- 1University of Lille, CNRS, Inserm, CHU Lille, Institut Pasteur Lille, U1019-UMR 9017-CIIL-Center for Infection and Immunity of Lille, Lille, France
- 2Univ. Lille, CNRS, Inserm, CHU Lille, Institut Pasteur de Lille, UMR9020-U1277-CANTHER-Cancer Heterogeneity Plasticity and Resistance to Therapies, Lille, France
Bordetella pertussis is a highly contagious respiratory pathogen responsible for whooping-cough or pertussis. Despite high vaccination coverage worldwide, this gram-negative bacterium continues to spread among the population. B. pertussis is transmitted by aerosol droplets from an infected individual to a new host and will colonize its upper respiratory tract. Alveolar macrophages (AMs) are effector cells of the innate immune system that phagocytose B. pertussis and secrete both pro-inflammatory and antimicrobial mediators in the lungs. However, understanding their role in B. pertussis pathogenesis at the molecular level is hampered by the limited number of primary AMs that can be collected in vivo. In order to decipher the regulation of innate response induced by B. pertussis infection, we used for the first time self-renewing, non-transformed cells, called Max Planck Institute (MPI) cells, which are phenotypically and functionally very close to pulmonary AMs. Using optimized infection conditions, we characterized the entry and the clearance of B. pertussis within MPI macrophages. We showed that under these conditions, MPI cells exhibit a pro-inflammatory phenotype with the production of TNF, IL-1β, IL-6 and MIP-2α, similarly to primary AMs purified from broncho-alveolar fluids of mice. In addition, we explored the yet uncharacterized role of the signal transduction activator of transcription (STAT) proteins family in the innate immune response to B. pertussis infection and showed for the first time the parallel regulation of pro-inflammatory cytokines by STAT3 and STAT5 in MPI macrophages infected by B. pertussis. Altogether, this work highlights the interest of using MPI cells for experiments optimization and preliminary data acquisition to understand B. pertussis interaction with AMs, and thus significantly reduce the number of animals to be sacrificed.
Introduction
Whooping cough or pertussis is a highly transmissible respiratory disease, responsible for more than 19 million cases worldwide and 117,000 deaths in children under 5 years old reported in 2019 (1). Despite being a vaccine-preventable disease, whooping cough still represents a major health problem due to an increasing incidence which has been observed since the early 2010s (2). The inability of current acellular pertussis vaccines to prevent nasal infection and transmission and to induce long-lasting protection are the main reasons for the resurgence of pertussis (3). Whooping cough is caused by the human-restricted pathogen Bordetella pertussis, a gram-negative bacterium transmitted by aerosol droplets (4). Once inhaled from an infected person, B. pertussis will express adhesins and toxins which will promote the colonization of the respiratory tract of the newly infected host and will induce damages in the first line of defense which is the airway epithelium. Resident and recruited innate immune cells such as alveolar macrophages and dendritic cells will be among the first immune cells to sense and to respond to B. pertussis infection in the lungs (5).
Alveolar macrophages (AMs) are the major macrophage population in the lungs (6). Their role during B. pertussis pathogenesis is twofold. These professional phagocytic cells play an important role in controlling infection, since AM depletion in mice promotes B. pertussis infection (7). This mechanism involves a Myd88-Adaptator-Like protein (MAL) dependent-pathway and the early induction of pro-inflammatory cytokines and chemokines (8). As well as contributing to protective immunity, AMs may also provide a niche for prolonged B. pertussis survival. Indeed, B. pertussis have been found inside AMs of infected infants and children with severe pertussis (9, 10). Similarly, intracellular survival was observed in mouse models (7, 11) as well as in vitro using human and mouse macrophages (8, 12).
Macrophages are a heterogeneous cell population which differ phenotypically and functionally depending on their origin and environment (13). AMs derived from embryonic progenitor and their development and self-renewal capacity depends on granulocyte colony-stimulating factor (GM-CSF) produced by type II alveolar epithelial cells (14, 15). Nevertheless, most of the current knowledge on macrophage responses to B. pertussis infection were extrapolated from data obtained using cells derived from human mononuclear cells (16), monocytic cell lines such as the THP1 (17, 18), or bone-marrow derived macrophages (8). Indeed, studying interactions between pathogens and alveolar macrophages is hampered by the limited number of AMs which can be obtained from naïve mice. In the present study, we used the recently described Max Planck Institute (MPI) cells to study the alveolar macrophage response to B. pertussis infection. MPI cells are non-transformed, GM-CSF-dependent murine macrophage cell line established from fetal liver cells which present phenotypical and immunological similarities with primary murine alveolar macrophages, such as the CD11c expression, low levels of CD14 and the pattern of pro-inflammatory cytokines production that differs from those of bone-marrow derived macrophages (19). Those cells have been shown to be a valuable model to study the host-pathogen interaction, such as with P. aeruginosa (20), M. tuberculosis (21), and more recently with S. pneumoniae (22). Here we set up an optimized protocol for infection of MPI cells by B. pertussis and characterized the process of bacterial internalization and clearance. We compared the production of pro-inflammatory cytokines and chemokines by infected MPI cells and primary AMs isolated from broncho-alveolar lavage fluids of mice. Using the valuable cellular model that are MPI cells, we explored the mechanism regulating the expression of pro-inflammatory cytokines by B. pertussis and the involvement of the family of STAT (signal transduction activator of transcription) proteins. Our results showed that the combined inhibition of STAT3 and STAT5 is associated with a high level of pro-inflammatory cytokine production by MPI macrophages in response to B. pertussis infection.
Materials and methods
Bordetella pertussis strain and growth conditions
Gentamicin-resistant derivative of B. pertussis B1917 bacteria (23, 24) were grown for 36h at 37°C on Bordet-Gengou (BG) agar (Difco) supplemented with 1% glycerol, 10% of Sheep Defibrinated Blood and 10 µg/ml gentamicin (Sigma-Aldrich). The bacteria were then scrapped off the plates and suspended in RPMI medium at the desired optical density.
MPI model
Max Plank Institute (MPI) macrophages are self-renewing, non-transformed, GM-CSF dependent murine cells, immunologically and phenotypically similar to murine alveolar macrophages (19). MPI were routinely cultivated in non-treated T-75 flasks (Sarstedt) at 37°C, 5% CO2 in RPMI 1640-Glutamax medium (Gibco), supplemented with 10% Fœtal Bovine Serum (Eurobio) and 30 ng/ml GM-CSF (Miltenyi). All experiments were performed using MPI cells from less than 40 passages.
For infection experiment, adherent cells were gently detached with Versene (Gibco) for 5 minutes at 4°C, recovered by centrifugation. and then seeded in 6 or 96-well plates in RPMI1640-Glutamax medium + 10% FBS, complemented or not with 15 ng/ml GM-CSF.
Isolation of primary murine alveolar macrophages
8-week old C57BL/6 mice were purchased from Charles River, Laboratories, France. All mice were maintained at the Institut Pasteur de Lille BSL-2 animal facility. Animal experiments were carried out according to the guidelines of the French Ministry of Research on animal experiments and with institutional regulations and ethical guidelines (B59-350009, Institut Pasteur de Lille, France). The protocols were approved by the Ethical Committees of the Region Nord-Pas-de-Calais and the Ministry of Research (agreement number APAFIS # 201603311654342_v2). To recover broncho-alveolar lavage fluids (BALFs), mice were euthanized by intraperitoneal injection of pentobarbital (Euthasol, TVM Lab). A canule was inserted in the trachea and BALFs were recovered by lavages with 800 µl of a pre-warmed solution of PBS (Gibco), 2% FBS and 2 mM EDTA and centrifuged at 300g, at 4°C for 5 min. Erythrocytes were lysed using ACK Lysing Buffer (Gibco). Cell pellets were resuspended in RPMI-1640-Glutamax, 10% FBS, 1 mM Pyruvate (Gibco) and GM-CSF (15 ng/ml) and were seeded in 96-well tissue-culture treated plates (CytoOne) at a final concentration of 2 x 105 cells/well. Purity of alveolar macrophages was assessed by Facs analysis and was shown to be higher than 90% (Figure S1).
Macrophages infection with B. pertussis
Macrophages were seeded in 6 or 96-well plates (CytoOne) at a concentration of 2 x 105 cells/well, with or without GM-CSF (15 ng/ml) and incubated at 37°C under 5% CO2. After 24h of incubation, the macrophages were infected with B. pertussis for 30 min, 1h or 2h at a multiplicity of infection (MOI) of 10, 50 or 100 bacteria/cell. The end of the contact time corresponds to T0. Non-adherent B. pertussis were removed by two successive washes with PBS and remaining extracellular bacteria were killed by 1h treatment with 100µg/ml polymyxin B (Euromedex) diluted in the culture medium. Cellular activity was assessed at different time points after infection using the MTT assay (Cell Kit Proliferation I, Roche) and measurement of the Optical Density (OD) on a plate reader at λ = 570nM. The results are expressed as the ratio of the (ODstimulated cells/ODunstimulated cells) *100. To evaluate the intracellular bacterial loads, macrophages were lysed at indicated times with 0,1% saponin (Sigma) and serial dilutions of the lysates were plated on BG agar medium. After 5 days of incubation at 37°C, the Colony Forming Units (CFU) were counted on the plates. Intracellular survival is calculated with the ratio of (CFUTime of interest/CFUT0)*100. In the experiments aiming to assess the impact of phagocytosis, MPI cells were pre-treated with 10µM of Cytochalasin D (Sigma-Aldrich) 30 min prior to B. pertussis infection. After 1h of infection, two washes were performed with PBS containing 100µg/ml polymyxin B prior to cells lysis.
Transmission electron microscopy
After 2h and 4h of contact with B. pertussis, MPI cells were fixed with 1% glutaraldehyde in 0.1 M sodium cacodylate pH 6.8 buffer, at 4°C overnight. Infected macrophages were post-fixed with 1% osmium tetroxide and 1.5% potassium ferricyanide then with 1% uranyl acetate, both in distilled water at room temperature in the dark, for 1h. After washing, samples were dehydrated with increasing ethanol-concentration solutions. Samples were finally infiltrated with epoxy resin and cured at 60°C for 24h. Sections of 70-80 nm thickness deposited on formvar-coated grids were observed at 80 kV with a Hitachi H7500 TEM (Milexia), and images were acquired with a 1 Mpixel digital camera from AMT (Milexia).
Immunoblot
MPI cells were lysed in 0.1% Triton X-100 (Sigma), 5mM EDTA, 50 mM NaCl, 20 mM Tris-HCl pH 7,4 and 1% of HALT protease/phosphatase inhibitor (Thermofisher) and the suspension was clarified by centrifugation. Protein concentration was determined using Pierce BCA Protein Assay Kit (Thermoscientific). Samples were boiled at 95°C for 10 min in Laemmli buffer containing 1M of dithiothreitol and proteins were separated on 4-15% Mini-protean TGX Gel (Bio-rad). Proteins were transferred to nitrocellulose membranes (Thermoscientific) which were blocked in PBS-Tween 0,1% containing 5% of non-fat milk for 1h and incubated overnight at 4°C with the following primary antibodies from Cell Signaling Technology diluted in the blocking solution: anti-β-actin (clone 8H10D10, dilution 1:1000), anti-pSTAT3 Tyr705 (clone 3E2, dilution 1:1000), anti-pSTAT3 Ser727 (ref 9134, dilution 1:1000), anti-pSTAT5 (clone C71E5 dilution 1:1000) anti-STAT3 (clone 124H6, dilution 1:1000) and anti-STAT5 (clone D2O6Y, dilution 1:1000). The membrane was then incubated with the appropriate HRP-conjugated secondary antibodies for 1h30 at room temperature. Revelation was done using SuperSignal West Femto Maximum Sensitive Substrate kit (Thermofisher) and images were acquired on Amersham Imager 600. Band intensity was quantified using ImageJ software and normalized to loading control β-actin. Relative expression for the condition of interest was then normalized to the one obtained for GM-CSF non-infected cells, which give the fold-protein expression.
Flow cytometry
To assess the rate of macrophages infected with B. pertussis, 109 bacteria were resuspended in 1 ml PBS containing 5 mM EDTA and incubated with 15µg/ml carboxyfluorescein diacetate succinimidyl ester (CFSE) (BD Horizon) at 37°C under agitation for 20 min. The CFSE fluorescence intensity was measured by Fluorescence-activated cell sorting (FACS) on Attune NxT (Thermofischer Scientific) to quantify the percentage of MPI infected cell. The bacteria were then diluted to the desired concentration prior to the infection.
For MPI cells phenotyping, cells were washed in PBS containing 3% FBS and 1 mg/ml sodium azide (Sigma). Cells were then incubated with Fc Block (BD Pharmingen, dilution 1:200), followed by staining with anti-SiglecF APC (Biolegend, clone S1700L dilution 1:400), anti-CD11c BV711 (Biolegend, clone N418 dilution 1:400), anti-CD11b BV605 (BD Horizon, clone M1/70 dilution:1:400), anti-CD16/CD32 FITC (BD Pharmingen, clone 2.4 G2 dilution 1:200), anti-Ly6G PE-CF594 (BD Horizon, clone 1A8 dilution 1:400) and anti-F4/80 PE (eBioscience, clone BM8 dilution 1:300). Cells were washed and fixed in Foxp3/Transcription Factor Staining Buffer Set (eBioscience). FACS samples were acquired on an Attune NxT using the Attune NxT Software (ThermoFisher scientific) and analyzed using the FlowJo v10 Software.
For cell cycle analysis, macrophages were harvested and fixed overnight in 70% ethanol at -20 °C. Cells were then washed in PBS and stained with 10 μg/mL propidium iodide (Sigma) containing RNase A (200 μg/mL; ThermoScientific) at 37 °C for 15 min in the dark. Cellular DNA content was measured from at least 10000 events per sample by LSR Fortessa (Beckman Coulter) and cell cycle distribution was analyzed with FlowJo v10 software with the Dean-Jett-Fox univariate model.
Cytokine analysis
To determine cytokine secretion level, cell culture supernatants were collected 24h after infection and analysed by enzyme-linked immunosorbent assay (ELISA) and Luminex technology according to the manufacturer’s instructions. The following cytokines were assessed by ELISA: TNF (Mouse TNF ELISA Set II, BD OptEIA), IL-6 (Mouse IL-6 ELISA Set, BD OptEIA), IL-1β (Mouse IL-1β Uncoated ELISA Kit, Invitrogen) and MIP-2α (DuoSet Mouse CXCL2/MIP-2, R&D Systems). When indicated, the production of cytokines was measured by Luminex technology using the following Milliplex Mouse magnetic bead panel (Merck, Millipore): TNF, IL-6, IL-1β, MIP-2α, IL12p40, IL-5, IL-10 and IL-22.
To measure the level of gene expression, total RNA was extracted 6h after B. pertussis infection and purified using the NucleoSpin kit (Macherey-Nagel). RNA quantification and RIN number were determined respectively with Nanodrop 2000c (Thermofisher) and the Bioanalyzer 2100 (Agilent). The RNAs were selected with a RIN number > 8. Reverse Transcription was then performed on 300 ng of purified RNA with the Verso cDNA Synthesis kit (Thermo Scientific). The obtained cDNA was mixed with the No ROX SYBR 2X MasterMix blue dTTP (Eurogentec-Takyo) and with specific primers for the genes of interest (GOI) (Supplementary Table 1) obtained from Eurogentec. qPCR was then realized with a LightCycler480. The Ct of all GOIs were substracted to the Ct of the macrophage housekeeping gene, Syntaxin-5 gene (stx5a) (Tanaka et al, 2017). The 2-ΔΔCt formula was then applied and considered as the fold-gene expression relative to the control condition.
Statistical analysis
Multiple independent groups were analyzed using first a Kruskal-Wallis test then a Conover post-hoc test. The Mann-Whitney U test was used to compare differences between two independent groups. If the p-value was 0.05 or less, the results were considered statistically significant. All the graphs, calculations and statistical analyses were performed by using GraphPad Prism software 141 version 9.3.1 and R software version 4.1.1.
Results
Optimization of B. pertussis infection in the MPI macrophage model
We first assessed MPI cell response to B. pertussis contact using as readout the secretion of TNF, a pro-inflammatory cytokine produced by B. pertussis-infected alveolar macrophages (8). MPI cells were cultured for 30 min with B1917, a virulent strain representative of current circulating B. pertussis bacteria (23). We compared increasing doses of B1917 corresponding to 10, 50 and 100 bacteria/cell. We showed that a multiplicity of infection (MOI) of 50 is required to induce significant secretion of TNF by MPI macrophages, and that the level of secreted TNF is increased at a MOI of 100 (Figure 1A). In parallel, we measured the impact of B. pertussis on MPI activity. Using the MTT assay, we observed a MOI-dependent increased cellular metabolic activity, indicating that B1917 stimulates MPI proliferation (Figure 1B). No significant difference was detected between a MOI of 50 and 100 suggesting that the maximum cellular activity level was already reached with the MOI of 50.
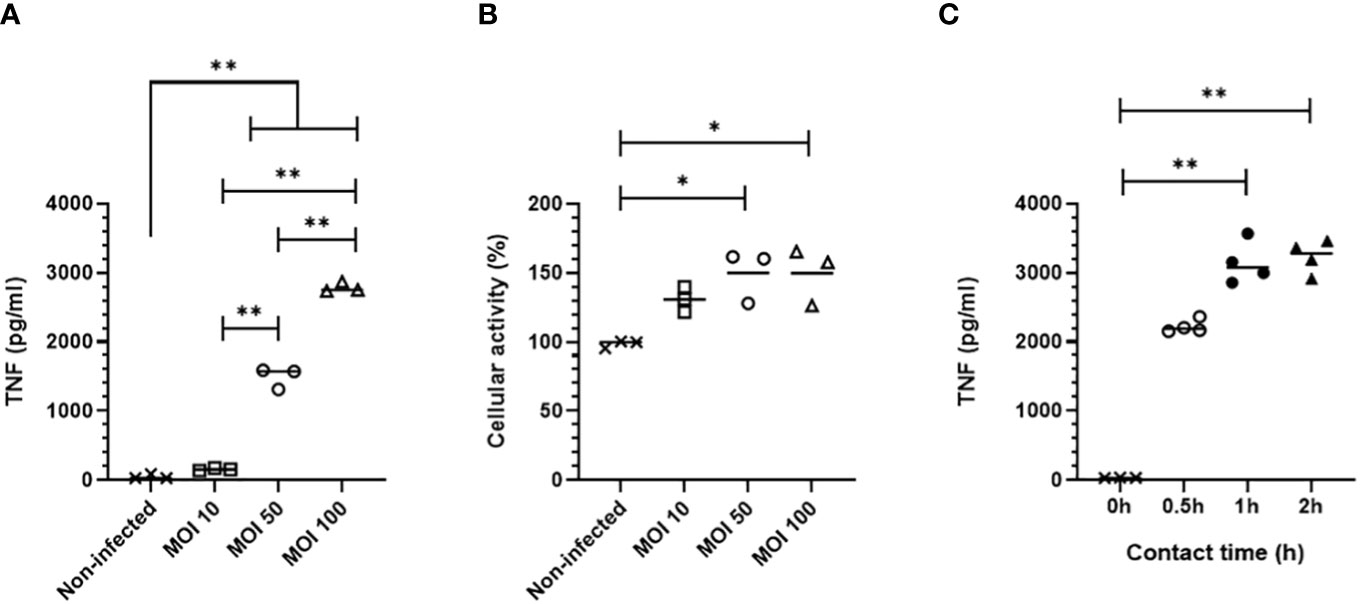
Figure 1 TNF production by MPI cells infected with B. pertussis. (A) TNF production 24h after infection measured by ELISA from cell culture supernatant of MPI cells incubated for 0,5h with different MOI of B. pertussis. (B) MPI cell activity assessed using MTT assay, 24h after infection of MPI cells with B. pertussis for 0,5h. (C) TNF production 24h post-infection measured by ELISA from cell culture supernatant of MPI cells incubated with B. pertussis for 0.5, 1 or 2h with a MOI of 50. Lines represent the median of triplicates or quadruplicates. Statistical test used: Kruskal-Wallis followed by a Conover’s test for multiple comparisons. Data are representatives of two independent experiments. *: p-value <0,05, **: p-value <0,01.
B. pertussis expresses several adhesins which will interact with receptors at the surface of macrophages to allow bacterial adherence (25–28). We determined the optimal contact time between B. pertussis and MPI macrophages to induce a significant TNF response. MPI cells were in contact with B1917 at MOI 50 for 30 min, 1h, or 2h before killing extracellular bacteria with polymyxin B. One day after infection, significant secretion of TNF was measured in cell culture supernatants in all conditions with a peak response induced after 1h of bacterium-cell contact (Figure 1C). MPI activity was not affected even after the longest contact time duration with B. pertussis (data not shown).
Taken together, these data indicate that the optimized protocol of infection of MPI macrophages with B. pertussis strain B1917 requires a contact time of 1h at a MOI of 50. This optimized protocol is used in all the following experiments described in this article.
Internalization and clearance of B. pertussis by MPI macrophages
Using transmission electron microscopy, we observed that interaction of B. pertussis with MPI induces cellular pseudopods extension which surround bacteria, leading to internalization of B. pertussis inside macrophages (Figure 2A). We measured the internalization efficiency of B. pertussis by MPI using the optimal conditions determined previously. Quantitative assessment by FACS analysis showed that in these conditions, more than 90% of MPI were infected with B. pertussis (Figure 2B).
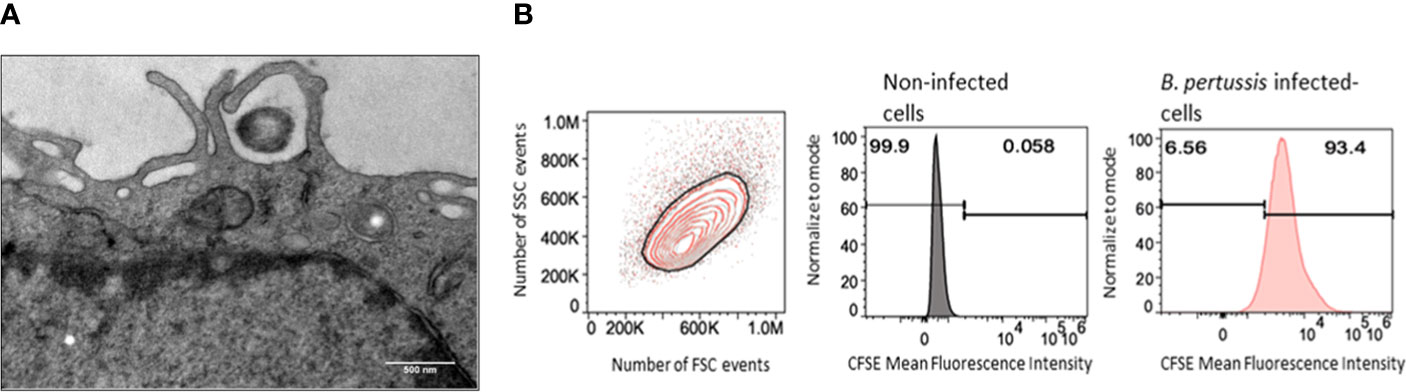
Figure 2 Internalization of B. pertussis by the MPI cells. (A) Electron micrograph of a cross-sectionned MPI cell incubated for 2h with B. pertussis at a MOI of 50. B. pertussis is entrapped by cytoplasmic protrusions synthesized by the cell. Scale bar, 500 nm. (B) Representative Flow cytometry chart of MPI cells infected for 1h with CFSE-stained-B. pertussis at a MOI of 50.
To assess the impact of B. pertussis internalization on our readout which is TNF secretion, we treated MPI with cytochalasin D, an inhibitor of actin polymerization and phagocytosis, prior to infection. In these experimental conditions, a non-significant but reproducible reduction in bacterial internalization rate of approximately 40% was observed in MPI treated with cytochalasin D leading to a significant decrease in TNF secretion after infection (Figures 3A, B). MPI metabolic activity was not affected by cytochalasin D treatment (data not shown). These data therefore suggest that internalization of B. pertussis increases the innate immune responses of infected MPI macrophages compared to stimulation by adherent bacteria alone.
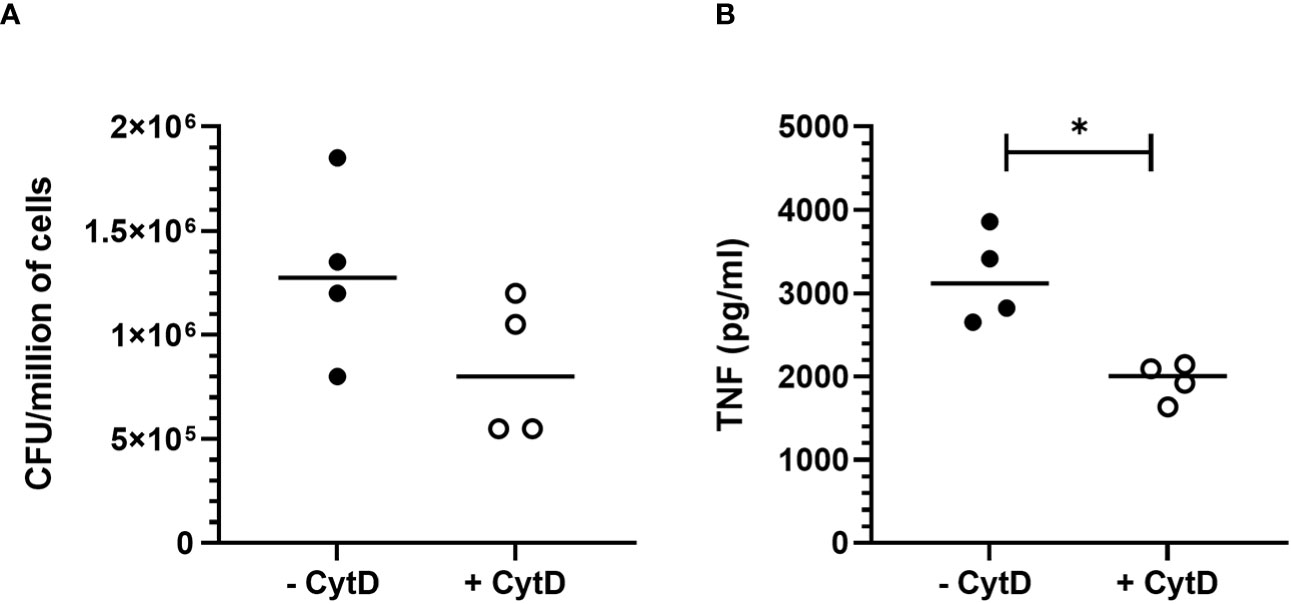
Figure 3 Impact of B. pertussis internalization on TNF response by MPI cells. (A) Number of colony-forming unit (CFU) internalized by MPI cells pre-treated or not with cytochalasin D (CytD) and incubated with B. pertussis for 1h at a MOI of 50. (B) TNF production 24h after B. pertussis infection measured by ELISA from cell culture supernatant of MPI cells pre-treated or not with Cyt D and incubated with B. pertussis for 1h with a MOI of 50. Lines represent the median of quadruplicates. Statistical test used: Mann-Whitney. Data are representatives of 2 experiments. *: p-value < 0,05.
We observed by transmission electron microscopy that following internalization, B. pertussis-containing phagosome fuses with lysosome to form a phagolysosome (Figure 4A). We monitored B. pertussis intracellular survival inside MPI cells and showed that B. pertussis is rapidly cleared by MPI macrophages, with less than 10% live bacteria recovered 24 hours after phagocytosis (Figure 4B). Only few live bacteria were recovered 96 hours after infection (<10 CFU/million of MPI cells).
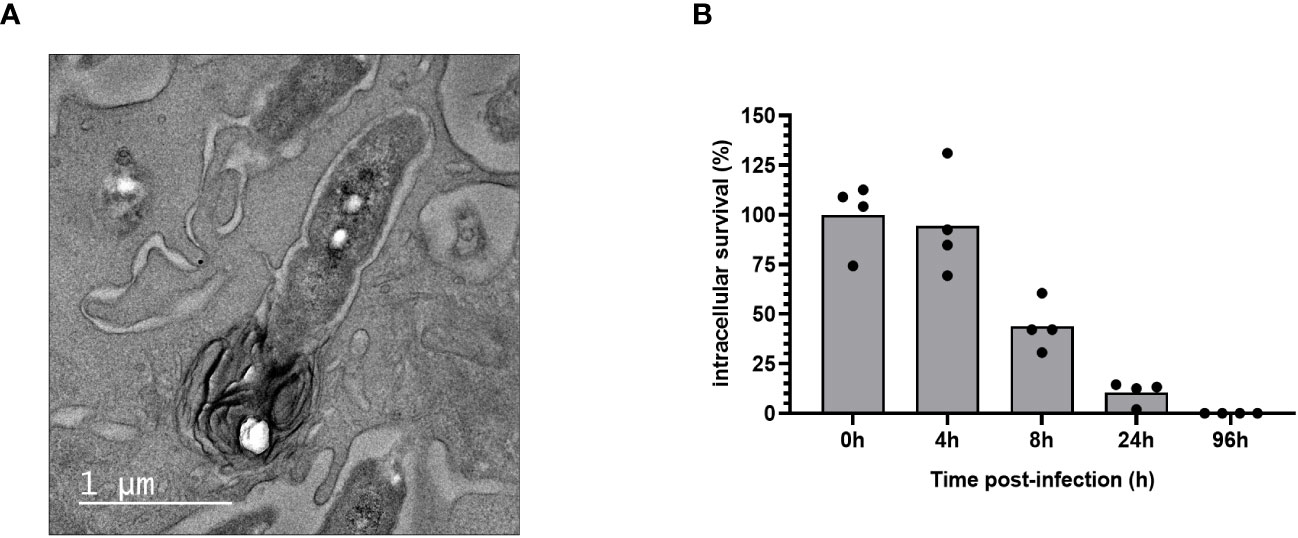
Figure 4 Intracellular clearance of B. pertussis by MPI cells. (A) Electron micrograph of a cross-sectioned MPI cells after 4h of incubation with B. pertussis at a MOI of 50, showing the fusion of a B. pertussis-containing phagosome with a lysosome. Scale bar, 500 nm. (B) Kinetics of intracellular survival of B. pertussis inside MPI cells after 1h of contact between cells and bacteria at a MOI of 50. The end of contact time corresponds to T0. Colony Forming Units of B. pertussis from infected-macrophages lysates were counted after 5 days of culture on BG agar plates. The percentage of intracellular survival was calculated with the ratio of (CFUTime of interest/CFUT0)*100. The bars represent the mean of quadruplicates. Data are representatives of 3 independent experiments.
MPI cells unlike primary alveolar macrophages express high level of pro-inflammatory cytokines but no IL-22 in response to B. pertussis infection
We compared after infection with B. pertussis¸ the levels of cytokines produced by MPI cells and by primary alveolar macrophages (AMs) purified from broncho-alveolar lavages of C57BL/6 mice. TNF secretion level was relatively higher in primary AMs infected with B. pertussis compared to infected-MPI macrophages even though the difference was not significant (Figure 5A). In contrast, MPI cells secreted significantly higher amount of the pro-inflammatory cytokines IL-1β and IL-6 as well as the chemokine MIP-2α (macrophage inflammatory protein 2α) involved in the recruitment of immune cells, than primary AMs in response to B. pertussis infection (Figures 5B–D). However, and in contrast to primary AMs (29), infected-MPI cells did not secrete IL-22 (Figure 5E). No secretion of IL12p40, IL-5 nor IL-10 was detected after B. pertussis infection of MPI or primary AMs (data not shown).
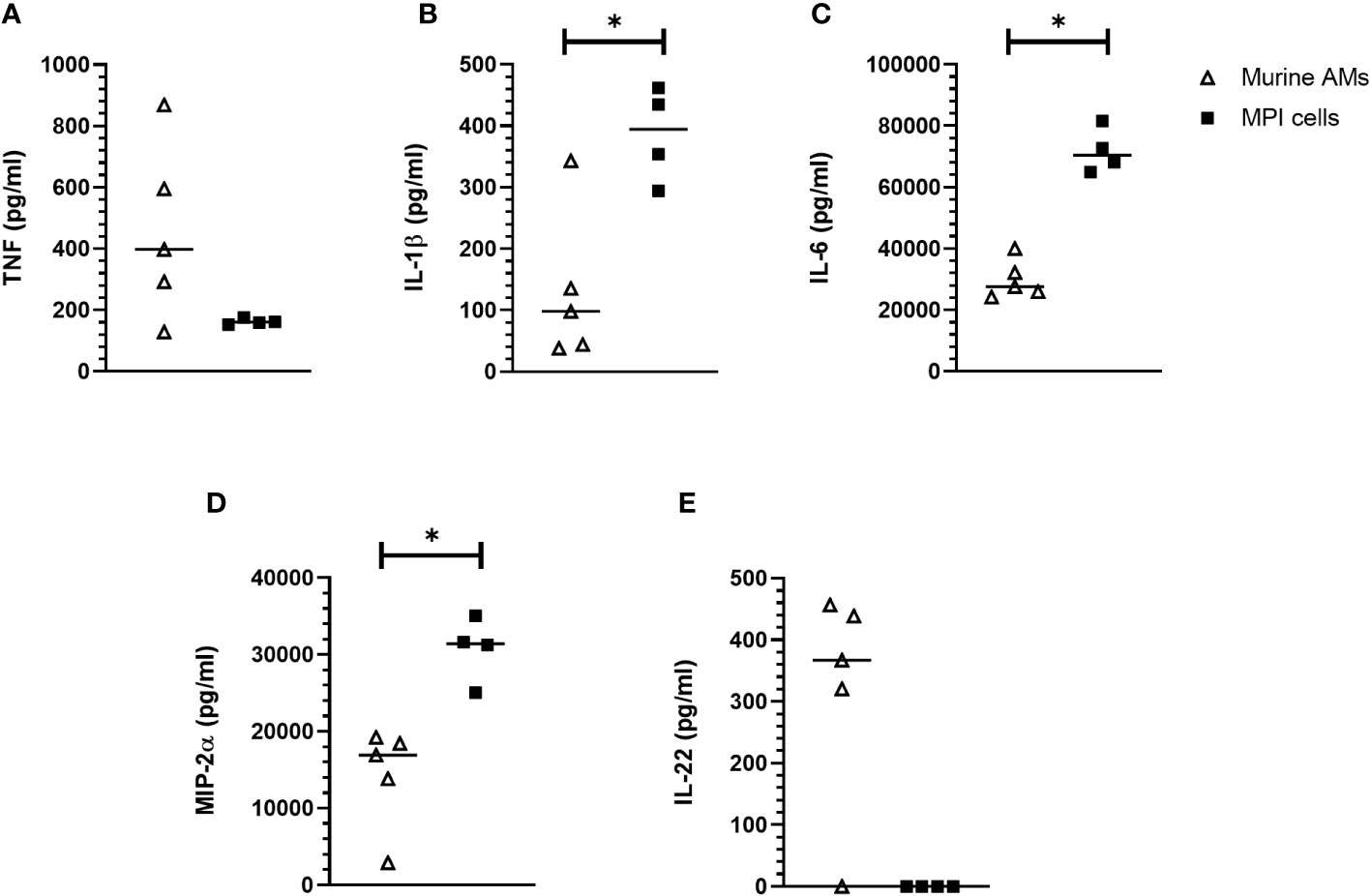
Figure 5 Cytokine responses of MPI cells and primary murine alveolar macrophages infected with B. pertussis. MPI cells and AM were incubated for 1h with B. pertussis at a MOI of 50. 24h post-infection, production of TNF (A), IL-1β (B), IL-6 (C), MIP-2α (D) and IL-22 (E) was measured by the Luminex technology. Lines represent the median of five biological replicates and the graph is representative of 2 experiments. Statistical test used: Mann-Whitney. *: p-value < 0,05.
Pro-inflammatory cytokines are produced by B. pertussis-infected MPI macrophages in the absence of GM-CSF-induced STAT5 activation
Regulation of signal transduction activator of transcription (STAT) protein family is critical for the induction of pro- or anti-inflammatory responses (30). Among the STAT family, STAT5 was shown to be activated by GM-CSF and to be essential for MPI pro-inflammatory phenotype (19). We therefore assessed the role of STAT5 in the induction of pro-inflammatory cytokine secretion in response to B. pertussis infection. Unexpectedly, we found that STAT5 activation, measured by its phosphorylation level, in MPI macrophages is downregulated after B. pertussis infection (Figures 6A, B). In order to confirm that the production of pro-inflammatory cytokines is independent of GM-CSF-induced STAT5 activation, we cultured MPI cells in medium depleted of GM-CSF before B. pertussis infection. As expected, removal of GM-CSF from MPI cells medium resulted in the absence of activation of STAT-5 in uninfected and B. pertussis-infected MPI lysates (Figures 6A, B). GM-CSF starvation being known to induce G0/G1 cell cycle arrest which could impact cellular properties and lead to comparison biases, we ensured that B. pertussis infection was able to induce cell cycle progression despite the removal of GM-CSF from culture medium. Facs analysis of MPI cells cultured in GM-CSF-depleted or complete medium showed that B. pertussis infection induced re-entry into the cell cycle as highlighted by an increased number of MPI-infected cells in G2/M phases in both conditions 24h after infection (Table 1; Figure S2). Moreover, B. pertussis killing inside infected-MPI cells cultured with GM-CSF was significantly less efficient than in MPI macrophages grown in a medium without GM-CSF (Figure 7). Cellular activity of infected-MPI cells was not affected by the presence or not of GM-CSF (Figure S3). Finally, MPI cell phenotype analysed by FACS after 24 hours of GM-CSF starvation showed lower level of CD11c and CD32 compared to MPI cells cultured in medium with GM-CSF while no difference was observed for the other markers studied (SiglecF, CD11b, F4/80, Ly6G) (Figure S4). The levels of expression and secretion of cytokines were then measured in B. pertussis-infected MPI cells cultured with or without GM-CSF. We showed that in response to B. pertussis, higher transcript levels of TNF, IL-1β, IL-6 and MIP-2α were detected 6 hours after infection when MPI were cultured without GM-CSF and therefore when STAT5 is inactivated (Figure 8A). In addition, 24h after B. pertussis infection, significantly higher levels of TNF, IL-1β, IL-6 and MIP-2α were secreted in supernatants of MPI cells cultured without GM-CSF (Figure 8B).
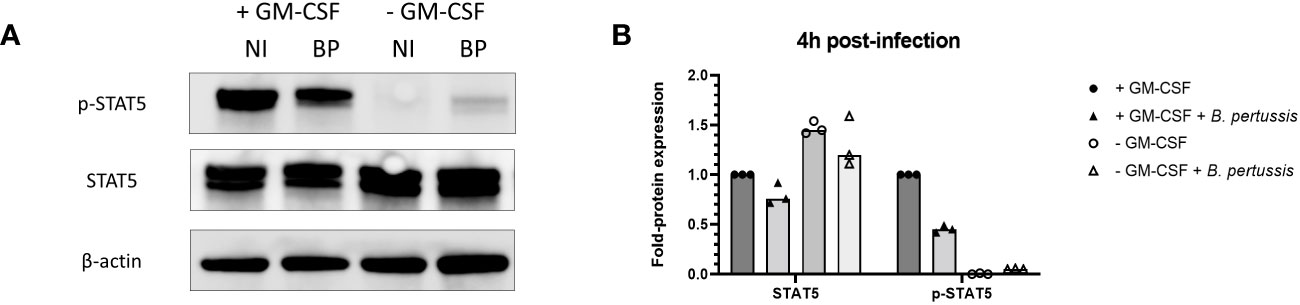
Figure 6 Inhibition of STAT5 phosphorylation in MPI cells cultured without GM-CSF and infected or not with B. pertussis. (A) Phospho-STAT5 (p-STAT5), STAT5 and β-actin levels detected by immunoblot 4h post-infection in lysates of MPI cells incubated for 1h with B. pertussis at a MOI of 50. The end of contact time corresponds to T0. Non-infected cells were added as control. NI: Non-infected cells, BP: B. pertussis infected-cells. (B) Fold-protein expression of STAT5 and p-STAT5 in B. pertussis-infected MPI cells cultured in the presence or not of GM-CSF. Bars represent the median of 3 independent experiments.
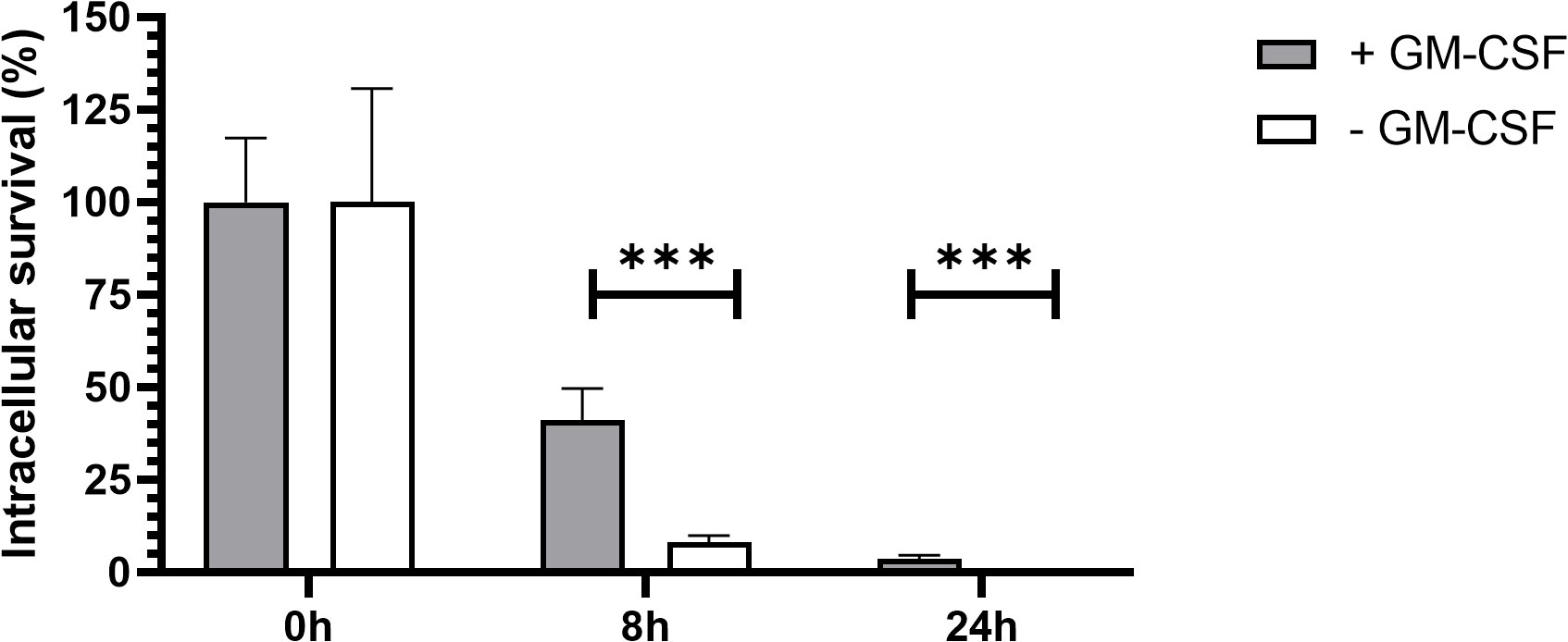
Figure 7 B. pertussis clearance in MPI cells with and without GM-CSF. Kinetics of intracellular survival of B. pertussis inside MPI cells. MPI cells were incubated for 1h with B. pertussis at a MOI of 50. The end of contact time corresponds to T0. Bars represent the mean of quadruplicates with the standard deviation (SD). Data are representatives of 3 independent experiments. ***: p-value <0,001.
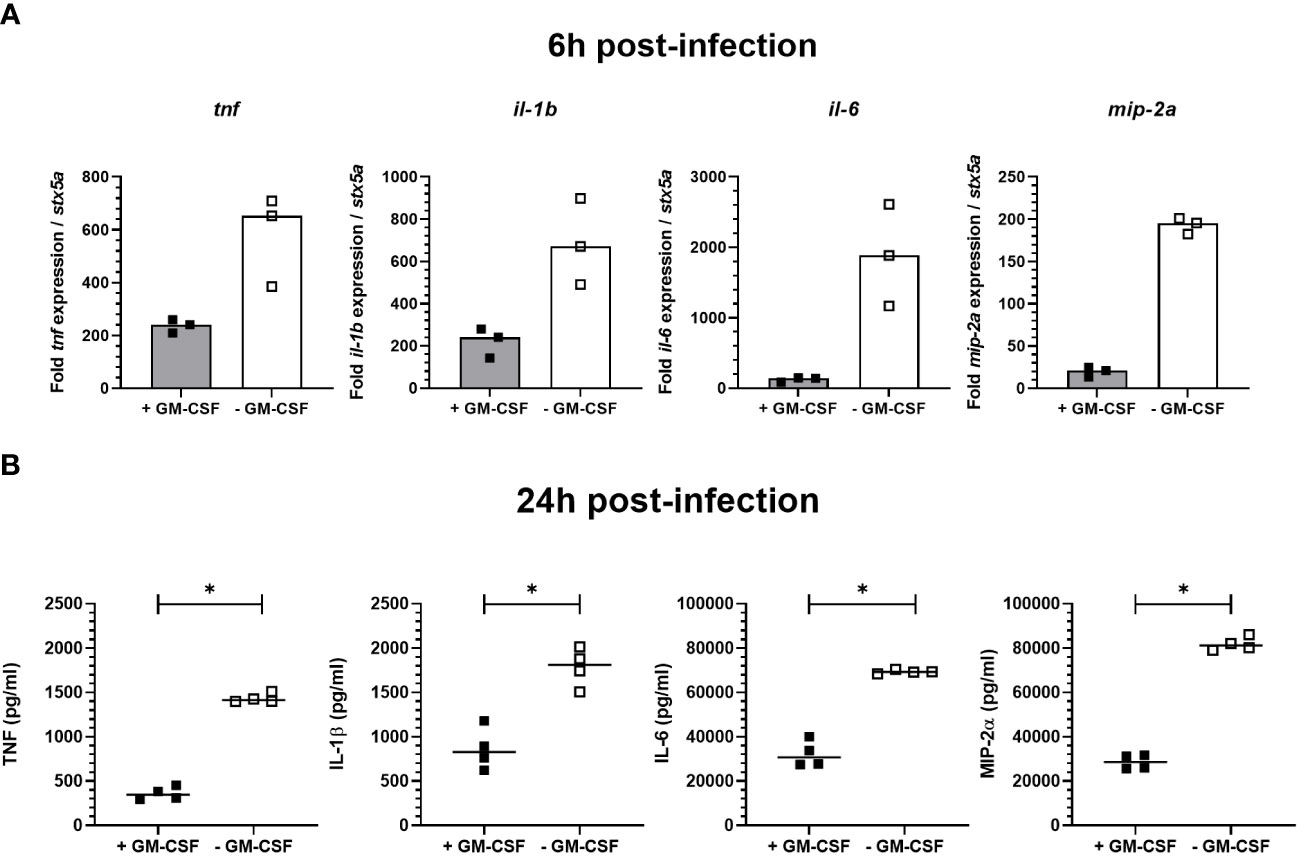
Figure 8 Cytokine expression and secretion by B. pertussis-infected MPI cells. MPI cells were incubated for 1h with B. pertussis at a MOI of 50. The end of contact time corresponds to T0. (A) Expression of tnf, il-1b, il-6 and mip-2a transcripts in MPI cells cultured in medium containing or not GM-CSF, 6h post-infection. Bars represent the median of triplicates (B) Cytokines production measured by ELISA in the supernatants of B. pertussis-infected cells 24h post-infection. Lines represent the median of quadruplicates. Statistical test used: Mann-Whitney. Data are representatives of 3 independent experiments. *: p-value < 0,05.
Combined downregulation of STAT5 and Tyr705 STAT3 phosphorylation, but not Ser727 STAT3, by B. pertussis infection is associated with an increased pro-inflammatory cytokine production by MPI macrophages
We hypothesized that the surprisingly higher pro-inflammatory cytokines production observed in the absence of GM-CSF-induced STAT5 activation might be linked to the parallel downregulation of STAT3. Indeed, it was shown in a model of intestinal myeloid cells and upon Salmonella typhimurium exposure that combined STAT3 and STAT5 inhibition resulted in high level of pro-inflammatory cytokine production (31). We therefore analysed STAT3 activation in B. pertussis-infected MPI cells cultured in medium without GM-CSF, conditions where we showed that STAT5 activation was inhibited. We found that similarly to STAT5, activation of STAT3 measured by the phosphorylation of Tyr705 was downregulated compared to B. pertussis-infected MPI cells cultured in complete medium (Figures 9A, C). STAT3 phosphorylation on Tyr705 induces its translocation to the nucleus to regulate transcription, while Ser727 phosphorylated STAT3 leads to mitochondrial metabolic reprogramming (32). Removal of GM-CSF from MPI cells medium resulted in the downregulation of Tyr705 but not Ser727 STAT-3 phosphorylation after B. pertussis infection (Figures 9B, C).
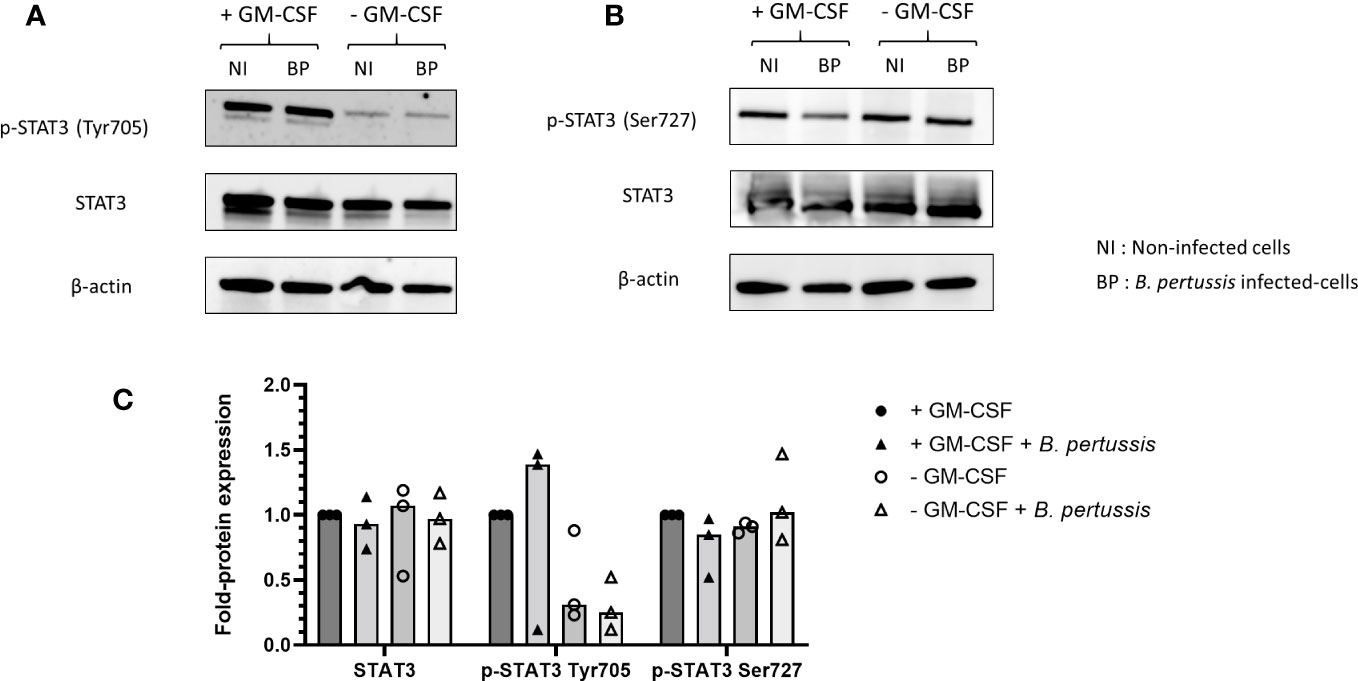
Figure 9 Inhibition of Tyr705 STAT3 phosphorylation, but not Ser727 STAT3, in MPI cells cultured without GM-CSF and infected or not with B. pertussis. MPI cells were incubated for 1h with B.pertussis at a MOI of 50. The end of contact time corresponds to T0. (A, B) Immunoblot of MPI cell lysates infected with B. pertussis 4h post-infection, using specific antibodies to detect (A) p-STAT3 (Tyr705), (B) p-STAT3 (Ser727), (A, B) STAT3 and β-actin. NI, Non-infected cells; BP, B. pertussis infected-cells. (C) Fold-protein expression of p-STAT3 (Tyr705), p-STAT3 (Ser727), STAT3. Results represent the median of 3 independent experiments.
Discussion
Alveolar macrophages are among the first innate cells to encounter B. pertussis when the bacteria reach the lungs. Their dual role as a potential reservoir of B. pertussis and as a key player in induction of inflammatory and immune responses make them a particularly attractive type of cells to study B. pertussis pathogenesis. Our current understanding of the molecular mechanisms induced in AMs in response to B. pertussis infection is nevertheless limited due to the constraints in collecting primary AMs which are present in low numbers in the lungs of naïve individuals. Moreover, in the context of 3Rs rules, the use of an alternative cellular model avoiding animals sacrifice is particularly recommended. In this paper, we describe the first data obtained with MPI cells, macrophages phenotypically similar to murine alveolar macrophages (19), in the context of B. pertussis infection. We showed in an optimized infection protocol that MPI cells can phagocytose and kill intracellular B. pertussis. We observed by transmission electron microscopy that phagosomes containing B. pertussis fused with lysosomes, which is a major driver of bacterial killing (33). This is consistent with the decrease in the number of viable intracellular bacteria in MPI cells within 24 hours of infection and is in agreement with a previous study showing colocalization of B. pertussis with late endosomal/lysosomal marker LAMP-1 and with Lysotracker, an acidotropic dye, indicating a lysosomal fusion in macrophages derived from blood mononuclear cells (34). In contrast to this study, we haven’t detected multiplication of B. pertussis inside MPI macrophages and only very few live bacteria were recovered from infected MPI after 3 days of infection. Macrophages represent a very heterogenous and specialized group of cells (35) and the difference observed between the two studies could be related to the distinct features of AMs compared to monocyte- or bone marrow-derived macrophages (36, 37). Interestingly, a recent study suggested that B. pertussis may survive in a dormant, non-culturable state, for a longer time inside macrophages (38). This observation opens a new axis that will have to be further explored in order to understand the intracellular fate of B. pertussis inside alveolar macrophages, which have been described as potential infectious reservoir, in particular because of their long lifespan (39).
Another characteristic of alveolar macrophages is that they play a central role in the orchestration of the innate immune response during respiratory infection. In response to B. pertussis infection, both MPI macrophages and primary AMs purified from broncho-alveolar lavages of mice exhibit pro-inflammatory phenotypes with the secretion of TNF, IL-1β and IL-6. In addition, we showed that both MPI and primary AMs expressed MIP-2α, also called CXCL2 (40), involved in the recruitment of neutrophils which play a key role in the clearance of B. pertussis (41, 42). IL-22 has also been shown to play an important role in mucosal response to gram-negative pathogens, as well as reducing lung injury by promoting mucosal barrier integrity (43). In addition, IL-22 was shown to synergize with IL-17, a cytokine which plays a key role in protective immunity against B. pertussis infection (44), to enhance antimicrobial peptide production and neutrophil recruitment (45). We showed here that primary AMs produce IL-22 in response to B. pertussis infection and may therefore contribute to the host defense response against B. pertussis. MPI cells were previously shown to be unable to produce IL-10 in response to various stimuli (19). Similarly, we did not detect IL-10 production in response to B. pertussis infection of MPI macrophages nor IL-22 which belongs to the same family of cytokines as IL-10 (46).
Regulation of cytokine production is a complex pathway, which has been surprisingly poorly studied during B. pertussis pathogenesis. In this work, we explored for the first time the role of the signal transduction activator of transcription (STAT) protein family in the innate immune response to B. pertussis infection. STAT proteins are part of the Janus kinase-signal transducer and activator of transcription (JAK-STAT) pathway, which is an evolutionary-conserved signalling pathway essential for the regulation of the innate immune response (47). Briefly, the JAKs are activated by binding of extracellular ligands, such as cytokines or growth factors, to transmembrane receptors. Activated JAKs then phosphorylate STAT proteins, which are translocated to the cell nucleus where they will bind to DNA and activate transcription of specific genes (47). Anti-inflammatory cytokines including IL-10 and IL-22 can activate STAT3 and STAT5 in an autocrine/paracrine manner and suppress pro-inflammatory cytokines in response to microbial stimulation in monocytes-derived macrophages (31). Reduced levels of pro-inflammatory cytokines produced by B. pertussis-infected primary AMs compared to infected MPI cells might thus be linked to the defect of IL-10 and IL-22 production by MPI macrophages. In addition, among the 7 members of the STAT family, STAT5 phosphorylation is dependent upon GM-CSF stimulation of cells and JAK2 activation (48). GM-CSF is critical for lung alveolar macrophage differentiation, and its presence in the culture medium is required for the proliferation of MPI cells (19, 49). Surprisingly and contrary to a previous study using various stimuli (19), we showed here that in the absence of GM-CSF in the culture medium, high levels of pro-inflammatory cytokines were induced by B. pertussis infection of MPI cells suggesting that the induction of pro-inflammatory cytokines by B. pertussis is independent of STAT5 activation. In neutrophils, GM-CSF was shown to induce the specific phosphorylation of STAT5 but also STAT3 (50).
Interestingly, STAT3 has been shown to cooperate with STAT5 to regulate the balance between pro- and anti-inflammatory cytokines response and particularly the parallel downregulation of STAT3 and STAT5 resulted in high level of pro-inflammatory cytokine production (31). Similarly, we showed that STAT5 and also STAT3 phosphorylation on Tyr705 was inhibited in MPI cells cultured in medium depleted in GM-CSF and infected with B. pertussis and that this combined inhibition was associated with an increase in production of pro-inflammatory cytokines.
GM-CSF specifically leads to tyrosine phosphorylation of JAK2 ( (50, 51) which activates, in addition to STAT5 and STAT3, other major signaling pathways such as PI3K/Akt and Ras/Raf-MAPK/ERK (52). The role of these signalling pathways in modulating the response of alveolar macrophages to B. pertussis infection needs to be further explored. In particular, the study of the PI3K/Akt/mTor pathway, involved in cell proliferation, macrophage polarization and in autophagy regulation (53, 54), could provide relevant data to decipher the intracellular survival mechanisms of B. pertussis inside alveolar macrophages.
In summary, our study described for the first time the use of a valuable model, called MPI cells, to study the interaction between B. pertussis and alveolar macrophages, sentinel cells of the lungs playing a key role in innate immune defense against infection. Future research using in vitro 2D or 3D platforms to mimic pulmonary environment will be necessary to further evaluate the role of the cross-talk between AMs and lung epithelial cells (55) in the induction of innate immunity in response to B. pertussis infections.
Using this in vitro model, we explored for the first time the role of two proteins from the STAT family in the regulation of pro-inflammatory cytokine production induced by B. pertussis infection of alveolar macrophages. Our study showed that induction of pro-inflammatory cytokines by B. pertussis-infected MPI cells was linked to the combined inhibition of STAT5 and STAT3 activation in the model of MPI alveolar macrophages. While further studies need to be done, this work paves the way toward a better understanding of the role of AMs in innate immunity and B. pertussis pathogenesis using MPI as a model to optimize experiments and obtain preliminary data while reducing the number of animals to be sacrificed.
Data availability statement
The raw data supporting the conclusions of this article will be made available by the authors, without undue reservation.
Ethics statement
The animal study was approved by Animal experiments were carried out according to the guidelines of the French Ministry of Research on animal experiments and with institutional regulations and ethical guidelines (B59-350009, Institut Pasteur de Lille, France). The protocols were approved by the Ethical Committees of the Region Nord-Pas-de-Calais and the Ministry of Research (agreement number APAFIS # 201603311654342_v2). The study was conducted in accordance with the local legislation and institutional requirements.
Author contributions
FK: Conceptualization, Data curation, Formal Analysis, Methodology, Validation, Writing – original draft, Writing – review & editing. ZK: Conceptualization, Data curation, Formal Analysis, Investigation, Methodology, Validation, Writing – review & editing. VD: Data curation, Formal Analysis, Investigation, Methodology, Validation, Writing – review & editing. SS: Data curation, Formal Analysis, Investigation, Methodology, Writing – review & editing. EP: Investigation, Methodology, Writing – review & editing. A-SD: Investigation, Methodology, Validation, Writing – review & editing. SC: Data curation, Methodology, Validation, Writing – review & editing. NB: Investigation, Writing – review & editing. CR: Conceptualization, Data curation, Formal Analysis, Investigation, Methodology, Supervision, Validation, Writing – review & editing. NM: Conceptualization, Data curation, Formal Analysis, Funding acquisition, Methodology, Supervision, Validation, Writing – original draft, Writing – review & editing.
Funding
This work was supported by the Center for Infection and Immunity of Lille (call for projects 2021). FK received PhD fellowship by Agence Régionale de Santé (ARS) and I-SITE Université Lille Nord-Europe (ULNE).
Acknowledgments
The authors acknowledge Dr. Jean-Claude Sirard at the CIIL for kindly providing the MPI cell line, Sophie Lecher for her help in protein expression analysis and Morgane Tardy for her help in animal experiments. The authors thank the animal facility (PLETHA) of the Institut Pasteur de Lille for animal maintenance and the BICeL platform of the UAR-PLBS for access to Facs equipment.
Conflict of interest
The authors declare that the research was conducted in the absence of any commercial or financial relationships that could be construed as a potential conflict of interest.
Publisher’s note
All claims expressed in this article are solely those of the authors and do not necessarily represent those of their affiliated organizations, or those of the publisher, the editors and the reviewers. Any product that may be evaluated in this article, or claim that may be made by its manufacturer, is not guaranteed or endorsed by the publisher.
Supplementary material
The Supplementary Material for this article can be found online at: https://www.frontiersin.org/articles/10.3389/fimmu.2023.1254276/full#supplementary-material
Supplementary Figure 1 | Representative flow cytometry plots showing gating for BAL cells from 8-week old naive mice. Alveolar macrophages were designated as SiglecF + CD11b-.
Supplementary Figure 2 | Characterisation of MPI cell cycle in FACS after GM-CSF starvation. Cell cycle analysis of MPI cells cultivated in the presence (A, B) of GM-CSF or after 24h starvation (C, D) by FACS. A and C show three-dimensional flow cytometry of propidium iodide stained cells. B and D show the relative proportion of cells in each phase of the cycle and was calculated from A and C with the Dean-Jett-Fox model using FlowJo software. Experiment was repeated at least three times, and the data shown here are representative of the results obtained.
Supplementary Figure 3 | MPI cell activity cultured with or without GM-CSF and infected with B. pertussis. MPI cell activity assessed using MTT assay, 24h after infection of MPI cells with B. pertussis at a MOI of 50 for 1h. Statistical test used: Kruskal-Wallis followed by a Dunn’s test for multiple comparisons. Lines represent the median of five biological replicates and the graph is representative of 2 experiments. ***: p-value <0,001.
Supplementary Figure 4 | Characterisation of MPI cell phenotype by FACS after GM-CSF starvation. Representative flow cytometry charts of SiglecF, CD11b, CD11c, CD32, F4/80, Ly6G expression by MPI cells cultured in presence of GM-CSF or after 24h of GM-CSF starvation. Data shown here are representative of the results obtained for 10 replicates.
Supplementary Table 1 | Primers for the genes of interest analyzed by qRT-PCR. SP: Sense primer; ASP: Anti-Sense primer.
References
1. Abbafati C, Abbas KM, Abbasi-Kangevari M, Abd-Allah F, Abdelalim A, Abdollahi M, et al. Global burden of 369 diseases and injuries in 204 countries and territories, 1990–2019: a systematic analysis for the Global Burden of Disease Study 2019. Lancet (2020) 396(10258):1204–22. doi: 10.1016/S0140-6736(20)30925-9
2. Tan T, Dalby T, Forsyth K, Halperin SA, Heininger U, Hozbor D, et al. Pertussis across the globe. Pediatr Infect Dis J [Internet] (2015) 34(9):e222–32. doi: 10.1097/INF.0000000000000795
3. Diavatopoulos DA, Mills KHG, Kester KE, Kampmann B, Silerova M, Heininger U, et al. PERISCOPE: road towards effective control of pertussis. Lancet Infect Dis [Internet] (2019) 19(5):e179–86. doi: 10.1016/S1473-3099(18)30646-7
4. Belcher T, Dubois V, Rivera-Millot A, Locht C, Jacob-Dubuisson F. Pathogenicity and virulence of Bordetella pertussis and its adaptation to its strictly human host. Virulence [Internet] (2021) 12(1):2608–32. doi: 10.1080/21505594.2021.1980987
5. Higgs R, Higgins SC, Ross PJ, Mills KHG. Immunity to the respiratory pathogen Bordetella pertussis. Mucosal Immunol [Internet] (2012) 5(5):485–500. doi: 10.1038/mi.2012.54
6. Aegerter H, Lambrecht BN, Jakubzick CV. Biology of lung macrophages in health and disease. Immun [Internet] (2022) 55(9):1564–80. doi: 10.1016/j.immuni.2022.08.010
7. Carbonetti NH, Artamonova GV, Van Rooijen N, Ayala VI. Pertussis toxin targets airway macrophages to promote Bordetella pertussis infection of the respiratory tract. Infect Immun (2007) 75(4):1713–20. doi: 10.1128/IAI.01578-06
8. Bernard NJ, Finlay CM, Tannahill GM, Cassidy JP, O’Neill LA, Mills KHG. A critical role for the TLR signaling adapter Mal in alveolar macrophage-mediated protection against Bordetella pertussis. Mucosal Immunol (2015) 8(5):982–92. doi: 10.1038/mi.2014.125
9. Paddock CD, Sanden GN, Cherry JD, Gal AA, Langston C, Tatti KM, et al. Pathology and pathogenesis of fatal bordetella pertussis infection in infants. Clin Infect Dis [Internet] (2008) 47(3):328–38. doi: 10.1086/589753
10. Bromberg K, Tannis G, Steiner P. Detection of Bordetella pertussis associated with the alveolar macrophages of children with human immunodeficiency virus infection. Infect Immun [Internet] (1991) 59(12):4715–9. doi: 10.1128/iai.59.12.4715-4719.1991
11. Hellwig SMM, Hazenbos WLW, Van de Winkel JGJ, Mooi FR. Evidence for an intracellular niche for Bordetella pertussis in broncho- alveolar lavage cells of mice. FEMS Immunol Med Microbiol (1999) 26(3–4):203–7. doi: 10.1111/j.1574-695X.1999.tb01391.x
12. Friedman RL, Nordensson K, Wilson L, Akporiaye ET, Yocum DE. Uptake and intracellular survival of Bordetella pertussis in human macrophages. Infect Immun (1992) 60(11):4578–85. doi: 10.1128/iai.60.11.4578-4585.1992
13. Hou F, Xiao K, Tang L, Xie L. Diversity of macrophages in lung homeostasis and diseases. Front Immunol (2021) 12:753940. doi: 10.3389/fimmu.2021.753940
14. Sieweke MH, Allen JE. Beyond stem cells: Self-renewal of differentiated macrophages. Science (2013) 342(6161):1242974. doi: 10.1126/science.1242974
15. Guilliams M, De Kleer I, Henri S, Post S, Vanhoutte L, De Prijck S, et al. Alveolar macrophages develop from fetal monocytes that differentiate into long-lived cells in the first week of life via GM-CSF. J Exp Med (2013) 210(10):1977–92. doi: 10.1084/jem.20131199
16. Valdez HA, Marin Franco JL, Gorgojo JP, Alvarez Hayes J, Balboa L, Fernandez Lahore M, et al. Human macrophage polarization shapes B. pertussis intracellular persistence. J Leukoc Biol (2022) 112(1):173–84. doi: 10.1002/JLB.4A0521-254R
17. Farman MR, Petráčková D, Kumar D, Držmíšek J, Saha A, Čurnová I, et al. Avirulent phenotype promotes Bordetella pertussis adaptation to the intramacrophage environment. Emerg Microbes Infect (2023) 12(1):e2146536. doi: 10.1080/22221751.2022.2146536
18. Valdez HA, Oviedo JM, Gorgojo JP, Lamberti Y, Rodriguez ME. Bordetella pertussis modulates human macrophage defense gene expression. Pathog Dis (2016) 74(6):1–14. doi: 10.1093/femspd/ftw073
19. Fejer G, Wegner MD, Györy I, Cohen I, Engelhard P, Voronov E, et al. Nontransformed, GM-CSF–dependent macrophage lines are a unique model to study tissue macrophage functions. Proc Natl Acad Sci [Internet] (2013) 110(24):E2191–8. doi: 10.1073/pnas.1302877110
20. Bastaert F, Kheir S, Saint-Criq V, Villeret B, Dang PMC, El-Benna J, et al. Pseudomonas aeruginosa LasB subverts alveolar macrophage activity by interfering with bacterial killing through downregulation of innate immune defense, reactive oxygen species generation, and complement activation. Front Immunol (2018) 9:1675. doi: 10.3389/fimmu.2018.01675
21. Woo M, Wood C, Kwon D, Park KHP, Fejer G, Delorme V. Mycobacterium tuberculosis infection and innate responses in a new model of lung alveolar macrophages. Front Immunol (2018) 9:438. doi: 10.3389/fimmu.2018.00438
22. MaChado MG, Patente TA, Rouillé Y, Heumel S, Melo EM, Deruyter L, et al. Acetate Improves the Killing of Streptococcus pneumoniae by Alveolar Macrophages via NLRP3 Inflammasome and Glycolysis-HIF-1α Axis. Front Immunol (2022) 13:773261. doi: 10.3389/fimmu.2022.773261
23. Bart MJ, Zeddeman A, van der Heide HGJ, Heuvelman K, van Gent M, Mooi FR. Complete genome sequences of bordetella pertussis isolates B1917 and B1920, representing two predominant global lineages. Genome Announc (2014) 2(6):2–3. doi: 10.1128/genomeA.01301-14
24. Dubois V, Chatagnon J, Thiriard A, Bauderlique-Le Roy H, Debrie AS, Coutte L, et al. Suppression of mucosal Th17 memory responses by acellular pertussis vaccines enhances nasal Bordetella pertussis carriage. NPJ Vaccines [Internet] (2021) 6(1):1–10. doi: 10.1038/s41541-020-00270-8
25. Relman D, Tuomanen E, Falkow S, Golenbock DT, Saukkonen K, Wright SD. Recognition of a bacterial adhesin by an integrin: Macrophage CR3 (αMβ2, CD11b CD18) binds filamentous hemagglutinin of Bordetella pertussis. Cell (1990) 61(7):1375–82. doi: 10.1016/0092-8674(90)90701-f
26. Saukkonen K, Cabellos C, Burroughs M, Prasad S, Tuomanen E. Integrin-mediated localization of Bordetella pertussis within macrophages: Role in pulmonary colonization. J Exp Med (1991) 173(5):1143–9. doi: 10.1084/jem.173.5.1143
27. Ishibashi Y, Relman DA, Nishikawa A. Invasion of human respiratory epithelial cells by Bordetella pertussis: Possible role for a filamentous hemagglutinin Arg-Gly-Asp sequence and α5β1 integrin. Microb Pathog (2001) 30(5):279–88. doi: 10.1006/mpat.2001.0432
28. Locht C, Berlin P, Menozzi FD, Renauld G. The filamentous haemagglutinin, a multifaceted adhesin produced by virulent Bordetella spp. Mol Microbiol (1993) 9(4):653–60. doi: 10.1111/j.1365-2958.1993.tb01725.x
29. Hansson M, Silverpil E, Lindén A, Glader P. Interleukin-22 produced by alveolar macrophages during activation of the innate immune response. Inflamm Res [Internet] (2013) 62(6):561–9. doi: 10.1007/s00011-013-0608-1
30. Platanitis E, Decker T. Regulatory networks involving STATs, IRFs, and NFκB in inflammation. Front Immunol (2018) 9:2542. doi: 10.3389/fimmu.2018.02542
31. Hedl M, Sun R, Huang C, Abraham C. STAT3 and STAT5 signaling thresholds determine distinct regulation for innate receptor–induced inflammatory cytokines, and STAT3 / STAT5 disease variants modulate these outcomes. J Immunol (2019) 203(12):3325–38. doi: 10.4049/jimmunol.1900031
32. Peron M, Dinarello A, Meneghetti G, Martorano L, Betto RM, Facchinello N, et al. Y705 and S727 are required for mitochondrial import and transcriptional activities of STAT3 and regulate proliferation of embryonic and tissue stem cells. Dev (2021) 148(17):1–13. doi: 10.1242/dev.199477
33. Nguyen JA, Yates RM. Better together: current insights into phagosome-lysosome fusion. Front Immunol (2021) 12:636078. doi: 10.3389/fimmu.2021.636078
34. Lamberti YA, Hayes JA, Perez Vidakovics ML, Harvill ET, Rodriguez ME. Intracellular trafficking of Bordetella pertussis in human macrophages. Infect Immun (2010) 78(3):907–13. doi: 10.1128/IAI.01031-09
35. Fejer G, Sharma S, Gyory I. Self-renewing macrophages - A new line of enquiries in mononuclear phagocytes. Immunobiology [Internet] (2015) 220(2):169–74. doi: 10.1016/j.imbio.2014.11.005
36. Jin M, Opalek JM, Marsh CB, Wu HM. Proteome comparison of alveolar macrophages with monocytes reveals distinct protein characteristics. Am J Respir Cell Mol Biol (2004) 31(3):322–9. doi: 10.1165/rcmb.2004-0080OC
37. Hussell T, Bell TJ. Alveolar macrophages: Plasticity in a tissue-specific context. Nat Rev Immunol (2014) 14(2):81–93. doi: 10.1038/nri3600
38. Novák J, Jurnečka D, Linhartová I, Holubová J, Staněk O, Štipl D, et al. A Mutation Upstream of the rplN-rpsD Ribosomal Operon Downregulates Bordetella pertussis Virulence Factor Production without Compromising Bacterial Survival within Human Macrophages. mSystems (2020) 5(6):1–21. doi: 10.1128/mSystems.00612-20
39. Murphy J, Summer R, Wilson AA, Kotton DN, Fine A. The prolonged life-span of alveolar macrophages. Am J Respir Cell Mol Biol (2008) 38(4):380–5. doi: 10.1165/rcmb.2007-0224RC
40. Gupta S, Feng L, Yoshimura T, Redick J, Fu SM, Rose CE. Intra-alveolar macrophage-inflammatory peptide 2 induces rapid neutrophil localization in the lung. Am J Respir Cell Mol Biol (1996) 15(5):656–63. doi: 10.1165/ajrcmb.15.5.8918372
41. Fang S, Ju D, Lin Y, Chen W. The role of interleukin-22 in lung health and its therapeutic potential for COVID-19. Front Immunol (2022) 13:951107. doi: 10.3389/fimmu.2022.951107
42. Andreasen C, Carbonetti NH. Role of neutrophils in response to bordetella pertussis infection in mice. Infect Immun (2009) 77(3):1182–8. doi: 10.1128/IAI.01150-08
43. Aujla SJ, Chan YR, Zheng M, Fei M, Askew DJ, Pociask DA, et al. IL-22 mediates mucosal host defense against Gram-negative bacterial pneumonia. Nat Med (2008) 14(3):275–81. doi: 10.1038/nm1710
44. Borkner L, Curham LM, Wilk MM, Moran B, Mills KHG. IL-17 mediates protective immunity against nasal infection with Bordetella pertussis by mobilizing neutrophils, especially Siglec-F+ neutrophils. Mucosal Immunol [Internet] (2021) 14(5):1183–202. doi: 10.1038/s41385-021-00407-5
45. Mcaleer JP, Kolls JK. Directing traffic: IL-17 and IL-22 coordinate pulmonary immune defense. Immunol Rev (2014) 260(1):129–44. doi: 10.1111/imr.12183
46. Ouyang W, O’Garra A. IL-10 family cytokines IL-10 and IL-22: from basic science to clinical translation. Immun [Internet] (2019) 50(4):871–91. doi: 10.1016/j.immuni.2019.03.020
47. Villarino AV, Kanno Y, Ferdinand JR, O’Shea JJ. Mechanisms of jak/STAT signaling in immunity and disease. J Immunol (2015) 194(1):21–7. doi: 10.4049/jimmunol.1401867
48. Lehtonen A, Matikainen S, Miettinen M, Julkunen I. induced STAT5 activation and target-gene expression during human monocyte / macrophage differentiation. J Leukoc Biol (2002) 71:511–9. doi: 10.1189/jlb.71.3.511
49. Shibata Y, Berclaz PY, Chroneos ZC, Yoshida M, Whitsett JA, Trapnell BC. GM-CSF regulates alveolar macrophage differentiation and innate immunity in the lung through PU.1. Immun (2001) 15(4):557–67. doi: 10.1016/s1074-7613(01)00218-7
50. Al-Shami A, Mahanna W, Naccache PH. Granulocyte-macrophage colony-stimulating factor-activated signaling pathways in human neutrophils. Selective activation of Jak2, Stat3, and Stat5B. J Biol Chem [Internet] (1998) 273(2):1058–63. doi: 10.1074/jbc.273.2.1058
51. Watanabe S, Itoh H, Arai K. Roles of JAK kinases in human GM-CSF receptor signal transduction. J Allergy Clin Immunol (1996) 98(6 PART 2):S183–91. doi: 10.1016/s0091-6749(96)70065-9
52. Levine RL, Pardanani A, Tefferi A, Gilliland DG. Role of JAK2 in the pathogenesis and therapy of myeloproliferative disorders. Nat Rev Cancer (2007) 7(9):673–83. doi: 10.1038/nrc2210
53. Vergadi E, Ieronymaki E, Lyroni K, Vaporidi K, Tsatsanis C. Akt signaling pathway in macrophage activation and M1/M2 polarization. J Immunol (2017) 198(3):1006–14. doi: 10.4049/jimmunol.1601515
54. Kma L, Baruah TJ. The interplay of ROS and the PI3K/Akt pathway in autophagy regulation. Biotechnol Appl Biochem (2022) 69(1):248–64. doi: 10.1002/bab.2104
Keywords: Bordetella pertussis, alveolar macrophages, infection, cytokines, STAT proteins
Citation: Khiter F, Kherrouche Z, Dubois V, Slupek S, Petit E, Debrie A-S, Cauchi S, Barois N, Rouanet C and Mielcarek N (2023) Combined regulation of pro-inflammatory cytokines production by STAT3 and STAT5 in a model of B. pertussis infection of alveolar macrophages. Front. Immunol. 14:1254276. doi: 10.3389/fimmu.2023.1254276
Received: 06 July 2023; Accepted: 14 September 2023;
Published: 28 September 2023.
Edited by:
Buka Samten, The University of Texas Health Science Center at Tyler, United StatesReviewed by:
Nicholas Carbonetti, University of Maryland, United StatesBernhard Kerscher, Paul-Ehrlich-Institut (PEI), Germany
Copyright © 2023 Khiter, Kherrouche, Dubois, Slupek, Petit, Debrie, Cauchi, Barois, Rouanet and Mielcarek. This is an open-access article distributed under the terms of the Creative Commons Attribution License (CC BY). The use, distribution or reproduction in other forums is permitted, provided the original author(s) and the copyright owner(s) are credited and that the original publication in this journal is cited, in accordance with accepted academic practice. No use, distribution or reproduction is permitted which does not comply with these terms.
*Correspondence: Nathalie Mielcarek, nathalie.mielcarek@inserm.fr
†These authors have contributed equally to this work and share last authorship