- 1Department of Neurosurgery, Tongji Hospital, Tongji Medical College, Huazhong University of Science and Technology, Wuhan, China
- 2Department of Oncology, Tongji Hospital, Tongji Medical College, Huazhong University of Science and Technology, Wuhan, China
- 3Department of Histology and Embryology, School of Basic Medicine, Tongji Medical College, Huazhong University of Science and Technology, Wuhan, China
Gliomas account for the majority of brain malignant tumors. As the most malignant subtype of glioma, glioblastoma (GBM) is barely effectively treated by traditional therapies (surgery combined with radiochemotherapy), resulting in poor prognosis. Meanwhile, due to its “cold tumor” phenotype, GBM fails to respond to multiple immunotherapies. As its capacity to prime T cell response, dendritic cells (DCs) are essential to anti-tumor immunity. In recent years, as a therapeutic method, dendritic cell vaccine (DCV) has been immensely developed. However, there have long been obstacles that limit the use of DCV yet to be tackled. As is shown in the following review, the role of DCs in anti-tumor immunity and the inhibitory effects of tumor microenvironment (TME) on DCs are described, the previous clinical trials of DCV in the treatment of GBM are summarized, and the challenges and possible development directions of DCV are analyzed.
1 Introduction
Diffuse glioma is diagnosed in approximately 100,000 people worldwide each year. Although it accounts for a small proportion (~1%) of all newly diagnosed cancers, diffuse glioma is related to high morbidity and mortality (1). According to the fifth edition of the World Health Organization (WHO) Classification of Tumors of the Central Nervous System, adult diffuse gliomas consist of three types: astrocytoma, IDH mutant (IDHmut); oligodendroglioma, IDHmut and 1p/19q co-deletion; glioblastoma (GBM), IDH wild type (IDHwt) (2). Glioblastoma is the most fatal subtype of glioma, accounting for 70 to 75% of all diffuse gliomas diagnosed, with a median overall survival range from 14 to 17 months (1).
Currently, first-line therapy for GBM typically consists of maximally safe resection followed by adjuvant temozolomide chemotherapy, concurrent fractionated radiotherapy, and maintenance temozolomide chemotherapy (3). This multimodality approach significantly improves survival. However, the prognosis is still quite poor and the relapse of GBM is common, with a median survival of only 6.2 months after relapse. To date, the main treatment options for recurrent GBM, including tumor-treating field (TTF) therapy, lomustine, carmustine, and the antiangiogenic agent bevacizumab (4, 5), are barely effective. Therefore, there is an urgent need to find more effective treatments for GBM.
Dendritic cells (DCs) are a kind of professional antigen-presenting cells (APCs) that are essential for the T cell response. They present extracellular antigens to CD4+ T helper (TH) cells via major histocompatibility complex (MHC) class II molecules, and present intracellular antigens to CD8+ T cells via MHC class I molecules. This so-called “cross-presentation” phenomenon, takes an important part in antitumor immune responses (6). DC vaccine is a kind of immunotherapy based on the effect of DC. The blueprint is that patients are administrated with DCs activated by tumor-associated antigens (TAAs), inducing an antitumor T cell response. This response eliminates tumor cells selectively and prevents tumor relapse because of immunologic memory (7, 8) (Figure 1).
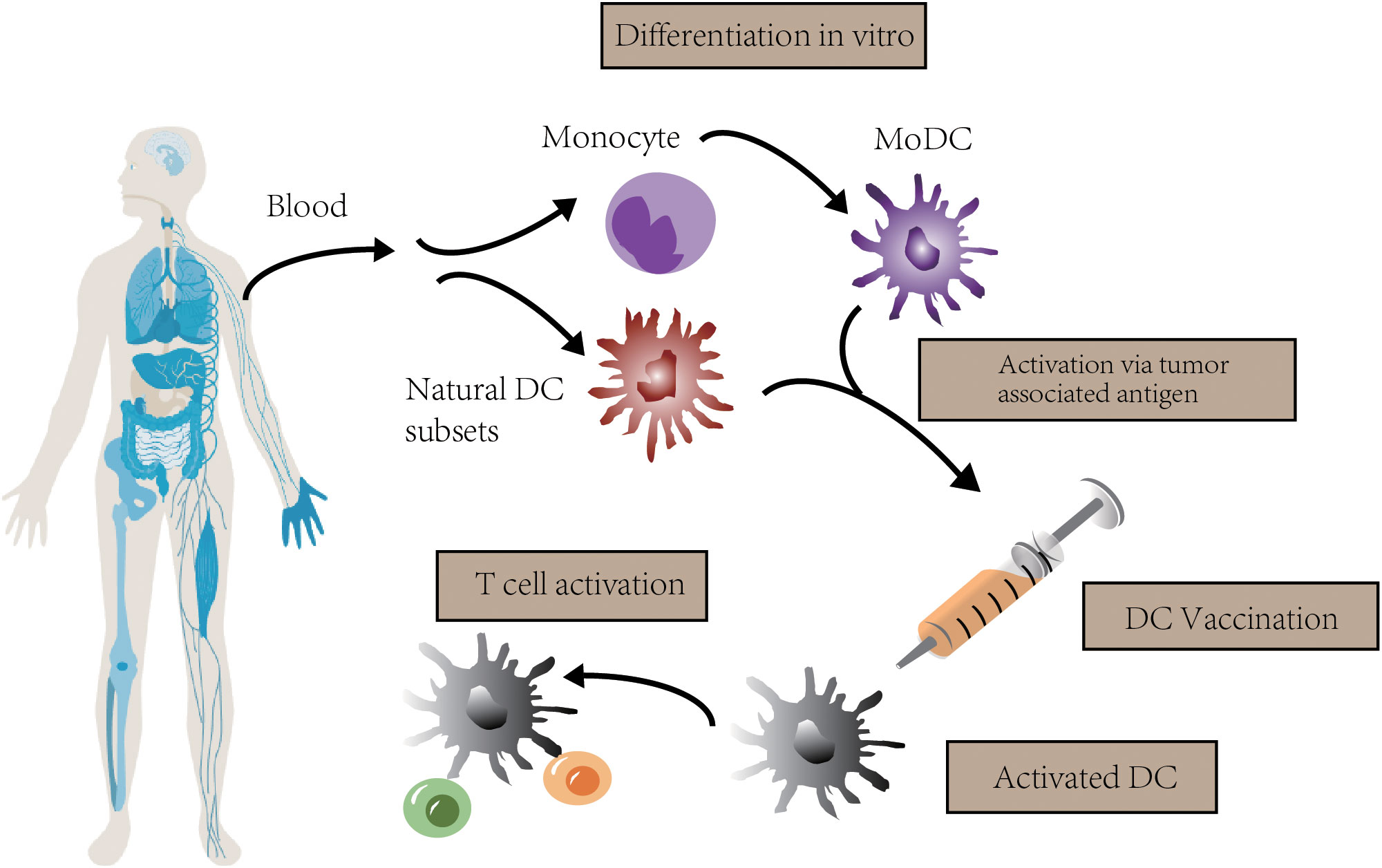
Figure 1 The concept of DC vaccination. In clinical trials, autologous monocytes are commonly used as the source of DC vaccines with differentiation and maturation in vitro. After loaded with tumor associated antigen, DC vaccines are infused to activate T cell response.
In recent decades, many advances have been achieved in the use of DC vaccines (DCV) in anti-tumor therapy. Since DCV made its debut in a B cell lymphoma clinical trial in 1996 (9), a large number of preclinical and clinical trials have been conducted using DCV for various tumors (10, 11). However, to date, only one tumor-targeted DCV therapy, sipuleucel-T, has Food and Drug Administration (FDA) approval to treat metastatic castration-resistant prostate cancer (12). The number of clinical trials using DCV has decreased significantly in recent years. This partly results from the rapid development of alternative immunotherapeutic methods, such as immune checkpoint inhibitors (ICIs) (13–15), and partly results from the disappointing clinical performance of DCV.
Nevertheless, due to the unique immune microenvironment of GBM, many immunotherapies that have successfully treated other tumors do not work in GBM (16, 17). As a result, there has been renewed interest in using DCV for treating GBM, particularly when it is combined with conventional therapies (e.g., chemotherapy, radiation) or other immunotherapies (e.g., ICIs) (18–20). Clinical trial results have varied widely, with clinical responses ranging from minimal to significant. Overall, although there are some promising results, conclusive evidence is still lacking. In this review, we analyze the role DC plays in antitumor immunity and the immunosuppressive effect of the tumor microenvironment (TME) on DC, summarize clinical trials that have used DCV for treating GBM in recent years, and propose the challenges and possible development directions of DCV.
2 DCs in anti-tumor immunology
At present, our understanding of DC subsets and functions comes mainly from murine models, while recently the number of studies aimed at assessing the biological properties of human DCs has significantly increased. According to the differences in development, phenotype, and function (See Table 1), DCs can be classified into several subtypes: classical, or conventional DCs (cDCs), plasmacytoid DCs (pDCs), and monocyte-derived DCs (MoDCs), etc (7). cDCs, which consist of two major subsets: cDC1s and cDC2s, develop from common DC precursor cells (CDPs) in the bone marrow (21). In humans, cDC1s can be recognized by highly specific cell surface markers such as CD141, XCR1, CLEC9A, and DEC205 (7, 21–23). cDC2s are more heterogeneous in cell surface markers and can be further classified into CD5+ cDC2s (DC2s) and CD5- cDC2s (DC3s) based on the presence or absence of CD5 expression (22, 24). It remains unknown whether DC3s are derived from CDPs (22). In addition, some studies have suggested the existence of other subsets of cDC2, such as DC4 (CD1C- CD141-) and DC5 (AXL+ SIGLEC6+), whose classification and function require further study (24). Although still controversial, current studies suggest that pDCs arise from both CDPs and lymphoid progenitors and that pDCs from different sources have different functions. During acute or chronic viral infection, mature pDC subsets of both different origins can secrete type I interferon, but only bone marrow-derived pDCs can process and present antigens (25, 26). Furthermore, pDCs are involved in the progression of autoimmune diseases (27), and the high frequency of pDCs in tumors is highly correlated with poor prognosis (28). In contrast to cDCs and pDCs, MoDCs originate in monocytes. Under the context of inflammation, monocytes in the blood are recruited through CC chemokine 2 (CCR2)-dependent pathways and differentiate into MoDCs in peripheral tissues (23). In response to inflammation, MoDCs allow CD4+ T cells’ differentiation into TH1, TH2, or IL-17-producing TH cell (TH17) phenotypes, depending on the context (29). Some investigators have suggested that CD16+ non-classical monocytes are also a type of DC, particularly those expressing carbohydrate-modified P-selectin glycoprotein ligand 1 (slanDCs) (23), which have potent pro-inflammatory properties.
DCs remain immature when pathophysiological stimuli are absent, and are crucial to immune surveillance (30, 31). Immature DCs (iDCs) are indispensable for maintaining tolerance to peripheral autoantigens (32). They can eliminate autoreactive T cells (33), and facilitate the expansion and differentiation of regulatory T cells (Tregs) (34). iDCs mature when they encounter microbial stimuli or endogenous stimuli associated with inflammation (35). Reduced phagocytic activity, increased expression of costimulatory ligands and MHC class I/II molecules on the cell surface, expression of chemokine receptors involved in lymph node homing and retention, and increased secretion of chemokines and proinflammatory cytokines are the main differences between mature DCs (mDCs) and iDCs (10).
cDC1s are of great importance in anti-tumor immunity and are the mere type of APC that effectively primes tumor-specific CD8+ T cells (36). In both murine and humans, cDC1s are crucial for the recruitment of CD8+ T cells to tumors (37). cDC1 is a major producer of IFN-λ, which induces TH1 responses (38, 39). cDC1s can also mediate TH type 1 (TH1) polarization of CD4+ T cells (40). Thus, the abundance of cDC1 in the tumor microenvironment (TME) has a positive correlation with patient survival (37). On the contrary, the understanding of the functions of cDC2s in antitumor responses is relatively new. cDC2s secrete a variety of cytokines, some of which are anti-inflammatory while some of which are pro-inflammatory, including interleukin-12 (IL-12), which is essential for the expansion and survival of T cells and natural killer (NK) cells (41, 42). cDC2s and MoDCs may also have the ability to cross-present antigens, and cDC2s appear to be required for initiating antitumor CD4+ T cell responses (7, 43). In addition, cDC2 and MoDC underlie direct or cross-presentation of TAAs after chemotherapy in some cancers (7, 44, 45). Among all the DC subtypes, pDCs have the strongest type I IFN responses and are the major producers of IFN-α (46). In anti-tumor immunity, type I IFNs are thought to be critical for immunogenic responses to anti-tumor therapies. However, high frequencies of pDCs in tumors are related to poor prognosis in a variety of cancers (47, 48). Persistent IFN-I response may be a key factor in immunodeficiency and treatment resistance, although the mechanism is not yet fully understood (49, 50).
3 The glioma microenvironment and DCs
3.1 The glioma microenvironment
Gliomas, especially GBM, have a unique TME compared to tumors at other sites. The central nervous system (CNS) used to be regarded as an immunologically privileged site. One of the reasons for this understanding is that the lymphatic drainage system of the brain has not been discovered for a long time. Another reason is the existence of the blood-brain barrier (BBB) (51). Recently, the discovery of the glial-lymphatic pathway has proposed a mechanism for connecting the parenchyma and interstitium with the cerebrospinal fluid space (52); meanwhile, the discovery of functional lymphatic vessels in the meninges confirms the existence of a direct drainage pathway for cerebrospinal fluid that contains solutes and immune cells from the brain to the cervical lymph nodes (53, 54). The brain is protected from pathogenic microorganisms by the BBB, consisting of pericytes, astrocyte processes, vascular endothelial cells, and extracellular matrix. Meanwhile, it makes it harder for drugs and peripheral immune cells to enter the CNS, facilitating tumor invasion and growth (55). However, it has been shown in recent studies that T cells can enter the brain and provide immune surveillance (56, 57), challenging the notion that the BBB is sealed to immune cell entry. Simultaneously, damaging the BBB by GBM itself can also limit the BBB’s ability to function (58). In summary, during inflammation, specific antigens are recognized by microglia, then microglia present them to activated lymphoid cells through the glial-lymphoid pathway, and subsequently more immune cells penetrate the BBB, leading to a more intense inflammatory response and following immune responses. Thus, CNS immunity is not so much “privileged” as it is “unique”.
However, compared to other tumor types, CNS tumors have lower levels of tumor-infiltrating lymphocytes (TILs) and other types of immune effector cells (59). This “cold tumor” phenotype has been related to poor response to immunostimulatory therapies such as ICIs (17, 60). Even when inducing T cells to respond to CNS cancer, the number of antigen-specific TILs remains relatively low, and the TILs present often exhibit a depleted phenotype (18, 61). Upon inflammatory stimulation, brain stromal cells produce high levels of classical immunosuppressive cytokines such as TGFβ. These cytokines neutralize inflammatory factors to maintain homeostasis (62). Glioma cells produce high levels of indoleamine-2,3-dioxygenase (IDO), which, besides promoting Treg accumulation, inhibits T-cell activity by depleting microenvironmental tryptophan (63). Microglia and tumor-infiltrating myeloid cells reduce the arginine level in the tissues by producing high levels of arginase, which further suppresses the proliferation and functions of T cells (64). Additionally, the compromised BBB suppresses glioma patients’ adaptive immune response by upregulating programmed death ligand 1/2 (PD-L1/2) expression to prevent effector T-cell from activation (65).
3.2 DCs in the glioma microenvironment
Normally, peripheral circulating DCs reach the vascular-rich compartment through the central lymphatic vessels and are virtually absent in the brain parenchyma (66). However, a recent study found that CD141+ cDC1 can infiltrate the region of GBM and present antigens to T cells in deep cervical lymph nodes (dcLNs) (67). Nonetheless, extracranial antigen presentation failed to facilitate tumor eradication in the absence of immunotherapy in a melanoma brain metastasis model (68). This indicates that the presentation of antigens in the periphery is probably not sufficient to induce immunity against brain tumors.
A major barrier to the application of DCs for the treatment of GBM is that DCs must have the capacity to induce anti-tumor immune responses under immunosuppressive conditions. The mechanism of immunosuppression in GBM involves both the glioma cells themselves and the cells in the TME (Figure 2).
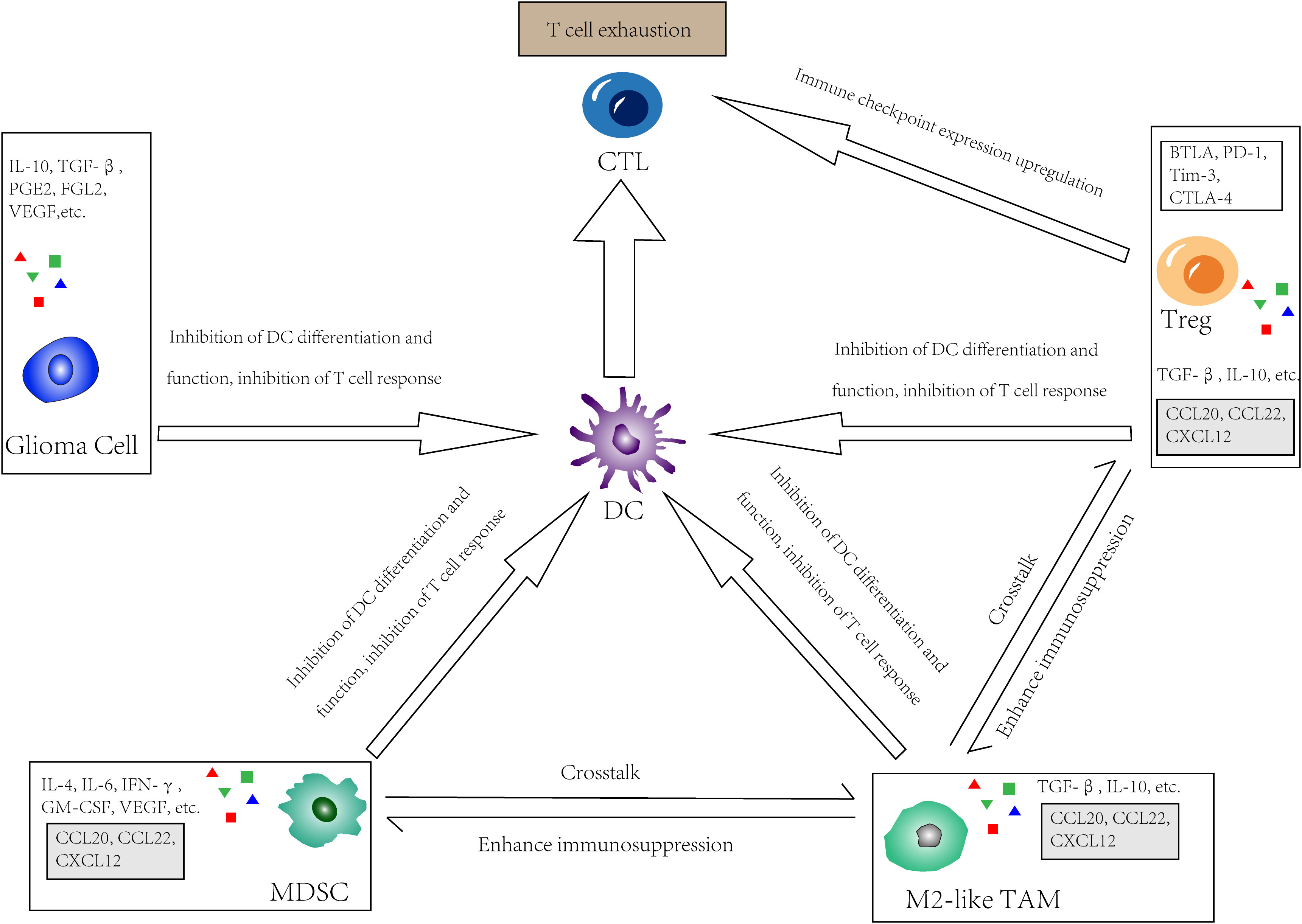
Figure 2 The immunosuppressive glioma microenvironment. In GBM, tumor cells, Tregs, M2-like tumor-associated macrophages (TAMs) as well as myeloid-derived suppressor cells (MDSCs) directly or indirectly inhibit the effect of DC by limit its differentiation and function, or inhibit recruitment, proliferation and function of T cells. Meanwhile, these cells upregulate immune checkpoint expression and interact with receptors on CTLs, thus lead to so called “T cell exhaustion”. There is also a crosstalk in the TME between those cells that secrete chemokines such as CCL20, CCL22, and CXCL12, which further enhancing immunosuppression.
3.2.1 glioma cells
Glioma cells secrete cytokines like TGFβ, IL-10, VEGF, and CSF-1, which inhibit the differentiation of DCs (69). Glioma cell-produced PGE2 can promote DC-producing IL-10, inhibiting effector T-cell responses (70). Tumor cells release IL-6, inhibiting the CD34+ cell differentiation into DCs and promoting the transition of these cells to the monocytic lineage with deficient APC function (71). Some glioma cell products are linked to DC dysfunction, including R-2-hydroxyglutarate (R-2-HG), fibrinogen-like protein 2 (FGL2), Nrf2, etc. In high grade glioma (HGG) patients with IDHmut, reprogramming mediated by the tumor metabolite R-2-HG leads to poor antigen presentation of DCs (66). FGL2 inhibits GM-CSF-induced CD103+ DC differentiation through inhibition of NF-κB, STAT1/5, and p38 activation (72). Glioma cells can induce DCs to overexpress Nrf, which inhibits DC maturation and reduces effector T-cell activation (73). Glioma cells can affect DC lipid metabolism, leading to lipid accumulation in DCs and limiting T-cell activation (74). The Warburg effect of glioma cells can lead to lactic acid accumulation, and low pH affects immune cell metabolism and function (75).
3.2.2 Cells in the glioma microenvironment
TME components such as Tregs, myeloid-derived suppressor cells (MDSCs), and tumor-associated macrophages (TAMs) can suppress antitumor immunity by reducing DC responses and causing T cell dysfunction, which is also known as “T cell exhaustion”.
Treg is an immunosuppressive T cell subset that helps to maintain immune tolerance, limits inordinate immune responses, and promotes homeostasis and tissue regeneration. In various solid tumors, the frequency of tumor-infiltrating effector Tregs is high, and the high proportion of Tregs: CD8+ T cells is inversely correlated with prognosis (76). Tregs are not detectable in normal brains and are seldom found in low-grade brain tumors. Intriguingly, despite lymphopenia, GBM patients have increased Treg frequencies in TME and blood (77). Treg frequencies vary by glioma subtypes, with higher frequencies in IDHwt than in IDHmut (78). In a murine model of astrocytoma, Tregs accumulate time-dependently after tumor cell implantation. The quantity of Tregs first increases in blood and then in tumor tissue during the asymptomatic phase (79). It can be seen that Tregs are recruited to tumors at an early stage when the number of tumor cells is still low. CD27(TNFRSF) expressed by Tregs can downregulate the expression of CD70 on the membrane of DCs, thereby limiting the activation of CD8+ T cells (80). The immune checkpoints BTLA, PD-1, Tim-3, and CTLA-4 expressed on Tregs also limit functions of DCs (65).
MDSCs are a population of immature bone marrow cells that are of high heterogeneity. In the TME, with their strong immunosuppressive activity, they continuously interact with infiltrating T cells, especially cytotoxic T lymphocyte (CTLs), inhibiting their function and thus promoting the growth and progression of tumors (81). MDSCs can be detected in patients with cancer or the setting of chronic inflammation when sustained low-level stimulation of bone marrow cell generation leads to the development of immunosuppressive bone marrow cells (82, 83). First develop in the bone marrow, they then infiltrate and accumulate in solid tumors via factors such as GM-CSF, G-CSF, M-CSF, VEGF, IFN-γ, IL-6, and IL-4, which are secreted by tumor cells or other TME components. In GBM, MDSC is one of the major immunosuppressive components of the TME (84). Recent studies have shown that MDSCs are present in GBM patients’ blood, but not in patients with low-grade gliomas or healthy people (85). MDSCs can prevent CTL entry into tumors and T cell responses to HLA stimulation through ROS- and NO-dependent pathways (86). Like Tregs, MDSCs produce immunosuppressive cytokines such as IL-10 and TGFβ. In addition, MDSCs express immune checkpoint regulatory pathway ligands, such as PD-L1/2 and CD155. These ligands inhibit T-cell responses. When interacting with receptors on T cells, they can even induce T cell apoptosis (87, 88).
TAMs, representing 50% of the total number of living cells in the entire GBM tumor, are the largest immune cell population in the TME of GBM (89). TAMs are a highly heterogeneous cell population, and overall, in both murine and human models, the majority of TAMs in brain tumors seem to originate in circulating monocytes, while approximately 15% of TAMs originate in brain-resident microglia (51, 90). In GBM, however, this heterogeneity depends on the context (e.g., Microglia are relatively abundant in primary GBM, whereas monocyte-derived macrophages predominate in recurrent GBM.) (89). In general, TAMs are thought to promote tumor growth, and the number of TAMs is correlated with tumor grade (91). As is shown in previous in vitro studies, macrophages can be classified into two groups: M1 and M2, and the growth-promoting activity of TAM correlates with the M2 macrophage phenotype that is anti-inflammatory. However, TAMs are neither M1-like nor M2-like but exhibit a mixed phenotype (91). In the TME, the majority of TAMs were M2-like cells. Yet, there are also proinflammatory TAMs capable of engulfing tumor cells (92). Immunosuppressive cytokines, such as TGFβ and IL-10, which are expressed by M2-like TAMs, suppress T cell proliferation and function. Meanwhile, they promote extensive crosstalk with Tregs and MDSCs, along with chemokines like CCL20, CCL22, and CXCL12, further enhancing immunosuppression (93, 94).
4 Overview of DCV clinical trials
4.1 The source of DCs
A large majority of DC vaccines in clinical trials are based on MoDCs. In particular, DCV trials in GBM now all use MoDCs. The common method is to collect autologous monocytes from patients, induce them to differentiate into immature DCs in vitro, expose them to TAAs after induction of maturation, and then transfuse them into the same patient. In trials that used mDCs, DC maturation was mostly induced by GM-CSF combined with IL-4, PGE2, TNF-α, or IFN-γ (95–99). There are couples of trials that induce DC maturation by using IL-6, IL-1β, TNF-α, or PGE2 without GM-CSF (100–102). Although cDCs may be superior to MoDCs in their ability to stimulate T cells (7, 103), there are currently no established protocols for isolating or differentiating these cells in vitro. Nonetheless, in some diseases, most notably melanoma, the use of cDCs and pDCs as DC vaccines has shown some encouraging early results that may be extended to GBM research in the future (104–107).
In addition, since iDCs are less capable of stimulating T cells than mDCs and may even induce tolerance, mDCs are used in most DCV trials. However, there are trials using iDCs that have reported clinical benefits (108, 109).
4.2 Tumor-associated antigens
By priming CD8+ T cells against TAA, DCs are an important part in antitumor immunity. Thus, the efficacy of DCV is related to the existence of TAAs, also known as neoantigens, in individual tumors. The overall mutational burden of GBM is very low, but patients who relapse after TMZ chemotherapy have an increased mutational burden (110).
Previous trials using DCV used tumor lysates, tumor cell apoptotic bodies, irradiated tumor cells, DC-tumor cell fusion, and tumor cell surface eluted peptides as whole tumor cell TAAs. Whole tumor cell-derived TAAs contain numerous TAAs, assuring the diversity of antigens and reducing the risk of TAA-loss variants escaping (111). However, due to the immunosuppressive factors produced by glioma cells, whole tumor cell-derived TAAs may inhibit the DC differentiation and maturation or alter the function of generated DCs (112). Furthermore, whole tumor cell-derived TAA vaccines produced using current methods are poorly immunogenic and difficult to induce potent and durable T cell responses (113).
Some DCV studies use molecularly defined TAAs, including specific peptides, proteins, and DCs transfected with TAA-coding mRNA. The source of molecularly defined TAAs is more standardized and reproducible, making it easier to monitor target-specific responses. In addition, they can be personalized for different individuals (114). However, compared to TAAs derived from whole tumor cells, molecularly defined TAAs lack diversity. Therefore, to reduce the risk of escape of TAA-loss mutants, several molecularly defined TAAs should be used.
4.3 Dose and route of application
To induce a T cell response in a healthy subject, the minimum DC dose is 2×10^6 DC/vaccine (115), while no study to date has achieved dose-limiting toxicity. While several clinical studies aiming at determining the best dose of DCV therapy have been conducted previously, and some of them have been completed (e. g. NCT00612001, NCT01171469, NCT00068510, NCT00107185), the relationship between clinical outcomes and DC dose, and the dose-response relationship of the optimal dose have remained inconclusive. Studies have shown that patients receiving lower doses of DC have longer survival (116); while some studies suggest that improving the efficiency of DC migrating to lymph nodes may increase patient survival (117). This may be because the DCV used in these studies was handled differently as well as the status of DC, making it difficult to compare to derive the optimal dose of DC. In the existing clinical trials, almost all patients received multiple vaccinations, mainly using the prime-boost method (18). Several studies have reported a trend toward improved survival in booster recipients (102).
Different routes of injection of DCV result in different distributions of DC in vivo (118, 119). Currently, the routes of administration used in clinical trials of DCV include intravenous injection, subcutaneous injection, and nodal injection. Subcutaneous injection is by far the most common route of administration, with up to 4% of DCV reaching the draining lymph nodes. Irrespective of the routes of administration, high numbers of DCs remained at the injection site, lost viability, and were eliminated by infiltrating CD163+ macrophages within 48 hours (120). The intranodal injection may allow more DCs to migrate to the T-cell region, but whether it is more effective in inducing antigen-specific immune responses remains to be determined (120).
4.4 Treatment options
Most patients underwent cytoreductive surgery before DCV, while some patients underwent biopsy alone or without surgery. The extent of surgical resection is positively associated with survival (121), while minimal residual disease status also favors DCV therapy (121, 122), which may be related to a reduction in local immunosuppression (123). Yet, other studies have shown that the extent of resection is not related to survival in DCV treatment (124). Therefore, in addition to the absolute volume of the residual tumor, other factors such as the composition of the residual tumor may influence the effect of DCV.
DCV treatment is often combined with radiotherapy or chemotherapy, or both. Tumor cell death after chemoradiotherapy releases tumor antigens, then the brain endothelium presents MHC class I antigens to circulating CD8+ T cells, which can enhance the tumor-specific effector CTL homing to brain tumors (125). The most widely used chemotherapeutic agent combined with DCV is TMZ, which has been used in all current DCV-controlled trials. TMZ can improve immunoreactivity by reducing Tregs and interfering with their recruitment to tumors (126). Although TMZ often induces lymphopenia, the lymphocyte zone restored after chemotherapy can still induce an antitumor response (117, 127). The specific efficacy is related to the dose of TMZ: for example, lower doses of TMZ help deplete Tregs, whereas myelosuppressive doses enhance the response to peptide vaccines (128). However, there is also evidence that CD8+ T cells expanded by DCV previously may be depleted by TMZ (100, 129). Moreover, only in the absence of TMZ was DCV able to generate IFN-γ-producing effector memory T cells, which was positively related to survival (130). Thus, the effect of TMZ on DCV efficacy remains inconclusive.
4.5 Safety
By far, no serious vaccine-related adverse events have been observed, except for a few studies that reported severe adverse events (grade ≥3) according to the National Cancer Institute Common Toxicity Criteria (NCI CTC). Some of these adverse events were severe peritumoral edema leading to other neurological symptoms (96, 131); some were allergies following co-injection of DCV and GM-CSF (132).
Commonly observed adverse reactions attributed to DCV are generally mild (≤ grade 2), including induration, pain, pruritus, and erythema in injection sites, as well as meningeal irritation, lymph node swelling, flu-like symptoms, edema, etc (95, 97, 98, 101, 102, 116, 133–143). These symptoms may be caused by disease progression or other concomitant therapies as well. All in all, DCV therapy was well tolerated as a therapeutic method (for a brief introduction of DCV clinical trials registered in clinical trials.gov, see Table 2 and Table 3).
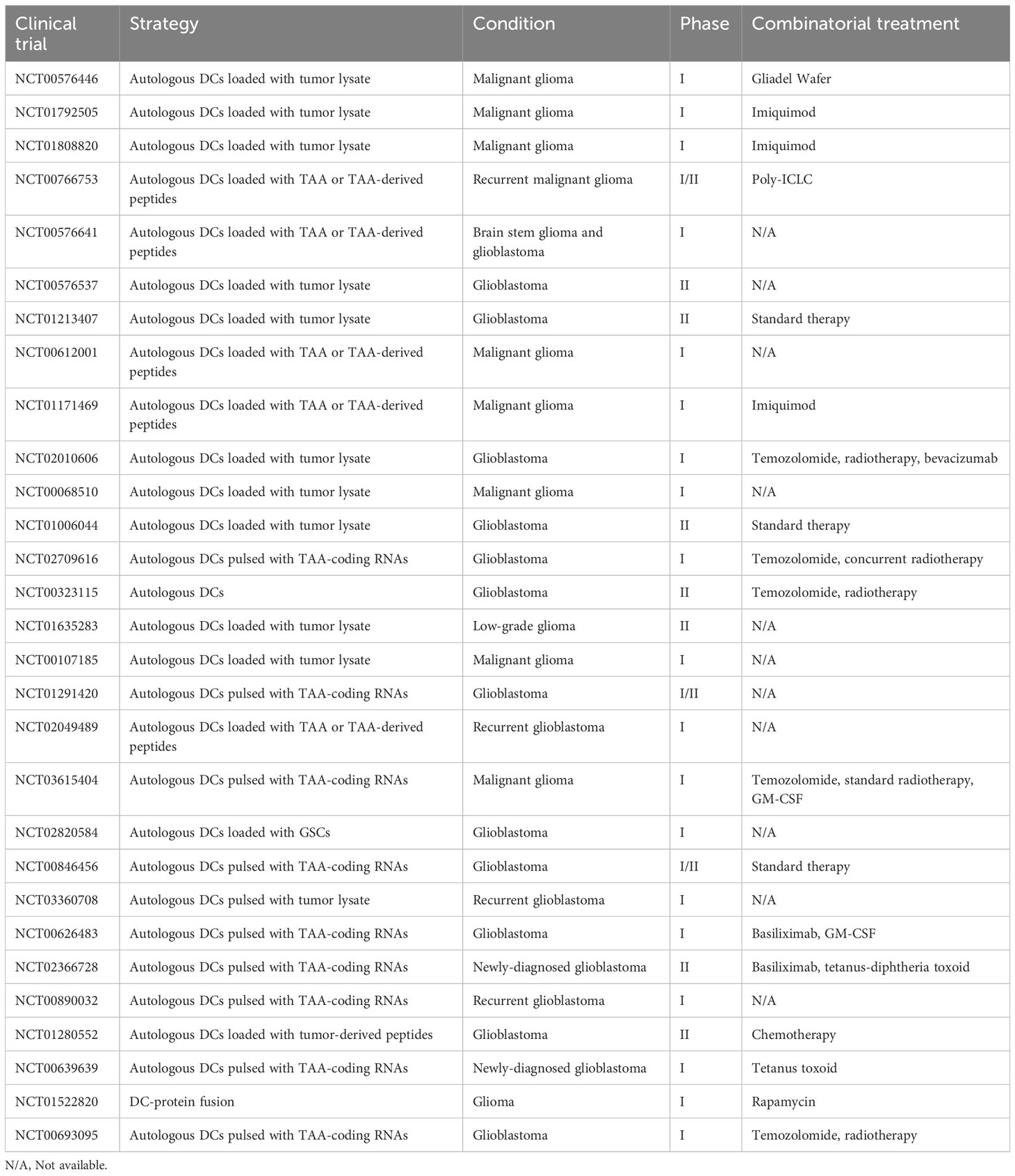
Table 2 Completed clinical trials registered on clinicaltrials.gov concerning dendritic cell vaccine in glioma patients.
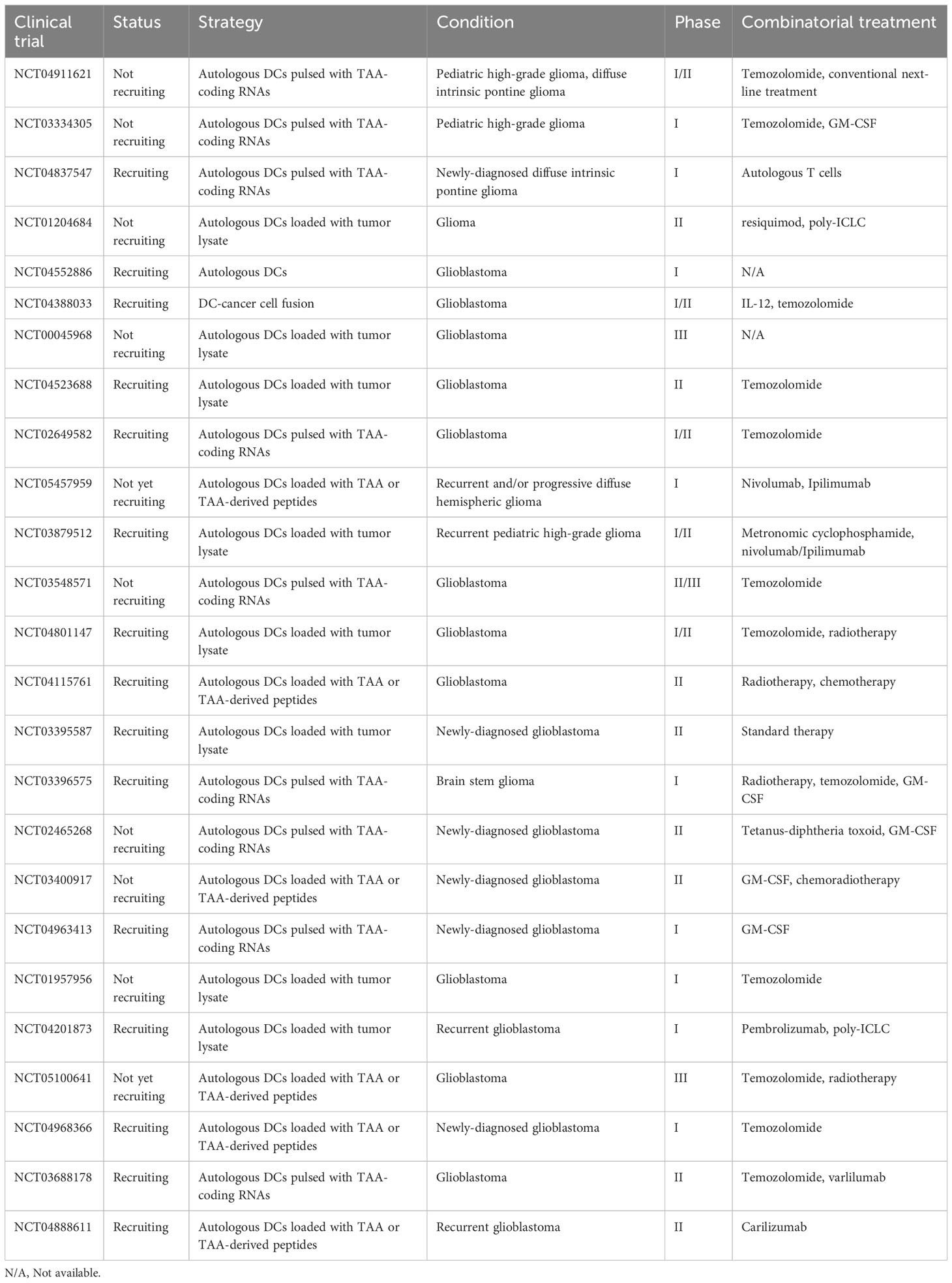
Table 3 Ongoing clinical trials registered on clinicaltrials.gov concerning dendritic cell vaccine in glioma patients.
5 Optimizing DCV therapy
5.1 Develop Other DC-derived vaccines
Although most DCVs use MoDCs that have been induced to differentiate in vitro, long-term in vitro culture can result in decreased MoDC migration and functional loss (7). Therefore, MoDC is probably not the best DC subpopulation for vaccine manufacturing, and the development of vaccines based on naturally circulating DC subtypes such as cDC, pDC, or Langhans cell may achieve better results. Among these DC subsets, it has been proven that cDCs have a stronger ability to induce CD8+ T cell response (7, 103). To date, the difficulty of producing cDC1/2 in large quantities from patients remains an obstacle to cDC-based DCVs. Therefore, future efforts should focus on solving the technical and cost issues of generating large numbers of cDCs.
5.2 Screen specific immune biomarkers
ICIs have achieved clinical success in effectively treating various cancers, which are related to specific immune biomarkers to guide application. Immune biomarkers such as tumor mutation burden and PD-L1 positivity provide accurate and non-invasive means for patient preselection (13, 15, 144), which are of great value to the success of antitumor immunotherapy. Unfortunately, the lack of strong patient-preselected biomarkers immensely limits the guide of application of DCV; therefore, there is a surge in urgency to screen out biomarkers that are most likely to predict a positive patient response to DCV. The selection of patient subgroups by specific biomarkers that improve the likelihood of a subject’s response to DCV will help guide the design of clinical trials.
5.3 Improve the function of DC in the glioma microenvironment
The glioma microenvironment is composed of various immunosuppressive cells, all of which are of great importance in disease progression. Targeting only one type of cell is not sufficient to modify the entire TME. Therefore, to improve DC function in the TME, it may be necessary to combine it with a variety of other immunotherapy methods to get over the negative effects of immunosuppression and immune checkpoint modulation.
In preclinical models, anti-CD25 antibodies are commonly used to deplete Tregs (145–147) or limit their immunosuppressive function by blocking molecules like PD-1, CTLA-4, and Tim-3 or enzymes such as IDO (146, 148, 149). However, it has been reported in murine models that high-dose unfractionated radiotherapy or low-dose TMZ or cyclophosphamide chemotherapy can deplete Tregs (150, 151). An encouraging approach is reducing the effect of Tregs by combining radiotherapy with anti-IDO, which eventually improves the survival of mice (152). When anti-CD25 therapy is combined, beneficial effects on survival caused by DCV have been reported by several other studies, especially when Tregs are depleted before vaccination (145, 153).
In vitro, paclitaxel promoted MDSC differentiation into DCs in a TLR4-independent manner (154). Docetaxel induces the transformation of MDSCs into M1-like macrophages and selectively enhances CTL responses (155). All-trans retinoic acid can promote MDSC maturation (156). In addition, low doses of 5-FU (157), capecitabine (158), etc. can deplete MDSCs. Pexidatinib reduced MDSCs and M2-like TAMs by blocking CSF-1 receptor signaling (159), while STAT3 inhibitors reduced MDSCs and impaired their function (160).
Blocking the CSF-1/CSF-1R axis prevents monocyte differentiation, thereby reducing the number of TAMs, while also reducing the survival of existing TAMs (161, 162). Blockade of the CCL2/CCR2 axis inhibited monocyte recruitment but did not affect the TAMs formed (163, 164). CD47 is a “don’t eat me” signal, and blocking CD47/SIRP enhances TAM phagocytosis of tumor cells (165). Oncolytic virotherapy repolarizes M2-like TAMs to M1-like TAMs (166, 167).
Although ICIs have achieved impressive results in various tumors, and immune checkpoint inhibitors have improved survival in a mouse GBM model (146, 148, 149, 168), ICIs alone have not been effective in the treatment of GBM (17, 169). However, the combination of ICI and DCV was more effective than DCV alone. Currently, the most commonly used target of ICIs is PD-1, followed by CTLA-4 (10).
5.4 New routes of administration
To date, there haven’t been any reported clinical trials for glioma using intratumoral injection of DCV yet. It has been shown that in an orthotopic GL261 glioma murine model, compared with subcutaneous injection of GL261 lysate-loaded DCs, intratumoral injection is less effective; however, combining these two administration routes is more effective than subcutaneous injection alone (170). Intratumoral injected DCs could be detected in the tumor parenchyma while not in the cervical lymph nodes. Therefore, intratumoral injection of DC may have a distinct mechanism to improve survival. This may be because intratumoral DC injection enhances the anti-tumor immune response induced by subcutaneous injection of DC by pro-immunomodulating cytokines in the TME, reducing Treg cells, and directly inhibiting tumor proliferation by TNF (170, 171). Therefore, combining the two in clinical trials may lead to better results.
6 Conclusion
Thousands of glioma patients have been treated with DCV over the past few decades. During this period of time, the methods of production and treatment of DCV have also been gradually diversified. Due to the weak immunogenicity of DCV produced by conventional methods, which cannot induce strong and durable T-cell responses, many efforts have been made to improve their immunogenicity (172, 173). Yet, those DCVs with higher immunogenicity don’t seem to be as clinically successful as expected. Thus, whether there is a better way to improve immunogenicity or whether immunogenicity doesn’t take a crucial part in the effect of DCV remains a question. Although no definitive conclusion can be made about the efficacy of DCV, some promising results still show the great potential of DCV as a therapeutic tool for GBM. To conclude, the reasons why the clinical application of DCV is not as good as expected may be related to the limitation of DC function by the immunosuppressive microenvironment, the lack of optimal dosage standards, and the lack of specific immune biomarkers. Either way, if future studies address the above issues, DCV will have a significant impact on GBM treatment and significantly improve patient outcomes.
Author contributions
SZ: Writing – review & editing. YZ: Writing – original draft. XM: Writing – review & editing. SF: Writing – review & editing. HZ: Writing – review & editing. XC: Writing – review & editing. XY: Writing – review & editing. KS: Writing – review & editing.
Funding
The authors declare financial support was received for the research, authorship, and/or publication of this article. This work was supported by the National Natural Science Foundation of China (82072805) and Hubei Natural Science Foundation (2020CFB678).
Conflict of interest
The authors declare that the research was conducted in the absence of any commercial or financial relationships that could be construed as a potential conflict of interest.
Publisher’s note
All claims expressed in this article are solely those of the authors and do not necessarily represent those of their affiliated organizations, or those of the publisher, the editors and the reviewers. Any product that may be evaluated in this article, or claim that may be made by its manufacturer, is not guaranteed or endorsed by the publisher.
References
1. Molinaro AM, Taylor JW, Wiencke JK, Wrensch MR. Genetic and molecular epidemiology of adult diffuse glioma. Nat Rev Neurol (2019) 15(7):405–17. doi: 10.1038/s41582-019-0220-2
2. Louis DN, Perry A, Wesseling P, Brat DJ, Cree IA, Figarella-Branger D, et al. The 2021 WHO classification of tumors of the central nervous system: a summary. Neuro Oncol (2021) 23(8):1231–51. doi: 10.1093/neuonc/noab106
3. Weller M, van den Bent M, Hopkins K, Tonn JC, Stupp R, Falini A, et al. EANO guideline for the diagnosis and treatment of anaplastic gliomas and glioblastoma. Lancet Oncol (2014) 15(9):e395–403. doi: 10.1016/S1470-2045(14)70011-7
4. Fisher JP, Adamson DC. Current FDA-approved therapies for high-grade Malignant gliomas. Biomedicines (2021) 9(3):324. doi: 10.3390/biomedicines9030324
5. Cruz Da Silva E, Mercier MC, Etienne-Selloum N, Dontenwill M, Choulier L. A systematic review of glioblastoma-targeted therapies in phases II, III, IV clinical trials. Cancers (Basel) (2021) 13(8):1795. doi: 10.3390/cancers13081795
6. Joffre OP, Segura E, Savina A, Amigorena S. Cross-presentation by dendritic cells. Nat Rev Immunol (2012) 12(8):557–69. doi: 10.1038/nri3254
7. Wculek SK, Cueto FJ, Mujal AM, Melero I, Krummel MF, Sancho D. Dendritic cells in cancer immunology and immunotherapy. Nat Rev Immunol (2020) 20(1):7–24. doi: 10.1038/s41577-019-0210-z
8. Datsi A, Sorg RV. Dendritic cell vaccination of glioblastoma: road to success or dead end. Front Immunol (2021) 12:770390. doi: 10.3389/fimmu.2021.770390
9. Hsu FJ, Benike C, Fagnoni F, Liles TM, Czerwinski D, Taidi B, et al. Vaccination of patients with B-cell lymphoma using autologous antigen-pulsed dendritic cells. Nat Med (1996) 2(1):52–8. doi: 10.1038/nm0196-52
10. Laureano RS, Sprooten J, Vanmeerbeerk I, Borras DM, Govaerts J, Naulaerts S, et al. Trial watch: Dendritic cell (DC)-based immunotherapy for cancer. OncoImmunology (2022) 11(1):2096363. doi: 10.1080/2162402X.2022.2096363
11. Sprooten J, Ceusters J, Coosemans A, Agostinis P, De Vleeschouwer S, Zitvogel L, et al. Trial watch: dendritic cell vaccination for cancer immunotherapy. Oncoimmunology (2019) 8(11):e1638212. doi: 10.1080/2162402X.2019.1638212
12. Kantoff PW, Higano CS, Shore ND, Berger ER, Small EJ, Penson DF, et al. Sipuleucel-T immunotherapy for castration-resistant prostate cancer. N Engl J Med (2010) 363(5):411–22. doi: 10.1056/NEJMoa1001294
13. Doroshow DB, Bhalla S, Beasley MB, Sholl LM, Kerr KM, Gnjatic S, et al. PD-L1 as a biomarker of response to immune-checkpoint inhibitors. Nat Rev Clin Oncol (2021) 18(6):345–62. doi: 10.1038/s41571-021-00473-5
14. Klempner SJ, Fabrizio D, Bane S, Reinhart M, Peoples T, Ali SM, et al. Tumor mutational burden as a predictive biomarker for response to immune checkpoint inhibitors: A review of current evidence. Oncologist (2020) 25(1):e147–59. doi: 10.1634/theoncologist.2019-0244
15. Sha D, Jin Z, Budczies J, Kluck K, Stenzinger A, Sinicrope FA. Tumor mutational burden as a predictive biomarker in solid tumors. Cancer Discov (2020) 10(12):1808–25. doi: 10.1158/2159-8290.CD-20-0522
16. Kurz SC, Cabrera LP, Hastie D, Huang R, Unadkat P, Rinne M, et al. PD-1 inhibition has only limited clinical benefit in patients with recurrent high-grade glioma. Neurology (2018) 91(14):e1355–9. doi: 10.1212/WNL.0000000000006283
17. Lim M, Weller M, Idbaih A, Steinbach J, Finocchiaro G, Raval RR, et al. Phase III trial of chemoradiotherapy with temozolomide plus nivolumab or placebo for newly diagnosed glioblastoma with methylated MGMT promoter. Neuro Oncol (2022) 24(11):1935–49. doi: 10.1093/neuonc/noac116
18. Harari A, Graciotti M, Bassani-Sternberg M, Kandalaft LE. Antitumour dendritic cell vaccination in a priming and boosting approach. Nat Rev Drug Discov (2020) 19(9):635–52. doi: 10.1038/s41573-020-0074-8
19. Liau LM, Ashkan K, Brem S, Campian JL, Trusheim JE, Iwamoto FM, et al. Association of autologous tumor lysate-loaded dendritic cell vaccination with extension of survival among patients with newly diagnosed and recurrent glioblastoma: A phase 3 prospective externally controlled cohort trial. JAMA Oncol (2023) 9(1):112–21. doi: 10.1001/jamaoncol.2022.5370
20. Liau LM, Ashkan K, Tran DD, Campian JL, Trusheim JE, Cobbs CS, et al. First results on survival from a large Phase 3 clinical trial of an autologous dendritic cell vaccine in newly diagnosed glioblastoma. J Transl Med (2018) 16(1):142. doi: 10.1186/s12967-018-1507-6
21. Schlitzer A, Sivakamasundari V, Chen J, Sumatoh HR, Schreuder J, Lum J, et al. Identification of cDC1- and cDC2-committed DC progenitors reveals early lineage priming at the common DC progenitor stage in the bone marrow. Nat Immunol (2015) 16(7):718–28. doi: 10.1038/ni.3200
22. Brown CC, Gudjonson H, Pritykin Y, Deep D, Lavallée VP, Mendoza A, et al. Transcriptional basis of mouse and human dendritic cell heterogeneity. Cell (2019) 179(4):846–863.e24. doi: 10.1016/j.cell.2019.09.035
23. Collin M, Bigley V. Human dendritic cell subsets: an update. Immunology (2018) 154(1):3–20. doi: 10.1111/imm.12888
24. Villani AC, Satija R, Reynolds G, Sarkizova S, Shekhar K, Fletcher J, et al. Single-cell RNA-seq reveals new types of human blood dendritic cells, monocytes, and progenitors. Science (2017) 356(6335):eaah4573. doi: 10.1126/science.aah4573
25. Rodrigues PF, Alberti-Servera L, Eremin A, Grajales-Reyes GE, Ivanek R, Tussiwand R. Distinct progenitor lineages contribute to the heterogeneity of plasmacytoid dendritic cells. Nat Immunol (2018) 19(7):711–22. doi: 10.1038/s41590-018-0136-9
26. Shigematsu H, Reizis B, Iwasaki H, Mizuno S, Hu D, Traver D, et al. Plasmacytoid dendritic cells activate lymphoid-specific genetic programs irrespective of their cellular origin. Immunity (2004) 21(1):43–53. doi: 10.1016/j.immuni.2004.06.011
27. Sever L, Radomir L, Stirm K, Wiener A, Schottlender N, Lewinsky H, et al. SLAMF9 regulates pDC homeostasis and function in health and disease. Proc Natl Acad Sci USA (2019) 116(33):16489–96. doi: 10.1073/pnas.1900079116
28. Mitchell D, Chintala S, Dey M. Plasmacytoid dendritic cell in immunity and cancer. J Neuroimmunol (2018) 322:63–73. doi: 10.1016/j.jneuroim.2018.06.012
29. Segura E, Touzot M, Bohineust A, Cappuccio A, Chiocchia G, Hosmalin A, et al. Human inflammatory dendritic cells induce Th17 cell differentiation. Immunity (2013) 38(2):336–48. doi: 10.1016/j.immuni.2012.10.018
30. Angelova M, Mascaux C, Galon J. Evasion before invasion: Pre-cancer immunosurveillance. Oncoimmunology (2021) 10(1):1912250. doi: 10.1080/2162402X.2021.1912250
31. Mahnke K, Schmitt E, Bonifaz L, Enk AH, Jonuleit H. Immature, but not inactive: the tolerogenic function of immature dendritic cells. Immunol Cell Biol (2002) 80(5):477–83. doi: 10.1046/j.1440-1711.2002.01115.x
32. Idoyaga J, Fiorese C, Zbytnuik L, Lubkin A, Miller J, Malissen B, et al. Specialized role of migratory dendritic cells in peripheral tolerance induction. J Clin Invest (2013) 123(2):844–54. doi: 10.1172/JCI65260
33. Steinman RM, Hawiger D, Nussenzweig MC. Tolerogenic dendritic cells. Annu Rev Immunol (2003) 21:685–711. doi: 10.1146/annurev.immunol.21.120601.141040
34. Roncarolo MG, Levings MK, Traversari C. Differentiation of T regulatory cells by immature dendritic cells. J Exp Med (2001) 193(2):F5–9. doi: 10.1084/jem.193.2.f5
35. Dalod M, Chelbi R, Malissen B, Lawrence T. Dendritic cell maturation: functional specialization through signaling specificity and transcriptional programming. EMBO J (2014) 33(10):1104–16. doi: 10.1002/embj.201488027
36. Bowman-Kirigin JA, Desai R, Saunders BT, Wang AZ, Schaettler MO, Liu CJ, et al. The conventional dendritic cell 1 subset primes CD8+ T cells and traffics tumor antigen to drive antitumor immunity in the brain. Cancer Immunol Res (2023) 11(1):20–37. doi: 10.1158/2326-6066.CIR-22-0098
37. Böttcher JP, Reis e Sousa C. The role of type 1 conventional dendritic cells in cancer immunity. Trends Cancer (2018) 4(11):784–92. doi: 10.1016/j.trecan.2018.09.001
38. Hubert M, Gobbini E, Couillault C, Manh TV, Doffin AC, Berthet J, et al. IFN-III is selectively produced by cDC1 and predicts good clinical outcome in breast cancer. Sci Immunol (2020) 5(46):eaav3942. doi: 10.1126/sciimmunol.aav3942
39. Lauterbach H, Bathke B, Gilles S, Traidl-Hoffmann C, Luber CA, Fejer G, et al. Mouse CD8alpha+ DCs and human BDCA3+ DCs are major producers of IFN-lambda in response to poly IC. J Exp Med. (2010) 207(12):2703–17. doi: 10.1084/jem.20092720
40. Wculek SK, Amores-Iniesta J, Conde-Garrosa R, Khouili SC, Melero I, Sancho D. Effective cancer immunotherapy by natural mouse conventional type-1 dendritic cells bearing dead tumor antigen. J Immunother Cancer (2019) 7(1):100. doi: 10.1186/s40425-019-0565-5
41. Reindl LM, Albinger N, Bexte T, Müller S, Hartmann J, Ullrich E. Immunotherapy with NK cells: recent developments in gene modification open up new avenues. Oncoimmunology (2020) 9(1):1777651. doi: 10.1080/2162402X.2020.1777651
42. Nizzoli G, Krietsch J, Weick A, Steinfelder S, Facciotti F, Gruarin P, et al. Human CD1c+ dendritic cells secrete high levels of IL-12 and potently prime cytotoxic T-cell responses. Blood (2013) 122(6):932–42. doi: 10.1182/blood-2013-04-495424
43. Binnewies M, Mujal AM, Pollack JL, Combes AJ, Hardison EA, Barry KC, et al. Unleashing type-2 dendritic cells to drive protective antitumor CD4(+) T cell immunity. Cell (2019) 177(3):556–571.e16. doi: 10.1016/j.cell.2019.02.005
44. Ma Y, Adjemian S, Mattarollo SR, Yamazaki T, Aymeric L, Yang H, et al. Anticancer chemotherapy-induced intratumoral recruitment and differentiation of antigen-presenting cells. Immunity (2013) 38(4):729–41. doi: 10.1016/j.immuni.2013.03.003
45. Casares N, Pequignot MO, Tesniere A, Ghiringhelli F, Roux S, Chaput N, et al. Caspase-dependent immunogenicity of doxorubicin-induced tumor cell death. J Exp Med (2005) 202(12):1691–701. doi: 10.1084/jem.20050915
46. Sprooten J, Agostinis P, Garg AD. Type I interferons and dendritic cells in cancer immunotherapy. Int Rev Cell Mol Biol (2019) 348:217–62. doi: 10.1016/bs.ircmb.2019.06.001
47. Gong W, Donnelly CR, Heath BR, Bellile E, Donnelly LA, Taner HF, et al. Cancer-specific type-I interferon receptor signaling promotes cancer stemness and effector CD8+ T-cell exhaustion. Oncoimmunology (2021) 10(1):1997385. doi: 10.1080/2162402X.2021.1997385
48. Zhang X, Wang S, Zhu Y, Zhang M, Zhao Y, Yan Z, et al. Double-edged effects of interferons on the regulation of cancer-immunity cycle. Oncoimmunology (2021) 10(1):1929005. doi: 10.1080/2162402X.2021.1929005
49. De Martino M, Vanpouille-Box C. Type I interferon induces cancer stem cells-mediated chemotherapy resistance. Oncoimmunology (2022) 11(1):2127274. doi: 10.1080/2162402X.2022.2127274
50. Musella M, Guarracino A, Manduca N, Galassi C, Ruggiero E, Potenza A, et al. Type I IFNs promote cancer cell stemness by triggering the epigenetic regulator KDM1B. Nat Immunol (2022) 23(9):1379–92. doi: 10.1038/s41590-022-01290-3
51. Sampson JH, Gunn MD, Fecci PE, Ashley DM. Brain immunology and immunotherapy in brain tumours. Nat Rev Cancer (2020) 20(1):12–25. doi: 10.1038/s41568-019-0224-7
52. Iliff JJ, Wang M, Liao Y, Plogg BA, Peng W, Gundersen GA, et al. A paravascular pathway facilitates CSF flow through the brain parenchyma and the clearance of interstitial solutes, including amyloid β. Sci Transl Med (2012) 4(147):147ra111. doi: 10.1126/scitranslmed.3003748
53. Aspelund A, Antila S, Proulx ST, Karlsen TV, Karaman S, Detmar M, et al. A dural lymphatic vascular system that drains brain interstitial fluid and macromolecules. J Exp Med (2015) 212(7):991–9. doi: 10.1084/jem.20142290
54. Louveau A, Herz J, Alme MN, Salvador AF, Dong MQ, Viar KE, et al. CNS lymphatic drainage and neuroinflammation are regulated by meningeal lymphatic vasculature. Nat Neurosci (2018) 21(10):1380–91. doi: 10.1038/s41593-018-0227-9
55. Srivastava S, Jackson C, Kim T, Choi J, Lim A. A characterization of dendritic cells and their role in immunotherapy in glioblastoma: from preclinical studies to clinical trials. Cancers (Basel) (2019) 11(4):537. doi: 10.3390/cancers11040537
56. Owens T, Bechmann I, Engelhardt B. Perivascular spaces and the two steps to neuroinflammation. J Neuropathol Exp Neurol (2008) 67(12):1113–21. doi: 10.1097/NEN.0b013e31818f9ca8
57. Schläger C, Körner H, Krueger M, Vidoli S, Haberl M, Mielke D, et al. Effector T-cell trafficking between the leptomeninges and the cerebrospinal fluid. Nature (2016) 530(7590):349–53. doi: 10.1038/nature16939
58. Watkins S, Robel S, Kimbrough IF, Robert SM, Ellis-Davies G, Sontheimer H. Disruption of astrocyte-vascular coupling and the blood-brain barrier by invading glioma cells. Nat Commun (2014) 5:4196. doi: 10.1038/ncomms5196
59. Gajewski TF, Schreiber H, Fu YX. Innate and adaptive immune cells in the tumor microenvironment. Nat Immunol (2013) 14(10):1014–22. doi: 10.1038/ni.2703
60. Gajewski TF, Corrales L, Williams J, Horton B, Sivan A, Spranger S. Cancer immunotherapy targets based on understanding the T cell-inflamed versus non-T cell-inflamed tumor microenvironment. Adv Exp Med Biol (2017) 1036:19–31. doi: 10.1007/978-3-319-67577-0_2
61. Keskin DB, Anandappa AJ, Sun J, Tirosh I, Mathewson ND, Li S, et al. Neoantigen vaccine generates intratumoral T cell responses in phase Ib glioblastoma trial. Nature (2019) 565(7738):234–9. doi: 10.1038/s41586-018-0792-9
62. Gong D, Shi W, ju Y, Chen H, Groffen J, Heisterkamp N. TGFβ signaling plays a critical role in promoting alternative macrophage activation. BMC Immunol (2012) 13:31. doi: 10.1186/1471-2172-13-31
63. Wainwright DA, Balyasnikova IV, Chang AL, Ahmed AU, Moon KS, Auffinger B, et al. IDO expression in brain tumors increases the recruitment of regulatory T cells and negatively impacts survival. Clin Cancer Res (2012) 18(22):6110–21. doi: 10.1158/1078-0432.CCR-12-2130
64. Zhang I, Alizadeh D, Liang J, Zhang L, Gao H, Song Y, et al. Characterization of arginase expression in glioma-associated microglia and macrophages. PloS One (2016) 11(12):e0165118. doi: 10.1371/journal.pone.0165118
65. Zhou J, Li L, Jia M, Liao Q, Peng G, Luo G, et al. Dendritic cell vaccines improve the glioma microenvironment: Influence, challenges, and future directions. Cancer Med (2023) 12(6):7207–21. doi: 10.1002/cam4.5511
66. Friedrich M, Hahn M, Michel J, Sankowski R, Kilian M, Kehl N, et al. Dysfunctional dendritic cells limit antigen-specific T cell response in glioma. Neuro-Oncology (2023) 25(2):263–76. doi: 10.1093/neuonc/noac138
67. Johanns TM, Ward JP, Miller CA, Wilson C, Kobayashi DK, Bender D, et al. Endogenous neoantigen-specific CD8 T cells identified in two glioblastoma models using a cancer immunogenomics approach. Cancer Immunol Res (2016) 4(12):1007–15. doi: 10.1158/2326-6066.CIR-16-0156
68. Taggart D, Andreou T, Scott KJ, Williams J, Rippaus N, Brownlie RJ, et al. Anti-PD-1/anti-CTLA-4 efficacy in melanoma brain metastases depends on extracranial disease and augmentation of CD8(+) T cell trafficking. Proc Natl Acad Sci USA (2018) 115(7):E1540–9. doi: 10.1073/pnas.1714089115
69. Cui X, Ma C, Vasudevaraja V, Serrano J, Tong J, Peng Y, et al. Dissecting the immunosuppressive tumor microenvironments in Glioblastoma-on-a-Chip for optimized PD-1 immunotherapy. Elife (2020) 9:e52253. doi: 10.7554/eLife.52253
70. Fu C, Jiang A. Dendritic cells and CD8 T cell immunity in tumor microenvironment. Front Immunol (2018) 9:3059. doi: 10.3389/fimmu.2018.03059
71. Patente TA, Pinho MP, Oliveira AA, Evangelista GCM, Bergami-Santos PC, Barbuto JAM. Human dendritic cells: their heterogeneity and clinical application potential in cancer immunotherapy. Front Immunol (2018) 9:3176. doi: 10.3389/fimmu.2018.03176
72. Yan J, Zhao Q, Gabrusiewicz K, Kong LY, Xia X, Wang J, et al. FGL2 promotes tumor progression in the CNS by suppressing CD103(+) dendritic cell differentiation. Nat Commun (2019) 10(1):448. doi: 10.1038/s41467-018-08271-x
73. Wang J, Liu P, Xin S, Wang Z, Li J. Nrf2 suppresses the function of dendritic cells to facilitate the immune escape of glioma cells. Exp Cell Res (2017) 360(2):66–73. doi: 10.1016/j.yexcr.2017.07.031
74. Kelly B, O’Neill LAJ. Metabolic reprogramming in macrophages and dendritic cells in innate immunity. Cell Res (2015) 25(7):771–84. doi: 10.1038/cr.2015.68
75. Boedtkjer E, Pedersen SF. The acidic tumor microenvironment as a driver of cancer. Annu Rev Physiol (2020) 82:103–26. doi: 10.1146/annurev-physiol-021119-034627
76. Shang B, Liu Y, juan JS, Liu Y. Prognostic value of tumor-infiltrating FoxP3+ regulatory T cells in cancers: a systematic review and meta-analysis. Sci Rep (2015) 5:15179. doi: 10.1038/srep15179
77. Fecci PE, Mitchell DA, Whitesides JF, Xie W, Friedman AH, Archer GE, et al. Increased regulatory T-cell fraction amidst a diminished CD4 compartment explains cellular immune defects in patients with Malignant glioma. Cancer Res (2006) 66(6):3294–302. doi: 10.1158/0008-5472.CAN-05-3773
78. Richardson LG, Nieman LT, Stemmer-Rachamimov AO, Zheng XS, Stafford K, Nagashima H, et al. IDH-mutant gliomas harbor fewer regulatory T cells in humans and mice. Oncoimmunology (2020) 9(1):1806662. doi: 10.1080/2162402X.2020.1806662
79. Tran Thang NN, Derouazi M, Philippin G, Arcidiaco S, Di Berardino-Besson W, Masson F, et al. Immune infiltration of spontaneous mouse astrocytomas is dominated by immunosuppressive cells from early stages of tumor development. Cancer Res (2010) 70(12):4829–39. doi: 10.1158/0008-5472.CAN-09-3074
80. Muth S, Klaric A, Radsak M, Schild H, Probst HC. CD27 expression on Treg cells limits immune responses against tumors. J Mol Med (Berl) (2022) 100(3):439–49. doi: 10.1007/s00109-021-02116-9
81. Qu P, Wang LZ, Lin PC. Expansion and functions of myeloid-derived suppressor cells in the tumor microenvironment. Cancer Lett (2016) 380(1):253–6. doi: 10.1016/j.canlet.2015.10.022
82. Talmadge JE, Gabrilovich DI. History of myeloid-derived suppressor cells. Nat Rev Cancer (2013) 13(10):739–52. doi: 10.1038/nrc3581
83. Gabrilovich DI. Myeloid-derived suppressor cells. Cancer Immunol Res (2017) 5(1):3–8. doi: 10.1158/2326-6066.CIR-16-0297
84. Gielen PR, Schulte BM, Kers-Rebel ED, Verrijp K, Petersen-Baltussen HM, ter Laan M, et al. Increase in both CD14-positive and CD15-positive myeloid-derived suppressor cell subpopulations in the blood of patients with glioma but predominance of CD15-positive myeloid-derived suppressor cells in glioma tissue. J Neuropathol Exp Neurol (2015) 74(5):390–400. doi: 10.1097/NEN.0000000000000183
85. Alban TJ, Bayik D, Otvos B, Rabljenovic A, Leng L, Jia-Shiun L, et al. Glioblastoma myeloid-derived suppressor cell subsets express differential macrophage migration inhibitory factor receptor profiles that can be targeted to reduce immune suppression. Front Immunol (2020) 11:1191. doi: 10.3389/fimmu.2020.01191
86. Gabrilovich DI, Nagaraj S. Myeloid-derived suppressor cells as regulators of the immune system. Nat Rev Immunol (2009) 9(3):162–74. doi: 10.1038/nri2506
87. Haist M, Stege H, Grabbe S, Bros M. The Functional Crosstalk between Myeloid-Derived Suppressor Cells and Regulatory T Cells within the Immunosuppressive Tumor Microenvironment. Cancers (Basel) (2021) 13(2):210. doi: 10.3390/cancers13020210
88. Gabrilovich DI, Ostrand-Rosenberg S, Bronte V. Coordinated regulation of myeloid cells by tumours. Nat Rev Immunol (2012) 12(4):253–68. doi: 10.1038/nri3175
89. Khan F, Pang L, Dunterman M, Lesniak MS, Heimberger AB, Chen P. Macrophages and microglia in glioblastoma: heterogeneity, plasticity, and therapy. J Clin Invest (2023) 133(1):e163446. doi: 10.1172/JCI163446
90. Chen Z, Feng X, Herting CJ, Garcia VA, Nie K, Pong WW, et al. Cellular and molecular identity of tumor-associated macrophages in glioblastoma. Cancer Res (2017) 77(9):2266–78. doi: 10.1158/0008-5472.CAN-16-2310
91. Hambardzumyan D, Gutmann DH, Kettenmann H. The role of microglia and macrophages in glioma maintenance and progression. Nat Neurosci (2016) 19(1):20–7. doi: 10.1038/nn.4185
92. Quail DF, Joyce JA. The microenvironmental landscape of brain tumors. Cancer Cell (2017) 31(3):326–41. doi: 10.1016/j.ccell.2017.02.009
93. Chen Y, Song Y, Du W, Gong L, Chang H, Zou Z. Tumor-associated macrophages: an accomplice in solid tumor progression. J BioMed Sci (2019) 26(1):78. doi: 10.1186/s12929-019-0568-z
94. Cendrowicz E, Sas Z, Bremer E, Rygiel TP. The role of macrophages in cancer development and therapy. Cancers (Basel) (2021) 13(8):1946. doi: 10.3390/cancers13081946
95. Yu JS, Wheeler CJ, Zeltzer PM, Ying H, Finger DN, Lee PK, et al. Vaccination of Malignant glioma patients with peptide-pulsed dendritic cells elicits systemic cytotoxicity and intracranial T-cell infiltration. Cancer Res (2001) 61(3):842–7.
96. Rutkowski S, De Vleeschouwer S, Kaempgen E, Wolff JE, Kühl J, Demaerel P, et al. Surgery and adjuvant dendritic cell-based tumour vaccination for patients with relapsed Malignant glioma, a feasibility study. Br J Cancer (2004) 91(9):1656–62. doi: 10.1038/sj.bjc.6602195
97. Kikuchi T, Akasaki Y, Irie M, Homma S, Abe T, Ohno T. Results of a phase I clinical trial of vaccination of glioma patients with fusions of dendritic and glioma cells. Cancer Immunol Immunother (2001) 50(7):337–44. doi: 10.1007/s002620100205
98. Yamanaka R, Homma J, Yajima N, Tsuchiya N, Sano M, Kobayashi T, et al. Clinical evaluation of dendritic cell vaccination for patients with recurrent glioma: results of a clinical phase I/II trial. Clin Cancer Res (2005) 11(11):4160–7. doi: 10.1158/1078-0432.CCR-05-0120
99. Wen PY, Reardon DA, Armstrong TS, Phuphanich S, Aiken RD, Landolfi JC, et al. A randomized double-blind placebo-controlled phase II trial of dendritic cell vaccine ICT-107 in newly diagnosed patients with glioblastoma. Clin Cancer Res (2019) 25(19):5799–807. doi: 10.1158/1078-0432.CCR-19-0261
100. Batich KA, Mitchell DA, Healy P, Herndon JE 2nd, Sampson JH. Once, twice, three times a finding: reproducibility of dendritic cell vaccine trials targeting cytomegalovirus in glioblastoma. Clin Cancer Res (2020) 26(20):5297–303. doi: 10.1158/1078-0432.CCR-20-1082
101. Jie X, Hua L, Jiang W, Feng F, Feng G, Hua Z. Clinical application of a dendritic cell vaccine raised against heat-shocked glioblastoma. Cell Biochem Biophys (2012) 62(1):91–9. doi: 10.1007/s12013-011-9265-6
102. Okada H, Kalinski P, Ueda R, Hoji A, Kohanbash G, Donegan TE, et al. Induction of CD8+ T-cell responses against novel glioma-associated antigen peptides and clinical activity by vaccinations with {alpha}-type 1 polarized dendritic cells and polyinosinic-polycytidylic acid stabilized by lysine and carboxymethylcellulose in patients with recurrent Malignant glioma. J Clin Oncol (2011) 29(3):330–6. doi: 10.1200/JCO.2010.30.7744
103. Laoui D, Keirsse J, Morias Y, Van Overmeire E, Geeraerts X, Elkrim Y, et al. The tumour microenvironment harbours ontogenically distinct dendritic cell populations with opposing effects on tumour immunity. Nat Commun (2016) 7:13720. doi: 10.1038/ncomms13720
104. Tel J, Aarntzen EHJG, Baba T, Schreibelt G, Schulte BM, Benitez-Ribas D, et al. Natural human plasmacytoid dendritic cells induce antigen-specific T-cell responses in melanoma patients. Cancer Res (2013) 73(3):1063–75. doi: 10.1158/0008-5472.CAN-12-2583
105. Bloemendal M, Bol KF, Boudewijns S, Gorris MAJ, de Wilt JHW, Croockewit SAJ, et al. Immunological responses to adjuvant vaccination with combined CD1c(+) myeloid and plasmacytoid dendritic cells in stage III melanoma patients. Oncoimmunology (2022) 11(1):2015113. doi: 10.1080/2162402X.2021.2015113
106. Schreibelt G, Bol KF, Westdorp H, Wimmers F, Aarntzen EH, Duiveman-de Boer T, et al. Effective clinical responses in metastatic melanoma patients after vaccination with primary myeloid dendritic cells. Clin Cancer Res (2016) 22(9):2155–66. doi: 10.1158/1078-0432.CCR-15-2205
107. Charles J, Chaperot L, Hannani D, Bruder Costa J, Templier I, Trabelsi S, et al. An innovative plasmacytoid dendritic cell line-based cancer vaccine primes and expands antitumor T-cells in melanoma patients in a first-in-human trial. Oncoimmunology (2020) 9(1):1738812. doi: 10.1080/2162402X.2020.1738812
108. Cho DY, Yang WK, Lee HC, Hsu DM, Lin HL, Lin SZ, et al. Adjuvant immunotherapy with whole-cell lysate dendritic cells vaccine for glioblastoma multiforme: a phase II clinical trial. World Neurosurg (2012) 77(5-6):736–44. doi: 10.1016/j.wneu.2011.08.020
109. Yao Y, Luo F, Tang C, Chen D, Qin Z, Hua W, et al. Molecular subgroups and B7-H4 expression levels predict responses to dendritic cell vaccines in glioblastoma: an exploratory randomized phase II clinical trial. Cancer Immunol Immunother (2018) 67(11):1777–88. doi: 10.1007/s00262-018-2232-y
110. Finocchiaro G, Langella T, Corbetta C, Pellegatta S. Hypermutations in gliomas: a potential immunotherapy target. Discovery Med (2017) 23(125):113–20.
111. Sampson JH, Heimberger AB, Archer GE, Aldape KD, Friedman AH, Friedman HS, et al. Immunologic escape after prolonged progression-free survival with epidermal growth factor receptor variant III peptide vaccination in patients with newly diagnosed glioblastoma. J Clin Oncol (2010) 28(31):4722–9. doi: 10.1200/JCO.2010.28.6963
112. Erhart F, Weiss T, Klingenbrunner S, Fischhuber K, Reitermaier R, Halfmann A, et al. Spheroid glioblastoma culture conditions as antigen source for dendritic cell-based immunotherapy: spheroid proteins are survival-relevant targets but can impair immunogenic interferon γ production. Cytotherapy (2019) 21(6):643–58. doi: 10.1016/j.jcyt.2019.03.002
113. Chiang CLL, Coukos G, Kandalaft LE. Whole tumor antigen vaccines: where are we? Vaccines (Basel) (2015) 3(2):344–72. doi: 10.3390/vaccines3020344
114. Wang QT, Nie Y, Sun SN, Lin T, Han RJ, Jiang J, et al. Tumor-associated antigen-based personalized dendritic cell vaccine in solid tumor patients. Cancer Immunol Immunother (2020) 69(7):1375–87. doi: 10.1007/s00262-020-02496-w
115. Dhodapkar MV, Steinman RM, Sapp M, Desai H, Fossella C, Krasovsky J, et al. Rapid generation of broad T-cell immunity in humans after a single injection of mature dendritic cells. J Clin Invest (1999) 104(2):173–80. doi: 10.1172/JCI6909
116. Prins RM, Soto H, Konkankit V, Odesa SK, Eskin A, Yong WH, et al. Gene expression profile correlates with T-cell infiltration and relative survival in glioblastoma patients vaccinated with dendritic cell immunotherapy. Clin Cancer Res (2011) 17(6):1603–15. doi: 10.1158/1078-0432.CCR-10-2563
117. Mitchell DA, Batich KA, Gunn MD, Huang MN, Sanchez-Perez L, Nair SK, et al. Tetanus toxoid and CCL3 improve dendritic cell vaccines in mice and glioblastoma patients. Nature (2015) 519(7543):366–9. doi: 10.1038/nature14320
118. Quillien V, Moisan A, Carsin A, Lesimple T, Lefeuvre C, Adamski H, et al. Biodistribution of radiolabelled human dendritic cells injected by various routes. Eur J Nucl Med Mol Imaging (2005) 32(7):731–41. doi: 10.1007/s00259-005-1825-9
119. Shemesh CS, Hsu JC, Hosseini I, Shen BQ, Rotte A, Twomey P, et al. Personalized cancer vaccines: clinical landscape, challenges, and opportunities. Mol Ther (2021) 29(2):555–70. doi: 10.1016/j.ymthe.2020.09.038
120. Verdijk P, Aarntzen EHJG, Lesterhuis WJ, Boullart AC, Kok E, van Rossum MM, et al. Limited amounts of dendritic cells migrate into the T-cell area of lymph nodes but have high immune activating potential in melanoma patients. Clin Cancer Res (2009) 15(7):2531–40. doi: 10.1158/1078-0432.CCR-08-2729
121. Jan CI, Tsai WC, Harn HJ, Shyu WC, Liu MC, Lu HM, et al. Predictors of response to autologous dendritic cell therapy in glioblastoma multiforme. Front Immunol (2018) 9:727. doi: 10.3389/fimmu.2018.00727
122. Pellegatta S, Eoli M, Frigerio S, Antozzi C, Bruzzone MG, Cantini G, et al. The natural killer cell response and tumor debulking are associated with prolonged survival in recurrent glioblastoma patients receiving dendritic cells loaded with autologous tumor lysates. Oncoimmunology (2013) 2(3):e23401. doi: 10.4161/onci.23401
123. Choi SH, Stuckey DW, Pignatta S, Reinshagen C, Khalsa JK, Roozendaal N, et al. Tumor resection recruits effector T cells and boosts therapeutic efficacy of encapsulated stem cells expressing IFNβ in glioblastomas. Clin Cancer Res (2017) 23(22):7047–58. doi: 10.1158/1078-0432.CCR-17-0077
124. Buchroithner J, Erhart F, Pichler J, Widhalm G, Preusser M, Stockhammer G, et al. Audencel immunotherapy based on dendritic cells has no effect on overall and progression-free survival in newly diagnosed glioblastoma: A phase II randomized trial. Cancers (Basel) (2018) 10(10):372. doi: 10.3390/cancers10100372
125. Galea I, Bernardes-Silva M, Forse PA, van Rooijen N, Liblau RS, Perry VH. An antigen-specific pathway for CD8 T cells across the blood-brain barrier. J Exp Med (2007) 204(9):2023–30. doi: 10.1084/jem.20070064
126. Jordan JT, Sun W, Hussain SF, DeAngulo G, Prabhu SS, Heimberger AB. Preferential migration of regulatory T cells mediated by glioma-secreted chemokines can be blocked with chemotherapy. Cancer Immunol Immunother (2008) 57(1):123–31. doi: 10.1007/s00262-007-0336-x
127. Sampson JH, Aldape KD, Archer GE, Coan A, Desjardins A, Friedman AH, et al. Greater chemotherapy-induced lymphopenia enhances tumor-specific immune responses that eliminate EGFRvIII-expressing tumor cells in patients with glioblastoma. Neuro Oncol (2011) 13(3):324–33. doi: 10.1093/neuonc/noq157
128. Sanchez-Perez LA, Choi BD, Archer GE, Cui X, Flores C, Johnson LA, et al. Myeloablative temozolomide enhances CD8+ T-cell responses to vaccine and is required for efficacy against brain tumors in mice. PloS One (2013) 8(3):e59082. doi: 10.1371/journal.pone.0059082
129. Pellegatta S, Eoli M, Cuccarini V, Anghileri E, Pollo B, Pessina S, et al. Survival gain in glioblastoma patients treated with dendritic cell immunotherapy is associated with increased NK but not CD8(+) T cell activation in the presence of adjuvant temozolomide. Oncoimmunology (2018) 7(4):e1412901. doi: 10.1080/2162402X.2017.1412901
130. Eoli M, Corbetta C, Anghileri E, Di Ianni N, Milani M, Cuccarini V, et al. Expansion of effector and memory T cells is associated with increased survival in recurrent glioblastomas treated with dendritic cell immunotherapy. Neurooncol Adv (2019) 1(1):vdz022. doi: 10.1093/noajnl/vdz022
131. De Vleeschouwer S, Fieuws S, Rutkowski S, Van Calenbergh F, Van Loon J, Goffin J, et al. Postoperative adjuvant dendritic cell-based immunotherapy in patients with relapsed glioblastoma multiforme. Clin Cancer Res (2008) 14(10):3098–104. doi: 10.1158/1078-0432.CCR-07-4875
132. Mitchell DA, Sayour EJ, Reap E, Schmittling R, DeLeon G, Norberg P, et al. Severe adverse immunologic reaction in a patient with glioblastoma receiving autologous dendritic cell vaccines combined with GM-CSF and dose-intensified temozolomide. Cancer Immunol Res (2015) 3(4):320–5. doi: 10.1158/2326-6066.CIR-14-0100
133. Yamanaka R, Abe T, Yajima N, Tsuchiya N, Homma J, Kobayashi T, et al. Vaccination of recurrent glioma patients with tumour lysate-pulsed dendritic cells elicits immune responses: results of a clinical phase I/II trial. Br J Cancer (2003) 89(7):1172–9. doi: 10.1038/sj.bjc.6601268
134. Kikuchi T, Akasaki Y, Abe T, Fukuda T, Saotome H, Ryan JL, et al. Vaccination of glioma patients with fusions of dendritic and glioma cells and recombinant human interleukin 12. J Immunother (2004) 27(6):452–9. doi: 10.1097/00002371-200411000-00005
135. Akasaki Y, Kikuchi T, Homma S, Koido S, Ohkusa T, Tasaki T, et al. Phase I/II trial of combination of temozolomide chemotherapy and immunotherapy with fusions of dendritic and glioma cells in patients with glioblastoma. Cancer Immunol Immunother (2016) 65(12):1499–509. doi: 10.1007/s00262-016-1905-7
136. Iwami K, Shimato S, Ohno M, Okada H, Nakahara N, Sato Y, et al. Peptide-pulsed dendritic cell vaccination targeting interleukin-13 receptor α2 chain in recurrent Malignant glioma patients with HLA-A*24/A*02 allele. Cytotherapy (2012) 14(6):733–42. doi: 10.3109/14653249.2012.666633
137. Sakai K, Shimodaira S, Maejima S, Udagawa N, Sano K, Higuchi Y, et al. Dendritic cell-based immunotherapy targeting Wilms’ tumor 1 in patients with recurrent Malignant glioma. J Neurosurg (2015) 123(4):989–97. doi: 10.3171/2015.1.JNS141554
138. Liau LM, Prins RM, Kiertscher SM, Odesa SK, Kremen TJ, Giovannone AJ, et al. Dendritic cell vaccination in glioblastoma patients induces systemic and intracranial T-cell responses modulated by the local central nervous system tumor microenvironment. Clin Cancer Res (2005) 11(15):5515–25. doi: 10.1158/1078-0432.CCR-05-0464
139. Khan JA, Yaqin S. Dendritic cell therapy with improved outcome in glioma multiforme–a case report. J Zhejiang Univ Sci B (2006) 7(2):114–7. doi: 10.1631/jzus.2006.B0114
140. Prins RM, Wang X, Soto H, Young E, Lisiero DN, Fong B, et al. Comparison of glioma-associated antigen peptide-loaded versus autologous tumor lysate-loaded dendritic cell vaccination in Malignant glioma patients. J Immunother (2013) 36(2):152–7. doi: 10.1097/CJI.0b013e3182811ae4
141. Okada H, Lieberman FS, Walter KA, Lunsford LD, Kondziolka DS, Bejjani GK, et al. Autologous glioma cell vaccine admixed with interleukin-4 gene transfected fibroblasts in the treatment of patients with Malignant gliomas. J Transl Med (2007) 5:67. doi: 10.1186/1479-5876-5-67
142. Ardon H, De Vleeschouwer S, Van Calenbergh F, Claes L, Kramm CM, Rutkowski S, et al. Adjuvant dendritic cell-based tumour vaccination for children with Malignant brain tumours. Pediatr Blood Cancer (2010) 54(4):519–25. doi: 10.1002/pbc.22319
143. Yu JS, Liu G, Ying H, Yong WH, Black KL, Wheeler CJ. Vaccination with tumor lysate-pulsed dendritic cells elicits antigen-specific, cytotoxic T-cells in patients with Malignant glioma. Cancer Res (2004) 64(14):4973–9. doi: 10.1158/0008-5472.CAN-03-3505
144. Chan TA, Yarchoan M, Jaffee E, Swanton C, Quezada SA, Stenzinger A, et al. Development of tumor mutation burden as an immunotherapy biomarker: utility for the oncology clinic. Ann Oncol (2019) 30(1):44–56. doi: 10.1093/annonc/mdy495
145. Fecci PE, Sweeney AE, Grossi PM, Nair SK, Learn CA, Mitchell DA, et al. Systemic anti-CD25 monoclonal antibody administration safely enhances immunity in murine glioma without eliminating regulatory T cells. Clin Cancer Res (2006) 12(14 Pt 1):4294–305. doi: 10.1158/1078-0432.CCR-06-0053
146. Grauer OM, Nierkens S, Bennink E, Toonen LW, Boon L, Wesseling P, et al. CD4+FoxP3+ regulatory T cells gradually accumulate in gliomas during tumor growth and efficiently suppress antiglioma immune responses. vivo. Int J Cancer (2007) 121(1):95–105. doi: 10.1002/ijc.22607
147. Mitchell DA, Cui X, Schmittling RJ, Sanchez-Perez L, Snyder DJ, Congdon KL, et al. Monoclonal antibody blockade of IL-2 receptor α during lymphopenia selectively depletes regulatory T cells in mice and humans. Blood (2011) 118(11):3003–12. doi: 10.1182/blood-2011-02-334565
148. Wainwright DA, Chang AL, Dey M, Balyasnikova IV, Kim CK, Tobias A, et al. Durable therapeutic efficacy utilizing combinatorial blockade against IDO, CTLA-4, and PD-L1 in mice with brain tumors. Clin Cancer Res (2014) 20(20):5290–301. doi: 10.1158/1078-0432.CCR-14-0514
149. Li J, Liu X, Duan Y, Liu Y, Wang H, Lian S, et al. Combined blockade of T cell immunoglobulin and mucin domain 3 and carcinoembryonic antigen-related cell adhesion molecule 1 results in durable therapeutic efficacy in mice with intracranial gliomas. Med Sci Monit (2017) 23:3593–602. doi: 10.12659/msm.903098
150. Banissi C, Ghiringhelli F, Chen L, Carpentier AF. Treg depletion with a low-dose metronomic temozolomide regimen in a rat glioma model. Cancer Immunol Immunother (2009) 58(10):1627–34. doi: 10.1007/s00262-009-0671-1
151. Riva M, Wouters R, Nittner D, Ceusters J, Sterpin E, Giovannoni R, et al. Radiation dose-escalation and dose-fractionation modulate the immune microenvironment, cancer stem cells and vasculature in experimental high-grade gliomas. J Neurosurg Sci (2023) 67(1):55–65. doi: 10.23736/S0390-5616.20.05060-2
152. Kesarwani P, Prabhu A, Kant S, Kumar P, Graham SF, Buelow KL, et al. Tryptophan metabolism contributes to radiation-induced immune checkpoint reactivation in glioblastoma. Clin Cancer Res (2018) 24(15):3632–43. doi: 10.1158/1078-0432.CCR-18-0041
153. Maes W, Rosas GG, Verbinnen B, Boon L, De Vleeschouwer S, Ceuppens JL, et al. DC vaccination with anti-CD25 treatment leads to long-term immunity against experimental glioma. Neuro Oncol (2009) 11(5):529–42. doi: 10.1215/15228517-2009-004
154. Michels T, Shurin GV, Naiditch H, Sevko A, Umansky V, Shurin MR. Paclitaxel promotes differentiation of myeloid-derived suppressor cells into dendritic cells in vitro in a TLR4-independent manner. J Immunotoxicol (2012) 9(3):292–300. doi: 10.3109/1547691X.2011.642418
155. Kodumudi KN, Woan K, Gilvary DL, Sahakian E, Wei S, Djeu JY. A novel chemoimmunomodulating property of docetaxel: suppression of myeloid-derived suppressor cells in tumor bearers. Clin Cancer Res (2010) 16(18):4583–94. doi: 10.1158/1078-0432.CCR-10-0733
156. Mirza N, Fishman M, Fricke I, Dunn M, Neuger AM, Frost TJ, et al. All-trans-retinoic acid improves differentiation of myeloid cells and immune response in cancer patients. Cancer Res (2006) 66(18):9299–307. doi: 10.1158/0008-5472.CAN-06-1690
157. Otvos B, Silver DJ, Mulkearns-Hubert EE, Alvarado AG, Turaga SM, Sorensen MD, et al. Cancer stem cell-secreted macrophage migration inhibitory factor stimulates myeloid derived suppressor cell function and facilitates glioblastoma immune evasion. Stem Cells (2016) 34(8):2026–39. doi: 10.1002/stem.2393
158. Peereboom DM, Alban TJ, Grabowski MM, Alvarado AG, Otvos B, Bayik D, et al. Metronomic capecitabine as an immune modulator in glioblastoma patients reduces myeloid-derived suppressor cells. JCI Insight (2019) 4(22):130748. doi: 10.1172/jci.insight.130748
159. Cannarile MA, Weisser M, Jacob W, Jegg AM, Ries CH, Rüttinger D. Colony-stimulating factor 1 receptor (CSF1R) inhibitors in cancer therapy. J Immunother Cancer (2017) 5(1):53. doi: 10.1186/s40425-017-0257-y
160. Abad C, Nobuta H, Li J, Kasai A, Yong WH, Waschek JA. Targeted STAT3 disruption in myeloid cells alters immunosuppressor cell abundance in a murine model of spontaneous medulloblastoma. J Leukoc Biol (2014) 95(2):357–67. doi: 10.1189/jlb.1012531
161. Pyonteck SM, Akkari L, Schuhmacher AJ, Bowman RL, Sevenich L, Quail DF, et al. CSF-1R inhibition alters macrophage polarization and blocks glioma progression. Nat Med (2013) 19(10):1264–72. doi: 10.1038/nm.3337
162. Stanley ER, Chitu V. CSF-1 receptor signaling in myeloid cells. Cold Spring Harb Perspect Biol (2014) 6(6):a021857. doi: 10.1101/cshperspect.a021857
163. Macanas-Pirard P, Quezada T, Navarrete L, Broekhuizen R, Leisewitz A, Nervi B, et al. The CCL2/CCR2 axis affects transmigration and proliferation but not resistance to chemotherapy of acute myeloid leukemia cells. PloS One (2017) 12(1):e0168888. doi: 10.1371/journal.pone.0168888
164. Zhu Y, Herndon JM, Sojka DK, Kim KW, Knolhoff BL, Zuo C, et al. Tissue-resident macrophages in pancreatic ductal adenocarcinoma originate from embryonic hematopoiesis and promote tumor progression. Immunity (2017) 47(2):323–338.e6. doi: 10.1016/j.immuni.2017.07.014
165. Willingham SB, Volkmer JP, Gentles AJ, Sahoo D, Dalerba P, Mitra SS, et al. The CD47-signal regulatory protein alpha (SIRPa) interaction is a therapeutic target for human solid tumors. Proc Natl Acad Sci USA (2012) 109(17):6662–7. doi: 10.1073/pnas.1121623109
166. Saha D, Martuza RL, Rabkin SD. Macrophage polarization contributes to glioblastoma eradication by combination immunovirotherapy and immune checkpoint blockade. Cancer Cell (2017) 32(2):253–267.e5. doi: 10.1016/j.ccell.2017.07.006
167. van den Bossche WBL, Kleijn A, Teunissen CE, Voerman JSA, Teodosio C, Noske DP, et al. Oncolytic virotherapy in glioblastoma patients induces a tumor macrophage phenotypic shift leading to an altered glioblastoma microenvironment. Neuro Oncol (2018) 20(11):1494–504. doi: 10.1093/neuonc/noy082
168. Fecci PE, Ochiai H, Mitchell DA, Grossi PM, Sweeney AE, Archer GE, et al. Systemic CTLA-4 blockade ameliorates glioma-induced changes to the CD4+ T cell compartment without affecting regulatory T-cell function. Clin Cancer Res (2007) 13(7):2158–67. doi: 10.1158/1078-0432.CCR-06-2070
169. Reardon DA, Brandes AA, Omuro A, Mulholland P, Lim M, Wick A, et al. Effect of nivolumab vs bevacizumab in patients with recurrent glioblastoma: the checkMate 143 phase 3 randomized clinical trial. JAMA Oncol (2020) 6(7):1003–10. doi: 10.1001/jamaoncol.2020.1024
170. Pellegatta S, Poliani PL, Stucchi E, Corno D, Colombo CA, Orzan F, et al. Intra-tumoral dendritic cells increase efficacy of peripheral vaccination by modulation of glioma microenvironment. Neuro Oncol (2010) 12(4):377–88. doi: 10.1093/neuonc/nop024
171. Masson F, Calzascia T, Di Berardino-Besson W, de Tribolet N, Dietrich PY, Walker PR. Brain microenvironment promotes the final functional maturation of tumor-specific effector CD8+ T cells. J Immunol (2007) 179(2):845–53. doi: 10.4049/jimmunol.179.2.845
172. Verheye E, Bravo Melgar J, Deschoemaeker S, Raes G, Maes A, De Bruyne E, et al. Dendritic cell-based immunotherapy in multiple myeloma: challenges, opportunities, and future directions. Int J Mol Sci (2022) 23(2):904. doi: 10.3390/ijms23020904
Keywords: dendritic cell vaccine, glioma microenvironment, immunotherapy, glioma, dendritic cell
Citation: Zheng Y, Ma X, Feng S, Zhu H, Chen X, Yu X, Shu K and Zhang S (2023) Dendritic cell vaccine of gliomas: challenges from bench to bed. Front. Immunol. 14:1259562. doi: 10.3389/fimmu.2023.1259562
Received: 16 July 2023; Accepted: 28 August 2023;
Published: 14 September 2023.
Edited by:
Yunlang She, Tongji University, ChinaReviewed by:
Quan Cheng, Central South University, ChinaWenchao Zhou, University of Science and Technology of China, China
Weiwei Tao, Huazhong Agricultural University, China
Copyright © 2023 Zheng, Ma, Feng, Zhu, Chen, Yu, Shu and Zhang. This is an open-access article distributed under the terms of the Creative Commons Attribution License (CC BY). The use, distribution or reproduction in other forums is permitted, provided the original author(s) and the copyright owner(s) are credited and that the original publication in this journal is cited, in accordance with accepted academic practice. No use, distribution or reproduction is permitted which does not comply with these terms.
*Correspondence: Suojun Zhang, zhangsuojun@tjh.tjmu.edu.cn