- 1Jiangsu Key Laboratory of Immunity and Metabolism, Jiangsu International Key Laboratory of Immunity and Metabolism, Department of Pathogen Biology and Immunology, Xuzhou Medical University, Xuzhou, Jiangsu, China
- 2The Second Clinical Medical College, Xuzhou Medical University, Xuzhou, Jiangsu, China
- 3Liangshan College (Li Shui) China, Lishui University, Lishui, Zhejiang, China
The preventive situation of parasitosis, a global public health burden especially for developing countries, is not looking that good. Similar to other infections, vaccines would be the best choice for preventing and controlling parasitic infection. However, ideal antigenic molecules for vaccine development have not been identified so far, resulting from the complicated life history and enormous genomes of the parasites. Furthermore, the suppression or down-regulation of anti-infectious immunity mediated by the parasites or their derived molecules can compromise the effect of parasitic vaccines. Comparing the early immune profiles of several parasites in the permissive and non-permissive hosts, a robust innate immune response is proposed to be a critical event to eliminate the parasites. Therefore, enhancing innate immunity may be essential for designing novel and effective parasitic vaccines. The newly emerging trained immunity (also termed innate immune memory) has been increasingly recognized to provide a novel perspective for vaccine development targeting innate immunity. This article reviews the current status of parasitic vaccines and anti-infectious immunity, as well as the conception, characteristics, and mechanisms of trained immunity and its research progress in Parasitology, highlighting the possible consideration of trained immunity in designing novel vaccines against parasitic diseases.
Introduction
As one of the most entrenched pathogens in the world, parasites have been posing severe threats to both individual health and the social economy for a long history. In 2018, malaria accounted for an estimated 228 million cases and 405,000 deaths globally, with even more people at considerable risk. According to the report of the World Health Organization (WHO), 11 parasitic diseases have been listed as Neglected Tropical Diseases (NTD) that infect over 1 billion people worldwide (1). Parasitic diseases have been threatening human health profoundly, causing physical pain and disability of substantial populations, especially in marginalized areas with poor sanitation and a backward economy. Therefore, reasonable solutions to control the global burden of parasitic diseases make great significance in individual and social welfare by improving health conditions, promoting productivity, slashing medical expenses, and alleviating general poverty.
Generally, the prevention and control strategies for parasitic diseases mainly rely on eliminating infection sources, blocking transmission routes, and protecting susceptible populations. Drug treatment has been the fundamental therapeutic avenue for quite some time. However, accumulating studies have reported drug resistance in both ecto- and endo-parasites, which undoubtedly impedes curing parasitic diseases (2–4). Other preventive measures (e.g., fecal management, water purification, pesticide sprays, intermediate host elimination) are labor and time-consuming, as well as financial resources. With the development of modern technology, a series of new avenues have been employed to improve efficiency (5–7). However, wide hygiene-based measures are crucial for the fight against parasitic diseases, and additional measures are needed to bring them under control.
Featuring simplicity, effectiveness, and long-term preventive capability, vaccines are undoubtedly a vital tool for controlling infectious diseases on a large scale and compensate for the deficiency of drug and surgical treatment to a large extent. Historically, the success of smallpox and polio vaccines has enabled us to eliminate these diseases. However, it is frustrating that no commercialized parasitic vaccine is available for humans, merely with several vaccines under different stages of clinical trials. RTS, S/AS01 (Mosquirix, GlaxoSmithKline) is the best example of a parasitic vaccine, the only malaria vaccine in the phase 3 clinical trial. It is reported that within 12 months after vaccination, the vaccine can halve the parasitic incidence in children aged 5 - 17 months and provide protection against severe malaria for children in Africa (8). However, the protective efficacy is only 28.3% among infants who received three doses and 36.3% for those given a fourth dose (9). Notably, a follow-up study over 7 years shows the vaccine efficacy wanes significantly over time, with 3.6% at year 7 in the low-exposure cohort and a negative rate at year 5 in the high-exposure cohort (10). Based on recent findings, a new generation of vaccine candidates has been developed, which contains antigenic domains similar to the RTS, S vaccine and can be expressed on mRNA or nanoparticles. According to the latest human trials in Burkina Faso with children injected with the nanoparticle R21 vaccine, the initial results showed 77% protection against severe malaria 1 year after 3 doses of the vaccine in children (11). Another example is the current status of the schistosomiasis vaccine. S. haematobium 28-kD glutathione S-transferase (rSh28GST), the first vaccine to enter a clinical trial for urinary schistosomiasis, has failed due to its lower safety and immunogenicity (12). Moreover, another three candidates (S. mansoni 14-kDa cytoplasmic fatty acid-binding protein, Sm14; S. mansoni 9-kDa tetraspanin surface protein, Sm-TSP-2; S. mansoni surface calpain, Sm-p80) are now under varying stages of clinical trials, of which the protective efficacy is unavailable and still needs further estimation (13–15).
With the development of research, several novel vaccines have emerged as a hot spot in parasitic vaccine development. Intriguingly, DNA vaccines effectively induce cell-mediated Th1 immune responses with increased proliferation of CD4+ T cells and CD8+ T cells in animals, and have a higher safety profile than vector vaccines (16–23). Additionally, DNA vaccines have a superior performance in terms of stability at ambient temperatures, rapid adaptation to new targets, and cost-effectiveness (18, 24). For example, the DNA vaccine pVIVO2-Sj23.EGFP against S. japonicum has a systemic and long-lasting protective effect and is safe for gut microbiota in mice (25). In the preclinical context, DNA vaccines have been developed against Schistosoma spp (26–29)., hookworms (30), and Fasciola hepatica (31). Unfortunately, many of these vaccines exhibit little reduction in parasite loads. Recently, the recombinant protein enhancing Sm-p80 DNA vaccine with CpG dinucleotides as an adjuvant, shows the most significant protective effect, reducing the parasite burden of S. mansoni by 47.34% (32). Moreover, viral vectored vaccines use infectious agents to deliver the vaccine target, leading to a potent cellular immune response, particularly CD8+ cytotoxic T cells (16). What’s more, viral vectored vaccines can mimic natural infections and are highly immunogenic (33). It is encouraging to note that viral vectored vaccines have been developed against malaria (34), Echinococcus granulosus (35) and taeniasis (36).
Despite the inability of the human host to develop a naturally acquired immune response to hookworms, it is feasible to develop a hookworm vaccine based on the success of immunizing experimental animals with an attenuated larval vaccine or with antigens extracted from the alimentary canal of adult blood-feeding stages (37). However, only recombinant proteins produced in eukaryotic expression systems (e.g., insect cells, baculoviruses, and yeast) are immunologically similar to the corresponding natural antigens (37). A challenge for vaccinologists is to formulate selected eukaryotic antigens with suitable adjuvants to induce the production of high litres of antibodies. Furthermore, veterinary vaccines can reduce disease prevalence in humans and animals. For example, Listeria monocytogenes vaccines expressing the tachyzoite surface antigen NcSAG1 (Lm3Dx_SAG1) plus kinase inhibitor BKI-1748, apical membrane antigen 1 (AMA-1) vaccine candidate, Trichobovis vaccine and Toxoplasma. gondii bradyzoite-formation deficient 1 (TgBFD1) vaccine candidate have all made significant progress in animals (38).
Insufficient efficacy is the major obstacle to the success of human parasite vaccines. For the unique biological characteristics of parasites, parasitic genomes, antigenic components, and life cycles are of more complexity and diversity in contrast with bacteria and viruses. Parasites express specific antigens and elicit differential host responses at different life stages. As a result, most parasitic antigens only induce partial protective immunity, which undoubtedly aggravates the difficulty in finding a qualified vaccine candidate to induce long-term, specific, and effective immune responses (39). On the other hand, many parasites utilize advanced strategies to regulate the host’s metabolism and immunity (40), compromising parasitic vaccines’ effect (41). Considering the status of the current parasitic vaccine, it is, therefore, essential to boost vaccines’ protective effect by other pathways. Trained Immunity (TI), also termed Innate Immune Memory, has attracted our interest because the novel discipline has been increasingly recognized to provide a particular perspective for vaccine development targeting innate immunity (42). Herein, we reviewed the anti-innate immunity of parasitic infection in hosts, as well as the conception, characteristics, and mechanisms of trained immunity and its advance in the field of Parasitology, highlighting the possible consideration of trained immunity in designing novel vaccines against parasitic diseases.
A compromised innate immunity response hampers parasite elimination in hosts
Adaptive immunity presents a significant “memory” trait, which induces stronger and faster immune responses upon second exposure to pathogens, thus providing long-term protection against infection. Evidence suggests that robust memory responses develop after reinfection of viruses and bacteria, whereas the host’s immune system appears to have only partial resistance to parasitic infection (43–45). The complete protective effect mediated by immunity (referred to as sterilizing immunity) is only observed in cutaneous leishmaniasis caused by tropical Leishmania (46). In contrast, most hosts establish non-sterilizing immunity, which maintains a lower parasite burden in hosts and fails to provide adequate protection against the reinfection of parasites. This results from the well-known fact that parasites are equipped with superior immunoregulatory skills, thereby suppressing and converting the host immune response away from lethality and creating surroundings favorable for survival.
Due to differential parasite size, antigen composition, and life cycles, host immune responses triggered by different types of parasites present specificity to some extent yet share many similarities. In general, the protozoan and the early stage of helminth infection tend to trigger Type 1 immunity (47). However, with the progress of helminth infection, the immune response is biased toward Type 2 immunity (48). Inflammatory monocytes (Mo) and macrophages (Mφ) are important in killing invasive parasites via two mechanisms. Upon infection, Mo triggers the production of reactive oxygen species (ROS), provoked by respiratory burst during phagocytosis, and contributes to early parasite control.
In contrast, Mφ synthesizes nitric oxide (NO) through inducible nitric oxide synthase (iNOS) after activation by IFN-γ (47). Following initial interaction between parasites and pattern recognition receptors (PRRs) (e.g., Toll-like receptors, TLRs; C-type lectin receptors), antigen-presenting cells (APCs), including Mo and DCs initiate and maintain Th1 immune responses through antigen presentation and production of inflammatory cytokines such as IL-12, TNF-α, IL-6. CD4+ T and CD8+ T cells and NK cells respond to IL-12 by secreting IFN-γ, which further instructs Mφ to activate the killing mechanism and enhance inflammatory cytokines production.
However, to resist hosts’ immune systems, parasites have evolved a series of immunomodulatory strategies to precisely suppress or downregulate immune responses, thereby escaping the elimination of immunity. The immunoregulatory events are initiated from the beginning post-infection. The outcome is an optimized environment featuring less aggressive immune cells, decreased pro-inflammatory cytokines (e.g., IFN-γ, IL-1, IL-12, TNF-α), and increased inhibitory cytokines (e.g., IL-10 and TGF-β). It is reported that Leishmania and Toxoplasma gondii can suppress the production of inflammatory cytokines, chemokines, and the expression of costimulatory molecules in Mφ via interference with MAPK, NF⁃κB, STAT3, or activation of STAT6-PPARγ/δ pathways, thus promoting Mφ to differentiate towards anti-inflammatory M2 phenotype. The activation of M2 Mφ is synchronized with the tilt of immune response to Th2, which is detrimental to the elimination of protozoa (49–59). Mφ and DCs are pivotal first responders of the innate immune system against early stage of malaria infection. Mφ controls the parasite burden in early infection stages primarily through phagocytic clearance. DCs are highly efficient in producing chemokines and cytokines in response to malaria infection, and interplay with other cells in the innate and adaptive immune systems. IL-12 produced by DCs activates NK cells, inducing secretion of IFN-γ, which facilitates Th1 and effector T cell responses (60). Massive production of IFN-γ contributes to the control of parasitemia by activating Mφ and neutrophils for enhanced phagocytic activity and consequently, parasite clearance (61, 62). However, Mφ becomes immunosuppressive following internalization of infected erythrocytes, hemozoin or merozoites (63–67), resulting in the inability to produce chemokines and cytokines, which is detrimental to the control of Plasmodium burden (68).
For helminths, the immunomodulatory strategies share many similarities with protozoa yet present quite diversities (69, 70). As the infection progresses, helminths induce the proliferation of Tregs and immunoregulatory Mo along with high levels of immunosuppressive cytokines TGF-β and IL-10 (48). Numerous studies have shown that helminths facilitate the activation and proliferation of Th2 cells and M2 Mφ. These cell types are involved in the anti-inflammatory responses to infection or tissue damage (71). Heligmosomoides polygyrus bakeri (Hpb) and its products are found to affect the metabolism and effector functions of eosinophils, group 2 innate lymphoid cells (ILC2), Mφs and neutrophils, which play important roles in the early type 2 immune response against helminth infection (72–74). Mice infected with Strongyloides venezuelensis (Sv) are protected from Nippostrongylus brasiliensis (Nb) infection for at least 3 months, and this protective effect may be related to the induction of long-term trained immunity programs in eosinophils and ILC2 (75, 76). House dust mite (HDM)-induced trained eosinophils provide protection against subsequent Ascaris infections in mice, and this protective effect against helminth infections is mainly dependent on Th2 cells (77–79). Thus, helminths and their products play key roles in the induction of trained type 2 immunity. It is found that Mφ trained with Fasciola hepatica total extract (FHTE) exhibit enhanced anti-inflammatory trained immunity that is mediated by histone methylation, suppressing effector Th1 and Th17 responses (80). In addition, Mφ from mice treated with FHTE express alternative activated macrophage (AAM) markers and produce large amounts of IL-10 and IL-1RA in response to FHTE or TLR ligands and inhibit the production of TNF and IL-12p40. Thus, by exposure to helminth products, innate immune cells can be trained in vivo or in vitro to be more anti-inflammatory, thereby protecting mice from the induction of T cell-mediated autoimmune diseases (80).
Taken together, parasites apply several tactics to subvert the early fatal Th1 response, inducing an environment characterized by a state of immunosuppression or a moderate Th2-biased immune response in hosts. Despite Th2-type immune response matters in protective immunity against helminth infection, it rarely kills parasites, only limiting their infection and weakening their survival and fertility. As a result, parasitic infection tends to cause long-term and asymptomatic infection in hosts, while the failure of elimination brings about chronic damage to hosts’ health and finally accumulates horrible consequences. Moreover, as the immune system cannot successfully control the parasitic infection in the early stage, parasites stand a chance to multiply rapidly in hosts, and the afterward inappropriate-activated systemic inflammation ultimately leads to immunopathological changes. In addition, suppression of the immune system also impairs vaccine efficacy to some extent, which undoubtedly intensifies the difficulty of preventing and controlling parasitic diseases (81–83). Consequently, a universal way to combat most types of parasites may be to reverse the suppressed state of the immune system and increase early Th1 or innate immunity to kill newly invading larvae, minimize the parasitic burden or even achieve complete prevention.
Interestingly, an analysis of the non-permissive host of Schistosoma japonicum-Microtus fortis is obtained from the comprehensive data of a cytokine chip assay, transcriptome, and metabolome (84), which may dramatically support our opinion. M. fortis presents natural resistance against S. japonicum, and the invading cercaria ultimately dies in the livers of M. fortis. After 12 days of infection, several white nodules composed of dead cercaria and a large number of surrounding inflammatory cells are observed on the surface of M. fortis liver and disappear for the next two weeks. In addition, no eggs, adult worms, or schistosomula are found in M. fortis. Furthermore, cytokines such as IL-1β, IL-2, IL-12, IFN-γ, GM-CSF, and MCP-1 are significantly higher than those in C57BL/6J mice (permissive host for S. japonicum) (Figure 1). Further analysis uncovers that differentially expressed metabolites, including unsaturated fatty acids, quasi-vitamins, and amino acids, are detected in the metabolomic pathway analyses, which relates to the strengthened function and activity of innate immune cells. These results indicate that early initiation of a solid anti-infectious immune response contributes to the natural resistance of M. fortis against S. japonicum infection. Further studies revealed that iNOS is highly expressed in Norway rats (85) (Figure 1), and iNOS interferes with mitochondrial respiration and energy production by producing NO (86, 87), thereby inhibiting S. japonicum growth (88), formation, and development of female and male reproductive organs, and oviposition (89), leading to the production of unfertilized eggs (90). The above effects significantly reduce the viability of S. japonicum (88, 91) and jointly disrupt the formation of granulomas induced by S. japonicum eggs (88). Moreover, NO can impair the tegument of S. japonicum (90), with adverse effects on its nutrient absorption and cholesterol metabolism (92, 93). Thus, enhancing the initial innate immune response may be essential to designing novel parasitic vaccines.
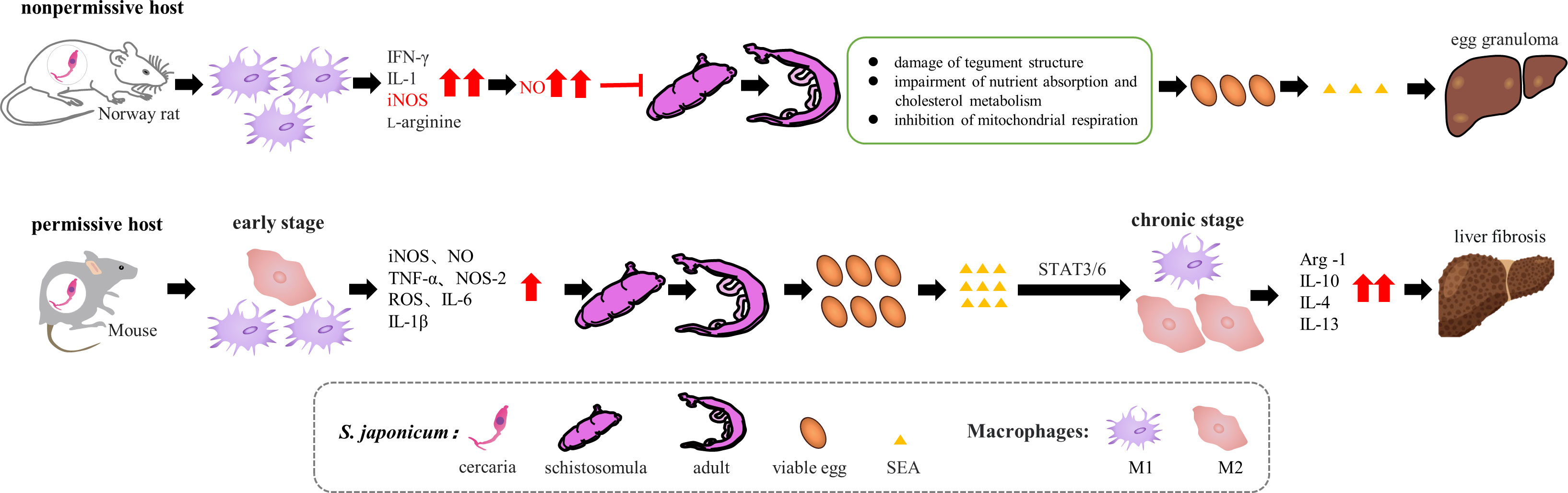
Figure 1 The immune profiles of S. japonicum infection in permissive and non-permissive hosts. In the Norway rats (non-permissive host), immune cells, mainly M1 polarized Mφ, produce nitric oxide (NO) through L-arginine metabolism by iNOS in response to cytokines (e.g., IFN-γ, IL-1). NO leads to impaired nutrient uptake and cholesterol metabolism in the parasite and the disruption of mitochondrial function and inhibition of mitochondrial respiration. These responses restrain the parasite’s viability in hosts, reducing the number of viable eggs and their produced soluble egg antigen (SEA), consequently leading to decreased liver fibrosis. In the permissive hosts (e. g C57BL/6J mice), the early stage of S. japonicum infection induces M1 Mφ polarization, leading to the secretion of inflammatory factors. However, In the chronic phase of infection, the SEA can induce M2 Mφ polarization through STAT3/6 pathways, followed by the secretion of anti-inflammatory factors, which promotes the progression of liver fibrosis.
Trained immunity boosts innate immune response via metabolic and epigenetic reprogramming
Previous opinions hold that only the adaptive system presents the “memory” trait when exposed to the reinfection of pathogens. Instead, trained immunity (TI), also termed innate immune memory, represents an adaptive form of innate host defense mechanisms or de facto innate immune memory. Following exposure to a specific infectious agent or vaccine, innate immune cells (mainly Mo/Mφ, NK cells, and innate lymphoid cells) can respond more rapidly and more strongly to secondary attacks by homologous or even heterologous pathogens (42, 94, 95). However, trained immunity can lead to aberrant inflammatory responses in cases such as autoimmunity (96). The mechanism of trained immunity involves long-term metabolic and epigenetic reprogramming of cells associated with strong immune responses (42, 97, 98).
In contrast with adaptive memory, which lies on the gene recombination of antigen receptors and proliferation of specific lymphocyte clones, TI is triggered by the interplay between pathogen-associated molecular patterns (PAMPs) or damage-associated molecular patterns (DAMPs) and pattern recognition receptors (PRRs) on the innate immune cells, followed by long-term metabolic and epigenetic changes (99, 100) (Figures 2, 3). TI can be initiated immediately after infection but usually lasts only weeks to months after stimulation. The bacterial or fungal ligands such as BCG and β-glucan have been identified as the inducers of TI (Figures 2, 3). However, on the opposite of the training event, a high amount of lipopolysaccharide (LPS) can induce epigenetic silencing and a weakened innate immune response toward the second challenge, which is called tolerance (100) (Figure 3).
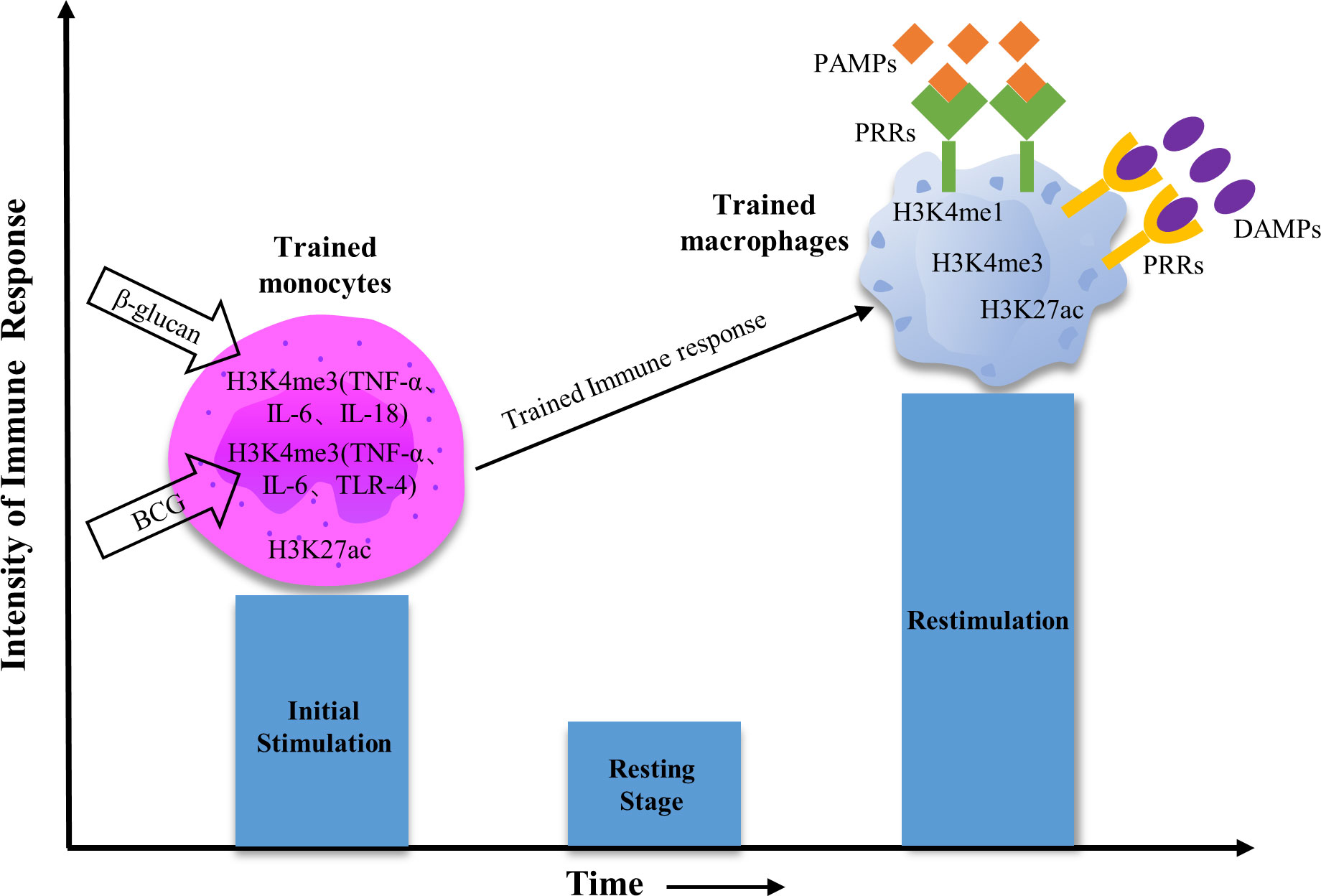
Figure 2 Epigenetic modifications in trained monocytes/macrophages. Initial stimulation of monocytes with β-glucan or BCG leads to histone modifications, which leave epigenetic reprogramming marks on activated genes. Among them, the increased H3K4me3 levels in the trained monocytes were mainly reflected in the promoter regions of genes associated with the expression of pro-inflammatory cytokines. Because chromatin markers are partially removed after primary stimulation, these genes are partially repressed during the resting period and cannot be transcribed. After re-stimulation with PAMPs or DAMPs, trained macrophages can undergo rapid gene transcription machinery and increase the acetylation, monomethylation, and trimethylation of gene promoter regions.
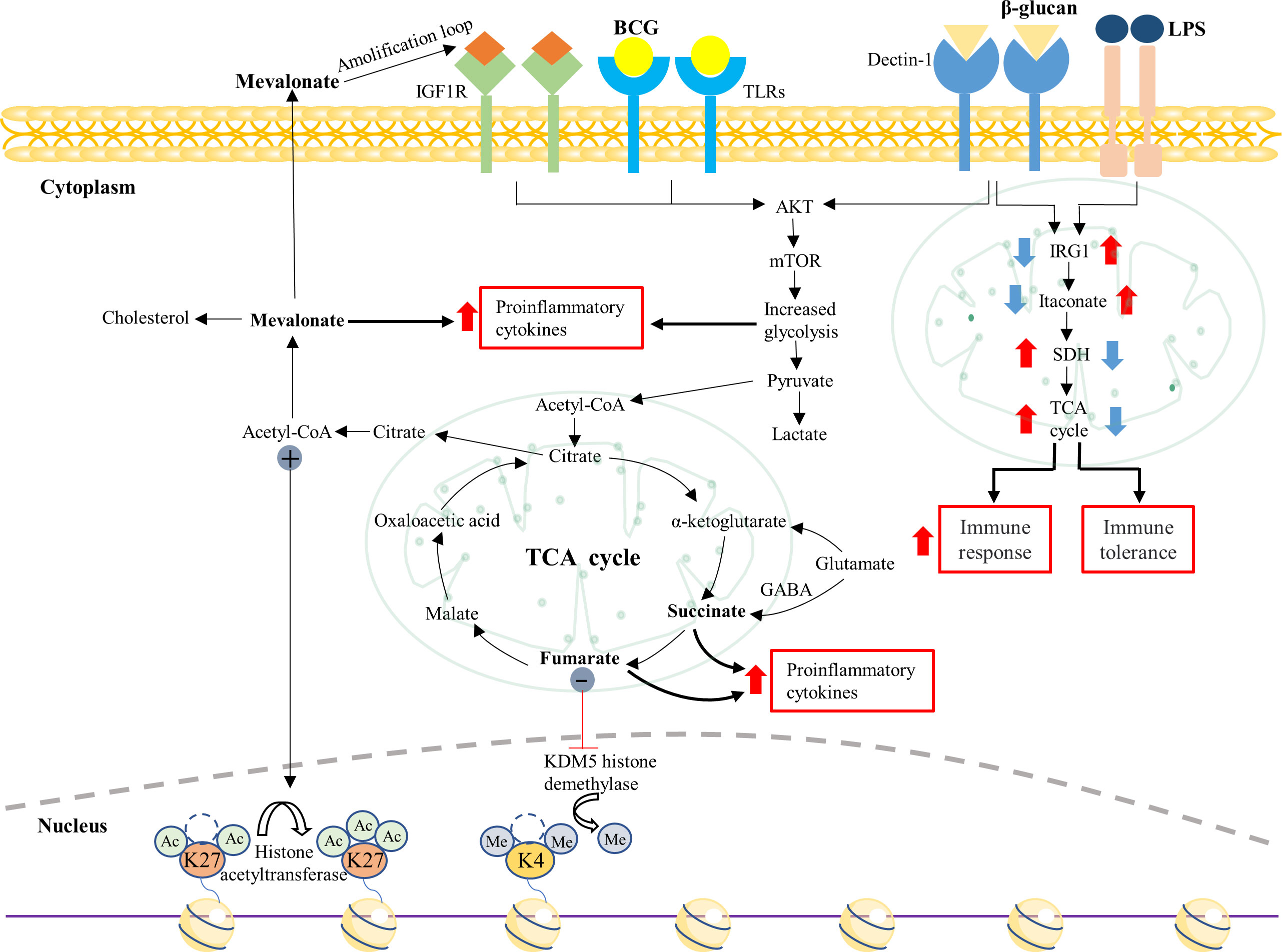
Figure 3 Interaction of metabolic and epigenetic events in trained monocytes/macrophages. Trained immunity is induced in Mo/Mφ by specific ligands (e.g., β-glucan and BCG) via binding to PRRs (e.g., Dectin-1 and TLRs), triggering a series of intracellular cascades. In the process, accumulated intermediate metabolites can regulate the innate immune response via complex mechanisms. For example, fumarate and acetyl-CoA can inhibit KDM5 histone demethylase and activate histone acetyltransferase, leading to specific changes in histone methylation and acetylation in the trained cells. The metabolite mevalonate can induce metabolic and epigenetic reprogramming in the monocytes/macrophages via interacting with IGF1R. In addition, β-glucan can regulate the IRG1-itaconate-SDH axis to enhance the pro-inflammatory response in trained monocytes/macrophages. PRR, pattern recognition receptor; TCA, tricarboxylic acid; IGF1R, insulin-like growth factor 1 receptor; IRG1, immune-responsive gene 1; SDH, succinate dehydrogenase.
The phenomenon of TI has been reported in Mo/Mφ, NK cells, and innate lymphoid cells (99, 101–104). Notably, the underlying mechanisms of TI in Mo/Mφ have been extensively investigated. Mo and Mφ belong to the mononuclear phagocyte system. Mo is an intermediate developmental stage between bone marrow precursor cells and tissue Mφ (105). Circulating Mo has critical biological functions, including maintaining homeostasis of the organism through patrol and repair functions (106) and generating inflammatory responses during infections in the organism (107). During infections, mature blood inflammatory Mo migrates to inflamed tissues and differentiates into Mo-derived Mφ, clearing pathogens and restoring tissue integrity (108). In an in vitro study, Mo was cultured in a human serum medium to induce their differentiation into Mφ (109). In the process, Mo that underwent transient priming by β-glucan differentiated into trained Mφ (101). It is reported that β-glucan induces an epigenetic modification involving many promoters and distal elements and the enhancers on differentiation-sensitive promoters (102). Thus, trained Mo and differentiated Mφ produce higher inflammatory factors in response to stimuli such as ligands targeting pattern recognition receptors (e.g., LPS and Pam3CSK4). This is beneficial for resistance to reinfection by the same or different pathogens, including parasites (110). Here, we reviewed how metabolic and epigenetic events determine TI in Mo/Mφ.
Metabolic reprogramming
(i) Glycolysis
In the resting state, the energy supply in Mo/Mφ relies on oxidative phosphorylation (OXPHOS); however, these cells prefer aerobic glycolysis upon activation (111, 112). The metabolic shift can provide Mo/Mφ with essential precursors for synthesizing nucleotides, amino acids, and lipids and is considered one of the prerequisites for TI (113). It is reported that the metabolic phenotype of β-glucan-trained Mo is characterized by increased glucose consumption, lactate production, the glycolytic pathway, pyruvate conversion, and decreased OXPHOS (114). β-glucan can act on Mo via DC-associated C-type lectin-1 (Dectin-1) receptor to activate the AKT-PI3K pathway, subsequently activating mTOR/HIF-1α (115). Moreover, several studies have demonstrated that the metabolic transition from OXPHOS to aerobic glycolysis mediated by Akt-mTOR-HIF-1α is the metabolic basis of TI (102, 116, 117) (Figure 3). In addition, BCG-induced TI also involves the genetic variation of glycolytic rate-limiting enzymes (e.g., hexokinase 2) in Mo and alteration of AKT/mTOR/HIF-1α pathway (118).
(ii) TCA cycle
Several intermediates produced in the TCA cycle can regulate the TI in Mo/Mφ (Figure 3). Citric acid is mainly converted from pyruvate and α-ketoglutarate, the metabolite of glutamine (119, 120). The citric acid in the cytosol can be converted to acetyl coenzyme A (AcCoA), which acts as an acetylation carrier to promote massive acetylation of histones (e.g., H3K27ac) (Figure 3) and is closely associated with the intrinsic epigenetics of trained immune cells (115, 121). Succinate and fumarate are significantly increased in trained Mo/Mφ (122) (Figure 3). On the one hand, large amounts of succinate and fumarate inhibit the degradation of HIF-1α by prolyl hydroxylase (PHD), thus maintaining the stability of HIF-1α. Then, HIF-1α can induce lactate production, disrupt TCA cycling, and upregulate IL-1 expression (123, 124). On the other hand, succinate and fumaric acid can act as antagonists of histone and DNA demethylases to promote the methylation of histones and DNA (102, 125), which leads to chromatin de-folding and promotes transcription and expression of pro-inflammatory factors (110).
Furthermore, the stimulation of LPS to Mo can induce increased expression of IRG1, which causes massive synthesis of itaconate (126), inhibiting the expression of SDH (127, 128). Itaconate can induce immune tolerance by inhibiting cytokine production (129). Inhibition of SDH expression causes an impeded conversion of succinate to fumarate, inhibiting the TCA cycle’s activity (127, 128) and leading to the development of immune tolerance. β-glucan-induced Mo can inhibit LPS-induced IRG1 expression, thereby blocking the detrimental effects of itaconate on immune tolerance (126) and increasing the expression of H3K27ac at the SDH level, restoring cellular metabolism (130), restoring the production of pro-inflammatory cytokines (131), and thereby reversing LPS-induced immune tolerance (Figure 3).
(iii) Glutamine metabolism
Glutamine (Gln) metabolism of β-glucan-trained or BCG-trained Mφ increases in some research (118) (Figure 3). Glutamate and α-KG that are catabolic products of Gln, as well as succinate generated by the gamma-aminobu-tyricacid (GABA) pathway, complement the intermediates in the TCA cycle (115, 132), which promotes TI. Furthermore, Gln catabolism products themselves can regulate TI through several pathways. Firstly, AcCoA produced from glutamate is a substrate for histone acetyltransferase, which promotes the acetylation of H3K27 (H3K27Ac) in histones in Mo (102, 133), in turn inducing TI. Secondly, glutamine supplementation of the TCA cycle generates large amounts of malate, which can be transported to the cytosol for pyruvate production. The massive synthesis of pyruvate and the activation of the mTOR-HIF-1α pathway will further promote the aerobic glycolytic process, facilitating the TI process (134, 135).
(iv) Cholesterol synthesis pathway
In the culture system of β-glucan-trained Mo/Mφ, those trained cells show enhanced glycolysis and disrupted TCA, which allows a large amount of pyruvate to be converted to AcCoA. Then, AcCoA can directly synthesize fatty acids or cholesterol via the mevalonate (MVA) pathway (136–140). In line with this, over half of the trained cells exhibit upregulation of genes associated with cholesterol synthesis-related pathways (115). Mevalonate (MVA), a key intermediate in this pathway, also accumulates in the Mo/Mφ. Notably, MVA is recently reported to activate the mTOR pathway by interacting with the Insulin-like growth factors-1-receptor (IGF1R) to induce TI in Mo/Mφ (141) (Figure 3).
Epigenetic reprogramming
Mo and Mφ express PRRs, which makes them able to accept and respond to PAMPs and DAMPs stimulation (142, 143). In this process, these cells undergo epigenomic programmed changes, referred to as epigenetic reprogramming, representing one important TI induction mechanism (Figure 2). For example, Mφ trained with β-glucan or BCG is often accompanied by changes in H3K4me3 at the promoters of genes encoding inflammatory cytokines and extensive changes in other chromatin markers (H3K4me1, H2K27ac, and H3K9me2, among others) (102, 114). These changes persist for weeks to months and determine the extent or type of immune response of the cells upon reinfection (144, 145). It is reported that Histone acetylation marks (e.g., H3K27ac) are short-lived and disappear upon removal of the stimulant, while methylation marks are long-lasting (146–149). H3K4me1, as an epigenetic marker, is actively present in enhancers, which maintains the long-term immune memory characteristics and enables Mo/Mφ cells to produce faster and more robust responses upon re-stimulation (148–150).
Furthermore, the immune tolerance process is accompanied by altered histone modification levels. In tolerance experiments with high doses of LPS-induced sepsis, changes in histone H3K4me3, H3K27me2, and histone methyltransferase complexes (102, 151, 152) can downregulate the genes encoding inflammatory factors while upregulating the anti-inflammatory factor genes (153, 154). On the contrary, the ultra-low doses of LPS induce a trained response and enhance the inflammatory response upon LPS re-stimulation (155, 156). In a few pretreatment experiments, low doses of LPS can protect against pathogen infections via epigenetic modifications to increase the expression of inflammatory factor genes, thereby enhancing the phagocytic and killing activity of Mφ (157).
The interaction between metabolic and epigenetic reprogramming
Mo and Mφ stimulated with microorganisms, or endogenous ligands, produce numerous metabolites, which can regulate the function of enzymes responsible for modifying histones and DNA, thereby altering the intrinsic epigenetic landscape. For example, α-KG acts as a cofactor for the activation of the histone demethylase JMJ family (Jumonji) to promote histone demethylation (102, 125); AcCoA provides acetyl substrate for histone acetyltransferases (158); succinate inhibits histone demethylation and inhibition of succinate dehydrogenase (SDH) which inhibits histone methylation (159). Moreover, histone lysine-specific demethylase 1 (LSD1) of the JmjC and JmjD families require α-KG as a cofactor for the demethylation process (156), whereas succinate and fumarate inhibit its demethylation process (160). It is reported that high concentrations of fumarate and succinate can enhance the 3-methylation of histones H3K4 and H3K27 by inhibiting KDM5 histone demethylase (responsible for H3K4 demethylation) and JMJD3 histone demethylase, respectively, which in turn promotes the expression of pro-inflammatory cytokine genes (99, 115, 125, 161, 162). Thus, metabolic and epigenetic reprogramming are tightly integrated and sequentially regulated to train the immune process.
Trained immunity provides a novel perspective for designing anti-parasitic vaccines
When it comes to advancing trained immunity in parasitic vaccines’ development, investigations are still in their infancy, yet with a steady-climbing trend. It is reported that stimulation of human adherent PBMCs with Plasmodium falciparum-infected red blood cells or the malaria crystal hemozoin followed by stimulation with TLR2 ligands can induce increased production of pro-inflammatory cytokines (163). This finding proposes the induction of trained immunity as a cutting edge in parasitic infection. Bacillus-Calmette Guérin (BCG) is a well-known inducer of trained immunity, which can induce long-lasting metabolic and epigenetic changes in Mo/Mφ or NK cells and, consequently, the non-specific memory features (102, 114). The demographic epidemiological survey has indicated that BCG vaccination reduces malaria-specific mortality in malaria-endemic areas (164). To support this hypothesis, BCG-mediated trained immunity boosts the pro-inflammatory cytokine responses (99, 165), thereby resisting the infection towards other pathogens (99, 165).
Moreover, BCG training can lead to early expression of CD69 in NK cells and HLA-DR expression in Mo, which can help reduce parasitemia (166). Furthermore, a recent study has shown that BCG-trained human Mo can enhance the killing of L. braziliensis, L. infantum, and L. amazonensis by increasing the production of reactive oxygen species (167). Moreover, BCG vaccination can reduce parasite load and lesion size in organisms infected with L. braziliensis and reduce the risk of transmission of L. amazonensis to other organs in infected mice (167). Furthermore, β-glucan can also induce trained immunity to increase resistance to Leishmania spp. It is reported that β-glucan-trained Mo, by interacting with dectin-1 and the complement receptor 3 (CR3) receptors (101), can increase cytokine production, especially IL-6, thereby improving the host’s ability of phagocytosis and killing of L. braziliensis (168). β-glucan training affects gene regulatory mechanisms, causing altered histone methylation (namely H3K4me1 enrichment), which leads to transcriptional induction of IL-32 by Mφ to produce higher levels of antimicrobial peptides such as cathelicidin and β-defensin 2 (168). These antimicrobial peptides can help Mφ kill and eliminate Leishmania spp (169, 170). In addition, β-glucan can stimulate a long-term pro-inflammatory Mφ phenotype (168), thereby enhancing the response to parasite infection, which is beneficial for controlling the parasite burden.
Given the potential role and promise of trained immunity in the control of parasitic infections, integration of the innate immune response in the form of trained immunity into the design of future vaccines could improve vaccine efficacy. It could be thus hypothesized that a vaccine targeting at the same time innate and adaptive immune memory would be more successful than existing vaccines against infections, including parasitic infections, that only aim to boost adaptive immune responses (155). It was found that rBCG strains first induced TI against Babesia in the early stages of parasitic infection and then specific antigens, thereby inducing acquired immune memory in the later stages of parasitic infection. The ability of BCG to deliver rhoptry-associated antigen 1 (RAP-1) of Babesia bovis was further confirmed in mice, which supported the hypothesis that rBCG could be used as a component of an anti-Babesia vaccine (171). Therefore, BCG-induced TI and rBCG may be novel strategies to induce protection against acute babesiosis in humans and cattle. This approach can be used either alone to control acute babesiosis infection and prevent deaths or with specific anti-babesiosis vaccines to prevent persistent babesiosis infection. The strategies described above have great potential to reduce the parasite load in the vertebrate host and reduce the risk of parasite transmission, thus favoring the control of disease progression. In addition, BCG-induced TI can play a considerable role in eradication protocols for babesiosis (172).
These studies demonstrate the powerful effect of trained immunity in defending against parasitic infections. It is most likely that boosting innate immunity via trained immunity may also contribute to eliminating a broader spectrum of parasites, although the main findings are only observed in Plasmodium, Leishmania, and Babesia-infected models.
Rewiring of metabolism and epigenetics of innate immune cells: a cutting edge approach for the development of novel parasitic vaccines
Considering the problems of parasitic vaccine development, it is very urgent to design more effective parasitic vaccines in view of novel insights. Epidemiological studies have indicated that, in addition to specific disease-protective effects, vaccines against infectious diseases have beneficial or detrimental non-specific effects on other pathogens (173–175). Intriguingly, the emerging trained immunity may provide a possible explanation for non-specific effects. The concept of “trained immunity-based vaccines (TIbV),” proposed by Sánchez-Ramón et al. in 2018, contributes to the exploitation of parasitic vaccines to expand their therapeutic efficacy (176). TIbV challenges traditional vaccines in several ways (176), although its clinical utility and immunological implications warrant further study. For example, TIbV may not be limited to the formulation of its antigen and is based on trained innate immune cells to provide non-specific protection against different pathogens. Furthermore, TIbV has self-adjuvant characteristics that enhance adaptive immune responses to autoantigens and bystander antigens. TIbV may open a new way to develop broad-spectrum parasitic vaccines in this context.
To develop effective vaccines by enhancing the anti-parasite immunity of the host, it is crucial to gain a deeper understanding of parasitic infection-driven immunomodulatory mechanisms. TI has been reported in a variety of cell populations, including Mo/Mφ, NK cells, innate lymphoid cells (ILCs) and polymorphonuclear leukocytes (77, 101, 116, 157, 177–182). This review focuses on the design of novel parasite vaccines based on Mo/Mφ, as the two types of cells are currently more comprehensively investigated in the field of parasitic infections and TI induction. Mφ can be polarized into classical activated Mφ (M1 Mφ) and alternative activated Mφ (M2 Mφ) under the stimulation of different environments (183) (Figure 4). In general, M1 Mφ is related to the initiation and maintenance of the inflammatory process and eliminates parasites by producing TNF⁃α, IL⁃12, and iNOS (184). However, M2 Mφ can metabolize L⁃arginine into proline and polyamine through Arg1 to promote the survival of parasites in the host, which is related to the regression of the inflammatory process and tissue repair (185). Thus, M1 Mφ is conducive to controlling parasite infection (184), and M2 Mφ is beneficial for parasite survival in the host (185). However, how to reverse Mφ M2 to M1 is a shortcoming in the field. Interestingly, the emerging discipline of TI may enhance the anti-infectious immunity of Mo/Mφ via epigenetic and metabolic events (186, 187) (Figure 3). Thus, rewiring metabolism and epigenetics in these innate cells may be the principle for designing novel parasitic vaccines.
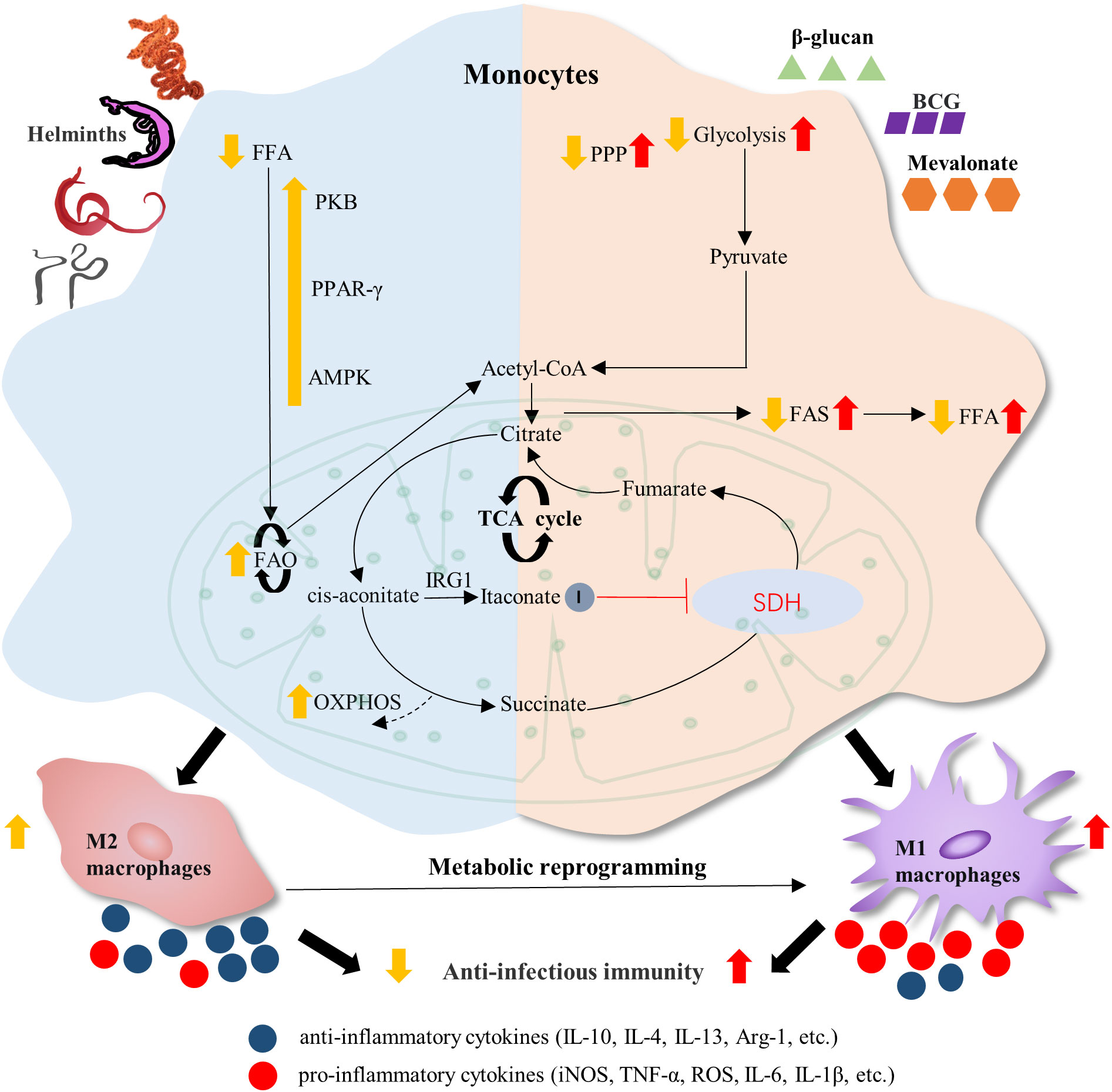
Figure 4 Proposed mechanisms of trained immunity in elevating anti-parasitic innate immunity. Helminth infection can induce the M2 polarization of macrophages in the chronic stage, producing a series of anti-inflammatory cytokines and weakening anti-infectious immunity. Stimulants such as β-glucan, BCG, and mevalonate may train the monocytes and alter the metabolic flux, which induces the M1 polarization of macrophages and enhance the anti-parasitic immunity. FFA, free fatty acid; PKB, protein kinase B; PPAR-γ, peroxisome proliferator–activated receptor-γ; AMPK, adenosine monophosphate (AMP)-activated protein kinase; FAO, fatty acid oxidation; PPP, pentose phosphate pathway; FAS, fatty acid synthesis; IRG1, immune-responsive gene 1; SDH, succinate dehydrogenase; OXPHOS, oxidative phosphorylation.
Induction of M1 Mφ
Mφ is an essential component of the innate immune system and the first line of defense against parasitic infections. Metabolic reprogramming is the critical event in the phenotypic differentiation of Mφ. The M1 type Mφ is associated with an inflammatory response with a predominantly glycolytic response and a disruption of TCA following citric and succinic acid that leads OXPHOS to be impaired. Glycolysis can supply M1 cells with energy to produce more pro-inflammatory factors to rapidly kill microorganisms and adapt to the hypoxic microenvironment of tissues (108, 188, 189). Activation of the pentose phosphate pathway (PPP) is beneficial to M1 cells to maintain inflammatory response (190) (Figure 4). However, M2 Mφ predominates in the chronic or advanced stages of helminth infection (Figure 4). It is reported that helminth infection promotes IL-4 production and maintains the OXPHOS of Mφ to differentiate into M2 Mφ (191–193). OXPHOS continuously provides a large amount of energy required for tissue remodeling and repair (108, 194, 195). Furthermore, both adults and eggs of Schistosoma mansoni can induce M2-type polarization of Mφ by peroxisome proliferator-activated receptor γ (PPARγ) activation, which is beneficial to parasite survival (70) (Figure 4). Thus, M1 Mφ plays a crucial role in anti-infectious immunity through metabolic reprogramming.
Over the past few years, discoveries in immunology have modified the current paradigm. It turns out that the innate immunity associated with hyper-responsiveness often leads to enhanced host defense against secondary infections, which is termed trained immunity (TI) (196). Immunomodulatory compounds, including β-glucan and BCG, are good inducers of TI via reprogramming the metabolic and epigenetic landscape in Mo and Mφ to augment the host response to parasitic infection (102, 114, 168, 197, 198). Mo trained by β-glucan can induce TI, causing increased H3K27ac modification and deposition of H3K4me1 and H3K4me3 gene promoters, leading to transcriptionally active chromatin (102, 197); it also can cause the increased expression of enhancer proximal genes, favoring the generation of a more robust transcriptional response (197). Moreover, β-glucan is reported to induce metabolic changes via the mTOR/HIF-1α pathway (114), resulting in a metabolic shift from OXPHOS to aerobic glycolysis (197), which facilitates the induction of Mo polarization into M1 Mφ. Trained Mφ will have elevated succinate levels in the TCA cycle, which can cause enhanced H3K27me3 in the M2 Mφ gene by inhibiting JMJD3 activity, leading to suppression of M2 Mφ expression (102, 199).
Furthermore, BCG recognizes different Mycobacterium tuberculosis molecular patterns through primed intracellular Toll-like receptors (TLRs) 7 and 9 in Mφ, activating intrinsic immune transcription and gene expression profiles through the NF⁃κB pathway to TLR signaling (200). BCG-trained Mφ cause increased expression of H3K4me3 and H3K27ac at the promoter sites of genes essential for glycolysis (114), as well as decreased expression of H3K9me3 (161), resulting in changes in cellular metabolism (118). Moreover, it is reported that the increased glycolysis and oxygen consumption (118) is induced through the Akt/mTOR pathway (114). Upregulation of glutamine metabolism and accumulation of succinate are involved in IL-1β production via upregulation of HIF-1α (124). Similarly, metabolites such as ferredoxic acid and 2-hydroxyglutaric acid can affect DNA demethylation enzymes (201), thus favoring chromatin condensation and preventing transcription factor binding and gene silencing (202, 203). The TI triggered by BCG leads to a sustained enrichment of H3K4me3, favoring the production of pro-inflammatory cytokines and enhancement of gene promoter regions associated with the expression of intracellular signaling molecules in Mφ (190). All these pathways induce Mφ polarization toward M1 Mφ. Thus, β-glucan and BCG can induce Mφ polarization toward M1 Mφ through the interplay and interaction between epigenetic reprogramming and cellular metabolic reprogramming to enhance anti-infectious immunity.
In addition, Mo and Mφ remove pathogens as part of innate immunity and contribute to adaptive immunity as antigen presentation cells. Upon pathogen invasion, PRRs (e.g., TLRs) expressed on the inflammatory Mφ initiate the innate immune signaling pathways and trigger a variety of pro-inflammatory cytokines production, which in turn instructs a pro-inflammatory adaptive immune response involving primarily Th1 cells (204, 205). During a Th1 response, T cells, specifically CD4+ Th1 cells, could play an integral role in suppressing parasite growth by producing IFN-γ and IL-2 (206–208). Therefore, inducing M1 Mφ may aid in optimizing vaccine strategy to elicit broadly protective innate immunity and parasite-specific adaptive immunity. In conclusion, future work on developing parasitic vaccines should further explore the pathways associated with the induction of Mφ polarization by parasite infection and focus on applying immune adjuvants such as BCG and β-glucan to alter the associated cellular metabolic and epigenetic reprogramming. Moreover, Mφ that undergoes metabolic reprogramming causes the accumulation of intermediate metabolites (124, 209–213). There is accumulating evidence showing that several metabolites are conducive to anti-infectious immunity due to their immunomodulatory properties (114, 115, 141), which may provide the development of vaccines with potent adjuvants.
Reversal of immune tolerance
Parasitic infections in chronic stages often compromise the host’s anti-parasite immunity, termed immune tolerance. For example, high levels of iNOS and NF⁃κB expression were shown in the peripheral blood of primary patients with Echinococcus granulosus, while their low expression occurred in the relapsed patients (214). Thus, reversing the immune tolerance can boost anti-infectious immunity. Interestingly, β-glucan binding to dectin-1 is reported to initiate the phagocytosis, produce the superoxide via NADPH oxidase (215–217), and convert Mφ to the M1 phenotype via NF–κB–autophagy-dependent pathway (218). Moreover, in a model of immune tolerance induced by LPS in volunteers, β-glucan can partially reverse LPS-induced immune tolerance by modulating the cellular epigenome, which allows elevated expression of H3K27ac, thereby restoring the ability of Mφ to produce pro-inflammatory cytokines (197). In addition, it is reported that in a human sepsis model, β-glucan inhibits the expression of immune response gene 1 (IRG1), thereby downregulating the synthesis of itaconate and increasing the expression of succinate dehydrogenase (SDH) (Figure 3). This metabolic alteration can recover the ability of Mo/Mφ to produce high levels of pro-inflammatory cytokines responsible for secondary stimulation (110, 126, 197), thereby enhancing the killing of pathogenic organisms. Taken together, β-glucan can prevent or reverse immune tolerance by altering the relevant metabolic and epigenetic pathways and TI induction. It is speculative that such an effect of β-glucan may be a breakthrough point to ameliorate the parasite-induced immune tolerance in the host.
Development of novel adjuvants
As a key vaccine component, the adjuvants can enhance the immune response through various activities (219, 220). They can bind to antigens and facilitate more accessible and efficient recognition of “non-self substances” by the body, thus triggering both innate and adaptive immune responses (221). Moreover, the adjuvants transport antigens to lymph nodes, thus facilitating T-cell antigen recognition. In addition, the adjuvants prolong the retention time of antigens, induce the release of pro-inflammatory factors, and increase the intensity of the local response at the injected site (222). Therefore, supplementation of adjuvants is necessary to develop a vaccine with high efficiency.
In the studies of parasitic vaccines, different types of adjuvants can significantly enhance the effectiveness of the vaccine. Monophosphoryl lipid A (MPL) is the component with adjuvant activity obtained after removing the toxic effects of bacterial lipopolysaccharides (223, 224). MPL® is formulated in a variety of adjuvant platforms, including AS01 (MPL® and QS-21 in phosphatidylcholine liposomes), AS02 (MPL® and saponin QS-21 in an oil-in-water emulsion containing squalene and α-tocopherol) and AS04 (MPL® adsorbed to aluminum oxyhydroxide). Numerous clinical studies have shown that TLR4 agonists such as AS01, AS04, and oil-in-water emulsion (GLA-SE) show excellent performance in the development of various vaccines against parasites (13, 225–233). In fact, the TLR4 ligand lipopolysaccharide is reported to induce epigenetic reprogramming of haematopoietic stem cells, leading to persistent changes in the accessibility of their myeloid lineage enhancers (234). Moreover, the TLR9 agonist CpG confers broad resistance to infection by inducing TI in Mφ through MyD88-dependent cascade conduction (235). These protective effects are partly mediated by metabolic reprogramming, characterized by sustained enhanced glycolysis, mitochondrial oxygen depletion (236, 237), and mTOR activation (235).
Interestingly, a recent study has demonstrated that mevalonate (MVA), a metabolite in the cholesterol synthesis pathway, can induce TI via activating the IGF1-R and mTOR pathways and subsequent histone modifications in Mo and Mφ (167) (Figure 3). In MVA-trained Mo, cellular metabolism switches from OXPHOS to aerobic glycolysis, and the enhanced glycolytic pathway also promotes the MVA metabolic pathway (140). Mevalonate can induce the enrichment of H3K4me3 on the IL-6 and TNF-α promoters (141), thereby enhancing the production of inflammatory factors. It is well-known that the enhanced glycolytic pathways favor the induction of Mφ polarization toward M1-type Mφ (238), as well as the production of various inflammatory cytokines, including IFN-γ and TNF-α. These cytokines eliminate the cellular internalized parasites by triggering the oxidative burst pathway (239). Moreover, there is accumulating evidence showing that TI can enhance innate immunity and reduce parasitemia in rodent models of B. microti and malaria (240–243). With the advance of TI research, it is believed that more and more metabolites with immunoregulatory activity will be identified in TI induction, which may be utilized to boost innate immunity in developing TIbV against parasitic diseases.
Stimulation of hematopoietic stem and multipotent progenitor cells
Hematopoietic stem cells (HSCs) in the bone marrow (BM) are pluripotent and self-renewing (244). A large body of recent evidence suggests that inflammation or infection elicits an immune response in the BM (245–247). Similar to TI in Mo and Mφ, several infectious stimuli can train HSCs and progenitor cells, thereby enhancing the strength of the response to secondary infections (244). In the model of severe infection or sepsis, hematopoietic stem and multipotent progenitor cells (HSPCs) in the BM are also activated to proliferate and prompt BM hematopoiesis, facilitating infection control (246, 247). Moreover, Plasmodium sporozoites lead to the sustained proliferation of Sca-1 HSPCs to deal with life-threatening infections (248). Studies have demonstrated that mice treated with Fasciola hepatica-excretory/secretory (Fh-ES) can imprint a lasting memory on HSCs in the BM through metabolic and transcriptional rewiring (249). Treating mice with Fh-ES has enhanced the expansion and proliferation of myeloid-committed precursors, which leads to the expansion of anti-inflammatory Mo. This helminth-induced anti-inflammatory trained immunity reduces the susceptibility of mice to experimental autoimmune encephalomyelitis (250). Recent studies have reported that HSPCs develop trained immunity after stimulation with β-glucan or BCG. In detail, BM stimulated by β-glucan induces expansion of CD41-biased HSCs and BM subpopulations, followed by enhanced metabolic pathways such as glycolysis, cholesterol synthesis, and mevalonate in HSPCs (251). The first exposure of BM to β-glucan increased the intensity of the response of HSPCs to secondary LPS infection by expanding lineage Sca-1c-Kit (LSK) and multipotent progenitors (MPPs) and by enhancing the DNA damage response (251). BCG trains HSCs and MPPs in the BM through an IFN-γ-mediated pathway, causing cell expansion and enhancing BM hematopoiesis (187). These trained HSCs gave rise to epigenetically modified Mφ, more resistant to Mycobacterium tuberculosis infection (187). Thus, trained HSPCs enhance the body’s resistance to infection, and the mature immune cells generated by their differentiation have a more robust response strength to secondary infections, ultimately improving the body’s defense against pathogenic microorganisms. Therefore, the better utilization of myeloid cells in future parasite vaccine development would have the potential to dramatically improve vaccine efficacy, which would help break the cycle of parasite transmission.
Myeloid-derived suppressor cells (MDSCs) arise from myeloid progenitor cells (252), which are a population of myeloid cells that arise in a range of pathological conditions such as cancer (253). These cells are divided into two main cell types: granulocytic (G-MDSC) and monocytic (M-MDSC) (253). MDSCs are powerful immunosuppressive cells (254), capable of suppressing the function of T cells and becoming regulators of the body’s immune function in many pathological situations (253). In S. japonicum-infected mice, G-MDSC was found to inhibit Tfh cell proliferation, possibly through the programmed cell death protein 1 (PD-1)/programmed cell death ligand 1 (PD-L1) pathway (255). In controlled human malaria infection (CHMI) experiments, a sustained increase in circulating G-MDSC was found in volunteers who developed Plasmodium falciparum parasitemia, which suppressed the proliferation of CD4+ and CD8+ T cells and led to a decrease of lymphocytes, interfering with the production of immune memory against the parasite and suppressing the body’s immune response (256). In T. cruzi-infected mice, expansion of iNOS-expressing MDSCs had an immunosuppressive effect. Expansion of MDSCs associated with the depletion of L-arg by Arg1 impeded nitric oxide production, further exacerbating immunosuppression in mice (257). Overall, MDSCs proliferate abnormally and exert immunosuppressive effects through the mechanisms described above during chronic parasite infections (255–257), which can significantly limit the effectiveness of parasite vaccines.
Interestingly, foreign agents like β-glucan can modulate this suppressive cell population in tumor models (258). In vivo oral administration of particulate β-glucan significantly reduced tumor weight and splenomegaly in tumor-bearing mice (259). Differentiated M-MDSC by β-glucan in vitro can induce antigen-specific Th1 and CD8+ T cell responses via interacting with dectin-1. Moreover, the M-MDSC stimulated by particulate β-glucan can differentiate into CD11c cells with high MHC class II expression in vivo, thereby reducing tumor growth (259). Another study reported that treatment with particulate β-glucan substantially reduces MDSCs in tumor-bearing mice but increases the infiltrated Mφ and DCs, thus inducing Th1 and CTL responses and suppressing the immunosuppressive effects of regulatory T cells (260). Overall, the discoveries in tumor models may offer the possibility of enhancing host defense against parasitic infection by β-glucan inhibiting the immunosuppressive functions of MDSCs.
Summary and perspectives
Because of the complex life history and large genome of parasites, it is difficult to find suitable parasite antigen molecules for effective vaccine development. Moreover, the parasite and some of its components can suppress the body’s immunity against infection, thus reducing the effectiveness of parasite vaccines. Interestingly, the description of TI has attracted extensive attention, representing a more effective innate immune response against the same or unrelated stimuli after a primary stimulation (94, 95). Therefore, enhancing the innate immune responses based on TI provides a unique perspective for parasite vaccine development. Enhanced innate immunity facilitates the killing of newly invading larvae at an early stage and substantially reduces the parasite burden on the organism to avoid parasite-induced type 2 immunity at a later stage (48, 69, 70). Therefore, there are promising directions for using TI to enhance the intrinsic immune response to improve the protection rate of parasite vaccines.
However, it should be pointed out that the progress of TI research is still in its infancy and faces important challenges. Research on the function and mechanism of TI in parasitic infections is just beginning, which will limit the rapid progress and practical application of TIbV. Due to the differences in immune evasion modalities of protozoan parasites and helminths (48–80), controlling parasitic infections through a strategy of TI against the same or unrelated stimuli after the initial stimulation (94, 95), is not a foolproof way. This results from the fact that parasites parasitizing different biological sites in hosts may trigger various immune responses (261). Moreover, helminth-mediated type 2 response is not always bad for hosts. For example, a short initial Th2 response is beneficial to worm expulsion, while a prolonged activation of the type 2 immune response may lead to a shift in the T-cell pool, thereby increasing the number of Treg cells (262). This hinders the over-activation of the immune system and consequently, limits the excessive collateral organismal tissue damage in chronic inflammation. Thus, how to keep the balance of type 1/type 2 response in the development of TIbV against parasitic infection, is still a big problem.
Furthermore, the future applicability of TIbV needs to be carefully considered due to the diverse disease spectrum in different regions. TIbV utilization may be more appropriate in the areas popular with parasitic and infectious diseases; however, it may not be suited in developed countries where non-communicable diseases are predominantly prevalent (263). Trained immunity can also have deleterious systemic consequences, as it may trigger enhanced tissue damage in chronic inflammatory states (95), such as increased susceptibility to atherosclerosis in patients with autoimmune or chronic inflammatory diseases (e.g., rheumatoid arthritis) (264). Moreover, western diets are reported to trigger maladaptive TI, an immune state that may be responsible for common inflammatory diseases (e.g., type 2 diabetes or Alzheimer’s disease) in developed countries (95). In addition, prolonged or excessive inflammatory responses can promote tumor progression (42). Overall, there are numerous challenges that need to be addressed before the clinical application of parasitic TIbV.
Author contributions
WP and DW conceived the manuscript. JZ, JL, and CY wrote the manuscript. All authors contributed to the article and approved the submitted version.
Funding
This work was supported by the National Natural Science Foundation of China (No. 81871670), the Open Competition Grant of Xuzhou Medical University, the Jiangsu Qing Lan Project, and the Liangshan Research Project (2021). The funders had no role in study design, data collection and analysis, decision to publish, or preparation of the manuscript.
Acknowledgments
We would like to thank Professor Mihai G Netea for providing the valuable comments on the manuscript.
Conflict of interest
The authors declare that the research was conducted in the absence of any commercial or financial relationships that could be construed as a potential conflict of interest.
Publisher’s note
All claims expressed in this article are solely those of the authors and do not necessarily represent those of their affiliated organizations, or those of the publisher, the editors and the reviewers. Any product that may be evaluated in this article, or claim that may be made by its manufacturer, is not guaranteed or endorsed by the publisher.
References
1. Varo R, Chaccour C, Bassat Q . Update on malaria. Med Clin (Barc) (2020) 155(9):395–402. doi: 10.1016/j.csbj.2017.02.003
2. Crellen T, Walker M, Lamberton PH, Kabatereine NB, Tukahebwa EM, Cotton JA, et al. Reduced efficacy of praziquantel against schistosoma mansoni is associated with multiple rounds of mass drug administration. Clin Infect Dis Off Publ Infect Dis Soc America (2016) 63(9):1151–9. doi: 10.1093/cid/ciw506
3. Wang W, Wang L, Liang YS. Susceptibility or resistance of praziquantel in human schistosomiasis: a review. Parasitol Res (2012) 111(5):1871–7. doi: 10.1007/s00436-012-3151-z
4. Black CL, Steinauer ML, Mwinzi PN, Evan SW, Karanja DM, Colley DG. Impact of intense, longitudinal retreatment with praziquantel on cure rates of schistosomiasis mansoni in a cohort of occupationally exposed adults in western Kenya. Trop Med Int Health (2009) 14(4):450. doi: 10.1111/j.1365-3156.2009.02234.x
5. Yu Q, Xiao N, Yang SJ, Han S. Deworming of stray dogs and wild canines with praziquantel-laced baits delivered by an unmanned aerial vehicle in areas highly endemic for echinococcosis in China. Infect Dis Poverty (2017) 6(1):117. doi: 10.1186/s40249-017-0329-8
6. Sun LP, Liang YS, Wu HH, Tian ZX, Dai JR, Yang K, et al. A Google Earth-based surveillance system for schistosomiasis japonica implemented in the lower reaches of the Yangtze River, China. Parasit Vectors (2011) 4:223. doi: 10.1186/1756-3305-4-223
7. Chen JH, Wang H, Chen JX, Bergquist R, Tanner M, Utzinger J, et al. Frontiers of parasitology research in the People's Republic of China: infection, diagnosis, protection and surveillance. Parasit Vectors (2012) 5:221. doi: 10.1186/1756-3305-5-221
8. Greenwood B, Doumbo OK. Implementation of the malaria candidate vaccine RTS,S/AS01. Lancet (2016) 387(10016):318–9. doi: 10.1016/S0140-6736(15)00807-7
9. Olotu A, Fegan G, Wambua J, Nyangweso G, Leach A, Lievens M, et al. Seven-year efficacy of RTS,S/AS01 malaria vaccine among young african children. N Engl J Med (2016) 374(26):2519–29. doi: 10.1056/NEJMoa1515257
10. Agnandji ST, Lell B, Soulanoudjingar SS, Fernandes JF, Abossolo BP, Conzelmann C, et al. First results of phase 3 trial of RTS,S/AS01 malaria vaccine in African children. N Engl J Med (2011) 365(20):1863–75. doi: 10.1056/NEJMoa1102287
11. Datoo MS, Natama MH, Somé A, Traoré O, Rouamba T, Bellamy D, et al. Efficacy of a low-dose candidate malaria vaccine, R21 in adjuvant Matrix-M, with seasonal administration to children in Burkina Faso: a randomised controlled trial. Lancet (2021) 397(10287):1809–18. doi: 10.1016/S0140-6736(21)00943-0
12. Riveau G, Schacht A-M, Dompnier J-P, Deplanque D, Seck M, Waucquier N, et al. Safety and efficacy of the rSh28GST urinary schistosomiasis vaccine: A phase 3 randomized, controlled trial in Senegalese children. PloS Negl Trop Dis (2018) 12(12):e0006968. doi: 10.1371/journal.pntd.0006968
13. Santini-Oliveira M, Coler RN, Parra J, Veloso V, Jayashankar L, Pinto PM, et al. Schistosomiasis vaccine candidate Sm14/GLA-SE: Phase 1 safety and immunogenicity clinical trial in healthy, male adults. Vaccine (2016) 34(4):586–94. doi: 10.1016/j.vaccine.2015.10.027
14. Hotez PJ, Bottazzi ME, Bethony J, Diemert DD. Advancing the development of a human schistosomiasis vaccine. Trends Parasitol (2019) 35(2):104–8. doi: 10.1016/j.pt.2018.10.005
15. Zhang W, Molehin AJ, Rojo JU, Sudduth J, Ganapathy PK, Kim E, et al. Sm-p80-based schistosomiasis vaccine: double-blind preclinical trial in baboons demonstrates comprehensive prophylactic and parasite transmission-blocking efficacy. Ann N Y Acad Sci (2018) 1425(1):38–51. doi: 10.1111/nyas.13942
16. Perera DJ, Ndao M. Promising technologies in the field of helminth vaccines. Front Immunol (2021) 12:711650. doi: 10.3389/fimmu.2021.711650
17. Lee LYY, Izzard L, Hurt AC. A review of DNA vaccines against influenza. Front Immunol (2018) 9:1568. doi: 10.3389/fimmu.2018.01568
18. Li L, Petrovsky N. Molecular mechanisms for enhanced DNA vaccine immunogenicity. Expert Rev Vaccines (2016) 15(3):313–29. doi: 10.1586/14760584.2016.1124762
19. Kim D, Wu Y, Kim YB, Oh YK. Advances in vaccine delivery systems against viral infectious diseases. Drug Delivery Transl Res (2021) 11(4):1401–19. doi: 10.1007/s13346-021-00945-2
20. Braathen R, Spång HCL, Hinke DM, Blazevski J, Bobic S, Fossum E, et al. A DNA vaccine that encodes an antigen-presenting cell-specific heterodimeric protein protects against cancer and influenza. Mol Ther Methods Clin Dev (2020) 17:378–92. doi: 10.1016/j.omtm.2020.01.007
21. Sharma A, Sharma P, Vishwakarma AL, Srivastava M. Functional Impairment of Murine Dendritic Cell Subsets following Infection with Infective Larval Stage 3 of Brugia malayi. Infect Immun (2017) 85(1):e00818-16. doi: 10.1128/IAI.00818-16
22. Joardar N, Mondal C, Sinha Babu SP. A review on the interactions between dendritic cells, filarial parasite and parasite-derived molecules in regulating the host immune responses. Scand J Immunol (2021) 93(4):e13001. doi: 10.1111/sji.13001
23. Gupta J, Misra S, Misra-Bhattacharya S. Immunization with Brugia malayi Myosin as Heterologous DNA Prime Protein Boost Induces Protective Immunity against B. malayi Infection in Mastomys coucha. PloS One (2016) 11(11):e0164991. doi: 10.1371/journal.pone.0164991
24. Chavda VP, Pandya R, Apostolopoulos V. DNA vaccines for SARS-CoV-2: toward third-generation vaccination era. Expert Rev Vaccines (2021) 20(12):1549–60. doi: 10.1080/14760584.2021.1987223
25. Liu HF, Li W, Lu MB, Yu LJ. Pharmacokinetics and risk evaluation of DNA vaccine against Schistosoma japonicum. Parasitol Res (2013) 112(1):59–67. doi: 10.1007/s00436-012-3104-6
26. Zhang W, Ahmad G, Torben W, Noor Z, Le L, Damian RT, et al. Sm-p80-based DNA vaccine provides baboons with levels of protection against Schistosoma mansoni infection comparable to those achieved by the irradiated cercarial vaccine. J Infect Dis (2010) 201(7):1105–12. doi: 10.1086/651147
27. Wang X, Dai Y, Zhao S, Tang J, Li H, Xing Y, et al. PAMAM-Lys, a novel vaccine delivery vector, enhances the protective effects of the SjC23 DNA vaccine against Schistosoma japonicum infection. PloS One (2014) 9(1):e86578. doi: 10.1371/journal.pone.0086578
28. Lei N, Liu FC, Ren CP, Shen JJ, Liu M. An efficient schistosoma japonicum bivalent membrane protein antigen DNA vaccine against schistosomiasis in mice. Med Sci Monit (2019) 25:9319–26. doi: 10.12659/MSM.919195
29. Gonçalves de Assis NR, Batistoni de Morais S, Figueiredo BC, Ricci ND, de Almeida LA, da Silva Pinheiro C, et al. DNA Vaccine Encoding the Chimeric Form of Schistosoma mansoni Sm-TSP2 and Sm29 Confers Partial Protection against Challenge Infection. PloS One (2015) 10(5):e0125075. doi: 10.1371/journal.pone.0125075
30. Wiśniewski M, Jaros S, Bąska P, Cappello M, Długosz E, Wędrychowicz H. Hamsters vaccinated with Ace-mep-7 DNA vaccine produced protective immunity against Ancylostoma ceylanicum infection. Exp Parasitol (2016) 163:1–7. doi: 10.1016/j.exppara.2016.01.006
31. Wesołowska A, Zawistowska-Deniziak A, Norbury LJ, Wilkowski P, Januszkiewicz K, Pyziel AM, et al. Immune responses in rats and sheep induced by a DNA vaccine containing the phosphoglycerate kinase gene of Fasciola hepatica and liver fluke infection. Acta Parasitol (2016) 61(2):212–20. doi: 10.1515/ap-2016-0030
32. Zhang W, Le L, Ahmad G, Molehin AJ, Siddiqui AJ, Torben W, et al. Fifteen Years of Sm-p80-Based Vaccine Trials in Nonhuman Primates: Antibodies From Vaccinated Baboons Confer Protection in vivo and in vitro From Schistosoma mansoni and Identification of Putative Correlative Markers of Protection. Front Immunol (2020) 11:1246. doi: 10.3389/fimmu.2020.01246
33. Ertl HC. Viral vectors as vaccine carriers. Curr Opin Virol (2016) 21:1–8. doi: 10.1016/j.coviro.2016.06.001
34. Yusuf Y, Yoshii T, Iyori M, Mizukami H, Fukumoto S, Yamamoto DS, et al. A viral-vectored multi-stage malaria vaccine regimen with protective and transmission-blocking efficacies. Front Immunol (2019) 10:2412. doi: 10.3389/fimmu.2019.02412
35. Dutton S, Fleming SB, Ueda N, Heath DD, Hibma MH, Mercer AA. Delivery of Echinococcus granulosus antigen EG95 to mice and sheep using recombinant vaccinia virus. Parasite Immunol (2012) 34(6):312–7. doi: 10.1111/j.1365-3024.2012.01360.x
36. Rothel JS, Boyle DB, Both GW, Pye AD, Waterkeyn JG, Wood PR, et al. Sequential nucleic acid and recombinant adenovirus vaccination induces host-protective immune responses against Taenia ovis infection in sheep. Parasite Immunol (1997) 19(5):221–7. doi: 10.1046/j.1365-3024.1997.d01-200.x
37. Hotez PJ, Zhan B, Bethony JM, Loukas A, Williamson A, Goud GN, et al. Progress in the development of a recombinant vaccine for human hookworm disease: the Human Hookworm Vaccine Initiative. Int J Parasitol (2003) 33(11):1245–58. doi: 10.1016/S0020-7519(03)00158-9
38. de Barros LD, Koutsodontis Cerqueira-Cézar C. Editorial: Vaccines against parasitic infections in domestic animals. Front Vet Sci (2023) 10:1144700. doi: 10.3389/fvets.2023.1144700
39. Arumugam TU, Ito D, Takashima E, Tachibana M, Ishino T, Torii M, et al. Application of wheat germ cell-free protein expression system for novel malaria vaccine candidate discovery. Expert Rev Vaccines (2014) 13(1):75–85. doi: 10.1586/14760584.2014.861747
40. Chen JY, Zhou JK, Pan W. Immunometabolism: towards a better understanding the mechanism of parasitic infection and immunity. Front Immunol (2021) 12:661241. doi: 10.3389/fimmu.2021.661241
41. McNeilly TN, Nisbet AJ. Immune modulation by helminth parasites of ruminants: implications for vaccine development and host immune competence. Parasite (2014) 21:51. doi: 10.1051/parasite/2014051
42. Netea MG, Domínguez-Andrés J, Barreiro LB, Chavakis T, Divangahi M, Fuchs E, et al. Defining trained immunity and its role in health and disease. Nat Rev Immunol (2020) 20(6):375–88. doi: 10.1038/s41577-020-0285-6
43. Whitmire JK, Murali-Krishna K, Altman J, Ahmed R. Antiviral CD4 and CD8 T-cell memory: differences in the size of the response and activation requirements. Philos Trans R Soc Lond B Biol Sci (2000) 355(1395):373–9. doi: 10.1098/rstb.2000.0577
44. Slifka MK. Immunological memory to viral infection. Curr Opin Immunol (2004) 16(4):443–50. doi: 10.1016/j.coi.2004.05.013
45. Zinkernagel RM, Hengartner H. On immunity against infections and vaccines: credo 2004. Scand J Immunol (2004) 60(1–2):9–13. doi: 10.1111/j.0300-9475.2004.01460.x
46. Scott P. Immunologic memory in cutaneous leishmaniasis. Cell Microbiol (2005) 7(12):1707–13. doi: 10.1111/j.1462-5822.2005.00626.x
47. Miller CM, Smith NC, Ikin RJ, Boulter NR, Dalton JP, Donnelly S. Immunological interactions between 2 common pathogens, Th1-inducing protozoan Toxoplasma gondii and the Th2-inducing helminth Fasciola hepatica. PloS One (2009) 4(5):e5692. doi: 10.1371/journal.pone.0005692
48. Allen JE, Maizels RM. Diversity and dialogue in immunity to helminths. Nat Rev Immunol (2011) 11(6):375–88. doi: 10.1038/nri2992
49. Denkers EY, Butcher BA, Del Rio L, Kim L. Manipulation of mitogen-activated protein kinase/nuclear factor-kappaB-signaling cascades during intracellular Toxoplasma gondii infection. Immunol Rev (2004) 201:191–205. doi: 10.1111/j.0105-2896.2004.00180.x
50. Shapira S, Speirs K, Gerstein A, Caamano J, Hunter CA. Suppression of NF-kappaB activation by infection with Toxoplasma gondii. J Infect Dis (2002) 185 Suppl 1:S66–72. doi: 10.1086/338000
51. Zhang AM, Shen Q, Li M, Xu XC, Chen H, Cai YH, et al. Comparative studies of macrophage-biased responses in mice to infection with Toxoplasma gondii ToxoDB 9 strains of different virulence isolated from China. Parasit Vectors (2013) 6(1):308. doi: 10.1186/1756-3305-6-308
52. He X, Gong P, Wei Z, Liu W, Wang W, Li J, et al. Peroxisome proliferator-activated receptor-γ-mediated polarization of macrophages in Neospora caninum infection. Exp Parasitol (2017) 178:37–44. doi: 10.1016/j.exppara.2017.05.002
53. Butcher BA, Kim L, Panopoulos AD, Watowich SS, Murray PJ, Denkers EY. IL-10-independent STAT3 activation by Toxoplasma gondii mediates suppression of IL-12 and TNF-alpha in host macrophages. J Immunol (2005) 174(6):3148–52. doi: 10.4049/jimmunol.174.6.3148
54. Denkers EY, Bzik DJ, Fox BA, Butcher BA. An inside job: hacking into Janus kinase/signal transducer and activator of transcription signaling cascades by the intracellular protozoan Toxoplasma gondii. Infect Immun (2012) 80(2):476–82. doi: 10.1128/IAI.05974-11
55. Chandra D, Naik S. Leishmania donovani infection down-regulates TLR2-stimulated IL-12p40 and activates IL-10 in cells of macrophage/monocytic lineage by modulating MAPK pathways through a contact-dependent mechanism. Clin Exp Immunol (2008) 154(2):224–34. doi: 10.1111/j.1365-2249.2008.03741.x
56. Srivastava N, Sudan R, Saha B. CD40-modulated dual-specificity phosphatases MAPK phosphatase (MKP)-1 and MKP-3 reciprocally regulate Leishmania major infection. J Immunol (2011) 186(10):5863–72. doi: 10.4049/jimmunol.1003957
57. Cameron P, McGachy A, Anderson M, Paul A, Coombs GH, Mottram JC, et al. Inhibition of lipopolysaccharide-induced macrophage IL-12 production by Leishmania mexicana amastigotes: the role of cysteine peptidases and the NF-kappaB signaling pathway. J Immunol (2004) 173(5):3297–304. doi: 10.4049/jimmunol.173.5.3297
58. Ben-Othman R, Dellagi K, Guizani-Tabbane L. Leishmania major parasites induced macrophage tolerance: implication of MAPK and NF-kappaB pathways. Mol Immunol (2009) 46(16):3438–44. doi: 10.1016/j.molimm.2009.05.337
59. Kong F, Saldarriaga OA, Spratt H, Osorio EY, Travi BL, Luxon BA, et al. Transcriptional profiling in experimental visceral leishmaniasis reveals a broad splenic inflammatory environment that conditions macrophages toward a disease-promoting phenotype. PloS Pathog (2017) 13(1):e1006165. doi: 10.1371/journal.ppat.1006165
60. Walsh KP, Mills KH. Dendritic cells and other innate determinants of T helper cell polarisation. Trends Immunol (2013) 34(11):521–30. doi: 10.1016/j.it.2013.07.006
61. Ing R, Stevenson MM. Dendritic cell and NK cell reciprocal cross talk promotes gamma interferon-dependent immunity to blood-stage Plasmodium chabaudi AS infection in mice. Infect Immun (2009) 77(2):770–82. doi: 10.1128/IAI.00994-08
62. King T, Lamb T. Interferon-γ: the jekyll and hyde of malaria. PloS Pathog (2015) 11(10):e1005118. doi: 10.1371/journal.ppat.1005118
63. Schwarzer E, Alessio M, Ulliers D, Arese P. Phagocytosis of the malarial pigment, hemozoin, impairs expression of major histocompatibility complex class II antigen, CD54, and CD11c in human monocytes. Infect Immun (1998) 66(4):1601–6. doi: 10.1128/IAI.66.4.1601-1606.1998
64. Skorokhod OA, Alessio M, Mordmüller B, Arese P, Schwarzer E. Hemozoin (malarial pigment) inhibits differentiation and maturation of human monocyte-derived dendritic cells: a peroxisome proliferator-activated receptor-gamma-mediated effect. J Immunol (2004) 173(6):4066–74. doi: 10.4049/jimmunol.173.6.4066
65. Schwarzer E, Bellomo G, Giribaldi G, Ulliers D, Arese P. Phagocytosis of malarial pigment haemozoin by human monocytes: a confocal microscopy study. Parasitology (2001) 123(Pt 2):125–31. doi: 10.1017/S0031182001008216
66. Schwarzer E, Turrini F, Ulliers D, Giribaldi G, Ginsburg H, Arese P. Impairment of macrophage functions after ingestion of Plasmodium falciparum-infected erythrocytes or isolated malarial pigment. J Exp Med (1992) 176(4):1033–41. doi: 10.1084/jem.176.4.1033
67. Wu X, Gowda NM, Gowda DC. Phagosomal acidification prevents macrophage inflammatory cytokine production to malaria, and dendritic cells are the major source at the early stages of infection: IMPLICATION FOR MALARIA PROTECTIVE IMMUNITY DEVELOPMENT. J Biol Chem (2015) 290(38):23135–47. doi: 10.1074/jbc.M115.671065
68. Gowda DC, Wu X. Parasite recognition and signaling mechanisms in innate immune responses to malaria. Front Immunol (2018) 9:3006. doi: 10.3389/fimmu.2018.03006
69. Raybarman C, Bhattacharjee S. Central and local controls of monocytopoiesis influence the outcome of Leishmania infection. Cytokine (2021) 147:155325. doi: 10.1016/j.cyto.2020.155325
70. Maizels RM, McSorley HJ. Regulation of the host immune system by helminth parasites. J Allergy Clin Immunol (2016) 138(3):666–75. doi: 10.1016/j.jaci.2016.07.007
71. Wiedemann M, Voehringer D. Immunomodulation and immune escape strategies of gastrointestinal helminths and schistosomes. Front Immunol (2020) 11:572865. doi: 10.3389/fimmu.2020.572865
72. de Los Reyes Jiménez M, Lechner A, Alessandrini F, Bohnacker S, Schindela S, Trompette A, et al. An anti-inflammatory eicosanoid switch mediates the suppression of type-2 inflammation by helminth larval products. Sci Transl Med (2020) 12(540):eaay0605. doi: 10.1126/scitranslmed.aay0605
73. Drurey C, Lindholm HT, Coakley G, Poveda MC, Löser S, Doolan R, et al. Intestinal epithelial tuft cell induction is negated by a murine helminth and its secreted products. J Exp Med (2022) 219(1):e20211140. doi: 10.1084/jem.20211140
74. Osbourn M, Soares DC, Vacca F, Cohen ES, Scott IC, Gregory WF, et al. HpARI protein secreted by a helminth parasite suppresses interleukin-33. Immunity (2017) 47(4):739–51.e5. doi: 10.1016/j.immuni.2017.09.015
75. Hartung F, Esser-von Bieren J. Trained immunity in type 2 immune responses. Mucosal Immunol (2022) 15(6):1158–69. doi: 10.1038/s41385-022-00557-0
76. Yasuda K, Adachi T, Koida A, Nakanishi K. Nematode-infected mice acquire resistance to subsequent infection with unrelated nematode by inducing highly responsive group 2 innate lymphoid cells in the lung. Front Immunol (2018) 9:2132. doi: 10.3389/fimmu.2018.02132
77. Martinez-Gonzalez I, Mathä L, Steer CA, Ghaedi M, Poon GF, Takei F. Allergen-experienced group 2 innate lymphoid cells acquire memory-like properties and enhance allergic lung inflammation. Immunity (2016) 45(1):198–208. doi: 10.1016/j.immuni.2016.06.017
78. Lechner A, Henkel FDR, Hartung F, Bohnacker S, Alessandrini F, Gubernatorova EO, et al. Macrophages acquire a TNF-dependent inflammatory memory in allergic asthma. J Allergy Clin Immunol (2022) 149(6):2078–90. doi: 10.1016/j.jaci.2021.11.026
79. Gazzinelli-Guimaraes PH, de Queiroz Prado R, Ricciardi A, Bonne-Année S, Sciurba J, Karmele EP, et al. Allergen presensitization drives an eosinophil-dependent arrest in lung-specific helminth development. J Clin Invest (2019) 129(9):3686–701. doi: 10.1172/JCI127963
80. Quinn SM, Cunningham K, Raverdeau M, Walsh RJ, Curham L, Malara A, et al. Anti-inflammatory trained immunity mediated by helminth products attenuates the induction of T cell-mediated autoimmune disease. Front Immunol (2019) 10:1109. doi: 10.3389/fimmu.2019.01109
81. Cortés A, Muñoz-Antoli C, Esteban JG, Toledo R. Th2 and th1 responses: clear and hidden sides of immunity against intestinal helminths. Trends Parasitol (2017) 33(9):678–93. doi: 10.1016/j.pt.2017.05.004
82. Jankovic D, Sher A, Yap G. Th1/Th2 effector choice in parasitic infection: decision making by committee. Curr Opin Immunol (2001) 13(4):403–9. doi: 10.1016/S0952-7915(00)00234-X
83. Mutapi F, Billingsley PF, Secor WE. Infection and treatment immunizations for successful parasite vaccines. Trends Parasitol (2013) 29(3):135–41. doi: 10.1016/j.pt.2013.01.003
84. Hu Y, Sun L, Yuan Z, Xu Y, Cao J. High throughput data analyses of the immune characteristics of Microtus fortis infected with Schistosoma japonicum. Sci Rep (2017) 7(1):11311. doi: 10.1038/s41598-017-11532-2
85. Li Z, Zhao ZJ, Zhu XQ, Ren QS, Nie FF, Gao JM, et al. Differences in iNOS and arginase expression and activity in the macrophages of rats are responsible for the resistance against T. gondii infection. PloS One (2012) 7(4):e35834. doi: 10.1371/journal.pone.0035834
86. McLaren DJ, James SL. Ultrastructural studies of the killing of schistosomula of Schistosoma mansoni by activated macrophages in vitro. Parasite Immunol (1985) 7(3):315–31. doi: 10.1111/j.1365-3024.1985.tb00079.x
87. James SL, Glaven J. Macrophage cytotoxicity against schistosomula of Schistosoma mansoni involves arginine-dependent production of reactive nitrogen intermediates. J Immunol (1989) 143(12):4208–12. doi: 10.4049/jimmunol.143.12.4208
88. Hu Y, Lu W, Shen Y, Xu Y, Yuan Z, Zhang C, et al. Immune changes of Schistosoma japonicum infections in various rodent disease models. Exp Parasitol (2012) 131(2):180–9. doi: 10.1016/j.exppara.2012.03.022
89. Huang SC, Freitas TC, Amiel E, Everts B, Pearce EL, Lok JB, et al. Fatty acid oxidation is essential for egg production by the parasitic flatworm Schistosoma mansoni. PloS Pathog (2012) 8(10):e1002996. doi: 10.1371/journal.ppat.1002996
90. Shen J, Lai D-H, Wilson RA, Chen Y-F, Wang L-F, Yu Z-L, et al. Nitric oxide blocks the development of the human parasite Schistosoma japonicum. Proc Natl Acad Sci United States America (2017) 114(38):10214–9. doi: 10.1073/pnas.1708578114
91. Knopf PM, Soliman M. Effects of host endocrine gland removal on the permissive status of laboratory rodents to infection by Schistosoma mansoni. Int J Parasitol (1980) 10(3):197–204. doi: 10.1016/0020-7519(80)90049-1
92. Pereira AS, Padilha RJ, Lima-Filho JL, Chaves ME. Scanning electron microscopy of the human low-density lipoprotein interaction with the tegument of Schistosoma mansoni. Parasitol Res (2011) 109(5):1395–402. doi: 10.1007/s00436-011-2386-4
93. Faghiri Z, Skelly PJ. The role of tegumental aquaporin from the human parasitic worm, Schistosoma mansoni, in osmoregulation and drug uptake. FASEB J (2009) 23(8):2780–9. doi: 10.1096/fj.09-130757
94. Netea MG, Quintin J, van der Meer JW. Trained immunity: a memory for innate host defense. Cell Host Microbe (2011) 9(5):355–61. doi: 10.1016/j.chom.2011.04.006
95. Netea MG, Joosten LA, Latz E, Mills KH, Natoli G, Stunnenberg HG, et al. Trained immunity: A program of innate immune memory in health and disease. Science (2016) 352(6284):aaf1098. doi: 10.1126/science.aaf1098
96. Bekkering S, Domínguez-Andrés J, Joosten LAB, Riksen NP, Netea MG. Trained immunity: reprogramming innate immunity in health and disease. Annu Rev Immunol (2021) 39:667–93. doi: 10.1146/annurev-immunol-102119-073855
97. Zhao S, Zhong Y, Fu X, Wang Y, Ye P, Cai J, et al. H3K4 methylation regulates LPS-induced proinflammatory cytokine expression and release in macrophages. Shock (2019) 51(3):401–6. doi: 10.1097/SHK.0000000000001141
98. Netea MG, Schlitzer A, Placek K, Joosten LAB, Schultze JL. Innate and adaptive immune memory: an evolutionary continuum in the host's response to pathogens. Cell Host Microbe (2019) 25(1):13–26. doi: 10.1016/j.chom.2018.12.006
99. Kleinnijenhuis J, Quintin J, Preijers F, Joosten LA, Ifrim DC, Saeed S, et al. Bacille Calmette-Guerin induces NOD2-dependent nonspecific protection from reinfection via epigenetic reprogramming of monocytes. Proc Natl Acad Sci U S A (2012) 109(43):17537–42. doi: 10.1073/pnas.1202870109
100. Ifrim DC, Quintin J, Joosten LA, Jacobs C, Jansen T, Jacobs L, et al. Trained immunity or tolerance: opposing functional programs induced in human monocytes after engagement of various pattern recognition receptors. Clin Vaccine Immunol (2014) 21(4):534–45. doi: 10.1128/CVI.00688-13
101. Quintin J, Saeed S, Martens JHA, Giamarellos-Bourboulis EJ, Ifrim DC, Logie C, et al. Candida albicans infection affords protection against reinfection via functional reprogramming of monocytes. Cell Host Microbe (2012) 12(2):223–32. doi: 10.1016/j.chom.2012.06.006
102. Saeed S, Quintin J, Kerstens HH, Rao NA, Aghajanirefah A, Matarese F, et al. Epigenetic programming of monocyte-to-macrophage differentiation and trained innate immunity. Science (2014) 345(6204):1251086. doi: 10.1126/science.1251086
103. O'Leary JG, Goodarzi M, Drayton DL, von Andrian UH. T cell- and B cell-independent adaptive immunity mediated by natural killer cells. Nat Immunol (2006) 7(5):507–16. doi: 10.1038/ni1332
104. Sun JC, Beilke JN, Lanier LL. Adaptive immune features of natural killer cells. Nature (2009) 457(7229):557–61. doi: 10.1038/nature07665
105. Lewis JS, Lee JA, Underwood JC, Harris AL, Lewis CE. Macrophage responses to hypoxia: relevance to disease mechanisms. J Leukoc Biol (1999) 66(6):889–900. doi: 10.1002/jlb.66.6.889
106. Geissmann F, Manz MG, Jung S, Sieweke MH, Merad M, Ley K. Development of monocytes, macrophages, and dendritic cells. Science (2010) 327(5966):656–61. doi: 10.1126/science.1178331
107. Auffray C, Sieweke MH, Geissmann F. Blood monocytes: development, heterogeneity, and relationship with dendritic cells. Annu Rev Immunol (2009) 27:669–92. doi: 10.1146/annurev.immunol.021908.132557
108. Sica A, Mantovani A. Macrophage plasticity and polarization: in vivo veritas. J Clin Invest (2012) 122(3):787–95. doi: 10.1172/JCI59643
109. Netea MG, Nold-Petry CA, Nold MF, Joosten LA, Opitz B, van der Meer JH, et al. Differential requirement for the activation of the inflammasome for processing and release of IL-1beta in monocytes and macrophages. Blood (2009) 113(10):2324–35. doi: 10.1182/blood-2008-03-146720
110. Riksen NP, Netea MG. Immunometabolic control of trained immunity. Mol Aspects Med (2021) 77:100897. doi: 10.1016/j.mam.2020.100897
111. Levine AJ, Puzio-Kuter AM. The control of the metabolic switch in cancers by oncogenes and tumor suppressor genes. Science (2010) 330(6009):1340–4. doi: 10.1126/science.1193494
112. Finlay DK. Regulation of glucose metabolism in T cells: new insight into the role of phosphoinositide 3-kinases. Front Immunol (2012) 3. doi: 10.3389/fimmu.2012.00247
113. Chen J, Ivashkiv LB. IFN-γ abrogates endotoxin tolerance by facilitating Toll-like receptor-induced chromatin remodeling. Proc Natl Acad Sci U S A (2010) 107(45):19438–43. doi: 10.1073/pnas.1007816107
114. Cheng SC, Quintin J, Cramer RA, Shepardson KM, Saeed S, Kumar V, et al. mTOR- and HIF-1α-mediated aerobic glycolysis as metabolic basis for trained immunity. Science (2014) 345(6204):1250684. doi: 10.1126/science.1250684
115. Arts RJ, Novakovic B, Ter Horst R, Carvalho A, Bekkering S, Lachmandas E, et al. Glutaminolysis and fumarate accumulation integrate immunometabolic and epigenetic programs in trained immunity. Cell Metab (2016) 24(6):807–19. doi: 10.1016/j.cmet.2016.10.008
116. Kleinnijenhuis J, Quintin J, Preijers F, Joosten LA, Jacobs C, Xavier RJ, et al. BCG-induced trained immunity in NK cells: Role for non-specific protection to infection. Clin Immunol (2014) 155(2):213–9. doi: 10.1016/j.clim.2014.10.005
117. Leentjens J, Bekkering S, Joosten LAB, Netea MG, Burgner DP, Riksen NP. Trained innate immunity as a novel mechanism linking infection and the development of atherosclerosis. Circ Res (2018) 122(5):664–9. doi: 10.1161/CIRCRESAHA.117.312465
118. Arts RJW, Carvalho A, La Rocca C, Palma C, Rodrigues F, Silvestre R, et al. Immunometabolic pathways in BCG-induced trained immunity. Cell Rep (2016) 17(10):2562–71. doi: 10.1016/j.celrep.2016.11.011
119. DeBerardinis RJ, Cheng T. Q's next: the diverse functions of glutamine in metabolism, cell biology and cancer. Oncogene (2010) 29(3):313–24. doi: 10.1038/onc.2009.358
120. Iacobazzi V, Infantino V. Citrate - new functions for an old metabolite. Biol Chem (2014) 395(4):387–99. doi: 10.1515/hsz-2013-0271
121. Morciano P, Carrisi C, Capobianco L, Mannini L, Burgio G, Cestra G, et al. A conserved role for the mitochondrial citrate transporter Sea/SLC25A1 in the maintenance of chromosome integrity. Hum Mol Genet (2009) 18(21):4180–8. doi: 10.1093/hmg/ddp370
122. Mills E, O'Neill LAJ. Succinate: a metabolic signal inflammation. Trends Cell Biol (2014) 24(5):313–20. doi: 10.1016/j.tcb.2013.11.008
123. Koivunen P, Tiainen P, Hyvarinen J, Williams KE, Sormunen R, Klaus SJ, et al. An endoplasmic reticulum transmembrane prolyl 4-hydroxylase is induced by hypoxia and acts on hypoxia-inducible factor alpha. J Biol Chem (2007) 282(42):30544–52. doi: 10.1074/jbc.M704988200
124. Tannahill GM, Curtis AM, Adamik J, Palsson-McDermott EM, McGettrick AF, Goel G, et al. Succinate is an inflammatory signal that induces IL-1β through HIF-1α. Nature (2013) 496(7444):238–42. doi: 10.1038/nature11986
125. Donohoe DR, Bultman SJ. Metaboloepigenetics: interrelationships between energy metabolism and epigenetic control of gene expression. J Cell Physiol (2012) 227(9):3169–77. doi: 10.1002/jcp.24054
126. Domínguez-Andrés J, Novakovic B, Li Y, Scicluna BP, Gresnigt MS, Arts RJW, et al. The itaconate pathway is a central regulatory node linking innate immune tolerance and trained immunity. Cell Metab (2019) 29(1):211–20.e5. doi: 10.1016/j.cmet.2018.09.003
127. Lampropoulou V, Sergushichev A, Bambouskova M, Nair S, Vincent EE, Loginicheva E, et al. Itaconate links inhibition of succinate dehydrogenase with macrophage metabolic remodeling and regulation of inflammation. Cell Metab (2016) 24(1):158–66. doi: 10.1016/j.cmet.2016.06.004
128. Cordes T, Wallace M, Michelucci A, Divakaruni AS, Sapcariu SC, Sousa C, et al. Immunoresponsive gene 1 and itaconate inhibit succinate dehydrogenase to modulate intracellular succinate levels. J Biol Chem (2016) 291(27):14274–84. doi: 10.1074/jbc.M115.685792
129. Li Y, Zhang P, Wang C, Han C, Meng J, Liu X, et al. Immune responsive gene 1 (IRG1) promotes endotoxin tolerance by increasing A20 expression in macrophages through reactive oxygen species. J Biol Chem (2013) 288(23):16225–34. doi: 10.1074/jbc.M113.454538
130. Lussey-Lepoutre C, Hollinshead KE, Ludwig C, Menara M, Morin A, Castro-Vega LJ, et al. Loss of succinate dehydrogenase activity results in dependency on pyruvate carboxylation for cellular anabolism. Nat Commun (2015) 6:8784. doi: 10.1038/ncomms9784
131. Mills EL, Kelly B, Logan A, Costa ASH, Varma M, Bryant CE, et al. Succinate dehydrogenase supports metabolic repurposing of mitochondria to drive inflammatory macrophages. Cell (2016) 167(2):457–70.e13. doi: 10.1016/j.cell.2016.08.064
132. van der Heijden C, Noz MP, Joosten LAB, Netea MG, Riksen NP, Keating ST. Epigenetics and trained immunity. Antioxid Redox Signal (2018) 29(11):1023–40. doi: 10.1089/ars.2017.7310
133. Garcia-Valtanen P, Guzman-Genuino RM, Williams DL, Hayball JD, Diener KR. Evaluation of trained immunity by β-1, 3 (d)-glucan on murine monocytes in vitro and duration of response in vivo. Immunol Cell Biol (2017) 95(7):601–10. doi: 10.1038/icb.2017.13
134. Lum JJ, Bui T, Gruber M, Gordan JD, DeBerardinis RJ, Covello KL, et al. The transcription factor HIF-1 alpha plays a critical role in the growth factor-dependent regulation of both aerobic and anaerobic glycolysis. Genes Dev (2007) 21(9):1037–49. doi: 10.1101/gad.1529107
135. Israel M, Schwartz L. The metabolic advantage of tumor cells. Mol Cancer (2011) 10:70. doi: 10.1186/1476-4598-10-70
136. Liu TF, Vachharajani VT, Yoza BK, McCall CE. NAD+-dependent sirtuin 1 and 6 proteins coordinate a switch from glucose to fatty acid oxidation during the acute inflammatory response. J Biol Chem (2012) 287(31):25758–69. doi: 10.1074/jbc.M112.362343
137. Kroemer G, Pouyssegur J. Tumor cell metabolism: cancer's Achilles' heel. Cancer Cell (2008) 13(6):472–82. doi: 10.1016/j.ccr.2008.05.005
138. Gruenbacher G, Thurnher M. Mevalonate metabolism governs cancer immune surveillance. Oncoimmunology (2017) 6(10):e1342917. doi: 10.1080/2162402X.2017.1342917
139. O'Sullivan D, van der Windt GJ, Huang SC, Curtis JD, Chang CH, Buck MD, et al. Memory CD8(+) T cells use cell-intrinsic lipolysis to support the metabolic programming necessary for development. Immunity (2014) 41(1):75–88. doi: 10.1016/j.immuni.2014.06.005
140. Thurnher M, Gruenbacher G. T lymphocyte regulation by mevalonate metabolism. Sci Signal (2015) 8(370):re4. doi: 10.1126/scisignal.2005970
141. Bekkering S, Arts RJW, Novakovic B, Kourtzelis I, van der Heijden C, Li Y, et al. Metabolic induction of trained immunity through the mevalonate pathway. Cell (2018) 172(1-2):135–46.e9. doi: 10.1016/j.cell.2017.11.025
142. Bianchi ME. DAMPs, PAMPs and alarmins: all we need to know about danger. J Leukocyte Biol (2007) 81(1):1–5. doi: 10.1189/jlb.0306164
143. Kawai T, Akira S. The role of pattern-recognition receptors in innate immunity: update on Toll-like receptors. Nat Immunol (2010) 11(5):373–84. doi: 10.1038/ni.1863
144. Yuan R, Li L. Dynamic modulation of innate immunity programming and memory. Sci China-Life Sci (2016) 59(1):38–43. doi: 10.1007/s11427-015-4998-x
145. DeBerardinis RJ, Mancuso A, Daikhin E, Nissim I, Yudkoff M, Wehrli S, et al. Beyond aerobic glycolysis: Transformed cells can engage in glutamine metabolism that exceeds the requirement for protein and nucleotide synthesis. Proc Natl Acad Sci United States America (2007) 104(49):19345–50. doi: 10.1073/pnas.0709747104
146. Nicodeme E, Jeffrey KL, Schaefer U, Beinke S, Dewell S, Chung C-w, et al. Suppression of inflammation by a synthetic histone mimic. Nature (2010) 468(7327):1119–23. doi: 10.1038/nature09589
147. Mehta S, Jeffrey KL. Beyond receptors and signaling: epigenetic factors in the regulation of innate immunity. Immunol Cell Biol (2015) 93(3):233–44. doi: 10.1038/icb.2014.101
148. Ostuni R, Piccolo V, Barozzi I, Polletti S, Termanini A, Bonifacio S, et al. Latent enhancers activated by stimulation in differentiated cells. Cell (2013) 152(1-2):157–71. doi: 10.1016/j.cell.2012.12.018
149. Incalcaterra S, Dominguez JA. Trained Immunity at a Glance; A Review on the Innate Immune Memory and its Potential Role in Infections, Diseases and New Therapeutic Strategies. Adv J Graduate Res (2020) 8(1):68–81. doi: 10.21467/ajgr.8.1.68-81
150. Barski A, Cuddapah S, Cui K, Roh T-Y, Schones DE, Wang Z, et al. High-resolution profiling of histone methylations in the human genome. Cell (2007) 129(4):823–37. doi: 10.1016/j.cell.2007.05.009
151. Carson WF, Cavassani KA, Dou Y, Kunkel SL. Epigenetic regulation of immune cell functions during post-septic immunosuppression. Epigenetics (2011) 6(3):273–83. doi: 10.4161/epi.6.3.14017
152. Park SH, Park-Min KH, Chen J, Hu X, Ivashkiv LB. Tumor necrosis factor induces GSK3 kinase-mediated cross-tolerance to endotoxin in macrophages. Nat Immunol (2011) 12(7):607–15. doi: 10.1038/ni.2043
153. Zhang X, Morrison DC. Lipopolysaccharide structure-function relationship in activation versus reprogramming of mouse peritoneal macrophages. J Leukocyte Biol (1993) 54(5):444–50. doi: 10.1002/jlb.54.5.444
154. Borriello F, Iannone R, Di Somma S, Loffredo S, Scamardella E, Galdiero MR, et al. GM-CSF and IL-3 modulate human monocyte TNF-alpha production and renewal in in vitro models of trained immunity. Front Immunol (2017) 7. doi: 10.3389/fimmu.2016.00680
155. Khader SA, Divangahi M, Hanekom W, Hill PC, Maeurer M, Makar KW, et al. Targeting innate immunity for tuberculosis vaccination. J Clin Invest (2019) 129(9):3482–91. doi: 10.1172/JCI128877
156. Morris M, Li L. Molecular mechanisms and pathological consequences of endotoxin tolerance and priming. Arch Immunol Ther Exp (Warsz) (2012) 60(1):13–8. doi: 10.1007/s00005-011-0155-9
157. Foster SL, Hargreaves DC, Medzhitov R. Gene-specific control of inflammation by TLR-induced chromatin modifications. Nature (2007) 447(7147):972–8. doi: 10.1038/nature05836
158. Lee KK, Workman JL. Histone acetyltransferase complexes: one size doesn't fit all. Nat Rev Mol Cell Biol (2007) 8(4):284–95. doi: 10.1038/nrm2145
159. Cervera AM, Bayley J-P, Devilee P, McCreath KJ. Inhibition of succinate dehydrogenase dysregulates histone modification in mammalian cells. Mol Cancer (2009) 8(1):89. doi: 10.1186/1476-4598-8-89
160. Lu C, Ward PS, Kapoor GS, Rohle D, Turcan S, Abdel-Wahab O, et al. IDH mutation impairs histone demethylation and results in a block to cell differentiation. Nature (2012) 483(7390):474–8. doi: 10.1038/nature10860
161. Arts RJ, Blok BA, Aaby P, Joosten LA, de Jong D, van der Meer JW, et al. Long-term in vitro and in vivo effects of γ-irradiated BCG on innate and adaptive immunity. J Leukoc Biol (2015) 98(6):995–1001. doi: 10.1189/jlb.4MA0215-059R
162. Carey BW, Finley LWS, Cross JR, Allis CD, Thompson CB. Intracellular alpha-ketoglutarate maintains the pluripotency of embryonic stem cells. Nature (2015) 518(7539):413–6. doi: 10.1038/nature13981
163. Schrum JE, Crabtree JN, Dobbs KR, Kiritsy MC, Reed GW, Gazzinelli RT, et al. Cutting edge: plasmodium falciparum induces trained innate immunity. J Immunol (2018) 200(4):1243–8. doi: 10.4049/jimmunol.1701010
164. Roth A, Gustafson P, Nhaga AR, Djana Q, Poulsen A, Garly ML, et al. BCG vaccination scar associated with better childhood survival in Guinea-Bissau. Int J Epidemiol (2005) 34(3):540–7. doi: 10.1093/ije/dyh392
165. Kleinnijenhuis J, Quintin J, Preijers F, Benn CS, Joosten LA, Jacobs C, et al. Long-lasting effects of BCG vaccination on both heterologous Th1/Th17 responses and innate trained immunity. J Innate Immun (2014) 6(2):152–8. doi: 10.1159/000355628
166. Walk J, de Bree LCJ, Graumans W, Stoter R, van Gemert G-J, van de Vegte-Bolmer M, et al. Outcomes of controlled human malaria infection after BCG vaccination. Nat Commun (2019) 10(1):874. doi: 10.1038/s41467-019-08659-3
167. Silva MVT, Dos Santos JC, Figueiredo AMB, Teufel LU, Pereira JX, Matos GG, et al. The role of IL-32 in Bacillus Calmette-Guérin (BCG)-induced trained immunity in infections caused by different Leishmania spp. Microb Pathog (2021) 158:105088. doi: 10.1016/j.micpath.2021.105088
168. Dos Santos JC, Barroso de Figueiredo AM, Teodoro Silva MV, Cirovic B, de Bree LCJ, Damen M, et al. β-Glucan-Induced Trained Immunity Protects against Leishmania Braziliensis Infection: a Crucial Role for IL-32. Cell Rep (2019) 28(10):2659–72.e6. doi: 10.1016/j.celrep.2019.08.004
169. Dos Santos JC, Heinhuis B, Gomes RS, Damen MS, Real F, Mortara RA, et al. Cytokines and microbicidal molecules regulated by IL-32 in THP-1-derived human macrophages infected with New World Leishmania species. PloS Negl Trop Dis (2017) 11(2):e0005413. doi: 10.1371/journal.pntd.0005413
170. Kulkarni MM, Barbi J, McMaster WR, Gallo RL, Satoskar AR, McGwire BS. Mammalian antimicrobial peptide influences control of cutaneous Leishmania infection. Cell Microbiol (2011) 13(6):913–23. doi: 10.1111/j.1462-5822.2011.01589.x
171. Santangelo MP, McIntosh D, Bigi F, Armôa GR, Campos AS, Ruybal P, et al. Mycobacterium bovis BCG as a delivery system for the RAP-1 antigen from Babesia bovis. Vaccine (2007) 25(6):1104–13. doi: 10.1016/j.vaccine.2006.09.069
172. Bastos RG, Alzan HF, Rathinasamy VA, Cooke BM, Dellagostin OA, Barletta RG, et al. Harnessing mycobacterium bovis BCG trained immunity to control human and bovine babesiosis. Vaccines (Basel) (2022) 10(1):123. doi: 10.3390/vaccines10010123
173. Benn CS, Netea MG, Selin LK, Aaby P. A small jab - a big effect: nonspecific immunomodulation by vaccines. Trends Immunol (2013) 34(9):431–9. doi: 10.1016/j.it.2013.04.004
174. Aaby P, Roth A, Ravn H, Napirna BM, Rodrigues A, Lisse IM, et al. Randomized trial of BCG vaccination at birth to low-birth-weight children: beneficial nonspecific effects in the neonatal period? J Infect Dis (2011) 204(2):245–52. doi: 10.1093/infdis/jir240
175. Aaby P, Martins CL, Garly ML, Balé C, Andersen A, Rodrigues A, et al. Non-specific effects of standard measles vaccine at 4.5 and 9 months of age on childhood mortality: randomised controlled trial. Bmj (2010) 341:c6495. doi: 10.1136/bmj.c6495
176. Sánchez-Ramón S, Conejero L, Netea MG, Sancho D, Palomares Ó, Subiza JL. Trained immunity-based vaccines: A new paradigm for the development of broad-spectrum anti-infectious formulations. Front Immunol (2018) 9:2936. doi: 10.3389/fimmu.2018.02936
177. Moorlag S, Rodriguez-Rosales YA, Gillard J, Fanucchi S, Theunissen K, Novakovic B, et al. BCG vaccination induces long-term functional reprogramming of human neutrophils. Cell Rep (2020) 33(7):108387. doi: 10.1016/j.celrep.2020.108387
178. Kalafati L, Hatzioannou A, Hajishengallis G, Chavakis T. The role of neutrophils in trained immunity. Immunol Rev (2023) 314(1):142–57. doi: 10.1111/imr.13142
179. Kalafati L, Kourtzelis I, Schulte-Schrepping J, Li X, Hatzioannou A, Grinenko T, et al. Innate immune training of granulopoiesis promotes anti-tumor activity. Cell (2020) 183(3):771–85.e12. doi: 10.1016/j.cell.2020.09.058
180. Halim TY, Steer CA, Mathä L, Gold MJ, Martinez-Gonzalez I, McNagny KM, et al. Group 2 innate lymphoid cells are critical for the initiation of adaptive T helper 2 cell-mediated allergic lung inflammation. Immunity (2014) 40(3):425–35. doi: 10.1016/j.immuni.2014.01.011
181. Serafini N, Jarade A, Surace L, Goncalves P, Sismeiro O, Varet H, et al. Trained ILC3 responses promote intestinal defense. Science (2022) 375(6583):859–63. doi: 10.1126/science.aaz8777
182. Sun JC, Madera S, Bezman NA, Beilke JN, Kaplan MH, Lanier LL. Proinflammatory cytokine signaling required for the generation of natural killer cell memory. J Exp Med (2012) 209(5):947–54. doi: 10.1084/jem.20111760
183. Cai J, Huang L, Wang LJ, Zheng MH, Liu H. The role of macrophage polarization in parasitic infections: a review. Zhongguo Xue Xi Chong Bing Fang Zhi Za Zhi Chin J Schistosomiasis Control (2020) 32(4):432–5. doi: 10.16250/j.32.1374.2019252
184. Hesse M, Modolell M, La Flamme AC, Schito M, Fuentes JM, Cheever AW, et al. Differential regulation of nitric oxide synthase-2 and arginase-1 by type 1/type 2 cytokines in vivo: granulomatous pathology is shaped by the pattern of L-arginine metabolism. J Immunol (2001) 167(11):6533–44. doi: 10.4049/jimmunol.167.11.6533
185. Muraille E, Leo O, Moser M. TH1/TH2 paradigm extended: macrophage polarization as an unappreciated pathogen-driven escape mechanism? Front Immunol (2014) 5:603. doi: 10.3389/fimmu.2014.00603
186. Riksen NP. Trained immunity and atherosclerotic cardiovascular disease. Curr Opin Lipidol (2019) 30(5):395–400. doi: 10.1097/MOL.0000000000000628
187. Kaufmann E, Sanz J, Dunn JL, Khan N, Mendonça LE, Pacis A, et al. BCG educates hematopoietic stem cells to generate protective innate immunity against tuberculosis. Cell (2018) 172(1-2):176–90.e19. doi: 10.1016/j.cell.2017.12.031
188. Nagy C, Haschemi A. Time and demand are two critical dimensions of immunometabolism: the process of macrophage activation and the pentose phosphate pathway. Front Immunol (2015) 6:164. doi: 10.3389/fimmu.2015.00164
189. Ganeshan K, Chawla A. Metabolic regulation of immune responses. Annu Rev Immunol (2014) 32:609–34. doi: 10.1146/annurev-immunol-032713-120236
190. O'Neill LA, Kishton RJ, Rathmell J. A guide to immunometabolism for immunologists. Nat Rev Immunol (2016) 16(9):553–65. doi: 10.1038/nri.2016.70
191. Pearce EL, Pearce EJ. Metabolic pathways in immune cell activation and quiescence. Immunity (2013) 38(4):633–43. doi: 10.1016/j.immuni.2013.04.005
192. O'Neill LAJ, Pearce EJ. Immunometabolism governs dendritic cell and macrophage function. J Exp Med (2016) 213(1):15–23. doi: 10.1084/jem.20151570
193. Odegaard JI, Chawla A. Alternative macrophage activation and metabolism. In: Abbas AK, Galli SJ, Howley PM, editors. Annual Review of Pathology: Mechanisms of Disease, Vol 6. Annual Review of Pathology-Mechanisms of Disease. USA: Annual Reviews Inc. (2011) 62011:275–97.
194. Hao J, Hu Y, Li Y, Zhou Q, Lv X. Involvement of JNK signaling in IL4-induced M2 macrophage polarization. Exp Cell Res (2017) 357(2):155–62. doi: 10.1016/j.yexcr.2017.05.010
195. Van den Bossche J, O'Neill LA, Menon D. Macrophage immunometabolism: where are we (Going). Trends Immunol (2017) 38(6):395–406. doi: 10.1016/j.it.2017.03.001
196. Xing Z, Afkhami S, Bavananthasivam J, Fritz DK, D'Agostino MR, Vaseghi-Shanjani M, et al. Innate immune memory of tissue-resident macrophages and trained innate immunity: Re-vamping vaccine concept and strategies. J Leukoc Biol (2020) 108(3):825–34. doi: 10.1002/JLB.4MR0220-446R
197. Novakovic B, Habibi E, Wang SY, Arts RJW, Davar R, Megchelenbrink W, et al. β-glucan reverses the epigenetic state of LPS-induced immunological tolerance. Cell (2016) 167(5):1354–68.e14. doi: 10.1016/j.cell.2016.09.034
198. Rusek P, Wala M, Druszczyńska M, Fol M. Infectious agents as stimuli of trained innate immunity. Int J Mol Sci (2018) 19(2):456. doi: 10.3390/ijms19020456
199. Bénit P, Letouzé E, Rak M, Aubry L, Burnichon N, Favier J, et al. Unsuspected task for an old team: succinate, fumarate and other Krebs cycle acids in metabolic remodeling. Biochim Biophys Acta (2014) 1837(8):1330–7. doi: 10.1016/j.bbabio.2014.03.013
200. Ferluga J, Yasmin H, Al-Ahdal MN, Bhakta S, Kishore U. Natural and trained innate immunity against Mycobacterium tuberculosis. Immunobiology (2020) 225(3):1326–38. doi: 10.1016/j.imbio.2020.151951
201. Xiao M, Yang H, Xu W, Ma S, Lin H, Zhu H, et al. Inhibition of α-KG-dependent histone and DNA demethylases by fumarate and succinate that are accumulated in mutations of FH and SDH tumor suppressors. Genes Dev (2012) 26(12):1326–38. doi: 10.1101/gad.191056.112
202. Jones PA. Functions of DNA methylation: islands, start sites, gene bodies and beyond. Nat Rev Genet (2012) 13(7):484–92. doi: 10.1038/nrg3230
203. Greer EL, Shi Y. Histone methylation: a dynamic mark in health, disease and inheritance. Nat Rev Genet (2012) 13(5):343–57. doi: 10.1038/nrg3173
204. Cui J, Chen Y, Wang HY, Wang RF. Mechanisms and pathways of innate immune activation and regulation in health and cancer. Hum Vaccin Immunother (2014) 10(11):3270–85. doi: 10.4161/21645515.2014.979640
205. Witztum JL, Lichtman AH. The influence of innate and adaptive immune responses on atherosclerosis. Annu Rev Pathol (2014) 9:73–102. doi: 10.1146/annurev-pathol-020712-163936
206. Sasai M, Pradipta A, Yamamoto M. Host immune responses to Toxoplasma gondii. Int Immunol (2018) 30(3):113–9. doi: 10.1093/intimm/dxy004
207. Toepp AJ, Petersen CA. The balancing act: Immunology of leishmaniosis. Res Vet Sci (2020) 130:19–25. doi: 10.1016/j.rvsc.2020.02.004
208. Sacks D, Noben-Trauth N. The immunology of susceptibility and resistance to Leishmania major in mice. Nat Rev Immunol (2002) 2(11):845–58. doi: 10.1038/nri933
209. Strelko CL, Lu W, Dufort FJ, Seyfried TN, Chiles TC, Rabinowitz JD, et al. Itaconic acid is a mammalian metabolite induced during macrophage activation. J Am Chem Soc (2011) 133(41):16386–9. doi: 10.1021/ja2070889
210. Pesce JT, Ramalingam TR, Mentink-Kane MM, Wilson MS, El Kasmi KC, Smith AM, et al. Arginase-1-expressing macrophages suppress Th2 cytokine-driven inflammation and fibrosis. PloS Pathog (2009) 5(4):e1000371. doi: 10.1371/journal.ppat.1000371
211. Rodríguez-Prados JC, Través PG, Cuenca J, Rico D, Aragonés J, Martín-Sanz P, et al. Substrate fate in activated macrophages: a comparison between innate, classic, and alternative activation. J Immunol (2010) 185(1):605–14. doi: 10.4049/jimmunol.0901698
212. McGettrick AF, O'Neill LA. How metabolism generates signals during innate immunity and inflammation. J Biol Chem (2013) 288(32):22893–8. doi: 10.1074/jbc.R113.486464
213. O'Neill LA. A critical role for citrate metabolism in LPS signalling. Biochem J (2011) 438(3):e5–6. doi: 10.1042/BJ20111386
214. Tilioua S, Mezioug D, Amir-Tidadini ZC, Medjdoub YM, Touil-Boukoffa C. Potential role of NF-κB pathway in the immuno-inflammatory responses during human cystic echinococcosis. Acta Trop (2020) 203:105306. doi: 10.1016/j.actatropica.2019.105306
215. Goodridge HS, Wolf AJ, Underhill DM. Beta-glucan recognition by the innate immune system. Immunol Rev (2009) 230(1):38–50. doi: 10.1111/j.1600-065X.2009.00793.x
216. Steele C, Marrero L, Swain S, Harmsen AG, Zheng M, Brown GD, et al. Alveolar macrophage-mediated killing of Pneumocystis carinii f. sp. muris involves molecular recognition by the Dectin-1 beta-glucan receptor. J Exp Med (2003) 198(11):1677–88. doi: 10.1084/jem.20030932
217. Brown GD, Herre J, Williams DL, Willment JA, Marshall AS, Gordon S. Dectin-1 mediates the biological effects of beta-glucans. J Exp Med (2003) 197(9):1119–24. doi: 10.1084/jem.20021890
218. Li X, Luo H, Ye Y, Chen X, Zou Y, Duan J, et al. β-glucan, a dectin-1 ligand, promotes macrophage M1 polarization via NF-κB/autophagy pathway. Int J Oncol (2019) 54(1):271–82. doi: 10.3892/ijo.2018.4630
219. O'Hagan DT, Valiante NM. Recent advances in the discovery and delivery of vaccine adjuvants. Nat Rev Drug Discovery (2003) 2(9):727–35. doi: 10.1038/nrd1176
220. Guimarães LE, Baker B, Perricone C, Shoenfeld Y. Vaccines, adjuvants and autoimmunity. Pharmacol Res (2015) 100:190–209. doi: 10.1016/j.phrs.2015.08.003
221. Shoenfeld Y, Agmon-Levin N. 'ASIA' - autoimmune/inflammatory syndrome induced by adjuvants. J Autoimmun (2011) 36(1):4–8. doi: 10.1016/j.jaut.2010.07.003
222. Schijns VE. Immunological concepts of vaccine adjuvant activity. Curr Opin Immunol (2000) 12(4):456–63. doi: 10.1016/S0952-7915(00)00120-5
223. Qureshi N, Takayama K, Ribi E. Purification and structural determination of nontoxic lipid A obtained from the lipopolysaccharide of Salmonella typhimurium. J Biol Chem (1982) 257(19):11808–15. doi: 10.1016/S0021-9258(18)33836-5
224. Takayama K, Ribi E, Cantrell JL. Isolation of a nontoxic lipid A fraction containing tumor regression activity. Cancer Res (1981) 41(7):2654–7.
225. RTS S Clinical Trials Partnership. Efficacy and safety of RTS,S/AS01 malaria vaccine with or without a booster dose in infants and children in Africa: final results of a phase 3, individually randomised, controlled trial. Lancet (2015) 386(9988):31–45. doi: 10.1016/S0140-6736(15)60721-8
226. Alonso PL, Sacarlal J, Aponte JJ, Leach A, Macete E, Milman J, et al. Efficacy of the RTS,S/AS02A vaccine against Plasmodium falciparum infection and disease in young African children: randomised controlled trial. Lancet (2004) 364(9443):1411–20. doi: 10.1016/S0140-6736(04)17223-1
227. Carter D, Fox CB, Day TA, Guderian JA, Liang H, Rolf T, et al. A structure-function approach to optimizing TLR4 ligands for human vaccines. Clin Transl Immunol (2016) 5(11):e108. doi: 10.1038/cti.2016.63
228. Lumsden JM, Pichyangkul S, Srichairatanakul U, Yongvanitchit K, Limsalakpetch A, Nurmukhambetova S, et al. Evaluation of the safety and immunogenicity in rhesus monkeys of a recombinant malaria vaccine for Plasmodium vivax with a synthetic Toll-like receptor 4 agonist formulated in an emulsion. Infect Immun (2011) 79(9):3492–500. doi: 10.1128/IAI.05257-11
229. Patra KP, Li F, Carter D, Gregory JA, Baga S, Reed SG, et al. Alga-produced malaria transmission-blocking vaccine candidate Pfs25 formulated with a human use-compatible potent adjuvant induces high-affinity antibodies that block Plasmodium falciparum infection of mosquitoes. Infect Immun (2015) 83(5):1799–808. doi: 10.1128/IAI.02980-14
230. Coler RN, Carter D, Friede M, Reed SG. Adjuvants for malaria vaccines. Parasite Immunol (2009) 31(9):520–8. doi: 10.1111/j.1365-3024.2009.01142.x
231. Fox CB, Moutaftsi M, Vergara J, Desbien AL, Nana GI, Vedvick TS, et al. TLR4 ligand formulation causes distinct effects on antigen-specific cell-mediated and humoral immune responses. Vaccine (2013) 31(49):5848–55. doi: 10.1016/j.vaccine.2013.09.069
232. Baldwin SL, Roeffen W, Singh SK, Tiendrebeogo RW, Christiansen M, Beebe E, et al. Synthetic TLR4 agonists enhance functional antibodies and CD4+ T-cell responses against the Plasmodium falciparum GMZ2.6C multi-stage vaccine antigen. Vaccine (2016) 34(19):2207–15. doi: 10.1016/j.vaccine.2016.03.016
233. Reed SG, Carter D, Casper C, Duthie MS, Fox CB. Correlates of GLA family adjuvants' activities. Semin Immunol (2018) 39:22–9. doi: 10.1016/j.smim.2018.10.004
234. de Laval B, Maurizio J, Kandalla PK, Brisou G, Simonnet L, Huber C, et al. C/EBPβ-dependent epigenetic memory induces trained immunity in hematopoietic stem cells. Cell Stem Cell (2023) 30(1):112. doi: 10.1016/j.stem.2022.12.005
235. Owen AM, Luan L, Burelbach KR, McBride MA, Stothers CL, Boykin OA, et al. MyD88-dependent signaling drives toll-like receptor-induced trained immunity in macrophages. Front Immunol (2022) 13:1044662. doi: 10.3389/fimmu.2022.1044662
236. McBride MA, Owen AM, Stothers CL, Hernandez A, Luan L, Burelbach KR, et al. The metabolic basis of immune dysfunction following sepsis and trauma. Front Immunol (2020) 11:1043. doi: 10.3389/fimmu.2020.01043
237. Fensterheim BA, Young JD, Luan L, Kleinbard RR, Stothers CL, Patil NK, et al. The TLR4 agonist monophosphoryl lipid A drives broad resistance to infection via dynamic reprogramming of macrophage metabolism. J Immunol (2018) 200(11):3777–89. doi: 10.4049/jimmunol.1800085
238. Yang D, Yang L, Cai J, Hu X, Li H, Zhang X, et al. A sweet spot for macrophages: Focusing on polarization. Pharmacol Res (2021) 167:105576. doi: 10.1016/j.phrs.2021.105576
239. Lima-Junior DS, Costa DL, Carregaro V, Cunha LD, Silva AL, Mineo TW, et al. Inflammasome-derived IL-1β production induces nitric oxide-mediated resistance to Leishmania. Nat Med (2013) 19(7):909–15. doi: 10.1038/nm.3221
240. Clark IA, Allison AC, Cox FE. Protection of mice against Babesia and Plasmodium with BCG. Nature (1976) 259(5541):309–11. doi: 10.1038/259309a0
241. Matsumoto S, Yukitake H, Kanbara H, Yamada H, Kitamura A, Yamada T. Mycobacterium bovis bacillus calmette-guérin induces protective immunity against infection by Plasmodium yoelii at blood-stage depending on shifting immunity toward Th1 type and inducing protective IgG2a after the parasite infection. Vaccine (2000) 19(7-8):779–87. doi: 10.1016/S0264-410X(00)00257-7
242. Murphy JR. Host defenses in murine malaria: nonspecific resistance to Plasmodium berghei generated in response to Mycobacterium bovis infection or Corynebacterium parvum stimulation. Infect Immun (1981) 33(1):199–211. doi: 10.1128/iai.33.1.199-211.1981
243. Parra M, Liu X, Derrick SC, Yang A, Tian J, Kolibab K, et al. Molecular analysis of non-specific protection against murine malaria induced by BCG vaccination. PloS One (2013) 8(7):e66115. doi: 10.1371/journal.pone.0066115
244. Sezaki M, Hayashi Y, Wang Y, Johansson A, Umemoto T, Takizawa H. Immuno-modulation of hematopoietic stem and progenitor cells in inflammation. Front Immunol (2020) 11:585367. doi: 10.3389/fimmu.2020.585367
245. Pietras EM. Inflammation: a key regulator of hematopoietic stem cell fate in health and disease. Blood (2017) 130(15):1693–8. doi: 10.1182/blood-2017-06-780882
246. Clapes T, Lefkopoulos S, Trompouki E. Stress and non-stress roles of inflammatory signals during HSC emergence and maintenance. Front Immunol (2016) 7:487. doi: 10.3389/fimmu.2016.00487
247. Takizawa H, Boettcher S, Manz MG. Demand-adapted regulation of early hematopoiesis in infection and inflammation. Blood (2012) 119(13):2991–3002. doi: 10.1182/blood-2011-12-380113
248. Vainieri ML, Blagborough AM, MacLean AL, Haltalli ML, Ruivo N, Fletcher HA, et al. Systematic tracking of altered haematopoiesis during sporozoite-mediated malaria development reveals multiple response points. Open Biol (2016) 6(6):160038 doi: 10.1098/rsob.160038
249. Cunningham KT, Finlay CM, Mills KHG. Helminth imprinting of hematopoietic stem cells sustains anti-inflammatory trained innate immunity that attenuates autoimmune disease. J Immunol (2021) 206(7):1618–30. doi: 10.4049/jimmunol.2001225
250. Doolan R, Putananickal N, Tritten L, Bouchery T. How to train your myeloid cells: a way forward for helminth vaccines? Front Immunol (2023) 14:1163364. doi: 10.3389/fimmu.2023.1163364
251. Mitroulis I, Ruppova K, Wang B, Chen LS, Grzybek M, Grinenko T, et al. Modulation of myelopoiesis progenitors is an integral component of trained immunity. Cell (2018) 172(1-2):147–61.e12. doi: 10.1016/j.cell.2017.11.034
252. Gabrilovich DI, Nagaraj S. Myeloid-derived suppressor cells as regulators of the immune system. Nat Rev Immunol (2009) 9(3):162–74. doi: 10.1038/nri2506
253. Veglia F, Perego M, Gabrilovich D. Myeloid-derived suppressor cells coming of age. Nat Immunol (2018) 19(2):108–19. doi: 10.1038/s41590-017-0022-x
254. Grover A, Sanseviero E, Timosenko E, Gabrilovich DI. Myeloid-derived suppressor cells: A propitious road to clinic. Cancer Discov (2021) 11(11):2693–706. doi: 10.1158/2159-8290.CD-21-0764
255. Zhang Y, Wu Y, Liu H, Gong W, Hu Y, Shen Y, et al. Granulocytic myeloid-derived suppressor cells inhibit T follicular helper cells during experimental Schistosoma japonicum infection. Parasit Vectors (2021) 14(1):497. doi: 10.1186/s13071-021-05006-8
256. Lamsfus Calle C, Fendel R, Singh A, Richie TL, Hoffman SL, Kremsner PG, et al. Expansion of functional myeloid-derived suppressor cells in controlled human malaria infection. Front Immunol (2021) 12:625712. doi: 10.3389/fimmu.2021.625712
257. Fresno M, Gironès N. Myeloid-derived suppressor cells in trypanosoma cruzi infection. Front Cell Infect Microbiol (2021) 11:737364. doi: 10.3389/fcimb.2021.737364
258. Tian X, Tian J, Tang X, Rui K, Zhang Y, Ma J, et al. Particulate β-glucan regulates the immunosuppression of granulocytic myeloid-derived suppressor cells by inhibiting NFIA expression. Oncoimmunology (2015) 4(9):e1038687. doi: 10.1080/2162402X.2015.1038687
259. Albeituni SH, Ding C, Liu M, Hu X, Luo F, Kloecker G, et al. Correction: yeast-derived particulate β-glucan treatment subverts the suppression of myeloid-derived suppressor cells (MDSC) by inducing polymorphonuclear MDSC apoptosis and monocytic MDSC differentiation to APC in cancer. J Immunol (2016) 196(9):3967. doi: 10.4049/jimmunol.1600346
260. Tian J, Ma J, Ma K, Guo H, Baidoo SE, Zhang Y, et al. β-Glucan enhances antitumor immune responses by regulating differentiation and function of monocytic myeloid-derived suppressor cells. Eur J Immunol (2013) 43(5):1220–30. doi: 10.1002/eji.201242841
262. Fitzsimmons CM, Falcone FH, Dunne DW. Helminth allergens, parasite-specific igE, and its protective role in human immunity. Front Immunol (2014) 5:61. doi: 10.3389/fimmu.2014.00061
263. Christ A, Latz E. The Western lifestyle has lasting effects on metaflammation. Nat Rev Immunol (2019) 19(5):267–8. doi: 10.1038/s41577-019-0156-1
Keywords: parasitic diseases, vaccine, trained immunity, innate immune memory, metabolic and epigenetic programming
Citation: Zhu J, Liu J, Yan C, Wang D and Pan W (2023) Trained immunity: a cutting edge approach for designing novel vaccines against parasitic diseases? Front. Immunol. 14:1252554. doi: 10.3389/fimmu.2023.1252554
Received: 04 July 2023; Accepted: 25 September 2023;
Published: 06 October 2023.
Edited by:
Tiago WP Mineo, Federal University of Uberlandia, BrazilReviewed by:
Lucienne Tritten, Swiss Tropical and Public Health Institute (Swiss TPH), SwitzerlandSuchandan Sikder, James Cook University, Australia
Copyright © 2023 Zhu, Liu, Yan, Wang and Pan. This is an open-access article distributed under the terms of the Creative Commons Attribution License (CC BY). The use, distribution or reproduction in other forums is permitted, provided the original author(s) and the copyright owner(s) are credited and that the original publication in this journal is cited, in accordance with accepted academic practice. No use, distribution or reproduction is permitted which does not comply with these terms.
*Correspondence: Wei Pan, panwei525@126.com; Dahui Wang, Leiningzi123@yeah.net