- 1Jilin Provincial Key Laboratory of Radiation Oncology & Therapy, The First Hospital of Jilin University, Changchun, China
- 2Department of Radiation Oncology, The First Hospital of Jilin University, Changchun, China
- 3National Health Commission (NHC) Key Laboratory of Radiobiology, School of Public Health of Jilin University, Changchun, China
Radiotherapy (RT) is an effective treatment option for cancer patients, which induces the production of reactive oxygen species (ROS) and causes oxidative stress (OS), leading to the death of tumor cells. OS not only causes apoptosis, autophagy and ferroptosis, but also affects tumor immune response. The combination of RT and immunotherapy has revolutionized the management of various cancers. In this process, OS caused by ROS plays a critical role. Specifically, RT-induced ROS can promote the release of tumor-associated antigens (TAAs), regulate the infiltration and differentiation of immune cells, manipulate the expression of immune checkpoints, and change the tumor immune microenvironment (TME). In this review, we briefly summarize several ways in which IR induces tumor cell death and discuss the interrelationship between RT-induced OS and antitumor immunity, with a focus on the interaction of ferroptosis with immunogenic death. We also summarize the potential mechanisms by which ROS regulates immune checkpoint expression, immune cells activity, and differentiation. In addition, we conclude the therapeutic opportunity improving radiotherapy in combination with immunotherapy by regulating OS, which may be beneficial for clinical treatment.
1 Introduction
Immunotherapy, a treatment that uses the immune system to eliminate cancer cells, has been touted as a potential cure for cancer in recent years (1). Immunotherapy eliminates cancer cells in the body by enhancing the recognition of tumor-associated antigens (TAAs) and specific killing effect of immune system (2). However, due to the heterogeneity of the tumor microenvironment (TME) and the immunosuppressive response of tumor cells, most patients do not benefit from the immunotherapy regimen.
Radiotherapy (RT) is a critical nonsurgical treatment for cancer. RT usually induces cell death by increasing the level of reactive oxygen species (ROS) in tumor cells (3). RT causes the production of a variety of ROS in tumor cells, which is one of the main ways of radiation-induced DNA damage and cell death (4). RT can directly induce DNA base damage. RT can also trigger ionization of water molecules, resulting in the production of large amounts of free radicals and ROS that damage DNA, lipids and proteins, leading to metabolic and functional changes and ultimately apoptosis (5). In addition, RT can favorably modulate immunological response, leading to increased tumor antigen presentation, priming of tumor-specific cytotoxic T cells, as well as enhanced T-cell homing, engraftment, and function in tumors (6).
Radiotherapy can enhance the effect of immunotherapy through a variety of mechanisms (Figure 1). Firstly, irradiation (IR) can increase the recognition of immune cells. IR damages DNA and proteins directly or indirectly through free radical production, which leads to an increase in neoantigens released by tumor cells for immune recognition. Tumor cells also release damage-associated molecular patterns (DAMPs) after IR, including high-mobility group box 1 (HMGB1), heat shock proteins (HSPs), and calreticulin (CRT), which mediate phagocytosis of antigen-presenting cells (APCs) and initiate tumor-specific T cell activation. In addition, T cell activation is mediated by the recognition and binding of major histocompatibility complex (MHC) molecules to which T cell receptor (TCR) bind peptides. IR can increase the expression of MHC-I in tumor cells, making it easier for cytotoxic T cells to recognize. Secondly, IR activates innate immune response and immune checkpoint upregulation. activation of the stimulator of interferon genes (STING) is an important part of innate immune response. The damaged DNA fragments after IR are released into the cytoplasm and recognized by cyclic GMP-AMP synthase (cGAS) to synthesize cyclic GMP-AMP (cGAMP), which induces the production of type I interferon (IFN) through the stimulation of STING-TBK1-IRF3 signal axis. Type I IFN regulates dendritic cells (DCs) function and helper T cell differentiation, mediating innate immune response. However, STING may also induce IR resistance and immunosuppression. On the one hand, STING can induce up-regulation of programmed cell death-ligand 1 (PD-L1) to promote immune escape. On the other hand, STING can promote IR and immune resistance of tumors through myeloid-derived suppressor cells (MDSCs) mobilization and indoleamine 2,3-dioxygenase (IDO) activation. IR-induced DNA damage can also directly up-regulate the expression of PD-L1 on tumor cells through ATM/ATR/Chk1 kinase, or indirectly regulate the expression of PD-L1 by increasing the secretion of IFN-γ. Thirdly, radiation-regulated immune microenvironments. IR can increase CD8+T cell infiltration and IFN-γ, promote the normalization of tumor vasculature and induce the polarization of M2-like macrophages towards M1 phenotype. However, IR can also promote the secretion of transforming growth factor β (TGF-β), inhibit CD8+T cells and increase the proportion of regulatory T cells (Tregs), leading to immunosuppression in tumors. To enhance the antitumor effect, the combination of RT and immunotherapy has become a new strategy for cancer care.
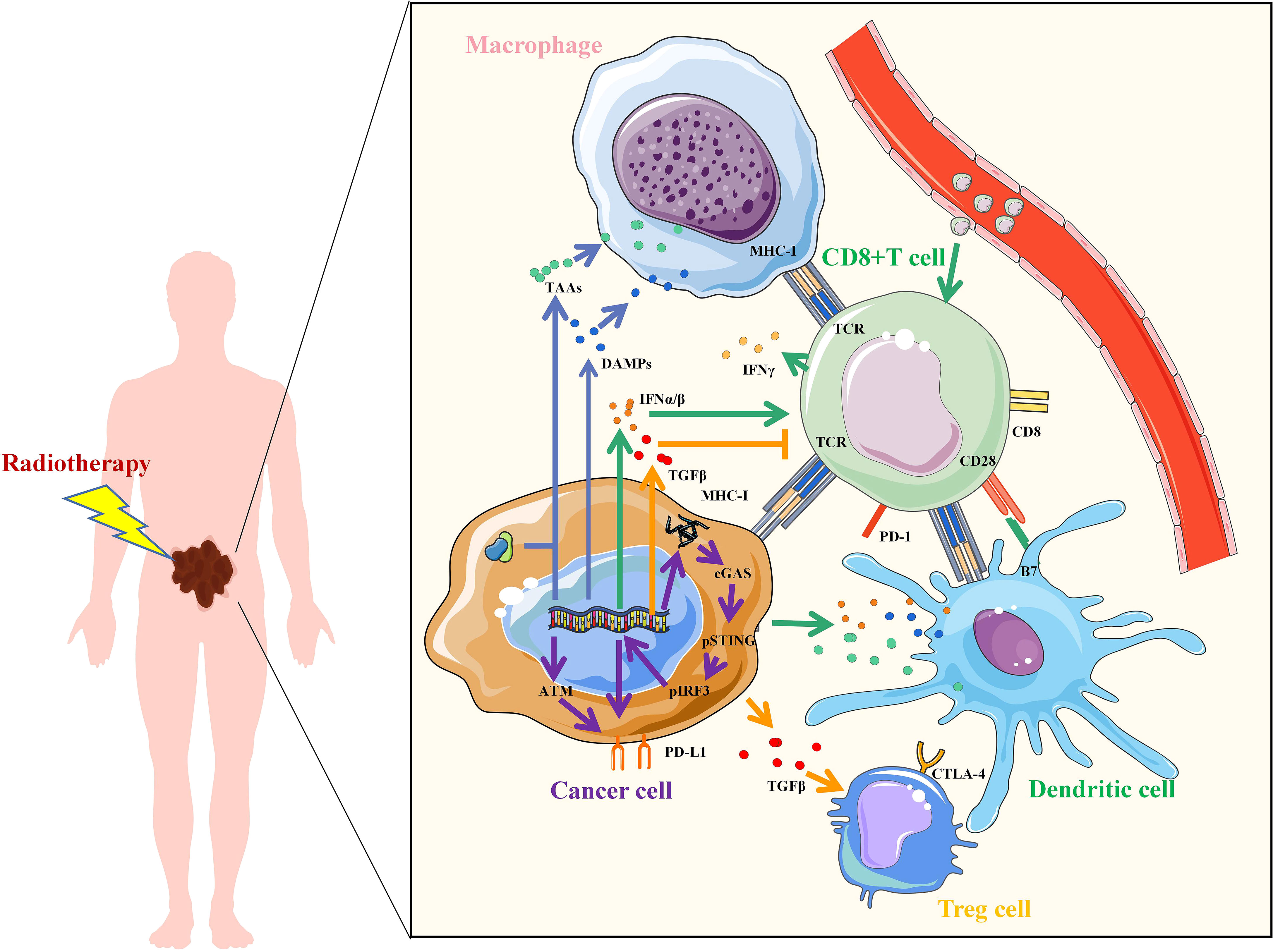
Figure 1 Mechanisms of radiotherapy to enhance immunotherapy. Irradiation increased the upregulation of tumor antigens, TAAs, tumor-associated antigens; DAMPs, damage-associated molecular patterns; IFN, interferon; TGF, transforming growth factor; MHC, major histocompatibility complex; TCR, T cell receptor; CD, Cluster of Differentiation; PD-L1, programmed cell death-ligand 1; PD-1, Programmed death 1; B7, CD80 and CD86; CTLA-4, cytotoxic T lymphocyte-associated antigen-4; cGAS, cyclic GMP-AMP synthase; STING, stimulator of interferon genes; IRF3, interferon regulatory factor 3; ATM, ataxia telangiectasia mutated protein.
Recent evidence suggests that ROS-induced oxidative stress (OS) can regulate multiple tumor immune responses. ROS plays a mediator role of pivotal functions such as phagocytosis, antigen presentation and recognition, cytolysis and phenotypical differentiation in immune cells (7). Moderate level of ROS contribute to activation and differentiation of T cells, whereas high level of ROS impair T cell survival and function (8). Tumor-associated macrophage (TAM), MDSCs and Tregs are the main immunosuppressive cells in TME (9). ROS is an important regulator of their immunosuppressive function. Increased ROS level in TME may promote the differentiation of TAM into M2 subtypes and regulate PD-L1 expression (10). Tregs are less susceptible to cell death induced by OS compared to other CD4+T cells and ROS may promote the expression of forkhead box protein 3 (FOXP3) in Tregs and maintain the immunosuppressive function (11, 12). ROS is essential for MDSCs in their undifferentiated state. The release of ROS molecules mediates the suppression of T cells and the activation of Tregs (9, 13). Production of several immunosuppressive cytokines is also regulated by ROS, such as IL-6, IL-10 and TGF-β, which can block the function of immune cells (14, 15). In addition, increased ROS can mediate the expression of immune checkpoints such as PD-L1 to promote tumor immune escape (16). Given the regulatory role of ROS in antitumor immunity, it is necessary to summarize the role of IR-induced OS in cancer immunotherapy.
In this review, we summarize several mechanisms by which RT induces ROS production and OS in tumors. The molecular mechanism underlying the interaction between OS and anti-tumor immunity is further discussed, with emphasis on the regulatory role of ROS on TME. On this basis, we attempted to find a treatment method for regulating OS during RT to enhance the efficacy of radiotherapy combined with immunotherapy.
2 IR-induced OS promotes tumor cell death
Increased ROS is a critical way to kill tumor cells exposed to RT. After exposure, water molecules are broken down to produce large amounts of ROS, including the superoxide anion, Hydroxyl agent and hydrogen peroxide, respectively (17). RT-induced ROS promotes the death of tumor cells mainly by damaging macromolecular substances such as DNA, lipids, and proteins in cells. When RT-induced ROS level exceed their own antioxidant level, tumor cells death will occur in a variety of ways, including apoptosis, autophagy, and ferroptosis and others. In addition, injured and dead tumor cells activate the anti-tumor immune mechanism and induce immunogenic death of tumor cells (Figure 2).
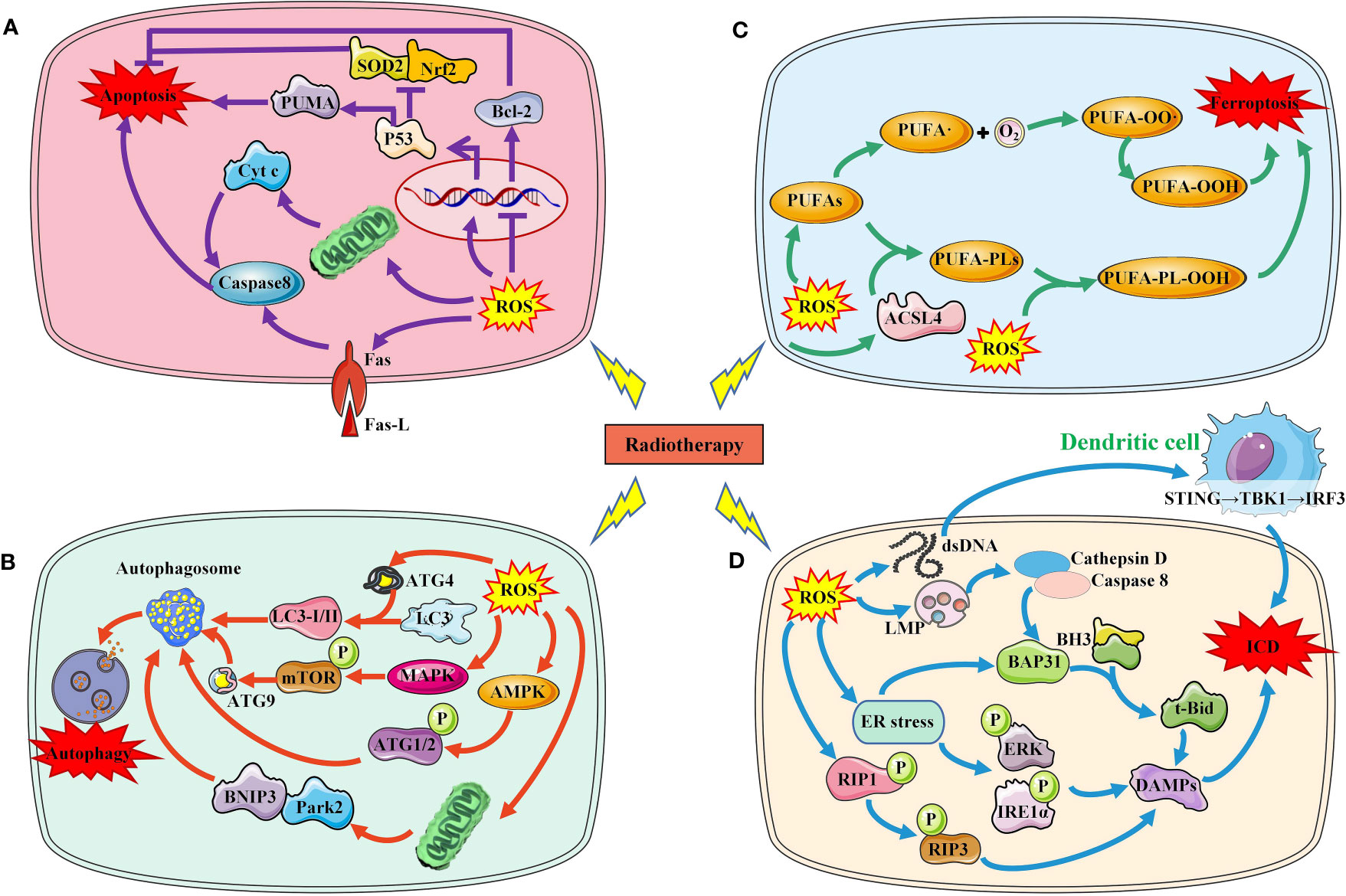
Figure 2 Several major ways in which RT-induced ROS induce tumor cell death. (A). RT-induced ROS induces tumor cell apoptosis through P53 pathway, mitochondrial pathway and death receptor ligand pathway. (B) RT-induced ROS induces autophagy of tumor cells by activating AMPK, MAPK and LC3. (C) RT-induced ROS increased lipid peroxidation induced tumor cell ferroptosis. (D) RT-induced ROS increased ICD by increasing DAMPs production and immune cell activation.SOD2, superoxide dismutase 2; Nrf2, nuclear factor‑erythroid 2 related factor 2; PUMA, p53 upregulated modulator of apoptosis; Bcl-2, B-cell lymphoma-2; Cty c, Cytochrome C; ROS, reactive oxygen species; ACSL4, acyl-CoA synthetase long-chain family member 4; PUFAs, polyunsaturated fatty acids; LMP, Lysosome membrane permeability; BH3,; BAP31, BCR associated protein Bap 31; ER, endoplasmic reticulum; RIP, receptor interacting protein; ERK, extracellular regulated protein kinases; IRE1, Inositol-requiring enzyme 1; DAMPs, damage-associated molecular patterns; ICD, immunogenic cell death; ATG, autophagy protein; MAPK, mitogen-activated protein kinase; AMPK, adenosine 5`-monophosphate-activated protein kinase; mTOR, mechanistic target of rapamycin; BNIP3, BCL2/adenovirus E1B 19kDa interacting protein 3; Park2, Recombinant Parkinson Disease Protein 2.
2.1 Apoptosis and autophagy
DNA is one of the main targets of ROS, which can induce DNA damage and induce DNA damage response (18). When the level of ROS-induced DNA damage exceeds the cell’s ability to repair it, the cell initiates the apoptosis program. P53 is an important molecule that regulates apoptosis. Increased ROS can lead to p53 activation and its downstream upregulation of p53 upregulated modulator of apoptosis, the pro-oxidant genes (19). Furthermore, ROS-induced p53 has been demonstrated to downregulate antioxidant proteins and the anti-oxidant transcriptional factor, including superoxide dismutase 2 and nuclear factor−erythroid 2 related factor 2 (Nrf2) (20, 21). Caspases are a family of proteases in cells, activated by death receptor-dependent pathway and mitochondrial-dependent pathway. ROS can cause the activation of caspase 8 by regulating the expression of the death receptors and its ligand such as Fas and Fas L. Another way of apoptosis is mediated by mitochondria. ROS can not only damage mitochondrial DNA, but also damage mitochondrial electron transport chain, causing mitochondrial dysfunction. Mitochondrial dysfunction results in the release of proapoptotic protein cytochrome c and activates caspases to induce apoptosis. B-cell lymphoma-2 (Bcl-2) is a key anti-apoptotic protein molecule in apoptosis cells. ROS can down-regulate the expression of Bcl-2 and promote apoptosis through oxidative modification.
Autophagy is another important form of RT-induced tumor cell death. ROS accumulation induced by IR can regulate autophagy through a variety of pathways. Autophagy protein (ATG)4 is the core of autophagy regulation, and ROS can directly oxidize ATG4, leading to the accumulation of autophagosomes. ROS can also activate adenosine 5’-monophosphate-activated protein kinase signaling and induce autophagy initiation through ATG1 complex. Similarly, radiation-induced ROS can also activate mitogen-activated protein kinase (MAPK) signaling pathway, which mediates the phosphorylation of mechanistic target of rapamycin (mTOR) and the transport of ATG9, thus promoting the initiation of autophagy. Mitophagy is mainly mediated by BCL2/adenovirus E1B 19kDa interacting protein 3/Nip3-like protein X, and Parkin/PINK1 (PTEN induced putative kinase 1) (22). Radiation induced the accumulation of mitochondrial ROS, which resulted in mitochondrial damage that was in turn recognized by Parkin (23).
2.2 Immunogenic cell death
Immunogenic cell death (ICD), known as immunogenic apoptotic cell death or immunogenic apoptosis, has been defined as a “form of regulated cell death that is sufficient to activate an adaptive immune response in immunocompetent hosts” (24). Cellular redistribution and extracellular release of DAMPs are the main features and mechanisms of ICD, including CRT, HSPs, HMGB1, adenosine 5-triphosphate (ATP), spliceosome-associated protein 130, defensins and S100 proteins.
IR-induced OS can induce immunogenic death of tumor cells through multiple mechanisms (25). (1) Endoplasmic reticulum (ER) stress, caused by overproduction of ROS, has been shown to be responsible for ICD (26–30). CRT is overexpressed and released during ER stress. IR-mediated ER stress produces DAMPs molecules via PERK and IRE1-α phosphorylation, especially CRT, acting as an ‘eat me’ signal to stimulate the antigen presenting function of dendritic cells, acting as an ‘eat me’ signal to stimulate the antigen presenting function of DCs (31). Currently, various therapies, including photodynamic therapy, hyperthermia, and nanomaterials have been explored as ways to enhance ER stress to promote ICD (26, 32, 33). (2) Mitochondria is another important pathway of ROS production induced by IR. Increased ROS level can be directly detected by receptor-interacting protein 1 and leading to autophosphorylation on serine residue 161 (34). And then recruits RIP3 and induces the formation of a functional necrosome with pore formation and the release of DAMPs (35, 36). After IR, mitochondrial DNA is oxidized in tumor cells, which will be phagocytosed by DCs and activate the STING-TBK1-IRF3-IFN-β pathway in DCs, which subsequently cross-presented irradiated tumor cell-derived antigens to CD8+T cells and elicited antitumor immunity (37). (3) Lysosome membrane permeability (LMP): After the radiation-induced ROS were perceived by the cells, LMP changed, which would trigger further downstream approaches to induce ICD (38). After the lysosomal membrane is destroyed, cathepsin D and the prozymogenic form of caspase 8 are released into the cytoplasm (39). Caspase 8 induces ER-associated BAP31 cleavage through downstream caspase activation and cleaves the BH3-only protein Bid into its truncated form, t-Bid, which promotes CRT expression on the cell surface by inducing mitochondrial outer membrane permeation and anterograde ER-Golgi traffic (39–41).
2.3 Ferroptosis
Ferroptosis is another way of radiation-induced tumor cell death, which is caused by lipid peroxidation caused by iron metabolism disorder and ROS accumulation. Lipid peroxidation is an important marker of ferroptosis. IR-induced ROS can remove electrons from polyunsaturated fatty acids (PUFAs) to form fatty acid free radicals (PUFA•), which rapidly react with molecular oxygen to form lipid peroxyls (PUFA-OO•) and form lipid hydroperoxides (PUFA-OOH) through Fenton reaction, which lead to lipid peroxidation of membrane phospholipids, eventually resulting in ferroptosis (42). In addition, IR-induced acyl-CoA synthetase long-chain family member 4 (ACSL4) expression increased PUFA-PL biosynthesis, which together with ROS drove PUFA-PL peroxidation (PUFA-PL-OOH) and ferroptosis (43, 44).
Ferroptosis is an important way of tumor cell death induced by RT, and lipid peroxidation induced by OS is the basis of ferroptosis. RT not only induced ROS production, but also induced the expression of ACSL4, a lipid metabolizing enzyme required for ferroptosis, leading to elevated lipid peroxidation and ferroptosis (44). IR-induced ROS attack PUFAs to produce lipid peroxides (L-OOH), resulting in the death of iron. The peroxidation process is mediated by lipoxyphenase and phosphatidyl ethanolamine binding protein 1. L-OOH can be reduced to the corresponding alcohol by glutathione peroxidase or by panthenol by Fenton reaction L-OO (45–47). Recombinant solute carrier family 7, member 11 (SLC7A11 or xCT) can promote glutathione (GSH) synthesis and reduce L-OOH production to reduce the occurrence of ferroptosis (48). Radiation activates the ataxic-telangiectasia mutant (ATM), which inhibits SLC7A11 expression and promotes ferroptosis (49). It has been suggested that tumor ferroptosis is a new intersection between RT and adaptive immune response. IFN-γ released by immunotherapy-activated CD8+T cells down-regulates the expression of SLC3A2 and SLC7A11, inhibits cystine uptake in cancer cells, and enhances ferroptosis specific lipid peroxidation in tumor cells (50). Nanoparticle loaded miR-21-3p increases ROS production by directly targeting thioredoxin reductase 1, thereby enhancing lipid peroxidation to promote IFN-γ-mediated ferroptosis and acting synergistically with anti-PD-1 antibodies (51). Radiation-induced ATM activation is also an important source of IFN-γ, which synergies with IFN-γ derived from CD8+T cells to inhibit SLC7A11 and increase ferroptosis in tumor cells (52). In an oxygen-deficient environment, neutrophils are able to transfer granules containing myeloperoxidase into tumor cells, thereby inducing the accumulation of lipid peroxides and iron in tumor cells and triggering ferroptosis (53). On the other hand, immune cells in the TME also undergo ferroptosis. CD36 mediates the uptake of fatty acids by tumor-infiltrating CD8+T cells, induces lipid peroxidation and ferroptosis, and leads to reduced cytotoxic cytokine production and reduced anti-tumor ability (54). Since Tregs are more resistant to ROS-induced ferroptosis, therapeutic strategies that can specifically induce lipid peroxidation and ferroptosis accumulation in Tregs need to be developed (55). Ferroptosis is more likely to occur in M2 TAM than M1 (56). Hsieh et al. (57) found that zero-valent iron (ZVI), a nanoparticle capable of inducing ferroptosis in tumor cells, was able to convert M2 type TAM into M1 type, lifting the inhibition of anti-tumor immunity. However, in pancreatic cancer, OS was able to induce secretion of KRASG12D-containing exosomes, which were taken up by macrophages to mediate autophagy-dependent ferroptosis and switch TAM to the M2 phenotype (58). In addition, in TME, the expression of proteins associated with ferroptosis and lipid peroxidation in natural killer cells (NKs) was increased, suggesting that the dysfunction of NKs may be related to ferroptosis (59). Similarly, ferroptosis in DCs decreases antigen presentation and antitumor ability, and prevents CD8+T cells from producing IFN-γ (60). RT is often used in combination with ICIs, but lacks the expected survival benefit in some tumors. In this subset of patients, combining ferroptosis inducers with immunotherapy and RT may be a good strategy for enhancing tumor ferroptosis and sensitizing such tumors to immunotherapy and RT. Drugs have also been developed to promote ferroptosis, inducing ferroptosis while also enhancing the immune response caused by immune checkpoint inhibitors (61). However, Due to the contradictory effects of ferroptosis on tumor cells and immune cells, the clinical application value of ferroptosis in immune cells needs further exploration (Figure 3).
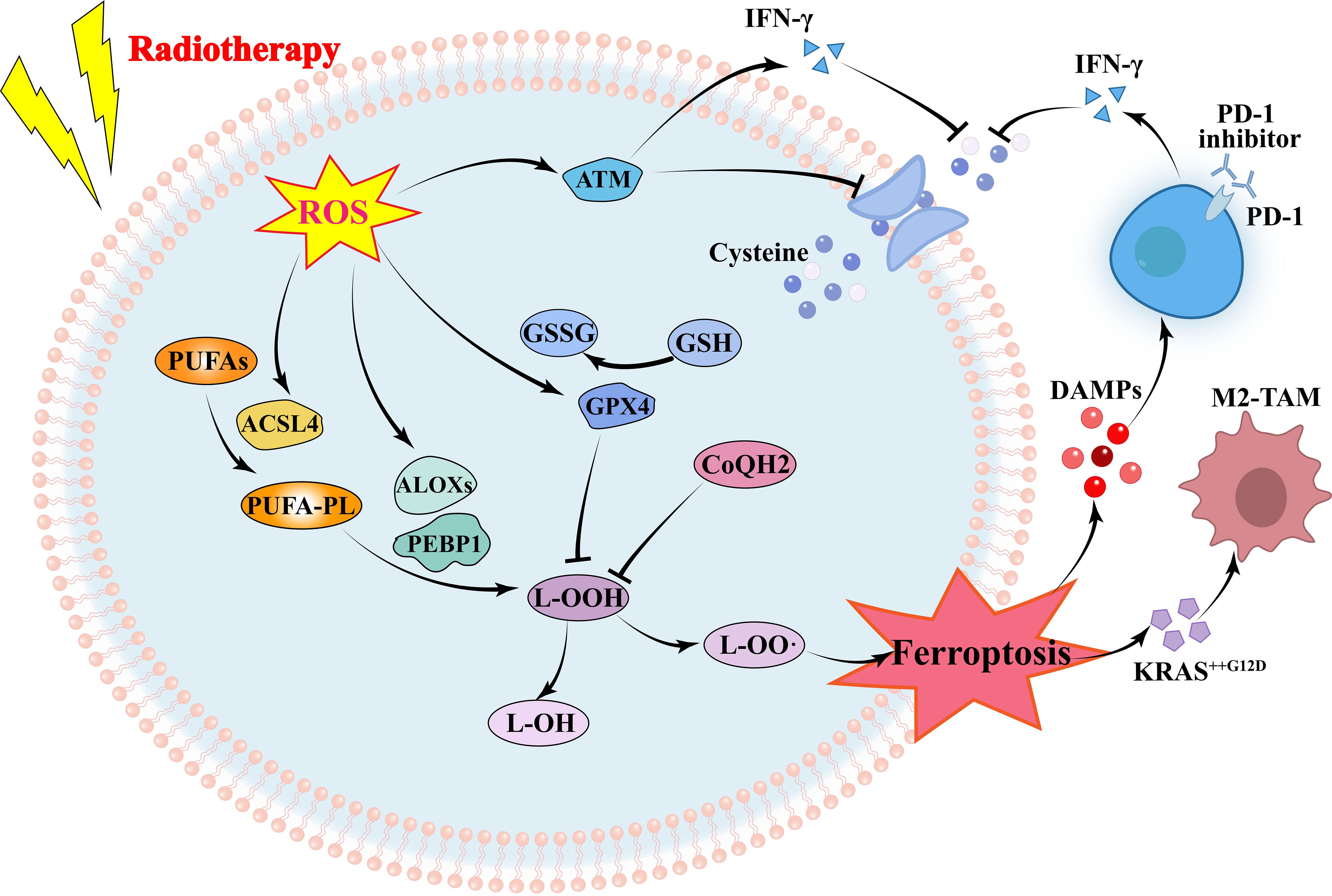
Figure 3 Mechanism of radiotherapy and immunotherapy in regulating ferroptosis of tumor cells. RT-induced ROS increases ferroptosis by increasing lipid peroxidation and decreasing glutathione. Immunotherapy and RT-activated T cells secreting IFN-γ inhibit glutathione synthesis by reducing glutamine transport, to promoting ferroptosis. ROS, reactive oxygen species; ATM, ataxia telangiectasia mutated protein; IFN, interferon; GSH, glutathione; GSSG, Glutathione Oxidized; GPX4, glutathione peroxidase 4; PUFAs, polyunsaturated fatty acids; ACSL4, acyl-CoA synthetase long-chain family member 4; ALOXs, Lipoxygenases; PEBP1, Phosphatidylethanolamine-binding Protein 1; DAMPs, damage-associated molecular patterns; TAM, tumor-associated macrophage.
3 OS regulates TME
3.1 OS regulates immune checkpoint expression
IR is considered as a precipitating factor of immune checkpoint expression (62, 63), and ROS plays a key role in this process (64). In macrophages, treatment with ROS inducers resulted in increased expression of PD-L1 (65). Mechanistically, ROS accumulation activated nuclear factor kappa-B (NF-κB) signaling to promote PD-L1 transcription and immunosuppressive chemokine release. In pancreatic cancer, ROS can also induce the expression of fibroblast growth factor receptor 1, which can directly induce the expression of PD-L1 or by activating protein kinase B (AKT) signaling (66). ROS induced by IR is one of the main causes of DNA double-strand breaks (DSBs). DSBs activate ATM kinase, which is able to convert signals to ataxia telangiectasia and Rad3-related (ATR), thereby upregulating checkpoint kinase 1 (Chk1) activity to promote PD-L1 expression, mediated through STAT1/3-IRF1 pathway (67). The fragment DNA produced by DSBs can also be sensed by intracellular cGAS, thereby activating STING-TBK1-IRF3 signal to increase the secretion of type I IFN, which is an important way to induce the expression of PD-L1 (68, 69). Similarly, during OS in mitochondria, oxidized mitochondria DNA is released into the cytosol and then it induces IFN signaling via cGAS-STING-TBK1, which upregulates PD-L1 and IDO-1 expression to inhibit T-cell activation (16). In addition to PD-L1, ATR-Chk1 activation also contributes to integrin-associated protein (CD47) expression, which is mediated by STAT3 (70). CD47 is another immunoglobulin highly expressed on the surface of tumor cells, which can inhibit macrophage-mediated phagocytosis by binding to its major ligand signal regulatory protein α, thereby negatively regulating anti-tumor immunity (71). However, it has also been reported that ROS can also down-regulate the expression of CD47, which is mediated by hypoxia-inducible factor 1α (HIF-1α) (72, 73).
3.2 OS regulates immune cells
IR-induced ROS also regulates the function and activation of immune cells (74). In TME, several major immune cells, such as T cells, Tregs, NKs, DCs, TAMs and MDSCs, are regulated by OS (Figure 4). Among T cells, CD8+T cells are the main specific immune killer cells that perform tumor killing. Of course, T cells also include many helper T cells. NKs are the main innate anti-tumor immune cells, which can directly kill tumor cells. DCs is the primary antigen presenting cell responsible for delivering TAAs to other immune killer cells. TAMs are divided into M1 type and M2 type, in which M1 type is an inflammatory phenotype and contributes to anti-tumor, while M2 type has the effect of promoting tumor immune escape. MDSCs and Tregs are the two main cells that inhibit anti-tumor immunity, and their increase often indicates immune escape of tumor cells.
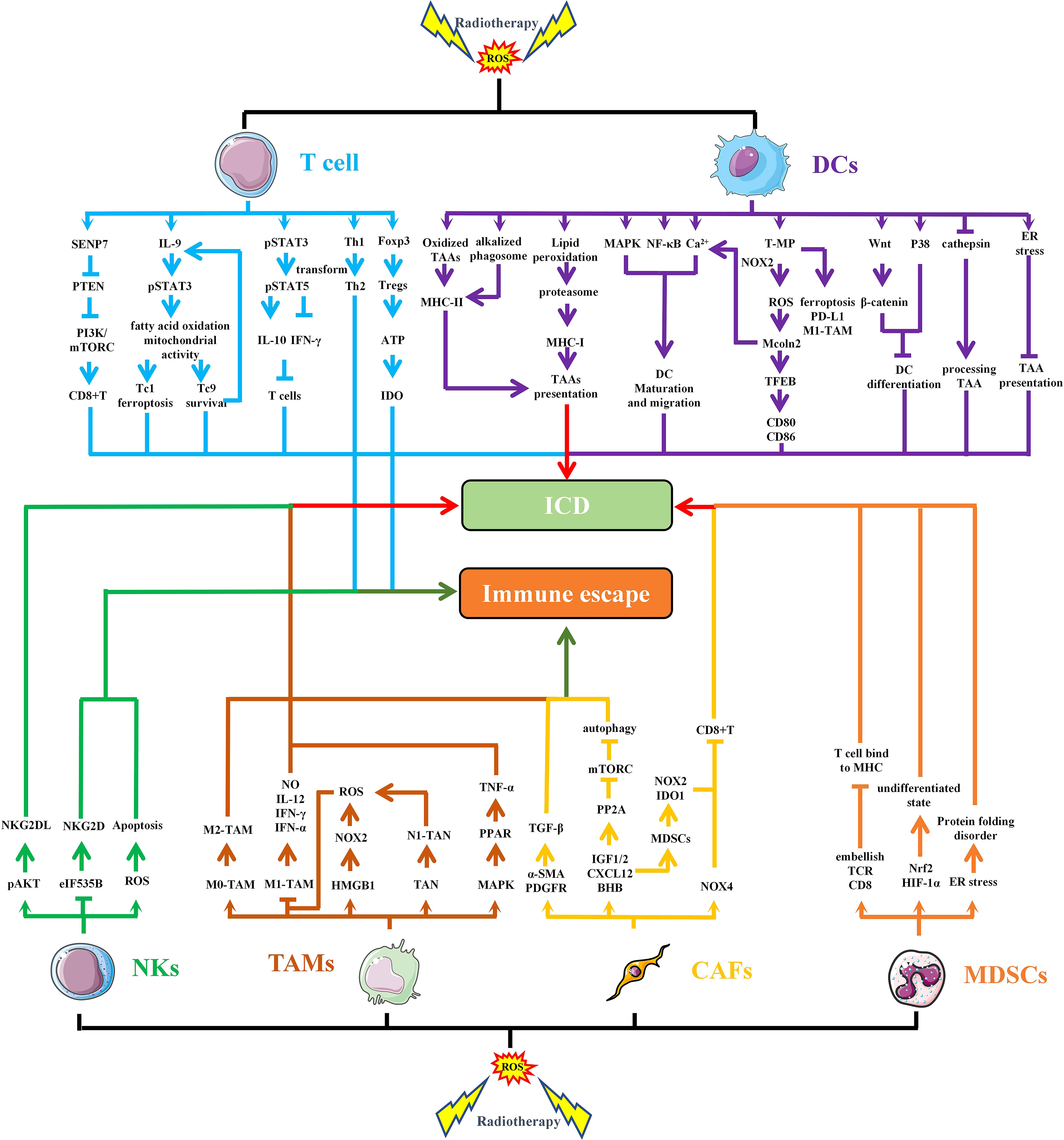
Figure 4 RT-induced ROS regulates the function and activation of immune cells. IR-induced ROS regulates the function of different cells in TME through multiple pathways, leading to immunogenic death or immune escape of tumor cells. SENP7, SUMO-specific protease 7; PTEN, phosphatase and tensin homolog deleted on chromosome 10; PI3K, Phosphoinositide-3 kinase; IL, interleukin; STAT, signal transducer and activator of transcription; IFN, interferon; CD, cluster of differentiation; Foxp3, forkhead box protein 3; ATP, adenosine 5-triphosphate; IDO, indoleamine 2,3-dioxygenase; MHC, major histocompatibility complex; TAAs, tumor-associated antigens; MAPK, mitogen-activated protein kinase; NF-κB, nuclear factor kappa-B; T-MP, tumor cell-derived microparticles; NOXs, NADPH oxidases; EB, transcription factor EB; ICD, immunogenic cell death; NO, nitrous oxide; TANs, Tumor-associated neutrophils; TNF, tumor necrosis factor; PPAR, peroxisome proliferator activated receptor; TGF, transforming growth factor; α-SMA, α-smooth muscle actin; PDGFR, platelet—derived growth factor receptor; PP2A, protein phospholipase; IGF, insulin like growth factor; CXCL, chemokine (C-X-C motif) ligand; BHB, β-hydroxybutyrate.
3.2.1 T cells
ROS concentration is a necessary precursor for the activation and function of CD8+T cells, especially during TCR signal transduction after tumor antigen stimulation (75). It is well known that phosphatase and tensin homolog deleted on chromosome 10 (PTEN) is an important inhibitory molecule in the PI3K/AKT/mTOC pathway and has immunosuppressive function. SUMO-specific protease 7 (SENP7), a reversible posttranslational modification protease, can sense OS to induce PTEN degradation, thereby maintaining metabolic fitness and effector functions of CD8+T cells (76). CD8+Tc9 (cytotoxic T lymphocyte subset 9) cells exert greater persistence and antitumor efficacy than Tc1 cells. Tc9 cell-derived IL-9 activated STAT3, upregulated fatty acid oxidation and mitochondrial activity, and rendered Tc9 cells with reduced lipid peroxidation and resistance to ROS-induced ferroptosis in the TME (77). The increase of ROS significantly down-regulates the expression of miR-155-5p in tumor cells. Targeting miR-155-5p may be an effective method to improve TME by down-regulating PD-L1 and increasing infiltration of CD8+T cells (78). Appropriate level of ROS is a key signaling molecule involved in directing T cell activation and differentiation. However, with too high level, it inhibits proliferation, damages DNA, and induces apoptosis (79). It has been suggested that elevated ROS is a driver of T cell depletion. ROS elevation drives elevated nuclear factor of activated T cells localization and sustained signaling, inducing depleted T cell phenotypes with elevated PD-1, T cell immunoglobulin domain and mucin domain-3, and T cell immunoreceptor with Ig and immunoreceptor tyrosine-based inhibition motif domains (80). In addition, ROS induced decreased IFN-γ secretion in T cells and increased IL-10 production through the STAT3-STAT5 axis (81). ROS can also cause changes in Th1/Th2 polarity, which is conducive to the production of more Th2 cells, which is conducive to the tumor (82). NF-κB is believed to be the key factor in inhibiting T cell function and inducing T cell death induced by OS (79). Chronic or persistent low-dose OS down-regulates NF-κB in naive and mature activated/memory T cells, and the synergistic effect of Ros-mediated NF-κB suppression and elevated TNF-α level leads to T cell death (83).
Tregs are recruited into the TME to mediate immunosuppression and are susceptible to OS in the TME (84). Cellular OS state induces and maintains the epigenetic modification, transcription, translation, and post-translational stability of FoxP3, the master regulator of Tregs (85). In TME with OS, Tregs are able to compete with DCs and effector T cells for cysteine, the feedstock of GSH, resulting in inhibition of DCs function and downregulation of T cell activation and proliferation (86). In addition, the weak Nrf2-related antioxidant system in Tregs transforms them into apoptotic Tregs in oxidized TME, which can self-supply and release ATP, and convert it to adenosine to mediate, sustain, and amplify powerful suppression in the TME (84)
3.2.2 DCs
DCs are fundamental for the initiation and maintenance of immune responses against cancer cells and have been shown to generate ROS during antigen presentation (87, 88). Immature DCs mainly have the ability to migrate, while mature DCs can mediate antigen presentation. During the differentiation of monocytes into DCs, expression of the DNA repair proteins XRCC1, ligase IIIα, poly (ADP-ribose) polymerase-1, and catalytic subunit of DNA-dependent protein kinase become up-regulated, making DCs repair-competent and ROS-resistant (89). Oxidized antigens have higher immunogenicity, which is reflected in enhanced MHC-II antigen processing and presentation by DCs (90). ROS can promote maturation of DCs and promote antigen presentation through phagosomal alkalinization (87). In DCs, ROS production and lipid peroxidation promote the escape of antigens from endosomes into the cytoplasm so that the proteasome can be degraded into peptides and processed into MHC I (91). In addition, transient intracellular ROS rapidly induced cytosolic mobilization of Ca2+, differential activation of mitogen-activated protein kinases, and nuclear translocation of NF-κB, which activate immature DCs to mature and potently enhance migration (92). DNA fragments generated by IR can promote the production of cGAMP, which can enter the TME and be ingested by DCs, activating STING signal (37, 93). STING-dependent cytosolic DNA sensing in DCs initiates antitumor immune responses, OS as a metabolic regulator promotes STING-mediated DCs antitumor immune responses and highlights SENP3 as an overflow valve for STING signaling induction in the metabolically abnormal TME (94). Tumor cell-derived microparticles (T-MP) increase lysosomal pH via NADPH oxidase 2 (NOX2, previously known as gp91phox)-catalyzed ROS production, promoting the formation of MHC class I-tumor antigen peptide complexes (95). It has been reported that radiation and chemotherapy can produce microparticles from tumor cells. Irradiation-induced T-MP can be taken up by tumor cells and TAMs, resulting in increased ferroptosis, PD-L1 expression, and M1 phenotype TAMs (96). Moreover, T-MP increased ROS activates the lysosomal Ca2+ channel Mcoln2, leading to Ca2+ release and activation of the transcription factor EB, a lysosomal master regulator that directly binds to CD80 and CD86 promoters and promotes gene expression, which facilitates antigen presentation (95). However, ROS has also been reported to have harmful effects on DCs. High glucose medium impaired the differentiation of monocytes to DCs by inducing ROS to activate Wnt/β-catenin and p38 MAPK (97). Overproduction of ROS also inhibits the hydrolytic function of cathepsin in DC, thus inhibiting antigen processing (98). Meanwhile, chronic activation of endoplasmic reticulum stress responses induced by excess ROS inhibits the ability of DC to present antigens to T cells (99, 100). Mitochondrial metabolism in plasmacytoid DCs (pDCs) which acquire the cross-presentation ability after stimulation by toll-like receptor (TLR) ligands is important to the induction of adaptive immune responses. The reduction of mitochondrial ROS production dramatically decreases the cross-presentation capacity of pDCs, leading to a strong reduction of their capacity to trigger CD8+T cells responses (101).
3.2.3 NKs
NKs are lymphocytes capable of eliminating tumor cells without prior sensitization to a specific antigen (102). However, in TME, ROS is quite detrimental to NK survival and function. ROS induced in NK promotes NK cell apoptosis, while activation of antioxidant pathway increases NK resistance to OS (103, 104). Inhibition of NOx2-derived ROS in combination with cell-activating cytokines or checkpoint inhibitors can enhance NK and T cell function (105). NKs-expressed activating receptor Natural Killer Group 2D (NKG2D) acts as a “master switch” in controlling the arousal state of NK cells. NKG2D-mediated cytotoxicity is related to the level of NKG2D ligands (NKG2DLs) expressed by tumor cells. It has been reported that ROS-induced AKT phosphorylation induces the expression of NKG2DLs, which would sensitize tumor cells to NKs (106). In breast cancer, ROS reduces translation-initiation rate-limiting factor (eIF535B) phosphorylation at Ser2 and down-regulates NKG2D expression to inhibit NK cell activity (107).
3.2.4 TAMs and tumor-associated neutrophils
In the tumor microenvironment, TAMs mainly include M1 and M2 phenotypes, among which M1 type has highly pro-inflammatory and anti-tumor functions, while M2 type contributes to suppress immune response. ROS plays a critical role in the differentiation of TAMs (108). M1 macrophages are more sensitive to OS, so ROS can polarize M0 macrophages into a tolerant M2 phenotype (109). It has also been reported that ROS is a critical trigger of cyclooxygenase-2–mediated macrophage differentiation from monocytes (108). Inhibition of ROS could transform TAMs into M1 phenotype, promote the secretion of inducible nitric oxide synthase, IL-12, IFN-γ and TNF-α (110). HMGB1 is a key molecule in irradiation induced ICD. A study has confirmed that in hepatocellular carcinoma, HMGB1 binds to TLR2 on the surface of TAMs to produce ROS via NOX2 (111). The increase of ROS promotes an increase in autophagy, which polarizes TAMs toward the M2 phenotype. In melanoma, ROS is also capable of ectopic peroxisome proliferator activated receptor-γ from the nucleus into the cytoplasm via mitogen-activated protein kinase kinase 1, thereby inducing an increase in TNF-α, which not only plays an antitumor role, but also converts TAMs into a phenotype that promotes tumor invasion (112).
Tumor-associated neutrophils (TANs) play an important role in TME. Irradiation can activate NOXs in neutrophils and produce ROS, which can increase phagocytosis (113). TANs include both antitumor (N1) and proto-tumor (N2) phenotypes, in which N1 TAN can be induced by RT. RT-induced N1 TAN produced more ROS, leading to tumor cell apoptosis and subsequent activation of tumor-specific cytotoxic T lymphocytes (CTLs) (114).
3.2.5 MDSCs
There is a close relationship between the immunosuppressive function of MDSCs and ROS. ROS molecules are essential for maintenance of MDSCs in their undifferentiated state. Scavenging ROS induces differentiation of immature myeloid cells into macrophages, while differentiate into macrophages and DCs in the absence of NOX activity (115, 116). In polymorphonuclear MDSC, ROS-induced ER stress is the main factor driving MDSC function. ROS accumulation can induce oxidative modification of ER coelomins and thus affect protein folding (117, 118). Clearing ROS significantly attenuates MDSC immunosuppression by inhibiting the unfolded protein response. In addition, the release of ROS by MDSCs is one of the main mechanisms used to inhibit human T cells. ROS produced and secreted by MDSCs can modify TCR and CD8 molecules in T cells, resulting in CD8+T cells losing the ability to bind MHC (119). Furthermore, beyond their role in MDSC-mediated immune-suppression, ROS molecules are intrinsically involved in activation of transcription factors such as Nrf2 and HIF-1α, which can induce transcriptional and metabolic reprogramming of MDSCs and influence their differentiation and maintenance (9).
3.2.6 Cancer-associated fibroblasts
Cancer-associated fibroblasts (CAFs) are key stromal cells in TME and promote tumor growth by releasing various growth factors. Myofibroblasts (an activated form of fibroblast) express smooth muscle actin (alpha-SMA) and platelet-derived growth factor receptors after IR, activating TGF-β signaling and angiogenesis via mitochondrial ROS to promote tumor growth (120). In addition, Insulin-like growth factor-1/2(IGF1/2), chemokine (C-X-C motif) ligand 12(CXCL12), and beta-hydroxybutyrate produced by CAFs can induce autophagy of cancer cells after RT, and promote recovery of irradiated cancer cells and tumor regeneration after RT. These CAFs-derived molecules, IGF1/2, CXCL12 and beta-hydroxybutyrate, increased ROS level after radiation, thereby enhancing protein phosphatases 2A activity, inhibiting mTOR activation, and increasing autophagy in cancer cells (121). CAFs are thought to promote immune escape through a variety of mechanisms and are regulated by ROS induced by NOX4 (122). NOX4 inhibition is capable of promoting CD8+T cell infiltration within tumors and restoring the immunotherapeutic response of CAFs-rich tumors. CAFs secrete CXCL2, facilitating the migration of monocytes to the local TME in lung squamous cell carcinoma and promoting monocyte-to-MDSCs differentiation. CAFs-induced MDSCs further inhibit CD8+T cells proliferation by upregulating the expression of NOX2 and IDO 1 to generate excessive ROS (123).
4 Regulating OS to enhance the efficacy of immunotherapy
Given the effect of OS on TME, regulation of OS is considered an effective protocol for enhancing immunotherapy efficacy (Table 1).
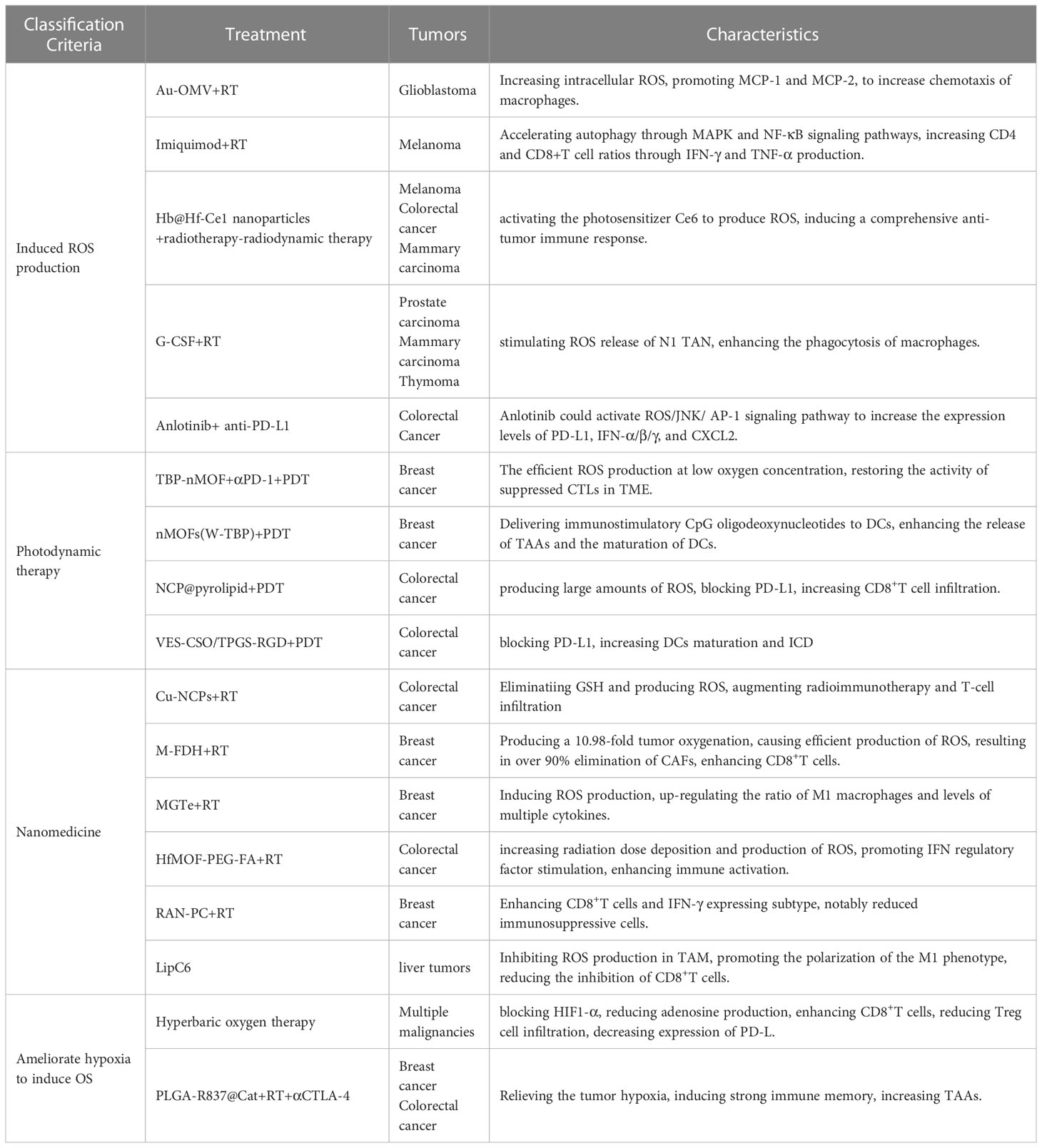
Table 1 Potential approachs to modulating oxidative stress to enhance radiotherapy in combination with immunotherapy.
4.1 Induced ROS production
The histopathological feature of glioblastoma is the significant invasion of microglia and peripheral macrophages (the primary innate immune cells of the central nervous system) resident in the tumor. Researchers have designed a compound Au-OMV using gold nanoparticles and bacterial outer membrane vesicles to increase intracellular ROS production (124). ROS induced OS promotes the expression of macrophage/monocyte chemoattractant protein-1 (MCP-1) and MCP-2. The combination of Au-OMV with RT increases chemotaxis of macrophages and radiation sensitivity in glioblastoma. TLR7 agonist imiquimod binding RT-induced ROS accelerates autophagy in melanoma through MAPK and NF-κB signaling pathways and increases CD4 and CD8+T cell ratios through IFN-γ and TNF-α production (125). Alternol is a naturally occurring compound that promotes the production of DAMPs and pro-inflammatory cytokines through elevated ROS, thereby promoting CD8+T cell initiation and T cell infiltration in tumors (126). To improve the therapeutic efficacy of RT-radiodynamic therapy, an oxygen-rich X-ray nanoprocessor Hb@Hf-Ce1 nanoparticles were designed in which an encapsulated oxygen carrier (hemoglobin, Hb) was used to regulate oxygen balance in anoxic TME. In contrast, radioluminescence stimulated by Hf under X-ray IR can activate the photosensitizer Ce6 to produce ROS, thus inducing a comprehensive anti-tumor immune response (127). G-CSF can stimulate the release of neutrophils and monocytes and enhance the phagocytosis of macrophages. It could amplify the tumor-specific immune response induced by RT, which is mediated by ROS production from N1 TAN stimulated by G-CSF (114). Anti-angiogenic drugs have the effect of normalizing blood vessels, which will improve tumor hypoxia and increase ROS production after radiotherapy (128). Immunotherapy also has this effect, so anti-angiogenic drugs (bevacizumab) can also increase the therapeutic efficacy of immune checkpoint inhibitors (129). Anlotinib is another antiangiogenic drug, and in colorectal cancer, Anlotinib benefits the anti-PD-L1 immunotherapy by activating ROS/JNK/AP-1 pathway to upregulate PD-L1 expression (130).
4.2 Photodynamic therapy
Photodynamic therapy (PDT) uses photosensitizers (PSs) to absorb certain wavelengths of ultraviolet light and produce free radicals or ions, which in turn produce ROS to suppress tumors (131). Importantly, PDT may well initiate ICD. First, PDT promotes the transfer of CRT from the endoplasmic reticulum to the surface of cell membranes and enhances the expression of HSP, providing “eat me” signals (132). In addition, PDT-induced ROS can promote the secretion of various cytokines (IFN-γ, IFN-α, etc.) by immune cells, and promote the infiltration of APCs and CD8+T cells (133). Many preclinical studies have found beneficial antitumor effects of PDT in combination with immunotherapy. In one study, Zhang et al. developed phenyporphyrin-based nMOFs, and PSs (TBP) combined with αPD-1 to restore the activity of suppressed CTLs in immunosuppressive TME (134). In another study, Lin et al. constructed a cationic nMOF (W-TBP) that is highly loaded with the anion CpG, which enhances the release of TAAs and effectively enhances the maturation of DCs (135). In addition, a self-assembled nano-coordination polymer (NCP) nanoparticle loaded with oxaliplatin induced an increase in CD8+T cell infiltration in both primary and remote tumors blocking PD-L1 and production of large amounts of ROS (136). Another temophen-supported nanodrug delivery system (VES-CSO/TPGS-RGD) has been shown in colorectal cancer to promote PD-L1 expression via HIF-1α and synergistically increase DCs maturation and ICD with PD-L1 blocking (137). Conventional PDT is limited in clinical application by the depth of light penetration, while X-rays can break shallow penetration limits as an energy source (138). In turn, ROS produced by PDT can increase DNA damage and lipid peroxidation damage, thus enhancing RT sensitivity (139). This suggests that the development of PDT is a potential sensitization method for radiotherapy combined with immunotherapy.
4.3 Nanomedicine
A variety of nanomaterials have been developed to amplify OS-induced ICDs during RT. Cu-based nanoscale coordination polymers (Cu-NCPS) with mixed-valence (Cu/Cu2+) have been shown to trigger GSH elimination and ROS production, to augmented radiotherapy combined with immunotherapy and T-cell infiltration (140). Hypoxic TME limits ROS production during RT. A microvesicle-inspired oxygen-delivering polyfluorocarbon nanosystem loading DiIC18(5) and halofuginone (M-FDH) produced a 10.98-fold enhancement of tumor oxygenation and caused efficient production of ROS upon radiation, which resulted in over 90% elimination of CAFs, enhancement of CD8+T cells, and elimination of suppressive immune cells (141). A hybrid nanoplatform (designated as MGTe) composed of tumor- and bacteria-derived immunomodulators and nano-radiosensitizer was acted as a radiosensitizer to enhance the efficacy of RT by ROS generation and ICD (142). MGTe-induced ROS would up-regulate the ratio of M1 macrophages and level of multiple cytokines. The hafnium foundation organic framework (HfMOF-PEG-FA) modified with folate increases radiation dose deposition and subsequent production of ROS, promotes IFN regulatory factor (IRF) stimulation, and in combination with the TLR7 agonist imiquimod enhances immune activation, characterized by an increase in CD8+ and proliferative T cells (143). A ROS-responsive albumin nanocomplex of anti-PD-L1 and cabazitaxel (RAN-PC) was designed to promote their intratumor delivery with controllable release capacity and potentiate radiation-mediated antitumor immunity for cancer therapy (144). RAN-PC+radiotherapy treatment produced a 3.61- and 5.10-fold enhancement in CD8+T cells and IFN-γ expressing subtype, respectively, and notably reduced versatile immunosuppressive cells. A nanoliposome loaded C6-ceremide (LipC6) was developed to inhibit ROS production in TAM to promote the polarization of the M1 phenotype and reduce the inhibition of CD8+T cells (110).
4.4 Ameliorate hypoxia to induce oxidative stress to enhance radiotherapy combined with immunotherapy
Hypoxia is a hallmark of solid tumors that reduces their susceptibility to radiation therapy and is a driver of immunotherapy resistance (145). Given the enormous benefits of targeting hypoxia for RT and immunotherapy, a number of regiments have been developed to ameliorate hypoxia to increase the efficacy of radiotherapy combined with immunotherapy, including increasing oxygen supply to tumors, inhibiting mitochondrial oxidative phosphorylation (OXPHOS), inhibiting HIF activity (146). Hyperbaric oxygen therapy can be used to counteract the anoxic effect in the TME to achieve the large number of ROS produced after IR, which can improve the efficacy of RT (147). In addition, hyperoxia blocks the hypoxia-HIF1α-adenosine pathway to reduce adenosine production, thereby enhancing CD8+T cells and reducing Treg cell infiltration. Meanwhile, oxygen-depleted MDSC inhibits antigen-specific and nonspecific T cell activity via HIF-1α, which can be inhibited by hyperoxygen, leading to decreased expression of PD-L1 (147). Core-shell nanoparticle based poly (lactate-glycolic) acid (PLGA) was prepared by encasing water-soluble catalase (Cat) to decompose H2O2 into O2, and imiquimote (R837), a toll-like receptor-7 agonist, was loaded into the shell. The formed PLGA-R837@Cat nanoparticles modulate the immunosuppressive TME by relieving tumor hypoxia. TAAs produced after RT induce intense ICD with the help of PLGA-R837@Cat. Combined with CTLA-4 checkpoint blocking, tumor metastasis is effectively inhibited through a powerful distant effect (148).
5 Conclusion
Radiotherapy can induce DNA strand breaks, damage of large molecules such as lipids and proteins, and other reactions. ROS is considered to be an important cytotoxic and signaling molecule in these reactions, inducing oxidative stress response of tumor cells. With the combination of radiotherapy and immunotherapy, the effect of oxidative stress on immune response has gained attention. In fact, apoptosis, autophagy and ferroptosis induced by oxidative stress are related to immunogenic death of tumor cells. A variety of immune cells and immune checkpoints in the immune microenvironment are also regulated by ROS. Appropriate ROS is a necessary condition for the activation of some immune cells, but excessive ROS may be an important reason why many immune cells have difficulty in performing anti-tumor functions and become resistant to immunotherapy. Therefore, a variety of therapeutic approaches are being explored to regulate ROS production during radiotherapy to enhance the efficacy of combination radiotherapy. In the future, while focusing on the changes of oxidative stress in tumor cells, we still need to pay attention to the influence of ROS in the TME on immunotherapy.
Author contributions
Conceptualization: XJ. Methodology: ZZ and JS. Software: XB. Validation: JX. Formal analysis: HW. Investigation: CB. Resources, XJ. Data curation: ZZ. Writing—original draft preparation: ZZ, JS, and CB. Writing—review and editing: XB, QZ, and XJ. Visualization: XJ. Supervision: XJ. Project administration: XJ. Funding acquisition: XJ. All authors contributed to the article and approved the submitted version.
Funding
This work was supported by the Jilin Provincial Science and Technology Foundation (grant number 20210402002GH and 20230508064RC), the Achievement Transformation Guiding Foundations of First Hospital of Jilin University (grant number CGZHYD202012-029).
Acknowledgments
We would like to thank Editage (www.editage.cn) for English language editing.
Conflict of interest
The authors declare that the research was conducted in the absence of any commercial or financial relationships that could be construed as a potential conflict of interest.
Publisher’s note
All claims expressed in this article are solely those of the authors and do not necessarily represent those of their affiliated organizations, or those of the publisher, the editors and the reviewers. Any product that may be evaluated in this article, or claim that may be made by its manufacturer, is not guaranteed or endorsed by the publisher.
Glossary
References
1. Decker WK, da Silva RF, Sanabria MH, Angelo LS, Guimarães F, Burt BM, et al. Cancer immunotherapy: historical perspective of a clinical revolution and emerging preclinical animal models. Front Immunol (2017) 8:829. doi: 10.3389/fimmu.2017.00829
2. Fang Y, Yu A, Ye L, Zhai G. Research progress in tumor targeted immunotherapy. Expert Opin Drug Deliv (2021) 18:1067–90. doi: 10.1080/17425247.2021.1882992
3. Azzam EI, Jay-Gerin JP and Pain D. Ionizing radiation-induced metabolic oxidative stress and prolonged cell injury. Cancer Lett (2012) 327:48–60. doi: 10.1016/j.canlet.2011.12.012
4. Delaney G, Jacob S, Featherstone C, Barton M. The role of radiotherapy in cancer treatment: estimating optimal utilization from a review of evidence-based clinical guidelines. Cancer (2005) 104:1129–37. doi: 10.1002/cncr.21324
5. Santivasi WL, Xia F. Ionizing radiation-induced DNA damage, response, and repair. Antioxid Redox Signal (2014) 21:251–9. doi: 10.1089/ars.2013.5668
6. Herrera FG, Bourhis J and Coukos G. Radiotherapy combination opportunities leveraging immunity for the next oncology practice. CA Cancer J Clin (2017) 67:65–85. doi: 10.3322/caac.21358
7. Kennel KB, Greten FR. Immune cell - produced ROS and their impact on tumor growth and metastasis. Redox Biol (2021) 42:101891. doi: 10.1016/j.redox.2021.101891
8. Murphy MP, Siegel RM. Mitochondrial ROS fire up T cell activation. Immunity (2013) 38:201–2. doi: 10.1016/j.immuni.2013.02.005
9. Ohl K, Tenbrock K. Reactive oxygen species as regulators of MDSC-mediated immune suppression. Front Immunol (2018) 9:2499. doi: 10.3389/fimmu.2018.02499
10. Li L, Sun F, Han L, Liu X, Xiao Y, Gregory AD, et al. PDLIM2 repression by ROS in alveolar macrophages promotes lung tumorigenesis. JCI Insight (2021) 6:20210308. doi: 10.1172/jci.insight.144394
11. Kunisada Y, Eikawa S, Tomonobu N, Domae S, Uehara T, Hori S, et al. Attenuation of CD4(+)CD25(+) regulatory T cells in the tumor microenvironment by metformin, a type 2 diabetes drug. EBioMedicine (2017) 25:154–64. doi: 10.1016/j.ebiom.2017.10.009
12. Mougiakakos D, Johansson CC, Kiessling R. Naturally occurring regulatory T cells show reduced sensitivity toward oxidative stress-induced cell death. Blood (2009) 113:3542–5. doi: 10.1182/blood-2008-09-181040
13. Chen X, Song M, Zhang B, Zhang Y. Reactive oxygen species regulate T cell immune response in the tumor microenvironment. Oxid Med Cell Longev (2016) 2016:1580967. doi: 10.1155/2016/1580967
14. Yoon S, Woo SU, Kang JH, Kim K, Kwon MH, Park S, et al. STAT3 transcriptional factor activated by reactive oxygen species induces IL6 in starvation-induced autophagy of cancer cells. Autophagy (2010) 6:1125–38. doi: 10.4161/auto.6.8.13547
15. Lee SY, Jeong EK, Ju MK, Jeon HM, Kim MY, Kim CH, et al. Induction of metastasis, cancer stem cell phenotype, and oncogenic metabolism in cancer cells by ionizing radiation. Mol Cancer (2017) 16:10. doi: 10.1186/s12943-016-0577-4
16. Cheng AN, Cheng LC, Kuo CL, Lo YK, Chou HY, Chen CH, et al. Mitochondrial Lon-induced mtDNA leakage contributes to PD-L1-mediated immunoescape via STING-IFN signaling and extracellular vesicles. J Immunother Cancer (2020) 8(2):e001372. doi: 10.1136/jitc-2020-001372
17. Zou Z, Chang H, Li H, Wang S. Induction of reactive oxygen species: an emerging approach for cancer therapy. Apoptosis (2017) 22:1321–35. doi: 10.1007/s10495-017-1424-9
18. Srinivas US, Tan BWQ, Vellayappan BA, Jeyasekharan AD. ROS and the DNA damage response in cancer. Redox Biol (2019) 25:101084. doi: 10.1016/j.redox.2018.101084
19. Yu L, Liu Z, Qiu L, Hao L, Guo J. Ipatasertib sensitizes colon cancer cells to TRAIL-induced apoptosis through ROS-mediated caspase activation. Biochem Biophys Res Commun (2019) 519:812–8. doi: 10.1016/j.bbrc.2019.09.063
20. Drane P, Bravard A, Bouvard V, May E. Reciprocal down-regulation of p53 and SOD2 gene expression-implication in p53 mediated apoptosis. Oncogene (2001) 20:430–9. doi: 10.1038/sj.onc.1204101
21. Faraonio R, Vergara P, Di Marzo D, Pierantoni MG, Napolitano M, Russo T, et al. p53 suppresses the Nrf2-dependent transcription of antioxidant response genes. J Biol Chem (2006) 281:39776–84. doi: 10.1074/jbc.M605707200
22. Yu L, Yang X, Li X, Qin L, Xu W, Cui H, et al. Pink1/PARK2/mROS-dependent mitophagy initiates the sensitization of cancer cells to radiation. Oxid Med Cell Longev (2021) 2021:5595652. doi: 10.1155/2021/5595652
23. Fernandez-Gil BI, Guerra-Librero A, Shen YQ, Florido J, Martínez-Ruiz L, García-López S, et al. Melatonin enhances cisplatin and radiation cytotoxicity in head and neck squamous cell carcinoma by stimulating mitochondrial ROS generation, apoptosis, and autophagy. Oxid Med Cell Longev (2019) 2019:7187128. doi: 10.1155/2019/7187128
24. Galluzzi L, Vitale I, Aaronson SA, Abrams JM, Adam D, Agostinis P, et al. Molecular mechanisms of cell death: recommendations of the Nomenclature Committee on Cell Death 2018. Cell Death Differ (2018) 25:486–541. doi: 10.1038/s41418-017-0012-4
25. Wang Y, Chen J, Duan R, Gu R, Wang W, Wu J, et al. High-Z-sensitized radiotherapy synergizes with the intervention of the pentose phosphate pathway for in situ tumor vaccination. Adv Mater (2022) 34:e2109726. doi: 10.1002/adma.202109726
26. Li W, Yang J, Luo L, Jiang M, Qin B, Yin H, et al. Targeting photodynamic and photothermal therapy to the endoplasmic reticulum enhances immunogenic cancer cell death. Nat Commun (2019) 10:3349. doi: 10.1038/s41467-019-11269-8
27. Kepp O, Menger L, Vacchelli E, Locher C, Adjemian S, Yamazaki T, et al. Crosstalk between ER stress and immunogenic cell death. Cytokine Growth Factor Rev (2013) 24:311–8. doi: 10.1016/j.cytogfr.2013.05.001
28. Jeong SD, Jung BK, Ahn HM, Lee D, Ha J, Noh I, et al. Immunogenic cell death inducing fluorinated mitochondria-disrupting helical polypeptide synergizes with PD-L1 immune checkpoint blockade. Adv Sci (Weinh) (2021) 8:2001308. doi: 10.1002/advs.202001308
29. Xu Z, Xu J, Sun S, Lin W, Li Y, Lu Q, et al. Mecheliolide elicits ROS-mediated ERS driven immunogenic cell death in hepatocellular carcinoma. Redox Biol (2022) 54:102351. doi: 10.1016/j.redox.2022.102351
30. Oshi M, Gandhi S, Yan L, Tokumaru Y, Wu R, Yamada A, et al. Abundance of reactive oxygen species (ROS) is associated with tumor aggressiveness, immune response, and worse survival in breast cancer. Breast Cancer Res Treat (2022) 194:231–41. doi: 10.1007/s10549-022-06633-0
31. Yang W, Xiu Z, He Y, Huang W, Li Y, Sun T. Bip inhibition in glioma stem cells promotes radiation-induced immunogenic cell death. Cell Death Dis (2020) 11:786. doi: 10.1038/s41419-020-03000-z
32. Ma H, Lu Y, Huang Z, Long S, Cao J, Zhang Z, et al. ER-targeting cyanine dye as an NIR photoinducer to efficiently trigger photoimmunogenic cancer cell death. J Am Chem Soc (2022) 144:3477–86. doi: 10.1021/jacs.1c11886
33. Deng H, Zhou Z, Yang W, Lin LS, Wang S, Niu G, et al. Endoplasmic reticulum targeting to amplify immunogenic cell death for cancer immunotherapy. Nano Lett (2020) 20:1928–33. doi: 10.1021/acs.nanolett.9b05210
34. Cerniglia GJ, Dey S, Gallagher-Colombo SM, Daurio NA, Tuttle S, Busch TM, et al. The PI3K/akt pathway regulates oxygen metabolism via pyruvate dehydrogenase (PDH)-E1α Phosphorylation. Mol Cancer Ther (2015) 14:1928–38. doi: 10.1158/1535-7163.Mct-14-0888
35. Krysko O, Aaes TL, Kagan VE, D'Herde K, Bachert C, Leybaert L, et al. Necroptotic cell death in anti-cancer therapy. Immunol Rev (2017) 280:207–19. doi: 10.1111/imr.12583
36. Ecker V, Stumpf M, Brandmeier L, Neumayer T, Pfeuffer L, Engleitner T, et al. Targeted PI3K/AKT-hyperactivation induces cell death in chronic lymphocytic leukemia. Nat Commun (2021) 12:3526. doi: 10.1038/s41467-021-23752-2
37. Fang C, Mo F, Liu L, Du J, Luo M, Men K, et al. Oxidized mitochondrial DNA sensing by STING signaling promotes the antitumor effect of an irradiated immunogenic cancer cell vaccine. Cell Mol Immunol (2021) 18:2211–23. doi: 10.1038/s41423-020-0456-1
38. Redza-Dutordoir M, Averill-Bates DA. Activation of apoptosis signalling pathways by reactive oxygen species. Biochim Biophys Acta (2016) 1863:2977–92. doi: 10.1016/j.bbamcr.2016.09.012
39. Kepp O, Senovilla L, Vitale I, Vacchelli E, Adjemian S, Agostinis P, et al. Consensus guidelines for the detection of immunogenic cell death. Oncoimmunology (2014) 3:e955691. doi: 10.4161/21624011.2014.955691
40. Chandra D, Choy G, Deng X, Bhatia B, Daniel P, Tang DG. Association of active caspase 8 with the mitochondrial membrane during apoptosis: potential roles in cleaving BAP31 and caspase 3 and mediating mitochondrion-endoplasmic reticulum cross talk in etoposide-induced cell death. Mol Cell Biol (2004) 24:6592–607. doi: 10.1128/mcb.24.15.6592-6607.2004
41. Iulianna T, Kuldeep N and Eric F. The Achilles' heel of cancer: targeting tumors via lysosome-induced immunogenic cell death. Cell Death Dis (2022) 13:509. doi: 10.1038/s41419-022-04912-8
42. Yang WS, Kim KJ, Gaschler MM, Patel M, Shchepinov MS, Stockwell BR. Peroxidation of polyunsaturated fatty acids by lipoxygenases drives ferroptosis. Proc Natl Acad Sci USA (2016) 113:E4966–4975. doi: 10.1073/pnas.1603244113
43. Doll S, Proneth B, Tyurina YY, Panzilius E, Kobayashi S, Ingold I, et al. ACSL4 dictates ferroptosis sensitivity by shaping cellular lipid composition. Nat Chem Biol (2017) 13:91–8. doi: 10.1038/nchembio.2239
44. Lei G, Zhang Y, Koppula P, Liu X, Zhang J, Lin SH, et al. The role of ferroptosis in ionizing radiation-induced cell death and tumor suppression. Cell Res (2020) 30:146–62. doi: 10.1038/s41422-019-0263-3
45. Wenzel SE, Tyurina YY, Zhao J, St Croix CM, Dar HH, Mao G, et al. PEBP1 wardens ferroptosis by enabling lipoxygenase generation of lipid death signals. Cell (2017) 171:628–641.e626. doi: 10.1016/j.cell.2017.09.044
46. Brigelius-Flohé R, Maiorino M. Glutathione peroxidases. Biochim Biophys Acta (2013) 1830:3289–303. doi: 10.1016/j.bbagen.2012.11.020
47. Zhao L, Zhou X, Xie F, Zhang L, Yan H, Huang J, et al. Ferroptosis in cancer and cancer immunotherapy. Cancer Commun (Lond) (2022) 42:88–116. doi: 10.1002/cac2.12250
48. Wang H, An P, Xie E, Wu Q, Fang X, Gao H, et al. Characterization of ferroptosis in murine models of hemochromatosis. Hepatology (2017) 66:449–65. doi: 10.1002/hep.29117
49. Chen PH, Wu J, Ding CC, Lin CC, Pan S, Bossa N, et al. Kinome screen of ferroptosis reveals a novel role of ATM in regulating iron metabolism. Cell Death Differ (2020) 27:1008–22. doi: 10.1038/s41418-019-0393-7
50. Wang W, Green M, Choi JE, Gijón M, Kennedy PD, Johnson JK, et al. CD8(+) T cells regulate tumour ferroptosis during cancer immunotherapy. Nature (2019) 569:270–4. doi: 10.1038/s41586-019-1170-y
51. Guo W, Wu Z, Chen J, Guo S, You W, Wang S, et al. Nanoparticle delivery of miR-21-3p sensitizes melanoma to anti-PD-1 immunotherapy by promoting ferroptosis. J Immunother Cancer (2022) 10(6):e004381. doi: 10.1136/jitc-2021-004381
52. Lang X, Green MD, Wang W, Yu J, Choi JE, Jiang L, et al. Radiotherapy and immunotherapy promote tumoral lipid oxidation and ferroptosis via synergistic repression of SLC7A11. Cancer Discov (2019) 9:1673–85. doi: 10.1158/2159-8290.Cd-19-0338
53. Yee PP, Wei Y, Kim SY, Lu T, Chih SY, Lawson C, et al. Neutrophil-induced ferroptosis promotes tumor necrosis in glioblastoma progression. Nat Commun (2020) 11:5424. doi: 10.1038/s41467-020-19193-y
54. Ma X, Xiao L, Liu L, Ye L, Su P, Bi E, et al. CD36-mediated ferroptosis dampens intratumoral CD8(+) T cell effector function and impairs their antitumor ability. Cell Metab (2021) 33:1001–1012.e1005. doi: 10.1016/j.cmet.2021.02.015
55. Mougiakakos D, Johansson CC, Jitschin R, Böttcher M, Kiessling R. Increased thioredoxin-1 production in human naturally occurring regulatory T cells confers enhanced tolerance to oxidative stress. Blood (2011) 117:857–61. doi: 10.1182/blood-2010-09-307041
56. Xu H, Ye D, Ren M, Zhang H, Bi F. Ferroptosis in the tumor microenvironment: perspectives for immunotherapy. Trends Mol Med (2021) 27:856–67. doi: 10.1016/j.molmed.2021.06.014
57. Hsieh CH, Hsieh HC, Shih FS, Wang PW, Yang LX, Shieh DB, et al. An innovative NRF2 nano-modulator induces lung cancer ferroptosis and elicits an immunostimulatory tumor microenvironment. Theranostics (2021) 11:7072–91. doi: 10.7150/thno.57803
58. Dai E, Han L, Liu J, Xie Y, Kroemer G, Klionsky DJ, et al. Autophagy-dependent ferroptosis drives tumor-associated macrophage polarization via release and uptake of oncogenic KRAS protein. Autophagy (2020) 16:2069–83. doi: 10.1080/15548627.2020.1714209
59. Poznanski SM, Singh K, Ritchie TM, Aguiar JA, Fan IY, Portillo AL, et al. Metabolic flexibility determines human NK cell functional fate in the tumor microenvironment. Cell Metab (2021) 33:1205–1220.e1205. doi: 10.1016/j.cmet.2021.03.023
60. Bai Y, Meng L, Han L, Jia Y, Zhao Y, Gao H, et al. Lipid storage and lipophagy regulates ferroptosis. Biochem Biophys Res Commun (2019) 508:997–1003. doi: 10.1016/j.bbrc.2018.12.039
61. Li Q, Su R, Bao X, Cao K, Du Y, Wang N, et al. Glycyrrhetinic acid nanoparticles combined with ferrotherapy for improved cancer immunotherapy. Acta Biomater (2022) 144:109–20. doi: 10.1016/j.actbio.2022.03.030
62. Oweida A, Lennon S, Calame D, Korpela S, Bhatia S, Sharma J, et al. Ionizing radiation sensitizes tumors to PD-L1 immune checkpoint blockade in orthotopic murine head and neck squamous cell carcinoma. Oncoimmunology (2017) 6:e1356153. doi: 10.1080/2162402x.2017.1356153
63. Kelly RJ, Zaidi AH, Smith MA, Omstead AN, Kosovec JE, Matsui D, et al. The dynamic and transient immune microenvironment in locally advanced esophageal adenocarcinoma post chemoradiation. Ann Surg (2018) 268:992–9. doi: 10.1097/sla.0000000000002410
64. Bailly C. Regulation of PD-L1 expression on cancer cells with ROS-modulating drugs. Life Sci (2020) 246:117403. doi: 10.1016/j.lfs.2020.117403
65. Roux C, Jafari SM, Shinde R, Duncan G, Cescon DW, Silvester J, et al. Reactive oxygen species modulate macrophage immunosuppressive phenotype through the up-regulation of PD-L1. Proc Natl Acad Sci USA (2019) 116:4326–35. doi: 10.1073/pnas.1819473116
66. Glorieux C, Xia X, He YQ, Hu Y, Cremer K, Robert A, et al. Regulation of PD-L1 expression in K-ras-driven cancers through ROS-mediated FGFR1 signaling. Redox Biol (2021) 38:101780. doi: 10.1016/j.redox.2020.101780
67. Sato H, Niimi A, Yasuhara T, Permata TBM, Hagiwara Y, Isono M, et al. DNA double-strand break repair pathway regulates PD-L1 expression in cancer cells. Nat Commun (2017) 8:1751. doi: 10.1038/s41467-017-01883-9
68. Reisländer T, Groelly FJ and Tarsounas M. DNA damage and cancer immunotherapy: A STING in the tale. Mol Cell (2020) 80:21–8. doi: 10.1016/j.molcel.2020.07.026
69. Du SS, Chen GW, Yang P, Chen YX, Hu Y, Zhao QQ, et al. Radiation Therapy Promotes Hepatocellular Carcinoma Immune Cloaking via PD-L1 Upregulation Induced by cGAS-STING Activation. Int J Radiat Oncol Biol Phys (2022) 112:1243–55. doi: 10.1016/j.ijrobp.2021.12.162
70. Hsieh RC, Krishnan S, Wu RC, Boda AR, Liu A, Winkler M, et al. ATR-mediated CD47 and PD-L1 up-regulation restricts radiotherapy-induced immune priming and abscopal responses in colorectal cancer. Sci Immunol (2022) 7:eabl9330. doi: 10.1126/sciimmunol.abl9330
71. Li Z, Li Y, Gao J, Fu Y, Hua P, Jing Y, et al. The role of CD47-SIRPα immune checkpoint in tumor immune evasion and innate immunotherapy. Life Sci (2021) 273:119150. doi: 10.1016/j.lfs.2021.119150
72. Shueng PW, Yu LY, Chiu HC, Chang HC, Chiu YL, Kuo TY, et al. Early phago-/endosomal escape of platinum drugs via ROS-responsive micelles for dual cancer chemo/immunotherapy. Biomaterials (2021) 276:121012. doi: 10.1016/j.biomaterials.2021.121012
73. Zhang H, Lu H, Xiang L, Bullen JW, Zhang C, Samanta D, et al. HIF-1 regulates CD47 expression in breast cancer cells to promote evasion of phagocytosis and maintenance of cancer stem cells. Proc Natl Acad Sci USA (2015) 112:E6215–6223. doi: 10.1073/pnas.1520032112
74. Li W, Wang L, Shen C, Xu T, Chu Y, Hu C. Radiation therapy-induced reactive oxygen species specifically eliminates CD19(+)IgA(+) B cells in nasopharyngeal carcinoma. Cancer Manag Res (2019) 11:6299–309. doi: 10.2147/cmar.S202375
75. Diebold L, Chandel NS. Mitochondrial ROS regulation of proliferating cells. Free Radic Biol Med (2016) 100:86–93. doi: 10.1016/j.freeradbiomed.2016.04.198
76. Wu Z, Huang H, Han Q, Hu Z, Teng XL, Ding R, et al. SENP7 senses oxidative stress to sustain metabolic fitness and antitumor functions of CD8+ T cells. J Clin Invest (2022) 132(7):e155224. doi: 10.1172/jci155224
77. Xiao L, Ma X, Ye L, Su P, Xiong W, Bi E, et al. IL-9/STAT3/fatty acid oxidation-mediated lipid peroxidation contributes to Tc9 cell longevity and enhanced antitumor activity. J Clin Invest (2022) 132(7):e153247. doi: 10.1172/jci153247
78. Li X, Wang S, Mu W, Barry J, Han A, Carpenter RL, et al. Reactive oxygen species reprogram macrophages to suppress antitumor immune response through the exosomal miR-155-5p/PD-L1 pathway. J Exp Clin Cancer Res (2022) 41:41. doi: 10.1186/s13046-022-02244-1
79. Bhattacharyya S, Saha J. Tumour, oxidative stress and host T cell response: cementing the dominance. Scand J Immunol (2015) 82:477–88. doi: 10.1111/sji.12350
80. Scharping NE, Rivadeneira DB, Menk AV, Vignali PDA, Ford BR, Rittenhouse NL, et al. Mitochondrial stress induced by continuous stimulation under hypoxia rapidly drives T cell exhaustion. Nat Immunol (2021) 22:205–15. doi: 10.1038/s41590-020-00834-9
81. Mohanty S, Barik P, Debata N, Nagarajan P, Devadas S. iCa(2+) flux, ROS and IL-10 determines cytotoxic, and suppressor T cell functions in chronic human viral infections. Front Immunol (2020) 11:83. doi: 10.3389/fimmu.2020.00083
82. King MR, Ismail AS, Davis LS, Karp DR. Oxidative stress promotes polarization of human T cell differentiation toward a T helper 2 phenotype. J Immunol (2006) 176:2765–72. doi: 10.4049/jimmunol.176.5.2765
83. Bhattacharyya S, Mandal D, Sen GS, Pal S, Banerjee S, Lahiry L, et al. Tumor-induced oxidative stress perturbs nuclear factor-kappaB activity-augmenting tumor necrosis factor-alpha-mediated T-cell death: protection by curcumin. Cancer Res (2007) 67:362–70. doi: 10.1158/0008-5472.Can-06-2583
84. Maj T, Wang W, Crespo J, Zhang H, Wang W, Wei S, et al. Oxidative stress controls regulatory T cell apoptosis and suppressor activity and PD-L1-blockade resistance in tumor. Nat Immunol (2017) 18:1332–41. doi: 10.1038/ni.3868
85. Patwardhan RS, Singh B, Pal D, Checker R, Bandekar M, Sharma D, et al. Redox regulation of regulatory T-cell differentiation and functions. Free Radic Res (2020) 54:947–60. doi: 10.1080/10715762.2020.1745202
86. Yan Z, Garg SK and Banerjee R. Regulatory T cells interfere with glutathione metabolism in dendritic cells and T cells. J Biol Chem (2010) 285:41525–32. doi: 10.1074/jbc.M110.189944
87. Paardekooper LM, Vos W, van den Bogaart G. Oxygen in the tumor microenvironment: effects on dendritic cell function. Oncotarget (2019) 10:883–96. doi: 10.18632/oncotarget.26608
88. Matsue H, Edelbaum D, Shalhevet D, Mizumoto N, Yang C, Mummert ME, et al. Generation and function of reactive oxygen species in dendritic cells during antigen presentation. J Immunol (2003) 171:3010–8. doi: 10.4049/jimmunol.171.6.3010
89. Bauer M, Goldstein M, Christmann M, Becker H, Heylmann D, Kaina B. Human monocytes are severely impaired in base and DNA double-strand break repair that renders them vulnerable to oxidative stress. Proc Natl Acad Sci USA (2011) 108:21105–10. doi: 10.1073/pnas.1111919109
90. Graciotti M, Marino F, Pak H, Baumgaertner P, Thierry AC, Chiffelle J, et al. Deciphering the mechanisms of improved immunogenicity of hypochlorous acid-treated antigens in anti-cancer dendritic cell-based vaccines. Vaccines (Basel) (2020) 8:20200602. doi: 10.3390/vaccines8020271
91. Lee W, Kingstad-Bakke B, Paulson B, Larsen A, Overmyer K, Marinaik CB, et al. Carbomer-based adjuvant elicits CD8 T-cell immunity by inducing a distinct metabolic state in cross-presenting dendritic cells. PloS Pathog (2021) 17:e1009168. doi: 10.1371/journal.ppat.1009168
92. Cheong TC, Shin EP, Kwon EK, Choi JH, Wang KK, Sharma P, et al. Functional manipulation of dendritic cells by photoswitchable generation of intracellular reactive oxygen species. ACS Chem Biol (2015) 10:757–65. doi: 10.1021/cb5009124
93. Deng L, Liang H, Xu M, Yang X, Burnette B, Arina A, et al. STING-dependent cytosolic DNA sensing promotes radiation-induced type I interferon-dependent antitumor immunity in immunogenic tumors. Immunity (2014) 41:843–52. doi: 10.1016/j.immuni.2014.10.019
94. Hu Z, Teng XL, Zhang T, Yu X, Ding R, Yi J, et al. SENP3 senses oxidative stress to facilitate STING-dependent dendritic cell antitumor function. Mol Cell (2021) 81:940–952.e945. doi: 10.1016/j.molcel.2020.12.024
95. Ma J, Wei K, Zhang H, Tang K, Li F, Zhang T, et al. Mechanisms by which dendritic cells present tumor microparticle antigens to CD8(+) T cells. Cancer Immunol Res (2018) 6:1057–68. doi: 10.1158/2326-6066.Cir-17-0716
96. Wan C, Sun Y, Tian Y, Lu L, Dai X, Meng J, et al. Irradiated tumor cell-derived microparticles mediate tumor eradication via cell killing and immune reprogramming. Sci Adv (2020) 6(13):eaay9789. doi: 10.1126/sciadv.aay9789
97. Gilardini Montani MS, Granato M, Cuomo L, Valia S, Di Renzo L, D'Orazi G, et al. High glucose and hyperglycemic sera from type 2 diabetic patients impair DC differentiation by inducing ROS and activating Wnt/β-catenin and p38 MAPK. Biochim Biophys Acta (2016) 1862:805–13. doi: 10.1016/j.bbadis.2016.01.001
98. Gondi CS, Rao JS. Cathepsin B as a cancer target. Expert Opin Ther Targets (2013) 17:281–91. doi: 10.1517/14728222.2013.740461
99. Mao D, Hu F, Yi Z, Kenry, Xu S, Yan S, et al. AIEgen-coupled upconversion nanoparticles eradicate solid tumors through dual-mode ROS activation. Sci Adv (2020) 6:eabb2712. doi: 10.1126/sciadv.abb2712
100. Cubillos-Ruiz JR, Silberman PC, Rutkowski MR, Chopra S, Perales-Puchalt A, Song M, et al. ER stress sensor XBP1 controls anti-tumor immunity by disrupting dendritic cell homeostasis. Cell (2015) 161:1527–38. doi: 10.1016/j.cell.2015.05.025
101. Oberkampf M, Guillerey C, Mouriès J, Rosenbaum P, Fayolle C, Bobard A, et al. Mitochondrial reactive oxygen species regulate the induction of CD8(+) T cells by plasmacytoid dendritic cells. Nat Commun (2018) 9:2241. doi: 10.1038/s41467-018-04686-8
102. Abel AM, Yang C, Thakar MS, Malarkannan S. Natural killer cells: development, maturation, and clinical utilization. Front Immunol (2018) 9:1869. doi: 10.3389/fimmu.2018.01869
103. Song H, Park H, Kim YS, Kim KD, Lee HK, Cho DH, et al. L-kynurenine-induced apoptosis in human NK cells is mediated by reactive oxygen species. Int Immunopharmacol (2011) 11:932–8. doi: 10.1016/j.intimp.2011.02.005
104. Yang Y, Neo SY, Chen Z, Cui W, Chen Y, Guo M, et al. Thioredoxin activity confers resistance against oxidative stress in tumor-infiltrating NK cells. J Clin Invest (2020) 130:5508–22. doi: 10.1172/jci137585
105. Grauers Wiktorin H, Aydin E, Hellstrand K, Martner A. NOX2-derived reactive oxygen species in cancer. Oxid Med Cell Longev (2020) 2020:7095902. doi: 10.1155/2020/7095902
106. Zhu Y, Zhao Z, Xue M, Wang D, Su G, Ju X, et al. Ciclopirox olamine sensitizes leukemia cells to natural killer cell-mediated cytolysis by upregulating NKG2DLs via the Akt signaling pathway. Biochem Biophys Res Commun (2023) 659:10–9. doi: 10.1016/j.bbrc.2023.03.062
107. Jin F, Wu Z, Hu X, Zhang J, Gao Z, Han X, et al. The PI3K/Akt/GSK-3β/ROS/eIF2B pathway promotes breast cancer growth and metastasis via suppression of NK cell cytotoxicity and tumor cell susceptibility. Cancer Biol Med (2019) 16:38–54. doi: 10.20892/j.issn.2095-3941.2018.0253
108. Zhang Y, Choksi S, Chen K, Pobezinskaya Y, Linnoila I, Liu ZG. ROS play a critical role in the differentiation of alternatively activated macrophages and the occurrence of tumor-associated macrophages. Cell Res (2013) 23:898–914. doi: 10.1038/cr.2013.75
109. Trzeciak ER, Zimmer N, Gehringer I, Stein L, Graefen B, Schupp J, et al. Oxidative stress differentially influences the survival and metabolism of cells in the melanoma microenvironment. Cells (2022) 11:20220308. doi: 10.3390/cells11060930
110. Li G, Liu D, Kimchi ET, Kaifi JT, Qi X, Manjunath Y, et al. Nanoliposome C6-ceramide increases the anti-tumor immune response and slows growth of liver tumors in mice. Gastroenterology (2018) 154:1024–1036.e1029. doi: 10.1053/j.gastro.2017.10.050
111. Shiau DJ, Kuo WT, Davuluri GVN, Shieh CC, Tsai PJ, Chen CC, et al. Hepatocellular carcinoma-derived high mobility group box 1 triggers M2 macrophage polarization via a TLR2/NOX2/autophagy axis. Sci Rep (2020) 10:13582. doi: 10.1038/s41598-020-70137-4
112. Lin X, Zheng W, Liu J, Zhang Y, Qin H, Wu H, et al. Oxidative stress in malignant melanoma enhances tumor necrosis factor-α secretion of tumor-associated macrophages that promote cancer cell invasion. Antioxid Redox Signal (2013) 19:1337–55. doi: 10.1089/ars.2012.4617
113. Owusu SB, Hudik E, Férard C, Dupré-Crochet S, Addison E, Preko K, et al. Radiation-induced reactive oxygen species partially assemble neutrophil NADPH oxidase. Free Radic Biol Med (2021) 164:76–84. doi: 10.1016/j.freeradbiomed.2020.12.233
114. Takeshima T, Pop LM, Laine A, Iyengar P, Vitetta ES, Hannan R. Key role for neutrophils in radiation-induced antitumor immune responses: Potentiation with G-CSF. Proc Natl Acad Sci USA (2016) 113:11300–5. doi: 10.1073/pnas.1613187113
115. Kusmartsev S, Gabrilovich DI. Inhibition of myeloid cell differentiation in cancer: the role of reactive oxygen species. J Leukoc Biol (2003) 74:186–96. doi: 10.1189/jlb.0103010
116. Corzo CA, Cotter MJ, Cheng P, Cheng F, Kusmartsev S, Sotomayor E, et al. Mechanism regulating reactive oxygen species in tumor-induced myeloid-derived suppressor cells. J Immunol (2009) 182:5693–701. doi: 10.4049/jimmunol.0900092
117. Condamine T, Dominguez GA, Youn JI, Kossenkov AV, Mony S, Alicea-Torres K, et al. Lectin-type oxidized LDL receptor-1 distinguishes population of human polymorphonuclear myeloid-derived suppressor cells in cancer patients. Sci Immunol (2016) 1:20160805. doi: 10.1126/sciimmunol.aaf8943
118. Chen X, Cubillos-Ruiz JR. Endoplasmic reticulum stress signals in the tumour and its microenvironment. Nat Rev Cancer (2021) 21:71–88. doi: 10.1038/s41568-020-00312-2
119. Nagaraj S, Gupta K, Pisarev V, Kinarsky L, Sherman S, Kang L, et al. Altered recognition of antigen is a mechanism of CD8+ T cell tolerance in cancer. Nat Med (2007) 13:828–35. doi: 10.1038/nm1609
120. Shimura T, Sasatani M, Kawai H, Kamiya K, Kobayashi J, Komatsu K, et al. Radiation-induced myofibroblasts promote tumor growth via mitochondrial ROS-activated TGFβ Signaling. Mol Cancer Res (2018) 16:1676–86. doi: 10.1158/1541-7786.Mcr-18-0321
121. Wang Y, Gan G, Wang B, Wu J, Cao Y, Zhu D, et al. Cancer-associated fibroblasts promote irradiated cancer cell recovery through autophagy. EBioMedicine (2017) 17:45–56. doi: 10.1016/j.ebiom.2017.02.019
122. Ford K, Hanley CJ, Mellone M, Szyndralewiez C, Heitz F, Wiesel P, et al. NOX4 inhibition potentiates immunotherapy by overcoming cancer-associated fibroblast-mediated CD8 T-cell exclusion from tumors. Cancer Res (2020) 80:1846–60. doi: 10.1158/0008-5472.Can-19-3158
123. Xiang H, Ramil CP, Hai J, Zhang C, Wang H, Watkins AA, et al. Cancer-associated fibroblasts promote immunosuppression by inducing ROS-generating monocytic MDSCs in lung squamous cell carcinoma. Cancer Immunol Res (2020) 8:436–50. doi: 10.1158/2326-6066.Cir-19-0507
124. Chen MH, Liu TY, Chen YC, Chen MH. Combining augmented radiotherapy and immunotherapy through a nano-gold and bacterial outer-membrane vesicle complex for the treatment of glioblastoma. Nanomaterials (Basel) (2021) 11:20210624. doi: 10.3390/nano11071661
125. Cho JH, Lee HJ, Ko HJ, Yoon BI, Choe J, Kim KC, et al. The TLR7 agonist imiquimod induces anti-cancer effects via autophagic cell death and enhances anti-tumoral and systemic immunity during radiotherapy for melanoma. Oncotarget (2017) 8:24932–48. doi: 10.18632/oncotarget.15326
126. Li C, Zhang Y, Yan S, Zhang G, Wei W, Qi Z, et al. Alternol triggers immunogenic cell death via reactive oxygen species generation. Oncoimmunology (2021) 10:1952539. doi: 10.1080/2162402x.2021.1952539
127. Sang W, Xie L, Wang G, Li J, Zhang Z, Li B, et al. Oxygen-enriched metal-phenolic X-ray nanoprocessor for cancer radio-radiodynamic therapy in combination with checkpoint blockade immunotherapy. Adv Sci (Weinh) (2021) 8:2003338. doi: 10.1002/advs.202003338
128. Liu Z, Zhao Q, Zheng Z, Liu S, Meng L, Dong L, et al. Vascular normalization in immunotherapy: A promising mechanisms combined with radiotherapy. BioMed Pharmacother (2021) 139:111607. doi: 10.1016/j.biopha.2021.111607
129. Fukumura D, Kloepper J, Amoozgar Z, Duda DG, Jain RK. Enhancing cancer immunotherapy using antiangiogenics: opportunities and challenges. Nat Rev Clin Oncol (2018) 15:325–40. doi: 10.1038/nrclinonc.2018.29
130. Luo B, Zhang S, Tan D, Yu X, Lin J, Wang M. Anlotinib benefits the αPDL1 immunotherapy by activating ROS/JNK/AP-1 pathway to upregulate PDL1 expression in colorectal cancer. Oxid Med Cell Longev (2022) 2022:8965903. doi: 10.1155/2022/8965903
131. Dolmans DE, Fukumura D, Jain RK. Photodynamic therapy for cancer. Nat Rev Cancer (2003) 3:380–7. doi: 10.1038/nrc1071
132. Kim D, Lee S and Na K. Immune stimulating antibody-photosensitizer conjugates via Fc-mediated dendritic cell phagocytosis and phototriggered immunogenic cell death for KRAS-mutated pancreatic cancer treatment. Small (2021) 17:e2006650. doi: 10.1002/smll.202006650
133. Min Y, Roche KC, Tian S, Eblan MJ, McKinnon KP, Caster JM, et al. Antigen-capturing nanoparticles improve the abscopal effect and cancer immunotherapy. Nat Nanotechnol (2017) 12:877–82. doi: 10.1038/nnano.2017.113
134. Zeng JY, Zou MZ, Zhang M, Wang XS, Zeng X, Cong H, et al. π-Extended benzoporphyrin-based metal-organic framework for inhibition of tumor metastasis. ACS Nano (2018) 12:4630–40. doi: 10.1021/acsnano.8b01186
135. Ni K, Luo T, Lan G, Culbert A, Song Y, Wu T, et al. A nanoscale metal-organic framework to mediate photodynamic therapy and deliver CpG oligodeoxynucleotides to enhance antigen presentation and cancer immunotherapy. Angew Chem Int Ed Engl (2020) 59:1108–12. doi: 10.1002/anie.201911429
136. He C, Duan X, Guo N, Chan C, Poon C, Weichselbaum RR, et al. Core-shell nanoscale coordination polymers combine chemotherapy and photodynamic therapy to potentiate checkpoint blockade cancer immunotherapy. Nat Commun (2016) 7:12499. doi: 10.1038/ncomms12499
137. Yuan Z, Fan G, Wu H, Liu C, Zhan Y, Qiu Y, et al. Photodynamic therapy synergizes with PD-L1 checkpoint blockade for immunotherapy of CRC by multifunctional nanoparticles. Mol Ther (2021) 29:2931–48. doi: 10.1016/j.ymthe.2021.05.017
138. Viswanath D, Won YY. Combining radiotherapy (RT) and photodynamic therapy (PDT): clinical studies on conventional RT-PDT approaches and novel nanoparticle-based RT-PDT approaches under preclinical evaluation. ACS Biomater Sci Eng (2022) 8:3644–58. doi: 10.1021/acsbiomaterials.2c00287
139. Wang GD, Nguyen HT, Chen H, Cox PB, Wang L, Nagata K, et al. X-ray induced photodynamic therapy: A combination of radiotherapy and photodynamic therapy. Theranostics (2016) 6:2295–305. doi: 10.7150/thno.16141
140. Wang Y, Ding Y, Yao D, Dong H, Ji C, Wu J, et al. Copper-based nanoscale coordination polymers augmented tumor radioimmunotherapy for immunogenic cell death induction and T-cell infiltration. Small (2021) 17:e2006231. doi: 10.1002/smll.202006231
141. Gong X, Li J, Xu X, Wu Y, Lei Y, Liu H, et al. Microvesicle-inspired oxygen-delivering nanosystem potentiates radiotherapy-mediated modulation of tumor stroma and antitumor immunity. Biomaterials (2022) 290:121855. doi: 10.1016/j.biomaterials.2022.121855
142. Pan P, Dong X, Chen Y, Ye JJ, Sun YX, Zhang XZ. A heterogenic membrane-based biomimetic hybrid nanoplatform for combining radiotherapy and immunotherapy against breast cancer. Biomaterials (2022) 289:121810. doi: 10.1016/j.biomaterials.2022.121810
143. Choi E, Landry M, Pennock N, Neufeld M, Weinfurter K, Goforth A, et al. Nanoscale hafnium metal-organic frameworks enhance radiotherapeutic effects by upregulation of type I interferon and TLR7 expression. Adv Healthc Mater (2023) 12(13):e2202830. doi: 10.1002/adhm.202202830
144. Wang J, Li J, Wu Y, Xu X, Qian X, Lei Y, et al. ROS-responsive nanocomplex of aPD-L1 and cabazitaxel improves intratumor delivery and potentiates radiation-mediated antitumor immunity. Nano Lett (2022) 22:8312–20. doi: 10.1021/acs.nanolett.2c03227
145. Eckert F, Zwirner K, Boeke S, Thorwarth D, Zips D, Huber SM. Rationale for combining radiotherapy and immune checkpoint inhibition for patients with hypoxic tumors. Front Immunol (2019) 10:407. doi: 10.3389/fimmu.2019.00407
146. Mudassar F, Shen H, Cook KM, Hau E. Improving the synergistic combination of programmed death-1/programmed death ligand-1 blockade and radiotherapy by targeting the hypoxic tumour microenvironment. J Med Imaging Radiat Oncol (2022) 66:560–74. doi: 10.1111/1754-9485.13416
147. Herrera-Campos AB, Zamudio-Martinez E, Delgado-Bellido D, Fernández-Cortés M, Montuenga LM, Oliver FJ, et al. Implications of hyperoxia over the tumor microenvironment: an overview highlighting the importance of the immune system. Cancers (Basel) (2022) 14:20220531. doi: 10.3390/cancers14112740
Keywords: radiotherapy, reactive oxygen species, oxidative stress, tumor immune microenvironment, immunotherapy
Citation: Zheng Z, Su J, Bao X, Wang H, Bian C, Zhao Q and Jiang X (2023) Mechanisms and applications of radiation-induced oxidative stress in regulating cancer immunotherapy. Front. Immunol. 14:1247268. doi: 10.3389/fimmu.2023.1247268
Received: 25 June 2023; Accepted: 21 July 2023;
Published: 04 August 2023.
Edited by:
Yafeng He, National Heart, Lung, and Blood Institute (NIH), United StatesReviewed by:
Qiao Qiao, China Medical University, ChinaYujin Xu, University of Chinese Academy of Sciences, China
Copyright © 2023 Zheng, Su, Bao, Wang, Bian, Zhao and Jiang. This is an open-access article distributed under the terms of the Creative Commons Attribution License (CC BY). The use, distribution or reproduction in other forums is permitted, provided the original author(s) and the copyright owner(s) are credited and that the original publication in this journal is cited, in accordance with accepted academic practice. No use, distribution or reproduction is permitted which does not comply with these terms.
*Correspondence: Qin Zhao, amx1emhhb3FpbjA5QGpsdS5lZHUuY24=; Xin Jiang, amlhbmd4QGpsdS5lZHUuY24=
†These authors have contributed equally to this work
‡ORCID: Xin Jiang, orcid.org/0000-0002-4613-7438