrophages, and DCs to express and secrete chemokines, i.e., CCL4, CCL2, CCL5, and CXCL8, which recruit more leukocytes for the reuptake of more vaccine antigens. Finally, this differentiation converts immune cells into antigen-presenting cells (APCs). Furthermore, these APCs migrate to lymph nodes where they induce an adaptive immune response as shown in Figure 2 (67, 73, 74).
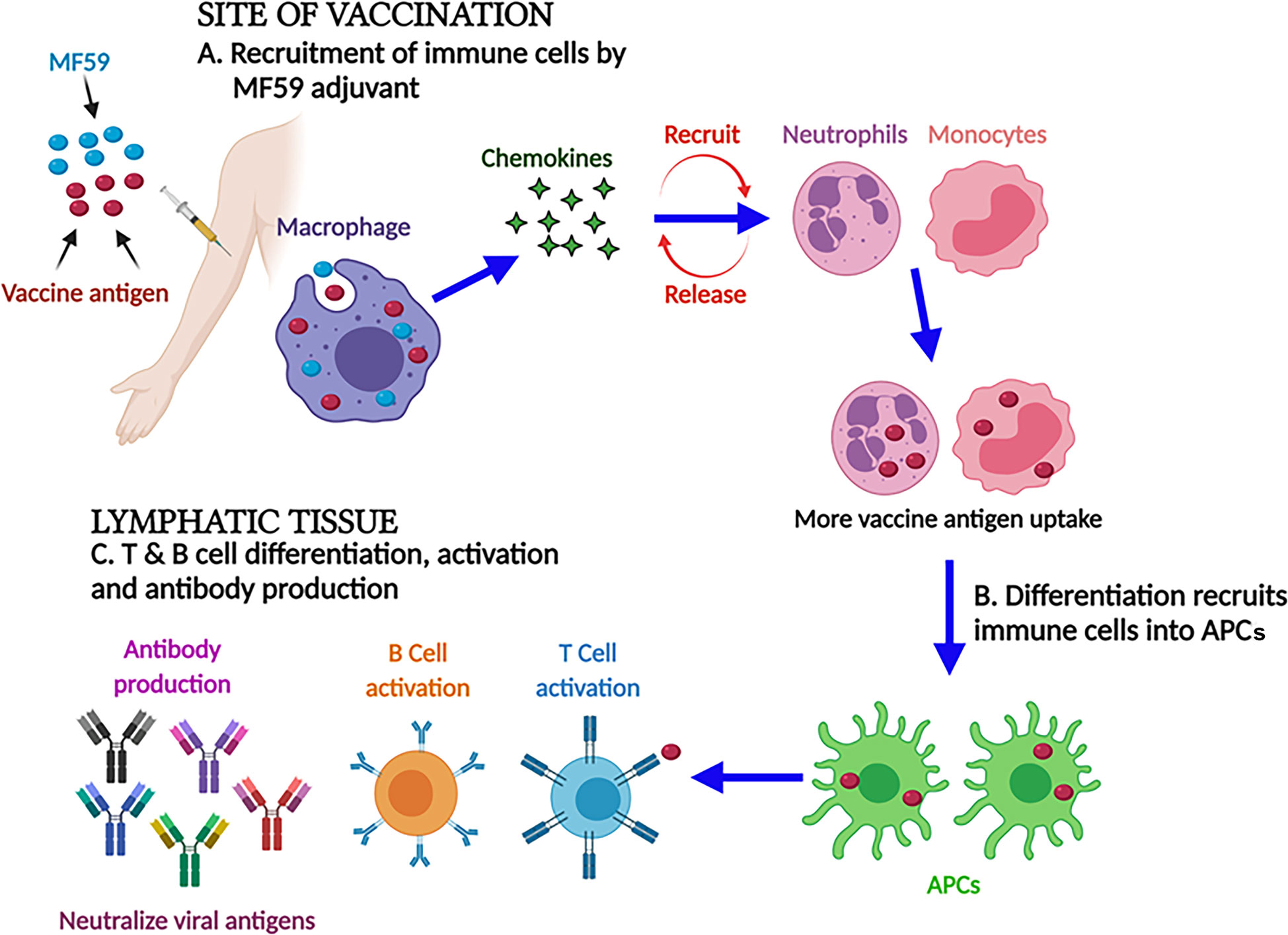
Figure 2 MF59 adjuvant and its mechanism of action. At the injection site, MF59 adjuvant-activated macrophages secrete the chemokines that stimulate and recruit the immune cells. Differentiation converts immune cells into antigen-presenting cells to activate B and T cells to impart strong humoral and cellular immune responses.
AS03 is also an oil-in-water emulsion adjuvant that includes squalene, α-tocopherol, and polysorbate 80 (75). The inclusion of α-tocopherol into the AS03 formulation made it different from other oil-in-water emulsion adjuvants (76). AS03 was first used in formulation with a vaccine against malaria (77). Recently, this adjuvant has been used for human vaccination against influenza. Recent clinical trials have shown that the AS03 adjuvant with the influenza vaccine elicited a strong immune response (78). In addition, the AS03 adjuvant vaccine was found to induce a robust immune response in infants as well (79). Furthermore, AS03 evokes immunity by stimulating Nuclear factor kappa B (NF-κB), a proinflammatory cytokine, and chemokines. Later, it recruits immune cells such as monocytes and macrophages. The advantage of applying this adjuvant in formulation with the pandemic vaccine is its capability of generating a strong humoral immune response with a lower dose of vaccine antigen, i.e., 3.75 or 7.5 μg per strain in comparison to 15 µg per strain in conventional trivalent inactivated influenza vaccines. Since 2009, ~4.7 million doses of AS03-adjuvanted A(H1N1) vaccines have been injected in children (80, 81).
Army liposome formulation
This formulation was developed by the U.S. military, made up of cholesterol and liposomes containing saturated phospholipids and monophosphoryl lipid A (MPLA) (82). There are improved versions of army liposome formulation (ALF), i.e., ALF adsorbed to aluminum hydroxide (ALFA), ALF containing the QS21 saponin (ALFQ), and ALFQ adsorbed to aluminum hydroxide (ALFQA) (83, 84). It has been found that the vaccine candidate circumsporozite protein of Plasmodium falciparum adjuvanted with ALFA imparted adequate humoral and cellular immune responses (85). The WRAIR (Walter Reed Army Institute of Research) malaria vaccine branch developed and tested the protective efficacy of FMP013 (falciparum malaria protein-013) and FMP014 (a self-assembling protein nanoparticle) SAPN (two new synthetic malarial antigens) with an ALFQ adjuvant (86–88).
Recombinant gp120 adjuvanted with ALFA induced cross-reactive antibodies against different subtypes of HIV-1 (89). As regards ALF and ALFQ in formulation with the recombinant HIV-1 envelope gp140 protein, ALF induces a dominant Th2 type of immune response, while ALFQ induces a more balanced Th1 and Th2 type of immunity (90, 91). In addition, the ALFQ adjuvant activates innate immune responses, upregulates APOBEC3 (apolipoprotein B mRNA-editing enzyme catalytic polypeptide-like family), an anti-HIV protein, and maintains a proinflammatory environment, as a result of which, MDMs (monocyte-derived macrophages) that are permissive to HIV-1 infection become capable of restricting HIV-1 infection (92).
Virosomes (lipids and glycoproteins)
Virosomes display the attributes of an adjuvant system and are known for their biodegradable and nontoxic qualities. Generally, virosomes do not generate anti-virosome antibodies (93). Virosomes are small spherical unilamellar lipid membrane vesicles (150 nm) embedded with viral envelope proteins, such as neuraminidase and hemagglutinin of the influenza virus. These proteins are integrated into phosphatidylcholine bilayer liposomes. These prepared virosomes are devoid of nucleocapsid including the genetic material of the source virus (94). These proteins facilitate virosome membranes to bind with cell receptors, mediating pH-dependent fusion with immune cells. Consequently, virosomes transport their contents, i.e., vaccine antigen(s), directly to their target cells, evoking an antigen-specific immune response yet carrying a weak immunogenic antigen (95). Virosomes are virus-like particles that allow the presentation of vaccine antigen to both major histocompatibility complex (MHC) class I and class II to elicit both B-cell and T-cell immune responses (95–99).
The types of immune response evoked by the virosome adjuvant system depend on whether the antigenic epitopes are outside or inside the virosome. There are few established examples such as PeviPRO™, which induces a humoral immune response (27). The antigen is degraded in endosomes of the cell and, hence, mainly generates an MHC II antigen presentation. PeviTER™ formulated antigens not only elicit a CD4+ and CD8+ T-cell immune response but also generate a strong cytotoxic T lymphocyte (CTL) response. Virosome adjuvant system encapsulation presents vaccine antigens via the MHC I route because the antigen is delivered naturally into the APC cytosol (95). The approved vaccines such as Epaxal® (for hepatitis A) and Inflexal®V (for influenza) (100) have successfully proven the excellence of virosomes. Thus far, these hepatitis A (Epaxal®) and influenza (Inflexal®V) vaccines have been authorized to be used in more than 45 countries, and more than 10 million people have been immunized to date. This new generation of vaccines offers additional benefits because they are effective even in immunosuppressed patients and in infants (101). Furthermore, they have a high safety profile as embedded viruses do not replicate. To the best of our knowledge, virosomes are no longer licensed to be used in humans.
AS04 (alum-adsorbed TLR-4 agonist)
To develop the novel adjuvant systems, aluminum salts have been used, mainly consisting of different Toll-like receptor (TLR) agonists absorbed on alum. Adjuvant system 04 (AS04) has already been approved for use in formulation with HPV (Cervarix) and HBV (Fendrix) vaccines. This adjuvant system includes alum in formulation with LPS that mainly constitutes 3-O-desacyl-4′-monophosphoryl lipid A (MPLA) from Salmonella minnesota. It is documented that MPLA retains the ability to stimulate innate immunity by interaction with TLR-4 (54). It further leads to induce NF-κB signaling and produce pro-inflammatory cytokines and chemokines that recruit the immune cells at the vaccination site and draining lymph nodes. An increase in the number of monocytes and DCs has been observed within a few hours of vaccination where they interact to stimulate antigen-specific T and B cells for strong cellular and humoral immune responses. There is no synergistic effect shown by alum with MPLA; however, a comparative study of MPLA and AS04 revealed that alum extends the cellular responses evoked by MPLA at the vaccination site. Hence, research findings suggest that the AS04 adjuvant induces innate immune responses by stimulating TLR-4 (54). The AS04-adjuvanted HBV vaccine elicits innate immune responses in humans (102). An elevated level of IL-6 and C-reactive protein was observed in the AS04-adjuvanted HBV immunized serum in comparison to the alum-adjuvanted HBV immunized serum. Yet, higher HBs Ag-specific T cells and antibodies were reported than those induced by the HBV vaccine in formulation with alum (103). Both HBV and HPV vaccines in formulation with AS04 elicit stronger humoral immune responses in comparison to the same vaccines when formulated with the alum adjuvant, signifying the importance of the TLR4 agonist MPLA adjuvant for human use (103–105).
RC-529 adjuvant
Sequential acid and base hydrolysis of LPS generates an MPLA that is known to retain various immunostimulatory functions of LPS. In vitro studies have shown that MPLA activates and stimulates the maturation of DCs and upregulates the human leukocyte antigen‐DR, CD80, CD86, CD40, and CD83 (106). MPLA is also known to induce the expression of Th1 and Th2 cytokines (106, 107) and augment the antigen-specific Tc cell response (108, 109). MPLA is an approved adjuvant and has been used in hepatitis B vaccine formulations. After this, synthetic mimetics such as aminoalkylglucosaminide 4-phosphates (AGPs) were characterized, and it was observed that they activate innate cells such as macrophages, DCs, B cells, and APCs. One such AGP compound, RC-529, was observed to activate the signal via TLR-4 and upregulate the costimulatory molecules on the cell surface including cytokines and chemokines (107). RC-529 was found to be an effective adjuvant in a clinical trial in which healthy volunteers were administered with a vaccine formulation against hepatitis B. In comparison to alum, RC-529 induced a significantly high production of antibodies in subjects who were administered with the vaccine formulation (108, 110). MPLA’s synthetic mimetic RC-529 was observed as safe and effective in clinical trials (111).
Mucosal vaccine adjuvants
These adjuvants evoke the innate immune system of the host and help to provide protection against pathogens. Whole-cell-based vaccines using live-attenuated or killed pathogens usually consist of endogenous adjuvants, such as the products of the bacterial cell wall and their genomic DNA/RNA. These adjuvants act as pathogen-associated molecular patterns (PAMPs) and are adequate to stimulate adaptive immunity. However, subunit vaccines usually miss, or are unable to induce, an innate immune response, and thus, the inclusion of an adjuvant is essential to deliver successful vaccines (112). Most of the pathogens enter the host via either the intranasal or the oral route. Therefore, an effective mucosal vaccine is of utmost importance to prevent such kinds of mucosal transmitted diseases. The mucosal vaccine, in comparison to the intramuscular/subunit vaccine, provides noteworthy benefits such as low cost, noninvasiveness, and, most importantly, very low risk of transmission of blood-borne infections, especially to young children. However, due to the lack of ideal mucosal adjuvants, only a few mucosal vaccines are approved for human use (113).
Generally, mucosal adjuvants have two roles: first, they act as delivery vehicles, and second, they act as immunostimulatory molecules. However, some mucosal adjuvants exhibit the characteristics of both immunostimulators and delivery systems, such as chitosan and its derivatives (114). It is documented that mucosal adjuvants can stimulate protective local and systemic immunity that is crucial for successful mucosal immunizations against various infectious diseases (115–118). In addition, mucosal adjuvants perform many vital roles to provide protection against infections at distant as well as local sites. For example, Cytosine-phosphorothioate-guanine (CpG) oligodeoxynucleotides act as effective mucosal adjuvants for vaccinations via the nasal route against pathogens transmitted by blood transfusion and sexual activities (119, 120). Moreover, mucosal adjuvants are strong stimulators of immunity against tumors (121, 122). Among many mucosal adjuvants, the agonists of TLR and mutant enterotoxins are the two most appealing types because they are not only effective but also comparatively safe (115). So far, bacterially derived Adenosine diphosphate (ADP)-ribosylating enterotoxins are the most characterized mucosal adjuvants. This class of adjuvant comprises Escherichia coli heat-labile enterotoxin (LT), cholera toxin (CT), and mutants/subunits of LT and CT. These toxins stimulate an antigen-specific cellular and humoral immune response that includes CTLs, Th1, Th2, and Th17. Most importantly, these adjuvants stimulate antigen-specific IgA antibodies and long-lasting memory cells to vaccine antigens when administered via the mucosal route (123).
How do heat-labile toxin/double-mutant heat-labile toxin mucosal adjuvants work?
The mucosal adjuvant LT is a polymeric protein of 84 kDa. This adjuvant retains an active form as AB5 that contains an A subunit and a pentameric B subunit. dmLT is a mutated form of its parent molecule LT (124, 125). The adjuvant dmLT immunomodulates the systemic as well as mucosal immune responses specific to the vaccine antigen after vaccination via the mucosal or parental route. It can simply be formulated with vaccine antigen(s) in an aqueous buffer. Due to the dual approach of the adjuvant, i.e., immunostimulatory characteristics and universal cell binding, the cell uptake of vaccine antigen(s) are many-fold high, and that is how the mucosal immunity is enhanced. This is the most suitable approach to deliver vaccine formulations, particularly for subunit vaccines to unapproachable sites, and specifically for sublingual (s.l.), oral (p.o.), and transcutaneous (t.c.i.) delivery. These strategies not only are needle-free but also reveal the capacity to enhance ease of administration and compliance. Moreover, it reduces the risk of transmission of the diseases from vaccinations using risky injections (126–129). The dmLT adjuvant evokes a strong Th17 response specific to the vaccine antigen and induces IL-17, and it provides protection against infections mainly in mucosal sites (130). The expression of IL-17 helps to increase the transport of secretory sIgA antibodies into the lumen of mucosal tissue by inducing B-cell differentiation into IgA-secreting cells (131–134). The dmLT adjuvant also stimulates the mucosal immune responses after parenteral vaccination (135–139). However, these studies have been tested only in animal models, and the use of this adjuvant in humans is yet to be determined.
LT, CT, and their related mutants have been very well characterized in the recent past (139–141). The subunits of the active form AB5 of LT or CT adjuvant function uniquely. Subunit B binds to the receptor and leads the entry into the cell. During mucosal vaccination, the B subunit helps in transporting the vaccine antigens throughout the mucosal sites (142). Subunit A is responsible for binding to ADP-ribosylation factors (ARFs), and ADP ribosylates Gsα, which results in an accumulation of Cyclic adenosine monophosphate (cAMP). Then, LT stimulates the activation of DCs, the expression of cytokines, and the stimulation of Th17 response (142, 143). It is also documented that the use of subunit A of LT alone evokes a mixed Th1/Th2/Th17 type of immune response but has a weaker impact than the active AB5 form of LT. The use of subunit B evokes a Th2/T regulatory cell (Treg)-skewed response (144). However, toxicity has always been a concern for these adjuvants.
How does the dmLT adjuvant induce the immune system? Figure 3 depicts the immunologic cascade. (I) At the vaccination site, after the uptake of the vaccine antigen, the activation of the innate immune system takes place. The epithelial cells secrete cytokines and chemokines such as IL-8 and granulocyte colony-stimulating factor (G-CSF). (II) The DCs are recruited to the vaccination site and activated for antigen processing and presentation to the major histocompatibility complex (Ag-MHC). The upregulation of CD80 and CD86 and the secretion of polarizing cytokines, e.g., IL-1, IL-23, IL-6, and G-CSF, take place. (III) The activated DCs carrying the vaccine antigen then migrate to the secondary lymphoid organs and facilitate the differentiation of antigen-specific T helper and B cells into plasma cells secreting IgA and IgG antibodies. Finally, a mixed Th1/Th2/Th17 type of immune response is imparted with specifically strong stimulation of Th17 cells (124, 145–147) and mucosal homing markers (135). It has been documented that Th17 cells are an essential part of immunity for promoting germinal center formation in secondary lymphoid organs and for augmenting IgA antibody secretion (132–134).
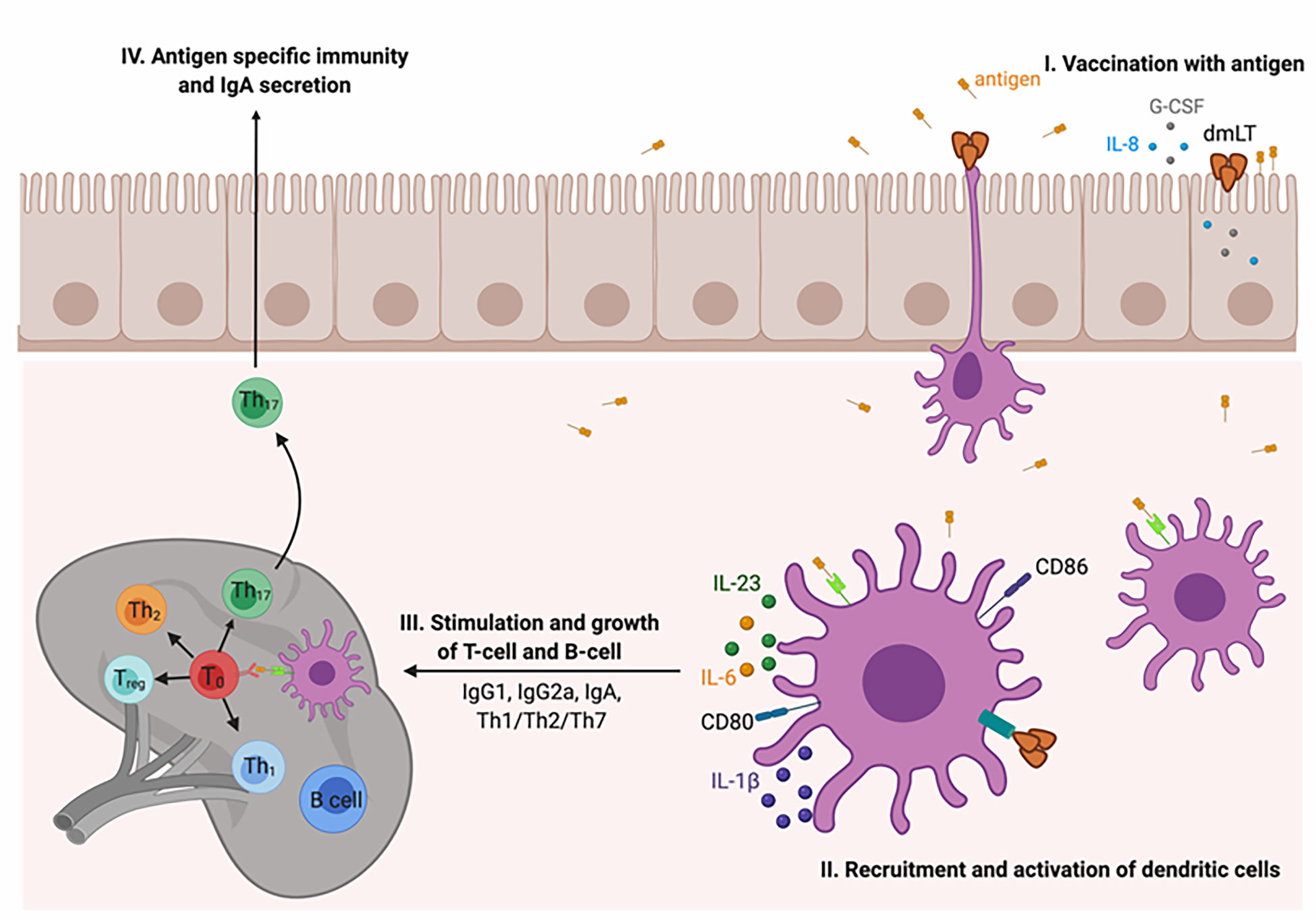
Figure 3 Mechanism of action of the dmLT adjuvant. (I) At the vaccination site, the dmLT adjuvant stimulates the innate immune response and activated epithelial cells secrete IL-8 and G-CSF. (II) Dendritic cells (DCs) are activated and recruited at the injection site where they process and present the antigen. It also upregulates the costimulatory molecules, i.e., CD80 and CD86, and stimulates the expression of IL-1, IL-6, IL-23, and G-CSF. (III) The activated DCs migrate to secondary lymphoid organs where they stimulate the differentiation of antibody-secreting plasma B cells and the generation of antigen-specific Th cells. Overall, a strong Th17 immune response is imparted. Th17 cells are identified as essential in immunity and in stimulating germinal center formation in secondary lymphoid organs as well as in enhancing IgA secretion.
The stimulated DCs by an LT/dmLT adjuvant induced a very high Th17 type of immune response due to the activation of caspase 1 inflammasome and, later, the expression of chemokines and cytokines such as IL-1 and IL-23. A scientific study reported that murine DCs activated with LT induced the expression of IL-1β, which is essential for the production of Th17 cells (148). Re-stimulation of human Peripheral blood mononuclear cells (PBMC) of immunized individuals with vaccine antigens in formulation with dmLT augmented the expression of IL-17A and IL-13 (146). In summary, a defined series of events takes place after immunization with a vaccine antigen in formulation with dmLT, which is an effective stimulator of APCs and vaccine immunity (123).
TLR agonists as a mucosal vaccine adjuvant
Vaccines in formulation with TLR ligand-based adjuvants trigger and activate an innate immune response that helps in the augmentation of a protective potential imparted by the vaccine candidate. TLR-based adjuvants require an additional adaptor protein, MyD88, which stimulates APCs and B cells for antibody production. In addition, MyD88 signaling also induces germinal center formation that is imperative to produce antibody-secreting cells (149). TLR ligand-based adjuvants include the TLR9-dependent adjuvant CpG DNA (CpG) and the TLR2-dependent adjuvant Porin B (PorB), both of which require MyD88 for proper stimulation of APCs. CpG is an unmethylated bacterial DNA motif while PorB is an outer-membrane protein of Neisseria meningitides. It is documented that TLRs are expressed on APCs, including macrophages, DCs, and B cells. Therefore, the main purpose of using a TLR agonist in vaccine preparation as an adjuvant is to stimulate these cells to impart a robust immune response, linking innate and adaptive immunity (149). Various ligands for TLR4 have been not only used in preclinical studies but also approved by the Food and Drug Administration for clinical trials like Bacillus Calmette–Guerin (BCG) and MPLA. LPS, a well-known ligand for TLR4, was used as an inducer of acute inflammation in vivo for tumor regression in a mouse model (150). It has been reported that the TLR4 agonist, a second-generation lipid adjuvant like SLA-SE, promotes an increment in mucosal antibodies elicited by intramuscular immunization with an enterotoxigenic E. coli (ETEC) vaccine antigen in a mouse model (151). To test the efficacy of L-pampo, a combination of TLR1/2 and TLR3 agonists for the SARS-CoV-2 ferret model was utilized, which elicited a vigorous humoral and cell-mediated immune response (152). More recently, an orally administered SARS-CoV-2 vaccine (VXA-CoV2-1), which is currently in Phase III clinical trial, used the double-stranded RNA adjuvant, which basically targets TLR3 and helps in the activation of the DC population owing to this receptor. The adjuvant used in this study not only broadens the pattern recognition receptor (PRR) target range but also is a promising alternative to toxoid-based adjuvants for oral vaccination (153). In our opinion, this advancement in adjuvant discovery for mucosal immunity can offer a new hope for combating pathogenic strains and can achieve a successful vaccine design.
The current status of adjuvants and clinical trials
Safety has always been a great concern in the process of developing novel vaccine adjuvants; therefore, many adjuvants have been comprehensively evaluated in both preclinical and clinical studies. A very commonly used adjuvant, e.g., alum, has been approved in the United States. Alum-adjuvanted vaccines were approved more than 70 years ago. Influenza vaccine in formulation with the emulsion-based vaccine adjuvant MF59 was permitted in 1997 and marketed in Europe. In addition, an emulsion-based vaccine adjuvant such as AS03 was adjuvanted with the influenza vaccine and licensed in 2009. Virosomes, a liposomal adjuvant, were approved in 2000 as an essential part of hepatitis A and influenza vaccines. In addition to this, AS04, a combination adjuvant containing MPLA adsorbed to alum, has been approved in Europe and licensed in the United States. Adjuvants that are licensed for use in humans are listed in Table 1.
Despite offering many noteworthy benefits such as reduced cost, high yield, and better safety of highly purified vaccine antigens, most of these provide a weaker vaccine potential due to the lack of intrinsic immunostimulatory factors like various PAMPs. It is essential to understand how immune cell receptors such as TLRs are stimulated by PAMPs, i.e., LPS of Gram-negative bacteria, and how the adaptive immune response is coordinated and regulated by the innate immune response (154). It has been reported that particulate material stimulates innate immune signals (155). Thus, adjuvants were identified for stimulating the specific receptors on innate immune cells that can easily sense danger signals and cellular stress.
Unfortunately, due to the high reactogenicity and poor tolerance, the early developed adjuvants could not reach clinical development. Because of the characterizations of HIV and malaria vaccines, the rationale that a combination of different adjuvants is a better option to synergistically promote a broad range of immune responses, i.e., humoral and cellular, emerged (80, 156, 157). This combination of adjuvants involved a delivery system, i.e., liposomes, alum, or emulsions, as well as a natural immune stimulator immune potentiator such as bacterial MPLA, viral dsRNA, or the plant Quillaja saponins. The new-generation adjuvants are mostly known as immune stimulators or immune potentiators, which have been tested in humans and are under clinical trials listed in Table 2. These new-generation adjuvant systems were designed based on existing drug delivery models and established pharmaceutical principles (170). Thus, the delivery system performed is mainly on immune signal activation that could be localized and focused on the formulated vaccine antigen (54, 76, 171–174). The adjuvant system (AS) of GSK is the best example of this advancement to make an effective vaccine against malaria (80). How important is the delivery system for vaccine development? It was mentioned and emphasized in the human clinical trial of two alternative vaccines of GSK. Both vaccines contain the same immune potentiators, but they were formulated differently. The vaccine that contained the AS01 adjuvant system (a liposome formulation) provided better protection in comparison to the vaccine containing the same constituents in the AS02 adjuvant system (an emulsion formulation) (158, 168). Therefore, the vaccine candidate RTS,S was formulated with the adjuvant system AS01, and this example proved that the combination of two immunopotentiators is necessary to achieve the goal. The adjuvant system AS01 contains both MPLA and the saponin QS-21. Studies suggest that when these two immune potentiators (MPLA and QS-21) were formulated in liposomes, they work synergistically and evoke an innate immune response that further augments the adaptive immune response (158, 168, 174–176). The capacity of the AS01 adjuvant system to stimulate the robust T-cell immune response in humans for various vaccine antigens is elucidated by the synergistic effect imparted by this unique combination of immunopotentiators, e.g., MPLA and QS-21. These findings open the doors and provide new avenues for the development of potential adjuvant systems (177).
The rationale of most of the adjuvants as a candidate or as a licensed vaccine (54, 76, 171–174, 178–187) became clear from the data collected from clinical studies (69, 188, 189). Typically, these adjuvants are known to stimulate the innate immune response very quickly and promptly at the immunization site, draining the stimulated immune cells into the lymph nodes, which is essential to augment the pathogen-specific adaptive immunity. The stimulation of PAMP and danger signal pathways, i.e., TLR, caspase-1, and lysosomal destabilization-Syk/Card9, promotes not only the recruitment of effector cells, including T and B cells, but also the recruitment and activation of APCs. It has been proven from many studies by targeted depletion of the activated APCs that this step is very important to induce vaccine antigen-specific T cells effectively. The type of antigen-specific T-cell phenotypes that will be produced will depend on the nature of innate immune signal activation. The secretion of IL-18 by stimulated subcapsular macrophages may promote the development of IFN-γ-secreting CD4+ T cells (178, 190). Similarly, the secretion of IL-6 or IL-12 by stimulated APCs may also promote the production of a follicular-helper phenotype of T cells (TFH), which promotes the secretion of high-avidity IgG by B cells, for example, influenza immunization (191). The adjuvant system that contained TLR ligands can modulate the clonal arrangement and quality of the repertoire of antigen-specific T cells by stimulating the expansion of clones of T cells with better TCR affinity (40, 192).
Novavax, Inc. has developed an important adjuvant named matrix-M. It is based on a saponin compound derived from the bark of Quillaja saponaria (Soapbark tree). Currently, the matrix-M adjuvant is being used in formulation with the recombinant spike (S) protein against SARS-CoV-2. At present, this vaccine formulation (COVID-19 vaccine) is in Phase I clinical trial. The matrix-M adjuvant not only induces the production of high neutralizing antibody titers but also induces a robust T-cell immune response and imparts high protective efficacy against various strains of coronavirus (193–195). Matrix-M has also been used in other vaccine formulations such as malaria vaccine (R21/Matrix-M), which is currently in Phase IIb, and influenza vaccine, which reached Phase I (196, 197). It has been observed that saponins such as Q-21 possess adjuvant activity by inducing an OVA-specific CTL response and high antibody titer by activating caspase 1 in subcapsular sinus macrophages (SSMs) in the draining lymph node (178, 198, 199), by activating tyrosine-protein kinase SYK through destabilizing lysosome upon endocytosis (181). In addition, saponins such as matrix-M play a crucial role in the activation of the innate immune system, allow for dose-sparing in vaccine usage, and induce both humoral and cell-mediated immune responses. However, the molecular mechanism of this adjuvant remains to be elucidated. The mechanism of action of this adjuvant can be defined by using a system vaccinology approach (32).
At present, the mechanistic approach and the rationale are correlated with the effectiveness and the acceptability of adjuvanted vaccines in humans. This piece of information might be helpful in providing a protocol on how to assess and characterize new adjuvant systems for vis-to-vis comparisons in nonclinical models (200–204) and in the clinic as well (69, 103). A recent study has shown that five different adjuvanted vaccines only affect the quantities of CD4+ T cells specific to vaccine antigen but failed to modulate the range of phenotypes of these cells (103). Hence, it is not always true that adjuvants affect the quality of the immune response for all antigens. In general, vaccine effectiveness is also heavily reliant on the vaccine antigen itself and on what kind of immunity is needed for protection, for example, HIV vaccine studies (204–206).
Use of adjuvants during the COVID-19 pandemic and future perspective
To date, we have achieved many insights into a new generation of adjuvants in terms of their immunology, and these insights may be quite helpful in providing future avenues to develop novel adjuvanted vaccines by selecting the suitable combination of adjuvants and antigens (207–209). With the advent of omics and system biology, one can easily elucidate the complicated human immune response stimulated by the ideal vaccines. Through these studies, a unique collection of molecular signatures can be compared with newly characterized vaccines (206, 209, 210). Moreover, compatible animal models can also be developed and advanced for the evaluation of potential adjuvanted vaccines. In addition, in the clinic, most of the molecular analyses are done based on peripheral blood samples and nonhuman primate models (203), which allows the evaluation of local immune response to immunization and provides the chance for logical validation of already available peripheral blood signatures. For the speedy development of new-generation adjuvants, nonclinical studies always play a crucial role in bridging clinical interpretations.
Adjuvants consist mostly of natural components. Current technologies are continuously evolving for the development of new adjuvants; soon, there will be important advancements for adjuvants containing synthetic components. While the approved adjuvants will always be considered valuable assets for the future, the key components of many of these adjuvants have been developed from natural resources and could be exchanged by synthetic molecules that possess a similar function. New molecules that have the potential to become more effective activators and agonists are currently characterized. These molecules can be efficiently produced in bulk at a low cost as they can be easily purified and evaluated and less susceptible to their intrinsic biological variability. In addition, the process for the large-scale production of these synthetic molecules may be less susceptible to the many difficulties and challenges of sourcing and extracting natural components. However, before replacing the natural components in existing adjuvants, the concern about safety and protective efficacy should be addressed in clinical trials for the vaccine in formulation with these new adjuvant candidates. As previously discussed, several promising adjuvant candidates have been studied over the past 100 years; however, only a few adjuvants were approved for human use. The complex acylated polysaccharide emulsan produced by a bacterium, Acinetobacter calcoaceticus, can be a good candidate due to its amenability to structural tailoring and its emulsification behavior. We found that emulsan activates macrophages and showed significant adjuvant activity as determined by hapten-specific antibody titers. This immune response was characterized by a high immunoglobulin G2a titer, consistent with a Th1 response. The significant immune potentiation demonstrated by this complex polymer establishes emulsan as an exciting new candidate adjuvant. We proposed that by manipulating the emulsan chemical structure, we can explore the physical basis of PRRs and macrophage activation (211).
In the last few years, we gained a deep understanding and immense knowledge of innate immune signals that provide new avenues to focus on adjuvant targets with higher potentials, i.e., TLR, stimulator of interferon genes (STING), retinoic acid-inducible gene I (RIG-I), C-type lectin receptors (CLRs), nucleotide-binding oligomerization domain, leucine-rich repeat and pyrin domain-containing (NLRP), and interferon-inducible protein (AIM2). In addition, some complex exploratory adjuvant concepts have been developed, including multimeric formulations containing many distinct components (212). Despite having encouraging results in preclinical studies, such adjuvants hardly play any role in ideal prophylactic vaccines, but with the advent of therapeutic vaccines, there may be additional avenues, if bulk production, robustness, reproducibility problems, etc., can be resolved. Despite having a huge advancement in adjuvants, there are some noteworthy limitations such as the incapability to stimulate a strong CTL response in humans. However, to stimulate a broad and diverse immune response, the adjuvants may also be utilized in prime/boost backgrounds with the inclusion of nucleic acids and vectors (213). Such an attempt has been made to develop an effective vaccine against HIV using the prime/boost technique, and an adjuvanted vaccine antigen is being evaluated as a booster protein after priming with a vector (214). Therefore, clinical studies are extremely essential to define the best combinations of a heterologous prime/boost setting.
Several basic problems can be solved by using a better approach to characterizing the newly developed adjuvants, i.e., by elucidating the signaling cascades specific to the innate immunity, which is required to support the potentiality of a specific vaccine. How is the impact of a quick and prompt stimulation specific to vaccine efficacy? Is there any redundancy among the signal pathways of innate immune response? Is it possible to provide a better result through a more specific stimulation? What are the exact temporal and spatial associations between the effectors of innate immunity such as cell–cell interaction and cytokine signaling, and the adaptive immune effectors such as B and T cells? Are these reactions time-dependent, in which vaccine antigens and adjuvants remain at the vaccination site, and how are the local APCs activated? In human populations, how are the adjuvants’ specific immune responses affected by ecological factors and individual genetic predispositions? What about the influence of several concurrent medications and already diseased individuals on immunization?
Conclusion
The ultimate objective of immunization is to provide effective and long-lasting protection against various infectious diseases. Such kind of immune protection can only be achieved by using vaccine formulations carrying suitable adjuvants and antigens. Adjuvants are essential components of vaccine formulations and can modulate the vaccine response. Earlier methods of vaccine formulations focused on a single type of adjuvant, i.e., alum or emulsion. However, the new vaccine formulations need to stimulate the well-defined cellular and humoral immune responses. Therefore, to trigger robust immune responses, such as humoral and cellular immune responses, there is an utmost need for new immune potentiators or immune stimulant adjuvants in vaccine formulations. The mucosal adjuvant dmLT is the outcome of 25 years of research. It has been proven by both preclinical and clinical studies that dmLT is a safe and potent adjuvant that can induce protective immunity in a suitable formulation. There is a need to focus on some unanswered questions for the improvement of the dmLT adjuvant such as safety and stability issues of antigen–adjuvant combinations. The mechanism of adjuvanticity and the induction of protective immunity imparted by dmLT also warrant further studies. It has been suggested that dmLT can promote both innate and adaptive immune responses in the case of infants. Vaccine preparation with dmLT has been found to be effective against mucosal infections. It has been concluded that dmLT will be useful to overcome hypo-responsiveness to other whole cell- or LPS-based vaccines such as cholera, Salmonella typhi, and Shigella vaccines in infants in economically less developed countries (215). We predict that the use of dmLT in vaccine preparations for infants will be highly effective in the future.
In the recent past, many insights into the area of adjuvant research have been obtained; now, there is a choice to select a suitable adjuvant rather than a classical adjuvant, such as immune potentiators or combinations thereof, to augment vaccine efficacy. Recently, alum, MF59, AS03, CpG, and matrix-M have been licensed and used in vaccine preparations worldwide against COVID-19 (216). It has been suggested that synthetic gene coding for the spike protein, whether prefusion stabilized or also receptor binding domain only, is used to engineer mammalian cells, baculovirus, or plant cells to produce the recombinant protein that then is purified, combined with adjuvants, and used as vaccine. During vaccine preparations against COVID-19, we have gained industrial and clinical knowledge with protein-based vaccines combined with licensed adjuvants, and we believe that, in the future, vaccine preparation based on these methods will be well tolerated, effective, and available in large quantities. Furthermore, the use of maturation of RNA and viral vectors to create vaccines during the COVID-19 pandemic has opened up new avenues to produce more effective vaccines in the future for emerging infections. Recently completed clinical studies with new adjuvants recommend that a panel of new immune potentiators or immune stimulators be used in vaccine formulations for human use in the future. The accessibility of these new-generation adjuvants in different combinations will enable rational strategies for the development of successful vaccines.
Author contributions
SV, AJ, and RR have initiated and conceptualized this review. MS was written by SV, AJ, PM, and RA. Figures and tables were created by NS, PM, and AG. Revisions were made by RA, PM, AJ, RR and SV. All the authors have designed and recommended the contents and approved the final version.
Funding
This work was supported by the University Grants Commission, Government of India, and Israel Science Foundation under grant F. No. 6-5/2016 (1C) to AKJ. This work was supported by the University Grants Commission and Prime Minister Research Fellowship (PMRF), Government of India (to PM and RA, respectively).
Acknowledgments
We thank the School of Biotechnology and School of Life Sciences, Jawaharlal Nehru University, New Delhi, India, for providing the facility necessary to write this manuscript.
Conflict of interest
Author RR is employed by GSK Vaccine, Italy.
The remaining authors declare that the research was conducted in the absence of any commercial or financial relationships that could be construed as a potential conflict of interest.
Publisher’s note
All claims expressed in this article are solely those of the authors and do not necessarily represent those of their affiliated organizations, or those of the publisher, the editors and the reviewers. Any product that may be evaluated in this article, or claim that may be made by its manufacturer, is not guaranteed or endorsed by the publisher.
References
1. Koff WC, Burton DR, Johnson PR, Walker BD, King CR, Nabel GJ, et al. Accelerating next- generationvaccine development for global disease prevention. Science (2013) 340:1232910. doi: 10.1126/science.1232910
2. Walker BD, Burton DR. Toward an AIDS vaccine. Science (2008) 320:760–4. doi: 10.1126/science.1152622
3. Nabel GJ. Designing tomorrow’s vaccines. N Engl J Med (2013) 68:551–60. doi: 10.1056/nejmra1204186
4. Barman S, Soni D, Brook B, Nanishi E, Dowling DJ. Precision vaccine development: Cues from natural immunity. Front Immunol (2022) 12:662218. doi: 10.3389/fimmu.2021.662218
5. Moyer TJ, Zmolek AC, Irvine DJ. Beyond antigens and adjuvants: formulating future vaccines. J Clin Invest (2016) 126:799–808. doi: 10.1172/jci81083
6. Chan EH, Brewer TF, Madoff LC, Pollack MP, Sonricker AL, Keller M, et al. Global capacity for emerging infectious disease detection. Proc Natl Acad Sci U.S.A (2010) 107:21701–6. doi: 10.1073/pnas.1006219107
8. Chatterjee P. Nipah virus outbreak in India. Lancet (2018) 391:2200. doi: 10.1016/s0140-6736(18)31252-2
9. World Health Organization. Coronavirus disease. (2019). Available at: https://www.who.int/emergencies/diseases/novel-coronavirus-2019/situation-reports.
10. Ebola response team WHO. Ebola Virus disease in West Africa–the first 9 months of the epidemic and forward projections. N Engl J Med (2014) 371:1481–95. doi: 10.1056/nejmoa1411100
11. Lee S, Nguyen MT. Recent advances of vaccine adjuvants for infectious diseases. Immune Netw (2015) 15(2), 51–7. doi: 10.4110/in.2015.15.2.51
12. Lurie N, Saville M, Hatchett R, Halton J. A strategic approach to COVID-19 vaccine R&D. Science (2020) 368:948–50. doi: 10.1126/science.abc5312
13. Riese P, Schulze K, Ebensen T, Prochnow B, A Guzmán C. Vaccine adjuvants: key tools for innovative vaccine design. Curr Top Med Chem (2013) 13:2562–80. doi: 10.2174/15680266113136660183
14. Chaudhary N, Weissman D, Whitehead KA. mRNA vaccines for infectious diseases: principles, delivery and clinical translation. Nat Rev Drug Discovery (2021) 20(11):817–38. doi: 10.1038/s41573-021-00283-5
15. Baxter D. Active and passive immunity, vaccine types,excipients and licensing. Occup. Med (Lond) (2007) 57:552–6. doi: 10.1093/occmed/kqm110
17. Reed SG, Orr MT, Fox CB. Key roles of adjuvants in modern vaccines. Nat Med (2013) 19:1597–608. doi: 10.1038/nm.3409
18. Kyriakidis NC, López-Cortés A, González EV, Grimaldos AB, Prado EO. SARS-CoV-2 vaccines strategies: a comprehensive review of phase 3 candidates. NPJ Vaccines (2021) 6(1):1–17. doi: 10.1038/s41541-021-00292-w
19. Kenney RT, Cross AS. Adjuvants for the future. In: Levine MM, et al, editors. New generation vaccines. New York: Informa Healthcare USA, Inc (2010). p. 250–62.
20. Pulendran B, Powell J, Flavell RA. Modulating vaccine responses with innate immunity. In: Levine MM, et al, editors. New generation vaccines. New York: Informa Healthcare USA, Inc. (2010). p. 183–90.
21. Rapaka RR, Cross AS, McArthur MA. Using adjuvants to drive T cell responses for next-generation infectious disease vaccines. Vaccines (2021) 9(8):820. doi: 10.3390/vaccines9080820
22. Facciolà A, Visalli G, Laganà A, Di Pietro A. An overview of vaccine adjuvants: current evidence and future perspectives. Vaccines (2022) 10(5):819. doi: 10.3390/vaccines10050819
23. Podda A. The adjuvanted influenza vaccines with novel adjuvants: Experience with the MF59-adjuvanted vaccine. Vaccine (2001) 19:2673–80. doi: 10.1016/s0264-410x(00)00499-0
24. Beran J. Safety and immunogenicity of a new hepatitis b vaccine for the protection of patients with renal insufficiency including pre-haemodialysis and haemodialysis patients. Expert Opin Biol Ther (2008) 8:235–47. doi: 10.1517/14712598.8.2.235
25. Boyle J, Eastman D, Millar C, Camuglia S, Cox J, Pearse M, et al. The utility of ISCOMATRIX adjuvant for dose reduction of antigen for vaccines requiring antibody responses. Vaccine (2007) 25:2541–4. doi: 10.1016/j.vaccine.2006.12.018
26. Banzhoff A, Gasparini R, Laghi-Pasini F, Staniscia T, Durando P, Montomoli E, et al. MF59-adjuvanted H5N1 vaccine induces immunologic memory and heterotypic antibody responses in non-elderly and elderly adults. PloS One (2009) 4:e4384. doi: 10.1371/journal.pone.0004384
27. Schwarz TF, Horacek T, Knuf M, Damman HG, Roman F, Dramé M, et al. Single dose vaccination with AS03-adjuvanted H5N1 vaccines in a randomized trial induces strong and broad immune responsiveness to booster vaccination in adults. Vaccine (2009) 27:6284–90. doi: 10.1016/j.vaccine.2009.01.040
28. Halperin SA, Dobson S, McNeil S, Langley JM, Smith B, McCall-Sani R, et al. Comparison of the safety and immunogenicity of hepatitis b virus surface antigen co-administered with an immunostimulatoryphosphorothioate oligonucleotide and alicensed hepatitis b vaccine in healthy young adults. Vaccine (2006) 24:20–6. doi: 10.1016/j.vaccine.2005.08.095
29. Ebensen T, Libanova R, Schulze K, Yevsa T, Morr M, Guzmán CA. Bis-(3′, 5′)-cyclic dimeric adenosine monophosphate: strong Th1/Th2/Th17 promoting mucosal adjuvant. Vaccine (2011) 29(32):5210–20. doi: 10.1016/j.vaccine.2011.05.026
30. Batra L, Verma SK, Nagar DP, Saxena N, Pathak P, Pant SC, et al. HSP70 domain II of Mycobacterium tuberculosis modulates immune response and protective potential of F1 and LcrV antigens of yersinia pestis in a mouse model. PloS Negl Trop Dis (2014) 8:e3322. doi: 10.1371/journal.pntd.0003322
31. Fisher EL, Otto M, Cheung GY. A recombinant trivalent fusion protein F1-LcrV-HSP70(II) augments humoral and cellular immune responses and imparts full protection against Yersinia pestis. Front Microbiol (2016) 7, 71053. doi: 10.3389/fmicb.2016.01053
32. Pulendran BS, Arunachalam P, O'Hagan DT. Emerging concepts in the science of vaccine adjuvants. Nat Rev Drug Discovery (2021) 20(6):454–75. doi: 10.1038/s41573-021-00163-y
33. Ong GH, Lian BS, Kawasaki T, Kawai T. Exploration of pattern recognition receptor agonists as candidate adjuvants. Front.Cell.Infect. Microbiol (2021) 968:745016. doi: 10.3389/fcimb.2021.745016
34. Vandepapelière P, Horsmans Y, Moris P, Van Mechelen M, Janssens M, Koutsoukos M, et al. Vaccine adjuvant systems containing monophosphoryl lipid a and QS21 induce strong and persistent humoral and T cell responses against hepatitis b surface antigen in healthy adult volunteers. Vaccine (2008) 26:1375–86. doi: 10.1016/j.vaccine.2007.12.038
35. Galli G, Medini D, Borgogni E, Zedda L, Bardelli M, Malzone C, et al. Adjuvanted H5N1 vaccine induces early CD4+ T cell response that predicts long-term persistence of protective antibody levels. Proc Natl Acad Sci USA (2009) 106:3877–82. doi: 10.1073/pnas.0813390106
36. Leroux-Roels I, Roman F, Forgus S, Maes C, De Boever F, Dramé M, et al. Priming with AS03 a-adjuvanted H5N1 influenza vaccine improves the kinetics, magnitude and durability of the immune response after a heterologous booster vaccination: An open non-randomised extension of a double-blind randomised primary study. Vaccine (2010) 28:849–57. doi: 10.1016/j.vaccine.2009.10.017
37. Huleatt JW, Jacobs AR, Tang J, Desai P, Kopp EB, Huang Y, et al. Vaccination with recombinant fusion proteins incorporating toll-like receptor ligands inducesrapid cellular and humoral immunity. Vaccine (2007) 25:763–75. doi: 10.1016/j.vaccine.2006.08.013
38. Galli G, Hancock K, Hoschler K, DeVos J, Praus M, Bardelli M, et al. Fast rise of broadly cross-reactive antibodies after boosting long-lived human memory b cells primed by an MF59 adjuvanted prepandemic vaccine. Proc Natl Acad Sci USA (2009) 106:7962–7. doi: 10.1073/pnas.0903181106
39. Khurana S, Chearwae W, Castellino F, Manischewitz J, King LR, Honorkiewicz A, et al. Vaccines with MF59 adjuvant expand the antibody repertoire to target protective sites of pandemic avian H5N1 influenza virus. Sci Transl Med (2010) 2:15ra15. doi: 10.1126/scitranslmed.3000624
40. Malherbe L, Mark L, Fazilleau N, McHeyzer-Williams LJ, McHeyzer-Williams MG. Vaccine adjuvants alter TCR-based selection thresholds. Immunity (2008) 28:698–709. doi: 10.1016/j.immuni.2008.03.014
41. HogenEsch H, O’Hagan DT, Fox CB. Optimizing the utilization of aluminum adjuvants in vaccines: you might just get what you want. NPJ Vaccines (2018) 3(1):1–11. doi: 10.1038/s41541-018-0089-x
42. Brewer JM. (How) do aluminium adjuvants work? Immunol Lett (2006) 102:10–5. doi: 10.1016/j.imlet.2005.08.002
43. Lindblad EB. Aluminium adjuvants–in retrospect andprospect. Vaccine (2004) 22:3658–68. doi: 10.1016/j.vaccine.2004.03.032
44. Pollard AJ, Bijker EM. A guide to vaccinology: from basic principles to new developments. Nat Rev Immunol (2021) 21(2):83–100. doi: 10.1038/s41577-020-00479-7
45. Wu Z, Hu Y, Xu M, Chen Z, Yang W, Jiang Z, et al. Safety, tolerability, and immunogenicity of an inactivated SARS-CoV-2 vaccine (CoronaVac) in healthy adults aged 60 years and older: a randomised, double-blind, placebo-controlled, phase 1/2 clinical trial. Lancet Infect Dis (2021) 21(6):803–12. doi: 10.1016/s1473-3099(20)30987-7
46. Xia S, Zhang Y, Wang Y, Wang H, Yang Y, Gao GF, et al. Safety and immunogenicity of an inactivated SARS-CoV-2 vaccine, BBIBP-CorV: A randomised, double-blind, placebo-controlled, phase 1/2 trial. Lancet Infect Dis (2021) 21(1):39–51. doi: 10.1016/s1473-3099(20)30831-8
47. Zhang Y, Zeng G, Pan H, Li C, Hu Y, Chu K, et al. Safety, tolerability, and immunogenicity of an inactivated SARS-CoV-2 vaccine in healthy adults aged 18–59 years: a randomised, double-blind, placebo-controlled, phase 1/2 clinical trial. Lancet Infect Dis (2021) 21(2):181–92. doi: 10.1016/s1473-3099(20)30843-4
48. Hutchison S, Benson RA, Gibson VB, Pollock AH, Garside P, Brewer JM. Antigen depot is not required for alum adjuvanticity. FASEB J (2012) 26:1272–9. doi: 10.1096/fj.11-184556
49. Awate S, Babiuk LA, Mutwiri G. Mechanisms of action of adjuvants. Front Immunol (2013) 4114:114. doi: 10.3389/fimmu.2013.00114
50. Marrack P, McKee AS, Munks MW. Towards an understanding of the adjuvant action of aluminium. Nat Rev Immunol (2009) 9:287–93. doi: 10.1038/nri2510
51. Lambrecht BN, Kool M, Willart MA, Hammad H. Mechanism of action of clinically approved adjuvants. Curr Opin Immunol (2009) 21:23–9. doi: 10.1016/j.coi.2009.01.004
52. Jiang H, Wang Q, Li L, Zeng Q, Li H, Gong T, et al. Turning the old adjuvant from gel to nanoparticles to amplify CD8+ T cell responses. Adv Sci (2018) 5(1):1700426. doi: 10.1002/advs.201700426
53. Gavin AL, Hoebe K, Duong B, Ota T, Martin C, Beutler B, et al. Adjuvant-enhanced antibody responses in the absence of toll-like receptor signalling. Science (2006) 314:1936–8. doi: 10.1126/science.1135299
54. Didierlaurent AM, Morel S, Lockman L, Giannini SL, Bisteau M, Carlsen H, et al. AS04, an aluminum salt- and TLR4 agonist-based adjuvant system, induces a transient localized innate immune response leading to enhanced adaptive immunity. J Immunol (2009) 183:6186–97. doi: 10.4049/jimmunol.0901474
55. Bonam SR, Partidos CD, Halmuthur SK, Muller S. An overview of novel adjuvants designed for improving vaccine efficacy. Trends Pharmacolsci (2017) 38(9):771–93. doi: 10.1016/j.tips.2017.06.002
56. Li H, Nookala S, Re F. Aluminum hydroxide adjuvants activate caspase-1 and induce IL-1beta andIL-18 release. J Immunol (2007) 178:5271–6. doi: 10.4049/jimmunol.178.8.5271
57. Reed SG, Tomai M, Gale MJ Jr. New horizons in adjuvants for vaccine development. Trends. Immunol (2009) 30:23–32. doi: 10.1016/j.it.2008.09.006
58. Sampath V, Rabinowitz G, Shah M, Jain S, Diamant Z, Jesenak M, et al. Vaccines and allergic reactions: The past, the current COVID-19 pandemic, and future perspectives. Allergy (2021) 76(6):1640–60. doi: 10.1111/all.14840
59. Mori A, Oleszycka E, Sharp FA, Coleman M, Ozasa Y, Singh M, et al. The vaccine adjuvant alum inhibits IL-12 by promoting PI 3 kinase signaling while chitosan does not inhibit IL-12 and enhances T h1 and T h17 responses. Eur J Immunol (2012) 42(10):2709–19. doi: 10.1002/eji.201242372
60. Khameneh HJ, Ho AW, Spreafico R, Derks H, Quek HQ, Mortellaro A. The syk–NFAT–IL-2 pathway in dendritic cells is required for optimal sterile immunity elicited by alum adjuvants. J Immunol (2017) 198(1):196–204. doi: 10.4049/jimmunol.1600420
61. Batty CJ, Heise MT, Bachelder EM, Ainslie KM. Vaccine formulations in clinical development for the prevention of severe acute respiratory syndrome coronavirus 2 infection. Adv Drug Del. Rev (2021) 169:168–89. doi: 10.1016/j.addr.2020.12.006
62. Carroll MC, Isenman DE. Regulation of humoral immunity by complement. Immunity (2012) 37(2):199–207. doi: 10.1016/j.immuni.2012.08.002
63. Güven E, Duus K, Laursen I, Højrup P, Houen G. Aluminum hydroxide adjuvant differentially activates the three complement pathways with major involvement of the alternative pathway. PloS One (2013) 8(9):e74445. doi: 10.1371/journal.pone.0074445
64. Gupta RK. Aluminum compounds as vaccine adjuvants. Adv Drug Deliv. Rev (1998) 32:155–72. doi: 10.1016/s0169-409x(98)00008-8
65. O'hagan DT, Wack A, Podda A. MF59 is a safe and potent vaccine adjuvant for flu vaccines in humans: what did we learn during its development? Clin Pharm Ther (2007) 82:740–4. doi: 10.1038/sj.clpt.6100402
66. Vesikari T, Pellegrini M, Karvonen A, Groth N, Borkowski A, O'Hagan DT, et al. Enhanced immunogenicity of seasonal influenza vaccines in young children using MF59 adjuvant. Pediatr Infect Dis J (2009) 28:563–71. doi: 10.1097/inf.0b013e31819d6394
67. O’Hagan DT, Ott GS, Nest GV, Rappuoli R, Giudice GD. The history of MF59 adjuvant: a phoenix that arose from the ashes. Expert Rev Vaccines (2013) 12:13–30. doi: 10.1586/erv.12.140
68. Vesikari T, Knuf M, Wutzler P, Karvonen A, Kieninger-Baum D, Schmitt HJ, et al. Oil-in-water emulsion adjuvant with influenza vaccine in young children. N Engl J Meds (2011) 365:1406–16. doi: 10.1056/nejmoa1010331
69. Nakaya HI, Clutterbuck E, Kazmin D, Wang L, Cortese M, Bosinger SE, et al. Systems biology of immunity to MF59-adjuvanted versus nonadjuvanted trivalent seasonal influenza vaccines in early childhood. Proc Natl Acad Sci USA (2016) 113:1853–8. doi: 10.1073/pnas.1519690113
70. Heineman TC, Clements-Mann ML, Poland GA, Jacobson RM, Izu AE, Sakamoto D, et al. A randomized, controlled study in adults of the immunogenicity of a novel hepatitis b vaccine containing MF59 adjuvant. Vaccine (1999) 17:2769–78. doi: 10.1016/s0264-410x(99)00088-2
71. Dupuis M, McDonald DM, Ott G. Distribution of adjuvant MF59 and antigen gD2 after intramuscular injection in mice. Vaccine (1999) 18:434–9. doi: 10.1016/s0264-410x(99)00263-7
72. Stephenson I, Bugarini R, Nicholson KG, Podda A, Wood JM, Zambon MC, et al. Crossreactivity to highly pathogenic avian influenza H5N1 viruses after vaccination with nonadjuvanted and MF59-adjuvanted influenza A/Duck/Singapore/97 (H5N3) vaccine: a potential priming strategy. J Infect Dis (2005) 191:1210–5. doi: 10.1086/428948
73. Seubert A, Monaci E, Pizza M, O’Hagan DT, Wack A. The adjuvants aluminum hydroxide and MF59 induce monocyte and granulocyte chemoattractants and enhance monocyte differentiation toward dendritic cells. J Immunol (2008) 180:5402–12. doi: 10.4049/jimmunol.180.8.5402
74. De Gregorio E, Caproni E, Ulmer JB. Vaccine adjuvants: Mode of action. Front Immunol (2013) 4:214. doi: 10.3389/fimmu.2013.00214
75. Garçon N, Vaughn DW, Didierlaurent AM. Development and evaluation of AS03, an adjuvant system containing α-tocopherol and squalene in an oil-in-water emulsion. Expert Rev Vaccines (2012) 11:349–66. doi: 10.1586/erv.11.192
76. Morel S, Didierlaurent A, Bourguignon P, Delhaye S, Baras B, Jacob V, et al. Adjuvant system AS03 containing a-tocopherol modulates innate immune response and leads to improved adaptive immunity. Vaccine (2011) 29:2461–73. doi: 10.1016/j.vaccine.2011.01.011
77. Stoute JA, Slaoui M, Heppner DG, Momin P, Kester KE, Desmons P, et al. A preliminary evaluation of a recombinant circumsporozoite protein vaccine against plasmodium falciparum malaria. N Engl J Med (1997) 336:86–91. doi: 10.1056/nejm199701093360202
78. Díez-Domingo J, Garcés-Sanchez M, Baldó JM, Planelles MV, Ubeda I, JuBert A, et al. Immunogenicity and safety of H5N1 A/vietnam/1194/2004 (clade 1) AS03-adjuvanted prepandemic candidate influenza vaccines in children aged 3 to 9 years: a phase II, randomized, open, controlled study. Pediatr Infect Dis J (2010) 29:e35–46. doi: 10.1097/INF.0b013e3181daf921
79. Carmona Martinez A, Salamanca de la Cueva I, Boutet P, Vanden Abeele C, Smolenov I, Devaster JM. A phase 1, open-label safety and immunogenicity study of an AS03-adjuvanted trivalent inactivated influenza vaccine in children aged 6 to 35 months. Hum Vaccin. Immunother (2014) 10:1959–68. doi: 10.4161/hv.28743
80. Garçon N, Di Pasquale A. Di pasquale from discovery to licensure, the adjuvant system story. Hum Vaccin. Immunother (2017) 13:19–33. doi: 10.1080/21645515.2016.1225635
81. Wilkins AL, Kazmin D, Napolitani G, Clutterbuck EA, Pulendran B, Siegrist CA, et al. AS03- and MF59-adjuvanted influenza vaccines in children. Front Immunol (2017) 13:1760. doi: 10.3389/fimmu.2017.01760
82. Alving CR, Peachman KK, Matyas GR, Rao M, Beck Z. Army liposome formulation (ALF) family of vaccine adjuvants. Expert Rev Vaccines (2020) 19(3):279–92. doi: 10.1080/14760584.2020.1745636
83. Alving CR, Richards RL, Moss J, Alving LI, Clements JD, Shiba T, et al. Effectiveness of liposomes as potential carriers of vaccines: applications to cholera toxin and human malaria sporozoite antigen. Vaccine (1986) 4(3):166–72. doi: 10.1016/0264-410x(86)90005-8
84. White WI, Cassatt DR, Madsen J, Burke SJ, Woods RM, Wassef NM, et al. Antibody and cytotoxic T-lymphocyte responses to a single liposome-associated peptide antigen. Vaccine (1995) 13(12):1111–22. doi: 10.1016/0264-410x(94)00058-u
85. Memvanga PB, Nkanga CI. Liposomes for malaria management: the evolution from 1980 to 2020. Malar. J (2021) 20(1):1–33. doi: 10.1186/s12936-021-03858-0
86. Genito CJ, Beck Z, Phares TW, Kalle F, Limbach KJ, Stefaniak ME, et al. Liposomes containing monophosphoryl lipid a and QS-21 serve as an effective adjuvant for soluble circumsporozoite protein malaria vaccine FMP013. Vaccine (2017) 35(31):3865–74. doi: 10.1016/j.vaccine.2017.05.070
87. Cawlfield A, Genito CJ, Beck Z, Bergmann-Leitner ES, Bitzer AA, Soto K, et al. Safety, toxicity and immunogenicity of a malaria vaccine based on the circumsporozoite protein (FMP013) with the adjuvant army liposome formulation containing QS21 (ALFQ). Vaccine (2019) 37(29):3793–803. doi: 10.1016/j.vaccine.2019.05.059
88. Seth L, Ferlez KM, Kaba SA, Musser DM, Emadi S, Matyas GR, et al. Development of a self-assembling protein nanoparticle vaccine targeting plasmodium falciparum circumsporozoite protein delivered in three army liposome formulation adjuvants. Vaccine (2017) 35(41):5448–54. doi: 10.1016/j.vaccine.2017.02.040
89. Rerks-Ngarm S, Pitisuttithum P, Nitayaphan S, Kaewkungwal J, Chiu J, Paris R, et al. Vaccination with ALVAC and AIDSVAX to prevent HIV-1 infection in Thailand. N Engl J Med (2009) 361(23):2209–20. doi: 10.1056/nejmoa0908492
90. Beck Z, Matyas GR, Jalah R, Rao M, Polonis VR, Alving CR. Differential immune responses to HIV-1 envelope protein induced by liposomal adjuvant formulations containing monophosphoryl lipid a with or without QS21. Vaccine (2015) 33(42):5578–87. doi: 10.1016/j.vaccine.2015.09.001
91. Beck Z, Torres OB, Matyas GR, Lanar DE, Alving CR. Immune response to antigen adsorbed to aluminum hydroxide particles: Effects of co-adsorption of ALF or ALFQ adjuvant to the aluminum-antigen complex. J Control Release (2018) 275:12–9. doi: 10.1016/j.jconrel.2018.02.006
92. Jobe O, Kim J, Pinto DO, Villar Z, Hewitt T, Duncan EH, et al. Army liposome formulation containing QS-21 render human monocyte-derived macrophages less permissive to HIV-1 infection by upregulating APOBEC3A. Sci Rep (2022) 12(1):1–17. doi: 10.1038/s41598-022-11230-8
93. Cryz SJ, Que JU, Glück R. A virosome vaccine antigen delivery system does not stimulate an antiphospholipid antibody response in humans. Vaccine (1996) 14:1381–3. doi: 10.1016/s0264-410x(96)00040-0
94. Mischler R, Metcalfe IC. Inflexal® V a trivalent virosome subunit influenza vaccine: production. Vaccine (2002) 20:B17–23. doi: 10.1016/s0264-410x(02)00512-1
95. Moser C, Amacker M, Kammer AR, Rasi S, Westerfeld N, Zurbriggen R. Influenza virosomes as a combined vaccine carrier and adjuvant system for prophylactic and therapeutic immunizations. Expert Rev Vaccines (2007) 6:711–21. doi: 10.1586/14760584.6.5.711
96. Arkema A, Huckriede A, Schoen P, Wilschut J, Daemen T. Induction of cytotoxic T lymphocyte activity by fusion-active peptide-containing virosomes. Vaccine (2000) 18:1327–33. doi: 10.1016/s0264-410x(99)00404-1
97. Cusi MG. Applications of influenza virosomes as a delivery system. Hum Vaccines (2006) 2 1 7, 1–7. doi: 10.4161/hv.2.1.2494
98. Bungener L, Serre K, Bijl L, Leserman L, Wilschut J, Daemen T, et al. Virosome-mediated delivery of protein antigens to dendritic cells. Vaccine (2002) 20:2287–95. doi: 10.1016/s0264-410x(02)00103-2
99. Huckriede A, Bungener L, Stegmann T, Daemen T, Medema J, Palache AM, et al. The virosome concept for influenza vaccines. Vaccine (2005) 23:S26–38. doi: 10.1016/j.vaccine.2005.04.026
100. Herzog C, Hartmann K, Künzi V, Kürsteiner O, Mischler R, Lazar H, et al. Eleven years of inflexal V-a virosomaladjuvanted influenza vaccine. Vaccine (2009) 27:4381–7. doi: 10.1016/j.vaccine.2009.05.029
101. Saroja CH, Lakshmi PK, Bhaskaran S. Recent trends in vaccine delivery systems: a review. Int J Pharm Investig (2011) 1(2):64. doi: 10.4103/2230-973x.82384
102. Burny W, Callegaro A, Bechtold V, Clement F, Delhaye S, Fissette L, et al. Different adjuvantsinduce common innate pathways that are associated with enhanced adaptive responses against amodel antigen in humans. Front Immunol (2017) 8:943. doi: 10.3389/fimmu.2017.00943
103. Leroux-Roels G, Marchant A, Levy J, Van Damme P, Schwarz TF, Horsmans Y, et al. Impact of adjuvants on CD4(+) T cell and b cell responses to a protein antigen vaccine: Results from a phase II, randomized, multicenter trial. Clin Immunol (2016) 169:16–27. doi: 10.1016/j.clim.2016.05.007
104. Giannini SL, Hanon E, Moris P, Van Mechelen M, Morel S, Dessy F, et al. Enhanced humoral and memory b cellular immunity using HPV16/18 L1 VLP vaccine formulated with the MPL/aluminium salt combination (AS04) compared to aluminium salt only. Vaccine (2006) 24:5937–49. doi: 10.1016/j.vaccine.2006.06.005
105. Del Giudice G, Rappuoli R, Didierlaurent AM. Correlates of adjuvanticity: A review on adjuvants in licensed vaccines. Semin Immunol (2018) 39:14–21. doi: 10.1016/j.smim.2018.05.001
106. Ismaili J, Rennesson J, Aksoy E, Vekemans J, Vincart B, Amraoui Z, et al. Monophosphoryl lipid a activates both human dendritic cells and T cells. J Immunol (2002) 168:926 –932. doi: 10.4049/jimmunol.168.2.926
107. De Becker G, Moulin V, Pajak B, Bruck C, Francotte M, Thiriart C, et al. The adjuvant monophosphoryl lipid a increases the function of antigen-presenting cells. Int Immunol (2000) 12:807–815. doi: 10.1093/intimm/12.6.807
108. Evans JT, Cluff CW, Johnson DA, Lacy MJ, Persing DH, Baldridge JR. Enhancement of antigen-specific immunity via the TLR4 ligands MPL adjuvant and Ribi.529. Expert Rev Vaccines (2003) 2:219 –229. doi: 10.1586/14760584.2.2.219
109. Baldridge JR, McGowan P, Evans JT, Cluff C, Mossman S, Johnson D, et al. Taking a toll on human disease: Toll-like receptor 4 agonists as vaccine adjuvants and monotherapeutic agents. Expert Opin Biol Ther (2004) 4:1129–38. doi: 10.1517/14712598.4.7.1129
111. Thompson BS, Chilton PM, Ward JR, Evans JT, Mitchell TC. The low-toxicity versions of LPS, MPL adjuvant and RC529, are efficient adjuvants for CD4+ T cells. J Leukoc. Biol (2005) 78:1273–80. doi: 10.1189/jlb.0305172
112. Aoshi T. Modes of action for mucosal vaccine adjuvants. Viral Immunol (2017) 30:463–70. doi: 10.1089/vim.2017.0026
113. Zeng L. Mucosal adjuvants: Opportunities and challenges. Hum.Vaccine Immunother (2016) 12:2456–8. doi: 10.1080/21645515.2016.1181236
114. Van der Lubben IM, Verhoef JC, Borchard G, Junginger HE. Chitosan and its derivatives in mucosal drug and vaccine delivery. Eur J Pharm Sci (2001) 14:201–7. doi: 10.1016/s0928-0987(01)00172-5
115. Lycke N. Recent progress in mucosal vaccine development: potential and limitations. Nat Rev Immunol (2012) 12:592–605. doi: 10.1038/nri3251
116. Nedrud JG, Bagheri N, Schön K, Xin W, Bergroth H, Eliasson DG, et al. Subcomponent vaccine based on CTA1-DD adjuvant with incorporated UreB class II peptides stimulates protective Helicobacter pylori immunity. PloS One (2013) 8:e83321. doi: 10.1371/journal.pone.0083321
117. Fujihashi K, Sato S, Kiyono H. Mucosal adjuvants for vaccines to control upper respiratory infections in the elderly. Exp Gerontol (2014) 54:21–6. doi: 10.1016/j.exger.2014.01.006
118. Sjökvist Ottsjö L, Flach CF, Clements J, Holmgren J, Raghavan S. A double mutant heat-labile toxin from Escherichia coli, LT (R192G/L211A), is an effective mucosal adjuvant for vaccination against helicobacter pylori infection. Infect Immun (2013) 81:1532–40. doi: 10.1128/iai.01407-12
119. Gallichan WS, Woolstencroft RN, Guarasci T, McCluskie MJ, Davis HL, Rosenthal KL. Intranasal immunization with CpGoligodeoxynucleotides as an adjuvant dramatically increases IgA and protection against herpes simplex virus-2 in the genital tract. J Immunol (2001) 166:3451–7. doi: 10.4049/jimmunol.166.5.3451
120. McCluskie MJ, Davis HL. Cutting edge: CpG DNA is a potent enhancer of systemic and mucosal immune responses against hepatitis b surface antigen with intranasal administration to mice. J Immunol (1998) 161:4463–6. doi: 10.4049/jimmunol.161.9.4463
121. Porgador A, Staats HF, Faiola B, Gilboa E, Palker TJ. Intranasal immunization with CTL epitope peptides from HIV-1 or ovalbumin and the mucosal adjuvant cholera toxin induces peptide-specific CTLs and protection against tumor development in vivo. J Immunol (1997) 158:834–841. doi: 10.4049/jimmunol.158.2.834
122. Ko SY, Ko HJ, Chang WS, Park SH, Kweon MN, Kang CY. Alpha-galactosylceramide can act as a nasal vaccine adjuvant inducing protective immune responses against viral infection and tumor. J Immunol (2005) 175:3309–17. doi: 10.4049/jimmunol.175.5.3309
123. Clements JD, Norton EB. The mucosal vaccine adjuvant LT(R192G/L211A) or dmLT. mSphere (2018) 3(4):e00215–18. doi: 10.1128/msphere.00215-18
124. Norton EB, Lawson LB, Freytag LC, Clements JD. Characterization of a mutant escherichia coli heat-labile toxin, LT(R192G/L211A), as a safe and effective oral adjuvant. Clin Vaccine Immunol (2011) 18:546–51. doi: 10.1128/cvi.00538-10
125. Merritt EA, Pronk SE, Sixma TK, Kalk KH, van Zanten BA, Hol WG. Structure of partially activated E. coli heat-labile enterotoxin (LT) at 2.6 a resolution. FEBS Lett (1994) 337:88–92. doi: 10.1016/0014-5793(94)80635-7
126. Coleman BL, McGeer AJ, Halperin SA, Langley JM, Shamout Y, Taddio, et al. A randomized control trial comparing immu- nogenicity, safety, and preference for self- versus nurse-administered intradermal influenza vaccine. Vaccine (2012) 30:6287–93. doi: 10.1016/j.vaccine.2012.08.006
127. Kim YC, Jarrahian C, Zehrung D, Mitragotri S, Prausnitz MR. Delivery systems for intradermal vaccination. Curr Top Microbiol Immunol (2012) 351:77–112. doi: 10.1007/82_2011_123
128. Singh J, Bhatia R, Gandhi JC, Kaswekar AP, Khare S, Patel SB, et al. Outbreak of viral hepatitis b in a rural community in India linked to inadequately sterilized needles and syringes. Bull World Health Organ (1998) 76:93–8.
129. Aylward B, Kane M, McNair-Scott R, Hu DH. Model-based estimates of the risk of human immunodeficiency virus and hepatitis b virus transmission through unsafe injections. . Int J Epidemiol (1995) 24:446–52. doi: 10.1093/ije/24.2.446
130. Veldhoen M. Interleukin 17 is a chief orchestrator of immunity. Nat Immunol (2017) 18:612–21. doi: 10.1038/ni.3742
131. Doreau A, Belot A, Bastid J, Riche B, Trescol-Biemont MC, Ranchin B, et al. Interleukin 17 acts in synergy with b cell-activating factor to influence b cell biology and the pathophysiology of systemic lupus erythematosus. Nat Immunol (2009) 10:778–85. doi: 10.1038/ni.1741
132. Mitsdoerffer M, Lee Y, Jäger A, Kim HJ, Korn T, Kolls JK, et al. Proinflammatory T helper type 17 cells are effective b-cell helpers. Proc Natl Acad Sci USA (2010) 107:14292–7. doi: 10.1073/pnas.1009234107
133. Jaffar Z, Ferrini ME, Girtsman TA, Roberts K. Antigen-specific treg regulate Th17-mediated lung neutrophilic inflammation. b-cell recruitment and polymeric IgA and IgM levels in the airways. Eur J Immunol (2009) 39:3307–14. doi: 10.1002/eji.200939498
134. Jaffar Z, Ferrini ME, Herritt LA, Roberts K. Cutting edge: lung mucosal Th17-mediated responses induce polymeric ig receptor expression by the airway epithelium and elevate secretory IgA levels. J Immunol (2009) 182:4507–11. doi: 10.4049/jimmunol.0900237
135. Frederick DR, Goggins JA, Sabbagh LM, Freytag LC, Clements JD, McLachlan JB. Adjuvant selection regulates gut migration and phenotypic diversity of antigen specific CD4(+) T cells following parenteral immunization. Mucosal. Immunol (2018) 11:549–61. doi: 10.1038/mi.2017.70
136. Norton EB, Bauer DL, Weldon WC, Oberste MS, Lawson LB, Clements JD. The novel adjuvant dmLT promotes dose sparing, mucosal immunity and longevity of antibody responses to the inactivated polio vac- cine in a murine model. Vaccine (2015) 33:1909 –1915. doi: 10.1016/j.vaccine.2015.02.069
137. Novotny LA, Clements JD, Bakaletz LO. Kinetic analysis and evaluation of the mechanisms involved in the resolution of experimental nontypeable haemophilus influenzae-induced otitis media after transcutaneous immunization. Vaccine (2013) 31:3417–26. doi: 10.1016/j.vaccine.2012.10.033
138. Lee S, Picking WL, Tzipori S. The immune response of two microbial antigens delivered intradermally, sublingually, or the combination thereof. Microbes Infect (2014) 16:796–803. doi: 10.1016/j.micinf.2014.07.013
139. Freytag LC, Clements JD. Mucosal adjuvants. Vaccine (2005) 23:1804–13. doi: 10.1016/j.vaccine.2004.11.010
140. Lawson LB, Norton EB, Clements JD. Defending the mucosa: adjuvant and carrier formulations for mucosal immunity. Curr Opin Immunol (2011) 23:414–420. doi: 10.1016/j.coi.2011.03.009
141. Norton EB, Clements JD, Voss TG, Cárdenas-Freytag L. Prophylactic administration of bacterially derived immunomodulators improves the outcome of influenza virus infection in a murine model. J Virol (2010) 84:2983–95. doi: 10.1128/jvi.01805-09
142. Anosova NG, Chabot S, Shreedhar V, Borawski JA, Dickinson BL, Neutra MR. Cholera toxin, E. coli heat-labile toxin, and non-toxic derivatives induce dendritic cell migration into the follicle-associated epithelium of peyer’s patches. Mucosal Immunol (2008) 1:59–67. doi: 10.1038/mi.2007.7
143. Bagley KC, Abdelwahab SF, Tuskan RG, Fouts TR, Lewis GK. Cholera toxin and heat-labile enterotoxin activate human monocyte-derived dendritic cells and dominantly inhibit cytokine production through a cyclic AMP-dependent pathway. Infect Immun (2002) 70:5533–9. doi: 10.1128/iai.70.10.5533-5539.2002
144. Norton EB, Lawson LB, Mahdi Z, Freytag LC, Clements JD. The a-subunit of escherichia coli heat-labile enterotoxin functions as a mucosal adjuvant and promotes IgG2a, IgA and Th17 responses to vaccine antigens. Infect Immun (2012) 80:2426 –2435. doi: 10.1128/iai.00181-12
145. Lu YJ, Yadav P, Clements JD, Forte S, Srivastava A, Thompson CM, et al. Options for inactivation, adjuvant, and route of topical administration of a killed, unencapsulated pneumococcal whole- cell vaccine. Clin Vaccine Immunol (2010) 17:1005–12. doi: 10.1128/cvi.00036-10
146. Leach S, Clements JD, Kaim J, Lundgren A. The adjuvant double mutant escherichia coli heat labile toxin enhances IL-17A production in human T cells specific for bacterial vaccine antigens. PloS One (2012) 7:e51718. doi: 10.1371/journal.pone.0051718
147. Da Fonseca DM, Hand TW, Han SJ, Gerner MY, Zaretsky AG, Byrd AL, et al. Microbiota-dependent sequelae of acute infection compromise tissue-specific immunity. Cell (2015) 163:354 –366. doi: 10.1016/j.cell.2015.08.030
148. Brereton CF, Sutton CE, Ross PJ, Iwakura Y, Pizza M, Rappuoli R, et al. Escherichia coli heat-labile enterotoxin promotes protective Th17 responses against infection by driving innate IL-1 and IL-23 production. J Immunol (2011) 186:5896–906. doi: 10.4049/jimmunol.1003789
149. Mosaheb MM, Reiser ML, Wetzler LM. Toll-like receptor ligand-based vaccine adjuvants require intact MyD88 signaling in antigen-presenting cells for germinal center formation and antibody production. Front Immunol (2017) 8:225. doi: 10.3389/fimmu.2017.00225
150. Shetab Boushehri MA, Lamprecht A. TLR4-based immunotherapeutics in cancer: a review of the achievements and shortcomings. Mol Pharm (2018) 15(11):4777–800. doi: 10.1021/acs.molpharmaceut.8b00691
151. Liang H, Poncet D, Seydoux E, Rintala ND, Maciel M, Ruiz S, et al. The TLR4 agonist adjuvant SLA-SE promotes functional mucosal antibodies against a parenterally delivered ETEC vaccine. NPG Vaccines (2019) 4(1):1–8. doi: 10.1038/s41541-019-0116-6
152. Jeong SK, Heo YK, Jeong JH, Ham SJ, Yum JS, Ahn BC, et al. COVID-19 subunit vaccine with a combination of TLR1/2 and TLR3 agonists induces robust and protective immunity. Vaccines (2021) 9(9):957. doi: 10.3390/vaccines9090957
153. Lavelle EC, Ward RW. Mucosal vaccines–fortifying the frontiers. Nat Rev Immunol (2022) 22(4):236–50. doi: 10.1038/s41577-021-00583-2
154. Paul WE. Bridging innate and adaptive immunity. Cell (2011) 147:1212–5. doi: 10.1016/j.cell.2011.11.036
155. Martinon F, Mayor A, Tschopp J. The inflammasomes: guardians of the body. Annu Rev Immunol (2009) 27:229–65. doi: 10.1146/annurev.immunol.021908.132715
156. Coffman RL, Sher A, Seder RA. Vaccine adjuvants: putting innate immunity to work. Immunity (2010) 33:492–503. doi: 10.1016/j.immuni.2010.10.002
157. Gordon DM. Use of novel adjuvants and delivery systems to improve the humoral and cellular immune response to malaria vaccine candidate antigens. Vaccine (1993) 11:591–3. doi: 10.1016/0264-410x(93)90239-t
158. Garcon N, Van Mechelen M. Recent clinical experience with vaccines using MPL- and QS-21-containing adjuvant systems. Expert Rev Vacc (2011) 10:471–86. doi: 10.1586/erv.11.29
159. Hung IF, Zhang AJ, To KK, Chan JF, Li P, Wong TL, et al. Topical imiquimod before intradermal trivalent influenza vaccine for protection against heterologous non-vaccine and antigenically drifted viruses: a single-centre, double-blind, randomised, controlled phase 2b/3 trial. Lancet Infect Dis (2016) 16(2):209–18. doi: 10.1016/S1473-3099(15)00354-0
160. O’Hagan D T, Friedland LR, Hanon E, Didierlaurent AM. Towards an evidence based approach for the development of adjuvanted vaccines. Curr Opin Immunol (2017) 47:93–102. doi: 10.1016/j.coi.2017.07.010
161. Pedersen GK, Höschler K, Solbak SM, Bredholt G, Pathirana RD, Afsar A, et al. Serum IgG titres, but not avidity, correlates with neutralizing antibody response after H5N1 vaccination. Vaccine (2014) 32(35):4550–7. doi: 10.1016/j.vaccine.2014.06.009
162. Day TA, Penn-Nicholson A, Luabeya AK, Fiore-Gartland A, Du Plessis N, Loxton AG, et al. Safety and immunogenicity of the adjunct therapeutic vaccine ID93 + GLA-SE in adults who have completed treatment for tuberculosis: a randomised, double-blind, placebo-controlled, phase 2a trial. Lancet Respir Med (2021) 9(4):373–86. doi: 10.1016/S2213-2600(20)30319-2
163. Jenum S, Tonby K, Rueegg CS, Rühwald M, Kristiansen MP, Bang P, et al. A phase I/II randomized trial of H56:IC31 vaccination and adjunctive cyclooxygenase-2-inhibitor treatment in tuberculosis patients. Nat Commun (2021) 12(1):6774. doi: 10.1038/s41467-021-27029-6
164. Pleguezuelos O, Dille J, de Groen S, Oftung F, Niesters HG, Islam MA, et al. Immunogenicity, safety, and efficacy of a standalone universal influenza vaccine, FLU-v, in healthy adults: A randomized clinical trial. Ann Intern Med (2020) 172(7):453–62. doi: 10.7326/M19-0735
165. Yang JX, Tseng JC, Yu GY, Luo Y, Huang CY, Hong YR, et al. Recent advances in the development of toll-like receptor agonist-based vaccine adjuvants for infectious diseases. Pharmaceutics (2022) 14(2):423. doi: 10.3390/pharmaceutics14020423
166. Overton ET, Goepfert PA, Cunningham P, Carter WA, Horvath J, Young D, et al. Intranasal seasonal influenza vaccine and a TLR-3 agonist, rintatolimod, induced cross-reactive IgA antibody formation against avian H5N1 and H7N9 influenza HA in humans. Vaccine (2014) 32(42):5490–5. doi: 10.1016/j.vaccine.2014.07.078
167. Gottlieb SL, Johnston C. Future prospects for new vaccines against sexually transmitted infections. Curr Opin Infect Dis (2017) 30(1):77–86. doi: 10.1097/QCO.0000000000000343
168. Kester KE, Cummings JF, Ofori-Anyinam O, Ockenhouse CF, Krzych U, Moris P, et al. Randomized, double-blind, phase 2a trial of falciparum malaria vaccines RTS,S/AS01B and RTS,S/AS02A in malaria- naive adults: safety, efficacy, and immunologic associates of protection. J Infect Dis (2009) 200:337–46. doi: 10.1086/600120
169. Mallory RM, Formica N, Pfeiffer S, Wilkinson B, Marcheschi A, Albert G, et al. Safety and immunogenicity following a homologous booster dose of a SARS-CoV-2 recombinant spike protein vaccine (NVX-CoV2373): A secondary analysis of a randomised, placebo-controlled, phase 2 trial. Lancet Infect Dis (2022) 22(11):1565–76. doi: 10.1016/S1473-3099(22)00420-0
170. Brito LA, Malyala P, O’Hagan DT. Vaccine adjuvant formulations: a pharmaceutical perspective. Semin Immunol (2013) 25:130–45. doi: 10.1016/j.smim.2013.05.007
171. Calabro S, Tortoli M, Baudner BC, Pacitto A, Cortese M, O’Hagan DT, et al. Vaccine adjuvants alum and MF59 induce rapid recruitment of neutrophils and monocytes that participate in antigen transport to draining lymph nodes. Vaccine (2011) 29:1812–1823. doi: 10.1016/j.vaccine.2010.12.090
172. Calabro S, Tritto E, Pezzotti A, Taccone M, Muzzi A, Bertholet S, et al. The adjuvant effect of MF59 is due to the oil-in-water emulsion formulation, none of the individual components induce a comparable adjuvant effect. Vaccine (2013) 31:3363–9. doi: 10.1016/j.vaccine.2013.05.007
173. Didierlaurent AM, Collignon C, Bourguignon P, Wouters S, Fierens K, Fochesato M, et al. Enhancement of adaptive immunity by the human vaccine adjuvant AS01 depends on activated dendritic cells. J Immunol (2014) 193:1920–30. doi: 10.4049/jimmunol.1400948
174. Duewell P, Kisser U, Heckelsmiller K, Hoves S, Stoitzner P, Koernig S, et al. ISCOMATRIX adjuvant combines immune activation with antigen delivery to dendritic cells in vivo leading to effective cross-priming of CD8+ T cells. J Immunol (2011) 187:55–63. doi: 10.4049/jimmunol.1004114
175. Dendouga N, Fochesato M, Lockman L, Mossman S, Giannini SL. Cell-mediated immune responses to a varicella-zoster virus glycoprotein e vaccine using both a TLR agonist and QS21 in mice. Vaccine (2012) 30:3126–35. doi: 10.1016/j.vaccine.2012.01.088
176. Coccia M, Collignon C, Hervé C, Chalon A, Welsby I, Detienne S, et al. Cellular and molecular synergy in AS01-adjuvanted vaccines results in an early IFN-γ response promoting vaccine immunogenicity. NPJ Vaccines (2017) 2:25. doi: 10.1038/s41541-017-0027-3
177. Didierlaurent AM, Laupèze B, Di Pasquale A, Hergli N, Collignon C, Garçon N. Adjuvant system AS01: helping to overcome the challenges of modern vaccines. Expert Rev Vaccines (2017) 1:55–63. doi: 10.1080/14760584.2016.1213632
178. Detienne S, Welsby I, Collignon C, Wouters S, Coccia M, Delhaye S, et al. Central role of CD169+ lymph node resident macrophages in the adjuvanticity of the QS-21 component of AS01. Sci Rep (2016) 6:39475. doi: 10.1038/srep39475
179. Kool M, Pétrilli V, De Smedt T, Rolaz A, Hammad H, Van Nimwegen M, et al. Cutting edge: alum adjuvant stimulates inflammatory dendritic cells through activation of the NALP3 inflammasome. J Immunol (2008) 181:3755–9. doi: 10.4049/jimmunol.181.6.3755
180. Wilson NS, Duewell P, Yang B, Li Y, Marsters S, Koernig S, et al. Inflammasome-dependent and -independent IL-18 production mediates immunity to the ISCOMATRIX adjuvant. J Immunol (2014) 192:3259–68. doi: 10.4049/jimmunol.1302011
181. Welsby I, Detienne S, N’Kuli F, Thomas S, Wouters S, Bechtold V, et al. Lysosome-dependent activation of human dendritic cells by the vaccine adjuvant QS-21. Front Immunol (2017) 7:663. doi: 10.3389/fimmu.2016.00663
182. Caproni E, Tritto E, Cortese M, Muzzi A, Mosca F, Monaci E, et al. MF59 and Pam3CSK4 boost adaptive responses to influenza subunit vaccine through an IFN type I-independent mechanism of action. J Immunol (2012) 188:3088–98. doi: 10.4049/jimmunol.1101764
183. Cauwelaert ND, Desbien AL, Hudson TE, Pine SO, Reed SG, Coler RN, et al. TLR4 agonist vaccine adjuvant, GLA-SE, requires anonical and atypical mechanisms of action for TH1 anduction. PloS One (2016) . 11:e0146372. doi: 10.1371/journal.pone.0146372
184. Kamath AT, Valenti MP, Rochat AF, Agger EM, Lingnau K, von Gabain A, et al. Protective anti-mycobacterial T cell responses through exquisite in vivo activation of vaccine-targeted dendritic cells. Eur J Immunol (2008) 38:1247–56. doi: 10.1002/eji.200737889
185. Kamath AT, Rochat AF, Christensen D, Agger EM, Andersen P, Lambert PH, et al. A liposome-based mycobacterial vaccine induces potent adult and neonatal multifunctional T cells through the exquisite targeting of dendritic cells. PloS One (2009) 4:e5771. doi: 10.1371/journal.pone.0005771
186. Kamath AT, Mastelic B, Christensen D, Rochat AF, Agger EM, Pinschewer DD, et al. Synchronization of dendritic cell activation and antigen exposure is required for the induction of Th1/Th17 responses. J Immunol (2012) 188:4828–37. doi: 10.4049/jimmunol.1103183
187. Werninghaus K, Babiak A, Groß O, Hölscher C, Dietrich H, Agger EM, et al. Adjuvanticity of a synthetic cord factor analogue for subunit mycobacterium tuberculosis vaccination requires FcRgamma-Syk-Card9-dependent innate immune activation. J Exp Med (2009) 206:89–97. doi: 10.1084/jem.20081445
188. Kazmin D, Nakaya HI, Lee EK, Johnson MJ, van der Most R, Van Den Berg RA, et al. Systems analysis of protective immune responses to RTS,S malaria vaccination in humans. Proc Natl Acad Sci USA (2017) 114:2425–30. doi: 10.1073/pnas.1621489114
189. Sobolev O, Binda E, O'farrell S, Lorenc A, Pradines J, Huang Y, et al. Adjuvanted influenza-H1N1 vaccination reveals lymphoid signatures of age-dependent early responses and of clinical adverse events. Nat Immunol (2016) 17:204–13. doi: 10.1038/ni.3328
190. Desbien AL, Dubois Cauwelaert N, Reed SJ, Bailor HR, Liang H, Carter D, et al. IL-18 and subcapsular lymph node macrophages are essential for enhanced b cell responses with TLR4 agonist adjuvants. J Immunol (2016) 197:4351–9. doi: 10.4049/jimmunol.1600993
191. Bentebibel SE, Khurana S, Schmitt N, Kurup P, Mueller C, Obermoser G, et al. ICOS(+)PD-1(+)CXCR3(+) T follicular helper cells contribute to the generation of high-avidity antibodies following influenza vaccination. Sci Rep (2016) 6:26494. doi: 10.1038/srep26494
192. Fazilleau N, McHeyzer-Williams LJ, Rosen H, McHeyzer-Williams MG. The function of follicular helper T cells is regulated by the strength of T cell antigen receptor binding. Nat Immunol (2009) 10:375–84. doi: 10.1038/ni.1704
193. Heath PT, Galiza EP, Baxter DN, Boffito M, Browne D, Burns F, et al. Safety and efficacy of NVX-CoV2373 covid-19 vaccine. N Engl J Med (2021) 385(13):1172–83. doi: 10.1056/nejmoa2107659
194. Sacks HS. The novavax vaccine had 90% efficacy against COVID-19≥ 7 d after the second dose. Ann Internal Med (2021) 174(11):JC124. doi: 10.7326/acpj202111160-124
195. Keech C, Albert G, Cho I, Robertson A, Reed P, Neal S, et al. Phase 1–2 trial of a SARS-CoV-2 recombinant spike protein nanoparticle vaccine. N Engl J Med (2020) 383(24):2320–32. doi: 10.1056/nejmoa2026920
196. Datoo MS, Natama MH, Somé A, Traoré O, Rouamba T, Bellamy D, et al. Efficacy of a low-dose candidate malaria vaccine, R21 in adjuvant matrix-m, with seasonal administration to children in Burkina Faso: a randomised controlled trial. Lancet (2021) 397(10287):1809–18. doi: 10.1016/s0140-6736(21)00943-0
197. Magnusson SE, Reimer JM, Karlsson KH, Lilja L, Bengtsson KL, Stertman L. Immune enhancing properties of the novel matrix-m™ adjuvant leads to potentiated immune responses to an influenza vaccine in mice. Vaccine (2013) 31(13):1725–33. doi: 10.1016/j.vaccine.2013.01.039
198. Newman MJ, Wu JY, Gardner BH, Munroe KJ, Leombruno D, Recchia J, et al. Saponin adjuvant induction of ovalbumin-specific CD8+ cytotoxic T lymphocyte responses. J Immunol (1992) 148(8):2357–62. doi: 10.4049/jimmunol.148.8.2357
199. Wu JY, Gardner BH, Murphy CI, Seals JR, Kensil CR, Recchia J, et al. Saponin adjuvant enhancement of antigen-specific immune responses to an experimental HIV-1 vaccine. J Immunol (1992) 148(5):1519–25. doi: 10.4049/jimmunol.148.5.1519
200. Mosca F, Tritto E, Muzzi A, Monaci E, Bagnoli F, Iavarone C, et al. Molecular and cellular signatures of human vaccine adjuvants. Proc Natl Acad Sci USA (2008) 105:10501–6. doi: 10.1073/pnas.0804699105
201. Olafsdottir TA, Lindqvist M, Nookaew I, Andersen P, Maertzdorf J, Persson J, et al. Comparative systems analyses reveal molecular signatures of clinically tested vaccine adjuvants. Sci Rep (2016) 6:39097. doi: 10.1038/srep39097
202. Rappuoli R, Medaglini D. ADITEC: joining forces for next-generation vaccines. Sci Transl Med (2012) 4:128cm4. doi: 10.1126/scitranslmed.3003826
203. Kwissa M, Nakaya HI, Oluoch H. Pulendran B.Distinct TLR adjuvants differentially stimulate systemic and local innate immune responses in nonhuman primates. Blood (2012) 119:2044–55. doi: 10.1182/blood-2011-10-388579
204. Vaccari M, Gordon SN, Fourati S, Schifanella L, Liyanage NP, Cameron M, et al. Adjuvant-dependent innate and adaptive immune signatures of risk of SIVmac251 acquisition. Nat Med (2016) 7:762–70. doi: 10.1038/nm.4105
205. Chung AW, Ghebremichael M, Robinson H, Brown E, Choi I, Lane S, et al. Polyfunctional fc-effector profiles mediated by IgG subclass selection distinguish RV144 and VAX003 vaccines. Sci Transl Med (2014) 6:228ra38. doi: 10.1126/scitranslmed.3007736
206. Chung A, Kumar M, Arnold K, Yu W, Schoen M, Dunphy L, et al. Dissecting polyclonal vaccine-induced humoral immunity against HIV using systems serology. Cell (2015) 163:988–98. doi: 10.1016/j.cell.2015.10.027
207. Della Cioppa G, Jonsdottir I, Lewis D. Challenges in early clinical development of adjuvanted vaccines. Vaccine (2015) 33(Suppl 2):B47–51. doi: 10.1016/j.vaccine.2015.02.031
208. Brito LA, O'Hagan DT. Designing and building the next generation of improved vaccine adjuvants. J Control Release (2014) 190:563–79. doi: 10.1016/j.jconrel.2014.06.027
209. Nakaya HI. Pulendran B.Vaccinology in the era of high- throughput biology. Philos Trans R Soc Lond B Biol Sci (2015) 370:20140146. doi: 10.1098/rstb.2014.0146
210. Olafsdottir T, Lindqvist M, Harandi AM. Molecular signatures of vaccine adjuvants. Vaccine (2015) 33:5302–7. doi: 10.1016/j.vaccine.2015.04.099
211. Panilaitis B, Johri A, Blank W, Kaplan D, Fuhrman J. Adjuvant activity of emulsan, a secreted lipopolysaccharide from. Clin Diagn. Lab Immunol (2002) 9(6):1240–7. doi: 10.1128/cdli.9.6.1240-1247.2002
212. Sarkar I, Garg R, van Drunen Little-van den Hurk S. Selection of adjuvants for vaccine targeting specific pathogens. Expert Rev Vaccines (2019) 18(5):505–21. doi: 10.1080/14760584.2019.1604231
213. Kardani K, Bolhassani A. Shahbazi S.Prime-boost vaccine strategy against viral infections: Mechanisms and benefits. Vaccine (2016) 34:413–23. doi: 10.1016/j.vaccine.2015.11.062
214. Omosa-Manyonyi G, Mpendo J, Ruzagira E, Kilembe W, Chomba E, Roman F, et al. A phase I double blind, placebo-controlled, randomized study of the safety and immunogenicity of an adjuvanted HIV-1 gag-Pol-Nef fusion protein and adenovirus 35 gag-RT-Int-Nef vaccine in healthy HIV-uninfected African adults. PloS One (2015) . 10:e0125954. doi: 10.1371/journal.pone.0125954
215. Akhtar M, Nizam NN, Basher SR, Hossain L, Akter S, Bhuiyan TR, et al. dmLT adjuvant enhances cytokine responses to T cell stimuli, whole cell vaccine antigens and lipopolysaccharide in both adults and infants. Front Immunol (2021) 12:654872. doi: 10.3389/fimmu.2021.654872
Keywords: improved vaccines, adjuvants, mucosal vaccine, immune response, infectious disease
Citation: Verma SK, Mahajan P, Singh NK, Gupta A, Aggarwal R, Rappuoli R and Johri AK (2023) New-age vaccine adjuvants, their development, and future perspective. Front. Immunol. 14:1043109. doi: 10.3389/fimmu.2023.1043109
Received: 13 September 2022; Accepted: 26 January 2023;
Published: 24 February 2023.
Edited by:
Denise L. Doolan, James Cook University, AustraliaReviewed by:
Yongjun Sui, National Cancer Institute (NIH), United StatesSlim Fourati, Emory University, United States
Copyright © 2023 Verma, Mahajan, Singh, Gupta, Aggarwal, Rappuoli and Johri. This is an open-access article distributed under the terms of the Creative Commons Attribution License (CC BY). The use, distribution or reproduction in other forums is permitted, provided the original author(s) and the copyright owner(s) are credited and that the original publication in this journal is cited, in accordance with accepted academic practice. No use, distribution or reproduction is permitted which does not comply with these terms.
*Correspondence: Atul Kumar Johri, akjohri14@yahoo.com; Shailendra Kumar Verma, skverma@lji.org
†Present Addresses: Shailendra Kumar Verma, Center for Infectious Disease and Vaccine Research, La Jolla Institute for Immunology, La Jolla, CA, United States
Rino Rappuoli, Fondazione Biotecnopolo di Siena, Siena, Italy