- 1Respiratory & Exercise Physiology, The Lundquist Institute for Biomedical Innovation at Harbor University of California Los Angeles (UCLA) Medical Center, Torrance, CA, United States
- 2Division of Respiratory and Critical Care Medicine and Physiology, David Geffen School of Medicine, University of California Los Angeles (UCLA), Los Angeles, CA, United States
Sensory neurons cooperate with barrier tissues and resident immune cells to form a significant aspect of defensive strategies in concert with the immune system. This assembly of neuroimmune cellular units is exemplified across evolution from early metazoans to mammalian life. As such, sensory neurons possess the capability to detect pathogenic infiltrates at barrier surfaces. This capacity relies on mechanisms that unleash specific cell signaling, trafficking and defensive reflexes. These pathways exploit mechanisms to amplify and enhance the alerting response should pathogenic infiltration seep into other tissue compartments and/or systemic circulation. Here we explore two hypotheses: 1) that sensory neurons’ potential cellular signaling pathways require the interaction of pathogen recognition receptors and ion channels specific to sensory neurons and; 2) mechanisms which amplify these sensing pathways require activation of multiple sensory neuron sites. Where possible, we provide references to other apt reviews which provide the reader more detail on specific aspects of the perspectives provided here.
Introduction
Without the ability to detect, combat and evade harmful pathogens, the survival of early metazoan and, evolution to mammalian life would not have been possible (1). The importance of pathogenic detection and mitigation is evidenced by innate systems, mostly confined to the earliest sensory units, which originated in early multicellular organisms such as Caenorhabditis elegans (2, 3) and Hydra (4, 5). The mammalian development of pathogenic control was enabled by the divergence from one to two systems which evolved in parallel but in a mutually interrelated manner (1, 3). Mammalian immune and nervous systems are organized in such a way that sentinel cells, with pathogenic detecting capabilities emanate alerting signals. When amplified chemical messengers are released to recruit combative cells that attack and/or eliminate invading threats (1).
Layers of defenses are involved in the intricacy of the immune system. Structural barrier cells within epithelial barrier layers coordinate a signalling cascade to recruit additional patrolling and cytotoxic cells, granulocytes, monocytes and lymphocytes to act and counter the invading threat. Following the cellular signalling posed by the epithelium, the immune system is then organized into lymphoid and secondary lymphoid organs, which harbor lymphoid-lineage cells that enable bi-directional communication with myeloid lineage cells, which have multiple methods to neutralize pathogens. Lymphoid lineage cells are triggered by numerous signals, simultaneously deployed at any one time, which was thought initially to be a failsafe system to back-up disruptions to poorly performing pathways (6, 7). However, the diversity and coordination of multiple cytokine and chemokine release appear to mediate the specificity of immune cell activation in response to pathogenic detection. This is due to the unique receptor gene expression profile on any single immune cell, providing a unique signature and the potential for differing modes of activation (8–10). Thus, deployment of a specific cytokine and chemokine pattern would then, presumably, activate a particular set of cells, in an exact way, to target a specific pathogen.
Like the immune system, the nervous system is partitioned to enable the sensing of the environment (sensory neurons within the peripheral nervous system), integrate and process information (medullary autonomic nervous system – and central hypothalamic centers) and finally, carry out signalling pathways (efferent nerves of the autonomic nervous system) to implement reflexes to receive information regarding deviations from homeostasis (1, 3). In the case of immediate pathogenic invasion, sensory neurons innervate all barrier (11–13) and mucosal surfaces (11–13) and patrol the bloodstream (14–16); interfaces which are vulnerable to infection and can come into contact with the outside environment. These important neural sensory clusters lie within the dorsal root ganglion (13), trigeminal ganglion (17), nodose/jugular ganglion (11, 18) and petrosal ganglion (14, 18), respectively. These neurons communicate with immune (19) and neuroendocrine cells (20, 21), either by the direct release of neurotransmitters into the immediate vicinity (19) or by relaying more extensive neural networks to innervate secondary lymphoid organs (22) and recruit other cells via secondary neurotransmitter release.
As such, it is clear that the anatomical association of sensory neurons with epithelial layers and indwelling immune cells form “neuroimmune cell units” (23) where their bidirectional communication between distinct neuronal and hematopoietic lineage cell types has afforded the ability to direct specific responses to a multitude of homeostatic disturbances. Prominent examples of the effects of these neuroimmune cell units are the cholinergic anti-inflammatory pathway (24, 25) or sympathetic influence on lymphopoiesis (26, 27). These modes of neural stimulation of immune function provide a sensory system which can quickly act should it identify pathogenic infiltration. Notably, the stimulation of this fast-acting sensory neuron pathogenic detection has been demonstrated to either assist with ‘immune’ cell recruitment (19) or provide antimicrobial protection in and of itself through antimicrobial neuropeptides (28). If the specific recruitment of immune cell subsets occurs due to the unique chemokine receptor expression signature on any immune cell (5, 6), what is the mode of specific sensory neuron activation in the face of pathogenic detection?
Early sensory systems are a blueprint for neuroimmune cell units
Pathogen recognition in early metazoan life likely developed in sensory neurons first (3). These sensory units acquired innate defenses to detect and counter initial pathogenic infiltration. The concurrent development of early PRRs and ion channels shows a sharing of potential physiologic outcomes in response to pathogen detection. Both pathogenic opsonization and sensory neuron depolarization induce avoidance behaviors brought on upon specific neurotransmitter release. This subsequent release of neuropeptides also demonstrates microbial neutralization properties akin to bacteriocins (29, 30); which appears to be conserved in mammalian life (28). Neuronal cell death to implement calcium release for both additional cell recruitment and adjacent neuron stimulation (31, 32) was an additional strategy of early alerting responses and, in select cases, is a last resort for mammalian defences (33). Intriguingly, it would appear that apoptotic signalling and not apoptosis per se, are necessary for neuroimmune interactions as blockade of cell death does not diminish immune cell trafficking (34). Nevertheless, these early alerting responses occur rapidly, and it has been suggested that the speed of the neural response may influence the speed of the subsequent immune response (3, 34); a relationship which appears to hold in mammalian life (19, 33). However, early strategies evolved and gained more specificity with the increased dispersion of duties amongst cell types. The conservation of intracellular signalling pathways throughout the evolution of defensive strategies is exemplified in neuroimmune cell units (11–13, 35–39). How these signalling systems interact, and their functional outcome are now the focus of intense investigation.
Hypotheses on the specificity of sensory neuron PRR sensing of microbes
In the case of sensory neuron depolarization, ion channels often form the terminal portion of the cell signalling cascade as these channels are phosphorylated by upstream kinases set in motion when receptors are activated (40). The phosphorylation of ion channels on sensory neurons then increases the probability of depolarization, leading to neuronal excitation and neurotransmitter release (41, 42). Neuronal ion channel expression has allowed the sorting/clustering of neuronal subsets (11–13, 35–39). It is likely that the complexity of neural depolarization mediated by the activation of multiple but sensory-specific ion channels in various ganglia would also enable a failsafe method to carry important information to the central nervous system (35, 43). It is equally likely that the specificity of neuronal activation also involves the activation of multiple receptors on any one neuron to offer specific neuron excitation. A notion carried from specific lymphoid cell activation afforded by chemokine receptor expression (8–10).
Furthermore, how and at which site of phosphorylation of each channel could offer another layer of complexity to add to the specific coding of neuronal activation by upstream receptors. Finally, subclusters of specific sensory neurons exist in multiple ganglia; can stimulation of neurons in multiple ganglia may augment neuroimmune reflexes? Therefore, the perspectives explored here are: whether the stimulation of multiple neurons within or between sensory ganglia, are phosphorylated differentially, and on differential phosphorylation sites, to provide a mechanism to code specific information to engage the appropriate neuroimmune reflex. In addition, the activation of ion channel phosphorylation on more than one set of nerves (ganglia) could augment these alerting reflexes.
Evidence for specificity of neuronal sub-clusters and ion channel phosphorylation as a means for specific neural responses
Detection of pathogenic invasion by sensory neurons, epithelial/barrier and innate antigen sensing/presenting immune cells is afforded by direct and indirect means (44). The direct recognition of pathogen-associated molecular patterns (PAMPs) and, therefore, neural activation involves four families of PRRs, including Toll-like receptors (TLRs), NOD-like receptors (NLRs), retinoic acid-inducible gene I (RIG-I)-like receptors (RLRs), formyl peptide receptors (FPRs) and C-lectin receptors (CLRs), each of which show notable differences regarding pathogen recognition, signal transduction, and intracellular downstream pathways (45–51) (Figure 1). The indirect activation of sensory neurons by pathogenic stimuli involves cytokines, chemokine, prostaglandin and, phospholipid stimulation of sensory neurons as a result of their release from either myeloid and lymphoid cells or, on neurons themselves (44, 80).
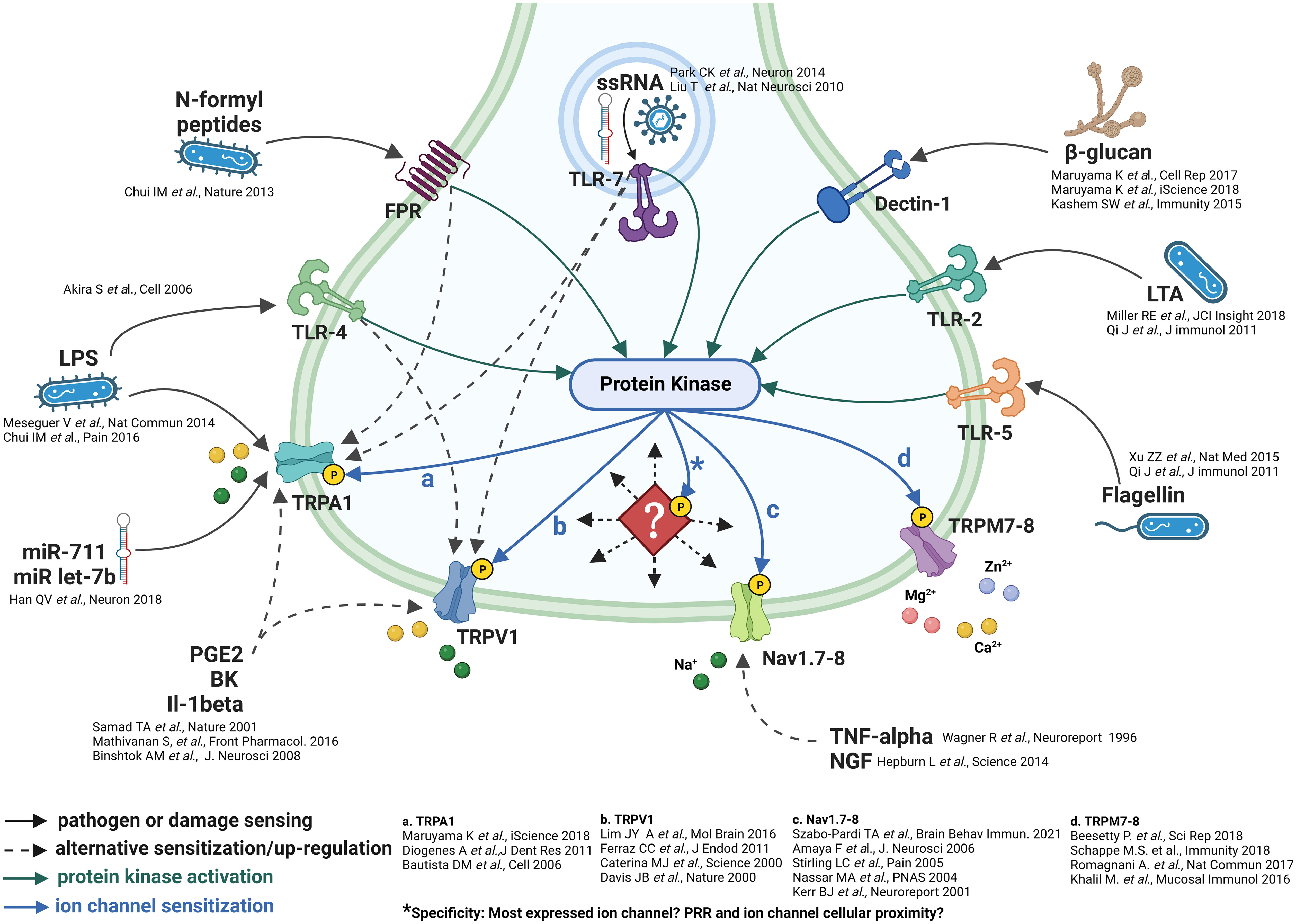
Figure 1 Neuronal pathogenic detection and cellular signaling pathways. Do ion channels incur specificity? The pathogenic sensing capability of sensory neurons is exemplified by the multitude of pathogenic detection receptors (51–79). These receptors appear to be linked spatially and by protein kinase stimulation/phosphorylation of downstream ion channels. *However, as indicated by the diamond, what forces direct the specific trafficking of protein kinases to specific phosphorylation sites on downstream ion channels? And, to which specific ion channel/set of ion channels?
The differential expression of PRRs, cytokine, chemokine, prostaglandin and phospholipid receptors in sensory neurons has been shown in DRGs and trigeminal ganglia, nodose/jugular ganglia and carotid chemoreceptors using a multitude of transcriptomic technologies including single-cell/differential RNA sequencing (sc/dRNA-seq), in situ hybridization, immunohistochemistry, and electrophysiology (11–13, 35–39). This potential for neuronal activation by multiple ligands is clearly a distinct possibility, both by direct (sensing of microbes by PRRs) and indirect (sensing of cytokines released by immune cell sensing of microbes and recognition of alarmins which are produced or released by damaged and dying cells) means. Is it possible that the composition of these molecules released upon initial immune cell sensing and the direct pathogenic stimulation of neurons can code specific neuronal signalling? To test this hypothesis would require a multitude of conditions with differing amounts and compositions of ligands in response to different pathogenic conditions; a difficult task which may be made easier with new-generation bioinformatic approaches involving RNA-protein interactions or the functional processing of neural activity. However, this same stimulation would also activate many other cell types with a similar receptor signature. Therefore, an additional layer of neuronal specificity is required to allow sensory neuron signaling specificity.
The composition of downstream ion channels is the defining characteristic of sensory neurons (11–13, 41). Protein phosphorylation is the most important post-translational protein modification which regulates enzymatic and ion channel activity, cell signalling and cellular localization (40, 81–83). Given the prominent role of protein kinases, which are readily activated as part of multiple signaling pathways (84–86), it is likely that their deployment can elicit downstream post-translational modification of ion channels to increase the probability of depolarization and/or neurotransmitter release which, in turn, could code specific nociceptor signals (Figure 1).
There are multiple examples of PRR receptors eliciting the engagement of kinases, including protein kinases, tyrosine, and Syk-family kinases as part of both PRR signalling (84–87) and indirect signaling from phospholipid, prostaglandin and cytokine receptors (87, 88). Further, the role phosphorylation plays in the modification of ion channel function in regards to neuronal depolarization has been documented in response, mainly, to GPCR mediated kinase activation such as prostaglandins and phospholipids (89, 90), and in response to some, but not all, neuroimmune conditions, examples include itch (91) and pain (92, 93). Subsequent activation of kinases in association with PRRs such as TLRs or CLRs and cytokines are certainly evidenced (87); whether their kinase activation results in ion channel depolarization or sensitization in these cases is not clear (Figure 1).
An additional possibility, in terms of the specificity of neuronal activation, appears to involve multiple phosphorylation sites per ion channel. The most prominent examples of these, within sensory neurons, lie with TRPV1, TRPC3 and Nav channels. Indeed, TRPV1 has three prominent phosphorylation sites, S502, S800 and T704 (94, 95), TRPC3 is phosphorylated on T11, S263, T646 and (96–98), and sensory neuron specific Nav channels, subtypes 1.8 and 1.9 are phosphorylated on D1-DII linker sites (99, 100). Each of these sites accepts kinases that appear to be directed in response to specific ligands such as prostaglandins and cytokines (40). This array of cellular signaling may be a manner in which multiple ion channels may be simultaneously phosphorylated and afford specificity similar to the multiple chemokine and cytokine stimulation of immune cells (44). The wide-ranging consequences of the multiplicity of signals contributing to ion channel phosphorylation could result in either pro- or anti-inflammatory responses. But an essential factor, which remains unanswered, is how the activation of kinases by specific receptors are directed/trafficked to particular phosphorylation sites on specific ion channels. There are examples of apparent coupling between PRRs and TRPs, such as TLR4 to TRPV1 and TLR7 to TRPA1 (44). What is a more probable cellular signalling scenario is that the dispersion of kinases, in response to stimulation, by a multitude of receptors, phosphorylates/post-translationally modifies the composition of channels present within each specific neuron subset. This would speak to the mode of specificity mentioned above. However, this hypothesis remains to be tested.
Evidence for multiple ganglion stimulation and alteration of defensive reflexes
In early eukaryotic life, the capability of neural signals to recruit cells from seemingly ‘distant’ sites (3, 101) demonstrated the capability for early neuroimmune cell unit coordination and broader protective capabilities. However, as eukaryotic life gained the complexity and partitioning of organ systems, both the immune and nervous systems organized themselves by sub-dividing specific hubs for either the storage of cells (e.g. secondary lymphoid organs, resident tissue cells) or to serve as a relay station for information (e.g. neural ganglia).
In the case of the sensory nervous system, necessary to form a part of the neuroimmune landscape, neurons are sorted and partitioned into different peripheral ganglia which innervate different organ systems or components of organ systems (19, 102). For example, the dorsal root ganglia innervate the skin (13, 102). In contrast, the vagus senses internal organs, and each neuron/neuron subset is responsible for a single organ system (11, 35, 102) and, possibly, tissue layer (11, 103, 104).
Signals from sensory neurons travel to the nucleus tractus solitarius and synapse onto the dorsal motor nucleus of the vagus (to elicit immediate efferent nerve activity) and the paraventricular nucleus within the hippocampus, amygdala and periaqueductal grey (to carry out grander neuroendocrine signalling involving the hypothalamic pituitary adrenal axis; HPA). Effective neural signalling of immunity is critically dependent on adrenal cortex release of glucocorticoids in response to a number of perturbations (105, 106) The integration of the neuroendocrine involvement in neuroimmune signalling is highlighted by stress responses (involved in a wide variety of threats to homeostasis) which signals the adrenal cortex to induce corticosteroid release which has a wide array of immune influence (105–107). Additionally, new and important reflexes of HPA involvement are highlighted with the cholinergic anti-inflammatory pathway (108) and humoral immunity (109) in response to infection.
Intriguingly, disease and its severity can often be magnified when it is ‘leaked’ beyond the initial site of infection into other tissue layers, organ systems and/or the blood; examples of these lie with infections that can quickly precipitate sepsis (16). Therefore, a situation may arise where multiple neurons that may signal differing ganglia could signal an alerting response should the disease spread beyond the initial site/tissue of infection.
One such case where infection could travel beyond the initial site of infection but remain within a single organ system, yet stimulate multiple sensory neurons which are housed in differing ganglia, is exemplified in the gut (103). Indeed, the colon is partitioned into intrinsic and extrinsic sensory neurons, with cell bodies in the intrinsic and nodose ganglia, respectively (103) (Figure 2). It is likely that stimulation of both ganglia by the detection of pathogenic harm by both sets of sensory neurons may provide an increased alerting response.
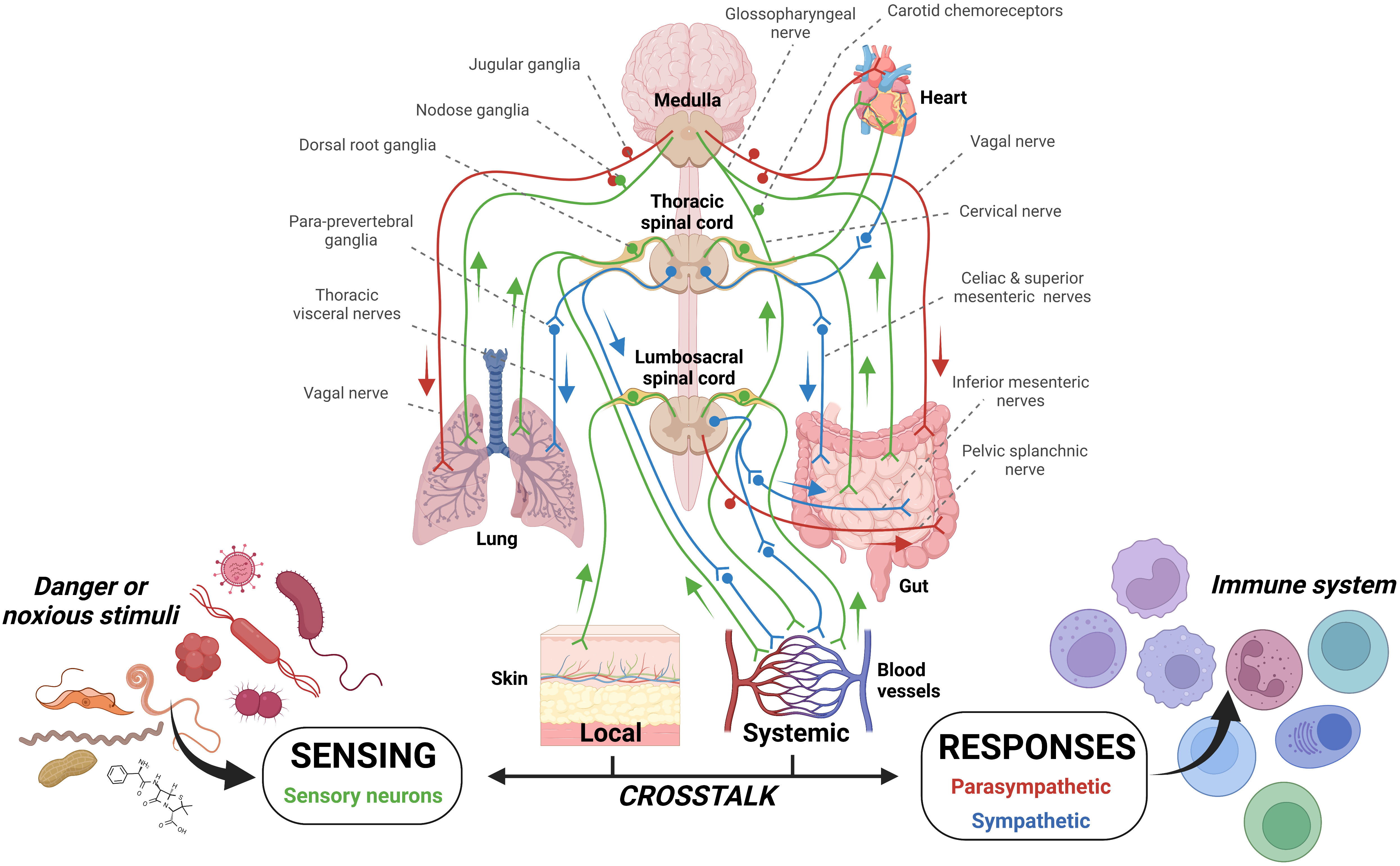
Figure 2 Sensory innervation of barrier tissues. The innervation of tissues by sensory neurons and the parasympathetic and sympathetic arms of the autonomic nervous system affords constant patrol of mammalian systems. In addition, the enteric nervous system has a layered system of innervation within the gut. These systems allow constant detection and also the possibility of dual stimulation should multiple neurons in different locations/tissues be stimulation. Can parallel and concurrent sensory neuron pathogenic stimulation increase signaling magnitude? The potential for this case has been examined previously (43, 103).
Indeed, the above discussion readily acknowledges that the first line of neuroimmune defence occurs with the release of these sensory neurotransmitters which act to recruit the appropriate cell to clear the pathogenic invasion. However, should disease progress to such a state where barrier surfaces become leaky/decoupled and allow the passage of microbial pathogens and/or cytokines into the bloodstream, additional defenses would be stimulated. In this regard, an additional line of defense would be recruited if pathogenic infiltration should enter the blood stream; the carotid chemoreceptors (16) the main sensory organ which patrols hemolytic homeostasis (15) would also be stimulated. Evidence demonstrates the carotid chemoreceptors’ ability to detect pathogenic patterns (16) as well as substances released in response to disease states/allergy (110, 111) as their composition of pathogenic detection is notable (38). We have demonstrated the possibility for this biologic scenario to occur in our dual vagus and carotid chemoreceptor preparation (43). Specifically, we showed that when the sensory neurons within the lungs are stimulated with either proteases or adenosine triphosphate and the carotid chemoreceptors with their known stimulus, low oxygen tension of their perfusate (blood), signals emanating to the efferent vagus are augmented, likely to regulate defensive reflexes such as mucus secretion and airway constriction (35) and/or to signal the cholinergic anti-inflammatory pathway (24, 25). This scenario shows that not only are subtypes of neurons within ganglia able to regulate and code specific neural signals in response to pathogenic stimulation but, also that augmentation of reflexes is possible when anatomically specific and important ganglia are stimulated at the same time. Therefore, the cascading stimulation of sensory neurons would provide the potential to augment and/or strengthen the neural coordination of immune cells, should pathogenic stimuli be sensed by multiple immune capable ganglia. In the context of specific coding of neural depolarization by the expression of differential receptors, ion channels and their respective phosphorylation, how would stimulation of two neuron sets with similar receptor/ion channel composition augment neuroimmune signalling? One possibility would simply be an increased rate of neuronal firing to elicit immunogenic neurotransmitter release. How would dual stimulation of two neuron sets with differing receptor/ion channel composition augment neuroimmune signalling? Would such an instance change the composition of neurotransmitter release and ultimately affect the chemotaxis of immune cells?
Perspectives/conclusions
Protection and evasion of disease has required an ever-evolving system with additive layers throughout the evolution of eukaryotic life. With the increasing complexity of mammalian body plans, host defence involves coordinating two defensive systems and organising cell clusters within and between systems. This perspectives essay has attempted to highlight how sensory neurons, an important part of the defensive response to microbial invasion, code specific information to either influence neuronal and immune cell stimulation at the site of infection. Additionally, should invasion extend beyond this site, larger reflexes which may be required to clear the pathogenic infiltration would become activated. However, much remains to be uncovered and, as recently posed by others (44), information regarding what this excitation means in a (patho)physiologic context and how it coordinates the release of neurotransmitter to act directly on immune cells or signal the central nervous system to coordinate grander neuro-immune regulation requires further intense investigation.
Data availability statement
The original contributions presented in the study are included in the article/supplementary material. Further inquiries can be directed to the corresponding author.
Author contributions
All authors contributed to the conception and composition of the manuscript. AM completed the figures. All authors approve the manuscript in the submitted form.
Funding
The Jendzjowsky laboratory is supported by NIH NIAID R21AI159221, TRDRP T32IP4707, T32KT4708 and UCLA CTSI UL1TR001881.
Acknowledgments
All images were created using Biorender. We thank Drs. Soula Danopoulos, Delphine Lee and Marc Swidergall (The Lundquist Institute) and Gabrielle Dyson for their critical review of our manuscript.
Conflict of interest
The authors declare that the research was conducted in the absence of any commercial or financial relationships that could be construed as a potential conflict of interest.
Publisher’s note
All claims expressed in this article are solely those of the authors and do not necessarily represent those of their affiliated organizations, or those of the publisher, the editors and the reviewers. Any product that may be evaluated in this article, or claim that may be made by its manufacturer, is not guaranteed or endorsed by the publisher.
References
1. Veiga-Fernandes H, Freitas AA. The s(c)ensory immune system theory. Trends Immunol (2017) 38(10):777–88. doi: 10.1016/j.it.2017.02.007
2. Wani KA, Goswamy D, Irazoqui JE. Nervous system control of intestinal host defense in c. elegans. Curr Opin Neurobiol (2020) 62:1–9. doi: 10.1016/j.conb.2019.11.007
3. Kraus A, Buckley KM, Salinas I. Sensing the world and its dangers: an evolutionary perspective in neuroimmunology. eLife (2021) 10:e66706. doi: 10.7554/eLife.66706
4. Klimovich AV, Bosch TCG. Rethinking the role of the nervous system: lessons from the hydra holobiont. Bioessays (2018) 40(9):e1800060. doi: 10.1002/bies.201800060
5. Franzenburg S, Fraune S, Künzel S, Baines JF, Domazet-Loso T, Bosch TCG. MyD88-deficient hydra reveal an ancient function of TLR signaling in sensing bacterial colonizers. Proc Natl Acad Sci U.S.A. (2012) 109(47):19374–9. doi: 10.1073/pnas.1213110109
6. O’Boyle G. The yin and yang of chemokine receptor activation. Br J Pharmacol (2012) 166(3):895–7. doi: 10.1111/j.1476-5381.2011.01759.x
7. Proudfoot AEI, Bonvin P, Power CA. Targeting chemokines: pathogens can, why can’t we? Cytokine (2015) 74(2):259–67. doi: 10.1016/j.cyto.2015.02.011
8. Dyer DP. Understanding the mechanisms that facilitate specificity, not redundancy, of chemokine-mediated leukocyte recruitment. Immunology (2020) 160(4):336–44. doi: 10.1111/imm.13200
9. Ridley AJ, Ou Y, Karlsson R, Pun N, Birchenough HL, Jowitt TA, et al. Chemokines form complex signals during inflammation and disease that can be decoded by extracellular matrix proteoglycans. bioRxiv (2022), 508420. doi: 10.1101/2022.09.20.508420
10. Dyer DP, Medina-Ruiz L, Bartolini R, Schuette F, Hughes CE, Pallas K, et al. Chemokine receptor redundancy and specificity are context dependent. Immunity (2019) 50(2):378–389.e5. doi: 10.1016/j.immuni.2019.01.009
11. Kupari J, Häring M, Agirre E, Castelo-Branco G, Ernfors P. An atlas of vagal sensory neurons and their molecular specialization. Cell Rep (2019) 27(8):2508–2523.e4. doi: 10.1016/j.celrep.2019.04.096
12. Häring M, Zeisel A, Hochgerner H, Rinwa P, Jakobsson JET, Lönnerberg P, et al. Neuronal atlas of the dorsal horn defines its architecture and links sensory input to transcriptional cell types. Nat Neurosci (2018) 21(6):869–80. doi: 10.1038/s41593-018-0141-1
13. Usoskin D, Furlan A, Islam S, Abdo H, Lönnerberg P, Lou D, et al. Unbiased classification of sensory neuron types by large-scale single-cell RNA sequencing. Nat Neurosci (2015) 18(1):145–53. doi: 10.1038/nn.3881
14. Ackland GL, Kazymov V, Marina N, Singer M, Gourine AV. Peripheral neural detection of danger-associated and pathogen-associated molecular patterns. Crit Care Med (2013) 41(6):e85–92. doi: 10.1097/CCM.0b013e31827c0b05
15. Prabhakhar NR, Joyner MJ. Tasting arterial blood: what do the carotid chemoreceptors sense? Front Physiol (2015) 5:524. doi: 10.3389/fphys.2014.00524
16. Katayama PL, Leirão IP, Kanashiro A, Luiz JPM, Cunha FQ, Navegantes LCC, et al. The carotid body detects circulating tumor necrosis factor-alpha to activate a sympathetic anti-inflammatory reflex. Brain Behav Immun (2022) 102:370–86. doi: 10.1016/j.bbi.2022.03.014
17. de Moraes ER, Kushmerick C, Naves LA. Morphological and functional diversity of first-order somatosensory neurons. Biophys Rev (2017) 9(5):847–56. doi: 10.1007/s12551-017-0321-3
18. Prescott SL, Liberles SD. Internal senses of the vagus nerve. Neuron (2022) 110:P579–599. doi: 10.1016/j.neuron.2021.12.020
19. Udit S, Blake K, Chiu IM. Somatosensory and autonomic neuronal regulation of the immune response. Nat Rev Neurosci. (2022) 23:57–171. doi: 10.1038/s41583-021-00555-4
20. Huang Y, Zhao C, Su X. Neuroimmune regulation of lung infection and inflammation. QJM (2019) 112(7):483–7. doi: 10.1093/qjmed/hcy154
21. Quatrini L, Vivier E, Ugolini S. Neuroendocrine regulation of innate lymphoid cells. Immunol Rev (2018) 286(1):120–36. doi: 10.1111/imr.12707
22. De Virgiliis F, Oliva VM, Kizil B, Scheiermann C. Control of lymph node activity by direct local innervation. Trends Neurosci (2022) 45(9):704–12. doi: 10.1016/j.tins.2022.06.006
23. Godinho-Silva C, Cardoso F, Veiga-Fernandes H. Neuro-immune cell units: a new paradigm in physiology. Annu Rev Immunol (2019) 37:19–46. doi: 10.1146/annurev-immunol-042718-041812
24. Tracey KJ. Approaching the next revolution? evolutionary integration of neural and immune pathogen sensing and response [Internet]. Cold Spring Harb Perspect Biol (2015) 7(2). doi: 10.1101/cshperspect.a016360
26. Hanoun M, Maryanovich M, Arnal-Estapé A, Frenette PS. Neural regulation of hematopoiesis, inflammation, and cancer. Neuron (2015) 86(2):360–73. doi: 10.1016/j.neuron.2015.01.026
27. Katayama Y, Battista M, Kao W-M, Hidalgo A, Peired AJ, Thomas SA, et al. Signals from the sympathetic nervous system regulate hematopoietic stem cell egress from bone marrow. Cell (2006) 124(2):407–21. doi: 10.1016/j.cell.2005.10.041
28. Lee EY, Chan LC, Wang H, Lieng J, Hung M, Srinivasan Y, et al. PACAP is a pathogen-inducible resident antimicrobial neuropeptide affording rapid and contextual molecular host defense of the brain. Proc Natl Acad Sci U.S.A. (2021) 118(1):e1917623117. doi: 10.1073/pnas.1917623117
29. Hanson MA, Lemaitre B, Unckless RL. Dynamic evolution of antimicrobial peptides underscores trade-offs between immunity and ecological fitness. Front Immunol (2019) 10:2620. doi: 10.3389/fimmu.2019.02620
30. Lezi E, Zhou T, Koh S, Chuang M, Sharma R, Pujol N, et al. An antimicrobial peptide and its neuronal receptor regulate dendrite degeneration in aging and infection. Neuron (2018) 97(1):125–138.e5. doi: 10.1016/j.neuron.2017.12.001
31. Ma Y, Li J, Chiu I, Wang Y, Sloane JA, Lü J, et al. Toll-like receptor 8 functions as a negative regulator of neurite outgrowth and inducer of neuronal apoptosis. J Cell Biol (2006) 175(2):209–15. doi: 10.1083/jcb.200606016
32. Kamm K, Schierwater B, DeSalle R. Innate immunity in the simplest animals - placozoans. BMC Genomics (2019) 20(1):5. doi: 10.1186/s12864-018-5377-3
33. Neel DV, Basu H, Gunner G, Chiu IM. Catching a killer: mechanisms of programmed cell death and immune activation in amyotrophic lateral sclerosis. Immunol Rev (2022) 311(1):130–50. doi: 10.1111/imr.13083
34. Sepahi A, Kraus A, Casadei E, Johnston CA, Galindo-Villegas J, Kelly C, et al. Olfactory sensory neurons mediate ultrarapid antiviral immune responses in a TrkA-dependent manner. PNAS (2019) 116(25):12428–36. doi: 10.1073/pnas.1900083116
35. Prescott SL, Umans BD, Williams EK, Brust RD, Liberles SD. An airway protection program revealed by sweeping genetic control of vagal afferents. Cell (2020) 181(3):574–589.e14. doi: 10.1016/j.cell.2020.03.004
36. Zeisel A, Hochgerner H, Lönnerberg P, Johnsson A, Memic F, van der Zwan J, et al. Molecular architecture of the mouse nervous system. Cell (2018) 174(4):999–1014.e22. doi: 10.1016/j.cell.2018.06.021
37. Zheng Y, Liu P, Bai L, Trimmer JS, Bean BP, Ginty DD. Deep sequencing of somatosensory neurons reveals molecular determinants of intrinsic physiological properties. Neuron (2019) 103(4):598–616.e7. doi: 10.1016/j.neuron.2019.05.039
38. Zhou T, Chien M, Kaleem S, Matsunami H. Single cell transcriptome analysis of mouse carotid body glomus cells. J Physiol (2016) 594(15):4225–51. doi: 10.1113/JP271936
39. Chang AJ. Acute oxygen sensing by the carotid body: from mitochondria to plasma membrane. J Appl Physiol (2017) 123(5):1335–43. doi: 10.1152/japplphysiol.00398.2017
40. Voolstra O, Huber A. Post-translational modifications of TRP channels. Cells (2014) 3(2):258–87. doi: 10.3390/cells3020258
41. Nilius B, Owsianik G. The transient receptor potential family of ion channels. Genome Biol (2011) 12(3):218. doi: 10.1186/gb-2011-12-3-218
42. Chahine M, O’Leary ME. Regulation/modulation of sensory neuron sodium channels. Handb Exp Pharmacol (2014) 221:111–35. doi: 10.1007/978-3-642-41588-3_6
43. Zyuzin J, Jendzjowsky N. Neuroanatomical and neurophysiological evidence of pulmonary nociceptor and carotid chemoreceptor convergence in the nucleus tractus solitarius and nucleus ambiguus. J Neurophysiol (2022) 127(6):1511–8. doi: 10.1152/jn.00125.2022
44. Donnelly CR, Chen O, Ji R-R. How do sensory neurons sense danger signals? Trends Neurosci (2020) 43(10):822–38. doi: 10.1016/j.tins.2020.07.008
45. Takeuchi O, Akira S. Pattern recognition receptors and inflammation. Cell (2010) 140(6):805–20. doi: 10.1016/j.cell.2010.01.022
46. Kumar H, Kawai T, Akira S. Pathogen recognition in the innate immune response. Biochem J (2009) 420(1):1–16. doi: 10.1042/BJ20090272
47. Carneiro L a. M, Magalhaes JG, Tattoli I, Philpott DJ, Travassos LH. Nod-like proteins in inflammation and disease. J Pathol (2008) 214(2):136–48. doi: 10.1002/path.2271
48. Kufer TA, Banks DJ, Philpott DJ. Innate immune sensing of microbes by nod proteins. Ann N Y Acad Sci (2006) 1072:19–27. doi: 10.1196/annals.1326.020
49. Goubau D, Deddouche S, Reis e Sousa C. Cytosolic sensing of viruses. Immunity (2013) 38(5):855–69. doi: 10.1016/j.immuni.2013.05.007
50. Abe T, Marutani Y, Shoji I. Cytosolic DNA-sensing immune response and viral infection. Microbiol Immunol (2019) 63(2):51–64. doi: 10.1111/1348-0421.12669
51. Chiu IM, Heesters BA, Ghasemlou N, Von Hehn CA, Zhao F, Tran J, et al. Bacteria activate sensory neurons that modulate pain and inflammation. Nature (2013) 501(7465):52–7. doi: 10.1038/nature12479
52. Akira S, Uematsu S, Takeuchi O. Pathogen recognition and innate immunity. Cell (2006) 124(4):783–801. doi: 10.1016/j.cell.2006.02.015
53. Meseguer V, Alpizar YA, Luis E, Tajada S, Denlinger B, Fajardo O, et al. TRPA1 channels mediate acute neurogenic inflammation and pain produced by bacterial endotoxins. Nat Commun (2014) 5:3125. doi: 10.1038/ncomms4125
54. Chiu IM, Pinho-Ribeiro FA, Woolf CJ. Pain and infection: pathogen detection by nociceptors. Pain (2016) 157(6):1192–3. doi: 10.1097/j.pain.0000000000000559
55. Han Q, Liu D, Convertino M, Wang Z, Jiang C, Kim YH, et al. miRNA-711 binds and activates TRPA1 extracellularly to evoke acute and chronic pruritus. Neuron (2018) 99(3):449–463.e6. doi: 10.1016/j.neuron.2018.06.039
56. Samad TA, Moore KA, Sapirstein A, Billet S, Allchorne A, Poole S, et al. Interleukin-1beta-mediated induction of cox-2 in the CNS contributes to inflammatory pain hypersensitivity. Nature (2001) 410(6827):471–5. doi: 10.1038/35068566
57. Mathivanan S, Devesa I, Changeux J-P, Ferrer-Montiel A. Bradykinin induces TRPV1 exocytotic recruitment in peptidergic nociceptors. Front Pharmacol (2016) 7:178. doi: 10.3389/fphar.2016.00178
58. Binshtok AM, Wang H, Zimmermann K, Amaya F, Vardeh D, Shi L, et al. Nociceptors are interleukin-1beta sensors. J Neurosci (2008) 28(52):14062–73. doi: 10.1523/JNEUROSCI.3795-08.2008
59. Kashem SW, Riedl MS, Yao C, Honda CN, Vulchanova L, Kaplan DH. Nociceptive sensory fibers drive interleukin-23 production from CD301b+ dermal dendritic cells and drive protective cutaneous immunity. Immunity (2015) 43(3):515–26. doi: 10.1016/j.immuni.2015.08.016
60. Wagner R, Myers RR. Endoneurial injection of TNF-alpha produces neuropathic pain behaviors. Neuroreport (1996) 7(18):2897–901. doi: 10.1097/00001756-199611250-00018
61. Hepburn L, Prajsnar TK, Klapholz C, Moreno P, Loynes CA, Ogryzko NV, et al. Innate immunity. a spaetzle-like role for nerve growth factor β in vertebrate immunity to staphylococcus aureus. Science (2014) 346(6209):641–6. doi: 10.1126/science.1258705
62. Qi J, Buzas K, Fan H, Cohen JI, Wang K, Mont E, et al. Painful pathways induced by TLR stimulation of dorsal root ganglion neurons. J Immunol (2011) 186(11):6417–26. doi: 10.4049/jimmunol.1001241
63. Miller JJ, Aoki K, Moehring F, Murphy CA, O’Hara CL, Tiemeyer M, et al. Neuropathic pain in a fabry disease rat model. JCI Insight (2018) 3(6):e99171, 99171. doi: 10.1172/jci.insight.99171
64. Xu Z-Z, Kim YH, Bang S, Zhang Y, Berta T, Wang F, et al. Inhibition of mechanical allodynia in neuropathic pain by TLR5-mediated a-fiber blockade. Nat Med (2015) 21(11):1326–31. doi: 10.1038/nm.3978
65. Maruyama K, Takayama Y, Sugisawa E, Yamanoi Y, Yokawa T, Kondo T, et al. The ATP transporter VNUT mediates induction of dectin-1-Triggered candida nociception. iScience (2018) 6:306–18. doi: 10.1016/j.isci.2018.08.007
66. Diogenes A, Ferraz CCR, Akopian AN, Henry MA, Hargreaves KM. LPS sensitizes TRPV1 via activation of TLR4 in trigeminal sensory neurons. J Dent Res (2011) 90(6):759–64. doi: 10.1177/0022034511400225
67. Bautista DM, Jordt S-E, Nikai T, Tsuruda PR, Read AJ, Poblete J, et al. TRPA1 mediates the inflammatory actions of environmental irritants and proalgesic agents. Cell (2006) 124(6):1269–82. doi: 10.1016/j.cell.2006.02.023
68. Lim JY, Choi S-I, Choi G, Hwang SW. Atypical sensors for direct and rapid neuronal detection of bacterial pathogens. Mol Brain (2016) 9:26. doi: 10.1186/s13041-016-0202-x
69. Caterina MJ, Leffler A, Malmberg AB, Martin WJ, Trafton J, Petersen-Zeitz KR, et al. Impaired nociception and pain sensation in mice lacking the capsaicin receptor. Science (2000) 288(5464):306–13. doi: 10.1126/science.288.5464.306
70. Davis JB, Gray J, Gunthorpe MJ, Hatcher JP, Davey PT, Overend P, et al. Vanilloid receptor-1 is essential for inflammatory thermal hyperalgesia. Nature (2000) 405(6783):183–7. doi: 10.1038/35012076
71. Szabo-Pardi TA, Barron LR, Lenert ME, Burton MD. Sensory neuron TLR4 mediates the development of nerve-injury induced mechanical hypersensitivity in female mice. Brain Behav Immun (2021) 97:42–60. doi: 10.1016/j.bbi.2021.06.011
72. Amaya F, Wang H, Costigan M, Allchorne AJ, Hatcher JP, Egerton J, et al. The voltage-gated sodium channel Na(v)1.9 is an effector of peripheral inflammatory pain hypersensitivity. J Neurosci (2006) 26(50):12852–60. doi: 10.1523/JNEUROSCI.4015-06.2006
73. Stirling LC, Forlani G, Baker MD, Wood JN, Matthews EA, Dickenson AH, et al. Nociceptor-specific gene deletion using heterozygous NaV1.8-cre recombinase mice. Pain (2005) 113(1–2):27–36. doi: 10.1016/j.pain.2004.08.015
74. Nassar MA, Stirling LC, Forlani G, Baker MD, Matthews EA, Dickenson AH, et al. Nociceptor-specific gene deletion reveals a major role for Nav1.7 (PN1) in acute and inflammatory pain. Proc Natl Acad Sci U.S.A. (2004) 101(34):12706–11. doi: 10.1073/pnas.0404915101
75. Kerr BJ, Souslova V, McMahon SB, Wood JN. A role for the TTX-resistant sodium channel nav 1.8 in NGF-induced hyperalgesia, but not neuropathic pain. Neuroreport (2001) 12(14):3077–80. doi: 10.1097/00001756-200110080-00019
76. Romagnani A, Vettore V, Rezzonico-Jost T, Hampe S, Rottoli E, Nadolni W, et al. TRPM7 kinase activity is essential for T cell colonization and alloreactivity in the gut. Nat Commun (2017) 8:1917. doi: 10.1038/s41467-017-01960-z
77. Beesetty P, Wieczerzak KB, Gibson JN, Kaitsuka T, Luu CT, Matsushita M, et al. Inactivation of TRPM7 kinase in mice results in enlarged spleens, reduced T-cell proliferation and diminished store-operated calcium entry. Sci Rep (2018) 8(1):1–22. doi: 10.1038/s41598-018-21004-w
78. Khalil M, Babes A, Lakra R, Försch S, Reeh PW, Wirtz S, et al. Transient receptor potential melastatin 8 ion channel in macrophages modulates colitis through a balance-shift in TNF-alpha and interleukin-10 production. Mucosal Immunol (2016) 9(6):1500–13. doi: 10.1038/mi.2016.16
79. Schappe MS, Szteyn K, Stremska ME, Mendu SK, Downs TK, Seegren PV, et al. Chanzyme TRPM7 mediates the Ca2+ influx essential for lipopolysaccharide-induced toll-like receptor 4 endocytosis and macrophage activation. Immunity (2018) 48(1):59–74.e5. doi: 10.1016/j.immuni.2017.11.026
80. Santoni G, Cardinali C, Morelli MB, Santoni M, Nabissi M, Amantini C. Danger- and pathogen-associated molecular patterns recognition by pattern-recognition receptors and ion channels of the transient receptor potential family triggers the inflammasome activation in immune cells and sensory neurons. J Neuroinflamm (2015) 12:21. doi: 10.1186/s12974-015-0239-2
81. Morrison RS, Kinoshita Y, Johnson MD, Uo T, Ho JT, McBee JK, et al. Proteomic analysis in the neurosciences. Mol Cell Proteomics (2002) 1(8):553–60. doi: 10.1074/mcp.R200004-MCP200
82. Pei Z, Pan Y, Cummins TR. Posttranslational modification of sodium channels. Handb Exp Pharmacol (2018) 246:101–24. doi: 10.1007/164_2017_69
83. Karve TM, Cheema AK. Small changes huge impact: the role of protein posttranslational modifications in cellular homeostasis and disease. J Amino Acids (2011) 2011:207691. doi: 10.4061/2011/207691
84. Dj L, Mr L. Protein kinase c and toll-like receptor signaling [Internet]. Enzyme Res (2011) 2011. doi: 10.4061/2011/537821
85. Tang J, Lin G, Langdon WY, Tao L, Zhang J. Regulation of c-type lectin receptor-mediated antifungal immunity. Front Immunol (2018) 9:123. doi: 10.3389/fimmu.2018.00123
86. Lim PS, Sutton CR, Rao S. Protein kinase c in the immune system: from signalling to chromatin regulation. Immunology (2015) 146(4):508–22. doi: 10.1111/imm.12510
87. Neagu M, Constantin C. Signal transduction in immune cells and protein kinases. Adv Exp Med Biol (2021) 1275:133–49. doi: 10.1007/978-3-030-49844-3_5
88. Jiang H, Galtes D, Wang J. Rockman HA. G protein-coupled receptor signaling: transducers and effectors. Am J Physiology-Cell Physiol (2022) 323(3):C731–48. doi: 10.1152/ajpcell.00210.2022
89. Kollarik M, Undem BJ. Activation of bronchopulmonary vagal afferent nerves with bradykinin, acid and vanilloid receptor agonists in wild-type and TRPV1-/- mice. J Physiol (Lond) (2004) 555(Pt 1):115–23. doi: 10.1113/jphysiol.2003.054890
90. Patil MJ, Meeker S, Bautista D, Dong X, Undem BJ. Sphingosine-1-phosphate activates mouse vagal airway afferent c-fibres via S1PR3 receptors. J Physiol (2019) 597(7):2007–19. doi: 10.1113/JP277521
91. Liu Q, Tang Z, Surdenikova L, Kim S, Patel KN, Kim A, et al. Sensory neuron-specific GPCR mrgprs are itch receptors mediating chloroquine-induced pruritus. Cell (2009) 139(7):1353–65. doi: 10.1016/j.cell.2009.11.034
92. Costello RW, Maloney M, Atiyeh M, Gleich G, Walsh MT. Mechanism of sphingosine 1-phosphate- and lysophosphatidic acid-induced up-regulation of adhesion molecules and eosinophil chemoattractant in nerve cells. Int J Mol Sci (2011) 12(5):3237–49. doi: 10.3390/ijms12053237
93. Juárez-Contreras R, Rosenbaum T, Morales-Lázaro SL. Lysophosphatidic acid and ion channels as molecular mediators of pain. Front Mol Neurosci (2018) 11:462. doi: 10.3389/fnmol.2018.00462
94. Numazaki M, Tominaga T, Toyooka H, Tominaga M. Direct phosphorylation of capsaicin receptor VR1 by protein kinase cϵ and identification of two target serine residues. J Biol Chem (2002) 277(16):13375–8. doi: 10.1074/jbc.C200104200
95. Jung J, Shin JS, Lee S-Y, Hwang SW, Koo J, Cho H, et al. Phosphorylation of vanilloid receptor 1 by Ca2+/calmodulin-dependent kinase II regulates its vanilloid binding. J Biol Chem (2004) 279(8):7048–54. doi: 10.1074/jbc.M311448200
96. Trebak M, Hempel N, Wedel BJ, Smyth JT, Bird GSJ, Putney JW. Negative regulation of TRPC3 channels by protein kinase c-mediated phosphorylation of serine 712. Mol Pharmacol (2005) 67(2):558–63. doi: 10.1124/mol.104.007252
97. Kwan H-Y, Huang Y, Yao X. Regulation of canonical transient receptor potential isoform 3 (TRPC3) channel by protein kinase G. Proc Natl Acad Sci U.S.A. (2004) 101(8):2625–30. doi: 10.1073/pnas.0304471101
98. Vazquez G, Wedel BJ, Kawasaki BT, Bird GSJ, Putney JW. Obligatory role of src kinase in the signaling mechanism for TRPC3 cation channels. J Biol Chem (2004) 279(39):40521–8. doi: 10.1074/jbc.M405280200
99. Scheuer T. Regulation of sodium channel activity by phosphorylation. Semin Cell Dev Biol (2011) 22(2):160–5. doi: 10.1016/j.semcdb.2010.10.002
100. Kerth CM, Hautvast P, Körner J, Lampert A, Meents JE. Phosphorylation of a chronic pain mutation in the voltage-gated sodium channel Nav1.7 increases voltage sensitivity. J Biol Chem (2020) 296:100227. doi: 10.1074/jbc.RA120.014288
101. Labed SA, Wani KA, Jagadeesan S, Hakkim A, Najibi M, Irazoqui JE. Intestinal epithelial wnt signaling mediates acetylcholine-triggered host defense against infection. Immunity (2018) 48(5):963–978.e3. doi: 10.1016/j.immuni.2018.04.017
102. Crawford LK, Caterina MJ. Functional anatomy of the sensory nervous system: updates from the neuroscience bench. Toxicol Pathol (2020) 48(1):174–89. doi: 10.1177/0192623319869011
103. Meerschaert KA, Adelman PC, Friedman RL, Albers KM, Koerber HR, Davis BM. Unique molecular characteristics of visceral afferents arising from different levels of the neuraxis: location of afferent somata predicts function and stimulus detection modalities. J Neurosci (2020) 40(38):7216–28. doi: 10.1523/JNEUROSCI.1426-20.2020
104. Su Y, Barr J, Jaquish A, Xu J, Verheyden JM, Sun X. Identification of lung innervating sensory neurons and their target specificity. Am J Physiol Lung Cell Mol Physiol (2022) 322(1):L50–63. doi: 10.1152/ajplung.00376.2021
105. Webster JI, Tonelli L, Sternberg EM. Neuroendocrine regulation of immunity. Annu Rev Immunol (2002) 20:125–63. doi: 10.1146/annurev.immunol.20.082401.104914
106. Mueller B, Figueroa A, Robinson-Papp J. Structural and functional connections between the autonomic nervous system, hypothalamic–pituitary–adrenal axis, and the immune system: a context and time dependent stress response network. Neurol Sci (2022) 43(2):951–60. doi: 10.1007/s10072-021-05810-1
107. Wohleb ES, McKim DB, Sheridan JF, Godbout JP. Monocyte trafficking to the brain with stress and inflammation: a novel axis of immune-to-brain communication that influences mood and behavior. Front Neurosci (2015) 8:447. doi: 10.3389/fnins.2014.00447
108. Souza ACP, Souza CM, Amaral CL, Lemes SF, Santucci LF, Milanski M, et al. Short-term high-fat diet consumption reduces hypothalamic expression of the nicotinic acetylcholine receptor α7 subunit (α7nAChR) and affects the anti-inflammatory response in a mouse model of sepsis. Front Immunol (2019) 10:565. doi: 10.3389/fimmu.2019.00565
109. Zhang X, Lei B, Yuan Y, Zhang L, Hu L, Jin S, et al. Brain control of humoral immune responses amenable to behavioural modulation. Nature (2020) 581(7807):204–8. doi: 10.1038/s41586-020-2235-7
110. Jendzjowsky NG, Roy A, Wilson RJA. Asthmatic allergen inhalation sensitises carotid bodies to lysophosphatidic acid. J Neuroinflamm (2021) 18:191. doi: 10.1186/s12974-021-02241-9
Keywords: pathogen recognition receptors (PRR), pathogen associated molecular patterns (PAMPs), transient receptor potential channel, sensory neuron, dorsal root ganglion (DRG), vagus, nodose ganglion (NG), carotid chemoreceptors
Citation: Millet A and Jendzjowsky N (2023) Pathogen recognition by sensory neurons: hypotheses on the specificity of sensory neuron signaling. Front. Immunol. 14:1184000. doi: 10.3389/fimmu.2023.1184000
Received: 10 March 2023; Accepted: 19 April 2023;
Published: 03 May 2023.
Edited by:
Guillermo Hernán Giambartolomei, National Scientific and Technical Research Council (CONICET), ArgentinaReviewed by:
Irene Salinas, University of New Mexico, United StatesOscar Bottasso, National University of Rosario, Argentina
Copyright © 2023 Millet and Jendzjowsky. This is an open-access article distributed under the terms of the Creative Commons Attribution License (CC BY). The use, distribution or reproduction in other forums is permitted, provided the original author(s) and the copyright owner(s) are credited and that the original publication in this journal is cited, in accordance with accepted academic practice. No use, distribution or reproduction is permitted which does not comply with these terms.
*Correspondence: Nicholas Jendzjowsky, bmljaG9sYXMuamVuZHpqb3dza3lAbHVuZHF1aXN0Lm9yZw==