- Department of Research and Development, Premas Biotech Pvt Ltd., Sector IV, Industrial Model Township (IMT), Manesar, Gurgaon, India
Viral infectious diseases threaten human health and global stability. Several vaccine platforms, such as DNA, mRNA, recombinant viral vectors, and virus-like particle-based vaccines have been developed to counter these viral infectious diseases. Virus-like particles (VLP) are considered real, present, licensed and successful vaccines against prevalent and emergent diseases due to their non-infectious nature, structural similarity with viruses, and high immunogenicity. However, only a few VLP-based vaccines have been commercialized, and the others are either in the clinical or preclinical phases. Notably, despite success in the preclinical phase, many vaccines are still struggling with small-scale fundamental research owing to technical difficulties. Successful production of VLP-based vaccines on a commercial scale requires a suitable platform and culture mode for large-scale production, optimization of transduction-related parameters, upstream and downstream processing, and monitoring of product quality at each step. In this review article, we focus on the advantages and disadvantages of various VLP-producing platforms, recent advances and technical challenges in VLP production, and the current status of VLP-based vaccine candidates at commercial, preclinical, and clinical levels.
1 Introduction
Traditional vaccines, such as inactivated or live-attenuated, have shown protective efficacy against several infectious diseases that are caused due to viral infections. However, they sometimes have safety concerns and deviations in the protective immune response. Recent advances in nucleic acid-based, subunit, and recombinant viral vector-based vaccines may overcome the limitations of traditional vaccines against infectious pathogens by enhancing immunity without compromising safety, efficacy, and tolerability and can also control infectious diseases during outbreaks or pandemics. The significance of such vaccine approaches in rapidly developing vaccine candidates for pandemic threats has been previously reported and is considered an approach for pandemic preparedness and response (1). Virus-like particles (VLP) differ from other subunit vaccines in that they have strong immunogenicity because they present repetitive antigenic epitopes to the immune system in more authentic confirmation and move in parallel with viral vector-based vaccines to produce protective, effective, and efficient vaccines against severe infectious diseases (2).
VLPs are non-infectious multiprotein structures that mimic native viruses but lack the disease-causing viral genome, making them safer vaccine candidates. Because of their optimal size (20–200 nm), they can practically display any epitope in a multivalent format that guarantees a high humoral or cell-mediated immune response in the host.
VLPs are categorized into enveloped and non-enveloped based on the presence or absence of lipid envelopes. Furthermore, these particles can be classified as homologous or heterologous according to their composition. Homologous VLPs contain proteins derived only from the native viruses. On the other hand, heterologous VLPs contain proteins from different sources, which increases their immunogenicity (3). They are further classified as single- or multi-capsid proteins. Simple VLPs are composed of a single capsid protein that is expressed in both eukaryotic and prokaryotic systems. In contrast, multi-capsid proteins show several striking structural characteristics, including many complicated concentric layers of different capsid proteins. Multi-capsid VLPs are usually produced in eukaryotic expression systems such as yeasts, insect cells, and plants. For example, various combinations of four different rotaviral capsid proteins can produce stable VLPs with double or triple capsid layers (4). Complex multi-capsid VLPs present higher production challenges. In this case, each protein should be expressed in stoichiometric amounts for efficient production because of limited cellular resources, as the expression of excess monomers does not improve VLP assembly (5). Enveloped VLPs (eVLPs) are poorly characterized at the biophysical level because they are structurally less uniform than non-enveloped VLPs. Technical challenges remain in the generation of eVLP-based vaccines in their design, treatment, and storage. Proper folding and glycosylation of viral surface antigens are important for defining the efficacy of eVLP-based vaccines because they are critical for stability, immune recognition, and pathogenicity. In general, eVLPs with host-derived envelopes are more sensitive to the external environment than non-enveloped VLP. Changes in conditions, such as temperature, shear force, and purification processes, can destroy the integrity and stability of particles, reducing eVLP immunogenicity. However, computational design, in vitro assembly of VLPs, removal or replacement of the transmembrane region, introduction of a suitable heterologous signal peptide, new bioprocessing modalities, and new macro-scale purification materials can address these problems robustly, at the expense of process complexity (6).
VLPs carry pathogen associated molecular patterns (PAMP), which are sensed by pathogen recognition receptors (PRRs) present either on the cell surface or endosomes of dendritic cells (DC). Presentation of VLP-peptides along with major histocompatibility complex (MHC) class I or class II molecules by DC induces CD8+ or CD4+ T cell responses, respectively (7). Simultaneously, co-stimulatory molecules present on DC activate T-helper (Th) cells, which are required for both cell-mediated and humoral immunity. In some cases, B cells are activated without innate immunity or any direction by Th cells (6). The addition of more immunogenic domains in VLP could further enhance their potency and efficacy, as seen in the case of hepatitis B core antigen (HBcAg). HBcAg-based VLP vaccines contain more immunogenic domains of hepatitis B surface antigen (HBsAg) and stimulate broad and specific humoral and cell-mediated responses against hepatitis B virus (HBV) (8). Because VLPs elicit a protective response at lower doses, they can significantly reduce vaccine costs (9).
Several platforms have been used for VLP production. The choice of the preferred platform provides flexibility in the manufacturing conditions required for the scaled-up production of VLP vaccines (7). Remarkably, VLPs can be recombinantly produced by expressing either a single type of coat protein, multiple structural proteins, or a combination of structural and nonstructural proteins required for VLP production. Examples include the first commercial VLP-based vaccine licensed for the HBV (9). To date, hundreds of VLP-based vaccine candidates have been studied for immunization against various human and animal diseases. However, only a few have been commercialized to target non-enveloped viruses. The structure of non-enveloped VLPs consists of either a single or multiple structural protein(s) of a specific virus and is relatively simple. In contrast, eVLPs have host cell components that pose potential challenges to their regulatory approval, scalability, and stability (10). Both types of VLPs can be engineered to form chimeric VLP, which display foreign antigens to enhance immune response and can also be used as nanocarriers for the delivery of desired antigens or therapeutic component of a molecule (11).
This article briefly presents the merits and demerits associated with various platforms, recent advances, and technical challenges in VLP production. The current scenario of VLP-based vaccine candidates at commercial, clinical, and preclinical levels are also summarized.
2 Expression platform for VLPs
VLP-based vaccines have been developed as the next-generation vaccines. However, to ensure optimal protein folding, post-translational modifications (PTMs), cost-effectiveness, and scale-up production of these vaccines in a timely manner, an appropriate platform must be selected. VLPs can be experimentally generated in the laboratory using recombinant viral proteins that are expressed in a range of expression systems including prokaryotic cells, yeast, insect cell lines, plants and mammalian cell lines. In addition, cell-free expression techniques have been used successfully. In these cases, VLP proteins are expressed first in a cell-based expression system, then assembled in a cell-free environment for proper folding (6). All systems have their own advantages and drawbacks, which are briefly listed in Table 1.
The optimal expression of each VLP is usually identified via trial-and-error by comparing the translated products of multiple expression systems. Expression in E. coli is often preferred for producing small proteins with limited PTMs; however, PTMs of larger proteins require a more complex expression system, such as yeast, baculovirus, and mammalian cells. Compared to higher eukaryotes, yeasts are considered a cost-effective production system. In addition, yeasts avoid endotoxin and viral contamination problems associated with bacterial and mammalian expression systems (13). Engerix-B (HBV vaccine) and Gardasil (Human Papilloma Virus (HPV) vaccine) are two FDA-approved vaccines that have been generated in yeast expression systems. Despite these achievements, the lack of complex PTMs is a major drawback of yeasts, which limits their use in generating non-enveloped VLPs. In contrast, animal cell expression platforms are attractive because of their ability to make complex and precise PTMs that are essential for proper protein folding, which can be used to produce multiple structural proteins of non-enveloped and enveloped VLPs (6).
Due to the ease and speed of baculovirus-based VLP expression, this system is suitable for manufacturing vaccines against viruses that rapidly change their surface antigens between outbreaks, such as influenza virus (6, 14). Cervarix is an FDA-approved HPV vaccine produced using this expression system. The baculovirus/insect cell platform has also been used as a prophylactic vaccine candidate against several infectious diseases such as human immunodeficiency virus (HIV) 1, influenza virus A, chikungunya virus, severe acute respiratory syndrome (SARS), Ebola virus, dengue fever virus, Rift Valley fever virus (RVFV), Norwalk virus, and hepatitis C virus (HCV) (6, 15). The major drawback of the baculovirus/insect cell platform is its simpler N-glycosylation pattern compared to that of mammalian cells, which can be a disadvantage for some VLP applications.
VLP production in transgenic plants has several interesting applications. For example, although plant cells lack mammalian-like PTMs, plant-specific glycosylation can have an immunostimulatory effect (13). Plants have been used for VLP production of the Norwalk virus (16, 17), HIV-1 (18), and influenza virus VLPs (Medicago). Another recently developed expression platform is the cell-free system, which usually consists of extracts from E. coli or yeast cells. This system is preferred for the production of viral capsid proteins with toxic intermediate forms. Owing to the non-replenishing nature of a cell-free system, this method is highly demanding, with scalability limitations. Examples of commercialized VLP vaccines produced in a cell-free expression system include the influenza vaccine Inflexal V (19) and the hepatitis A vaccine Epaxal (20).
3 Culture mode used for the production of multimeric VLP
Three culture modes were used to produce the VLPs: batch, fed-batch, and continuous cultivation. Process design is of crucial importance because it influences yield, productivity, and final product concentration. The cost of raw materials can be decreased by maintaining a yield close to the maximum theoretical value. Simultaneously, an increase in high product concentration and productivity, and a reduction in non-productive periods can reduce the cost of downstream processing and contribute to the efficient use of manufacturing equipment. Owing to its simplicity and low risk of contamination, the batch mode is preferred for many industrial processes. In batch culture, all media components are added to the bioreactor at the start of the cultivation process, with no extra nutrients supplied until the end. However, the maximum potential of this process is often not achieved. Substrate and product inhibition, low virus titers, temperature sensitivity, low productivity (21) and ineffective use of time between batches are major problems in the batch mode. For efficient performance of batch fermentation, all non-productive steps (such as cleaning and filling of bioreactor, media formulation, etc.) should be minimized while targeting the maximum yield of the end product (22). This approach has been used to test a variety of VLPs, including HIV (23), chikungunya virus (24) and Ebola (25). In a fed-batch process, substrate inhibition is avoided by reducing the lag phase, while correctly designed feeding of concentrated nutrients increases product concentration and yield. The rate of the reaction is determined by the amount of limiting nutrients in the feed medium. In a baculovirus/insect cell expression system, this culture mode was tested for the production of parvovirus-like particles (26) and recombinant HBsAg (27). The highest productivity is achieved in continuous mode because the culture is in the log phase. In this mode, fresh medium is supplied and the conditioned medium is extracted. Continuous production is obtained using this method, and the product must be stored under proper conditions while being produced. This approach has been used for producing rabies VLPs in HEK293 cells (28). There are many types of continuous modes such as chemostat, turbidostat, stressostat, and morbidostat. Chemostat is the most widely used continuous cultivation method (29). It is possible to control the specific growth rates and product formation in a chemostat by changing the concentration of the limiting substrate for a specific strain. However, the increased risk of contamination, high volumes of low-concentration broth from the system, and low product concentrations are problematic (30). The fermentation process should be selected by considering the advantages and disadvantages of each process. The major criterion for the selection of a bioreactor/fermentation process is the minimal capital cost per unit product yield. For example, the design of a continuous production process for measles virus is more challenging because of its size (300 nm – 1 μm) and lysis of host cells. Cell lysis leads to rapid accumulation of host cell proteins (HCPs) and cell debris in the surrounding medium. Therefore, filtration during cultivation is challenging because the filter pores are blocked by debris or HCPs. Discontinuous filtration or repeated batch mode is more appropriate for this virus (21, 31). The corresponding yields from the different culture modes are summarized in Table 2.
The bioreactors used in the manufacturing of VLP-based vaccines are made of stainless-steel vessels. However, single-use technology is gaining importance since it eliminates the need for in-situ cleaning and sterilizing, which reduces cross-contamination, investment, and operational costs. However, single-use vessels are less useful (14). Each of the three culture modes have several advantages and disadvantages (Table 3).
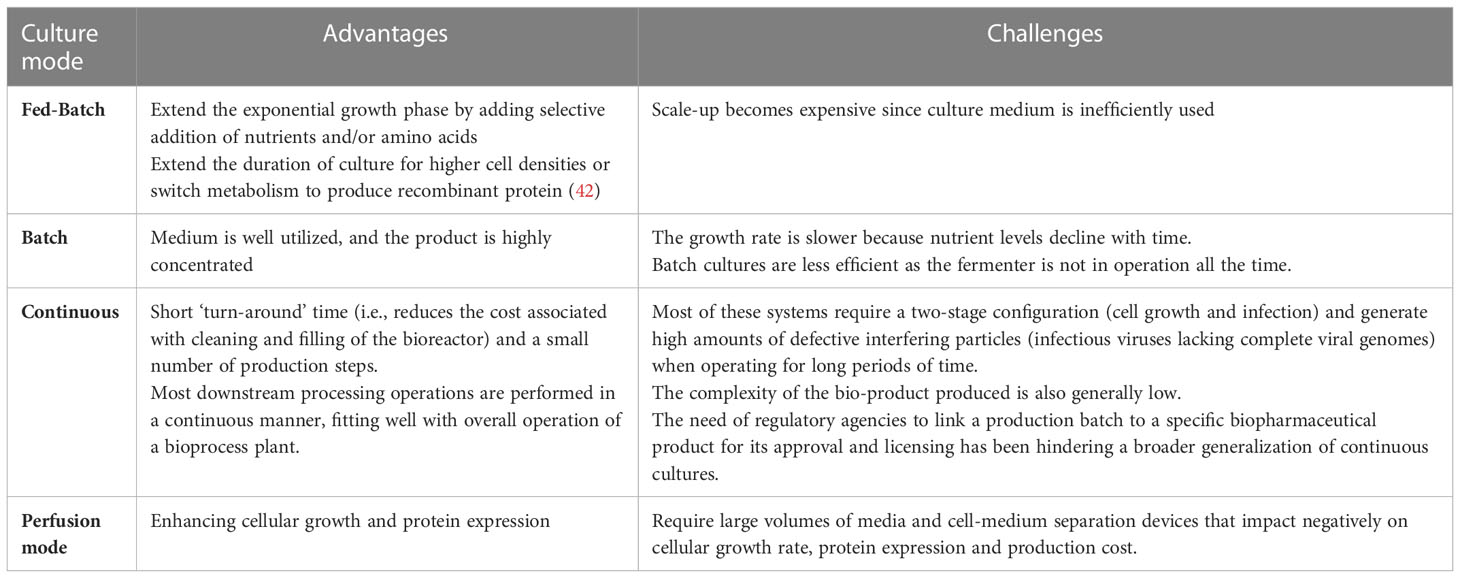
Table 3 Advantages/challenges associated with the various culture modes used for the production of VLPs.
4 Recombinant DNA technology applied to different host systems for VLP vaccine production
Bacterial systems (mainly E. coli) are used to produce approximately 28% of all VLPs (43). In these systems, viral genes are codon-optimized for bacteria and cloned into commercial plasmids with strong promoters to generate higher yields and easy initial purification (44). However, a major drawback of these systems is their inability to induce post-translational glycosylation in the eukaryotic proteins and the endotoxins created during production of recombinant proteins. Little progress has been made in introducing glycosylation into E. coli-based cell-free systems. Asparagine-linked glycosylation is the most general and universal PTMs that determines the folding, stability, and catalytic activity of a recombinant protein. Protein glycosylation relies on the activity of pglB oligosaccharyltransferase (Pilin glycosylation B OTase), which transfers oligosaccharides to N-linked asparagine, thereby facilitating glycosylation (45, 46). In Campylobacter jejuni, a single pglB gene encodes all enzymes required for the biosynthesis of N-linked glycans. The promiscuity of C. jejuni PglB OTase has exhibited efficiency in E. coli (47). Subsequently, a glycoengineered E. coli strain, CLM24, was developed to express both Cj PglB OTase and Cj lipid-linked oligosaccharides (48, 49). The glycosylation components of the cell-free glycoprotein synthesis (CFGpS) system do not require a purification step and thus are easy to use and cost-efficient (48). The CFGpS system is the first prokaryotic cell-free system capable of cell-free transcription, translation, and glycosylation of proteins. Recently, a glycolic platform was developed that facilitates the glycosylation of VLPs in the E. coli cytoplasm (50). This glycolic platform uses the asparagine glucosyltransferase of Actinobacillus pleuropneumoniae, which is expressed in E. coli and catalyzes the transfer of single beta-linked glucose onto recombinant proteins. Similarly, to overcome the drawbacks of endotoxins, several genes such as LPS that participate in the expression of endotoxins are interrupted to eliminate endotoxin contamination and produce better recombinant proteins in E. coli, as shown in the case of the production of a 9-valent HPV vaccine candidate in E. coli ER2566 strain using a chromosomal integration approach, with markedly reduced residual endotoxin levels (51).
Insect, yeast, and mammalian platforms have also been used for the production of VLPs since the 1980s (43). Recombinant insect cells are a promising alternative platform to the baculovirus–insect cell system because target proteins can be produced simply by culturing the cells (52). For example, the secretory form of influenza VLPs consisting of hemagglutinin (HA) and matrix proteins was successfully produced by recombinant insect cells (53). Manipulating genes in yeasts is comparatively straightforward, and transformed cells can easily grow to extremely high densities. Therefore, VLPs can be produced in large volumes using commercial-scale fermenters. Among the many yeast species, Saccharomyces cerevisiae and Pichia pastoris have emerged as the most promising candidates for developing VLP-based vaccines. Noticeable changes in the production of VLP-based vaccines involve recombinant strategies that induce the secretion and production of multilayered VLPs, which are typically composed of more than one type of structural protein. For example, co-expression of four structural proteins in yeast expression systems effectively produces enterovirus 71 (EV71) VLPs (54). EV71 causes hand, foot, and mouth disease (HFMD), which is associated with severe neurological complications. VLP-based vaccines have shown great potential for preventing EV71 infections. Moreover, there have been a number of attempts to produce VLPs using new expression hosts, such as Hansenula polymorpha (methylotrophic yeast) is particularly striking (55). H. polymorpha has several unique characteristics such as thermostability, avoidance of hyperglycosylation, and use of several carbon sources (56). This platform has been established for the cost-effective production of VLPs at a larger scale (40 mg/100 g) (57).
Several mammalian cells, such as Chinese hamster ovary (CHO), HEK293, amniotic fluid CAP-T, and Vero cells, are used to produce VLPs (2, 14). These cells can produce complex and accurate post-translational modifications, and therefore, are preferred for the production of complex eVLPs containing multiple structural proteins. CHO cells are not human-derived; thus, compared with other mammalian cells, they have the advantage of a lower risk of contamination by human viruses. However, low yield (0.018–10 μg/mL) (14), difficulty in scale-up, and high cost are several factors that need attention. It has been shown that the CHO‐derived viral‐like particles for hepatitis B include a combination of glycosylated and non‐glycosylated HBsAg proteins, similar to those in patients’ sera. Examples of CHO‐based vaccines are GenHevac B® and Sci‐B‐Vac™. These vaccines contain the HBsAg small and middle proteins (GenHevac B) or middle and large proteins (Sci‐B‐Vac) (58, 59). Similarly, hantavirus VLPs have been designed to co-express the nucleocapsid protein and glycoproteins (Gn and Gc) in CHO cells. These VLPs produce both humoral and cell-mediated immune responses against hantavirus (60). However, CHO-derived VLPs may be contaminated with HCPs. Certain HCPs, if not removed during subsequent purification processes, have been shown to induce immunogenic responses in patients. Others can shorten the shelf life of VLP vaccines via various mechanisms, including polysorbate degradation. Anti-CHO antibodies have been identified in 54% of individuals with no history of exposure to protein therapeutics (61). Therefore, a reduction in HCPs to minimal levels is required. While most HCPs are removed during downstream purification steps, certain ‘difficult-to-remove’ HCPs often remain (62). Additionally, stable transfection of mammalian cells is time-consuming, transient transfection has been used to produce VLPs in several studies. For example, Wu et al. demonstrated the effective production of influenza VLPs resembling original viruses in size, structure, glycosylation, and host factor composition (63). Similarly, severe acute respiratory syndrome coronavirus-2 (SARS-CoV-2) VLPs was constructed by co-expressing four SARS-CoV-2 structural proteins in Vero E6 cells making it stable and unified, as compared to those from HEK293T cells.
5 Technical challenges in VLP production
5.1 Challenges in the transduction related parameters
Cell concentration at infection (CCI), multiplicity of infection (MOI), and time of harvest (TOH) in the baculovirus system strongly influence protein expression and, consequently, assembly of VLP. 72–120 h post-infection is considered the optimal time of harvest. Outside this period, various parameters can hinder productivity, such as cell lysis, protein degradation, and difficulties in downstream processing owing to the presence of contaminant proteins, hosts, or viral DNA. Incomplete VLP may also be released into the medium along with target mature VLP and can hinder the downstream processing of VLP (64–66). Differences in volumetric productivity, either due to low or high MOI, can be counterbalanced by modulating CCI. However, CCI is inversely correlated with productivity. Although perfusion or fed-batch techniques may overcome these limitations, they are neither practical nor economically feasible. Therefore, the development of novel techniques for process optimization is required.
5.2 Challenges in VLP assembly
The assembly of VLPs involves assembling single or multiple structural capsid proteins in appropriate production hosts (in-vivo assembly) or in cell-free conditions (in-vitro assembly). The nature of the stabilizing contacts can influence the VLP assembly route (67). Protein-protein interactions protect the viral genetic material from the degradation and facilitate the establishment of a relatively stable carrier/delivery system. Similarly, viral genomes coevolution with their own structural elements has resulted in interactions in which nucleic acids are the primary driving mechanism for VLP formation. For example, the formation of HCV VLP is dependent on the interaction of highly basic N-terminal 120 (68)or 124 (69) amino acids of the core protein with a 5′ untranslated region containing expected secondary structural elements. The assembly and disassembly of VLPs may also depend on the ionic or reducing conditions in which they are assembled. In more complex viruses, scaffolding proteins play an essential structural role in ensuring correct assembly of large viruses. For example, P22a is a critical scaffolding protein in the particle assembly of the herpes simplex virus (70). Assembly is not usually a single high‐order reaction, but can sometimes include a cascade of low‐order intermediate reactions. These intermediate reactions at various phases of assembly can be examined or trapped by mutagenesis of structural proteins and their expression in a suitable system. The uniformity and self-assembly of VLPs, along with their ability to withstand chemical modifications primarily on the outer surface, make them flexible and stable alternatives to nanoparticles, such as liposomes and metal assemblies (1, 8).
Self-assembly of VLPs is driven by interactions between capsid proteins and often with the viral genome. For example, approximately 25% of the HBsAg monomers in the RTS,S (Mosquirix™; the first vaccine for malaria) are genetically fused to the truncated circumsporozoite protein (CSP) and serve as protein carriers. The region of the CSP included in the RTS,S vaccine includes immunodominant B-cell epitopes (last 18 four amino acids (NANP) repeats) and three known T-cell epitopes: a highly variable CD4+ T-cell epitope before the Thrombospondin (TSP)-like domain, a highly variable CD8+ T-cell epitope within the TSP-like domain, and a conserved “universal” CD4+ T cell epitope at the C-terminus. These epitopes of Mosquirix enhanced antigen presentation to the immune system and likely facilitated strong anti-CSP antibody and T-cell responses measured in vaccinated individuals. Mosquirix has received approval from the regulatory authorities (71, 72).
To obtain superior assembly effects, cell-free or in vitro assembly of VLPs requires control of variables such as temperature, pH, and specific assembly solution (73). The assembly of VLPs in vivo necessitates a suitable thermodynamic environment for the host, which might result in the formation of malformed structures and pose technical challenges in VLP assembly and production. In this context, thermodynamic studies may be useful for identifying the most suitable environmental conditions for production during upstream processing as well as for maintaining stability throughout downstream processing (DSP) and storage. Various studies have demonstrated the effects of several physicochemical parameters (pH, ionic strength, temperature, and correct stoichiometric ratio) on the formation of VLPs (74, 75). When optimal conditions are not available, VLP destruction increases considerably. Additionally, owing to their large structure, VLPs may occasionally lose their self-assembling property or cause protein misfolding, resulting in the formation of defective VLPs (76). Time is another factor that renders antigen assembly laborious. Therefore, optimization is essential for appropriate VLP assembly and should be investigated further.
5.3 Challenges in upstream processing
Several process parameters, such as dissolved oxygen concentration, pH, temperature, agitation rate, inlet gas flow, and composition, influence VLP production and pose technical challenges either by hindering the cell growth or metabolic state of the cells or by interfering with the mechanisms responsible for post-translational modification. Limited or excess oxygen induces proteases that can degrade the product of interest (77). When oxygen-derived free radicals are formed owing to a high concentration of dissolved oxygen, they either induce oxidative stress in cells or cause oxidative damage to proteins (78). Temperature is another parameter that can control oxygen solubility (79).
The use of headspace aeration (bubble-free systems) or nonionic copolymers may provide a solution depending on the degree of sensitivity of the cells (77, 79–81). Cellular damage can be eliminated in a bubble-free system, but productivity decreases due to inadequate levels of dissolved oxygen. However, nonionic copolymers do not interfere with dissolved oxygen. These copolymers lower the surface tension of the culture medium and increase cell membrane rigidity, thereby inhibiting the attachment of cells to bubbles and increasing cell resistance to hydrodynamic forces.
5.4 Challenges in downstream processing of VLPs
The separation of a complex mixture of proteins from VLP using transduction is challenging because of their similar size and molecular weight. The same problem does not exist in the case of transfection, which thus, significantly decreases the complexity of downstream processing. Serum-free medium further improves or facilitates downstream processing because it does not contain any animal-derived supplements. Controlling various process conditions, such as optimal salt, pH, and ion concentrations, can reduce or avoid protein aggregation, which is a major challenge in downstream processing. However, aggregation during large-scale production of VLPs, owing to their larger size, leads to the loss of a considerable portion of the target protein or reduced immunogenicity (82). In a recent study, a new membrane chromatography procedure was developed to produce nano-VLPs against Ebola. This method reduces VLP size to a more manageable range, where it can not only retain its temperature stability and antigen structure, but also stimulate immunity in mice. In addition, lyophilization can be used to increase its thermostability. Lyophilization and freeze-drying are used worldwide. However, they are especially effective for tropical countries that do not have a reliable “cold-chain” of refrigeration (83). A patent was filed for the methodology against the Ebola virus in 2015 (US20170274063A1); however, in 2017, it was abandoned. Tangential flow filtration, gel permeation, ion exchange, size exclusion chromatography, and mixed-mode chromatography, including the use of improved disposable membrane technologies, can improve the productivity of purified VLP (84–86).
5.5 Challenges in biochemical and biophysical characterization of VLPs
The biochemical characterization of VLPs is usually performed using various techniques such as sodium dodecyl sulfate (SDS)- polyacrylamide gel electrophoresis, western blotting (WB), enzyme-linked immunoassay (ELISA), and bicinchoninic acid (BCA) protein quantification assays. Flow cytometry is now being exploited as a tool for the study and validation of VLPs (87). However, these techniques cannot differentiate between unassembled and partially or completely assembled VLPs and are time-consuming, have low sensitivity, and require high sample volumes. This leads to either the over-or underestimation of proteins, compromising downstream processing. Some of the issues mentioned above have been effectively visualized and addressed using Transmission electron microscopy (TEM). Particle size analysis using light-scattering techniques is commonly adopted to measure the hydrodynamic size and homogeneity (polydispersity) of VLPs in solution. Additionally, orthogonal methods, such as high-performance liquid chromatography (HPLC), SDS-capillary gel electrophoresis, MALDI-TOF MS, and capillary zone electrophoresis, have been developed to overcome the limitations posed by SDS-PAGE, WB, ELISA etc. Two-dimensional fluorometry is an optical fiber-based technology that can monitor several compounds, either present outside or inside cells. Therefore, in situ monitoring of the kinetics of VLPs is possible using this technology (88–93).
5.6 Challenges in critical quality attributes in VLP production
Critical Quality Attributes (CQAs), such as the biophysical, biochemical, structural, and immunochemical properties of VLPs, are critical parameters in developing vaccine candidates with good stability and delivering consistent products for the preclinical and clinical stages. In addition, these parameters also help in post-licensure life-cycle management to support market demand by upgrading the process or scale-up.
Various methods are used to characterize the physical and structural attributes of VLPs, such as transmission electron microscopy (TEM), Atomic force microscopy (AFM) for morphology, size-exclusion chromatography-high performance liquid chromatography (SEC-HPLC) for monodispersity (94), dynamic light scattering (DLS), cloud point, surface plasmon resonance (SPR) to check propensity of aggregation and circular dichroism (CD) (95) and differential scanning calorimetry (DSC) to check thermal stability (96, 97). However, it should be noted that drying of samples is required during TEM or AFM, which can introduce artifacts caused by the dehydration of VLPs. Cryo-EM can overcome the drawbacks of TEM and AFM because it preserves the VLP structure in its native form. However, cryo-EM is only useful if the VLP preparation is sufficiently homogeneous, although a pleomorphic nature can also be observed. Similarly, the AFM in the solution also reduced the drying artifacts. Another major challenge in VLP characterization is accurate measurement or quantification of VLPs to derive an accurate dose. Nanoparticle tracking analysis (NTA)/tunable resistive pulse sensing (TRPS) (98), electrospray-differential mobility analysis (ES-DMA) (99, 100) and asymmetric flow field flow fractionation with multi-angle light scattering detection (AF4-MALS) (100, 101) have been used to accurately measure the size distribution accurately. These methods are cost-efficient compared to TEM and are used to quantitatively monitor batch consistency for new vaccines.
Activity-related attributes are also important for checking the structure–function relationship in VLP-based vaccines or the integrity of key epitopes. Tools such as bilayer interferometry (BLI) and SPR are used to check epitope-specific antigenicity and epitope mapping (102). In vitro relative potency or mouse potency assays are used to test the release and stability of VLPs. These assays are required to define the quality and quantity of epitopes on recombinant antigens. Therefore, they play a major role in the implementation of process improvements or scale-up.
Additionally, expressing VLPs in a suitable host to provide an appropriate environment and post-purification disassembly/reassembly are essential for enhancing the stability, homogeneity, and immunogenicity of VLPs. For example, HBsAg particles contain 30% lipid by mass; therefore, a suitable host that can provide an appropriate lipid composition should be chosen. Similarly, HPV VLPs derived from E. coli are heterogeneous in nature because of the many ill-formed or incomplete particles. This heterogeneity can be reduced by post-purification disassembly followed by reassembly, as reported previously (97).
Demonstration and maintenance of comparability between different lots of preclinical/clinical-grade materials and between small-scale preclinical and large-scale clinical trial materials is a regulatory requirement. Process changes are implemented based on the lot-to-lot comparability exercise, an important component of chemistry, manufacturing, and control (CMC) batch analysis. Lot-to-lot comparability relies on the physical and functional properties of the vaccine, which are responsible for predicting the consistency of batches across different lots. This analysis ensures that vaccine lots are equivalent in potency, purity, and physicochemical integrity. Any process modification and formulation change between these phases must also be verified by CQA-based comparability, which is required for Good Manufacturing Practice (GMP) (103).
5.7 Challenges in the production of Chimeric VLPs
Chimeric VLPs are produced by assembling the structural proteins or epitopes of different viruses. For example, in the M2-HPV model, the M2e influenza epitope was inserted into the HPV 16 L1 sequence in Nicotiana benthamiana plants (104). L1 is the major capsid protein of HPV. The production process for chimeric VLPs depends on various factors, such as glycosylation efficiency, intermolecular chemical bonding, steric hindrance, and cell type; therefore, it is highly unpredictable. This unpredictability may lead to the formation of heterogeneous VLPs. Adding nonpeptide antigens to chimeric VLPs is another challenge because introduced antigens cannot adopt a quaternary structure and thus lead to inappropriate folding of the protein. These epitopes are not optimally exposed; therefore, the immune responses obtained against such epitopes are often limited. Thus, a VLP pseudotyping strategy or modular molecular assembly approach is a viable solution for overcoming this drawback (105, 106). In the first step of the two-step process, native VLPs and target antigens are synthesized separately. In the second step, in vitro assembly of the two components is performed by either covalent or noncovalent binding, which links the target antigen to the surface of the preassembled VLP. This approach significantly enhances the combination of a full-length correctly folded target protein with VLPs. Chemical cross-linking is the most commonly used method to facilitate the binding of antigens and native VLPs. Two examples of chemical crosslinking are the M2-HPV VLP conjugate vaccines of Merck & Co, Inc. and Cytos Biotechnology AG, where the M2 peptide of influenza A is conjugated to VLP (7, 107). Another example is the chemical coupling of the receptor-binding domain (RBD) of SARS-CoV-2 to cucumber mosaic virus derived virus-like particles (CuMVTTVLP) by a Swiss biotech company, Saiba-AG (108). These CuMVTT VLPs incorporate a universal T-cell epitope derived from the tetanus toxin. In addition, these VLPs package bacterial RNA, which is a ligand for Toll-like receptor (TLR) 7/8 and serves as a potent adjuvant by engaging these innate receptors in specific B cells (108).
5.8 Challenges in maintaining stability of VLPs
VLPs can become very unstable and tend to phase separately in solution. The stability of VLPs is essential for producing viable antigens for VLP-based vaccine candidates. This is only possible after obtaining a stable formulation of VLPs. Therefore, preserving critical epitopes on VLPs, along with immunogenicity, is equally important. The introduction of nonionic surfactants into HPV VLP aqueous solutions significantly enhanced their stabilization and reduced aggregation due to heat stress and physical agitation. Generally, eVLPs are more susceptible to external environmental conditions than non-enveloped VLPs because of various factors, such as temperature changes, shear stress, fluid dynamics, agitation rate, chemical treatment, and dissolved oxygen, all of which affect the integrity and stability of VLP particles. Several modifications have been made to improve the thermostability of the eVLPs. A typical procedure involves insertion of stabilizing mutations. A study discovered (109) that inserting a stabilizing mutation in the coat proteins of poliovirus type 3 VLPs increases their stability when compared to wild-type VLPs. Because adjuvants are primarily used to enhance the stability of VLPs, proper dissolution conditions for the removal of adjuvants are also required for morphological and antigenicity analysis of VLPs. In-vitro relative potency (IVRP) or mouse potency under proper dissolution conditions to fully recover the antigen is used to assess the stability of vaccines over many months (110).
Another important parameter is to define the stability of VLPs in three storage conditions: real-time (long-term), accelerated, and stress (high-temperature) conditions (111). Long-term stability studies define the conditions needed for vaccine preservation and expiration date. Accelerated and stress condition tests are used to define the stability of the vaccine in the short-term deviation from preservation and extreme conditions, respectively. However, defining stability under these conditions is challenging because a conceptualized matrix is not available for VLPs owing to their non-linear nature in response to thermal and other external factors and may be very specific to the nature and composites of VLP. The stability tests include identity, appearance, volume of fill, pH, osmolarity, adjuvant content, detergent content, sterility test, endotoxin, impact on potency, and toxicity (111).
Similarly, to avoid the freezing–de-freezing cycle, critical attention should be paid to choosing appropriate and compatible cryoprotectants to preserve the native structure of VLPs.
6 Recent advances to enhance productivity and immunogenicity of VLP expression systems
6.1 Productivity
Several purification steps, such as ion exchange, size-exclusion chromatography, and ultracentrifugation, are used to obtain purified VLPs. Furthermore, HCP are removed by polishing. This step is followed by sterile filtration and formulation to obtain a safe and effective vaccine. At the industrial scale, size-exclusion chromatographic purification or sucrose gradient ultracentrifugation steps are used, which increase production costs. Many scientists are working on replacing these steps with more robust purification steps. For example, a robust purification protocol obtained approximately 90% pure foot and mouth disease virus (FMDV) VLPs. This protocol does not include non-preferred industrial size-exclusion chromatographic purification or sucrose gradient ultracentrifugation steps (32). This approach could be used for large-scale industrial production of E. coli-based VLPs against FMDV infections of different serotypes, making this approach cost-effective. Carvalho et al. showed that the use of sulfated cellulose membrane adsorbers (SCMA) enhances the scalability of vaccines compared to conventional ion exchanger membrane adsorbers by reducing the number of purification steps. This study suggested that SCMA could qualify as a generic platform for purifying VLP-based influenza vaccines (112). However, if the same techniques can be used to produce several different VLPs, it has not been tested and needs further investigation.
Similarly, a chromatographic procedure was used to purify HIV-1 gag VLPs using a mammalian platform, in which the clarified and filtered cell culture supernatant was directly processed on an anion-exchange monolith. Gag, or group-specific antigen, is the major structural protein of HIV-1 and all other retroviruses, and comprises approximately 50% of the mass of a viral particle. This method showed a 220-fold improvement in productivity compared to density gradient centrifugation (35). Plants such as tobacco and related Nicotiana species are commonly used to generate plant-made pharmaceutical proteins (PMPs). However, these plants have high levels of phenolics and toxic alkaloids; therefore, the downstream processing of target PMPs is hindered by technical and regulatory difficulties. Hence, lettuce-based production systems are used instead of tobacco-based platforms because of their low levels of secondary metabolites (113). More complex VLPs have production challenges owing to the incorporation of envelope proteins or their stable nature. Therefore, new technologies must be developed. For example, in the case of enveloped HIV VLP, crossflow filtration across a hollow-fiber cartridge membrane under low-stress conditions concentrates the desired material with 95% recovery.
6.2 Immunogenicity
VLPs have intrinsic immunostimulatory properties because of their ability to activate antigen-presenting cells directly and therefore have great potential for use as antigenic platforms (114). This immunogenicity can be further improved and oriented by incorporating non-coding RNAs (ncRNAs) that act as TLR7/8 ligands. For example, the incorporation of ncRNA into murine leukemia virus (MLV)-derived VLPs provides further TLR-mediated activation and Th1-type CD4+ and CD8+ T-cell response orientation (115). TLRs are expressed by innate immune cells, predominantly antigen-presenting cells (APCs), and recognize various pathogen components. Upon binding to PAMPs, TLR signaling activates APCs, which leads to the production of cytokines, increased co-stimulatory molecule expression, and an enhanced capacity to present and cross-present antigens on MHC class I molecules (116).
The expression of antigens either in their core or on the surface of VLPs significantly improves their immunogenicity, favoring the induction of both B and T-cell-mediated immune responses. Compared to non-enveloped VLPs, eVLPs have the added advantage of presenting the surface proteins of enveloped viruses in their native form. These features can enhance both the humoral and cell-mediated immune responses. For example, the gag protein of Moloney MLV in HEK-293 cells was cleaved to generate a group of proteins that function to create the structured inner core of a VLP. These include matrix, capsid, and nucleocapsid proteins. The capsid protein is the main structural element of a mature virus particle, forming the core-shell around the nucleocapsid-RNA complex, whereas the matrix protein remains linked to the viral lipid bilayer. The commercial name of this vaccine is VBI-1501, which showed high immunogenicity in animals and was used in a phase I clinical trial. Another eVLP vaccine candidate, VBI-1901, is undergoing phase II testing for therapeutic immunization against glioblastoma. Similarly, two other vaccines, VBI-2902a and VBI-2905a, are eVLP-based COVID-19 vaccines for Wuhan and B.1.351 (Beta) variants of SARS-CoV-2, respectively. These vaccines contain a stabilized form of the spike protein of SARS-CoV-2 inserted into the eVLP lipid bilayer (117, 118).
The immunogenicity of VLPs can be increased by engineering VLPs, allowing for a more versatile display of antigens (119). To maximize versatility in displaying foreign antigens of varied compositions, sizes, and structures, the recombinant fusion approach is adopted. In this approach, foreign antigens are inserted into sites inside the viral structural protein to display them on the surface of the resulting VLP’s. However, because peptide insertions into viral capsid proteins frequently result in protein-folding failures, peptide insertions frequently impact the ability of recombinant proteins to assemble into VLPs. To avoid these failures, preformed VLPs might be employed as scaffolding. Foreign antigens are attached to the VLP scaffold using various conjugation techniques (120–123). Similarly, the magnitude and longevity of immune responses of VLPs can also be enhanced by modifying VLPs in such a way that their interactions with specific receptors on or within immune cells (124), interactions with molecules that regulate trafficking (125), and compatibility with adjuvants can be increased. Many VLPs have molecular and structural properties that can spontaneously stimulate the immune system without adjuvants. Nevertheless, the use of adjuvants in VLPs vaccine formulations may enhance immunogenicity and stimulate a specific immune response.
Table 4 summarizes the most recent methods for increasing VLP productivity/immunogenicity.
7 VLP-based vaccines in clinical and preclinical stages
Several VLP-based vaccine candidates tested in clinical and preclinical trials are summarized in Tables 5A, B, respectively. Several VLP-based vaccines have been developed against SARS-CoV-2, five of which are currently undergoing clinical trials. The main antigenic component of these vaccines is the spike protein, specifically the RBD, which is involved in viral entry and antibody neutralization. By displaying multiple full-length spike proteins or only RBDs on the VLPs surfaces, especially on eVLPs, these vaccines have shown high immunogenicity in clinical trials, generating neutralizing antibodies and inducing cell-mediated responses. However, introducing additional structural proteins as antigens in eVLP-based vaccines may lead to more complex and native-like structures and generate broad-spectrum immunity against various SARS-CoV-2 variants (3, 146).
8 Conclusion
Currently, only a few VLP-based vaccines are commercially available against various pathogens such as zika virus, HCV, HBV, and HPV due to various hurdles in their development processes. Therefore, these hurdles must be addressed to enhance commercialization. Researchers are trying to refine VLP-based vaccines at the formulation level by selecting appropriate adjuvants and stabilizers/protectants, administration routes, and delivery vehicles, such as liposomes, poly(lactide–co-glycolide) microparticles, or alginates to reduce the number of vaccine shots and dosage. Concomitantly, methods that can enhance the shelf life of vaccines and avoid cold chains are under investigation.
From an economic point of view, Gardasil is the most successful VLP-based vaccine, yielding over $48.7 billion in 2021. Gardasil has been replaced by Gardasil9 that is an adjuvanted non-infectious recombinant 9-valent vaccine. Gardasil9 has increased concentrations of L1 VLPs for HPV 16 and 18 in order to induce antibody responses in comparison to Gardasil (179). L1, is a ~55 kDa protein with the ability to spontaneously self-assemble into VLPs. In contrast, in other VLP based vaccines generated in the last three years, such as Cervarix for HPV and Engerix-B, Recombivax HB, and Hepavax-Gene for HBV, the revenue has not been reported. Cervarix contains purified proteins from two types of human papillomaviruses (types 16 and 18) (180). It is made using monophosporyl lipid A (MPL), a purified lipid (fat-like substance) extracted from bacteria, which enhances the immune response of the vaccine. VLPs and MPL are then fixed onto an aluminum compound to stimulate a better immune response. MPL is a heterogeneous mixture of congeneric lipid A with 4′-phophoryl groups and varying numbers of acyl chains (181). MPL adjuvant in the form of AS04 mimics a Toll-like receptor 4 agonist providing direct stimulation of antigen presenting cells, pronounced cellular and humoral immune responses, and long lasting antibody responses (179).The Hepavax-Gene (thiomersal free) and Engerix B are non-infectious recombinant hepatitis B vaccines that contain highly purified HBsAg. Engerix-B and Recombivax vaccines differ only in the concentration of HBsAg and the nature of the aluminum adjuvant. Engerix-B uses aluminum hydroxide as an adjuvant and trace amounts of thimerosal from the manufacturing process (182). On the other hand, Recombivax contains aluminum hydrophosphate sulfate. Currently, both academic and industry partners collaborate to produce cheaper vaccines that are affordable to low- and middle-income countries (LMIC). However, based on past events (e.g., HBV vaccination), this appears to not have occurred before. For example, life-saving vaccines such as the HPV VLPs Gardasil and Cervarix were first introduced at relatively high prices in industrialized countries and private sectors in developing countries. Therefore, reaching LMICs with these vaccines took longer than expected. The financial viability of biopharmaceutical companies is the main hurdle in this process and needs to be addressed. Commercial names of various licensed VLP vaccines are given in Table 6.
Author contributions
PK, NA, KA, and RG were responsible for the conceptualization of the manuscript. SR, AJ, RR and RG: Contributed to latest literature review and manuscript drafting. All authors contributed to the article and approved the submitted version.
Conflict of interest
All the authors were employed by Premas Biotech Private Limited.
Publisher’s note
All claims expressed in this article are solely those of the authors and do not necessarily represent those of their affiliated organizations, or those of the publisher, the editors and the reviewers. Any product that may be evaluated in this article, or claim that may be made by its manufacturer, is not guaranteed or endorsed by the publisher.
Glossary
References
1. Loomis RJ, Stewart-Jones GBE, Tsybovsky Y, Caringal RT, Morabito KM, McLellan JS, et al. Structure-based design of nipah virus vaccines: A generalizable approach to paramyxovirus immunogen development. Front Immunol (2020) 11:842. doi: 10.3389/fimmu.2020.00842
2. Tariq H, Batool S, Asif S, Ali M, Abbasi BH. Virus-like particles: Revolutionary platforms for developing vaccines against emerging infectious diseases. Front Microbiol (2021) 12:790121. doi: 10.3389/fmicb.2021.790121
3. Prates-Syed WA, Chaves LCS, Crema KP, Vuitika L, Lira A, Cortes N, et al. VLP-based COVID-19 vaccines: An adaptable technology against the threat of new variants. Vaccines (Basel) (2021) 9(12). doi: 10.3390/vaccines9121409
4. Crawford SE, Labbe M, Cohen J, Burroughs MH, Zhou YJ, Estes MK. Characterization of virus-like particles produced by the expression of rotavirus capsid proteins in insect cells. J Virol (1994) 68(9):5945–52. doi: 10.1128/jvi.68.9.5945-5952.1994
5. Fernandes F, Teixeira AP, Carinhas N, Carrondo MJ, Alves PM. Insect cells as a production platform of complex virus-like particles. Expert Rev Vaccines (2013) 12(2):225–36. doi: 10.1586/erv.12.153
6. Nooraei S, Bahrulolum H, Hoseini ZS, Katalani C, Hajizade A, Easton AJ, et al. Virus-like particles: preparation, immunogenicity and their roles as nanovaccines and drug nanocarriers. J Nanobiotechnol (2021) 19(1):59. doi: 10.1186/s12951-021-00806-7
7. Chackerian B. Virus-like particles: flexible platforms for vaccine development. Expert Rev Vaccines (2007) 6(3):381–90. doi: 10.1586/14760584.6.3.381
8. Mobini S, Chizari M, Mafakher L, Rismani E, Rismani E. Computational design of a novel VLP-based vaccine for hepatitis b virus. Front Immunol (2020) 11:2074. doi: 10.3389/fimmu.2020.02074
9. Roldao A, Mellado MC, Castilho LR, Carrondo MJ, Alves PM. Virus-like particles in vaccine development. Expert Rev Vaccines (2010) 9(10):1149–76. doi: 10.1586/erv.10.115
10. Fernandez-Presas AM, Padilla-Noriega L, Becker I, Robert L, Jimenez JA, Solano S, et al. Enveloped and non-enveloped viral-like particles in trypanosoma cruzi epimastigotes. Rev Inst Med Trop Sao Paulo (2017) 59:e46. doi: 10.1590/s1678-9946201759046
11. Qian C, Liu X, Xu Q, Wang Z, Chen J, Li T, et al. Recent progress on the versatility of virus-like particles. Vaccines (Basel) (2020) 8(1). doi: 10.3390/vaccines8010139
12. Kulagina N, Besseau S, Godon C, Goldman GH, Papon N, Courdavault V. Yeasts as biopharmaceutical production platforms. Front Fungal Biol (2021) 2. doi: 10.3389/ffunb.2021.733492
13. Braeden Donaldson FA-B, Young V, Scullion S, Ward V, Young S. Virus-like particles, a versatile subunit vaccine platform. Subunit Vaccine Delivery (2015), 159–80. doi: 10.1007/978-1-4939-1417-3_9
14. Fuenmayor J, Godia F, Cervera L. Production of virus-like particles for vaccines. N Biotechnol (2017) 39(Pt B):174–80. doi: 10.1016/j.nbt.2017.07.010
15. Baumert TF, Ito S, Wong DT, Liang TJ. Hepatitis c virus structural proteins assemble into viruslike particles in insect cells. J Virol (1998) 72(5):3827–36. doi: 10.1128/JVI.72.5.3827-3836.1998
16. Tacket CO. Plant-based vaccines against diarrheal diseases. Trans Am Clin Climatol Assoc (2007) 118:79–87.
17. Tacket CO, Mason HS, Losonsky G, Estes MK, Levine MM, Arntzen CJ. Human immune responses to a novel norwalk virus vaccine delivered in transgenic potatoes. J Infect Dis (2000) 182(1):302–5. doi: 10.1086/315653
18. Scotti N, Alagna F, Ferraiolo E, Formisano G, Sannino L, Buonaguro L, et al. High-level expression of the HIV-1 Pr55gag polyprotein in transgenic tobacco chloroplasts. Planta (2009) 229(5):1109–22. doi: 10.1007/s00425-009-0898-2
19. Herzog C, Hartmann K, Kunzi V, Kursteiner O, Mischler R, Lazar H, et al. Eleven years of inflexal V-a virosomal adjuvanted influenza vaccine. Vaccine (2009) 27(33):4381–7. doi: 10.1016/j.vaccine.2009.05.029
20. Bovier PA. Recent advances with a virosomal hepatitis a vaccine. Expert Opin Biol Ther (2008) 8(8):1177–85. doi: 10.1517/14712598.8.8.1177
21. Grein TA, Weidner T, Czermak P. Concepts for the production of viruses and viral vectors in cell cultures. In: Gowder SJT editor. (London, UK: IntechOpen) New insights into cell culture technology (2017).
22. Paulová L, Patáková P, Brányik T. Chapter 4: Advanced fermentation processes. In: Teixeira J, Vicente A, editors, Engineering Aspects of Food Biotechnology. Boca Raton: CRC Press (2013). p. 89–105. doi: 10.1201/b15426-6
23. Gutierrez-Granados S, Cervera L, Segura Mde L, Wolfel J, Godia F. Optimized production of HIV-1 virus-like particles by transient transfection in CAP-T cells. Appl Microbiol Biotechnol (2016) 100(9):3935–47. doi: 10.1007/s00253-015-7213-x
24. Metz SW, Gardner J, Geertsema C, Le TT, Goh L, Vlak JM, et al. Effective chikungunya virus-like particle vaccine produced in insect cells. PloS Negl Trop Dis (2013) 7(3):e2124. doi: 10.1371/journal.pntd.0002124
25. Ye L, Lin J, Sun Y, Bennouna S, Lo M, Wu Q, et al. Ebola Virus-like particles produced in insect cells exhibit dendritic cell stimulating activity and induce neutralizing antibodies. Virology (2006) 351(2):260–70. doi: 10.1016/j.virol.2006.03.021
26. Maranga L, Brazao TF, Carrondo MJ. Virus-like particle production at low multiplicities of infection with the baculovirus insect cell system. Biotechnol Bioeng (2003) 84(2):245–53. doi: 10.1002/bit.10773
27. Gurramkonda C, Adnan A, Gabel T, Lunsdorf H, Ross A, Nemani SK, et al. Simple high-cell density fed-batch technique for high-level recombinant protein production with pichia pastoris: Application to intracellular production of hepatitis b surface antigen. Microb Cell Fact (2009) 8:13. doi: 10.1186/1475-2859-8-13
28. Fontana D, Kratje R, Etcheverrigaray M, Prieto C. Immunogenic virus-like particles continuously expressed in mammalian cells as a veterinary rabies vaccine candidate. Vaccine (2015) 33(35):4238–46. doi: 10.1016/j.vaccine.2015.03.088
30. Paulova L, Chmelik J, Branska B, Patakova P, Drahokoupil M, Melzoch K. Comparison of lactic acid production by l. casei in batch, fed-batch and continuous cultivation, testing the use of feather hydrolysate as a complex nitrogen source. Braz Arch Biol Technol (2020) 63:1–12. doi: 10.1590/1678-4324-2020190151
31. Weiss K, Gerstenberger J, Salzig D, Mühlebach MD, Cichutek K, Pörtner R, et al. Oncolytic measles viruses produced at different scales under serum-free conditions. Engineering in Life Sci (2015) 15(4):425–36. doi: 10.1002/elsc.201400165
32. Xiao Y, Chen HY, Wang Y, Yin B, Lv C, Mo X, et al. Large-Scale production of foot-and-mouth disease virus (serotype Asia1) VLP vaccine in escherichia coli and protection potency evaluation in cattle. BMC Biotechnol (2016) 16(1):56. doi: 10.1186/s12896-016-0285-6
33. Fernandez E, Toledo JR, Mendez L, Gonzalez N, Parra F, Martin-Alonso JM, et al. Conformational and thermal stability improvements for the large-scale production of yeast-derived rabbit hemorrhagic disease virus-like particles as multipurpose vaccine. PloS One (2013) 8(2):e56417. doi: 10.1371/journal.pone.0056417
34. Yang Z, Gao F, Wang X, Shi L, Zhou Z, Jiang Y, et al. Development and characterization of an enterovirus 71 (EV71) virus-like particles (VLPs) vaccine produced in pichia pastoris. Hum Vaccin Immunother (2020) 16(7):1602–10. doi: 10.1080/21645515.2019.1649554
35. Steppert P, Burgstaller D, Klausberger M, Berger E, Aguilar PP, Schneider TA, et al. Purification of HIV-1 gag virus-like particles and separation of other extracellular particles. J Chromatogr A (2016) 1455:93–101. doi: 10.1016/j.chroma.2016.05.053
36. Wu PC, Chen TY, Chi JN, Chien MS, Huang C. Efficient expression and purification of porcine circovirus type 2 virus-like particles in escherichia coli. J Biotechnol (2016) 220:78–85. doi: 10.1016/j.jbiotec.2016.01.017
37. Aucoin MG, Jacob D, Chahal PS, Meghrous J, Bernier A, Kamen AA. Virus-like particle and viral vector production using the baculovirus expression vector system/insect cell system: adeno-associated virus-based products. Methods Mol Biol (2007) 388:281–96. doi: 10.1007/978-1-59745-457-5_14
38. Wagner JM, Pajerowski JD, Daniels CL, McHugh PM, Flynn JA, Balliet JW, et al. Enhanced production of chikungunya virus-like particles using a high-pH adapted spodoptera frugiperda insect cell line. PloS One (2014) 9(4):e94401. doi: 10.1371/journal.pone.0094401
39. Lai CC, Cheng YC, Chen PW, Lin TH, Tzeng TT, Lu CC, et al. Process development for pandemic influenza VLP vaccine production using a baculovirus expression system. J Biol Eng (2019) 13:78. doi: 10.1186/s13036-019-0206-z
40. Rodriguez-Limas WA, Tyo KE, Nielsen J, Ramirez OT, Palomares LA. Molecular and process design for rotavirus-like particle production in saccharomyces cerevisiae. Microb Cell Fact (2011) 10:33. doi: 10.1186/1475-2859-10-33
41. Mohsen MO, Balke I, Zinkhan S, Zeltina V, Liu X, Chang X, et al. A scalable and highly immunogenic virus-like particle-based vaccine against SARS-CoV-2. Allergy (2022) 77(1):243–57. doi: 10.1111/all.15080
42. Krause M, Ukkonen K, Haataja T, Ruottinen M, Glumoff T, Neubauer A, et al. A novel fed-batch based cultivation method provides high cell-density and improves yield of soluble recombinant proteins in shaken cultures. Microb Cell Fact (2010) 9:11. doi: 10.1186/1475-2859-9-11
43. Huang X, Wang X, Zhang J, Xia N, Zhao Q. Escherichia coli-derived virus-like particles in vaccine development. NPJ Vaccines (2017) 2:3. doi: 10.1038/s41541-017-0006-8
44. Le DT, Muller KM. In vitro assembly of virus-like particles and their applications. Life (Basel) (2021) 11(4). doi: 10.3390/life11040334
45. Schwarz F, Aebi M. Mechanisms and principles of n-linked protein glycosylation. Curr Opin Struct Biol (2011) 21(5):576–82. doi: 10.1016/j.sbi.2011.08.005
46. Wacker M, Linton D, Hitchen PG, Nita-Lazar M, Haslam SM, North SJ, et al. N-linked glycosylation in campylobacter jejuni and its functional transfer into e. coli. Science (2002) 298(5599):1790–3. doi: 10.1126/science.298.5599.1790
47. Nothaft H, Szymanski CM. Bacterial protein n-glycosylation: new perspectives and applications. J Biol Chem (2013) 288(10):6912–20. doi: 10.1074/jbc.R112.417857
48. Jaroentomeechai T, Stark JC, Natarajan A, Glasscock CJ, Yates LE, Hsu KJ, et al. Single-pot glycoprotein biosynthesis using a cell-free transcription-translation system enriched with glycosylation machinery. Nat Commun (2018) 9(1):2686. doi: 10.1038/s41467-018-05110-x
49. Smolskaya S, Logashina YA, Andreev YA. Escherichia coli extract-based cell-free expression system as an alternative for difficult-to-Obtain protein biosynthesis. Int J Mol Sci (2020) 21(3). doi: 10.3390/ijms21030928
50. Tytgat HLP, Lin CW, Levasseur MD, Tomek MB, Rutschmann C, Mock J, et al. Cytoplasmic glycoengineering enables biosynthesis of nanoscale glycoprotein assemblies. Nat Commun (2019) 10(1):5403. doi: 10.1038/s41467-019-13283-2
51. Wang K, Zhou L, Chen T, Li Q, Li J, Liu L, et al. Engineering for an HPV 9-valent vaccine candidate using genomic constitutive over-expression and low lipopolysaccharide levels in escherichia coli cells. Microb Cell Fact (2021) 20(1):227. doi: 10.1186/s12934-021-01719-8
52. Yamaji H. Suitability and perspectives on using recombinant insect cells for the production of virus-like particles. Appl Microbiol Biotechnol (2014) 98(5):1963–70. doi: 10.1007/s00253-013-5474-9
53. Matsuda T, Tanijima T, Hirose A, Masumi-Koizumi K, Katsuda T, Yamaji H. Production of influenza virus-like particles using recombinant insect cells. Biochem Eng J (2020) 163:107757. doi: 10.1016/j.bej.2020.107757
54. Wang X, Xiao X, Zhao M, Liu W, Pang L, Sun X, et al. EV71 virus-like particles produced by co-expression of capsid proteins in yeast cells elicit humoral protective response against EV71 lethal challenge. BMC Res Notes (2016) 9:42. doi: 10.1186/s13104-015-1780-x
55. Kim HJ, Kim HJ. Yeast as an expression system for producing virus-like particles: what factors do we need to consider? Lett Appl Microbiol (2017) 64(2):111–23. doi: 10.1111/lam.12695
56. Manfrao-Netto JHC, Gomes AMV, Parachin NS. Advances in using hansenula polymorpha as chassis for recombinant protein production. Front Bioeng Biotechnol (2019) 7:94. doi: 10.3389/fbioe.2019.00094
57. Wetzel D, Rolf T, Suckow M, Kranz A, Barbian A, Chan JA, et al. Establishment of a yeast-based VLP platform for antigen presentation. Microb Cell Fact (2018) 17(1):17. doi: 10.1186/s12934-018-0868-0
58. Shirbaghaee Z, Bolhassani A. Different applications of virus-like particles in biology and medicine: Vaccination and delivery systems. Biopolymers (2016) 105(3):113–32. doi: 10.1002/bip.22759
59. Shouval D, Roggendorf H, Roggendorf M. Enhanced immune response to hepatitis b vaccination through immunization with a pre-S1/Pre-S2/S vaccine. Med Microbiol Immunol (2015) 204(1):57–68. doi: 10.1007/s00430-014-0374-x
60. Li C, Liu F, Liang M, Zhang Q, Wang X, Wang T, et al. Hantavirus-like particles generated in CHO cells induce specific immune responses in C57BL/6 mice. Vaccine (2010) 28(26):4294–300. doi: 10.1016/j.vaccine.2010.04.025
61. Ingerslev J, Christiansen K, Ravn HB, Bray GL, Gomperts ED, Recombinate Study G. Antibodies to heterologous proteins in hemophilia a patients receiving recombinant factor VIII (Recombinate). Thromb Haemost (2002) 87(4):626–34. doi: 10.1055/s-0037-1613059
62. MacDonald ML, Hamaker N, Lee KH. Bioinformatic analysis of Chinese hamster ovary host cell protein lipases. AIChE J (2018) 64(12):4247–54. doi: 10.1002/aic.16378
63. Wu CY, Yeh YC, Yang YC, Chou C, Liu MT, Wu HS, et al. Mammalian expression of virus-like particles for advanced mimicry of authentic influenza virus. PloS One (2010) 5(3):e9784. doi: 10.1371/journal.pone.0009784
64. Bernal V, Carinhas N, Yokomizo AY, Carrondo MJ, Alves PM. Cell density effect in the baculovirus-insect cells system: a quantitative analysis of energetic metabolism. Biotechnol Bioeng (2009) 104(1):162–80. doi: 10.1002/bit.22364
65. Cruz PE, Cunha A, Peixoto CC, Clemente J, Moreira JL, Carrondo MJ. Optimization of the production of virus-like particles in insect cells. Biotechnol Bioeng (1998) 60(4):408–18. doi: 10.1002/(SICI)1097-0290(19981120)60:4<408::AID-BIT2>3.0.CO;2-Q
66. Power JF, Reid S, Radford KM, Greenfield PF, Nielsen LK. Modeling and optimization of the baculovirus expression vector system in batch suspension culture. Biotechnol Bioeng (1994) 44(6):710–9. doi: 10.1002/bit.260440607
67. Palucha A, Loniewska A, Satheshkumar S, Boguszewska-Chachulska AM, Umashankar M, Milner M, et al. Virus-like particles: models for assembly studies and foreign epitope carriers. Prog Nucleic Acid Res Mol Biol (2005) 80:135–68. doi: 10.1016/S0079-6603(05)80004-2
68. Lorenzo LJ, Duenas-Carrera S, Falcon V, Acosta-Rivero N, Gonzalez E, de la Rosa MC, et al. Assembly of truncated HCV core antigen into virus-like particles in escherichia coli. Biochem Biophys Res Commun (2001) 281(4):962–5. doi: 10.1006/bbrc.2001.4449
69. Kunkel M, Lorinczi M, Rijnbrand R, Lemon SM, Watowich SJ. Self-assembly of nucleocapsid-like particles from recombinant hepatitis c virus core protein. J Virol (2001) 75(5):2119–29. doi: 10.1128/JVI.75.5.2119-2129.2001
70. Thomsen DR, Newcomb WW, Brown JC, Homa FL. Assembly of the herpes simplex virus capsid: requirement for the carboxyl-terminal twenty-five amino acids of the proteins encoded by the UL26 and UL26.5 genes. J Virol (1995) 69(6):3690–703. doi: 10.1128/jvi.69.6.3690-3703.1995
71. Almeida MEM, Vasconcelos MGS, Tarrago AM, Mariuba LAM. Circumsporozoite surface protein-based malaria vaccines: a review. Rev Inst Med Trop Sao Paulo (2021) 63:e11. doi: 10.1590/s1678-9946202163011
72. Laurens MB. RTS,S/AS01 vaccine (Mosquirix): an overview. Hum Vaccin Immunother (2020) 16(3):480–9. doi: 10.1080/21645515.2019.1669415
73. van Rosmalen MGM, Li C, Zlotnick A, Wuite GJL, Roos WH. Effect of dsDNA on the assembly pathway and mechanical strength of SV40 VP1 virus-like particles. Biophys J (2018) 115(9):1656–65. doi: 10.1016/j.bpj.2018.07.044
74. Roldao A, Mellado MC, Lima JC, Carrondo MJ, Alves PM, Oliveira R. On the effect of thermodynamic equilibrium on the assembly efficiency of complex multi-layered virus-like particles (VLP): the case of rotavirus VLP. PloS Comput Biol (2012) 8(2):e1002367. doi: 10.1371/journal.pcbi.1002367
75. Samandoulgou I, Fliss I, Jean J. Zeta potential and aggregation of virus-like particle of human norovirus and feline calicivirus under different physicochemical conditions. Food Environ Virol (2015) 7(3):249–60. doi: 10.1007/s12560-015-9198-0
76. Wang Y, Wang G, Duan WT, Sun MX, Wang MH, Wang SH, et al. Self-assembly into virus-like particles of the recombinant capsid protein of porcine circovirus type 3 and its application on antibodies detection. AMB Express (2020) 10(1):3. doi: 10.1186/s13568-019-0940-0
77. Roldão ACS A, Mellado MCM. Viruses and virus-like particles in biotechnology: Fundamentals and applications. Compr Biotechnol (2017) 1:633–56. doi: 10.1016/B978-0-12-809633-8.09046-4
78. Konz JO, King J, Cooney CL. Effects of oxygen on recombinant protein expression. Biotechnol Prog (1998) 14(3):393–409. doi: 10.1021/bp980021l
79. Xie L, Metallo C, Warren J, Pilbrough W, Peltier J, Zhong T, et al. Large-Scale propagation of a replication-defective adenovirus vector in stirred-tank bioreactor PER.C6 cell culture under sparging conditions. Biotechnol Bioeng (2003) 83(1):45–52. doi: 10.1002/bit.10644
80. Murhammer D, Goochee C. Scale up of insect cell cultures: Protective effects of pluronic f-68. Biotechnology (1988) 6:1411–8.
81. Hu W, Berdugo C, Chalmers JJ. The potential of hydrodynamic damage to animal cells of industrial relevance: current understanding. Cytotechnology (2011) 63(5):445–60. doi: 10.1007/s10616-011-9368-3
82. Hosseini SN, Sarvari T, Bashiri G, Khatami M, Shojaosadati SA. Assessing virus like particles formation and r-HBsAg aggregation during large scale production of recombinant hepatitis b surface antigen from pichia pastoris. Int J Biol Macromol (2019) 139:697–711. doi: 10.1016/j.ijbiomac.2019.08.019
83. Carra JH, Martins KA, Schokman RD, Robinson CG, Steffens JT, Bavari S. A thermostable, chromatographically purified Ebola nano-VLP vaccine. J Transl Med (2015) 13:228. doi: 10.1186/s12967-015-0593-y
84. Peixoto C, Ferreira TB, Sousa MF, Carrondo MJ, Alves PM. Towards purification of adenoviral vectors based on membrane technology. Biotechnol Prog (2008) 24(6):1290–6. doi: 10.1002/btpr.25
85. Morenweiser R. Downstream processing of viral vectors and vaccines. Gene Ther (2005) 12 Suppl 1:S103–10. doi: 10.1038/sj.gt.3302624
86. Hammonds J, Chen X, Zhang X, Lee F, Spearman P. Advances in methods for the production, purification, and characterization of HIV-1 gag-env pseudovirion vaccines. Vaccine (2007) 25(47):8036–48. doi: 10.1016/j.vaccine.2007.09.016
87. Gonzalez-Dominguez I, Puente-Massaguer E, Cervera L, Godia F. Quality assessment of virus-like particles at single particle level: A comparative study. Viruses (2020) 12(2). doi: 10.3390/v12020223
88. Vieira HL, Estevao C, Roldao A, Peixoto CC, Sousa MF, Cruz PE, et al. Triple layered rotavirus VLP production: kinetics of vector replication, mRNA stability and recombinant protein production. J Biotechnol (2005) 120(1):72–82. doi: 10.1016/j.jbiotec.2005.03.026
89. Mena JA, Ramirez OT, Palomares LA. Quantification of rotavirus-like particles by gel permeation chromatography. J Chromatogr B Analyt Technol BioMed Life Sci (2005) 824(1-2):267–76. doi: 10.1016/j.jchromb.2005.07.034
90. Mellado MC, Franco C, Coelho A, Alves PM, Simplicio AL. Sodium dodecyl sulfate-capillary gel electrophoresis analysis of rotavirus-like particles. J Chromatogr A (2008) 1192(1):166–72. doi: 10.1016/j.chroma.2008.03.035
91. Franco CF, Mellado MC, Alves PM, Coelho AV. Monitoring virus-like particle and viral protein production by intact cell MALDI-TOF mass spectrometry. Talanta (2010) 80(4):1561–8. doi: 10.1016/j.talanta.2009.06.081
92. Teixeira AP, Portugal CA, Carinhas N, Dias JM, Crespo JP, Alves PM, et al. In situ 2D fluorometry and chemometric monitoring of mammalian cell cultures. Biotechnol Bioeng (2009) 102(4):1098–106. doi: 10.1002/bit.22125
93. Teixeira AP, Oliveira R, Alves PM, Carrondo MJT. Advances in on-line monitoring and control of mammalian cell cultures: Supporting the PAT initiative. Biotechnol Adv (2009) 27(6):726–32. doi: 10.1016/j.biotechadv.2009.05.003
94. Ladd Effio C, Oelmeier SA, Hubbuch J. High-throughput characterization of virus-like particles by interlaced size-exclusion chromatography. Vaccine (2016) 34(10):1259–67. doi: 10.1016/j.vaccine.2016.01.035
95. Samandoulgou I, Hammami R, Morales Rayas R, Fliss I, Jean J. Stability of secondary and tertiary structures of virus-like particles representing noroviruses: Effects of pH, ionic strength, and temperature and implications for adhesion to surfaces. Appl Environ Microbiol (2015) 81(22):7680–6. doi: 10.1128/AEM.01278-15
96. Zhao Q, Li S, Yu H, Xia N, Modis Y. Virus-like particle-based human vaccines: quality assessment based on structural and functional properties. Trends Biotechnol (2013) 31(11):654–63. doi: 10.1016/j.tibtech.2013.09.002
97. Zhao Q, Allen MJ, Wang Y, Wang B, Wang N, Shi L, et al. Disassembly and reassembly improves morphology and thermal stability of human papillomavirus type 16 virus-like particles. Nanomedicine (2012) 8(7):1182–9. doi: 10.1016/j.nano.2012.01.007
98. Steppert P, Burgstaller D, Klausberger M, Tover A, Berger E, Jungbauer A. Quantification and characterization of virus-like particles by size-exclusion chromatography and nanoparticle tracking analysis. J Chromatogr A (2017) 1487:89–99. doi: 10.1016/j.chroma.2016.12.085
99. Guha S, Li M, Tarlov MJ, Zachariah MR. Electrospray-differential mobility analysis of bionanoparticles. Trends Biotechnol (2012) 30(5):291–300. doi: 10.1016/j.tibtech.2012.02.003
100. Pease LF 3rd, Lipin DI, Tsai DH, Zachariah MR, Lua LH, Tarlov MJ, et al. Quantitative characterization of virus-like particles by asymmetrical flow field flow fractionation, electrospray differential mobility analysis, and transmission electron microscopy. Biotechnol Bioeng (2009) 102(3):845–55. doi: 10.1002/bit.22085
101. Chuan YP, Fan YY, Lua L, Middelberg AP. Quantitative analysis of virus-like particle size and distribution by field-flow fractionation. Biotechnol Bioeng (2008) 99(6):1425–33. doi: 10.1002/bit.21710
102. Towne V, Zhao Q, Brown M, Finnefrock AC. Pairwise antibody footprinting using surface plasmon resonance technology to characterize human papillomavirus type 16 virus-like particles with direct anti-HPV antibody immobilization. J Immunol Methods (2013) 388(1-2):1–7. doi: 10.1016/j.jim.2012.11.005
103. Sanyal G, Sarnefalt A, Kumar A. Considerations for bioanalytical characterization and batch release of COVID-19 vaccines. NPJ Vaccines (2021) 6(1):53. doi: 10.1038/s41541-021-00317-4
104. Matic S, Rinaldi R, Masenga V, Noris E. Efficient production of chimeric human papillomavirus 16 L1 protein bearing the M2e influenza epitope in nicotiana benthamiana plants. BMC Biotechnol (2011) 11:106. doi: 10.1186/1472-6750-11-106
105. Bellier B, Klatzmann D. Virus-like particle-based vaccines against hepatitis c virus infection. Expert Rev Vaccines (2013) 12(2):143–54. doi: 10.1586/erv.13.10
106. Garrone P, Fluckiger AC, Mangeot PE, Gauthier E, Dupeyrot-Lacas P, Mancip J, et al. A prime-boost strategy using virus-like particles pseudotyped for HCV proteins triggers broadly neutralizing antibodies in macaques. Sci Transl Med (2011) 3(94):94ra71. doi: 10.1126/scitranslmed.3002330
107. Schotsaert M, De Filette M, Fiers W, Saelens X. Universal M2 ectodomain-based influenza a vaccines: preclinical and clinical developments. Expert Rev Vaccines (2009) 8(4):499–508. doi: 10.1586/erv.09.6
108. Zha L, Chang X, Zhao H, Mohsen MO, Hong L, Zhou Y, et al. Development of a vaccine against SARS-CoV-2 based on the receptor-binding domain displayed on virus-like particles. Vaccines (Basel) (2021) 9(4). doi: 10.3390/vaccines9040395
109. Marsian J, Fox H, Bahar MW, Kotecha A, Fry EE, Stuart DI, et al. Plant-made polio type 3 stabilized VLPs-a candidate synthetic polio vaccine. Nat Commun (2017) 8(1):245. doi: 10.1038/s41467-017-00090-w
110. Shank-Retzlaff M, Wang F, Morley T, Anderson C, Hamm M, Brown M, et al. Correlation between mouse potency and in vitro relative potency for human papillomavirus type 16 virus-like particles and gardasil vaccine samples. Hum Vaccin (2005) 1(5):191–7. doi: 10.4161/hv.1.5.2126
111. Liu YY, Zhang HJ, Shen EC, Chen D, Wang Y, Fu SJ, et al. Stability of trivalent human papillomavirus (types 16, 18, 58) recombinant vaccine (Escherichia coli). Chin Med J (Engl) (2021) 134(24):3020–2. doi: 10.1097/CM9.0000000000001659
112. BC S, Fortuna AR, Wolff MW, Peixoto C, MA P, Reichl U, et al. Purification of influenza virus-like particles using sulfated cellulose membrane adsorbers. J Chem Technol Biotechnol (2018) 93(7):1988–96. doi: 10.1002/jctb.5474
113. Lai H, He J, Engle M, Diamond MS, Chen Q. Robust production of virus-like particles and monoclonal antibodies with geminiviral replicon vectors in lettuce. Plant Biotechnol J (2012) 10(1):95–104. doi: 10.1111/j.1467-7652.2011.00649.x
114. Bellier B, Dalba C, Clerc B, Desjardins D, Drury R, Cosset FL, et al. DNA Vaccines encoding retrovirus-based virus-like particles induce efficient immune responses without adjuvant. Vaccine (2006) 24(14):2643–55. doi: 10.1016/j.vaccine.2005.11.034
115. Pitoiset F, Vazquez T, Levacher B, Nehar-Belaid D, Derian N, Vigneron J, et al. Retrovirus-based virus-like particle immunogenicity and its modulation by toll-like receptor activation. J Virol (2017) 91(21). doi: 10.1128/JVI.01230-17
116. Kawasaki T, Kawai T. Toll-like receptor signaling pathways. Front Immunol (2014) 5:461. doi: 10.3389/fimmu.2014.00461
117. Diaz-Mitoma F. Enveloped virus-like particles as a platform for vaccine development. Int J Noncommunicable Dis (2021) 6:89–94. doi: 10.4103/2468-8827.330656
118. Pitoiset F, Vazquez T, Bellier B. Enveloped virus-like particle platforms: vaccines of the future? Expert Rev Vaccines (2015) 14(7):913–5. doi: 10.1586/14760584.2015.1046440
119. Frietze KM, Peabody DS, Chackerian B. Engineering virus-like particles as vaccine platforms. Curr Opin Virol (2016) 18:44–9. doi: 10.1016/j.coviro.2016.03.001
120. Zakeri B, Fierer JO, Celik E, Chittock EC, Schwarz-Linek U, Moy VT, et al. Peptide tag forming a rapid covalent bond to a protein, through engineering a bacterial adhesin. Proc Natl Acad Sci U S A (2012) 109(12):E690–7. doi: 10.1073/pnas.1115485109
121. Hatlem D, Trunk T, Linke D, Leo JC. Catching a SPY: Using the SpyCatcher-SpyTag and related systems for labeling and localizing bacterial proteins. Int J Mol Sci (2019) 20(9). doi: 10.3390/ijms20092129
122. Brune KD, Leneghan DB, Brian IJ, Ishizuka AS, Bachmann MF, Draper SJ, et al. Plug-and-Display: decoration of virus-like particles via isopeptide bonds for modular immunization. Sci Rep (2016) 6:19234. doi: 10.1038/srep19234
123. Chevillard C, Amen A, Besson S, Hannani D, Bally I, Dettling V, et al. Elicitation of potent SARS-CoV-2 neutralizing antibody responses through immunization with a versatile adenovirus-inspired multimerization platform. Mol Ther (2022) 30(5):1913–25. doi: 10.1016/j.ymthe.2022.02.011
124. Manolova V, Flace A, Bauer M, Schwarz K, Saudan P, Bachmann MF. Nanoparticles target distinct dendritic cell populations according to their size. Eur J Immunol (2008) 38(5):1404–13. doi: 10.1002/eji.200737984
125. Link A, Zabel F, Schnetzler Y, Titz A, Brombacher F, Bachmann MF. Innate immunity mediates follicular transport of particulate but not soluble protein antigen. J Immunol (2012) 188(8):3724–33. doi: 10.4049/jimmunol.1103312
126. Imagawa T, Ito M, Matsuda M, Nakashima K, Tokunaga Y, Ohta I, et al. Virus-like particles with FLAG-tagged envelope protein as a tetravalent dengue vaccine candidate. Sci Rep (2021) 11(1):17542. doi: 10.1038/s41598-021-97038-4
127. Yang M, Lai H, Sun H, Chen Q. Virus-like particles that display zika virus envelope protein domain III induce potent neutralizing immune responses in mice. Sci Rep (2017) 7(1):7679. doi: 10.1038/s41598-017-08247-9
128. Liu D, Zhang S, Poteet E, Marin-Muller C, Chen C, Yao Q. Sublingual immunization with chimeric C1q/CD40 Ligand/HIV virus-like particles induces strong mucosal immune responses against HIV. Vaccines (Basel) (2021) 9(11). doi: 10.3390/vaccines9111236
129. Zamora-Ceballos M, Moreno N, Gil-Cantero D, Caston JR, Blanco E, Barcena J. Immunogenicity of multi-target chimeric RHDV virus-like particles delivering foreign b-cell epitopes. Vaccines (Basel) (2022) 10(2). doi: 10.3390/vaccines10020229
130. Zhang B, Yin S, Wang Y, Su Z, Bi J. Cost-effective purification process development for chimeric hepatitis b core (HBc) virus-like particles assisted by molecular dynamic simulation. Eng Life Sci (2021) 21(6):438–52. doi: 10.1002/elsc.202000104
131. Chauveau L, Bridgeman A, Tan TK, Beveridge R, Frost JN, Rijal P, et al. Inclusion of cGAMP within virus-like particle vaccines enhances their immunogenicity. EMBO Rep (2021) 22(8):e52447. doi: 10.15252/embr.202152447
132. Guo M, Li J, Teng Z, Ren M, Dong H, Zhang Y, et al. Four simple biomimetic mineralization methods to improve the thermostability and immunogenicity of virus-like particles as a vaccine against foot-and-Mouth disease. Vaccines (Basel) (2021) 9(8). doi: 10.3390/vaccines9080891
133. Anwar MN, Jiang C, Di D, Zhang J, Guo S, Wang X, et al. A novel recombinant virus-like particles displaying b and T cell epitopes of Japanese encephalitis virus offers protective immunity in mice and Guinea pigs. Vaccines (Basel) (2021) 9(9). doi: 10.3390/vaccines9090980
134. Cregg JFT JM, Stillman C, Siegel R, Akong M, Craig WS, Buckholz RG, et al. High–level expression and efficient assembly of hepatitis b surface antigen in the methylotrophic yeast, pichia pastoris. Bio/Technology (1987) 5:479–85. doi: 10.1038/nbt0587-479
135. Zhou Y, Shen C, Zhang C, Zhang W, Wang L, Lan K, et al. Yeast-produced recombinant virus-like particles of coxsackievirus A6 elicited protective antibodies in mice. Antiviral Res (2016) 132:165–9. doi: 10.1016/j.antiviral.2016.06.004
136. Mazumder S, Rastogi R, Undale A, Arora K, Arora NM, Pratim B, et al. PRAK-03202: A triple antigen virus-like particle vaccine candidate against SARS CoV-2. Heliyon (2021) 7(10):e08124. doi: 10.1016/j.heliyon.2021.e08124
137. CG A, Roesti ES, El-Turabi A, Bachmann MF. Type of RNA packed in VLPs impacts IgG class switching-implications for an influenza vaccine design. Vaccines (Basel) (2019) 7(2). doi: 10.3390/vaccines7020047
138. Nardin EH, Oliveira GA, Calvo-Calle JM, Wetzel K, Maier C, Birkett AJ, et al. Phase I testing of a malaria vaccine composed of hepatitis b virus core particles expressing plasmodium falciparum circumsporozoite epitopes. Infect Immun (2004) 72(11):6519–27. doi: 10.1128/IAI.72.11.6519-6527.2004
139. Klimek L, Willers J, Hammann-Haenni A, Pfaar O, Stocker H, Mueller P, et al. Assessment of clinical efficacy of CYT003-QbG10 in patients with allergic rhinoconjunctivitis: a phase IIb study. Clin Exp Allergy (2011) 41(9):1305–12. doi: 10.1111/j.1365-2222.2011.03783.x
140. Lee YT, Kim KH, Ko EJ, Lee YN, Kim MC, Kwon YM, et al. New vaccines against influenza virus. Clin Exp Vaccine Res (2014) 3(1):12–28. doi: 10.7774/cevr.2014.3.1.12
141. Qiao YL, Wu T, Li RC, Hu YM, Wei LH, Li CG, et al. Efficacy, safety, and immunogenicity of an escherichia coli-produced bivalent human papillomavirus vaccine: An interim analysis of a randomized clinical trial. J Natl Cancer Inst (2020) 112(2):145–53. doi: 10.1093/jnci/djz074
142. Hildesheim A, Gonzalez P, Kreimer AR, Wacholder S, Schussler J, Rodriguez AC, et al. Impact of human papillomavirus (HPV) 16 and 18 vaccination on prevalent infections and rates of cervical lesions after excisional treatment. Am J Obstet Gynecol (2016) 215(2):212 e1– e15. doi: 10.1016/j.ajog.2016.02.021
143. Rusmil K, Gunardi H, Fadlyana E, Soedjatmiko, Dhamayanti M, Sekartini R, et al. The immunogenicity, safety, and consistency of an Indonesia combined DTP-HB-Hib vaccine in expanded program on immunization schedule. BMC Pediatr (2015) 15:219. doi: 10.1186/s12887-015-0525-2
144. Valero-Pacheco N, Perez-Toledo M, Villasis-Keever MA, Nunez-Valencia A, Bosco-Garate I, Lozano-Dubernard B, et al. Antibody persistence in adults two years after vaccination with an H1N1 2009 pandemic influenza virus-like particle vaccine. PloS One (2016) 11(2):e0150146. doi: 10.1371/journal.pone.0150146
145. Bernstein DI, El Sahly HM, Keitel WA, Wolff M, Simone G, Segawa C, et al. Safety and immunogenicity of a candidate parvovirus B19 vaccine. Vaccine (2011) 29(43):7357–63. doi: 10.1016/j.vaccine.2011.07.080
146. Sharifzadeh M, Mottaghi-Dastjerdi N, Soltany Rezae Raad M. A review of virus-like particle-based SARS-CoV-2 vaccines in clinical trial phases. Iran J Pharm Res (2022) 21(1):e127042. doi: 10.5812/ijpr-127042
147. Piroth L, Launay O, Michel ML, Bourredjem A, Miailhes P, Ajana F, et al. Vaccination against hepatitis b virus (HBV) in HIV-1-Infected patients with isolated anti-HBV core antibody: The ANRS HB EP03 CISOVAC prospective study. J Infect Dis (2016) 213(11):1735–42. doi: 10.1093/infdis/jiw011
148. Bennett SR, McCarty JM, Ramanathan R, Mendy J, Richardson JS, Smith J, et al. Safety and immunogenicity of PXVX0317, an aluminium hydroxide-adjuvanted chikungunya virus-like particle vaccine: a randomised, double-blind, parallel-group, phase 2 trial. Lancet Infect Dis (2022) 22(9):1343–55. doi: 10.1016/S1473-3099(22)00226-2
149. Fluckiger AC, Ontsouka B, Bozic J, Diress A, Ahmed T, Berthoud T, et al. An enveloped virus-like particle vaccine expressing a stabilized prefusion form of the SARS-CoV-2 spike protein elicits highly potent immunity. Vaccine (2021) 39(35):4988–5001. doi: 10.1016/j.vaccine.2021.07.034
150. Hager KJ, Perez Marc G, Gobeil P, Diaz RS, Heizer G, Llapur C, et al. Efficacy and safety of a recombinant plant-based adjuvanted covid-19 vaccine. N Engl J Med (2022) 386(22):2084–96. doi: 10.1056/NEJMoa2201300
151. Ward BJ, Seguin A, Couillard J, Trepanier S, Landry N. Phase III: Randomized observer-blind trial to evaluate lot-to-lot consistency of a new plant-derived quadrivalent virus like particle influenza vaccine in adults 18-49 years of age. Vaccine (2021) 39(10):1528–33. doi: 10.1016/j.vaccine.2021.01.004
152. D'Aoust MA, Couture MM, Charland N, Trepanier S, Landry N, Ors F, et al. The production of hemagglutinin-based virus-like particles in plants: a rapid, efficient and safe response to pandemic influenza. Plant Biotechnol J (2010) 8(5):607–19. doi: 10.1111/j.1467-7652.2009.00496.x
153. Shukla R, Beesetti H, Brown JA, Ahuja R, Ramasamy V, Shanmugam RK, et al. Dengue and zika virus infections are enhanced by live attenuated dengue vaccine but not by recombinant DSV4 vaccine candidate in mouse models. EBioMedicine (2020) 60:102991. doi: 10.1016/j.ebiom.2020.102991
154. Swaminathan S, Khanna N. Dengue vaccine development: Global and Indian scenarios. Int J Infect Dis (2019) 84S:S80–S6. doi: 10.1016/j.ijid.2019.01.029
155. Barsoe S, Skovgaard K, Sepulveda D, Stratmann A, Vendramin N, Lorenzen N. Nervous necrosis virus-like particle (VLP) vaccine stimulates European Sea bass innate and adaptive immune responses and induces long-term protection against disease. Pathogens (2021) 10(11). doi: 10.3390/pathogens10111477
156. Yang L, Xiao A, Wang H, Zhang X, Zhang Y, Li Y, et al. A VLP-based vaccine candidate protects mice against Japanese encephalitis virus infection. Vaccines (Basel) (2022) 10(2). doi: 10.3390/vaccines10020197
157. Du J, Gu Q, Liu Y, Li Q, Guo T, Liu Y. The endemic GII.4 norovirus-like-particle induced-antibody lacks of cross-reactivity against the epidemic GII.17 strain. J Med Virol (2021) 93(6):3974–9. doi: 10.1002/jmv.26474
158. Murata K, Lechmann M, Qiao M, Gunji T, Alter HJ, Liang TJ. Immunization with hepatitis c virus-like particles protects mice from recombinant hepatitis c virus-vaccinia infection. Proc Natl Acad Sci U S A (2003) 100(11):6753–8. doi: 10.1073/pnas.113192910
159. Elmowalid GA, Qiao M, Jeong SH, Borg BB, Baumert TF, Sapp RK, et al. Immunization with hepatitis c virus-like particles results in control of hepatitis c virus infection in chimpanzees. Proc Natl Acad Sci U S A (2007) 104(20):8427–32. doi: 10.1073/pnas.0702162104
160. Kumar A, Das S, Mullick R, Lahiri P, Tatineni R, Goswami D, et al. Immune responses against hepatitis c virus genotype 3a virus-like particles in mice: A novel VLP prime-adenovirus boost strategy. Vaccine (2016) 34(8):1115–25. doi: 10.1016/j.vaccine.2015.11.061
161. Dora EG, Rossi SL, Weaver SC, Tucker SN, Mateo R. An adjuvanted adenovirus 5-based vaccine elicits neutralizing antibodies and protects mice against chikungunya virus-induced footpad swelling. Vaccine (2019) 37(24):3146–50. doi: 10.1016/j.vaccine.2019.04.069
162. Cimica V, Saleem S, Matuczinski E, Adams-Fish D, McMahon C, Rashid S, et al. A virus-like particle-based vaccine candidate against the tick-borne powassan virus induces neutralizing antibodies in a mouse model. Pathogens (2021) 10(6). doi: 10.3390/pathogens10060680
163. Beltran-Pavez C, Bontjer I, Gonzalez N, Pernas M, Merino-Mansilla A, Olvera A, et al. Potent induction of envelope-specific antibody responses by virus-like particle immunogens based on HIV-1 envelopes from patients with early broadly neutralizing responses. J Virol (2022) 96(1):e0134321. doi: 10.1128/JVI.01343-21
164. McGregor J, Hardy JM, Lay CS, Boo I, Piontek M, Suckow M, et al. Virus-like particles containing the E2 core domain of hepatitis c virus generate broadly neutralizing antibodies in Guinea pigs. J Virol (2022) 96(5):e0167521. doi: 10.1128/jvi.01675-21
165. Cabral-Miranda G, Lim SM, Mohsen MO, Pobelov IV, Roesti ES, Heath MD, et al. Zika virus-derived e-DIII protein displayed on immunologically optimized VLPs induces neutralizing antibodies without causing enhancement of dengue virus infection. Vaccines (Basel) (2019) 7(3). doi: 10.3390/vaccines7030072
166. Li G, Liu L, Xu B, Hu J, Kuang H, Wang X, et al. Displaying epitope b and epitope 7 of porcine reproductive and respiratory syndrome virus on virus like particles of porcine circovirus type 2 provides partial protection to pigs. J Vet Med Sci (2021) 83(8):1263–72. doi: 10.1292/jvms.20-0543
167. Liu J, Zhang P, Chen Y, Zhong W, Li B, Pi M, et al. Vaccination with virus-like particles of atypical porcine pestivirus inhibits virus replication in tissues of BALB/c mice. Arch Virol (2021) 166(10):2733–41. doi: 10.1007/s00705-021-05185-w
168. Webster E, Seiger KW, Core SB, Collar AL, Knapp-Broas H, Graham J, et al. Immunogenicity and protective capacity of a virus-like particle vaccine against chlamydia trachomatis type 3 secretion system tip protein, CT584. Vaccines (Basel) (2022) 10(1). doi: 10.3390/vaccines10010111
169. Warner NL, Frietze KM. Development of bacteriophage virus-like particle vaccines displaying conserved epitopes of dengue virus non-structural protein 1. Vaccines (Basel) (2021) 9(7). doi: 10.3390/vaccines9070726
170. Malm M, Vesikari T, Blazevic V. Simultaneous immunization with multivalent norovirus VLPs induces better protective immune responses to norovirus than sequential immunization. Viruses (2019) 11(11). doi: 10.3390/v11111018
171. Zhang N, Zheng T, Chen Y, Zhu H, Qu Y, Zheng H, et al. Coxsackievirus B5 virus-like particle vaccine exhibits greater immunogenicity and immunoprotection than its inactivated counterpart in mice. Vaccine (2021) 39(39):5699–705. doi: 10.1016/j.vaccine.2021.07.095
172. Go HJ, Park BJ, Ahn HS, Kim DH, Kim DY, Kim JH, et al. Pigs immunized with the virus-like particle vaccine are protected against the hepatitis e-3 virus. Vaccines (Basel) (2021) 9(11). doi: 10.3390/vaccines9111265
173. Kang YM, Cho HK, Kim JH, Lee SJ, Park SJ, Kim DY, et al. Single dose of multi-clade virus-like particle vaccine protects chickens against clade 2.3.2.1 and clade 2.3.4.4 highly pathogenic avian influenza viruses. Sci Rep (2021) 11(1):13786. doi: 10.1038/s41598-021-93060-8
174. Lee SH, Chu KB, Kang HJ, Quan FS. Protection and alleviated inflammation induced by virus-like particle vaccines containing plasmodium berghei MSP-8, MSP-9 and RAP1. Vaccines (Basel) (2022) 10(2). doi: 10.3390/vaccines10020203
175. Kang HJ, Chu KB, Yoon KW, Eom GD, Mao J, Kim MJ, et al. Multiple neuraminidase containing influenza virus-like particle vaccines protect mice from avian and human influenza virus infection. Viruses (2022) 14(2). doi: 10.3390/v14020429
176. Hu J, Peng P, Li J, Zhang Q, Li R, Wang X, et al. Single dose of bivalent H5 and H7 influenza virus-like particle protects chickens against highly pathogenic H5N1 and H7N9 avian influenza viruses. Front Vet Sci (2021) 8:774630. doi: 10.3389/fvets.2021.774630
177. Tuse D, Malm M, Tamminen K, Diessner A, Thieme F, Jarczowski F, et al. Safety and immunogenicity studies in animal models support clinical development of a bivalent norovirus-like particle vaccine produced in plants. Vaccine (2022) 40(7):977–87. doi: 10.1016/j.vaccine.2022.01.009
178. Stander J, Chabeda A, Rybicki EP, Meyers AE. A plant-produced virus-like particle displaying envelope protein domain III elicits an immune response against West Nile virus in mice. Front Plant Sci (2021) 12:738619. doi: 10.3389/fpls.2021.738619
179. Harper DM, DeMars LR. HPV vaccines - a review of the first decade. Gynecol Oncol (2017) 146(1):196–204. doi: 10.1016/j.ygyno.2017.04.004
180. Monie A, Hung CF, Roden R, Wu TC. Cervarix: a vaccine for the prevention of HPV 16, 18-associated cervical cancer. Biologics (2008) 2(1):97–105.
181. Wang YQ, Bazin-Lee H, Evans JT, Casella CR, Mitchell TC. MPL adjuvant contains competitive antagonists of human TLR4. Front Immunol (2020) 11:577823. doi: 10.3389/fimmu.2020.577823
183. Wu T, Huang SJ, Zhu FC, Zhang XF, Ai X, Yan Q, et al. Immunogenicity and safety of hepatitis e vaccine in healthy hepatitis b surface antigen positive adults. Hum Vaccin Immunother (2013) 9(11):2474–9. doi: 10.4161/hv.25814
184. Li SW, Zhang J, Li YM, Ou SH, Huang GY, He ZQ, et al. A bacterially expressed particulate hepatitis e vaccine: antigenicity, immunogenicity and protectivity on primates. Vaccine (2005) 23(22):2893–901. doi: 10.1016/j.vaccine.2004.11.064
185. Van Den Ende C, Marano C, Van Ahee A, Bunge EM, De Moerlooze L. The immunogenicity and safety of GSK's recombinant hepatitis b vaccine in adults: a systematic review of 30 years of experience. Expert Rev Vaccines (2017) 16(8):811–32. doi: 10.1080/14760584.2017.1338568
186. Hussain Z, Ali SS, Husain SA, Raish M, Sharma DR, Kar P. Evaluation of immunogenicity and reactogenicity of recombinant DNA hepatitis b vaccine produced in India. World J Gastroenterol (2005) 11(45):7165–8. doi: 10.3748/wjg.v11.i45.7165
187. Shivananda, Somani V, Srikanth BS, Mohan M, Kulkarni PS. Comparison of two hepatitis b vaccines (GeneVac-b and engerix-b) in healthy infants in India. Clin Vaccine Immunol (2006) 13(6):661–4. doi: 10.1128/CVI.00087-06
188. Zhao Q, Towne V, Brown M, Wang Y, Abraham D, Oswald CB, et al. In-depth process understanding of RECOMBIVAX HB(R) maturation and potential epitope improvements with redox treatment: multifaceted biochemical and immunochemical characterization. Vaccine (2011) 29(45):7936–41. doi: 10.1016/j.vaccine.2011.08.070
189. Kushnir N, Streatfield SJ, Yusibov V. Virus-like particles as a highly efficient vaccine platform: diversity of targets and production systems and advances in clinical development. Vaccine (2012) 31(1):58–83. doi: 10.1016/j.vaccine.2012.10.083
190. Thakur V, Pati NT, Gupta RC, Sarin SK. Efficacy of shanvac-b recombinant DNA hepatitis b vaccine in health care workers of northern India. Hepatobil Pancreat Dis Int (2010) 9(4):393–7. doi: 10.37757/MR2021.V23.N1.6
191. Hernandez-Bernal F, Aguilar-Betancourt A, Aljovin V, Arias G, Valenzuela C, de Alejo KP, et al. Comparison of four recombinant hepatitis b vaccines applied on an accelerated schedule in healthy adults. Hum Vaccin (2011) 7(10):1026–36. doi: 10.4161/hv.7.10.15989
192. Penton-Arias E, Aguilar-Rubido JC. Cuban Prophylactic and therapeutic vaccines for controlling hepatitis b. MEDICC Rev (2021) 23(1):21–9. doi: 10.37757/MR2021.V23.N1.6
193. Rebedea I, Diaconescu IG, Bach D, Bartelsen O, Arndtz N. Comparison of thiomersal-free and thiomersal-containing formulations of a recombinant hepatitis b vaccine (Hepavax-gene) in healthy adults. Vaccine (2006) 24(25):5320–6. doi: 10.1016/j.vaccine.2006.04.018
194. Villa LL, Costa RLR, Petta CA, Andrade RP, Ault KA, Giuliano AR, et al. Prophylactic quadrivalent human papillomavirus (types 6, 11, 16 and 18) L1 virus-like particle vaccine in young women: A randomized double-blind placebo-controlled multicentre phase II efficacy trial. Lancet Oncol (2005) 6:271–8. doi: 10.1016/S1470-2045(05)70101-7
Keywords: virus like particles, enveloped VLP, non-enveloped VLP, chimeric VLP, vaccine, recombinant DNA technology
Citation: Gupta R, Arora K, Roy SS, Joseph A, Rastogi R, Arora NM and Kundu PK (2023) Platforms, advances, and technical challenges in virus-like particles-based vaccines. Front. Immunol. 14:1123805. doi: 10.3389/fimmu.2023.1123805
Received: 14 December 2022; Accepted: 30 January 2023;
Published: 09 February 2023.
Edited by:
Piero Pileri, Toscana Life Sciences, ItalyReviewed by:
Marc Paul Girard, Université Paris Cité, FranceBertrand Bellier, Sorbonne Universités, France
Pascal Fender, UMR5075 Institut de Biologie Structurale (IBS), France
Copyright © 2023 Gupta, Arora, Roy, Joseph, Rastogi, Arora and Kundu. This is an open-access article distributed under the terms of the Creative Commons Attribution License (CC BY). The use, distribution or reproduction in other forums is permitted, provided the original author(s) and the copyright owner(s) are credited and that the original publication in this journal is cited, in accordance with accepted academic practice. No use, distribution or reproduction is permitted which does not comply with these terms.
*Correspondence: Prabuddha K. Kundu, c2NpZW5jZWFkdjEzQGdtYWlsLmNvbQ==; cHJhYnVkZGhhLmt1bmR1QHByZW1hc2Jpb3RlY2guY29t