- 1Institute of Drug Metabolism and Pharmaceutical Analysis, College of Pharmaceutical Sciences, Zhejiang University, Hangzhou, China
- 2Key Laboratory of Clinical Cancer Pharmacology and Toxicology Research of Zhejiang, Department of Clinical Pharmacy, Affiliated Hangzhou First People’s Hospital, Cancer Center, Zhejiang University School of Medicine, Hangzhou, China
- 3Center for Clinical Pharmacy, Cancer Center, Department of Pharmacy, Zhejiang Provincial People's Hospital (Affiliated People's Hospital), Hangzhou Medical College, Hangzhou, Zhejiang, China
- 4The Fourth Affiliated Hospital, School of Medicine, Zhejiang University, Jinhua, China
- 5Department of Neurosurgery, Sir Run Run Shaw Hospital, Zhejiang University School of Medicine, Hangzhou, Zhejiang, China
- 6Department of Pharmacy, Shaoxing People’s Hospital (Shaoxing Hospital, Zhejiang University School of Medicine), Shaoxing, China
- 7Westlake Laboratory of Life Sciences and Biomedicine of Zhejiang Province, Hangzhou, China
- 8Department of Pharmacy, Second Affiliated Hospital, School of Medicine, Zhejiang University, Hangzhou, China
The tumor microenvironment (TME) is a crucial driving factor for tumor progression and it can hinder the body’s immune response by altering the metabolic activity of immune cells. Both tumor and immune cells maintain their proliferative characteristics and physiological functions through transporter-mediated regulation of nutrient acquisition and metabolite efflux. Transporters also play an important role in modulating immune responses in the TME. In this review, we outline the metabolic characteristics of the TME and systematically elaborate on the effects of abundant metabolites on immune cell function and transporter expression. We also discuss the mechanism of tumor immune escape due to transporter dysfunction. Finally, we introduce some transporter-targeted antitumor therapeutic strategies, with the aim of providing new insights into the development of antitumor drugs and rational drug usage for clinical cancer therapy.
1 Introduction
The tumor microenvironment (TME) is a complex ecosystem that supports tumor cell survival and development. Tumor cells constitute the majority of the TME, along with peripheral blood vessels, stromal cells, extracellular matrix, and the secretion products (such as cellular metabolites and cytokines) of various cells (1). As a fundamental component of stromal cells, immune cells are indispensable for inhibiting the occurrence and development of tumors.
Tumor immune escape refers to the process whereby tumor cells escape the immune system’s “surveillance” instead of being eliminated, enabling them to proliferate and divide rapidly. The mechanisms of tumor immune escape can be divided into two main categories. One is that the TME suppresses antitumor immune responses by altering the function of immune cells, and the other is that tumor cells avoid being attacked by the immune system by decreasing the expression levels of self-antigens, creating defects in the antigen presentation machinery, or reducing their immunogenicity (2).
Transporters are membrane-binding proteins that mediate the passage of substrates through biological membranes. Transporters play integral roles in the cellular uptake of nutrients and the efflux of metabolic waste. Therefore, changes in the expression levels of transporters regulate the growth and function of cells. The TME has been found to affect the expression of transporters in immune cells, which can impair the ability of immune cells to kill tumor cells and control tumor progression.
This review provides an overview of the reciprocal regulatory mechanisms among metabolites in the TME, transporters in immune cells, and immune cell functions. In addition, we discuss potential cancer treatment regimens that target transporters to modulate immune responses, thus laying the groundwork for more efficient and safe antitumor therapies.
2 The tumor microenvironment and immune cells
2.1 Metabolic characteristics of the tumor microenvironment
The oxidative decomposition of glucose provides the primary energy source for cells. Normal cells acquire energy by thoroughly oxidizing glucose to CO2 and water under aerobic conditions, whereas tumor cells prefer to convert glucose into lactate via glycolysis, even under oxygen-sufficient conditions. This is referred to as the Warburg effect (3). Compared with oxidative phosphorylation (OXPHOS), aerobic glycolysis results in inefficient, but rapid, ATP production, accompanied by the production of intermediates and metabolites that act as major raw materials for cellular biosynthesis (4, 5). Collectively, aerobic glycolysis is conducive to the rapid growth and proliferation of tumor cells. Notably, the Warburg effect is not an exclusive feature of tumor cell metabolism, because cells in different tumor tissue regions preferentially utilize distinct metabolic pathways depending on the TME conditions and nutrient availability (6). Previous research has shown that oxygenated tumor cells produce large amounts of ATP through the oxidative phosphorylation of glucose, whereas hypoxic tumor cells mainly utilize glycolysis to supply energy and produce lactate for oxygenated tumor cells to fuel oxidative metabolism (7). This coupled metabolic pattern is of great significance for tumor cell growth and proliferation.
Glucose deficiency is a striking feature of the TME. The rapid proliferation of tumor cells enhances glucose uptake through the overexpression of the glucose transporters, GLUT1 and GLUT3, to relieve glucose dependence, resulting in glucose deficiency in the TME. It has been suggested that upregulated expression levels of glucose transporters in tumor cells is closely associated with poor cancer prognosis (8). Increased oxygen consumption resulting from tumor cell hyperproliferation creates a hypoxic microenvironment that contributes to tumor invasion and metastasis (9). In addition, both aerobic glycolysis and glutamine catabolism in tumor cells produce large amounts of lactate, rendering the TME acidic (10). Although most cancer-metabolism-related studies have considered lactate as a metabolic waste product in the Warburg effect, recent studies have suggested that lactate is a potential fuel (11).
Lipids are another abundant metabolite in the TME, and their accumulation is closely related to immune cell dysfunction and tumor cell survival (12). Amino acids are primarily involved in cellular component synthesis and energy metabolism (such as the TCA cycle), which, along with their metabolites, contribute to various effects on antitumor immune responses and tumor growth in the TME (13). Moreover, the high accumulation of nucleosides (especially ATP) in the TME contributes to tumor immune escape (14, 15).
In general, the characteristics of the TME, including hypoxia, acidification, lack of nutrients (such as glucose and amino acids), and massive accumulation of immunosuppressive metabolites (such as lactate, lipids, and nucleosides), effectively promote tumor progression and influence antitumor immune responses.
2.2 Functions of immune cells in the TME
Immune cells in the TME are divided into three categories based on their functions in tumor cells. The first group comprises immune cells with antitumor effects, mainly CD8+ T cells, CD4+ Th1 cells, natural killer (NK) cells, and dendritic cells (DCs). The second group consists of tumor-promoting immune cells, including CD4+CD25+ T cells (Tregs), myeloid-derived suppressor cells (MDSCs), and mast cells. Additionally, highly heterogeneous macrophages and neutrophils exert distinct immune functions via phenotypic changes in different local tissue microenvironments.
T cells are essential immune cells that can differentiate into CD8+ T cells with cytotoxic activity and CD4+ T cells with helper functions. CD8+ and CD4+ T cells have multiple cell subtypes that play multifaceted roles in tumorigenesis according to the different cytokines they produce (Table 1). The infiltration of CD8+ T cells and CD4+ Th1 cells at the tumor site is a hallmark of a favorable tumor prognosis (51), due to their production of the cytokines IFNγ and TNFα, which can effectively induce the cell cycle of tumor cells to arrest in the G1/G0 phase (20, 52). As the most powerful antigen-presenting cells, DCs play key roles in innate and adaptive immunity, as they can effectively activate resting T cells to transform them into cytotoxic T lymphocytes to promote an antitumor immune response (53). NK cells are also an integral part of the antitumor immune response. They exert cytotoxic effects on their target cells by forming immune synapses and directionally secreting lysed particles, such as perforin and granzyme (54).
Tregs inhibit autoimmune reactions and maintain immune homeostasis in vivo (55). Tregs expressing the transcription factor Foxp3 are closely associated with a poor prognosis in multiple cancers (56). Similarly, Th2 and Th17 cells contribute to tumor occurrence and development (51). MDSCs are myeloid-derived cells, including polymorphonuclear MDSCs (PMN-MDSCs) and mononuclear MDSCs (M-MDSCs) (57). Tumor-infiltrating MDSCs exert tumor-promoting effects by inducing the generation of immunosuppressive Tregs and M2-type macrophages and inhibiting T cell activation (58). Mast cells, also known as basophils, are derived from myeloid cells and they secrete several bioactive molecules with tumor-promoting functions, such as angiogenic factors and matrix metalloproteinases (59). In addition, IL-33-activated mast cells promote tumor outgrowth by secreting macrophage chemokines to facilitate tumor-associated macrophages (TAMs) recruitment to tumor sites (60).
Macrophages and neutrophils perform distinct functions through environment-dependent phenotypic transformations. It is generally believed that macrophages mainly fall into two categories: type 1 (M1) and type 2 (M2) macrophages. M1 macrophages with proinflammatory and tumor-suppressive effects primarily rely on aerobic glycolysis for their energy supply, whereas M2 macrophages utilize fatty acid oxidation (FAO) to fuel mitochondrial OXPHOS, which is beneficial for repairing tissues, maintaining metabolic homeostasis, and enhancing tumor progression (61). Tumor cells recruit TAMs with an M2-like phenotype to the tumor microenvironment to disrupt immune surveillance (62). Similar to TAMs, tumor-associated neutrophils (TANs) can be divided into an N1 phenotype with antitumor activity and an N2 phenotype with protumor activity. The immunosuppressive cytokine TGF-β, which is overexpressed by tumor cells, can induce TAN polarization toward the N2 phenotype (63).
2.3 Effects of metabolites on immune cell function in the TME
2.3.1 Lipids
Lipids in the TME are pivotal for the suppression of antitumor immune responses. Lipids reduce CD8+ T cell cytotoxicity by inducing lipid peroxidation and ferroptosis (64, 65). Cholesterol accumulation in tumor-infiltrating CD8+ T cells is also closely related to the increased expression levels of immune checkpoint factors and cell exhaustion (66). Excessive intracellular lipids in DCs damage their antigen-presenting function and further inhibit T cell priming in the TME (67, 68). In NK cells, high lipid levels lead to a decrease in IFN-γ levels and the induction of metabolic reprogramming, thus significantly blunting their cytotoxic effects on tumor cells (69). The TME induces a phenotypic switch from M1 to M2 macrophages with lipid dependency. Lipid accumulation in TAMs is required for cell differentiation and tumor-promoting function (70). In addition, lipid-overloaded MDSCs have a stronger immunosuppressive effect (71).
2.3.2 Glucose
Glucose is the major nutrient that fuels cellular metabolic activity. Competition between tumor cells and T cells for glucose leads to the exhaustion of tumor-infiltrating T lymphocytes in the TME (72). At the same time, T cells derived from the TME have metabolic defects that manifest as decreased expression levels of glucose transporters and metabolic enzymes, which are detrimental to antitumor immune responses (73, 74). Because the increased metabolic demands for glucose and glutamine in activated T cells cannot be satisfied in the nutrient-deficient TME, protein glycosylation, cell differentiation, and growth of T cells are maintained (75, 76). Activated NK cells regulate metabolic reprogramming toward glycolysis via mTORC1, to adapt to the glucose-deficient TME (77). Similarly, Tregs inhibit glycolysis by highly expressing Foxp3 to achieve better metabolic adaptation to the low-glucose TME (78). Tumor-derived exosomes (TDEs) endow TAMs with immunosuppressive phenotypes by enhancing glucose uptake and metabolism (79).
2.3.3 Lactate
Excess lactate excretion improves tumor progression by enhancing acidification and regulating multiple signaling pathways in different cells (9). Lactate accumulation in the TME limits its efflux in a concentration-dependent manner and promotes the expression of lactate-uptake transporters, resulting in elevated levels of intracellular lactate, which interfere with cell metabolism and immune function (80, 81). Similarly, lactate is essential for the maintenance of Treg activity. Tumor-infiltrating Tregs exhibit upregulated expression levels of lactate-uptake- and metabolism-related genes to acquire adaptability and exert their immunosuppressive function (82). Additionally, in an environment with high lactate concentrations, macrophages are prone to M2 polarization, whereas the maturation of DCs is attenuated. These findings are consistent with immunosuppression generated by the TME (83).
2.3.4 Amino acids
Amino acids are closely associated with tumor development, and amino acid deficiency in the TME typically triggers immune cell dysfunction. Notably, the accumulation of certain amino acids, such as kynurenic acid (Kyn), are closely correlated with tumor development and progression. Kyn-mediated AHR signal conduction is a key mechanism that regulates the interaction between Tregs, TAMs, and CD8+ T cells to endow the TME with immunosuppressive properties (84–88). Branched-chain amino acids (BCCAs) are essential contributors to tumor growth. Upregulation of the BCCA transaminase 1, which is involved in BCCA catabolism, has been observed in various tumors (89). The accumulation of the BCCA metabolites, branch chain keto acids (BCKAs), excreted by glioblastoma cells via monocarboxylate transporter 1 (MCT1), suppresses antitumor immune responses by attenuating the phagocytic activity of macrophages (90).
2.3.5 Nucleosides
Hypoxic conditions induce ATP accumulation in the TME. Extracellular ATP can be rapidly degraded into adenosine, an immunosuppressive effector that participates in key signaling pathways that regulate tumor immune responses (15). In lymphocytes, signal transduction between adenosine and the A2A adenosine receptor inhibits cytotoxic T cell activation, while favoring Treg proliferation and immunosuppressive activity (91, 92). Regarding myeloid cells, adenosine enhances the IL-10-induced activation of M2 macrophages and PMN-MDSC expansion (93, 94). The ectonucleoside triphosphate diphosphohydrolase, ENTPD2, which is highly expressed in many types of tumors, hydrolyzes extracellular ATP into 5’-AMP to maintain the immunosuppressive function of M-MDSCs (95).
3 Effects of metabolic transporters on immune cells in the tumor microenvironment
3.1 Lipid transporters
According to the “Comprehensive Classification System for Lipids” published by the International Lipid Classification and Nomenclature Committee in 2005, lipids can be divided into the following eight categories: fatty acyls, glycerolipids, glycerophospholipids, sphingolipids, sterol lipids, prenol lipids, saccharolipids, and polyketides (96). Since lipids are cellular nutrients and signaling molecules, functional changes in lipid transporters are key modulators of the immune response. Several transmembrane and intracellular transporters mediate lipid transport (Figure 1). The CD36 transporter and fatty acid transport proteins (FATPs) are major lipid uptake transporters. Lipids are ingested intracellularly by the CD36 transporter in the form of low-density lipoprotein (LDL), very-low-density lipoprotein (VLDL), and fatty acids (61, 70). The FATP family, which is composed of six members (FATP1-6), mediates exogenous fatty acid uptake with different tissue expression patterns (97). Fatty acid binding proteins (FABPs) are a family of small proteins that act as intracellular fatty acid transporters (98), while ABCA1 and ABCG1 can synergistically mediate intracellular cholesterol efflux (99).
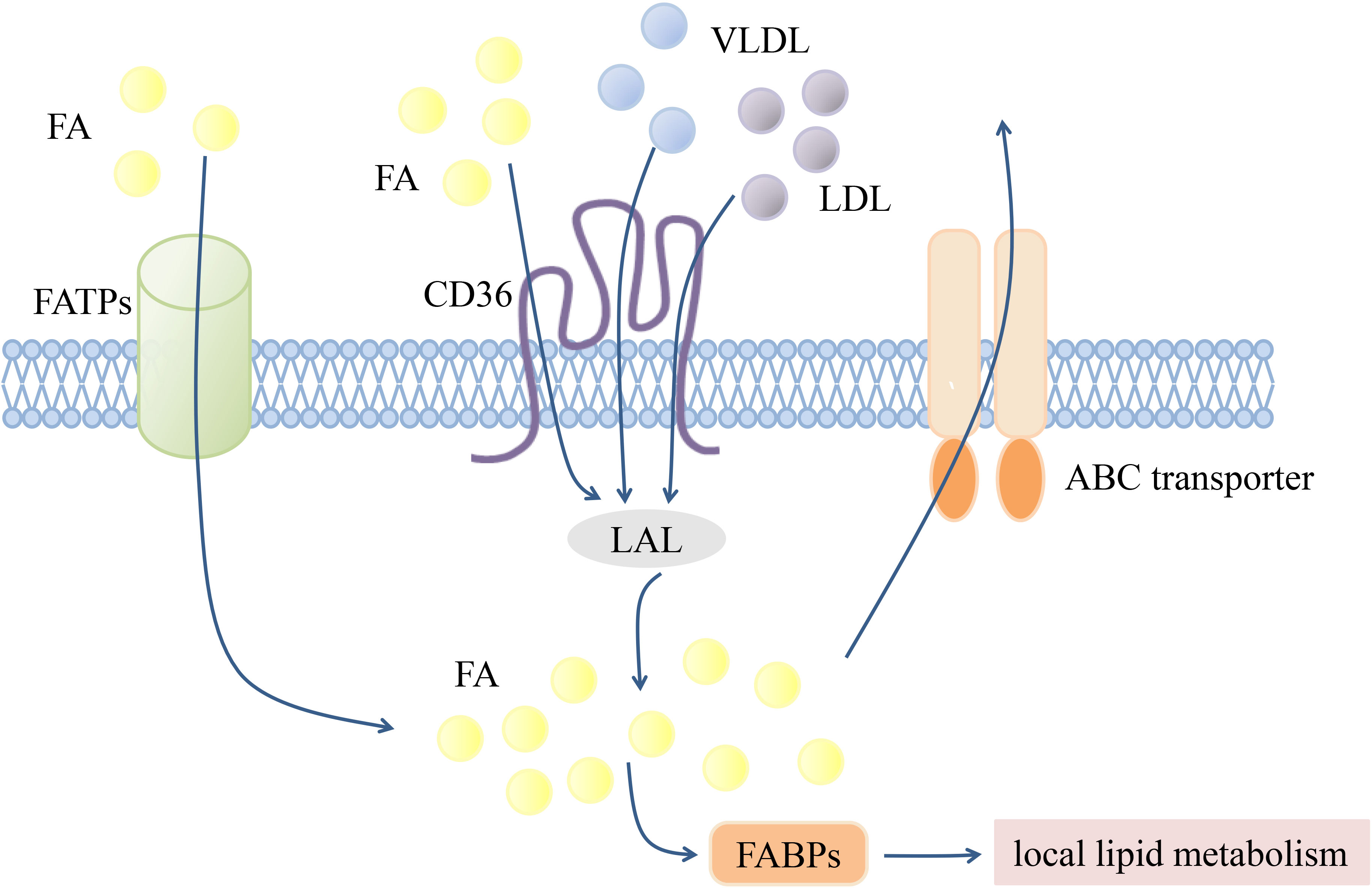
Figure 1 Transmembrane transportation of lipids. Transmembrane transport of lipids is mediated by various transport proteins. FATPs and CD36 are mainly responsible for mediating exogenous FA uptake. LDL and VLDL can also achieve intracellular transport through CD36, and then hydrolyzed by LAL into FA. Intracellular FA can be transported to different organelles or compartments for subsequent local lipid metabolism by FABPs, or excreted by ABC transporters. Fatty acid (FA), The fatty acid transporter family (FATPs), The fatty acid binding proteins (FABPs), Lysosomal acidic lipase (LAL), Low-density lipoprotein (LDL), Very low-density lipoprotein (VLDL).
3.1.1 CD36
The scavenger receptor, CD36, which has a lipid transport function, is widely expressed in various types of immune cells. The TME promotes tumor cell proliferation by interfering with CD36-mediated lipid metabolism in immune cells. Increased CD36 expression levels in CD8+ T cells allows the accumulation of lipid peroxides in cells and leads to the decreased secretion of cytotoxic factors, causing the dysfunction of tumor-infiltrating CD8+ T cells (64, 65). CD36 also functions as a regulator of tumor-infiltrating Tregs. The CD36-PPARβ signaling pathway mediates mitochondrial fitness and NAD production to support Tregs, which are more adaptable to low glucose and high lactate levels (78, 100). M2 macrophage activation is dependent on FAO. M2 macrophages increase LDL and VLDL uptake by upregulating CD36 expression levels, followed by lysosomal acidic lipase hydrolysis to provide fatty acids as substrates for FAO (61). CD36 directly mediates fatty acid transport. Increased CD36 expression levels in TAMs is conducive to an immunosuppressive function and cancer progression by enhancing fatty acid absorption and FAO (70). As a crucial regulator of TAM polarization, S100A4 upregulates CD36 expression levels by acting on PPAR-γ to induce FAO and then drives the M2-like polarization of TAMs (101). Lipid accumulation associated with the upregulation of CD36 and CD204 in DCs reduces antigen processing by DCs, limiting T cell priming (67, 68). MDSCs increase exogenous lipid uptake by upregulating the expression of multiple lipid transporters involved in metabolic reprogramming to support their immunosuppressive functions (71, 102). CD36 upregulation is closely related to Pim1-PPARγ signal transduction by PIM1 kinase, thus enhancing lipid metabolism and the immunosuppressive function of MDSCs (103). Similarly, lipid accumulation in NK cells caused by high CD36 expression levels, also attenuates the toxic effects on cancer cells (104).
3.1.2 The FATP/FABP family
FATPs encoded by the SLC27A family mediate the uptake of exogenous fatty acids and exhibit acetyl-CoA synthetase activity (105). Veglia et al. reported that the high expression levels of FATP2 (SLC27A2) in tumor-infiltrating PMN-MDSCs results in enhanced arachidonic acid uptake and prostaglandin E2 synthesis, thus endowing PMN-MDSCs with immunosuppressive activity (106). Subsequent studies by Adeshakin et al. showed that lipid accumulation in MDSCs induces immunosuppressive activity by enhancing mitochondrial function and activating reactive oxygen species (105).
FABPs encoded by FABP1-9 genes are other key proteins involved in intracellular lipid transportation, with a high affinity for long-chain fatty acids (98). Previous studies have found that M2 macrophages preferentially upregulate FABP4 expression and play a tumor-promoting role through FABP4-dependent IL-6/STAT3 signaling (107). In contrast, FABP5 in M1 macrophages enhances the recruitment of tumor-killer immune cells, such as effector T cells and NK cells, to the tumor site by inducing IFN-β secretion, thus promoting antitumor immune responses (108). FABP5 has been implicated as a regulator of macrophage phenotypes, contributing to M2 polarization, and FABP5 deficiency is hostile to the anti-inflammatory response of murine macrophages (109). High FABP5 expression levels in tumor-infiltrating Tregs enhance mitochondrial disturbances, type I IFN signaling, and immunosuppressive activity (110).
3.1.3 ATP-binding cassette transporters
ATP-binding cassette (ABC) transporters are members of the ABC protein superfamily. They couple ATP hydrolysis with substrate transport. As an integral component of the cell membrane structure, cholesterol is a key regulatory effector of the immune response. For example, T cell activation mediated by T cell receptor (TCR) signaling relies on intracellular cholesterol (111, 112). The ABC transporters ABCA1 and ABCG1 are the predominant cholesterol efflux transporters in immune cells. ABCG1 is a negative regulator of lymphocyte proliferation, and the loss of ABCG1 leads to enhanced TCR signaling in CD4+ T cells and promotes cell proliferation by promoting the accumulation of cholesterol (113). However, the effect of cholesterol on CD8+-T-cell-mediated antitumor immune responses remains controversial. The reduction in cholesterol uptake by CD8+ T cells induced by the TME inhibits TCR signaling and antitumor activity (114). Previous studies have also demonstrated that intracellular cholesterol negatively regulates the antitumor function of CD8+ T cells (66, 115). Accordingly, further studies are needed to determine the regulatory effects of cholesterol on T cell function.
ABCA1 and ABCG1 mediate cholesterol efflux from macrophages (99). Cholesterol metabolites and oxysterols inhibit T cell proliferation and immune effector capacity by activating LXR to induce ABCA1 and ABCG1 upregulation in macrophages (116). In ABCG1-deficient macrophages, cholesterol accumulation promotes proinflammatory gene expression mediated by NF-κB, thereby inducing macrophage polarization to the M1 phenotype (117). In ABCA1/G1-deficient DCs, accumulated cholesterol activates the DC inflammasome and promotes the secretion of inflammatory cytokines, leading to autoimmune diseases (118). ABCG1 deficiency is associated with the proliferation and maturation of invariant NK T (iNKT) cells (119). Additionally, ABCA7, another ABC family transporter in iNKT cells, regulates cellular functions by mediating the efflux of phospholipid complexes (120).
3.2 Glucose transporters
As a vital nutrient that supports energy production and biomass synthesis in cells, glucose is mainly transported into cells through GLUTs, a family of glucose transporters encoded by the SLC2A gene family (121). Activated CD4+ T cells support metabolic reprogramming toward aerobic glycolysis by upregulating GLUT1 expression levels to rapidly proliferate and differentiate into immune-promoting effector T (Teff) cells and immune-inhibiting Treg cells (122). Decreased GLUT1 expression levels in T cells from cancer patients may be closely related to the expression levels of the inhibitory receptors, PD-1 and TIM3 (73, 74, 123). A study of lung squamous cell carcinoma identified GLUT3 as the main glucose transporter in immune cells of the TME (124). Increased glucose uptake by GLUT3 in T cells inhibits GLUT3-mediated glucose uptake by cancer cells, indicating that immune cells with high GLUT3 expression levels promote effective antitumor immunity (124). Teff cells selectively depend on the glucose transporter GLUT1 for proliferation and inflammatory responses (122, 125). In addition, Tregs with increased GLUT1 expression levels and glycolytic reactions in the TME are functionally unaffected by GLUT1 deficiency, possibly because the energy supplementation pathway involves lipid metabolism (122, 126). Compared to normal neutrophils, neutrophils with pro-tumor functions exhibit higher GLUT1 expression levels and glucose metabolism (127). Similarly, TDE-induced macrophages achieve metabolic reprogramming by increasing the expression levels of GLUT1, HIF1-α, and lactose dehydrogenase A, which polarizes TAMs toward protumor phenotypes (79).
3.3 Lactate transporters
Monocarboxylate transporters (MCTs) belong to the SLC solute carrier family and comprise 14 members. MCT1–4 are proton-coupled monocarboxylate transporters capable of mediating the bidirectional transport of lactate, driven by a transmembrane concentration gradient (128). MCT1 and MCT4 are the most extensively studied monocarboxylate transporters involved in human cancers. MCT1 plays a major role in lactate uptake by glycolytic cells, whereas MCT4, which has a low affinity for lactate, is not easily saturated by high intracellular lactate levels and promotes lactate efflux in glycolysis-dependent cells, such as tumor cells (128, 129). High expression levels of MCT1 and MCT4 have been observed in many types of tumor cells (128). The Na-coupled transporters SMCT1 (SLC5A8) and SMCT2 (SLC5A12) also participate in lactate transport (129, 130).
Excess lactate accumulation in the TME inhibits the antitumor effects of immune cells. For activated T cells, increased lactate in the TME leads to an impaired lactate efflux function of MCT1, thus affecting glycolysis and the immune function of cytotoxic T cells (80). For NK cells, the downregulation of MCT4 expression levels in tumor cells can effectively restore their tumor-killing function by reversing the immunosuppressive TME (131). Conditions of high lactate concentration affect the antigenic phenotype and functional activity of monocyte-derived DCs, and induce MCT1 expression in plasma cell-like dendritic cells (pDCs), thereby facilitating tumor cells to evade immune surveillance (132, 133). Moreover, lactate inhibits the TLR stimulation response and IFN-α secretion through MCT1-mediated intracellular lactate transport and promotes pDCs to induce Tregs (133). Tregs with high MCT1 expression levels upregulate PD-1 expression and maintain their immunosuppressive activity in the glucose-deficient TME (82, 134). Additionally, lactate facilitates tumor immune escape by upregulating MCT1 expression levels in macrophages, thereby enabling M2 macrophage polarization (135, 136). Increased MCT4 expression levels in macrophages enhances lactate efflux, which is necessary for macrophage activation, glycolysis, and inflammatory responses (137).
To date, there has been no detailed investigation of the changes in SMCT expression levels in immune cells. It has been proposed that lactate accumulation upregulates SMCT2 expression levels in CD4+ T cells to enhance lactate uptake and the production of the proinflammatory cytokine IL-17, resulting in decreased glycolysis, cell activity, and migration (81, 138). DCs and macrophages also express SLC5A8 and SLC5A12, but they do not appear to exert a lactate transport function (130, 139).
3.4 Amino acid transporters
Amino acids are involved in a series of complex physiological processes, such as protein synthesis, metabolism, and signal transduction. There are three major classes of amino acid transporters in the SLC superfamily. Based on their properties, amino acid transporters can be divided into neutral, basic, and acidic types. Depending on whether the transport process requires coupling with Na+, they can be further divided into Na+- and non-Na+-dependent transporters (13). When comprehensively considering substrate specificity and transport mechanisms, they can be further divided into multiple systems, such as A, N, ASC, B, L, T, xc−, and y+ (140). The relationship between amino acids and their transporters is not a one-to-one relationship (Figure 2), making research targeting amino acid transporters more challenging (13).
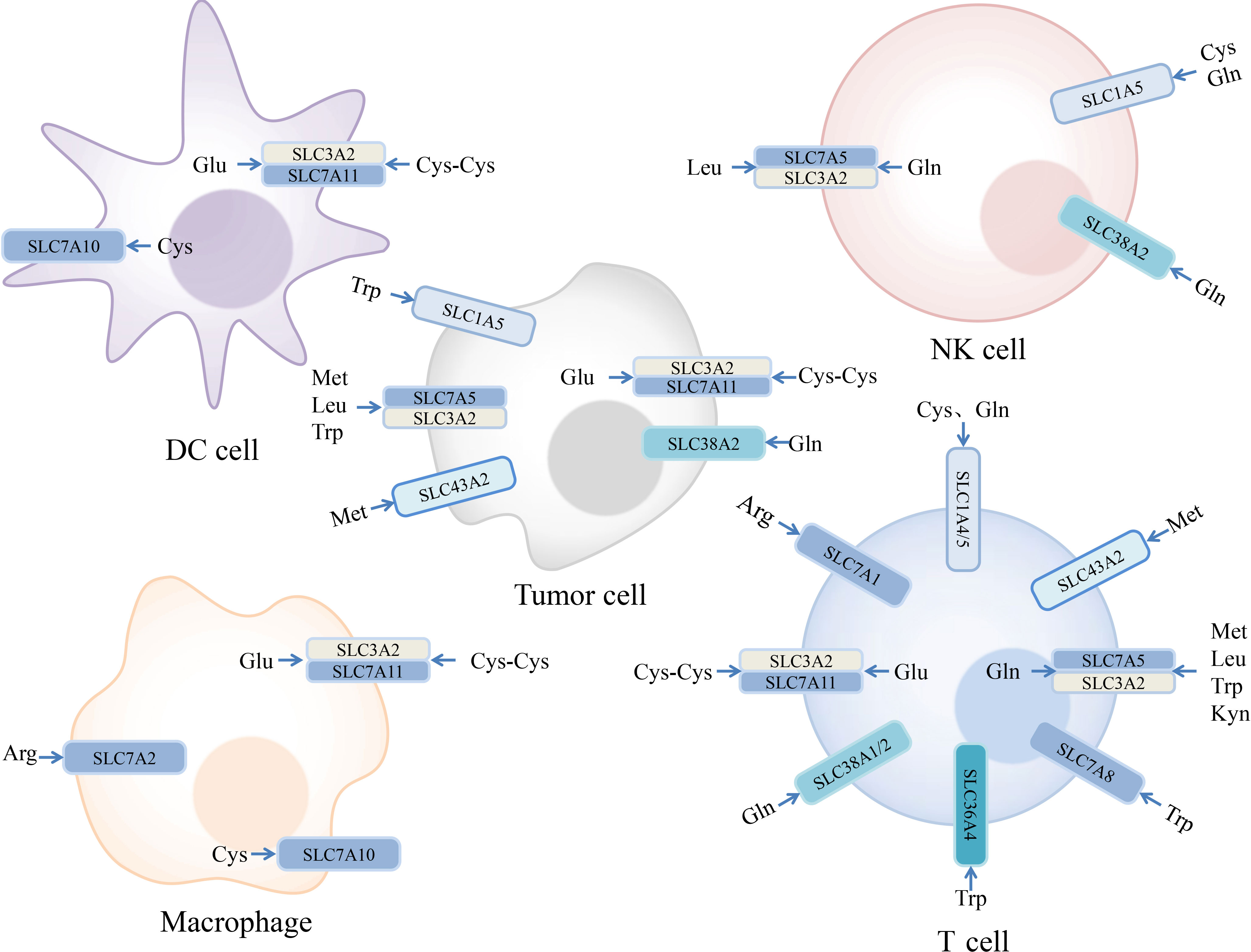
Figure 2 Expression of amino acid transporters in tumor and immune cells and their substrates. Some amino acids and their transporters are engaged in the regulation of the immune responses in the tumor microenvironment. Examples we mentioned are depicted in the figure. Arginine (Arg), Cysteine (Cys), Cystine (Cys-Cys), Glutamate (Glu), Glutamine (Gln), Kynurenine (Kyn), Leucine (Leu), Methionine (Met), Tryptophan (Trp).
3.4.1 Cysteine and cystine transporters
Typically, cystine is rapidly reduced to cysteine, which is necessary for the formation of the antioxidant glutathione (GSH) in cells (141). System xc−, which is composed of xCT (SLC7A11) and CD98 (SLC3A2) subunits, is a major plasma membrane antiporter responsible for the cellular uptake of cystine in exchange for intracellular glutamate (142). NaïveT cells cannot utilize cystine and methionine to synthesize cysteine because of a lack of cystathionase and complete xc− transporters. In this setting, T cell activation requires the neutral amino acid transporters ASCT1 (SLC1A4) and ASCT2 (SLC1A5) for the cellular uptake of cysteine excreted by macrophages and DCs through ASC transporters (SLC7A10) (143). Activated T cells upregulate the expression levels of members of the xc− system and neutral amino acid transporters to ingest cysteine for proliferation, thus deviating from a dependency on extracellular cysteine (144, 145). Activated monocytes and macrophages also ingest cystine mainly through the xc− system and upregulate xc− component expression levels in response to inflammatory stimulation to exercise immune functions (146, 147). The highly expressed xc− system in mature DCs plays a significant role in mediating the differentiation of monocytes into DCs (148). MDSCs deplete cysteine in the TME and block T cell activation by rendering DCs and macrophages unable to support T cells (143). CD8+ T cells induce lipid peroxidation and ferroptosis through the downregulation of SLC3A2 and SLC7A11 levels in tumor cells by secreting IFN-γ, thereby mediating effective antitumor responses (149). Elevated expression levels of SLC7A11 in tumor cells contribute to tumor invasion and metastasis, which are also correlated with poor cancer prognosis (150). Although SLC7A11 deletion in tumor cells results in impaired tumor growth, neither systemic nor T-cell-specific knockout of SLC7A11 affects T cell proliferation or the antitumor immune response, suggesting that further investigation of the interplay between SLC7A11 and T cell function should be conducted (13, 151).
3.4.2 Glutamine transporters
Glutamine is a nonessential amino acid that regulates vital cellular activities, such as energy metabolism and biosynthesis. As the deaminated form of glutamine, glutamate is further converted into ketoglutarate, which enters the TCA cycle, where it serves as a substrate for fatty acid, amino acid, and nucleotide synthesis (152, 153). The amino acid transporter system L, composed of LAT1 (SLC7A5) and CD98 (SLC3A2), exchanges glutamine for essential amino acids to regulate cell proliferation and function, indicating that intracellular glutamine is vital for amino acid transport (154–157). Glutamine is indispensable for T cell activation; therefore, most studies on glutamine transporters in immune cells have focused on T cells. TCR and CD28 activate T cells by stimulating the downstream MAPK family member, ERK, to induce the expression of the glutamine transporters SNAT1 (SLC38A1) and SNAT2 (SLC38A2). SNAT1 displays higher expression levels because of its faster induction (153). Similarly, ASCT2-mediated glutamine uptake plays a constructive role in T cell activation mediated by the mTORC1 signaling pathway. Therefore, ASCT2 deficiency suppresses the inflammatory response by inhibiting CD4+ T cell differentiation into Th1 and Th17 cells (155). LAT1 deletion also inhibits CD4+ T cell expansion and decreases the secretion of inflammatory factors (158). Amino acid transport mediated by ASCT2 and transporter system L is crucial for maintaining cellular metabolism and function in NK cells by regulating cMyc expression (154, 159).
3.4.3 Methionine transporters
Methionine is a sulfur-containing proteinogenic amino acid required for the synthesis of spermine and the major reducing agent GSH (160). In addition, methionine serves as the only source of the intracellular universal methyl donor S-adenosylmethionine (SAM), which plays an integral role in regulating gene expression and basic metabolic pathways in cells (161). Activated T cells increase methionine uptake by upregulating SLC7A5 expression levels and producing methyl donors for DNA and RNA methylation (162). In a hypoxic microenvironment, upregulated SLC7A5 expression contributes to the accumulation of methionine in tumor cells, which leads to methionine depletion in the TME (163). Therefore, decreased methylation in T cells may be attributed to an insufficient level of methionine, which affects cell proliferation and function (164). Since another methionine transporter encoded by SLC43A2 is also highly expressed in tumor cells, tumor-infiltrating effector T cells with low SLC43A2 expression levels do not compete with tumor cells for methionine. Consequently, low methionine and SAM levels and weakened H3K79me2 signaling in the promoter region downregulate STAT5 expression, which in turn affects T cell survival and function (165). Tumor cells escape immune surveillance by inducing T cell exhaustion through the cellular methionine metabolites SAM and 5-methylthioadenosine (166).
3.4.4 Leucine transporters
Transport of the essential branched-chain amino acid, leucine, and other large neutral amino acids is predominantly mediated by the amino acid transporter system L (167, 168). The mTORC1 signaling pathway is crucial for T cell activation and differentiation. Previous studies have shown that T cells upregulate SLC7A5 expression under sustained immune activation via TCR or IL-2 to improve leucine uptake and activate mTOR signaling (168). Notably, leucine uptake mediated by system L must be coupled with glutamine efflux, which is dependent on ASCT2-mediated glutamine uptake (169). This conclusion was confirmed in a follow-up study in which Nakaya et al. effectively activated the mTORC1 signaling pathway in SLC1A5-deficient T cells by exogenous supplementation with leucine (155). The inhibition of SLC7A5 expression affects the NK cell effector function, resulting in lower C-MYC protein expression levels and mTORC1 signaling in NK cells (167). Overexpression of LAT1 is also closely related to the proliferation of cancer cells, such as lymphoma, esophageal cancer, lung cancer, and prostate cancer cells (156), owing to the critical role of SLC7A5 in activating mTORC1 signaling and metastasis in tumors (156, 170).
3.4.5 Tryptophan and kynurenine transporters
Tryptophan metabolism through indoleamine 2,3-dioxygenase (IDO) and tryptophan 2,3-dioxygenase (TDO), which are involved in the kynurenine pathway, is postulated to be a leading cause of tumor immune escape (171). Tryptophan is transported via two pathways. One is the amino acid transporter system L, which is ubiquitously expressed in mammalian cells, and is the only conventional transport system expressed by resting human T cells. The other is a novel transport system upregulated by IDO in tumor cells and human monocyte-derived macrophages, with a high affinity for tryptophan (172, 173). Tumor cells upregulate the expression of multiple transporters that rely on the transcription factor ATF4 to enhance tryptophan and glutamine uptake under IDO-induced tryptophan deficiency (171). Moreover, overexpression of the tryptophan-transport- and metabolism-related genes SLC1A5, SLC7A5, SLC7A8, and TDO2 in tumor cells also provides potential strategies for cancer therapy (174, 175). SLC1A5 expression is also upregulated in a highly tumorigenic subpopulation of tumor cells known as tumor-repopulating cells (175). Correspondingly, enhanced tryptophan uptake and metabolism in tumor cells leads to kynurenine accumulation in the TME, thereby dampening antitumor immune responses. Extensive research has shown that kynurenine transport in activated T cells is mediated by transporters encoded by SLC7A5, SLC7A8, and SLC36A4 genes (175, 176). As LAT1 simultaneously mediates the transport of multiple amino acid substrates, high levels of kynurenine in the TME exhibit competitive inhibition with other substrates, thus providing a new explanation for the immunosuppressive mechanism mediated by kynurenine accumulation in the TME (176).
3.4.6 Arginine transporters
The conditionally essential amino acid arginine is the main substrate for the biosynthesis of proteins, creatine, nitric oxide, and polyamines, and is involved in activating the metabolic checkpoint, mTOR, for cell proliferation (177, 178). Arginine uptake is mainly mediated by transporter system y (CAT1-3), encoded by the SLC7A1-3 gene (179). Arginine metabolism is essential for sustaining tumor metabolism and survival (180, 181). CAT1 is the only transporter responsible for L-arginine uptake in chronic lymphocytic leukemia (CLL) cells. Previous studies have shown that downregulating CAT1 expression in mouse CLL cells has a significant tumor-inhibition effect (182). Therefore, CAT1 may serve as a novel therapeutic target for CLL treatment. Similarly, arginase produced by tumor cells and MDSCs inhibits T cell proliferation and leads to tumor immune escape (183–185). In addition to arginine, creatine and polyamines also have immunomodulatory properties. The upregulation of CAT1 in activated T cells results in increased L-arginine uptake, which contributes to T cell proliferation and tumor-suppressive functions (186, 187). Polyamine metabolism affects transcriptome and epigenome remodeling from Th17 cells to Tregs (188). The expression of CAT2 is induced in both M1 and M2 macrophages (189). Additionally, macrophages ingest creatine, a metabolite of arginine, in a process mediated by the transporter CRT (SLC6A8), to polarize them toward the M2 phenotype (190).
3.5 Nucleoside transporters
Equilibrative nucleoside transporters (ENT1–4) encoded by the SLC29 family and condensed nucleoside transporters (CNT1-3) encoded by the SLC28 family are the major nucleotide transporters in the human body. ENTs have been implicated in the bidirectional transport of various purines and pyrimidines to maintain intracellular and extracellular nucleoside homeostasis. CNTs mediate the unidirectional uptake of nucleosides in an Na+- and H+-coupled manner (191, 192). Nucleoside transporters play a central role in transporting nucleosides, nucleobases, and nucleoside analogs, with effects on cell metabolism and signal transduction (192–194). Several studies have suggested that decreased ENT1 and CNT1 expression levels in tumor cells may be associated with drug resistance (192, 193, 195, 196). In addition, the RFC1 transporter encoded by SLC19A1 mediates the uptake of the immune transmitter cGAMP into monocyte-derived U937 cells to elicit antitumor immune responses via immune cell recruitment. However, whether other immune cells utilize this mechanism to achieve cGAMP uptake requires further investigation (197).
Hypoxia is a common feature of the TME, with inhibitory effects on cellular ENT1 expression (198–200). For example, nucleotide metabolic enzymes CD73 and CD39, expressed by immune cells, are upregulated under the regulation of HIF1-α. This leads to adenosine accumulation in the TME, which negatively affects the immune activity of Teff cells (198, 199, 201). In mice with inflammatory diseases, the ENT1-targeted drug decitabine selectively depletes Teff cells with high ENT1 expression levels and promotes the proliferation of immunosuppressive Treg cells, which may be one of the mechanisms responsible for immune tolerance (202). Macrophages simultaneously express ENTs and CNTs, and selectively express specific nucleoside transporters according to the requirements related to their growth, development, and metabolism, with regulators including lipopolysaccharide, TNF-α, IFN-γ, and M-CSF (203, 204).
4 Tumor therapeutic regimens targeting transporters
Metabolic reprogramming of tumor and immune cells occurs owing to the rapid proliferation of tumor cells, ultimately creating an immunosuppressive TME with complex interactions between metabolite abundance and transporter expression. Therefore, targeting transporters expressed in the cells of the TME to regulate cellular metabolism may provide powerful support for cancer treatment. Various types of transporter-targeted antitumor drugs have been developed, including chemical drugs and antibodies (Figure 3). In addition, the combination of transporter-targeted drugs with existing chemotherapeutic drugs or immune checkpoint inhibitors may provide new strategies for clinical cancer treatment.
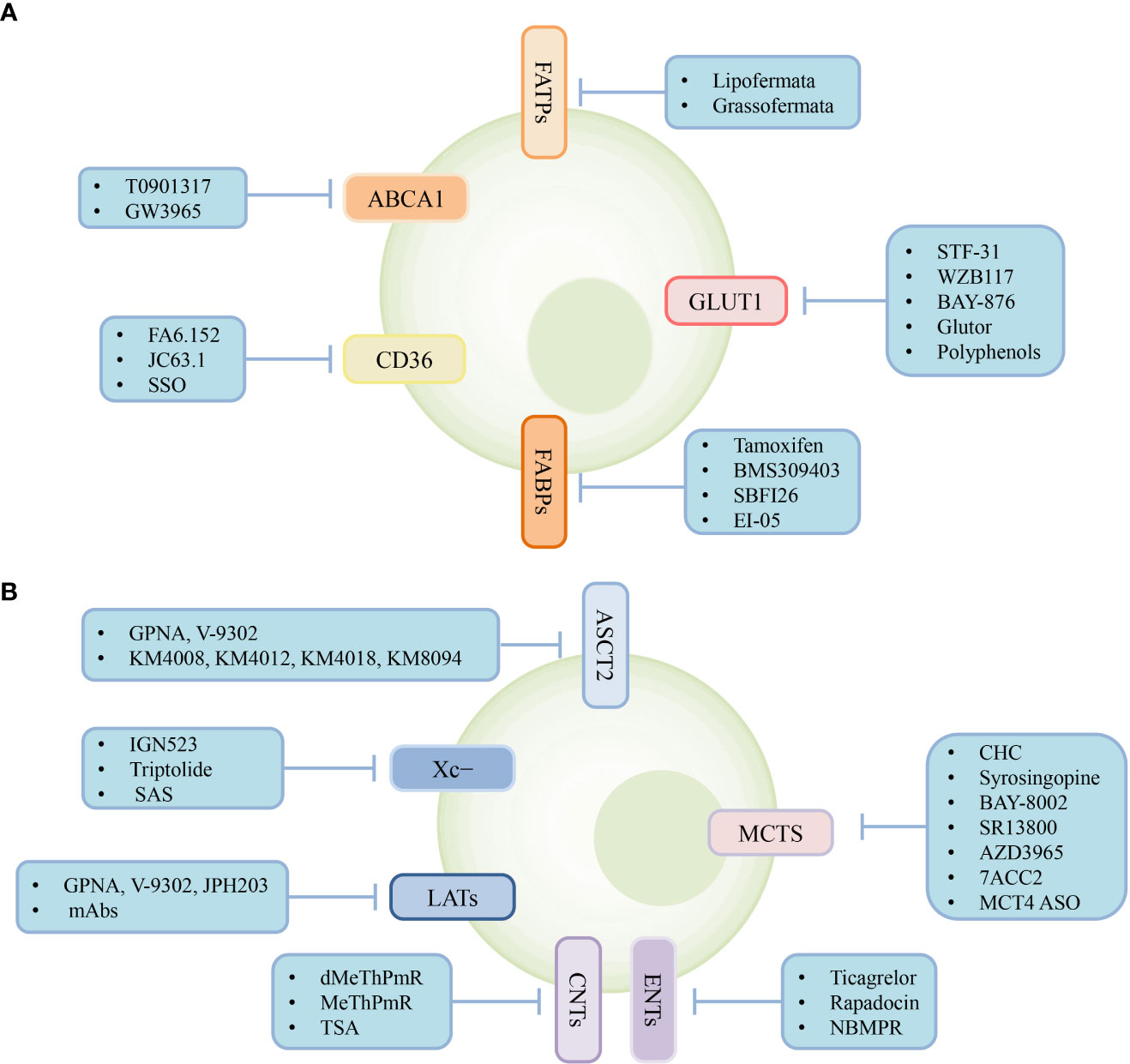
Figure 3 Transporter-targeted antitumor drugs. Current approaches including chemical drugs and antibodies for transporter-targeted antitumor drugs. (A) Lipid and glucose transporters-targeted drugs. (B) Lactate, amio acid and nucleoside transporters-targeted drugs.
4.1 Targeting lipid transporters
The irreversible CD36 inhibitor sulfo-n-succiniminooleate (SSO) inhibits the uptake of oxidized low-density lipoprotein by macrophages (205). Experimental studies in mice have shown that the CD36-neutralizing antibodies FA6.152 and JC63.1 hold promise for inhibiting cancer cell metastasis in various cancer types (206).
Lipofermata and grassofermata specifically inhibit FATP2-mediated fatty acid transport and cytotoxic reactions caused by excessive fatty acid accumulation (106, 207). Moreover, FATP2 inhibitors, in combination with checkpoint inhibitors, alleviate the suppressive activity of MDSCs against T cells and significantly enhance antitumor immune responses (105, 106). Lipofermata may also be exploited to inhibit FATP1 because of the similarity between FATP1 and FATP2, which contributes to the effective inhibition of FATP1-mediated fatty acid uptake, thereby reducing melanoma cell proliferation (208). However, lipofermata shows no obvious therapeutic effects in tumor-bearing mice owing to its short half-life (208). Pharmacological inhibitors of FABP4, such as tamoxifen and BMS309403, effectively inhibit tumor progression (209–211). The chemical inhibitor SBFI26 restricts tumor proliferation by targeting FABP5-PPARγ-VEGF signaling (212). High FABP5 expression levels confer enhanced antitumor activity to macrophages. The small-molecule compound EI-05, identified by Rao et al., significantly increase FABP5 expression levels in macrophages and inhibits breast tumor growth in mice (213).
The ABCA1 transporter is also a potential target for tumor treatment. ABCA1 suppresses tumor growth by mediating cholesterol efflux in tumor cells to promote cell death (214, 215). Missense mutations in ABCA1 contribute to tumor progression in patients with chronic myelomonocytic leukemia (216). The tumor suppressor P53 induces ABCA1 expression, and loss of P53 or ABCA1 promotes liver tumor cell proliferation in mice (217). As the target gene of the nuclear receptor LXR, ABCA1 expression can be increased by LXR activation. Therefore, the LXR agonists T0901317 and GW3965 play crucial roles in the anti-proliferative effects on tumor cells (218). LXR agonists combined with first-line therapeutic drugs for melanoma, such as dacarbazine, vemurafenib, and anti-CTLA-4 antibodies, improve the clinical efficacy of monotherapies (219). Similarly, the combination of GW3965 and gemcitabine exhibits a more significant antitumor effect than two single-agent treatments on pancreatic cancer cell lines (220).
4.2 Targeting glucose transporters
Treatments targeting glucose transporters mainly include drug therapies targeting GLUT1 and those providing RNA interference. Compound STF-31, identified by Chan et al., exhibits synthetic lethality in cancer cells with high GLUT1 expression levels and glycolysis dependence (221). WZB117, a small-molecule inhibitor, exerts anticancer effects by reducing GLUT1 expression levels, intracellular ATP levels, and glycolytic enzyme levels in tumor cells (222). BAY-876 is a highly selective inhibitor of GLUT1 with favorable pharmacokinetic properties both in vitro and in vivo and it exhibits potent antitumor effects against a variety of solid tumors (223–226). Polyphenols, such as resveratrol, hesperetin, catechin, and quercetin, also possess antitumor functions by reducing GLUT1 mRNA and protein expression levels in tumor cells (227). The growth of tumor cells treated with an anti-SLC2A1 short hairpin RNA (shRNA) is markedly suppressed (228). Furthermore, an shRNA targeting GLUT1 enhances cisplatin sensitivity in head and neck cancer cells (229). Collectively, RNA combined with chemotherapeutic drugs is expected to result in improved therapeutic effects against cancer. Notably, in addition to GLUT1, tumor cells upregulate GLUT3 expression levels under conditions of glucose deprivation. Therefore, drugs specifically targeting GLUT1 may be insufficient to inhibit tumor growth and may require high concentrations to elicit therapeutic effects. These issues can be overcome by a novel pan-GLUT inhibitor, Gluor, which targets the glucose transporters GLUT-1, GLUT-2, and GLUT-3, with antitumor effects at nanomolar concentrations. However, detailed investigations of the effect of Gluor in vivo are limited (230, 231).
4.3 Targeting lactate transporters
As the predominant lactate transporters in tumor cells, MCT1 and MCT4 prevent intracellular acidification and maintain normal tumor cell growth. Consequently, MCT inhibitors have great potential for clinical applications in antitumor treatments. α-Cyano-4-hydroxycinnamate (CHC) is a selective and reversible inhibitor of MCT1 that can significantly inhibit tumor growth and reverse hypoxic conditions in the TME (7). Choi et al. effectively inhibits tumor growth by reducing MCT4 expression levels in tumor-bearing nude mice treated with an MCT4-targeting antisense oligonucleotide (MCT4 ASO) (232). Various potent MCT1 inhibitors have been reported, including SR13800, AZD3965, BAY-8002, and 7ACC2 (233). AZD3965 is an orally bioavailable MCT1 inhibitor in phase I clinical trials that effectively increases T cell infiltration, reduces lactate efflux from tumor cells, and reverses the immunosuppressive microenvironment of solid tumors (234). Moreover, syrosingopine has dual inhibitory effects on MCT1 and MCT4. In combination with metformin, which inhibits oxidative phosphorylation, sylrosiglitazone induces significant cytotoxic reactions and reduces the effective concentration of metformin (235)
4.4 Targeting amino acid transporters
Overexpression of CD98, an integral subunit of system xc−, is often closely associated with poor prognosis in many cancers. The humanized monoclonal antibody IGN523, which targets CD98, has been reported to have significant antitumor activity, and a phase I clinical trial of this antibody in patients with acute myeloid leukemia is currently underway (236). xCT, another functional subunit of the xc− transport system, affects intracellular redox homeostasis by regulating GSH metabolism. The transcription factor Nrf2 controls the antioxidant pathway in eukaryotic cells by regulating the expression of SLC7A11 (237). Therefore, the Nrf2 inhibitor triptolide disrupts GSH synthesis in tumor cells and is synthetically lethal in malignant tumors with IDH1 mutations (237). Many studies of inhibitors targeting system xc− combined with other therapeutic approaches are also currently underway. Clinical trials of an FDA-approved xCT inhibitor, sulfasalazine (SAS), have been conducted in patients with recurrent glioblastoma. However, a recent study on melanoma indicated that xCT inhibition by SAS allows tumor cells to secrete PD-L1 via exosomes, inducing the M2 polarization of macrophages and leading to the development of resistance to anti-PD-1/PD-L1 therapy (238). Therefore, more detailed studies on the effects of SLC7A11 inhibitors as combination drugs in antitumor immunotherapy are required.
ASCT2-mediated glutamine uptake is inseparable from tumor cell growth and metabolism. GPNA was initially recognized as a commercially available competitive inhibitor of ASCT2, and has since been used in many cancer model studies. However, GPNA is not highly selective for ASCT2 and previous studies have shown that LAT1 and LAT2 are also inhibited by GPNA (239). V-9302 is considered an efficient ASCT2 competitive antagonist with significant inhibitory effects on tumor cell growth and proliferation, both in vivo and in vitro, while also inducing tumor cell death and oxidative stress (240). Nonetheless, follow-up studies have found that V-9302 has the same inhibitory function on SNAT2 and LAT1 (241). Therefore, specific ASCT2 inhibitors need to be further developed. Recently, monoclonal antibody (mAb) therapies targeting ASCT2 have been preliminarily studied. Suzuki et al. isolated three specific anti-ASCT2 mAbs, KM4008, KM4012, and KM4018, from CHO cells expressing ASCT2, and these effectively inhibited colorectal tumor growth in vitro (242). Another humanized anti-ASCT2 mAb, KM8094, exerts antitumor effects in gastric cancer patient-derived xenograft models, indicating its potential application in gastric cancer treatment (243).
L-type amino acid transporters are responsible for the uptake of the large neutral amino acids required for cell growth. By evaluating the antitumor effects of multiple anti-LAT1 mAbs, one study demonstrated that a novel anti-LAT1 mAb effectively inhibited tumor development and thus, it holds promise for cancer therapy (244). The small-molecule LAT1 inhibitor, JPH203, inhibits the growth of multiple solid tumors. Current phase I clinical trials of JPH203 suggest promising applications for the treatment of advanced biliary tract cancer (245).
Immune checkpoint blockade therapy can also be an effective cancer treatment by affecting transporter functions. For instance, immune checkpoint factors PD-1 and CTLA4 trigger immunosuppressive signaling pathways to inhibit the expression of the glucose transporter, GLUT1, and the glutamine transporters, SNAT1 and SNAT2, in T cells, thus limiting nutrient uptake and T cell activation, indicating that mAb drugs targeting immune checkpoints can restore T cell function (246).
4.5 Targeting nucleoside transporters
Currently, there is limited published information regarding nucleoside transporter inhibitors. As a selective ENT inhibitor, NBMPR has been widely used in physiological and pharmacological studies of ENT function (247). The FDA-approved antiplatelet drug, ticagrelor, can inhibit ENT1 activity in human erythrocytes and platelets (248, 249). Rapamycin, another potent ENT1 inhibitor, interacts with FKBP to exert its biological function. The efficacy of rapamycin therapy at affecting cellular adenosine uptake and subsequent adenosine signaling suggests a potential new therapeutic strategy for regulating cellular adenosine signaling (250). The fused-pyrimidine nucleoside analog, thienopyrimidine 2′-deoxynucleoside (dMeThPmR) and its ribonucleoside analog (MeThPmR) are inhibitors with high affinity for hCNT1 (251). In colorectal cancer, the histone deacetylase inhibitor trichostatin A (TSA) effectively reverses the downregulation of CNT2 expression mediated by histone deacetylation and enhances the sensitivity of colorectal cancer cells to the chemotherapeutic drug cladribine (196).
5 Summary and prospects
Taken together, changes in the expression levels of transporters in the TME are important in regulating tumor progression and immune responses. In the TME, peculiar metabolic pathways and metabolites are major barriers to antitumor immunotherapy, as they affect cellular metabolic activity by regulating the expression of transporters. Tumor proliferation and invasion, as well as immune cell functions, are inseparable from transporter-mediated substrate transport. Current studies on transporter-targeted antitumor drugs have made some progress; however, the evaluation of drug efficacy has mainly focused on tumor cells rather than on the regulation of transporter expression in immune cells. Therefore, more detailed investigations of targeted drugs for selective cytotoxicity, specific inhibitory activity, and sensitivity to metabolites in the TME are required.
Furthermore, changes in metabolite abundance and immune cell activity in the complex and dynamic TME should be considered when describing the effects of changes in transporter expression levels on tumor progression. Metabolism-related genes are currently used to assess immunotherapy efficacy and patient prognosis (252–254). Whether the expression levels of transporters on immune cells can also be used as prognostic indicators for tumor immunotherapy requires further study.
Transporter-targeted drugs combined with chemoradiotherapy and immunotherapy have been shown to have good clinical application potential. Future research should be directed toward the discovery of additional transporters as biomarkers or therapeutic targets, and existing clinical cancer treatments should be optimized by exploring different combination regimens. Rational strategies for tumor therapy may also focus on cellular metabolic features mediated by changes in transporter expression levels in immune cells, to reverse the immunosuppressive TME, thereby enabling more effective antitumor immune responses.
Author contributions
LY and LC conceived, designed, and supervised the project. LC and YW wrote the manuscript. LC, YW, QH, YL, XQ, ZT, HH, NL, SZ and LY provided critical discussion and revised the manuscript. All authors contributed to the article and approved the submitted version.
Funding
This work was supported by the Ten-thousand Talents Program of Zhejiang Province (2021R52013), the grants from National Natural Science Foundation of China (82003845, 82274003, 81973390), the Zhejiang Provincial Natural Science Foundation of China (LQ21H310003), and the Zhejiang Provincial Medical and Health Technology Project (2022485016).
Conflict of interest
The authors declare that the research was conducted in the absence of any commercial or financial relationships that could be construed as a potential conflict of interest.
Publisher’s note
All claims expressed in this article are solely those of the authors and do not necessarily represent those of their affiliated organizations, or those of the publisher, the editors and the reviewers. Any product that may be evaluated in this article, or claim that may be made by its manufacturer, is not guaranteed or endorsed by the publisher.
References
1. Hui L, Chen Y. Tumor microenvironment: sanctuary of the devil. Cancer Lett (2015) 368(1):7–13. doi: 10.1016/j.canlet.2015.07.039
2. Iorgulescu JB, Braun D, Oliveira G, Keskin DB, Wu CJ. Acquired mechanisms of immune escape in cancer following immunotherapy. Genome Med (2018) 10(1):87. doi: 10.1186/s13073-018-0598-2
3. Weinhouse S. On respiratory impairment in cancer cells. Science (1956) 124(3215):267–9. doi: 10.1126/science.124.3215.267
4. Martinez-Outschoorn UE, Peiris-Pagés M, Pestell RG, Sotgia F, Lisanti MP. Cancer metabolism: A therapeutic perspective. Nat Rev Clin Oncol (2017) 14(1):11–31. doi: 10.1038/nrclinonc.2016.60
5. Dey P, Kimmelman AC, DePinho RA. Metabolic codependencies in the tumor microenvironment. Cancer Discovery (2021) 11(5):1067–81. doi: 10.1158/2159-8290.Cd-20-1211
6. Villalba M, Rathore MG, Lopez-Royuela N, Krzywinska E, Garaude J, Allende-Vega N. From tumor cell metabolism to tumor immune escape. Int J Biochem Cell Biol (2013) 45(1):106–13. doi: 10.1016/j.biocel.2012.04.024
7. Sonveaux P, Végran F, Schroeder T, Wergin MC, Verrax J, Rabbani ZN, et al. Targeting lactate-fueled respiration selectively kills hypoxic tumor cells in mice. J Clin Invest (2008) 118(12):3930–42. doi: 10.1172/jci36843
8. Barron CC, Bilan PJ, Tsakiridis T, Tsiani E. Facilitative glucose transporters: implications for cancer detection, prognosis and treatment. Metabolism (2016) 65(2):124–39. doi: 10.1016/j.metabol.2015.10.007
9. Jing XM, Yang FM, Shao CC, Wei K, Xie MY, Shen H, et al. Role of hypoxia in cancer therapy by regulating the tumor microenvironment. Mol Cancer (2019) 18(1):157. doi: 10.1186/s12943-019-1089-9
10. Wise DR, DeBerardinis RJ, Mancuso A, Sayed N, Zhang XY, Pfeiffer HK, et al. Myc regulates a transcriptional program that stimulates mitochondrial glutaminolysis and leads to glutamine addiction. Proc Natl Acad Sci U.S.A. (2008) 105(48):18782–7. doi: 10.1073/pnas.0810199105
11. Faubert B, Li KY, Cai L, Hensley CT, Kim J, Zacharias LG, et al. Lactate metabolism in human lung tumors. Cell (2017) 171(2):358–71.e9. doi: 10.1016/j.cell.2017.09.019
12. Yu W, Lei Q, Yang L, Qin G, Liu S, Wang D, et al. Contradictory roles of lipid metabolism in immune response within the tumor microenvironment. J Hematol Oncol (2021) 14(1):187. doi: 10.1186/s13045-021-01200-4
13. Wang W, Zou W. Amino acids and their transporters in T cell immunity and cancer therapy. Mol Cell (2020) 80(3):384–95. doi: 10.1016/j.molcel.2020.09.006
14. Di Virgilio F, Sarti AC, Falzoni S, De Marchi E, Adinolfi E. Extracellular ATP and P2 purinergic signalling in the tumour microenvironment. Nat Rev Cancer (2018) 18(10):601–18. doi: 10.1038/s41568-018-0037-0
15. Vijayan D, Young A, Teng MWL, Smyth MJ. Targeting immunosuppressive adenosine in cancer. Nat Rev Cancer (2017) 17(12):709–24. doi: 10.1038/nrc.2017.110
16. Sawant DV, Yano H, Chikina M, Zhang Q, Liao M, Liu C, et al. Adaptive plasticity of IL-10(+) and IL-35(+) T(Reg) cells cooperatively promotes tumor T cell exhaustion. Nat Immunol (2019) 20(6):724–35. doi: 10.1038/s41590-019-0346-9
17. Fu Q, Xu L, Wang Y, Jiang Q, Liu Z, Zhang J, et al. Tumor-associated macrophage-derived interleukin-23 interlinks kidney cancer glutamine addiction with immune evasion. Eur Urol (2019) 75(5):752–63. doi: 10.1016/j.eururo.2018.09.030
18. Saleh R, Elkord E. Foxp3(+) T regulatory cells in cancer: prognostic biomarkers and therapeutic targets. Cancer Lett (2020) 490:174–85. doi: 10.1016/j.canlet.2020.07.022
19. Sakaguchi S, Mikami N, Wing JB, Tanaka A, Ichiyama K, Ohkura N. Regulatory T cells and human disease. Annu Rev Immunol (2020) 38:541–66. doi: 10.1146/annurev-immunol-042718-041717
20. Braumüller H, Wieder T, Brenner E, Aßmann S, Hahn M, Alkhaled M, et al. T-helper-1-cell cytokines drive cancer into senescence. Nature (2013) 494(7437):361–5. doi: 10.1038/nature11824
21. Saigusa R S, Winkels H, Ley K. T cell subsets and functions in atherosclerosis. Nat Rev Cardiol (2020) 17(7):387–401. doi: 10.1038/s41569-020-0352-5
22. Taylor A, Verhagen J, Blaser K, Akdis M, Akdis CA. Mechanisms of immune suppression by interleukin-10 and transforming growth factor-beta: the role of T regulatory cells. Immunology (2006) 117(4):433–42. doi: 10.1111/j.1365-2567.2006.02321.x
23. Boieri M, Malishkevich A, Guennoun R, Marchese E, Kroon S, Trerice KE, et al. CD4+ T helper 2 cells suppress breast cancer by inducing terminal differentiation. J Exp Med (2022) 219(7):e20201963. doi: 10.1084/jem.20201963
24. Kitajima M, Ito T, Tumes DJ, Endo Y, Onodera A, Hashimoto K, et al. Memory type 2 helper T cells induce long-lasting antitumor immunity by activating natural killer cells. Cancer Res (2011) 71(14):4790–8. doi: 10.1158/0008-5472.Can-10-1572
25. Aspord C, Pedroza-Gonzalez A, Gallegos M, Tindle S, Burton EC, Su D, et al. Breast cancer instructs dendritic cells to prime interleukin 13-secreting CD4+ T cells that facilitate tumor development. J Exp Med (2007) 204(5):1037–47. doi: 10.1084/jem.20061120
26. Geskin LJ, Viragova S, Stolz DB, Fuschiotti P. Interleukin-13 is overexpressed in cutaneous T-cell lymphoma cells and regulates their proliferation. Blood (2015) 125(18):2798–805. doi: 10.1182/blood-2014-07-590398
27. Yoshie O. CCR4 as a therapeutic target for cancer immunotherapy. Cancers (Basel) (2021) 13(21):5542. doi: 10.3390/cancers13215542
28. Chen T, Guo J, Cai Z, Li B, Sun L, Shen Y, et al. Th9 cell differentiation and its dual effects in tumor development. Front Immunol (2020) 11:1026. doi: 10.3389/fimmu.2020.01026
29. Liang SC, Tan XY, Luxenberg DP, Karim R, Dunussi-Joannopoulos K, Collins M, et al. Interleukin (IL)-22 and IL-17 are coexpressed by Th17 cells and cooperatively enhance expression of antimicrobial peptides. J Exp Med (2006) 203(10):2271–9. doi: 10.1084/jem.20061308
30. Liu C, Liu R, Wang B, Lian J, Yao Y, Sun H, et al. Blocking IL-17A enhances tumor response to anti-PD-1 immunotherapy in microsatellite stable colorectal cancer. J Immunother Cancer (2021) 9(1):e001895. doi: 10.1136/jitc-2020-001895
31. McGeachy MJ, Cua DJ, Gaffen SL. The IL-17 family of cytokines in health and disease. Immunity (2019) 50(4):892–906. doi: 10.1016/j.immuni.2019.03.021
32. Liu L, Ge D, Ma L, Mei J, Liu S, Zhang Q, et al. Interleukin-17 and prostaglandin E2 are involved in formation of an M2 macrophage-dominant microenvironment in lung cancer. J Thorac Oncol (2012) 7(7):1091–100. doi: 10.1097/JTO.0b013e3182542752
33. Benchetrit F, Ciree A, Vives V, Warnier G, Gey A, Sautès-Fridman C, et al. Interleukin-17 inhibits tumor cell growth by means of a T-cell-dependent mechanism. Blood (2002) 99(6):2114–21. doi: 10.1182/blood.v99.6.2114
34. Lin Y, Xu J, Su H, Zhong W, Yuan Y, Yu Z, et al. Interleukin-17 is a favorable prognostic marker for colorectal cancer. Clin Transl Oncol (2015) 17(1):50–6. doi: 10.1007/s12094-014-1197-3
35. Perez LG, Kempski J, McGee HM, Pelzcar P, Agalioti T, Giannou A, et al. TGF-β Signaling in Th17 cells promotes IL-22 production and colitis-associated colon cancer. Nat Commun (2020) 11(1):2608. doi: 10.1038/s41467-020-16363-w
36. Sabat R, Ouyang W, Wolk K. Therapeutic opportunities of the IL-22-IL-22R1 system. Nat Rev Drug Discovery (2014) 13(1):21–38. doi: 10.1038/nrd4176
37. Crotty S. T follicular helper cell biology: A decade of discovery and diseases. Immunity (2019) 50(5):1132–48. doi: 10.1016/j.immuni.2019.04.011
38. Cui C, Wang J, Fagerberg E, Chen PM, Connolly KA, Damo M, et al. Neoantigen-driven B cell and CD4 T follicular helper cell collaboration promotes anti-tumor CD8 T cell responses. Cell (2021) 184(25):6101–18.e13. doi: 10.1016/j.cell.2021.11.007
39. Shirota H, Klinman DM, Ito SE, Ito H, Kubo M, Ishioka C. IL4 from T follicular helper cells downregulates antitumor immunity. Cancer Immunol Res (2017) 5(1):61–71. doi: 10.1158/2326-6066.Cir-16-0113
40. Pandey S, Mourcin F, Marchand T, Nayar S, Guirriec M, Pangault C, et al. IL-4/CXCL12 loop is a key regulator of lymphoid stroma function in follicular lymphoma. Blood (2017) 129(18):2507–18. doi: 10.1182/blood-2016-08-737239
41. Bauer L, Müller LJ, Volkers SM, Heinrich F, Mashreghi MF, Ruppert C, et al. Follicular helper-like T cells in the lung highlight a novel role of B cells in sarcoidosis. Am J Respir Crit Care Med (2021) 204(12):1403–17. doi: 10.1164/rccm.202012-4423OC
42. Kemp RA, Ronchese F. Tumor-specific Tc1, but not Tc2, cells deliver protective antitumor immunity. J Immunol (2001) 167(11):6497–502. doi: 10.4049/jimmunol.167.11.6497
43. Arra A, Lingel H, Kuropka B, Pick J, Schnoeder T, Fischer T, et al. The differentiation and plasticity of Tc17 cells are regulated by CTLA-4-mediated effects on stats. Oncoimmunology (2017) 6(2):e1273300. doi: 10.1080/2162402x.2016.1273300
44. St Paul M, Ohashi PS. The roles of CD8(+) T cell subsets in antitumor immunity. Trends Cell Biol (2020) 30(9):695–704. doi: 10.1016/j.tcb.2020.06.003
45. Dobrzanski MJ, Reome JB, Hylind JC, Rewers-Felkins KA. CD8-mediated type 1 antitumor responses selectively modulate endogenous differentiated and nondifferentiated T cell localization, activation, and function in progressive breast cancer. J Immunol (2006) 177(11):8191–201. doi: 10.4049/jimmunol.177.11.8191
46. Dobrzanski MJ, Reome JB, Dutton RW. Role of effector cell-derived IL-4, IL-5, and perforin in early and late stages of type 2 CD8 effector cell-mediated tumor rejection. J Immunol (2001) 167(1):424–34. doi: 10.4049/jimmunol.167.1.424
47. Lu Y, Hong B, Li H, Zheng Y, Zhang M, Wang S, et al. Tumor-specific IL-9-producing CD8+ Tc9 cells are superior effector than type-I cytotoxic Tc1 cells for adoptive immunotherapy of cancers. Proc Natl Acad Sci U.S.A. (2014) 111(6):2265–70. doi: 10.1073/pnas.1317431111
48. Chang SY, Song JH, Guleng B, Cotoner CA, Arihiro S, Zhao Y, et al. Circulatory antigen processing by mucosal dendritic cells controls CD8(+) T cell activation. Immunity (2013) 38(1):153–65. doi: 10.1016/j.immuni.2012.09.018
49. Huber M, Heink S, Grothe H, Guralnik A, Reinhard K, Elflein K, et al. A Th17-like developmental process leads to CD8(+) Tc17 cells with reduced cytotoxic activity. Eur J Immunol (2009) 39(7):1716–25. doi: 10.1002/eji.200939412
50. St Paul M, Saibil SD, Lien SC, Han S, Sayad A, Mulder DT, et al. IL6 induces an IL22(+) CD8(+) T-cell subset with potent antitumor function. Cancer Immunol Res (2020) 8(3):321–33. doi: 10.1158/2326-6066.Cir-19-0521
51. Fridman WH, Pages F, Sautes-Fridman C, Galon J. The immune contexture in human tumours: impact on clinical outcome. Nat Rev Cancer (2012) 12(4):298–306. doi: 10.1038/nrc3245
52. Matsushita H, Hosoi A, Ueha S, Abe J, Fujieda N, Tomura M, et al. Cytotoxic T lymphocytes block tumor growth both by lytic activity and IFNγ-dependent cell-cycle arrest. Cancer Immunol Res (2015) 3(1):26–36. doi: 10.1158/2326-6066.Cir-14-0098
53. Chiang CLL, Balint K, Coukos G, Kandalaft LE. Potential approaches for more successful dendritic cell-based immunotherapy. Expert Opin Biol Ther (2015) 15(4):569–82. doi: 10.1517/14712598.2015.1000298
54. Orange JS. Formation and function of the lytic NK-cell immunological synapse. Nat Rev Immunol (2008) 8(9):713–25. doi: 10.1038/nri2381
55. Delgoffe GM, Woo SR, Turnis ME, Gravano DM, Guy C, Overacre AE, et al. Stability and function of regulatory T cells is maintained by a neuropilin-1-semaphorin-4a axis. Nature (2013) 501(7466):252–6. doi: 10.1038/nature12428
56. Saito T, Nishikawa H, Wada H, Nagano Y, Sugiyama D, Atarashi K, et al. Two FOXP3(+)CD4(+)T cell subpopulations distinctly control the prognosis of colorectal cancers. Nat Med (2016) 22(6):679–84. doi: 10.1038/nm.4086
57. Yang ZZ, Guo JC, Weng LL, Tang WX, Jin SL, Ma W. Myeloid-derived suppressor cells-new and exciting players in lung cancer. J Hematol Oncol (2020) 13(1):10. doi: 10.1186/s13045-020-0843-1
58. Groth C, Hu XY, Weber R, Fleming V, Altevogt P, Utikal J, et al. Immunosuppression mediated by myeloid-derived suppressor cells (MDSCs) during tumour progression. Br J Cancer (2019) 120(1):16–25. doi: 10.1038/s41416-018-0333-1
59. Crivellato E, Nico B, Ribatti D. Mast cells and tumour angiogenesis: new insight from experimental carcinogenesis. Cancer Lett (2008) 269(1):1–6. doi: 10.1016/j.canlet.2008.03.031
60. Eissmann MF, Dijkstra C, Jarnicki A, Phesse T, Brunnberg J, Poh AR, et al. IL-33-mediated mast cell activation promotes gastric cancer through macrophage mobilization. Nat Commun (2019) 10(1):2735. doi: 10.1038/s41467-019-10676-1
61. Huang SC, Everts B, Ivanova Y, O'Sullivan D, Nascimento M, Smith AM, et al. Cell-intrinsic lysosomal lipolysis is essential for alternative activation of macrophages. Nat Immunol (2014) 15(9):846–55. doi: 10.1038/ni.2956
62. Quail DF, Joyce JA. Microenvironmental regulation of tumor progression and metastasis. Nat Med (2013) 19(11):1423–37. doi: 10.1038/nm.3394
63. Fridlender ZG, Sun J, Kim S, Kapoor V, Cheng GJ, Ling LN, et al. Polarization of tumor-associated neutrophil phenotype by TGF-β: “N1” Versus “N2” Tan. Cancer Cell (2009) 16(3):183–94. doi: 10.1016/j.ccr.2009.06.017
64. Ma X, Xiao L, Liu L, Ye L, Su P, Bi E, et al. CD36-mediated ferroptosis dampens intratumoral CD8(+) T cell effector function and impairs their antitumor ability. Cell Metab (2021) 33(5):1001–12.e5. doi: 10.1016/j.cmet.2021.02.015
65. Xu S, Chaudhary O, Rodriguez-Morales P, Sun X, Chen D, Zappasodi R, et al. Uptake of oxidized lipids by the scavenger receptor CD36 promotes lipid peroxidation and dysfunction in CD8(+) T cells in tumors. Immunity (2021) 54(7):1561–77.e7. doi: 10.1016/j.immuni.2021.05.003
66. Ma X, Bi E, Lu Y, Su P, Huang C, Liu L, et al. Cholesterol induces CD8(+) T cell exhaustion in the tumor microenvironment. Cell Metab (2019) 30(1):143–56.e5. doi: 10.1016/j.cmet.2019.04.002
67. Herber DL, Cao W, Nefedova Y, Novitskiy SV, Nagaraj S, Tyurin VA, et al. Lipid accumulation and dendritic cell dysfunction in cancer. Nat Med (2010) 16(8):880–6. doi: 10.1038/nm.2172
68. Oh DS, Lee HK. Autophagy protein ATG5 regulates CD36 expression and anti-tumor MHC class II antigen presentation in dendritic cells. Autophagy (2019) 15(12):2091–106. doi: 10.1080/15548627.2019.1596493
69. Hu X, Jia X, Xu C, Wei Y, Wang Z, Liu G, et al. Downregulation of NK cell activities in apolipoprotein C-III-induced hyperlipidemia resulting from lipid-induced metabolic reprogramming and crosstalk with lipid-laden dendritic cells. Metabolism (2021) 120:154800. doi: 10.1016/j.metabol.2021.154800
70. Su P, Wang Q, Bi EG, Ma XZ, Liu LT, Yang MJ, et al. Enhanced lipid accumulation and metabolism are required for the differentiation and activation of tumor-associated macrophages. Cancer Res (2020) 80(7):1438–50. doi: 10.1158/0008-5472.Can-19-2994
71. Cao W, Gabrilovich D. Contribution of fatty acid accumulation to myeloid-derived suppressor cell function in cancer. Cancer Res (2011) 71(8 Supplement):3649. doi: 10.1158/1538-7445.Am2011-3649
72. Ho PC, Bihuniak JD, Macintyre AN, Staron M, Liu XJ, Amezquita R, et al. Phosphoenolpyruvate is a metabolic checkpoint of anti-tumor T cell responses. Cell (2015) 162(6):1217–28. doi: 10.1016/j.cell.2015.08.012
73. Siska PJ, van der Windt GJ, Kishton RJ, Cohen S, Eisner W, MacIver NJ, et al. Suppression of glut1 and glucose metabolism by decreased akt/mTORC1 signaling drives T cell impairment in B cell leukemia. J Immunol (2016) 197(6):2532–40. doi: 10.4049/jimmunol.1502464
74. Song M, Sandoval TA, Chae CS, Chopra S, Tan C, Rutkowski MR, et al. IRE1α-XBP1 controls T cell function in ovarian cancer by regulating mitochondrial activity. Nature (2018) 562(7727):423–8. doi: 10.1038/s41586-018-0597-x
75. Swamy M, Pathak S, Grzes KM, Damerow S, Sinclair LV, van Aalten DMF, et al. Glucose and glutamine fuel protein O-Glcnacylation to control T cell self-renewal and malignancy. Nat Immunol (2016) 17(6):712–20. doi: 10.1038/ni.3439
76. Araujo L, Khim P, Mkhikian H, Mortales CL, Demetriou M. Glycolysis and glutaminolysis cooperatively control T cell function by limiting metabolite supply to N-glycosylation. Elife (2017) 6:e21330. doi: 10.7554/eLife.21330
77. Donnelly RP, Loftus RM, Keating SE, Liou KT, Biron CA, Gardiner CM, et al. mTORC1-dependent metabolic reprogramming is a prerequisite for NK cell effector function. J Immunol (2014) 193(9):4477–84. doi: 10.4049/jimmunol.1401558
78. Angelin A, Gil-de-Gomez L, Dahiya S, Jiao J, Guo L, Levine MH, et al. Foxp3 reprograms T cell metabolism to function in low-glucose, high-lactate environments. Cell Metab (2017) 25(6):1282–93.e7. doi: 10.1016/j.cmet.2016.12.018
79. Morrissey SM, Zhang F, Ding C, Montoya-Durango DE, Hu X, Yang C, et al. Tumor-derived exosomes drive immunosuppressive macrophages in a pre-metastatic niche through glycolytic dominant metabolic reprogramming. Cell Metab (2021) 33(10):2040–58.e10. doi: 10.1016/j.cmet.2021.09.002
80. Fischer K, Hoffmann P, Voelkl S, Meidenbauer N, Ammer J, Edinger M, et al. Inhibitory effect of tumor cell-derived lactic acid on human T cells. Blood (2007) 109(9):3812–9. doi: 10.1182/blood-2006-07-035972
81. Pucino V, Certo M, Bulusu V, Cucchi D, Goldmann K, Pontarini E, et al. Lactate buildup at the site of chronic inflammation promotes disease by inducing CD4(+) T cell metabolic rewiring. Cell Metab (2019) 30(6):1055–74.e8. doi: 10.1016/j.cmet.2019.10.004
82. Watson MJ, Vignali PDA, Mullett SJ, Overacre-Delgoffe AE, Peralta RM, Grebinoski S, et al. Metabolic support of tumour-infiltrating regulatory T cells by lactic acid. Nature (2021) 591(7851):645–51. doi: 10.1038/s41586-020-03045-2
83. Sangsuwan R, Thuamsang B, Pacifici N, Allen R, Han H, Miakicheva S, et al. Lactate exposure promotes immunosuppressive phenotypes in innate immune cells. Cell Mol Bioeng (2020) 13(5):541–57. doi: 10.1007/s12195-020-00652-x
84. Greene LI, Bruno TC, Christenson JL, D'Alessandro A, Culp-Hill R, Torkko K, et al. A role for tryptophan-2,3-dioxygenase in CD8 T-cell suppression and evidence of tryptophan catabolism in breast cancer patient plasma. Mol Cancer Res (2019) 17(1):131–9. doi: 10.1158/1541-7786.Mcr-18-0362
85. Mezrich JD, Fechner JH, Zhang X, Johnson BP, Burlingham WJ, Bradfield CA. An interaction between kynurenine and the aryl hydrocarbon receptor can generate regulatory T cells. J Immunol (2010) 185(6):3190–8. doi: 10.4049/jimmunol.0903670
86. Takenaka MC, Gabriely G, Rothhammer V, Mascanfroni ID, Wheeler MA, Chao CC, et al. Control of tumor-associated macrophages and T cells in glioblastoma via AHR and CD39. Nat Neurosci (2019) 22(5):729–40. doi: 10.1038/s41593-019-0370-y
87. Campesato LF, Budhu S, Tchaicha J, Weng CH, Gigoux M, Cohen IJ, et al. Blockade of the AHR restricts a treg-macrophage suppressive axis induced by L-kynurenine. Nat Commun (2020) 11(1):4011. doi: 10.1038/s41467-020-17750-z
88. Triplett TA, Garrison KC, Marshall N, Donkor M, Blazeck J, Lamb C, et al. Reversal of indoleamine 2,3-dioxygenase-mediated cancer immune suppression by systemic kynurenine depletion with a therapeutic enzyme. Nat Biotechnol (2018) 36(8):758–64. doi: 10.1038/nbt.4180
89. Xu Y, Yu WM, Yang TT, Zhang MZ, Liang C, Cai XL, et al. Overexpression of BCAT1 is a prognostic marker in gastric cancer. Hum Pathol (2018) 75:41–6. doi: 10.1016/j.humpath.2018.02.003
90. Silva LS, Poschet G, Nonnenmacher Y, Becker HM, Sapcariu S, Gaupel AC, et al. Branched-chain ketoacids secreted by glioblastoma cells via MCT1 modulate macrophage phenotype. EMBO Rep (2017) 18(12):2172–85. doi: 10.15252/embr.201744154
91. Ohta A, Kini R, Ohta A, Subramanian M, Madasu M, Sitkovsky M. The development and immunosuppressive functions of CD4(+) CD25(+) foxP3(+) regulatory T cells are under influence of the adenosine-A2A adenosine receptor pathway. Front Immunol (2012) 3:190. doi: 10.3389/fimmu.2012.00190
92. Cekic C, Linden J. Adenosine A2A receptors intrinsically regulate CD8(+) T cells in the tumor microenvironment. Cancer Res (2014) 74(24):7239–49. doi: 10.1158/0008-5472.Can-13-3581
93. Koscso B, Csoka B, Kokai E, Nemeth ZH, Pacher P, Virag L, et al. Adenosine augments IL-10-induced STAT3 signaling in M2c macrophages. J Leukoc Biol (2013) 94(6):1309–15. doi: 10.1189/jlb.0113043
94. Ryzhov S, Novitskiy SV, Goldstein AE, Biktasova A, Blackburn MR, Biaggioni I, et al. Adenosinergic regulation of the expansion and immunosuppressive activity of CD11b(+)Gr1(+) cells. J Immunol (2011) 187(11):6120–9. doi: 10.4049/jimmunol.1101225
95. Chiu DKC, Tse APW, Xu IMJ, Di Cui J, Lai RKH, Li LL, et al. Hypoxia inducible factor HIF-1 promotes myeloid-derived suppressor cells accumulation through ENTPD2/CD39L1 in hepatocellular carcinoma. Nat Commun (2017) 8(1):517. doi: 10.1038/s41467-017-00530-7
96. Fahy E, Subramaniam S, Murphy RC, Nishijima M, Raetz CR, Shimizu T, et al. Update of the lipid maps comprehensive classification system for lipids. J Lipid Res (2009) 50 Suppl(Suppl):S9–14. doi: 10.1194/jlr.R800095-JLR200
97. Duttaroy AK. Transport of fatty acids across the human placenta: A review. Prog Lipid Res (2009) 48(1):52–61. doi: 10.1016/j.plipres.2008.11.001
98. Huang X, Zhou Y, Sun Y, Wang Q. Intestinal fatty acid binding protein: A rising therapeutic target in lipid metabolism. Prog Lipid Res (2022) 87:101178. doi: 10.1016/j.plipres.2022.101178
99. Kennedy MA, Barrera GC, Nakamura K, Baldan A, Tarr P, Fishbein MC, et al. ABCG1 has a critical role in mediating cholesterol efflux to hdl and preventing cellular lipid accumulation. Cell Metab (2005) 1(2):121–31. doi: 10.1016/j.cmet.2005.01.002
100. Wang H, Franco F, Tsui YC, Xie X, Trefny MP, Zappasodi R, et al. CD36-mediated metabolic adaptation supports regulatory T cell survival and function in tumors. Nat Immunol (2020) 21(3):298–308. doi: 10.1038/s41590-019-0589-5
101. Liu S, Zhang H, Li Y, Zhang Y, Bian Y, Zeng Y, et al. S100A4 enhances protumor macrophage polarization by control of PPAR-γ-dependent induction of fatty acid oxidation. J Immunother Cancer (2021) 9(6):e002548. doi: 10.1136/jitc-2021-002548
102. Al-Khami AA, Zheng L, Del Valle L, Hossain F, Wyczechowska D, Zabaleta J, et al. Exogenous lipid uptake induces metabolic and functional reprogramming of tumor-associated myeloid-derived suppressor cells. Oncoimmunology (2017) 6(10):e1344804. doi: 10.1080/2162402X.2017.1344804
103. Xin G, Chen Y, Topchyan P, Kasmani MY, Burns R, Volberding PJ, et al. Targeting PIM1-mediated metabolism in myeloid suppressor cells to treat cancer. Cancer Immunol Res (2021) 9(4):454–69. doi: 10.1158/2326-6066.CIR-20-0433
104. Michelet X, Dyck L, Hogan A, Loftus RM, Duquette D, Wei K, et al. Metabolic reprogramming of natural killer cells in obesity limits antitumor responses. Nat Immunol (2018) 19(12):1330–40. doi: 10.1038/s41590-018-0251-7
105. Adeshakin AO, Liu W, Adeshakin FO, Afolabi LO, Zhang M, Zhang G, et al. Regulation of ROS in myeloid-derived suppressor cells through targeting fatty acid transport protein 2 enhanced anti-PD-L1 tumor immunotherapy. Cell Immunol (2021) 362:104286. doi: 10.1016/j.cellimm.2021.104286
106. Veglia F, Tyurin VA, Blasi M, De Leo A, Kossenkov AV, Donthireddy L, et al. Fatty acid transport protein 2 reprograms neutrophils in cancer. Nature (2019) 569(7754):73–8. doi: 10.1038/s41586-019-1118-2
107. Hao J, Yan F, Zhang Y, Triplett A, Zhang Y, Schultz DA, et al. Expression of adipocyte/macrophage fatty acid-binding protein in tumor-associated macrophages promotes breast cancer progression. Cancer Res (2018) 78(9):2343–55. doi: 10.1158/0008-5472.Can-17-2465
108. Zhang Y, Sun Y, Rao E, Yan F, Li Q, Zhang Y, et al. Fatty acid-binding protein E-FABP restricts tumor growth by promoting IFN-β Responses in tumor-associated macrophages. Cancer Res (2014) 74(11):2986–98. doi: 10.1158/0008-5472.Can-13-2689
109. Moore SM, Holt VV, Malpass LR, Hines IN, Wheeler MD. Fatty acid-binding protein 5 limits the anti-inflammatory response in murine macrophages. Mol Immunol (2015) 67(2, Part B):265–75. doi: 10.1016/j.molimm.2015.06.001
110. Field CS, Baixauli F, Kyle RL, Puleston DJ, Cameron AM, Sanin DE, et al. Mitochondrial integrity regulated by lipid metabolism is a cell-intrinsic checkpoint for treg suppressive function. Cell Metab (2020) 31(2):422–37.e5. doi: 10.1016/j.cmet.2019.11.021
111. Wang F, Beck-Garcia K, Zorzin C, Schamel WWA, Davis MM. Inhibition of T cell receptor signaling by cholesterol sulfate, a naturally occurring derivative of membrane cholesterol. Nat Immunol (2016) 17(7):844–50. doi: 10.1038/ni.3462
112. Yang W, Bai Y, Xiong Y, Zhang J, Chen S, Zheng X, et al. Potentiating the antitumour response of CD8(+) T cells by modulating cholesterol metabolism. Nature (2016) 531(7596):651–5. doi: 10.1038/nature17412
113. Armstrong AJ, Gebre AK, Parks JS, Hedrick CC. ATP-binding cassette transporter G1 negatively regulates thymocyte and peripheral lymphocyte proliferation. J Immunol (2010) 184(1):173–83. doi: 10.4049/jimmunol.0902372
114. Yuan J, Cai T, Zheng X, Ren Y, Qi J, Lu X, et al. Potentiating CD8(+) T cell antitumor activity by inhibiting PCSK9 to promote LDLR-mediated TCR recycling and signaling. Protein Cell (2021) 12(4):240–60. doi: 10.1007/s13238-021-00821-2
115. Ma X, Bi E, Huang C, Lu Y, Xue G, Guo X, et al. Cholesterol negatively regulates IL-9-producing CD8(+) T cell differentiation and antitumor activity. J Exp Med (2018) 215(6):1555–69. doi: 10.1084/jem.20171576
116. Ma LQ, Wang L, Nelson AT, Han C, He SS, Henn MA, et al. 27-hydroxycholesterol acts on myeloid immune cells to induce T cell dysfunction, promoting breast cancer progression. Cancer Lett (2020) 493:266–83. doi: 10.1016/j.canlet.2020.08.020
117. Sag D, Cekic C, Wu RP, Linden J, Hedrick CC. The cholesterol transporter ABCG1 links cholesterol homeostasis and tumour immunity. Nat Commun (2015) 6:6354. doi: 10.1038/ncomms7354
118. Westerterp M, Gautier EL, Ganda A, Molusky MM, Wang W, Fotakis P, et al. Cholesterol accumulation in dendritic cells links the inflammasome to acquired immunity. Cell Metab (2017) 25(6):1294–304.e6. doi: 10.1016/j.cmet.2017.04.005
119. Sag D, Wingender G, Nowyhed H, Wu RP, Gebre AK, Parks JS, et al. ATP-binding cassette transporter G1 intrinsically regulates invariant NKT cell development. J Immunol (2012) 189(11):5129–38. doi: 10.4049/jimmunol.1201570
120. Nowyhed HN, Chandra S, Kiosses W, Marcovecchio P, Andary F, Zhao M, et al. ATP binding cassette transporter ABCA7 regulates NKT cell development and function by controlling CD1d expression and lipid raft content. Sci Rep (2017) 7:40273. doi: 10.1038/srep40273
121. Ancey PB, Contat C, Meylan E. Glucose transporters in cancer - from tumor cells to the tumor microenvironment. FEBS J (2018) 285(16):2926–43. doi: 10.1111/febs.14577
122. Macintyre AN, Gerriets VA, Nichols AG, Michalek RD, Rudolph MC, Deoliveira D, et al. The glucose transporter glut1 is selectively essential for CD4 T cell activation and effector function. Cell Metab (2014) 20(1):61–72. doi: 10.1016/j.cmet.2014.05.004
123. Lee MJ, Yun SJ, Lee B, Jeong E, Yoon G, Kim K, et al. Association of TIM-3 expression with glucose metabolism in jurkat T cells. BMC Immunol (2020) 21(1):48. doi: 10.1186/s12865-020-00377-6
124. Na KJ, Choi H, Oh HR, Kim YH, Lee SB, Jung YJ, et al. Reciprocal change in glucose metabolism of cancer and immune cells mediated by different glucose transporters predicts immunotherapy response. Theranostics (2020) 10(21):9579–90. doi: 10.7150/thno.48954
125. Michalek RD, Gerriets VA, Jacobs SR, Macintyre AN, MacIver NJ, Mason EF, et al. Cutting edge: distinct glycolytic and lipid oxidative metabolic programs are essential for effector and regulatory CD4+ T cell subsets. J Immunol (2011) 186(6):3299–303. doi: 10.4049/jimmunol.1003613
126. Pacella I, Procaccini C, Focaccetti C, Miacci S, Timperi E, Faicchia D, et al. Fatty acid metabolism complements glycolysis in the selective regulatory T cell expansion during tumor growth. Proc Natl Acad Sci U.S.A. (2018) 115(28):E6546–E55. doi: 10.1073/pnas.1720113115
127. Ancey PB, Contat C, Boivin G, Sabatino S, Pascual J, Zangger N, et al. GLUT1 expression in tumor-associated neutrophils promotes lung cancer growth and resistance to radiotherapy. Cancer Res (2021) 81(9):2345–57. doi: 10.1158/0008-5472.CAN-20-2870
128. Payen VL, Mina E, Van Hee VF, Porporato PE, Sonveaux P. Monocarboxylate transporters in cancer. Mol Metab (2020) 33:48–66. doi: 10.1016/j.molmet.2019.07.006
129. Brown TP, Ganapathy V. Lactate/GPR81 signaling and proton motive force in cancer: role in angiogenesis, immune escape, nutrition, and warburg phenomenon. Pharmacol Ther (2020) 206:107451. doi: 10.1016/j.pharmthera.2019.107451
130. Weiss HJ, Angiari S. Metabolite transporters as regulators of immunity. Metabolites (2020) 10(10):418. doi: 10.3390/metabo10100418
131. Long Y, Gao Z, Hu X, Xiang F, Wu Z, Zhang J, et al. Downregulation of MCT4 for lactate exchange promotes the cytotoxicity of NK cells in breast carcinoma. Cancer Med (2018) 7(9):4690–700. doi: 10.1002/cam4.1713
132. Gottfried E, Kunz-Schughart LA, Ebner S, Mueller-Klieser W, Hoves S, Andreesen R, et al. Tumor-derived lactic acid modulates dendritic cell activation and antigen expression. Blood (2006) 107(5):2013–21. doi: 10.1182/blood-2005-05-1795
133. Raychaudhuri D, Bhattacharya R, Sinha BP, Liu CSC, Ghosh AR, Rahaman O, et al. Lactate induces pro-tumor reprogramming in intratumoral plasmacytoid dendritic cells. Front Immunol (2019) 10:1878. doi: 10.3389/fimmu.2019.01878
134. Kumagai S, Koyama S, Itahashi K, Tanegashima T, Lin YT, Togashi Y, et al. Lactic acid promotes PD-1 expression in regulatory T cells in highly glycolytic tumor microenvironments. Cancer Cell (2022) 40(2):201–18.e9. doi: 10.1016/j.ccell.2022.01.001
135. Zhang J, Muri J, Fitzgerald G, Gorski T, Gianni-Barrera R, Masschelein E, et al. Endothelial lactate controls muscle regeneration from ischemia by inducing M2-like macrophage polarization. Cell Metab (2020) 31(6):1136–53.e7. doi: 10.1016/j.cmet.2020.05.004
136. Li B, Yang Q, Li Z, Xu Z, Sun S, Wu Q, et al. Expression of monocarboxylate transporter 1 in immunosuppressive macrophages is associated with the poor prognosis in breast cancer. Front Oncol (2020) 10:574787. doi: 10.3389/fonc.2020.574787
137. Tan Z, Xie N, Banerjee S, Cui H, Fu M, Thannickal VJ, et al. The monocarboxylate transporter 4 is required for glycolytic reprogramming and inflammatory response in macrophages. J Biol Chem (2015) 290(1):46–55. doi: 10.1074/jbc.M114.603589
138. Haas R, Smith J, Rocher-Ros V, Nadkarni S, Montero-Melendez T, D'Acquisto F, et al. Lactate regulates metabolic and proinflammatory circuits in control of T cell migration and effector functions. PloS Biol (2015) 13(7):e1002202. doi: 10.1371/journal.pbio.1002202
139. Manoharan I, Prasad PD, Thangaraju M, Manicassamy S. Lactate-dependent regulation of immune responses by dendritic cells and macrophages. Front Immunol (2021) 12:691134. doi: 10.3389/fimmu.2021.691134
140. Kandasamy P, Gyimesi G, Kanai Y, Hediger MA. Amino acid transporters revisited: new views in health and disease. Trends Biochem Sci (2018) 43(10):752–89. doi: 10.1016/j.tibs.2018.05.003
141. Mattox ML, D'Angelo JA, Grimes ZM, Fiebiger E, Dickinson BL. The cystine/glutamate antiporter regulates indoleamine 2,3-dioxygenase protein levels and enzymatic activity in human dendritic cells. Am J Clin Exp Immunol (2012) 1(2):113–23.
142. Huang Y, Dai Z, Barbacioru C, Sadee W. Cystine-glutamate transporter SLC7A11 in cancer chemosensitivity and chemoresistance. Cancer Res (2005) 65(16):7446–54. doi: 10.1158/0008-5472.CAN-04-4267
143. Srivastava MK, Sinha P, Clements VK, Rodriguez P, Ostrand-Rosenberg S. Myeloid-derived suppressor cells inhibit T-cell activation by depleting cystine and cysteine. Cancer Res (2010) 70(1):68–77. doi: 10.1158/0008-5472.CAN-09-2587
144. Levring TB, Hansen AK, Nielsen BL, Kongsbak M, von Essen MR, Woetmann A, et al. Activated human CD4+ T cells express transporters for both cysteine and cystine. Sci Rep (2012) 2:266. doi: 10.1038/srep00266
145. Garg SK, Yan ZH, Vitvitsky V, Banerjee R. Differential dependence on cysteine from transsulfuration versus transport during T cell activation. Antioxid Redox Signal (2011) 15(1):39–47. doi: 10.1089/ars.2010.3496
146. Kobayashi S, Hamashima S, Homma T, Sato M, Kusumi R, Bannai S, et al. Cystine/glutamate transporter, system xc(-), is involved in nitric oxide production in mouse peritoneal macrophages. Nitric Oxide (2018) 78:32–40. doi: 10.1016/j.niox.2018.05.005
147. Pampliega O, Domercq M, Soria FN, Villoslada P, Rodriguez-Antiguedad A, Matute C. Increased expression of cystine/glutamate antiporter in multiple sclerosis. J Neuroinflamm (2011) 8:63. doi: 10.1186/1742-2094-8-63
148. D'Angelo JA, Dehlink E, Platzer B, Dwyer P, Circu ML, Garay J, et al. The cystine/glutamate antiporter regulates dendritic cell differentiation and antigen presentation. J Immunol (2010) 185(6):3217–26. doi: 10.4049/jimmunol.1001199
149. Wang W, Green M, Choi JE, Gijon M, Kennedy PD, Johnson JK, et al. CD8(+) T cells regulate tumour ferroptosis during cancer immunotherapy. Nature (2019) 569(7755):270–4. doi: 10.1038/s41586-019-1170-y
150. Koppula P, Zhuang L, Gan B. Cystine transporter SLC7A11/xCT in cancer: ferroptosis, nutrient dependency, and cancer therapy. Protein Cell (2021) 12(8):599–620. doi: 10.1007/s13238-020-00789-5
151. Arensman MD, Yang XS, Leahy DM, Toral-Barza L, Mileski M, Rosfjord EC, et al. Cystine-glutamate antiporter xct deficiency suppresses tumor growth while preserving antitumor immunity. Proc Natl Acad Sci U.S.A. (2019) 116(19):9533–42. doi: 10.1073/pnas.1814932116
152. Song W, Li D, Tao L, Luo Q, Chen L. Solute carrier transporters: the metabolic gatekeepers of immune cells. Acta Pharm Sin B (2020) 10(1):61–78. doi: 10.1016/j.apsb.2019.12.006
153. Carr EL, Kelman A, Wu GS, Gopaul R, Senkevitch E, Aghvanyan A, et al. Glutamine uptake and metabolism are coordinately regulated by ERK/MAPK during T lymphocyte activation. J Immunol (2010) 185(2):1037–44. doi: 10.4049/jimmunol.0903586
154. Loftus RM, Assmann N, Kedia-Mehta N, O'Brien KL, Garcia A, Gillespie C, et al. Amino acid-dependent cMyc expression is essential for NK cell metabolic and functional responses in mice. Nat Commun (2018) 9(1):2341. doi: 10.1038/s41467-018-04719-2
155. Nakaya M, Xiao Y, Zhou X, Chang JH, Chang M, Cheng X, et al. Inflammatory T cell responses rely on amino acid transporter ASCT2 facilitation of glutamine uptake and mTORC1 kinase activation. Immunity (2014) 40(5):692–705. doi: 10.1016/j.immuni.2014.04.007
156. Rosilio C, Nebout M, Imbert V, Griessinger E, Neffati Z, Benadiba J, et al. L-type amino-acid transporter 1 (LAT1): A therapeutic target supporting growth and survival of T-cell lymphoblastic lymphoma/T-cell acute lymphoblastic leukemia. Leukemia (2015) 29(6):1253–66. doi: 10.1038/leu.2014.338
157. Nachef M, Ali AK, Almutairi SM, Lee SH. Targeting SLC1A5 and SLC3A2/SLC7A5 as a potential strategy to strengthen anti-tumor immunity in the tumor microenvironment. Front Immunol (2021) 12:624324. doi: 10.3389/fimmu.2021.624324
158. Cibrian D, Castillo-González R, Fernández-Gallego N, de la Fuente H, Jorge I, Saiz ML, et al. Targeting L-type amino acid transporter 1 in innate and adaptive T cells efficiently controls skin inflammation. J Allergy Clin Immunol (2020) 145(1):199–214.e11. doi: 10.1016/j.jaci.2019.09.025
159. Jensen H, Potempa M, Gotthardt D, Lanier LL. Cutting edge: IL-2-induced expression of the amino acid transporters SLC1A5 and CD98 is a prerequisite for NKG2D-M ediated activation of human NK cells. J Immunol (2017) 199(6):1967–72. doi: 10.4049/jimmunol.1700497
160. Cellarier E, Durando X, Vasson MP, Farges MC, Demiden A, Maurizis JC, et al. Methionine dependency and cancer treatment. Cancer Treat Rev (2003) 29(6):489–99. doi: 10.1016/s0305-7372(03)00118-x
161. Sanderson SM, Gao X, Dai Z, Locasale JW. Methionine metabolism in health and cancer: A nexus of diet and precision medicine. Nat Rev Cancer (2019) 19(11):625–37. doi: 10.1038/s41568-019-0187-8
162. Sinclair LV, Howden AJ, Brenes A, Spinelli L, Hukelmann JL, Macintyre AN, et al. Antigen receptor control of methionine metabolism in T cells. Elife (2019) 8:e44210. doi: 10.7554/eLife.44210
163. Xu Q, Liu Y, Sun W, Song T, Jiang X, Zeng K, et al. Blockade LAT1 mediates methionine metabolism to overcome oxaliplatin resistance under hypoxia in renal cell carcinoma. Cancers (Basel) (2022) 14(10):2551. doi: 10.3390/cancers14102551
164. Roy DG, Chen J, Mamane V, Ma EH, Muhire BM, Sheldon RD, et al. Methionine metabolism shapes T helper cell responses through regulation of epigenetic reprogramming. Cell Metab (2020) 31(2):250–66.e9. doi: 10.1016/j.cmet.2020.01.006
165. Bian Y, Li W, Kremer DM, Sajjakulnukit P, Li S, Crespo J, et al. Cancer SLC43A2 alters T cell methionine metabolism and histone methylation. Nature (2020) 585(7824):277–82. doi: 10.1038/s41586-020-2682-1
166. Hung MH, Lee JS, Ma C, Diggs LP, Heinrich S, Chang CW, et al. Tumor methionine metabolism drives T-cell exhaustion in hepatocellular carcinoma. Nat Commun (2021) 12(1):1455. doi: 10.1038/s41467-021-21804-1
167. Ansari RE, Craze ML, Althobiti M, Alfarsi L, Ellis IO, Rakha EA, et al. Enhanced glutamine uptake influences composition of immune cell infiltrates in breast cancer. Br J Cancer (2020) 122(1):94–101. doi: 10.1038/s41416-019-0626-z
168. Sinclair LV, Rolf J, Emslie E, Shi YB, Taylor PM, Cantrell DA. Control of amino-acid transport by antigen receptors coordinates the metabolic reprogramming essential for T cell differentiation. Nat Immunol (2013) 14(5):500–8. doi: 10.1038/ni.2556
169. Nicklin P, Bergman P, Zhang B, Triantafellow E, Wang H, Nyfeler B, et al. Bidirectional transport of amino acids regulates mTOR and autophagy. Cell (2009) 136(3):521–34. doi: 10.1016/j.cell.2008.11.044
170. Wang Q, Holst J. L-type amino acid transport and cancer: targeting the mTORC1 pathway to inhibit neoplasia. Am J Cancer Res (2015) 5(4):1281–94.
171. Timosenko E, Ghadbane H, Silk JD, Shepherd D, Gileadi U, Howson LJ, et al. Nutritional stress induced by tryptophan-degrading enzymes results in ATF4-dependent reprogramming of the amino acid transporter profile in tumor cells. Cancer Res (2016) 76(21):6193–204. doi: 10.1158/0008-5472.CAN-15-3502
172. Seymour RL, Ganapathy V, Mellor AL, Munn DH. A high-affinity, tryptophan-selective amino acid transport system in human macrophages. J Leukoc Biol (2006) 80(6):1320–7. doi: 10.1189/jlb.1205727
173. Silk JD, Lakhal S, Laynes R, Vallius L, Karydis I, Marcea C, et al. IDO induces expression of a novel tryptophan transporter in mouse and human tumor cells. J Immunol (2011) 187(4):1617–25. doi: 10.4049/jimmunol.1000815
174. Tina E, Prosen S, Lennholm S, Gasparyan G, Lindberg M, Gothlin Eremo A. Expression profile of the amino acid transporters SLC7A5, SLC7A7, SLC7A8 and the enzyme TDO2 in basal cell carcinoma. Br J Dermatol (2019) 180(1):130–40. doi: 10.1111/bjd.16905
175. Liu Y, Liang X, Dong W, Fang Y, Lv J, Zhang T, et al. Tumor-repopulating cells induce PD-1 expression in CD8(+) T cells by transferring kynurenine and ahR activation. Cancer Cell (2018) 33(3):480–94.e7. doi: 10.1016/j.ccell.2018.02.005
176. Sinclair LV, Neyens D, Ramsay G, Taylor PM, Cantrell DA. Single cell analysis of kynurenine and system L amino acid transport in T cells. Nat Commun (2018) 9(1):1981. doi: 10.1038/s41467-018-04366-7
177. Delage B, Fennell DA, Nicholson L, McNeish I, Lemoine NR, Crook T, et al. Arginine deprivation and argininosuccinate synthetase expression in the treatment of cancer. Int J Cancer (2010) 126(12):2762–72. doi: 10.1002/ijc.25202
178. Chantranupong L, Scaria SM, Saxton RA, Gygi MP, Shen K, Wyant GA, et al. The castor proteins are arginine sensors for the mtorc1 pathway. Cell (2016) 165(1):153–64. doi: 10.1016/j.cell.2016.02.035
179. Thompson RW, Pesce JT, Ramalingam T, Wilson MS, White S, Cheever AW, et al. Cationic amino acid transporter-2 regulates immunity by modulating arginase activity. PloS Pathog (2008) 4(3):e1000023. doi: 10.1371/journal.ppat.1000023
180. De Santo C, Booth S, Vardon A, Cousins A, Tubb V, Perry T, et al. The arginine metabolome in acute lymphoblastic leukemia can be targeted by the pegylated-recombinant arginase I BCT-100. Int J Cancer (2018) 142(7):1490–502. doi: 10.1002/ijc.31170
181. Poillet-Perez L, Xie XQ, Zhan L, Yang Y, Sharp DW, Hu ZS, et al. Autophagy maintains tumour growth through circulating arginine. Nature (2018) 563(7732):569–73. doi: 10.1038/s41586-018-0697-7
182. Werner A, Pieh D, Echchannaoui H, Rupp J, Rajalingam K, Theobald M, et al. Cationic amino acid transporter-1-mediated arginine uptake is essential for chronic lymphocytic leukemia cell proliferation and viability. Front Oncol (2019) 9:1268. doi: 10.3389/fonc.2019.01268
183. Czystowska-Kuzmicz M, Sosnowska A, Nowis D, Ramji K, Szajnik M, Chlebowska-Tuz J, et al. Small extracellular vesicles containing arginase-1 suppress T-cell responses and promote tumor growth in ovarian carcinoma. Nat Commun (2019) 10(1):3000. doi: 10.1038/s41467-019-10979-3
184. Mussai F, Egan S, Hunter S, Webber H, Fisher J, Wheat R, et al. Neuroblastoma arginase activity creates an immunosuppressive microenvironment that impairs autologous and engineered immunity. Cancer Res (2015) 75(15):3043–53. doi: 10.1158/0008-5472.Can-14-3443
185. Arlauckas SP, Garren SB, Garris CS, Kohler RH, Oh J, Pittet MJ, et al. Arg1 expression defines immunosuppressive subsets of tumor-associated macrophages. Theranostics (2018) 8(21):5842–54. doi: 10.7150/thno.26888
186. Geiger R, Rieckmann JC, Wolf T, Basso C, Feng YH, Fuhrer T, et al. L-Arginine modulates T cell metabolism and enhances survival and anti-tumor activity. Cell (2016) 167(3):829–42.e13. doi: 10.1016/j.cell.2016.09.031
187. Werner A, Amann E, Schnitzius V, Habermeier A, Luckner-Minden C, Leuchtner N, et al. Induced arginine transport via cationic amino acid transporter-1 is necessary for human T-cell proliferation. Eur J Immunol (2016) 46(1):92–103. doi: 10.1002/eji.201546047
188. Wagner A, Wang C, Fessler J, DeTomaso D, Avila-Pacheco J, Kaminski J, et al. Metabolic modeling of single Th17 cells reveals regulators of autoimmunity. Cell (2021) 184(16):4168–85.e21. doi: 10.1016/j.cell.2021.05.045
189. Yeramian AE, Martin L, Serrat N, Arpa L, Soler C, Bertran J, et al. Arginine transport via cationic amino acid transporter 2 plays a critical regulatory role in classical or alternative activation of macrophages1. J Immunol (2006) 176(10):5918–24. doi: 10.4049/jimmunol.176.10.5918
190. Ji LL, Zhao XB, Zhang B, Kang L, Song WX, Zhao BH, et al. Slc6a8-mediated creatine uptake and accumulation reprogram macrophage polarization via regulating cytokine responses. Immunity (2019) 51(2):272–84.e7. doi: 10.1016/j.immuni.2019.06.007
191. Young JD, Yao SY, Baldwin JM, Cass CE, Baldwin SA. The human concentrative and equilibrative nucleoside transporter families, SLC28 and SLC29. Mol Aspects Med (2013) 34(2-3):529–47. doi: 10.1016/j.mam.2012.05.007
192. Koltai T, Reshkin SJ, Carvalho TMA, Di Molfetta D, Greco MR, Alfarouk KO, et al. Resistance to gemcitabine in pancreatic ductal adenocarcinoma: A physiopathologic and pharmacologic review. Cancers (Basel) (2022) 14(10):2486. doi: 10.3390/cancers14102486
193. Koikawa K, Kibe S, Suizu F, Sekino N, Kim N, Manz TD, et al. Targeting pin1 renders pancreatic cancer eradicable by synergizing with immunochemotherapy. Cell (2021) 184(18):4753–71.e27. doi: 10.1016/j.cell.2021.07.020
194. Molina-Arcas M, Bellosillo B, Casado FJ, Montserrat E, Gil J, Colomer D, et al. Fludarabine uptake mechanisms in B-cell chronic lymphocytic leukemia. Blood (2003) 101(6):2328–34. doi: 10.1182/blood-2002-07-2236
195. Skrypek N, Duchene B, Hebbar M, Leteurtre E, van Seuningen I, Jonckheere N. The MUC4 mucin mediates gemcitabine resistance of human pancreatic cancer cells via the concentrative nucleoside transporter family. Oncogene (2013) 32(13):1714–23. doi: 10.1038/onc.2012.179
196. Ye C, Han K, Lei J, Zeng K, Zeng S, Ju H, et al. Inhibition of histone deacetylase 7 reverses concentrative nucleoside transporter 2 repression in colorectal cancer by up-regulating histone acetylation state. Br J Pharmacol (2018) 175(22):4209–17. doi: 10.1111/bph.14467
197. Ritchie C, Cordova AF, Hess GT, Bassik MC, Li LY. SLC19A1 is an importer of the immunotransmitter cGAMP. Mol Cell (2019) 75(2):372–81. doi: 10.1016/j.molcel.2019.05.006
198. Casanello P, Torres A, Sanhueza F, Gonzalez M, Farias M, Gallardo V, et al. Equilibrative nucleoside transporter 1 expression is downregulated by hypoxia in human umbilical vein endothelium. Circ Res (2005) 97(1):16–24. doi: 10.1161/01.RES.0000172568.49367.f8
199. Eltzschig HK, Abdulla P, Hoffman E, Hamilton KE, Daniels D, Schonfeld C, et al. HIF-1-dependent repression of equilibrative nucleoside transporter (ENT) in hypoxia. J Exp Med (2005) 202(11):1493–505. doi: 10.1084/jem.20050177
200. Kobayashi S, Zimmermann H, Millhorn DE. Chronic hypoxia enhances adenosine release in rat PC12 cells by altering adenosine metabolism and membrane transport. J Neurochem (2000) 74(2):621–32. doi: 10.1046/j.1471-4159.2000.740621.x
201. Fu Z, Mowday AM, Smaill JB, Hermans IF, Patterson AV. Tumour hypoxia-mediated immunosuppression: mechanisms and therapeutic approaches to improve cancer immunotherapy. Cells (2021) 10(5):1006. doi: 10.3390/cells10051006
202. Huang YS, Tseng WY, Clanchy FIL, Topping LM, Ogbechi J, McNamee K, et al. Pharmacological modulation of T cell immunity results in long-term remission of autoimmune arthritis. Proc Natl Acad Sci U.S.A. (2021) 118(19):e2100939118. doi: 10.1073/pnas.2100939118
203. Soler C, Valdes R, Garcia-Manteiga J, Xaus J, Comalada M, Casado FJ, et al. Lipopolysaccharide-Induced Apoptosis of Macrophages Determines the up-Regulation of Concentrative Nucleoside Transporters Cnt1 and Cnt2 through Tumor Necrosis Factor-Alpha-Dependent and -Independent Mechanisms. J Biol Chem (2001) 276(32):30043–9. doi: 10.1074/jbc.M101807200
204. Soler C, García-Manteiga J, Valdés R, Xaus J, Comalada M, Casado FJ, et al. Macrophages require different nucleoside transport systems for proliferation and activation. FASEB J (2001) 15(11):1979–88. doi: 10.1096/fj.01-0022com
205. Kuda O, Pietka TA, Demianova Z, Kudova E, Cvacka J, Kopecky J, et al. Sulfo-N-succinimidyl oleate (SSO) inhibits fatty acid uptake and signaling for intracellular calcium via binding CD36 lysine 164. J Biol Chem (2013) 288(22):15547–55. doi: 10.1074/jbc.M113.473298
206. Pascual G, Avgustinova A, Mejetta S, Martin M, Castellanos A, Attolini CSO, et al. Targeting metastasis-initiating cells through the fatty acid receptor CD36. Nature (2017) 541(7635):41–5. doi: 10.1038/nature20791
207. Black PN, Ahowesso C, Montefusco D, Saini N, DiRusso CC. Fatty acid transport proteins: targeting FATP2 as a gatekeeper involved in the transport of exogenous fatty acids. Medchemcomm (2016) 7(4):612–22. doi: 10.1039/c6md00043f
208. Zhang M, Di Martino JS, Bowman RL, Campbell NR, Baksh SC, Simon-Vermot T, et al. Adipocyte-derived lipids mediate melanoma progression via FATP proteins. Cancer Discovery (2018) 8(8):1006–25. doi: 10.1158/2159-8290.Cd-17-1371
209. Gharpure KM, Pradeep S, Sans M, Rupaimoole R, Ivan C, Wu SY, et al. FABP4 as a key determinant of metastatic potential of ovarian cancer. Nat Commun (2018) 9(1):2923. doi: 10.1038/s41467-018-04987-y
210. Nie J, Zhang J, Wang L, Lu L, Yuan Q, An F, et al. Adipocytes promote cholangiocarcinoma metastasis through fatty acid binding protein 4. J Exp Clin Cancer Res (2017) 36(1):183. doi: 10.1186/s13046-017-0641-y
211. Huang M, Narita S, Inoue T, Koizumi A, Saito M, Tsuruta H, et al. Fatty acid binding protein 4 enhances prostate cancer progression by upregulating matrix metalloproteinases and stromal cell cytokine production. Oncotarget (2017) 8(67):111780–94. doi: 10.18632/oncotarget.22908
212. Al-Jameel W, Gou X, Forootan SS, Al Fayi MS, Rudland PS, Forootan FS, et al. Inhibitor SBFI26 suppresses the malignant progression of castration-resistant PC3-M cells by competitively binding to oncogenic FABP5. Oncotarget (2017) 8(19):31041–56. doi: 10.18632/oncotarget.16055
213. Rao E, Singh P, Zhai X, Li Y, Zhu G, Zhang Y, et al. Inhibition of tumor growth by a newly-identified activator for epidermal fatty acid binding protein. Oncotarget (2015) 6(10):7815–27. doi: 10.18632/oncotarget.3485
214. Lee BH, Taylor MG, Robinet P, Smith JD, Schweitzer J, Sehayek E, et al. Dysregulation of cholesterol homeostasis in human prostate cancer through loss of ABCA1. Cancer Res (2013) 73(3):1211–8. doi: 10.1158/0008-5472.Can-12-3128
215. Guo DL, Reinitz F, Youssef M, Hong C, Nathanson D, Akhavan D, et al. An LXR agonist promotes glioblastoma cell death through inhibition of an EGFR/AKT/SREBP-1/LDLR-dependent pathway. Cancer Discovery (2011) 1(5):442–56. doi: 10.1158/2159-8290.Cd-11-0102
216. Viaud M, Abdel-Wahab O, Gall J, Ivanov S, Guinamard R, Sore S, et al. ABCA1 exerts tumor-suppressor function in myeloproliferative neoplasms. Cell Rep (2020) 30(10):3397–410. doi: 10.1016/j.celrep.2020.02.056
217. Moon SH, Huang CH, Houlihan SL, Regunath K, Freed-Pastor WA, Morris JPT, et al. P53 represses the mevalonate pathway to mediate tumor suppression. Cell (2019) 176(3):564–80.e19. doi: 10.1016/j.cell.2018.11.011
218. Lin C-Y, Gustafsson J-Å. Targeting liver X receptors in cancer therapeutics. Nat Rev Cancer (2015) 15(4):216–24. doi: 10.1038/nrc3912
219. Pencheva N, Buss Colin G, Posada J, Merghoub T, Tavazoie Sohail F. Broad-spectrum therapeutic suppression of metastatic melanoma through nuclear hormone receptor activation. Cell (2014) 156(5):986–1001. doi: 10.1016/j.cell.2014.01.038
220. Candelaria NR, Addanki S, Zheng J, Nguyen-Vu T, Karaboga H, Dey P, et al. Antiproliferative effects and mechanisms of liver X receptor ligands in pancreatic ductal adenocarcinoma cells. PloS One (2014) 9(9):e106289. doi: 10.1371/journal.pone.0106289
221. Chan DA, Sutphin PD, Nguyen P, Turcotte S, Lai EW, Banh A, et al. Targeting GLUT1 and the warburg effect in renal cell carcinoma by chemical synthetic lethality. Sci Transl Med (2011) 3(94):94ra70. doi: 10.1126/scitranslmed.3002394
222. Liu Y, Cao YY, Zhang WH, Bergmeier S, Qian YR, Akbar H, et al. A small-molecule inhibitor of glucose transporter 1 downregulates glycolysis, induces cell-cycle arrest, and inhibits cancer cell growth in vitro and in vivo. Mol Cancer Ther (2012) 11(8):1672–82. doi: 10.1158/1535-7163.Mct-12-0131
223. Siebeneicher H, Cleve A, Rehwinkel H, Neuhaus R, Heisler I, Muller T, et al. Identification and optimization of the first highly selective GLUT1 inhibitor BAY-876. ChemMedChem (2016) 11(20):2261–71. doi: 10.1002/cmdc.201600276
224. Wu Q, ba-alawi W, Deblois G, Cruickshank J, Duan SL, Lima-Fernandes E, et al. GLUT1 inhibition blocks growth of RB1-positive triple negative breast cancer. Nat Commun (2020) 11(1):4205. doi: 10.1038/s41467-020-18020-8
225. Wang XJ, He HC, Rui WB, Zhang N, Zhu Y, Xie X. TRIM38 triggers the uniquitination and degradation of glucose transporter type 1 (GLUT1) to restrict tumor progression in bladder cancer. J Transl Med (2021) 19(1):508. doi: 10.1186/s12967-021-03173-x
226. Ma YB, Wang W, Idowu MO, Oh U, Wang XY, Temkin SM, et al. Ovarian cancer relies on glucose transporter 1 to fuel glycolysis and growth: anti-tumor activity of BAY-876. Cancers (Basel) (2019) 11(1):33. doi: 10.3390/cancers11010033
227. Shin E, Koo JS. Glucose metabolism and glucose transporters in breast cancer. Front Cell Dev Biol (2021) 9:728759. doi: 10.3389/fcell.2021.728759
228. Young CD, Lewis AS, Rudolph MC, Ruehle MD, Jackman MR, Yun UJ, et al. Modulation of glucose transporter 1 (GLUT1) expression levels alters mouse mammary tumor cell growth in vitro and in vivo. PloS One (2011) 6(8):e23205. doi: 10.1371/journal.pone.0023205
229. Wang YD, Li SJ, Liao JX. Inhibition of glucose transporter 1 (GLUT1) chemosensitized head and neck cancer cells to cisplatin. Technol Cancer Res Treat (2013) 12(6):525–35. doi: 10.7785/tcrt.2012.500343
230. Reckzeh ES, Karageorgis G, Schwalfenberg M, Ceballos J, Nowacki J, Stroet MCM, et al. Inhibition of glucose transporters and glutaminase synergistically impairs tumor cell growth. Cell Chem Biol (2019) 26(9):1214–28.e25. doi: 10.1016/j.chembiol.2019.06.005
231. Temre MK, Yadav S, Goel Y, Pandey SK, Kumar A, Singh SM. Glutor, a glucose transporter inhibitor, exerts antineoplastic action on tumor cells of thymic origin: implication of modulated metabolism, survival, oxidative stress, mitochondrial membrane potential, ph homeostasis, and chemosensitivity. Front Oncol (2022) 12:925666. doi: 10.3389/fonc.2022.925666
232. Choi SYC, Xue H, Wu R, Fazli L, Lin D, Collins CC, et al. The MCT4 gene: A novel, potential target for therapy of advanced prostate cancer. Clin Cancer Res (2016) 22(11):2721–33. doi: 10.1158/1078-0432.Ccr-15-1624
233. Polanski R, Hodgkinson CL, Fusi A, Nonaka D, Priest L, Kelly P, et al. Activity of the monocarboxylate transporter 1 inhibitor AZD3965 in small cell lung cancer. Clin Cancer Res (2014) 20(4):926–37. doi: 10.1158/1078-0432.Ccr-13-2270
234. Huang T, Feng Q, Wang Z, Li W, Sun Z, Wilhelm J, et al. Tumor-targeted inhibition of monocarboxylate transporter 1 improves T-cell immunotherapy of solid tumors. Adv Healthc Mater (2021) 10(4):e2000549. doi: 10.1002/adhm.202000549
235. Benjamin D, Robay D, Hindupur SK, Pohlmann J, Colombi M, El-Shemerly MY, et al. Dual inhibition of the lactate transporters MCT1 and MCT4 is synthetic lethal with metformin due to NAD(+) depletion in cancer cells. Cell Rep (2018) 25(11):3047–58.e4. doi: 10.1016/j.celrep.2018.11.043
236. Hayes GM, Chinn L, Cantor JM, Cairns B, Levashova Z, Tran H, et al. Antitumor activity of an anti-CD98 antibody. Int J Cancer (2015) 137(3):710–20. doi: 10.1002/ijc.29415
237. Yu D, Liu Y, Zhou YQ, Ruiz-Rodado V, Larion M, Xu GW, et al. Triptolide suppresses IDH1-mutated malignancy via Nrf2-driven glutathione metabolism. Proc Natl Acad Sci U.S.A. (2020) 117(18):9964–72. doi: 10.1073/pnas.1913633117
238. Liu N, Zhang JL, Yin MZ, Liu H, Zhang X, Li JD, et al. Inhibition of XCT suppresses the efficacy of anti-PD-1/L1 melanoma treatment through exosomal PD-L1-induced macrophage M2 polarization. Mol Ther (2021) 29(7):2321–34. doi: 10.1016/j.ymthe.2021.03.013
239. Chiu M, Sabino C, Taurino G, Bianchi MG, Andreoli R, Giuliani N, et al. GPNA inhibits the sodium-independent transport system L for neutral amino acids. Amino Acids (2017) 49(8):1365–72. doi: 10.1007/s00726-017-2436-z
240. Schulte ML, Fu A, Zhao P, Li J, Geng L, Smith ST, et al. Pharmacological blockade of ASCT2-dependent glutamine transport leads to antitumor efficacy in preclinical models. Nat Med (2018) 24(2):194–202. doi: 10.1038/nm.4464
241. Broer A, Fairweather S, Broer S. Disruption of amino acid homeostasis by novel ASCT2 inhibitors involves multiple targets. Front Pharmacol (2018) 9:785. doi: 10.3389/fphar.2018.00785
242. Suzuki M, Toki H, Furuya A, Ando H. Establishment of monoclonal antibodies against cell surface domains of ASCT2/SLC1A5 and their inhibition of glutamine-dependent tumor cell growth. Biochem Biophys Res Commun (2017) 482(4):651–7. doi: 10.1016/j.bbrc.2016.11.089
243. Kasai N, Sasakawa A, Hosomi K, Poh TW, Chua BL, Yong WP, et al. Anti-tumor efficacy evaluation of a novel monoclonal antibody targeting neutral amino acid transporter ASCT2 using patient-derived xenograft mouse models of gastric cancer. Am J Transl Res (2017) 9(7):3399–410.
244. Ueda S, Hayashi H, Miyamoto T, Abe S, Hirai K, Matsukura K, et al. Anti-tumor effects of MAb against L-type amino acid transporter 1 (LAT1) bound to human and monkey LAT1 with dual avidity modes. Cancer Sci (2019) 110(2):674–85. doi: 10.1111/cas.13908
245. Okano N, Naruge D, Kawai K, Kobayashi T, Nagashima F, Endou H, et al. First-in-human phase I study of JPH203, an L-type amino acid transporter 1 inhibitor, in patients with advanced solid tumors. Invest New Drugs (2020) 38(5):1495–506. doi: 10.1007/s10637-020-00924-3
246. Patsoukis N, Bardhan K, Chatterjee P, Sari D, Liu B, Bell LN, et al. PD-1 alters T-cell metabolic reprogramming by inhibiting glycolysis and promoting lipolysis and fatty acid oxidation. Nat Commun (2015) 6:6692. doi: 10.1038/ncomms7692
247. Karbanova S, Sorf A, Jiraskova L, Lalinska A, Ptackova Z, Staud F, et al. S-(4-Nitrobenzyl)-6-thioinosine (NBMPR) is not a selective inhibitor of equilibrative nucleoside transporters but also blocks efflux activity of breast cancer resistance protein. Pharm Res (2020) 37(3):58. doi: 10.1007/s11095-020-2782-5
248. Armstrong D, Summers C, Ewart L, Nylander S, Sidaway JE, van Giezen JJJ. Characterization of the adenosine pharmacology of ticagrelor reveals therapeutically relevant inhibition of equilibrative nucleoside transporter 1. J Cardiovasc Pharmacol Ther (2014) 19(2):209–19. doi: 10.1177/1074248413511693
249. Aungraheeta R, Conibear A, Butler M, Kelly E, Nylander S, Mumford A, et al. Inverse agonism at the P2Y(12) receptor and ENT1 transporter blockade contribute to platelet inhibition by ticagrelor. Blood (2016) 128(23):2717–28. doi: 10.1182/blood-2016-03-707844
250. Guo ZF, Hong SY, Wang JX, Rehan S, Liu WK, Peng HJ, et al. Rapamycin-inspired macrocycles with new target specificity. Nat Chem (2019) 11(3):254–63. doi: 10.1038/s41557-018-0187-4
251. Damaraju VL, Smith KM, Mowles D, Nowak I, Karpinski E, Young JD, et al. Interaction of fused-pyrimidine nucleoside analogs with human concentrative nucleoside transporters: high-affinity inhibitors of human concentrative nucleoside transporter 1. Biochem Pharmacol (2011) 81(1):82–90. doi: 10.1016/j.bcp.2010.09.009
252. Zhang P, Pei S, Gong Z, Ren Q, Xie J, Liu H, et al. The integrated single-cell analysis developed a lactate metabolism-driven signature to improve outcomes and immunotherapy in lung adenocarcinoma. Front Endocrinol (Lausanne) (2023) 14:1154410. doi: 10.3389/fendo.2023.1154410
253. Zhang P, Pei S, Gong Z, Feng Y, Zhang X, Yang F, et al. By integrating single-cell RNA-seq and bulk RNA-seq in sphingolipid metabolism, CACYBP was identified as a potential therapeutic target in lung adenocarcinoma. Front Immunol (2023) 14:1115272. doi: 10.3389/fimmu.2023.1115272
Keywords: tumor immune evasion, transporters, metabolites, the tumor microenvironment, cancer therapy
Citation: Chen L, Wang Y, Hu Q, Liu Y, Qi X, Tang Z, Hu H, Lin N, Zeng S and Yu L (2023) Unveiling tumor immune evasion mechanisms: abnormal expression of transporters on immune cells in the tumor microenvironment. Front. Immunol. 14:1225948. doi: 10.3389/fimmu.2023.1225948
Received: 20 May 2023; Accepted: 06 July 2023;
Published: 21 July 2023.
Edited by:
Peter J. Siska, University Medical Center Regensburg, GermanyReviewed by:
Yosra Bouraoui, Jendouba University, TunisiaPengpeng Zhang, Tianjin Medical University, China
Copyright © 2023 Chen, Wang, Hu, Liu, Qi, Tang, Hu, Lin, Zeng and Yu. This is an open-access article distributed under the terms of the Creative Commons Attribution License (CC BY). The use, distribution or reproduction in other forums is permitted, provided the original author(s) and the copyright owner(s) are credited and that the original publication in this journal is cited, in accordance with accepted academic practice. No use, distribution or reproduction is permitted which does not comply with these terms.
*Correspondence: Lushan Yu, eXVsc0B6anUuZWR1LmNu
†These authors have contributed equally to this work and share first authorship