- 1Department of Ecology and Evolutionary Biology, University of Tennessee, Knoxville, Knoxville, TN, United States
- 2Smithsonian Tropical Research Institute, Balboa, Ancón, Panama
Animals often mount complex immune responses to infections. Aside from cellular and molecular defense mechanisms, animals can alter their behavior in response to infection by avoiding, resisting, or tolerating negative effects of pathogens. These behaviors are often connected to cellular and molecular immune responses. For instance, sickness behaviors are a set of behavioral changes triggered by the host inflammatory response (e.g., cytokines) and could aid in resisting or tolerating infection, as well as affect transmission dynamics if sick animals socially withdraw or are being avoided by others. To fully understand the group and population level transmission dynamics and consequences of pathogen infections in bats, it is not only important to consider cellular and molecular defense mechanisms, but also behavioral mechanisms, and how both interact. Although there has been increasing interest in bat immune responses due to their ability to successfully cope with viral infections, few studies have explored behavioral anti-pathogen defense mechanisms. My main objective is to explore the interaction of cellular and molecular defense mechanisms, and behavioral alterations that results from infection in bats, and to outline current knowledge and future research avenues in this field.
Introduction
Animals often change their behavior in response to infection, which can have profound impacts on how individuals recover, or transmit pathogens (1–5). These behaviors can aid in avoiding, resisting, or tolerating negative fitness consequences of pathogenic infections and are often tightly connected to cellular and molecular immune responses such as inflammatory processes (2, 6, 7). For instance, sickness behaviors such as lethargy, anorexia or social withdrawal are triggered by a complex interaction of host inflammatory responses, neuroendocrinological mechanisms and the brain (1, 2, 4, 8, 9). Sickness behaviors could help the infected individual increase its tolerance to the infection (10) or resist the pathogen by diverting energetic resources to a costly immune response (5). These same behaviors can also affect uninfected conspecifics if infected individuals withdraw from social situations and, hence, are less likely to infect others (11–14). Uninfected conspecifics can also detect behavioral changes or other cues in their infected conspecifics and avoid them (3, 15).
Despite mounting interest in how bats harbor highly pathogenic viruses and what immunological mechanisms are involved in controlling viral pathogenesis (16–19), there are relatively few examples that explore behavioral anti-pathogen defense mechanisms and their connection to host immunology in bats (but see (11, 20)). Here, I will explore how infection and subsequent immune responses may lead to behavioral defense mechanisms in bats and affect their social interactions. I will mainly focus on the behavioral component of this interaction, as in vitro and in vivo studies on cellular and molecular immune responses in bats have been reviewed rigorously elsewhere (see (16, 21)). While I will outline existing studies, I will also highlight gaps in our knowledge and behavioral mechanisms to be further explored (Figure 1). Overall, I aim to connect research studying cellular and molecular defense mechanisms in bats and research trying to tie these immune traits to behavioral alterations that might affect downstream transmission dynamics and host-pathogen co-evolution.
Behavioral changes as a response to infection
Upon infection with pathogens (or exposure to inflammatory triggers), the body initiates a cascade of innate responses, which lead to physiological changes in the infected animal and ultimately affect their behaviors (2, 4, 7). Conserved receptors on immune cells (pathogen recognition receptors, PRRs) recognize equally conserved pathogen-associated molecular patterns (PAMPs) and initiate inflammatory responses such as the secretion of pro-inflammatory cytokines, including Interleukin-1 beta (IL-1β), Interleukin-6 (IL-6), Tumor necrosis factor alpha (TNF-α), and Interferons such as Interferon gamma (INF-γ), among others (2, 7, 22).
Cytokine secretion not only helps the animal to reduce pathogen proliferation in early stages of the infection by protecting and preparing nearby cells, but also initiates the adaptive arm of the immune system (23, 24). Importantly, these early immune responses also change the behavior of the host. Sickness behaviors such as lethargy and social withdrawal among others, are a direct result of in increased circulation of proinflammatory cytokines acting in the periphery and in the brain (2, 4, 6–8, 22), and recent research has begun to identify specific neuronal populations that are likely involved in triggering and mediating sickness behaviors (25, 26).
While the adaptiveness of sickness behaviors is still debated (4, 27, 28), one way they could function to increase an individual’s resistance is by helping to clear the infection. For instance, sickness-induced lethargy could divert energy to metabolically costly responses such as fevers (4). Sickness behaviors could also increase tolerance of the host by promoting stress tolerance in tissues (10) or affect transmission to conspecifics if sick individual withdraw socially or move less (1, 12, 13, 27, 29).
Toll-like receptors that are crucial in the recognition mechanisms that mediate sickness behaviors are highly conserved and are present throughout the animal kingdom (23). While in many cases functional studies of the specific signaling pathways are still lacking in bats (Figure 2, (16)), there has been increased interest in identifying bat PRRs that are involved in the recognition of pathogens (especially viral pathogens, reviewed in (16)). For instance, in the black flying fox (Pteropus Alecto) a full set of TLRs (1–10) transcripts has been sequenced (30), and functional studies in different bat cell cultures confirm that sensing of a PAMP, double-stranded RNA (dsRNA) and complete viruses, is likely conserved between humans and bats (31–35). In vivo studies that use injections of PAMPs (e.g., Lipopolysaccharide, Poly I:C, Zymogen) elicit typical innate immune responses and physiological symptoms, such as increases in white blood cell counts, weight loss, fever, and increased oxidative stress in a wide range of bat species, with intriguing species differences that need to be further explored (36–46). This suggests that bat innate immune systems are recognizing PAMPs and mounting acute phase responses. Studies on behavioral changes that accompany this acute phase response and could lead to changes in social interactions, however, are less common and limited to only a few species. Both vampire bats (Desmodus rotundus) and Egyptian fruit bats (Rosettus aegyptiacus) express sickness behaviors such as lethargy and social withdrawal in response to injections with bacterial LPS (11, 20, 47–49). Similarly, rabies-infected vampire bats show lower levels of social interactions (50) and little brown bats (Myotis lucifugus) are less active when infected with Pseudogymnoascus destructans, the fungus that causes White-nose Syndrome (51). While such studies suggest that sickness behaviors can occur during immune responses in bats, further studies across a wider range of bat species are needed to evaluate how sickness behaviors are connected to inflammatory traits in bats (Figure 1).
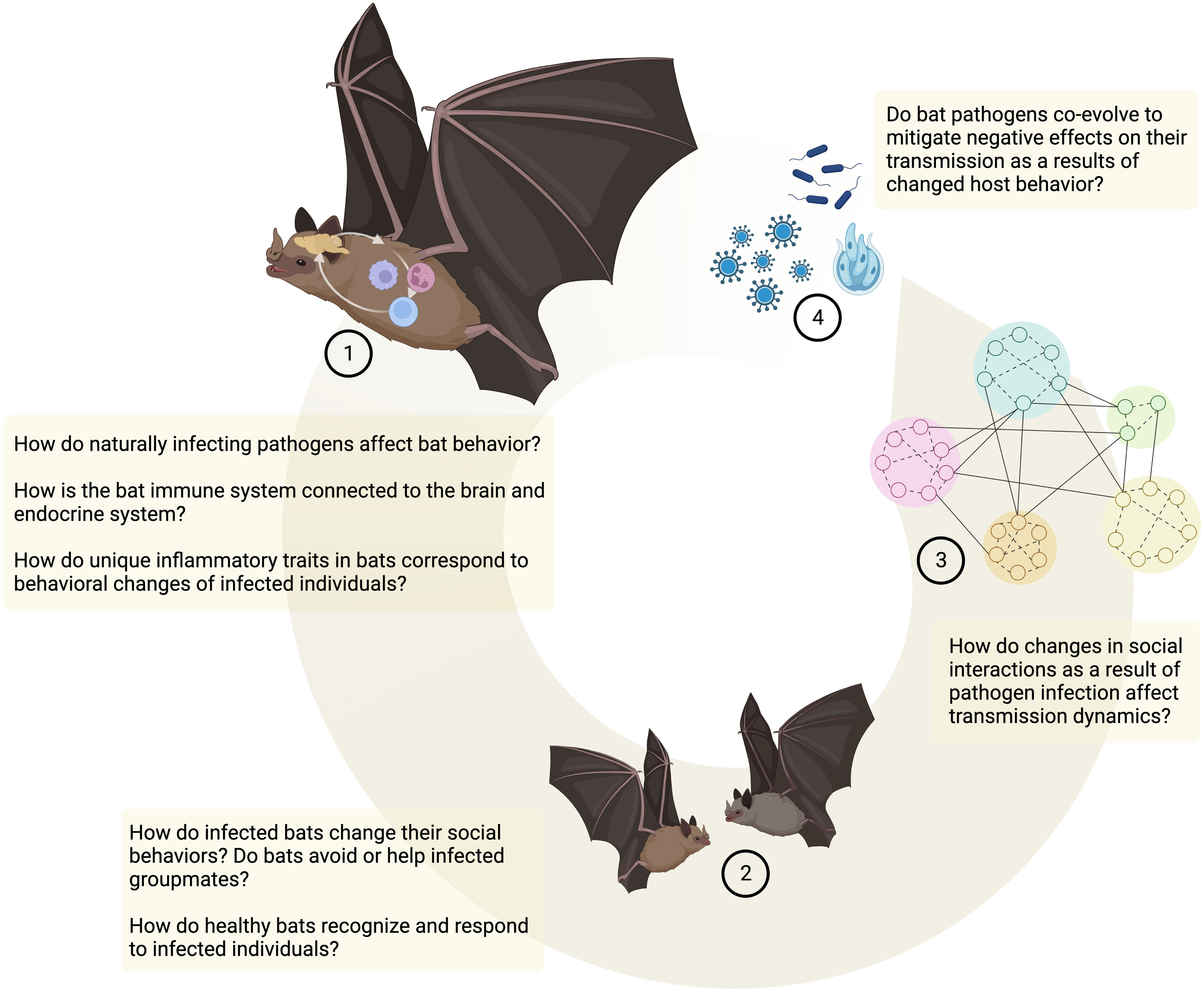
Figure 1 Behavioral anti-pathogen defenses in bats. Open questions and future directions. Created in BioRender.
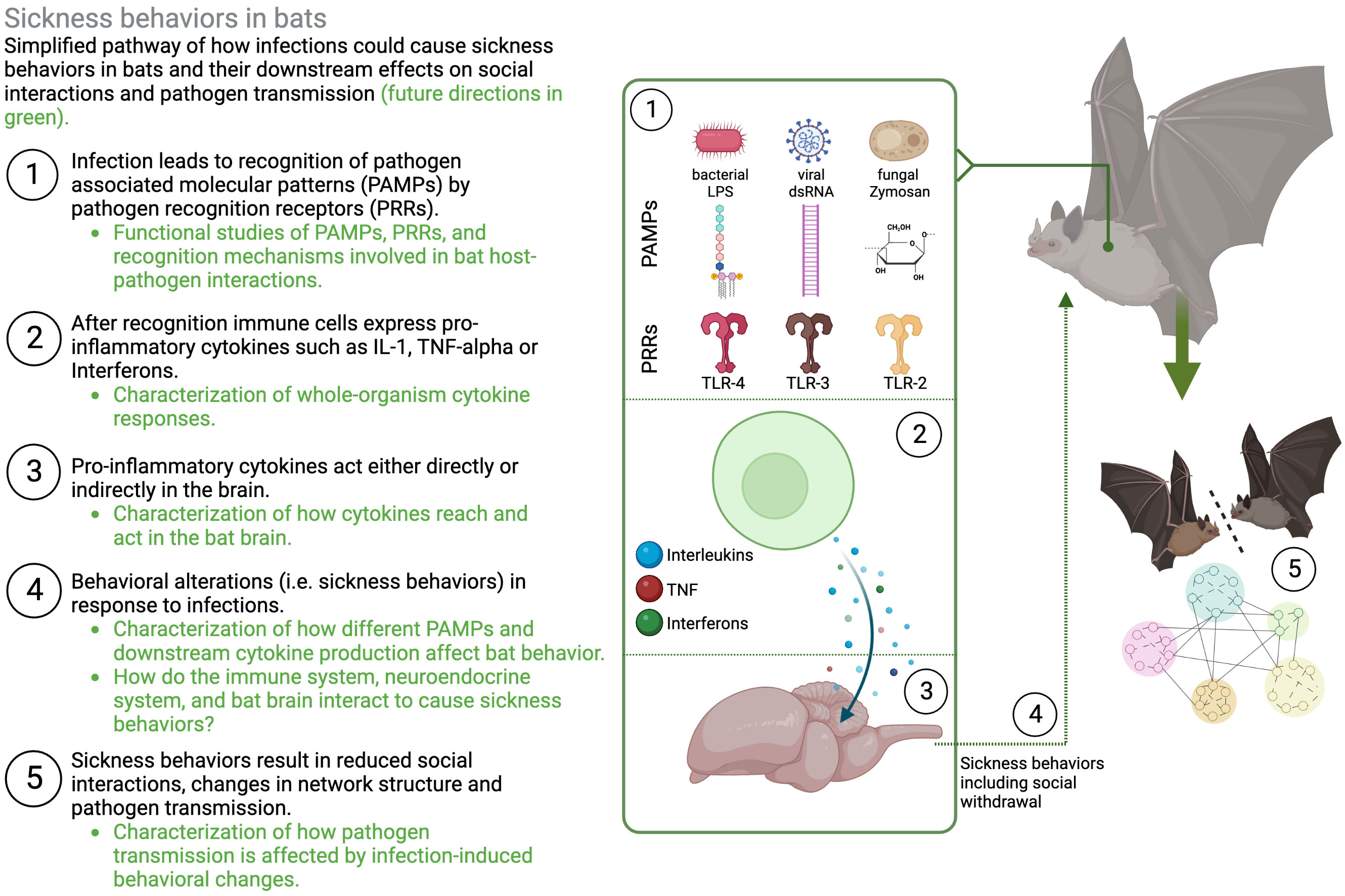
Figure 2 Schematic representation of sickness behaviors in bats. Illustrating the need to understand different layers of how molecular and cellular immune responses lead to changes in behavior and downstream transmission dynamics. Figure created in BioRender.
In recent years, bats have been identified as potential reservoirs for emerging viruses (52–54), which has sparked interest in what makes them immunologically unique in tolerating pathogens that are often lethal to other species (reviewed in (16–18)). Recent studies suggest that one mechanism by which bats tolerate otherwise highly pathogenic viruses is through a sophisticated regulation of the inflammatory response, which prevents damage to the host while also limiting viral propagation (18, 19, 55, 56). Bats that are experimentally infected with viruses often do not show any symptoms of illness (57–61), and both in vitro and in vivo studies suggest that inflammatory responses to viral triggers are often dampened (for detailed reviews of signaling pathways and immunological mechanisms, see (16, 21)). This raises the question of whether dampened inflammatory responses do or do not translate into behavioral symptoms.
Viral infections often result in behavioral changes in different taxa (2, 62). For example, in mice, systemic injection of Poly I:C (synthetic dsRNA, a viral mimetic) causes physiological symptoms such as elevated levels of IFN-γ and sickness behaviors (63). Similarly, TLR-3 deficient mice have attenuated sickness behaviors in response to influenza infections (64). These findings suggest that stimulation of TLR-3 and downstream cytokine expression in the periphery can change host behavior. But, what if the inflammatory response to viral antigens is uniquely regulated as described above? Do inhibited inflammatory responses found in bats translate into less severe behavioral symptoms? What are the downstream effects of inhibited inflammation and, potentially, reduced symptoms on social interactions and transmission in bats?
Physiological and behavioral changes that lead to infection recognition
Infection can not only change the behavior of hosts but can also cause visual, auditory, or olfactory cues that may be recognized by uninfected conspecifics. Such conspecific responses can range from conspecific avoidance (3, 65, 66) to aid provided to the sick conspecific (67), which can help them tolerate negative effects of an infection (1, 67, 68).
Before even having to engage in an immunological response, hosts could benefit from avoiding infected conspecifics (1, 3, 65, 66). Such conspecific avoidance is a widely observed behavior in vertebrates (e.g (69–73)), and offers a first line of defense. Infection itself can produce a variety of cues (olfactory (69, 72, 74); visual (75, 76); auditory (47, 77); and behavioral (2, 3)) that could lead to conspecific avoidance (3). Importantly, while avoidance of sick conspecifics has been shown in many different taxa, exact mechanisms of recognition are less well understood (but see (69)). Some studies in bats have examined conspecific responses to immune-challenged individuals (20, 47, 49). In these cases, reduced contact appeared to be primarily driven by the sick individual not engaging in social interactions (e.g., through lethargy, reduced movement, or social withdrawal) and less by conspecific avoidance. Importantly, these studies used immune-challenges as opposed to naturally infecting pathogens that might produce different infection cues (e.g., visible lesions, respiratory or neurological symptoms).
Some unanswered questions remain in bats: Do bats avoid their sick conspecifics? What are the cues and the mechanisms of recognition that mediate avoidance? What is the cost-benefit balance between conspecific avoidance and forgoing beneficial social interactions (e.g., see (49))? Given that bat species vary in which sensory system is predominantly used, does infection perception occur in the predominantly used sensory modality or in a single system (e.g., olfactory perception) regardless of which sensory modality is most frequently used in other aspects of the bats’ life?
Most bat species are social (78) and their propensity to live in social groups could (i) affect their immune-system and increase resistance, and/or (ii) help them mitigate negative effects of infection (i.e., increased tolerance), Research suggests that social integration as opposed to isolation or social adversity has a range of fitness benefits and can alter the regulation of the immune response (reviewed in (79)). For instance, experimental studies in primates suggest that increased social adversity leads to increased expression of genes linked to the inflammatory response (80, 81). While research aimed at understanding the interplay of social group living and immunity is gaining traction, studies in bats are lacking. How does living in social groups affect bat health positively or negatively, and how are immunological defense mechanisms affected by bat social interactions? Because bat species vary in their level of sociality (78), this could be addressed in comparative studies evaluating immune traits in relation to sociality. Another intriguing research avenue is “immune-priming” in response to perceived infection of conspecifics (82). This anticipatory response has been observed in several taxa (82–84). For instance, in humans, perception of disease related cues triggers the immune system to mount a stronger response (83). Due to their high levels of sociality, bats are presumably often exposed to infected conspecifics. How does their immune system respond to this exposure? Similarly, social interactions with infected others (e.g., food sharing or grooming) could lead to low-dose exposure, priming the immune system for subsequent encounters with the same pathogen.
Helping infected conspecifics can increase their tolerance by maintaining their fitness despite negative effects of infections (i.e., high pathogen load). Such help could manifest in the form of food provisions, temperature regulation, or even just in the maintenance of “normal” levels of social interactions that benefit the infected individual (i.e., some individuals might not choose to avoid their infected conspecifics). Theoretically, healthy individuals might help infected individuals tolerate infections if the individual costs are not too high (e.g., low virulence, (5, 68)) or if they indirectly benefit from helping specific individuals (e.g., socially bonded partners or kin (85)). Vampire bats will share food with immune-challenged conspecifics (49), though, here, immune-challenged bats were simultaneously fasted which makes it difficult to discern the exact cause of food sharing behaviors. The question remains if and under which social circumstances bats do not avoid, or even aid their infected conspecifics, and how this affects individual tolerance of pathogens and, potentially, supports recovery.
Group- and population-level effects of infection-induced behavioral changes
Whether individuals socially withdraw as a response to infection, avoid infected conspecifics, or increase contact with them to provide aid, will affect how pathogens spread through their social networks (1). In humans, behavioral symptoms as a results of influenza infections reduce the number of social contacts and predicted R0 (13). Similarly, immune-challenged mice move less, are less connected, and predicted pathogen transmission is therefore contained within few animals (12). While the behavioral effects of infection, or immune-challenges on individuals and their downstream effects on group characteristics and dynamics have been described in other species (12, 86, 87), this has rarely been done in bats (but see 48), especially with pathogens that infect them naturally. The question overall remains, how do sick bats and their groupmates behave, and how does this affect transmission to others? If sickness behaviors are connected to an inflammatory response, they can have downstream effects on how pathogens are transmitted illustrating the need to understand different layers of how inflammatory responses affect (i) the expression of sickness behaviors, (ii) interactions with others, and (ii) group of population-level transmission dynamics (Figure 2).
Many bats live in fission-fusion type social organizations or disperse, involving frequent movement of individuals to other roosts (78). How does reduced movement because of infections affect spread to other roosts and new individuals? New methods will allow for answers to some of these questions. For instance, the questions of how sick bats interact and move and how this could affect pathogen transmission to others might be answered using combinations of diagnostic methods to detect active infection in bats, and next-generation animal tracking methods such as GPS loggers and proximity sensors that quantify movement and host-host contacts (20, 48, 88).
Importantly, these infection-induced changes in behavior can also affect host-pathogen co-evolution if they affect transmission of the pathogen. Pathogens could evolve changes in virulence, pre- or asymptomatic periods, and reductions in infection-induced cues to avoid detection (1, 89, 90). The ability of viruses to mitigate sickness behaviors that might affect their transmission has recently been suggested in the case of SARS-CoV2, where a viral protein can help silence pain which could theoretically reduce sickness behaviors and increase transmission (91).
Concluding remarks
While the unique ability to deal with certain pathogens has triggered an interest in bat's inflammatory traits (16–19, 21), there has been far less interest in how these immune responses affect bat behavior even though behavioral alterations might affect pathogen transmission and host-pathogen co-evolution (1). Future research on immunological responses in live bats, and especially studies using experimental infections with bat-infecting pathogens, should include behavioral components to answer questions of how sick bats behave and how this affects their healthy conspecifics and groups. Behavioral observations could range from scoring of individual or social behaviors (11, 50) to more sophisticated methods such as proximity sensors used to quantify individual-individual contacts and network-wide effects on transmission (48, 92), or GPS tracking to quantify bat movement in relation to their infection, or immune status (20).
Here, I argue that to understand the group- and population-level transmission dynamics and consequences of pathogenic infections in bats, it is not only important to study their molecular and cellular defense mechanisms, but also behavioral defence mechanisms, and how they interact.
Author contributions
The author confirms being the sole contributor of this work and has approved it for publication.
Funding
Partial funding for open access to this research was provided by University of Tennessee’s Open Publishing Support Fund.
Acknowledgments
The author is thankful for comments from Patricia Lopes and Claire Hemingway.
Conflict of interest
The author declares that the research was conducted in the absence of any commercial or financial relationships that could be construed as a potential conflict of interest.
Publisher’s note
All claims expressed in this article are solely those of the authors and do not necessarily represent those of their affiliated organizations, or those of the publisher, the editors and the reviewers. Any product that may be evaluated in this article, or claim that may be made by its manufacturer, is not guaranteed or endorsed by the publisher.
References
1. Stockmaier S, Stroeymeyt N, Shattuck EC, Hawley DM, Ancel Meyers L, Bolnick DI. Infectious diseases and social distancing in nature. Science (2021) 6533:eabc8881. doi: 10.1126/science.abc8881
2. Lopes PC, French SS, Woodhams DC, Binning SA. Sickness behaviors across vertebrate taxa: proximate and ultimate mechanisms. J Exp Biol (2021) 224(9):jeb225847. doi: 10.1242/jeb.225847
3. Lopes PC, Woodhams DC, Binning SA. Infection avoidance behaviors across vertebrate taxa: patterns, processes and future directions. In: Animal behavior and parasitism. (Oxford, UK: Oxford University Press) (2022).
4. Hart BL. Biological basis of the behavior of sick animals. Neurosci Biobehav Rev (1988) 12(2):123–37. doi: 10.1016/s0149-7634(88)80004-6
5. Hart BL. Behavioral adaptations to pathogens and parasites: five strategies. Neurosci Biobehav Rev (1990) 14(3):273–94. doi: 10.1016/s0149-7634(05)80038-7
6. Shattuck EC, Muehlenbein MP. Human sickness behavior: Ultimate and proximate explanations. Am J Phys Anhropol (2015) 157(1):1–18. doi: 10.1002/ajpa.22698
7. Kelley KW, Bluthe RM, Dantzer R, Zhou JH, Shen WH, Johnson RW, et al. Cytokine-induced sickness behavior. Brain Behav Immun (2003) 1:S112–8. doi: 10.1016/s0889-1591(02)00077-6
8. Kelley KW, Kent S. The legacy of sickness behaviors. Front Psychiatry (2020) 11:607269. doi: 10.3389/fpsyt.2020.607269
9. Adelman JS, Martin LB. Vertebrate sickness behaviors: Adaptive and integrated neuroendocrine immune responses. Integr Comp Biol (2009) 49(3):202–14. doi: 10.1093/icb/icp028
10. Medzhitov R, Schneider DS, Soares MP. Disease tolerance as a defense strategy. Science (2012) 335(6071):936–41. doi: 10.1126/science.1214935
11. Stockmaier S, Bolnick DI, Page RA, Carter GG. An immune challenge reduces social grooming in vampire bats. Anim Behav (2018) 140:141–9. doi: 10.1016/j.anbehav.2018.04.021
12. Lopes PC, Block P, Konig B. Infection-induced behavioural changes reduce connectivity and the potential for disease spread in wild mice contact networks. Sci Rep (2016) 6:31790. doi: 10.1038/srep31790
13. Van Kerckhove K, Hens N, Edmunds WJ, Eames KTD. The impact of illness on social networks: implications for transmission and control of influenza. Am J Epidemiol (2013) 178(11):1655–62. doi: 10.1093/aje/kwt196
14. Bos N, Lefevre T, Jensen AB, d’Ettorre P. Sick ants become unsociable. J Evol Biol (2012) 25(2):342–51. doi: 10.1111/j.1420-9101.2011.02425.x
15. Zylberberg M, Klasing KC, Hahn TP. House finches (Carpodacus mexicanus) balance investment in behavioural and immunological defences against pathogens. Biol Lett (2013) 9(1):20120856. doi: 10.1098/rsbl.2012.0856
16. Banerjee A, Baker ML, Kulcsar K, Misra V, Plowright R, Mossman K. Novel insights into immune systems of bats. Front Immunol (2020) 11:26. doi: 10.3389/fimmu.2020.00026
17. Hayman DTS. Bat tolerance to viral infections. Nat Microbiol (2019) 4(5):728–9. doi: 10.1038/s41564-019-0430-9
18. Irving AT, Ahn M, Goh G, Anderson DE, Wang LF. Lessons from the host defences of bats, a unique viral reservoir. Nature (2021) 589(7842):363–70. doi: 10.1038/s41586-020-03128-0
19. Schountz T, Baker ML, Butler J, Munster V. Immunological control of viral infections in bats and the emergence of viruses highly pathogenic to humans. Front Immunol (2017) 8:1098. doi: 10.3389/fimmu.2017.01098
20. Moreno KR, Weinberg M, Harten L, Salinas Ramos VB, Herrera MLG, Czirják GÁ, et al. Sick bats stay home alone: fruit bats practice social distancing when faced with an immunological challenge. Ann N Y Acad Sci (2021) 1505(1):178–90. doi: 10.1111/nyas.14600
21. Gonzalez V, Banerjee A. Molecular, ecological, and behavioral drivers of the bat-virus relationship. iScience (2022) 25(8):104779. doi: 10.1016/j.isci.2022.104779
22. Tizard I. Sickness behavior, its mechanisms and significance. Anim Health Res Rev (2008) 9(1):87–99. doi: 10.1017/S1466252308001448
23. Medzhitov R. Toll-like receptors and innate immunity. Nat Rev Immunol (2001) 1(2):135–45. doi: 10.1038/35100529
24. Janeway CA, Medzhitov R. Innate immune recognition. Annu Rev Immunol (2002) 20(1):197–216. doi: 10.1146/annurev.immunol.20.083001.084359
25. Osterhout JA, Kapoor V, Eichhorn SW, Vaughn E, Moore JD, Liu D, et al. A preoptic neuronal population controls fever and appetite during sickness. Nature (2022) 606(7916):937–44. doi: 10.1038/s41586-022-04793-z
26. Ilanges A, Shiao R, Shaked J, Luo JD, Yu X, Friedman JM. Brainstem ADCYAP1+ neurons control multiple aspects of sickness behaviour. Nature (2022) 609(7928):761–71. doi: 10.1038/s41586-022-05161-7
27. Shakhar K, Shakhar G. Why do we feel sick when infected–can altruism play a role? PloS Biol (2015) 13(10):e1002276. doi: 10.1371/journal.pbio.1002276
28. Poulin R. “Adaptive” changes in the behaviour of parasitized animals: A critical review. Int J Parasitol (1995) 25(12):1371–83. doi: 10.1016/0020-7519(95)00100-x
29. Fairbanks BM, Hawley DM, Alexander KA. The impact of health status on dispersal behavior in Banded Mongooses (Mungos mungo). EcoHealth (2014) 11(2):258–62. doi: 10.1007/s10393-014-0912-4
30. Cowled C, Baker M, Tachedjian M, Zhou P, Bulach D, Wang LF. Molecular characterisation of Toll-like receptors in the black flying fox Pteropus alecto. Dev Comp Immunol (2011) 35(1):7–18. doi: 10.1016/j.dci.2010.07.006
31. Banerjee A, Rapin N, Miller M, Griebel P, Zhou Y, Munster V, et al. Generation and characterization of Eptesicus fuscus (Big brown bat) kidney cell lines immortalized using the Myotis polyomavirus large T-antigen. J Virol Methods (2016) 237:166–73. doi: 10.1016/j.jviromet.2016.09.008
32. Cowled C, Baker ML, Zhou P, Tachedjian M, Wang LF. Molecular characterisation of RIG-I-like helicases in the black flying fox. Pteropus alecto. Dev Comp Immunol (2012) 36(4):657–64. doi: 10.1016/j.dci.2011.11.008
33. Crameri G, Todd S, Grimley S, McEachern JA, Marsh GA, Smith C, et al. Establishment, immortalisation and characterisation of Pteropid bat cell lines. PloS One (2009) 4(12):e8266. doi: 10.1371/journal.pone.0008266
34. Omatsu T, Bak EJ, Ishii Y, Kyuwa S, Tohya Y, Akashi H, et al. Induction and sequencing of Rousette bat interferon α and β genes. Vet Immunol Immunopathol (2008) 124(1):169–76. doi: 10.1016/j.vetimm.2008.03.004
35. Banerjee A, Rapin N, Bollinger T, Misra V. Lack of inflammatory gene expression in bats: a unique role for a transcription repressor. Sci Rep (2017) 7(1):2232. doi: 10.1038/s41598-017-01513-w
36. Schneeberger K, Czirjak GA, Voigt CC. Inflammatory challenge increases measures of oxidative stress in a free-ranging, long-lived mammal. J Exp Biol (2013) 216(Pt 24):4514–9. doi: 10.1242/jeb.090837
37. Seltmann A, Troxell SA, SChad J, Fritze M, Bailey LD, Voigt CC, et al. Differences in acute phase response to bacterial, fungal and viral antigens in greater mouse-eared bats (Myotis myotis). Sci Rep (2022) 12:15259. doi: 10.1038/s41598-022-18240-6
38. Stockmaier S, Dechmann DK, Page RA, O’Mara MT. No fever and leucocytosis in response to a lipopolysaccharide challenge in an insectivorous bat. Biol Lett (2015) 11(9):20150576. doi: 10.1098/rsbl.2015.0576
39. Cabrera-Martinez LV, Herrera MLG, Cruz-Neto AP. The energetic cost of mounting an immune response for Pallas’s long-tongued bat (Glossophaga soricina). PeerJ (2018) 6:e4627. doi: 10.7717/peerj.4627
40. Melhado G, Herrera MLG, da Cruz-Neto AP. Bats respond to simulated bacterial infection during the active phase by reducing food intake. J Exp Zool A Ecol Integr Physiol (2020) 333(8):536–42. doi: 10.1002/jez.2399
41. Otálora-Ardila A, Herrera MLG, Flores-Martínez JJ. Metabolic cost of the activation of immune response in the Fish-Eating Myotis (Myotis vivesi): the effects of inflammation and the acute phase response. PloS One (2016) 11(10):e0164938–e0164938. doi: 10.1371/journal.pone.0164938
42. Otálora-Ardila A, Herrera MLG, Flores-Martínez JJ, Welch KC. The effect of short-term food restriction on the metabolic cost of the acute phase response in the fish-eating Myotis (Myotis vivesi). Mamm Biol (2017) 82:41–7. doi: 10.1016/j.mambio.2016.11.002
43. Voigt CC, Fritze M, Lindecke O, Costantini D, Pētersons G, Czirják GÁ.The immune response of bats differs between pre-migration and migration seasons. Sci Rep (2020) 10(1):17384doi: 10.1038/s41598-020-74473-3
44. Guerrero A, Rivera D, Díaz V, Triana C, Nino A. Metabolic cost of acute phase response in the frugivorous bat, Artibeus lituratus. Mamm Res (2018) 63:397–404. doi: 10.1007/s13364-018-0375-z
45. Weise P, Czirják GA, Lindecke O, Bumrungsri S, Voigt CC. Simulated bacterial infection disrupts the circadian fluctuation of immune cells in wrinkle-lipped bats (Chaerephon plicatus). PeerJ (2017) 5:e3570. doi: 10.7717/peerj.3570
46. Costantini D, Weinberg M, Jordán L, Moreno KR, Yovel Y, Czirják GÁ.Induced bacterial sickness causes inflammation but not blood oxidative stress in Egyptian fruit bats (Rousettus aegyptiacus). Conserv Physiol (2022) 10(1):coac028doi: 10.1093/conphys/coac028
47. Stockmaier S, Bolnick DI, Page RA, Josic D, Carter GG. Immune-challenged vampire bats produce fewer contact calls. Biol Lett (2020) 16(7):20200272. doi: 10.1098/rsbl.2020.0272
48. Ripperger SP, Stockmaier S, Carter GG. Tracking sickness effects on social encounters via continuous proximity sensing in wild vampire bats. Behav Ecol (2020) 31:araa111. doi: 10.1093/beheco/araa111
49. Stockmaier S, Bolnick DI, Page RA, Carter GG. Sickness effects on social interactions depend on the type of behaviour and relationship. J Anim Ecol (2020) 89(6):1387–94. doi: 10.1111/1365-2656.13193
50. Cárdenas-Canales EM, Stockmaier S, Cronin E, Rocke TE, Osorio JE, Carter GG. Social effects of rabies infection in male vampire bats (Desmodus rotundus). Biol Lett (2022) 18(9):20220298. doi: 10.1098/rsbl.2022.0298
51. Bohn SJ, Turner JM, Warnecke L, Mayo C, McGuire LP, Misra V, et al. Evidence of ‘sickness behaviour’ in bats with white-nose syndrome. Behaviour (2016) 153(8):981–1003. doi: 10.1163/1568539X-00003384
52. Li W, Shi Z, Yu M, Ren W, Smith C, Epstein JH, et al. Bats are natural reservoirs of SARS-like coronaviruses. Science (2005) 310(5748):676–9. doi: 10.1126/science.1118391
53. Field H, Young P, Yob JM, Mills J, Hall L, Mackenzie J. The natural history of Hendra and Nipah viruses. Microbes Infect (2001) 3(4):307–14. doi: 10.1016/s1286-4579(01)01384-3
54. Calisher CH, Childs JE, Field HE, Holmes KV, Schountz T. Bats: important reservoir hosts of emerging viruses. Clin Microbiol Rev (2006) 19(3):531–45. doi: 10.1128/CMR.00017-06
55. Ahn M, Anderson DE, Zhang Q, Tan CW, Lim BL, Luko K, et al. Dampened NLRP3-mediated inflammation in bats and implications for a special viral reservoir host. Nat Microbiol (2019) 4(5):789–99. doi: 10.1038/s41564-019-0371-3
56. Pavlovich SS, Lovett SP, Koroleva G, Guito JC, Arnold CE, Nagle ER, et al. The Egyptian Rousette genome reveals unexpected features of bat antiviral immunity. Cell (2018) 173(5):1098–1110.e18. doi: 10.1016/j.cell.2018.03.070
57. Paweska JT, Storm N, Grobbelaar AA, Markotter W, Kemp A, Jansen van Vuren P. Experimental inoculation of Egyptian fruit bats (Rousettus aEgyptiacus) with Ebola Virus. Viruses (2016) 8(2):29. doi: 10.3390/v8020029
58. Munster VJ, Adney DR, van Doremalen N, Brown VR, Miazgowicz KL, Milne-Price S, et al. Replication and shedding of MERS-CoV in Jamaican fruit bats (Artibeus jamaicensis). Sci Rep (2016) 6(1):21878. doi: 10.1038/srep21878
59. Middleton DJ, Morrissy CJ, van der Heide BM, Russell GM, Braun MA, Westbury HA, et al. Experimental Nipah virus infection in Pteropid bats (Pteropus poliocephalus). J Comp Pathol (2007) 136(4):266–72. doi: 10.1016/j.jcpa.2007.03.002
60. Halpin K, Hyatt AD, Fogarty R, Middleton D, Bingham J, Epstein JH, et al. Pteropid Bats are confirmed as the reservoir hosts of Henipaviruses: a comprehensive experimental study of virus transmission. Am J Trop Med Hyg (2011) 85(5):946–51. doi: 10.4269/ajtmh.2011.10-0567
61. Davis A, Bunning M, Gordy P, Panella N, Blitvich B, Bowen R. Experimental and natural infection of North American bats with West Nile virus. Am J Trop Med Hyg (2005) 73(2):467–9. doi: 10.4269/ajtmh.2005.73.467
62. Krapić M, Kavazović I, Wensveen FM. Immunological mechanisms of sickness behavior in viral infection. Viruses (2021) 13(11):2245. doi: 10.3390/v13112245
63. Cunningham C, Campion S, Teeling J, Felton L, Perry VH. The sickness behaviour and CNS inflammatory mediator profile induced by systemic challenge of mice with synthetic double-stranded RNA (poly I:C). Brain Behav Immun (2007) 21(4):490–502. doi: 10.1016/j.bbi.2006.12.007
64. Majde JA, Kapás L, Bohnet SG, De A, Krueger JM. Attenuation of the influenza virus sickness behavior in mice deficient in Toll-like receptor 3. Brain Behav Immun (2010) 24(2):306–15. doi: 10.1016/j.bbi.2009.10.011
65. Curtis VA. Infection-avoidance behaviour in humans and other animals. Trends Immunol (2014) 35(10):457–64. doi: 10.1016/j.it.2014.08.006
66. Gibson AK, Amoroso CR. Evolution and ecology of parasite avoidance. Annu Rev Ecol Evol Syst (2022) 53(1):47–67. doi: 10.1146/annurev-ecolsys-102220-020636
67. Loehle C. Social barriers to pathogen transmission in wild animal populations. Ecology (1995) 76(2):326–35. doi: 10.2307/1941192
68. Almberg ES, Cross PC, Dobson AP, Smith DW, Metz MC, Stahler DR, et al. Social living mitigates the costs of a chronic illness in a cooperative carnivore. Ecol Lett (2015) 18(7):660–7. doi: 10.1111/ele.12444
69. Boillat M, Challet L, Rossier D, Kan C, Carleton A, Rodriguez I. The vomeronasal system mediates sick conspecific avoidance. Curr Biol (2015) 25(2):251–5. doi: 10.1016/j.cub.2014.11.061
70. Behringer DC, Butler MJ, Shields JD. Ecology: avoidance of disease by social lobsters. Nature (2006) 441(7092):421. doi: 10.1038/441421a
71. Kiesecker JM, Skelly DK, Beard KH, Preisser E. Behavioral reduction of infection risk. Proc Natl Acad Sci USA (1999) 96(16):9165–8. doi: 10.1073/pnas.96.16.9165
72. Poirotte C, Massol F, Herbert A, Willaume E, Bomo PM, Kappeler PM, et al. Mandrills use olfaction to socially avoid parasitized conspecifics. Sci Adv (2017) 3(4):e1601721. doi: 10.1126/sciadv.1601721
73. Stephenson JF, Perkins SE, Cable J. Transmission risk predicts avoidance of infected conspecifics in Trinidadian guppies. J Anim Ecol (2018) 87(6):1525–33. doi: 10.1111/1365-2656.12885
74. Shirasu M, Touhara K. The scent of disease: volatile organic compounds of the human body related to disease and disorder. J Biochem (2011) 150(3):257–66. doi: 10.1093/jb/mvr090
75. Bouwman KM, Hawley DM. Sickness behaviour acting as an evolutionary trap? Male house finches preferentially feed near diseased conspecifics. Biol Lett (2010) 6(4):462–5. doi: 10.1098/rsbl.2010.0020
76. Kollias GV, Sydenstricker KV, Kollias HW, Ley DH, Hosseini PR, Connolly V, et al. Experimental infection of house finches with Mycoplasma gallisepticum. J Wildl Dis (2004) 40(1):79–86. doi: 10.7589/0090-3558-40.1.79
77. Lopes PC, König B. Choosing a healthy mate: sexually attractive traits as reliable indicators of current disease status in house mice. Anim Behav (2016) 111:119–26. doi: 10.1016/j.anbehav.2015.10.011
78. Kerth G. Causes and consequences of sociality in bats. BioSci (2008) 58(8):737–46. doi: 10.1641/B580810
79. Snyder-Mackler N, Burger JR, Gaydosh L, Belsky DW, Noppert GA, Campos FA, et al. Social determinants of health and survival in humans and other animals. Science (2020) 368(6493):eaax9553. doi: 10.1126/science.aax9553
80. Tung J, Barreiro LB, Johnson ZP, Hansen KD, Michopoulos V, Toufexis D, et al. Social environment is associated with gene regulatory variation in the rhesus macaque immune system. Proc Natl Acad Sci USA (2012) 109(17):6490–5. doi: 10.1073/pnas.1202734109
81. Snyder-Mackler N, Sanz J, Kohn JN, Brinkworth JF, Morrow S, Shaver AO, et al. Social status alters immune regulation and response to infection in macaques. Science (2016) 354(6315):1041–5. doi: 10.1126/science.aah3580
82. Lopes PC. Anticipating infection: how parasitism risk changes animal physiology. Fun Ecol (2023) 37:821–30. doi: 10.1111/1365-2435.14155
83. Schaller M, Miller GE, Gervais WM, Yager S, Chen E. Mere visual perception of other people’s disease symptoms facilitates a more aggressive immune response. Psychol Sci (2010) 21(5):649–52. doi: 10.1177/0956797610368064
84. Love AC, Grisham K, Krall JB, Goodchild CG, DuRant SE. Perception of infection: disease-related social cues influence immunity in songbirds. Biol Lett (2021) 17(6):20210125. doi: 10.1098/rsbl.2021.0125
85. Stockmaier S, Ulrich Y, Albery GF, Cremer S, Lopes PC. Behavioural defences against parasites across host social structures. Fun Ecol (2023) 37:809–20. doi: 10.1111/1365-2435.14310
86. Stroeymeyt N, Grasse AV, Crespi A, Mersch DP, Cremer S, Keller L. Social network plasticity decreases disease transmission in a eusocial insect. Science (2018) 362(6417):941. doi: 10.1126/science.aat4793
87. Alciatore G, Ugelvig LV, Frank E, Bidaux J, Gal A, Schmitt T, et al. Immune challenges increase network centrality in a queenless ant. Proc Biol Sci (2021) 288(1958):20211456. doi: 10.1098/rspb.2021.1456
88. O’Mara MT, Wikelski M, Dechmann DKN. 50 years of bat tracking: device attachment and future directions. Methods Ecol Evol (2014) 5(4):311–9. doi: 10.1111/2041-210X.12172
89. Hawley DM, Gibson AK, Townsend AK, Craft ME, Stephenson JF. Bidirectional interactions between host social behaviour and parasites arise through ecological and evolutionary processes. Parasitology (2020) 148(3):274–88. doi: 10.1017/S0031182020002048
90. Ezenwa VO, Archie EA, Craft ME, Hawley DM, Martin LB, Moore J, et al. Host behaviour–parasite feedback: an essential link between animal behaviour and disease ecology. Proc Biol Sci (2016) 283(1828):20153078. doi: 10.1098/rspb.2015.3078
91. Moutal A, Martin LF, Boinon L, Gomez K, Ran D, Zhou Y, et al. SARS-CoV-2 spike protein co-opts VEGF-A/neuropilin-1 receptor signaling to induce analgesia. Pain (2021) 162(1):243–52. doi: 10.1097/j.pain.0000000000002097
Keywords: sickness behavior, bats (Chiroptera), inflammatory response, avoidance, infection-induced behavior, bat immunology
Citation: Stockmaier S (2023) Bat behavioral immune responses in social contexts: current knowledge and future directions. Front. Immunol. 14:1232556. doi: 10.3389/fimmu.2023.1232556
Received: 31 May 2023; Accepted: 31 July 2023;
Published: 17 August 2023.
Edited by:
Hannah Frank, Tulane University, New Orleans, United StatesReviewed by:
Luis Gerardo Herrera M., Universidad Nacional Autónoma de México, MexicoCopyright © 2023 Stockmaier. This is an open-access article distributed under the terms of the Creative Commons Attribution License (CC BY). The use, distribution or reproduction in other forums is permitted, provided the original author(s) and the copyright owner(s) are credited and that the original publication in this journal is cited, in accordance with accepted academic practice. No use, distribution or reproduction is permitted which does not comply with these terms.
*Correspondence: Sebastian Stockmaier, c3N0b2NrbWFAdXRrLmVkdQ==