- 1Department of Applied Biology, Institute of Environmentally Friendly Agriculture (IEFA), College of Agriculture and Life Sciences, Chonnam National University, Gwangju, Republic of Korea
- 2Life & Medical Sciences Institute (LIMES) Development, Genetics & Molecular Physiology Unit, University of Bonn, Bonn, Germany
The gut is a crucial organ in insect defense against various pathogens and harmful substances in their environment and diet. Distinct insect gut compartments possess unique functionalities contributing to their physiological processes, including immunity. The insect gut’s cellular composition is vital for cellular and humoral immunity. The peritrophic membrane, mucus layer, lumen, microvilli, and various gut cells provide essential support for activating and regulating immune defense mechanisms. These components also secrete molecules and enzymes that are imperative in physiological activities. Additionally, the gut microbiota initiates various signaling pathways and produces vitamins and minerals that help maintain gut homeostasis. Distinct immune signaling pathways are activated within the gut when insects ingest pathogens or hazardous materials. The pathway induced depends on the infection or pathogen type; include immune deficiency (imd), Toll, JAK/STAT, Duox-ROS, and JNK/FOXO regulatory pathways. These pathways produce different antimicrobial peptides (AMPs) and maintain gut homeostasis. Furthermore, various signaling mechanisms within gut cells regulate insect gut recovery following infection. Although some questions regarding insect gut immunity in different species require additional study, this review provides insights into the insect gut’s structure and composition, commensal microorganism roles in Drosophila melanogaster and Tenebrio molitor life cycles, different signaling pathways involved in gut immune systems, and the insect gut post-infection recovery through various signaling mechanisms.
1 Introduction
Food is vital for life. To thrive in their natural environment and ensure survival, insects must obtain nourishment through various ways such as adaptations, physiological processes, and reproductive activities. Consequently, insects are compelled to gather food from diverse environmental outlets that often harbor harmful microorganisms that can invade the insect’s digestive system. Therefore, insect digestive systems must employ expansive defense mechanisms to counteract infection risks or potential harm (1).
The insect gut composition is crucial for orchestrating defense mechanisms. The peritrophic membrane, comprising chitin and protein, collaborates with glycosylated protein-based mucus, intestinal epithelial cells, and intestinal stem cells to establish a robust physical shield against invading pathogenic microorganisms (2). The peritrophic membrane is the foremost defense and a formidable barrier that safeguards the midgut epithelium from abrasive food particles and lethal pathogen incursions (3). The mucosal layer, enriched with mucins, concurrently lubricates the luminal surface and fortifies the epithelium, impeding mechanical damage, pathogens, and toxic molecules (4). Given an insect’s diverse dietary repertoire of decaying plants, fungal matter, and pathogenic bacteria, these organisms have evolved an impressive digestive enzyme arsenal for efficient carbohydrate, protein, and lipid processing for complex food sources. Intricate intestinal pH regulation is also pivotal in the initial food digestion stages, with a pH of 5 in the foregut, 7 to 9 in the midgut, and 5 in the hindgut. However, these pH fluctuations physically and chemically affect ingested food material, influencing its overall integrity (1).
In the face of pathogen attacks on host gut epithelial cells are tackled with a pathogen outbreak, cascades of immune responses are triggered, involving the activation of key immune genes. Notably, pattern recognition receptors (PRRs) and antimicrobial peptides (AMPs) are among the immune factors that spring into action. The immune deficiency pathway, orchestrated by the Nuclear Factor-kappaB (NF-kB) transcription factors REL2 and REL2-F, is stimulated, bolstering the host’s defense mechanisms (5). Furthermore, the Dualoxidase (Duox) pathway works diligently as another line of defense to maintain gut stability by safeguarding gut mucosa against reactive oxygen species (ROS)-sensitive pathogens (6). Moreover, the intricate protein interplay, including peptidoglycan receptor proteins (PGRPs), serine proteases, serpins, and a range of other molecules, reinforces the immune bolstering process through melanization within the prophenoloxidase (PPO) pathway, which varies in insect species (7). Additionally, the Janus kinase (JAK/STAT) signaling pathway, responsible for regulating cell growth, differentiation, apoptosis, and inflammatory reactions, triggers diverse antimicrobial peptide production, thereby instigating potent intestinal immunity in insects (8).
Various microorganism types have established symbiotic relationships with their hosts throughout evolution, providing extensive support. These microorganisms, commonly known as “commensal/microbiota,” are integral for enhancing host fecundity, development, and growth. Specifically, they contribute to food digestion, vitamin production, host gut defense against pathogens, harmful substance detoxification, and stimulating host immune responses (9). Gut microbiota has garnered recognition as a pivotal component for biological control and waste biodegradation while deterring insect-borne disease transmission (9). Furthermore, the gut microbiota provides significant functionalities in synthesizing proteins, catecholamine cross-linkers, and chitin, inducing various immune regulatory pathways to aid recovery from infection (10). The gut microbiota supports host defense by modulating pH and digestive enzyme levels. They actively compete with pathogens for resources, such as living space and nutrients, while producing antimicrobial substances (11).
This review highlights contemporary advancements in understanding the insect gut’s immune mechanisms when combating pathogenic threats. Specifically, we delve into the physical, biochemical, and intricate gut immune responses observed in model organisms, such as D. melanogaster and T. molitor, and further insight into post-infection recovery processes.
2 Insect gut structure and function
2.1 Insect gut compartments
The insect intestinal tract maintains a compartmentalized organization comprising three distinct sections: the foregut (stomodeum), midgut (mesenteron), and hindgut (proctodeum) (Figure 1). The foregut encompasses anatomical components, including the mouth, pharynx, esophagus, crop, and proventriculus. The midgut’s peritrophic matrix and mucins compose a formidable physical barrier, impeding pathogen intrusion. Lastly, the hindgut is essential for nutrient absorption and selective superfluous material exclusion (13).
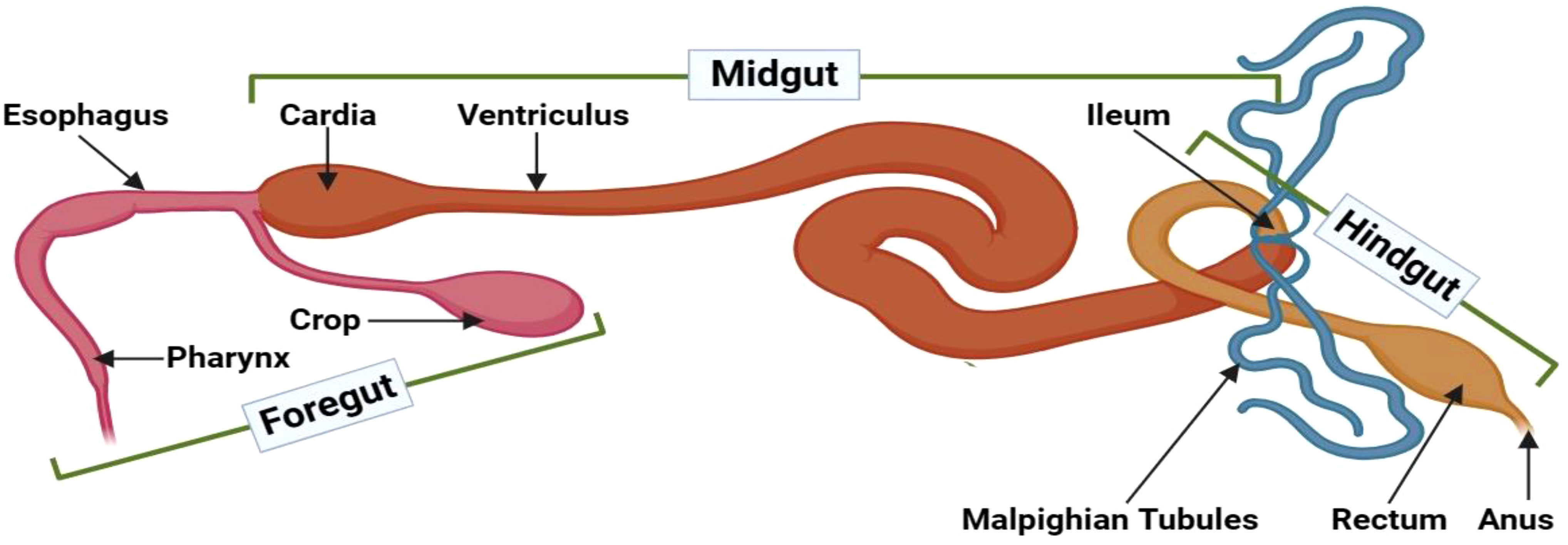
Figure 1 Gut compartments of adult D. melanogaster. The insect intestinal tract can be anatomically divided into three distinct compartments: the foregut, midgut, and hindgut. The foregut encompasses vital structures, such as the mouth, pharynx, esophagus, crop, and proventriculus, with robust cuticles that form a protective barrier against invading pathogens. Moreover, the foregut facilitates enzymatic compound secretions from the buccal cavity and salivary glands. The midgut begins at the gastric caeca and segments into anterior, median, and posterior regions, determined by their respective lengths. This section is notable for its relatively high permeability. The midgut’s epithelial lining incorporates diverse cell types with crucial functions, including nutrient digestion and absorption, pH regulation, endocrine modulation, epithelial growth, and safeguarding against pathogenic threats. Lastly, the hindgut starts where the Malpighian tubules and the muscular pyloric sphincter connect, followed by the ileum, colon, and rectum. Distinctive physiological processes in this compartment primarily focus on reabsorbing water, electrolytes, and other beneficial substances from the excreta. This illustration has been adopted from T. H. Napoleao et al (12).
2.1.1 Foregut
The foregut represents the insect gut threshold, featuring an impermeable cuticle that imparts mechanical and chemical digestion properties facilitated by enzymes secreted from the buccal cavity. This section comprises salivary glands, reservoirs, and stomodeal valves that direct food flow toward the midgut (12). The crop is also integral to this region as it provides initial food storage, while the proventriculus acts as a valve that modifies ingested foodstuff (14). Certain insect species, such as Carpenter bees, symbiotically associate with Lactobacillus and Enterobacteriaceae bacteria predominantly located within the foregut. These microorganisms give support the bees’ digestive processes, nutrient delivery, pathogen defense, and immune signaling mechanisms (15). Moreover, the foregut in Bulbitermes spp is a site for xenobiotic degradation and initial metabolic processes (16). In Anopheles culicifacies, the foregut’s salivary gland harbors a more diverse symbiotic microorganism array than the midgut and hindgut, responsible for food acquisition, ingestion, and digestion (17). Research on Cephalotes rohweri indicates that the proventriculus valve, positioned between the crop and midgut, filters and effectively prevents pathogenic bacteria and particles larger than 0.2 µm from entering, protecting the host and maintaining host-microbe fidelity (18). Nutrient uptake and digestion are vital physiological processes across various life forms, and the gut-brain axis ensures gut homeostasis by regulating these processes through a neurohumoral communication system. Different neuropeptide classes govern the release of digestive enzymes, muscle activity, and engorgement, with specific neuropeptides presenting dynamic contributions to ecological pest management (19). Notably, Drosophila spp.’s gastric valve and crop function analogously to the stomach. A study investigating Drosophila stomach stem cells unveiled that JAK-STAT signaling regulates stem cell proliferation, Wingless signaling controls self-renewal, and hedgehog signaling governs cellular differentiation (20).
2.1.2 Midgut
The midgut is a crucial component of the insect’s digestive tract that starts from the gastric caeca and serves as a primary site for digestion and nutrient absorption (12). Structurally, the midgut is divided into anterior, median, and posterior regions based on length (21). Comprising a diverse cell array, the midgut epithelium digests and absorbs nutrients and maintains luminal pH levels, endocrine regulation, and epithelial growth in this middle region (22). Within specific regions of this organ, messenger RNA expression governs enzyme secretion tailored for breaking down specific food components, such as proteins, carbohydrates, and lipids. These enzymes, along with the luminal pH, significantly contribute to eradicating pathogenic microorganisms that are ingested with food (22). Moreover, the midgut epithelium employs various secretion methods, including merocrine, apocrine, and micro-apocrine mechanisms. Diverse symbiotic microorganisms reside in the anterior midgut’s endoperitrophic space, assisting for digestion, and prompting immune responses (21). Regarding Drosophila, the midgut encompasses 14 distinct subregions that manifest distinct morphological, histological, and genetic properties. Damage to this compartment disrupts gut homeostasis, triggers stem cell proliferation, and stimulates various immune signaling pathways (23). When Nasutitermes takasagoensis digests cellulose, both endogenous endo-b-1,4-glucanase and b-glucosidase genes are uniformly expressed in the midgut, ensuring consistent enzyme production (24). In Locusta migratoria, the lethal giant larvae (Lgl) protein facilitates midgut morphology maintenance and ds-RNA-based insecticide development (25). Throughout Drosophila spp.’s lifespan, numerous regulatory signaling pathways operate within the midgut: the Delta/Notch signaling pathway regulates midgut progenitor cell differentiation, the Wingless and Int-1 (WNT) signaling pathway is responsible for intestinal stem cell (ISC) proliferation and maintenance, the Cytokine/Jak/Stat and Epidermal Growth Factor Receptor (EGFR) signaling pathways govern ISC proliferation, the JNK signaling pathway coordinates stress response and ISC proliferation; the Hippo/Salvador/Warts signaling pathway regulates midgut regeneration; and the cellular redox state influences ISC proliferation (26).
2.1.3 Hindgut
The insect hindgut is structurally divided into the ileum, colon, and rectum, beginning at the pyloric valve after the midgut. Malpighian tubules in this region establish the excretory organs responsible for removing nitrogenous waste as uric acid from the hemolymph. This gut section is entirely covered by epithelial cells, with a muscular layer surrounding the basal membrane. The hindgut sustains greater permeability than the foregut, primarily absorbs nutrients and minerals, and eliminates feces (12). Additionally, the hindgut facilitates salt and amino acid reabsorption to maintain the hemolymph’s osmotic balance (27). Prophenoloxidase is a notable melanin production enzyme and serves as an immune response element at wound sites, functioning as the last line of defense against pathogens (26). The hindgut also harbors enteric bacteria, such as Pantoea agglomerans, which induce diverse immune responses (14).
2.2 The insect gut’s general cellular composition
The insect intestinal system comprises a myriad of cell types, including absorptive enterocytes, secretory enteroendocrine cells, and pluripotent intestinal stem cells. Additionally, muscle cells are situated beneath epithelial cells’ basement membranes (Figure 2). A semipermeable non-cellular structure, known as the peritrophic matrix/membrane, is positioned between the lumen and the epithelial layer within the insect gut, protecting enterocytes against abrasive particles and pathogens. Furthermore, a mucus layer between the peritrophic matrix and the enterocytes extends throughout the gut (28). Neurons and trachea are intricately integrated into the muscle layer beneath intestinal cells’ basement membrane, aiding the insect intestinal system’s overall functionality and coordination (1).
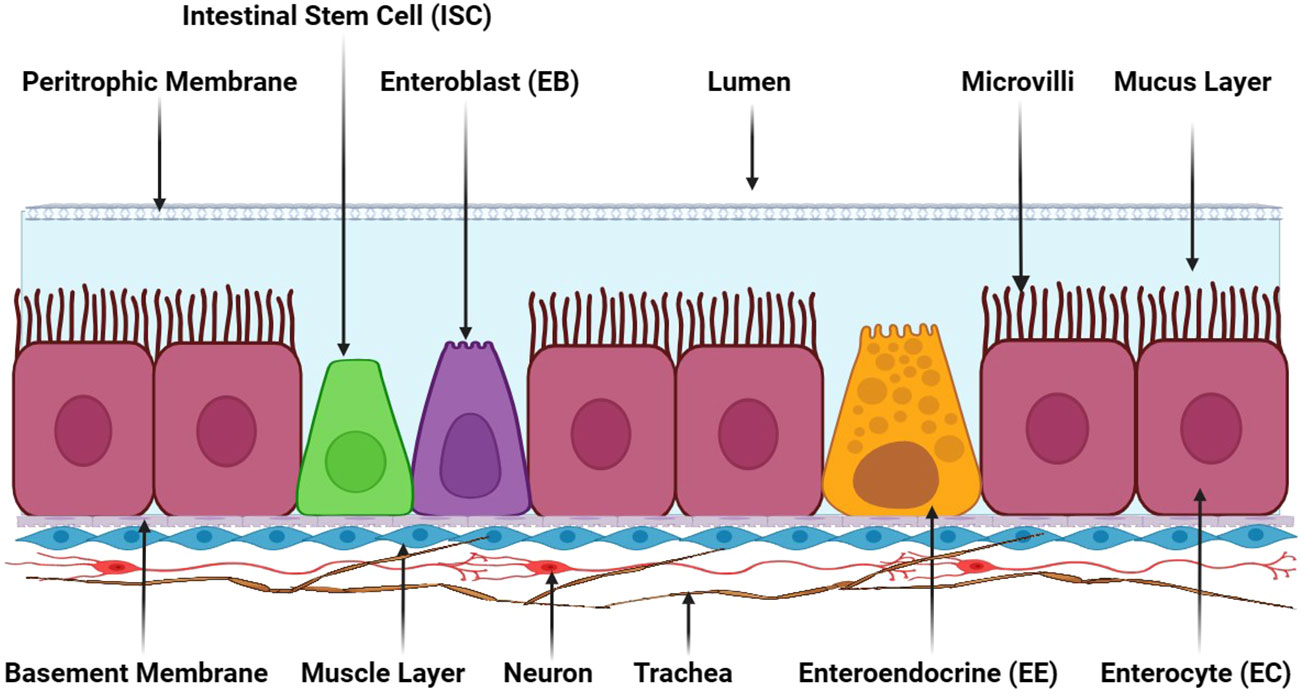
Figure 2 The adult insect gut’s general cellular composition. Adult insect gastrointestinal tracts comprise various cell types, namely absorptive enterocytes (ECs), secretory enteroendocrine cells (EEs), pluripotent intestinal stem cells (ISCs), and enteroblasts (EBs). These cells are collectively essential for an insect’s digestive process. The peritrophic membrane situated between the lumen and epithelial cells is a semipermeable non-cellular structure that serves as a protective barrier, shielding enterocytes from abrasive particles and invading pathogens. Adjacent to the peritrophic membrane lies a mucus layer that further engenders a protective environment for enterocyte cells. Beneath epithelial cells’ basement membranes is a muscle cell layer responsible for mechanical movements and contractions in the gut. Positioned underneath the muscle layer, neurons and trachea are present, providing innervation and oxygen supply to the gastrointestinal tract. This illustration has been adopted from T. Kuraishi et al (28).
2.2.1 Peritrophic membrane
The peritrophic membrane (PM) serves as a protective lining within the insect gut that separates the digestive compartment from the midgut lumen. Its primary functions include luminal surface lubrication to safeguard against food abrasion and pathogen invasion, as well as regulating immune responses. This membrane is composed of chitin fibers cross-linked by chitin-binding PM proteins (PMP); the PM forms a three-dimensional meshwork with water-filled pores. The mucin-like PMPs contain extensively O-glycosylated linker and chitin-binding domains (ChtBD2). In particular, these O-glycans are crucial for lubrication and controlling exclusion (29). Chitin and glycoproteins form the intestinal PM, which activates various regulatory pathways to defend against pathogens and prevent gut tissue damage caused by pore-forming toxins (3). For example, the peritrophic membrane in mosquitos is a vital gut homeostasis regulator and immune response mediator (30). Furthermore, Heme-dependent peroxidase 1 (HPx1) promotes PM assembly and enhances antioxidant capacity while modulating vector competence. The E75 transcription factor induces transcriptional HPx1 activation, and HPx1 knockdown accelerates DUOX-NADPH oxidase’s gut reactive oxygen species (ROS) production, thereby enhancing the immunological sensing of microbial infection (31). The peritrophic membrane of insects, exposed mucins and non-mucin protein receptors, functions as a binding site for various insect pathogens, such as Junonia coenia densovirus (JcDV) (32). Different protein classes within the insect PM exhibit distinct activities: Class I PM proteins contribute to PM integrity and function; Class II proteins faintly interact with the PM through adsorption; Class III proteins are released under harsher conditions; and Class IV proteins are covalently linked to other PM proteins or chitin, making their removal challenging (33). Seventeen peritrophic membrane proteins have been identified in the Manduca sexta midgut, and genes encoding peritrophic matrix proteins in Tribolium castaneum are directly associated with insect survival (34).
2.2.2 Mucus layer and mucin
Mucus production is mediated by mucus-forming mucins (Mf-mucins). These proteins are glycosylated and form a protective layer on epithelial cells that line the respiratory, digestive, and urogenital tracts in vertebrates. Their primary function is to protect the organ cell lining against infections, dehydration, physical or chemical damage, and other threats while facilitating nutrient flow through the intestinal tract. In particular, studies have evidenced that the mucus layer is involved in Mf-mucins activation (35). While the mucus layer’s composition varies by organism, certain molecules remain consistent, particularly proteins extensively modified with N-acetylgalactosamine-based sugars attached to serines or threonines through O-linked glycosylation. Mucin proteins and their constituent O-glycans are fundamental for protecting and maintaining internal epithelial tissue vitality (36). In female insects, mucin proteins are localized in swollen crypts and are associated with symbiotic bacteria that stimulate gut immune responses (37).
2.2.3 Microvilli
Microvilli form the inner intestinal cell surface and are intricately linked to the mucus layer. Microvilli contains jelly-associated enzymes composed of membrane-bound material. These enzymes are either bound to the cell membrane or entrapped within the glycocalyx and predicate microvilli formation and structure. The glycocalyx refers to the carbohydrate chain connected to integral proteins and glycolipids on the microvilli. Digestion primarily transpires on the microvilli surface, and insights into microvillar enzymology are invaluable for proteomics studies (38). Moreover, digestive enzymes are synthesized in the rough endoplasmic reticulum, processed in the Golgi complex, and packaged into secretory vesicles. Gut microvillar membranes’ physiological operations vary among insect taxa, influencing digestion, absorption, ion homeostasis, signaling, and secretory mechanisms. For instance, hemipteran gut cells manage various secretion types, including exocytic, apocrine, micro-apocrine with budding or pinched-off vesicles, and modified exocytic secretions (39).
2.2.4 Insect gut cells
Enterocytes (ECs), enteroendocrine cells (EEs), ISCs, and enteroblasts (EBs) are the key cellular components involved in regulating the various activities and immune responses within the insect gut. Enterocytes primarily participate in absorption, whereas enteroendocrine cells secrete a diverse array of secretory molecules. ISCs are crucial for intestinal epithelium regeneration influenced by intrinsic factors in addition local and systemic stimuli (40). ISCs continuously generate progenitor cells that differentiate into multiple cell types within the epithelium, thereby maintaining homeostasis (41). Enteroblasts are transient progenitors that differentiate into ECs (42).
3 Insect gut microbiota
3.1 Gut microbiota in D. melanogaster
An insect encounters diverse environmental conditions and various foodstuffs harboring assorted microorganisms throughout its life. While most of these microorganisms pass through the insect gut’s lumen or interact with the host immune system, certain species are permitted to establish and proliferate within the gut environment called “commensal”. These commensal microbes provide metabolic assistance, secondary metabolite production, immune system support, pathogen clearance, gut homeostasis maintenance, intestinal tissue regeneration, and other valuable contributions to the host (43–45). In Drosophila, the gut microbiota encompasses 5-20 commensal bacteria species, mainly from the Acetobacteraceae and Enterobacteriaceae families or the Lactobacillale order, including Lactobacillus plantarum, L. brevis, Acetobacter pomorum, Gluconobacter morbifer, and Commensalibacter intestine (45). Although gut microbiota compositions vary due to fluctuations in environmental conditions and food availability, numerous studies have consistently identified the following dominant bacterial families in the Drosophila’s gut: Acetobacteraceae (55%), Lactobacillaceae (31%), Leuconostocaceae (4%), Enterobacteriaceae (3%), and Enterococceae (2%) (46). Furthermore, ingesting microorganisms, such as Rhodiola crenulate, enhances survival rates and induces antimicrobial peptide gene expressions in D. melanogaster following exposure to pathogens or toxic compounds (47).
Multiple studies have revealed that the gut microbiota influences host immune responses and various physiological activities that include larvae development, nutrient uptake, behavior, and environmental signal responses (48). The gut microbiota produces distinct metabolites sensed by host intestinal receptors, thereby triggering host metabolism (49). Interactions among microbes can influence triglyceride content, highlighting the microbiota’s influence on host metabolic processes (50). Notably, Acetobacter persici in the Drosophila gut prompts age-dependent metabolic purine level shifts by activating the innate immune signaling imd pathway in renal tubules (51). Furthermore, selective consumption of dietary components mediated by microbiota can modify the nutritional balance of food and ultimately impact the nutritional status of the host (52). Microbiota-induced signaling and regulatory networks involving genes associated with nutritional traits contribute to the host’s nutritional status (53). A substantial larvae accumulation in a specific area can alter food’s chemical and bacterial composition, effectuating growth time, pupation height, viability, and body mass alterations without affecting gut microbiota (54). The gut microbial community’s composition in insects depends on host-specific factors, with colonization transpiring in specific gut regions (55). Additionally, food sources can influence a microbiome’s establishment by reducing predominant member abundance and allowing new microorganisms to colonize (56). Introducing a single microorganism to the D. melanogaster gut can alter the microbial community’s symbiotic composition without exhibiting extensive antibacterial activity, potentially influenced by food sources. For example, Rhodiola rosea significantly increases Acetobacter abundance while diminishing Lactobacillales, engendering a symbiotic association (57).
Drosophila spp. exhibits bidirectional signaling through the “microbiota-gut-vagus-brain axis,” where gut microbiota can activate host neural circuitry and influence foraging behavior, stress-anxiety responses, and empathy development (58). During undernutrition, specific microbiomes, such as L. plantarum, induce the insulin/IGF-1 signaling (IIS) pathway, regulate glucose import and glycogen synthesis, and increase free amino acid levels by promoting gut peptidase expression via the imd pathway. A genetic L. plantarum screening identified an operon responsible for modifying bacterial cell walls, incorporating D-alanylated teichoic acids, which stimulate host enterocytes to induce gut peptidases (59). The gut microbiota, including Acetobacter spp. and yeast, also modifies host chemosensory responses, feeding preferences, and behavior, depending on the host-microbial history and identity. Different olfactory receptors and pathways are involved in sensing and responding to these changes, known as Olfactory-Guided Microbial Preferences by the host (60).
In addition, the Drosophila gut microbiota manages learning, memory, and sleep homeostasis aspects dependent on the host’s genetic background (61). Aggressive behaviors in both male and female Drosophila can be regulated by the microbiome, swaying mate selection during development (62). Interactions between Drosophila and its gut microbiome are foundations for developing new therapeutic drugs and understanding downstream consequences for host health through genome analysis. Notably, these findings have also extended to human precision medicine (63). Various factors influence the interplay between microbiota and their host concerning immune induction. The immune response in D. melanogaster incorporates both humoral and cellular components, including the production of antimicrobial peptides, ROS, reactive chlorine species (RCS), phagocytic cells, and melanization. The gut microbiota directly or indirectly aids in activating the immune response (64, 65). Among prevalent D. melanogaster microbiota, Lactobacilli spp. activate the NADPH Oxidases (NOX) pathway in gut tissue, releasing ROS and reducing microbiota overgrowth. Conversely, pathogenic bacteria stimulate ROS and RCS production through the dual oxidase system (Duox), which helps maintain host gut homeostasis (64, 65).
Microbiota and pathogens trigger the humoral immune response, inducing the production of antimicrobial peptides through imd and Toll regulatory pathways. These processes are initiated by PRRs in response to conserved microbial-associated molecular patterns (MAMPs) (64, 65). PGRPs, such as PGRP-LC, PGRP-LE, PGRP-SD, PGRP-LB, and PGRP-SC, within the IMD signaling cascade also recognize peptidoglycans from the microbiota. Notably, PGRP-LB has been found to protect the host from the harmful peptidoglycan effects on the hemolymph and other organ systems (64, 65). The EGFR and JAK-STAT signaling pathways are responsible for gut repair prompted by gut microbiota and pathogens, and the pathway activated depends on the damage sustained. Additionally, histone demethylase KDM5 deficiency begets an overactive IMD response and dysbiosis, whereas L. plantarum normalizes IMD activation and reduces dysbiosis (65). Vibrio cholerae’s Type 6 Secretion System (T6SS) and its interaction with Acetobacter pasteurianus microbiota induce the imd pathway in D. melanogaster, triggering immune responses (66). L. plantarum, a gut microbiota, can protect D. melanogaster from common pathogens, such as Pseudomonas aeruginosa and Serratia marcescens, by inducing the host’s immune response (56). Moreover, certain yeast commensals in D. melanogaster protect against the pathogenic fungus Aspergillus flavus (67). L. plantarum also mitigates D. melanogaster’s susceptibility to the pathogenic fungus Diaporthe FY (68). Furthermore, Erwinia carotovora pathogen infection and commensal microbes can trigger lipid utilization through enterocytes, but with distinct outcomes prevalent upon microbial cues, imd signaling, ROS production, and lipid utilization (64). Cell type-specific responses within the gut can also induce immune responses and are recognized by the gut microbiota. For instance, imd pathway activation in enterocytes invokes an immune response, activation in enteroendocrine cells promotes lipid utilization and improves anabolic growth (64). The microenvironment is also significant in maintaining constitutive immunity and gut microbiota concentration (69). Similarly, antimicrobial peptides and lysozymes, two immune effector families, regulate gut microbiota composition and abundance (70).
3.2 Gut microbiota in T. molitor
Like Drosophila, commensal microorganisms in T. molitor also affect gut immune responses and various physiological activities. The surrounding environment is a substantial source of diverse beneficial and pathogenic microorganisms. Different microorganism types could enter the T. molitor gut and establish themselves as symbionts or pathogens. Symbiotic microorganisms contribute to numerous physiological activities and environmentally friendly actions, such as detoxification in phytophagous insects, lignocellulose degradation in xylophagous insects, and antimicrobial compound production to protect against pathogens (71).
Experimental data has revealed that T. molitor harbors Bacillus, Lactococcus, Weissella, Escherichia, and Clostridiaceae spp. in an open environment but is dominated by Enterococcus spp. and some unclassified Enterobacteriaceae in a closed ecosystem. Microorganisms in the open environment actively contribute to host metabolism and immune activity, whereas the closed ecosystem offers the opportunity to select probiotic strains for improving growth efficiency (72). Another study on the gut of T. molitor identified fermentative bacteria, such as Weissella and Lactococcus spp.; proteolytic bacteria responsible for protein degradation, like Rahnella and Cronobacter spp.; and other microbiota groups, including Lactobacillus, Bacillus, Spiroplasma, Clostridium, Enterobacter, and Pantoea found in different concentrations across gut regions, with the hindgut containing the most diversity (73). Moreover, the gut microbiota in T. molitor was revealed to have significant plasticity in response to environmental factors, particularly soil. The composition of microbiota can rapidly fluctuate from one commensal group to another based on alterations in soil composition (74).
T. molitor and its gut microbiota degrade polystyrene (PS), polyvinyl chloride (PVC), polyethylene (PE), acrylonitrile butadiene styrene (ABS), polyurethane (PU), bio-based cross-linked polymers, and other plastic polymers (75). Research findings have identified eight specific microorganisms responsible for polystyrene biodegradation in T. molitor: Citrobacter freundii, Klebsiella aerogenes, S. marcescens, Stenotrophomonas maltophilia, Bacillus thuringiensis, P. aeruginosa, Enterococcus faecalis, and Enterobacter asburiae. It has been studied that T. molitor gut secretory factors and gut microbiota contribute to plastic degradation. Emulsifying factors with 30-100 kDa molecular weights were found in the T. molitor gut. In contrast, gut microbiome-produced secretory factors with molecular weights below 30 kDa were responsible for degrading plastic molecules (76). In addition, T. molitor’s gut microenvironments may be plays a crucial for plastic biodegradation. Therefore, combining T. molitor, specific gut microbiota, and an artificial microenvironment’s design will be a promising chamber for effective plastic degradation (76, 77).
Moreover, studies have demonstrated that although polystyrene provides some nutrients to T. molitor, but polystyrene has negative impacts on growth and development (78). T. molitor cannot survive on a diet consisting solely of plastic; additional essential nutrients are required (79). For example, different food supplements, such as bran with a 7:1 (w/w) plastic ratio, can promote T. molitor growth (80). Another study revealed that for polystyrene degradation, Erwinia olea, Lactococcus lactis, and Lactococcus garviae were the dominant microbiota in the gut of T. molitor (81). Similarly, for polyurethane degradation by T. molitor’s different gut microbiota, such as Paraclostridium, Chryseobacterium, Kosakonia, and Pseudomonas, were found to be responsible. During the degradation process, they can degrade the polymer by 35% within 17 days, but it experiences a weight loss of 14% (82). On the other hand, T. molitor’s gut microbiota breakdown long-chain and branched polymers by diffusing extracellular depolymerase, producing shorter chains containing 16 carbon atoms. The microbiota utilizes fatty acid degradation pathways to generate intermediate products during plastic degradation, while T. molitor acts as a downstream decomposer (79). Additionally, T. molitor’s gut microbiota can create cracks and holes in plastic sheets, form biofilms, and utilize them as a carbon source for growth (83). Furthermore, plastic molecular weight critically affects biodegradation. Plastic with low, medium, high, and ultra-high molecular weights can significantly influence metabolic pathways, induce intestinal dysbiosis, and alter gut microbiome composition (81, 84). Generally, T. molitor employs an extensive depolymerization process for plastic biodegradation, although the depolymerization of ultra-high molecular weight plastics is limited (84).
In addition, for plastic degradation, the gut microbiota involves the secretion of siderophores such as enterobactin and yersiniabactin, enzymes, and specific enzymatic genes such as undecaprenyl-phosphate galactose phosphotransferase [EC:2.7.8.6]. Furthermore, the supernatant of intestinal content acts as a surfactant, enhancing the hydrophilicity of plastic and providing physical support for the gut microbiomes (77). A fascinating discovery revealed that Enterobacter hormaechei LG3 produces a biofilm on the plastic’s surface for degradation under anaerobic conditions. In this study, researchers identified degradative enzyme-expressing genes of the peroxidase family, including tpx, ahpC, and bcp (85). Another study demonstrated that phosphatases, esterases, leucine arylamidase, ß-galactosidase, ß-glucuronidase, α-glucosidase, ß-glucosidase, chitinase, α-mannosidase, α-fucosidase, and numerous other enzymes are involved in plastic degradation. Furthermore, the study suggested that enzymatic activity plays a more substantial role in plastic biodegradation than gut microbiota (86).
Moreover, to the environmental considerations regarding plastic degradation, T. molitor holds promise and has been approved by the European Union as a protein source to meet global food demand. Recent efforts have focused on enhancing T. molitor’s nutritional value by incorporating probiotic strains, such as Pediococcus pentosacceus KVL-B19-01 and Enterococcus faecium 669, as commensal gut bacteria. These strains contribute as gut microbiota and promote host growth and development. Moreover, they protect against entomopathogenic fungi, such as Metarhizium brunneum (87). P. pentosaceus significantly protects T. molitor from six different insect pathogenic strains, including B. thuringiensis and species of Serratia and Pseudomonas bacteria (88). Another research study demonstrated that introducing Lactobacillus and Bifidobacterium strains, such as Lactobacillus casei 01 and Bifidobacterium animalis ssp. lactis Bb1 into the T. molitor gut, optimized them as gut microbiota without adversely affecting the host. In addition, these introduced strains can produce short-chain fatty acids (SCFA) and lactate (89).
J. Poveda et al. investigated T. molitor frass’ potentiality, including gut microbiota, as a biofertilizer and verified that it can enhance plant growth, even under stressful conditions (90). Conversely, parasitic symbionts hinder T. molitor mass production, as these symbionts can transfer to humans when T. molitor is used as a food source (91). When parasites are introduced into the insect gut microbiota, they stimulate the innate immune system through nonspecific cellular constituents known as hemocyte. Hemocytes respond to infectious agents by activating the phenoloxidase enzymatic cascade, ultimately producing specific antimicrobial peptides. However, parasites can alter the gut microbiota due to their transient nature, and in some cases, the host gut microbiota participates in establishing these parasites (92). Furthermore, T. molitor gut microbiota assists in detoxifying toxic food-derived compounds, such as plant-derived glucoside salicin. In summary, T. molitor’s immune mechanisms and gut microbiota are strikingly adaptable as vital host defense components (93).
4 D. melanogaster’s and T. molitor’s gut immune response
Diverse immune signaling pathways are intricately involved in mediating the gut immune response in D. melanogaster and T. molitor. These pathways are triggered by various signaling molecules released by pathogens and are sometimes associated with commensal microorganisms. The specific immune signaling pathways activated upon gut infection depend on the infecting microorganism’s nature and composition. The following sections explore the immune signaling pathways crucial for maintaining gut homeostasis and defense mechanisms.
4.1 Imd signaling pathway in D. melanogaster
Peptidoglycan, a bacterial cell wall component derived from Gram-negative bacteria and Gram-positive bacilli, recognition mediates the immune response of gut cells in D. melanogaster. The peptidoglycan structure consists of 1,4-linked N-acetylglucosamine and N-acetylmuramic acid cross-linked sugar chains, four amino acids, and meso-diaminopimelic acid, forming what is known as DAP-type peptidoglycan. The detection of this peptidoglycan by Drosophila gut cells relies on the presence of PGRPs. Notably, the genes responsible for encoding PGRP are categorized by their transcript lengths. Upon DAP-type peptidoglycan binding, PGRP-LC and PGRP-LE transmembrane receptors activate the imd pathway (Figure 3). This binding event generates a signaling complex involving IMD, Dredd, and fas-associated protein and the death domain (FADD), subsequently translocating to the plasma membrane. Dredd is activated through K63-ubiquitination mediated by the E3-ligase inhibitor of apoptosis 2 (IAP2). Dredd cleaves IMD at its N-terminus, revealing an IAP2-binding motif and facilitating its interaction with IAP2’s BIR domain. As a result, the TAK1/TAB2 complex is recruited to the signaling complex. TAK1 then functions as a MAPKKK kinase and activates the IKK complex, which comprises the catalytic activity subunit IRD5 and the regulatory subunit “Kenny”. Next, the activated IKK complex phosphorylates Relish at multiple sites, activating various transcription factors, including RNA polymerase II. Negative imd pathway regulation incorporates the amidase activity of specific PGRPs, including PGRP-LB, PGRP-SC1a, PGRP-SC1b, and PGRP-SC2, to reduce bacterial peptidoglycan levels. Additional negative regulators include defense repressor 1 (Dnr1) to inhibit Dredd, Caspar for impeding Dredd-dependent Relish cleavage, Trabid at the TAK1 level, the deubiquitinating enzyme cylindromatosis (CYLD), SkpA (a subunit of the SCF E3 ubiquitin ligase targeting Relish), and transcriptional repressors that include caudal and the Oct1 homolog Nubbin (45, 96, 97).
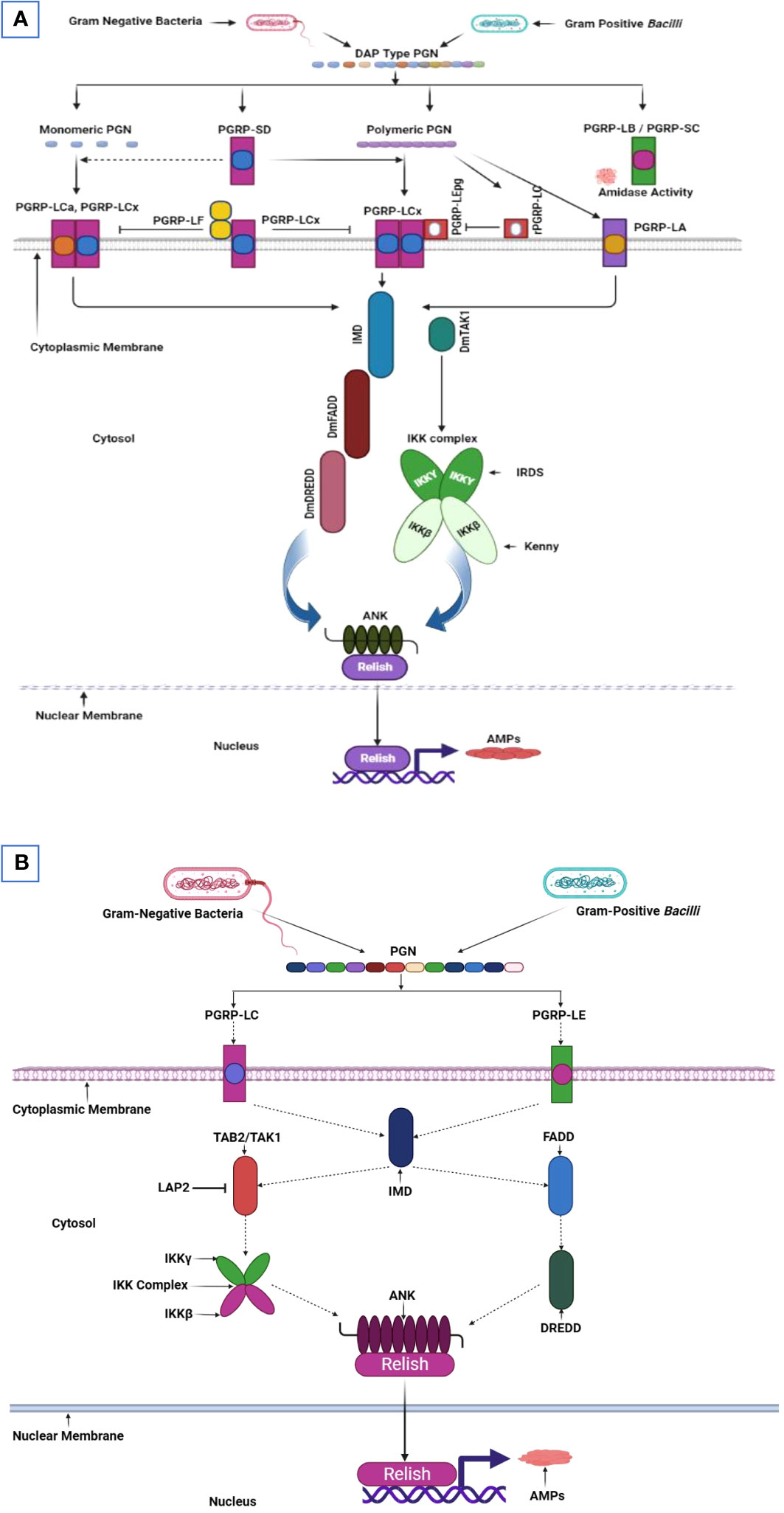
Figure 3 Imd pathway in D. melanogaster and T. molitor. (A) The immune deficiency (imd) pathway in the Drosophila midgut concurrently initiates in the proventriculus, anterior, median, and posterior regions. All receptors in these regions are essential for imd pathway activation. Peptidoglycan (PGN) recognition proteins (PGRP)-LEs and PGRP-LCs within the insect gut identify the polymeric and monomeric diaminopimelic acid (DAP) type PGN derived from gram-negative bacteria and gram-positive bacilli. These recognition signals are then translocated to the imd pathway within the cytoplasm. The intracellular domain of PGRP-LCs is subsequently activated and recruit IMD and, form a complex with the fas-associated protein and the death domain (dFADD). This complex facilitates cleaving phosphorylated Relish, a protein with an N-terminal Rel DNA-binding domain and inhibitory ankyrin (ANK) repeats. The Relish domain is translocated to the nucleus, where it binds to NF-κB-response elements and begins transcribing genes encoding antimicrobial peptides. Meanwhile, the inhibitory Relish domain remains in the cytoplasm. Relish phosphorylation is mediated by a κB kinase (IKK) complex inhibitor, comprising immune responses deficient 5 (Ird5) and Kenny. This complex is activated by the mitogen-activated protein (MAP) kinase transforming growth factor (TGF)-β activated kinase1 (dTAK1), operating in an IMD and FADD-dependent manner. (B) The imd pathway activates in response to Gram-negative bacteria and Gram-positive bacilli infection in T. molitor’s gut. Signal transduction within gut cells hypothetically incorporates imd pathway activation, which comprises a death domain that facilitates TmFADD (TAK1 activator) and TmDredd (a caspase) recruitment. The activated TmTAK1, a protein kinase, then phosphorylates Relish through TmIKK-β, -γ, and -ϵ. This phosphorylation event translocates the Rel domain into the nucleus, initiating antimicrobial peptide gene transcription. This illustration has been adopted from M. A. M. Kojour et al and Y. Lu et al (94, 95).
The predominant intracellular receptor for monomeric peptidoglycan (PGN) in the Drosophila midgut is PGRP-LE. Conversely, in the proventriculus and hindgut, PGRP-LC binds to PGRP-LE in the ventriculus, facilitating the identification of monomeric and polymeric PGN structures. Commensal bacteria commonly present in the Drosophila gut, such as Acetobacter and Lactobacilli spp., harbor DAP-type PGNs. These bacteria maintain basal imd pathway activation and contribute to gut homeostasis (96). A comprehensive investigation concluded that the imd pathway is pivotal in governing antimicrobial peptide production and preserving barrier integrity within the gut. Remarkably, the coordinated imd and JNK signaling activities have been implicated in gut cell shedding regulation and controlled transcription factor GATAe expression, effectively controlling pathogen infections (98). Intriguingly, another experimental study revealed that microbial metabolic signals, specifically the short-chain fatty acid acetate, can induce imd pathway activation in enteroendocrine cells. This activation subsequently triggers endocrine peptide Tachykinin (Tk) upregulation, promoting larval development by influencing lipid metabolism and insulin signaling (99).
The imd pathway is a crucial immune signaling pathway in D. melanogaster, strictly regulated by various factors. Negative regulators, such as Pirk and PGRP-LB, balance bacterial infection and gut microbiota tolerance, while PGRP-LE acts as a sensor (100). In investigating the response to killed bacteria in Drosophila, specifically Escherichia coli and Staphylococcus aureus, gender-specific variations were observed due to differential receptor, signaling molecule, and protein expressions (101). Several common pathogenic microbes inhabit Drosophila’s intestinal tract, including Pseudomonas entomophila, Vibrio cholerae, Candida albicans, Enterococcus faecalis, and Staphylococcus xylosus. Certain pathogens, like S. marcescens and P. aeruginosa, can breach the intestinal barrier and activate the imd pathway (102, 103). While gram-positive bacteria generally activate the Toll pathway, a study demonstrated that the imd pathway and ROS partake in clearing ingested S. aureus, highlighting their versatile roles in insect gut immune defense (104). Microbial metabolites, such as acetate, can stimulate the imd pathway in anterior midgut enteroendocrine cells, producing antimicrobial peptides to protect against pathogens (105). In addition, Histone acetyltransferase Tip60 is an essential regulator in downregulating the imd signaling pathway by blocking dietary acetate and other acetyl-CoA sources, thereby blocking the pathway and fine-tuning gut immunity (106). On the other hand, the commensal gut microbiota can induce necrosis, activate the imd pathway, and alter bacterial populations, impacting D. melanogaster’s lifespan (107). Furthermore, the imd pathway and mustard mutants suppress V. cholerae infection and cholera toxins by inducing intestinal stem cell proliferation. This protective mechanism facilitates epithelial regeneration, protecting against pathogenic invasion (108). The imd signaling pathway is also vital for maintaining gut microbiota lysozyme concentrations, further contributing to gut homeostasis (70). The basic similarities and differences of imd and Toll signaling pathways in D. melanogaster and T. molitor have mentioned below in consideration of recognition receptor, adapter molecule, regulatory molecule, transcription factor and proteolytic cascades (Table 1). Experimental studies reveal that gut and Malpighian tubules of D. melanogaster are not directly related to the expression of genes related Toll signaling pathway. However, in consideration of T. molitor the gut immune response is stimulated by the Toll and imd immune signaling pathways (Table 2). The imd is the major regulatory pathway for gut and Malpighian tubules in D. melanogaster.
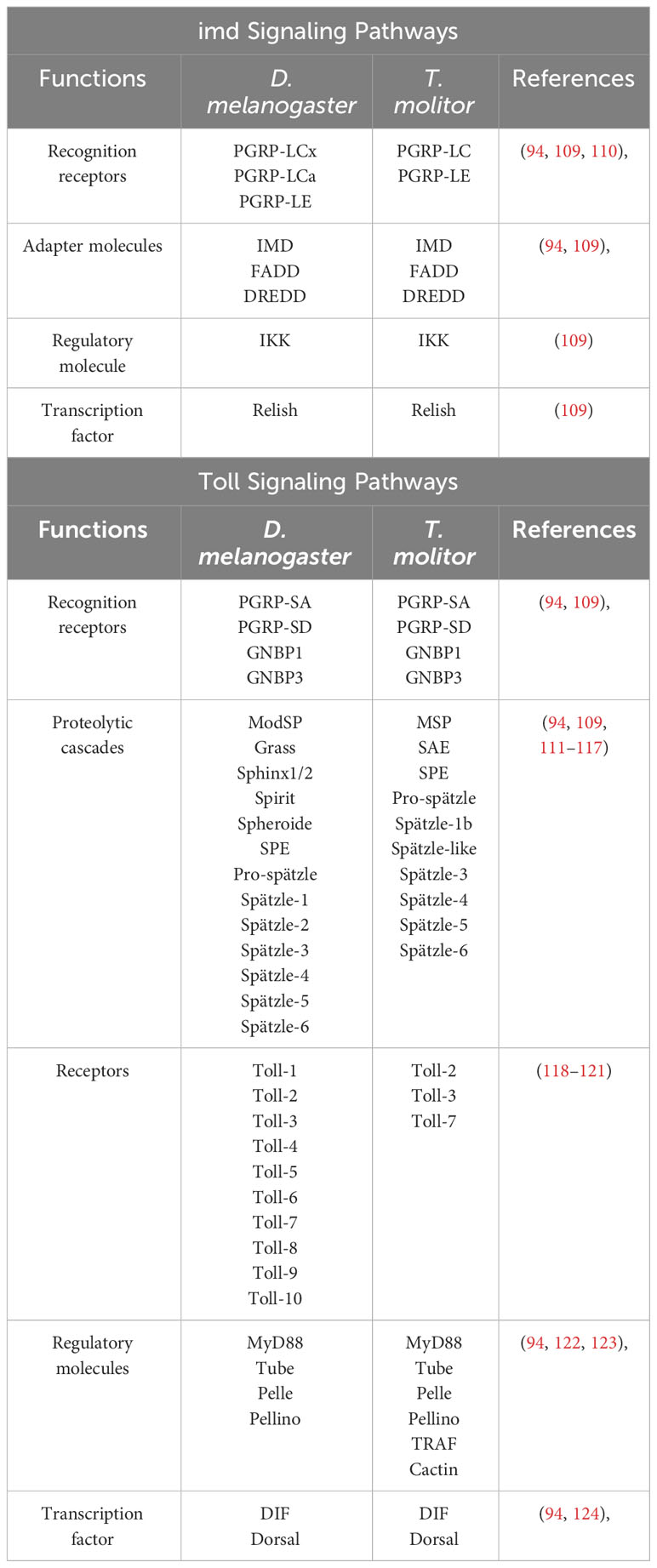
Table 1 Basic similarities and differences of imd and Toll signaling pathway in D. melanogaster and T. molitor.
4.2 Imd signaling pathway T. molitor
The imd pathway in T. molitor is activated upon the recognition of pathogens by PRRs, such as PGRP-LC or PGRP LE. An intracellular signal is transmitted to the adaptor protein IMD. The IMD forms a signal complex with dFADD, K63, and caspase DREDD which induces IMD cleavage (Figure 3). Then, the K63-polyubiquitin molecule activates TAK1 and TAB2’s ubiquitin-binding domain. Relish phosphorylation and the subsequent translocation, which produce antimicrobial peptides for pathogen clearance, depend on the Imd signaling upstream receptors; PGRP LE/LC, relevant adaptor molecules; FADD, DREDD, TAB2, TAK1, and the IKK complex comprising TmIKK- and (94). Moreover, IKK/NEMO as a non-catalytic regulatory subunit is pivotal in the NF-ĸB pathway signaling (136).
Specific immune responses in various T. molitor tissues, particularly the gut, are mediated by nine distinct AMP genes: Tenecin1, Tenecin4, Attacin1a, Attacin1b, Attacin2, ColeoptericinA, ColeoptericinC, Defensin, and Defensin-like. Notably, suppressing IMD expression during E. coli infection exacerbates mortality rates and downregulates these nine AMP genes. Similarly, C. albicans infection reduces five AMP gene expressions, namely Tenecin2, Defensin-like, ColeoptericinA, Attacin1a, and Attacin2 (137, 138). A comprehensive scientific study has elucidated TmPGRP-LE’s significance as a sensor for inducing AMP production via the Imd pathway in T. monitor’s gut. TmPGRP-LE mRNA levels increase during Listeria monocytogenes, E. coli, and C. albicans infections, influencing TmRelish, TmDorX1, and TmDorX2 expression levels in T. molitor’s gut (139, 140). Furthermore, TmRelish’s mRNA expression is upregulated in the gut following gram-negative bacteria infection, such as E. coli, and downregulation of TmRelish leads to repression of the nine AMP genes (141).
4.3 JAK/STAT signaling pathway in D. melanogaster
When D. melanogaster ingests gram-negative bacteria, the imd signaling pathway activates, producing various antimicrobial peptides. The JAK/STAT signaling pathway synthesizes antibacterial and Drosomycin-like peptides with antifungal properties, specifically within D. melanogaster’s anterior midgut (142, 143). Scientific investigations have demonstrated that the JAK/STAT, JNK, and DUOX pathways contribute to epithelial renewal through cell proliferation, ensuring gut homeostasis when faced with pathogenic infections. The JAK/STAT signaling pathway’s activation is facilitated by hop Tum-l or upd-3 expressions, encouraging gut renewal during infection, and may also be involved in non-infectious stages (143).
The JAK-STAT pathway, consisting of hopscotch/hop (JAK) and Stat92E (STAT transcription factor), in D. melanogaster responds to IL-3, IL-5, and IFN-γ hematopoietic cytokines and growth factors including granulocyte-macrophage colony-stimulating factor (GM-CSF), erythropoietin (EPO), growth hormone (GH), and prolactin. JAK activates when cytokines bind to their respective receptors, leading to receptor dimerization and subsequent association with the receptor’s cytoplasmic tail (Figure 4). Consequently, JAKs undergo phosphorylation at tyrosine residues, which serve as docking sites for STAT molecules (Stat92E)’s Src homology 2 (SH2) domains. Simultaneously, STAT is phosphorylated at tyrosine (Tyr-704) by JAKs, enabling STAT dimer formation and translocation into the nucleus. Subsequently, the STAT dimers bind to specific genes with the TTCCCGGAA binding sequence (108). Notably, a scientific study highlights the JAK-STAT pathway’s prominence in controlling intestinal stem cell differentiation, gut immunity, tissue regeneration, cell fate determination, and overall cell homeostasis in D. melanogaster. Additionally, D. melanogaster enteroendocrine cells secrete assorted neuropeptides responsible for regulating gut physiology and promoting growth (145).
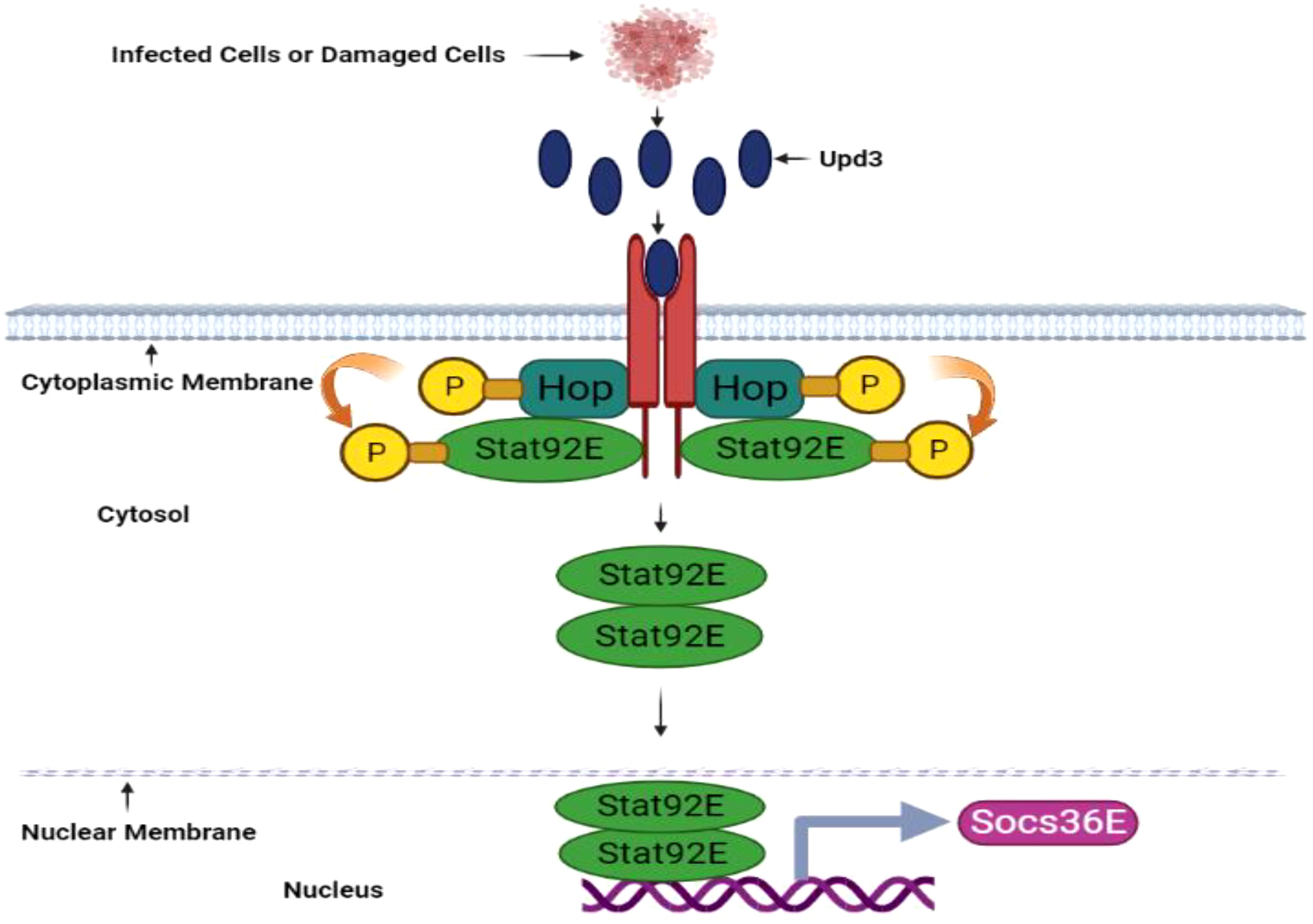
Figure 4 JAK/STAT pathway in D. melanogaster. The JAK/STAT pathway is activated upon pathogen invasion and produces antimicrobial peptides in the midgut, including antifungal Drosomycin-like peptides. This pathway is also vital for cell proliferation. Tissue damage prompted by pathogenic infection induces upd3 cytokine expression. Upon upd3 cytokine binding, the dimerized domeless receptors Hopscotch (Hop)/JAK activate, and Hopscotch phosphorylates itself and specific tyrosine residues on the receptors in cytoplasmic regions. These phosphorylated tyrosine residues serve as Stat92E binding sites, a transcription factor component of the STAT family. Hopscotch further phosphorylates Stat92E at tyrosine residues, promoting its dimerization and subsequent translocation into the nucleus. Once in the nucleus, Stat92E binds to the promoter regions of its target genes. The JAK/STAT signaling pathway also upregulates Socs36E gene expression This illustration has been adopted from C. Kietz and A. Meinander et al (144).
4.4 Duox-ROS defense mechanism in D. melanogaster
A rapid reactive oxygen species burst is crucial for controlling bacterial growth in D. melanogaster’s gut. This ROS surge is facilitated by membrane-associated dual oxidase (Duox), a member of the nicotinamide adenine dinucleotide phosphate (NADPH) oxidase family (Figure 5). The p38, PGN-dependent, and PGN-independent pathways, which are integrated with the MEKK1-MEK3-p38-ATF2 pathway, regulate DUOX gene transcription (147). Certain gut-pathogenic bacteria, such as Candida intestine, Vibrio, Klebsiella, Shigella, Pseudomonas, and Serratia, secrete uracil, a nucleobase found in uridine. Moreover, DUOX activity is regulated by calcium ions released from the host gut cells’ endoplasmic reticulum, controlled by the inositol 1,4,5-triphosphate (IP3) receptor, phospholipase C-ß (PLCß), and G protein α-q (Gαq). Hence, researchers propose that DUOX-ROS activation transpires through the peptidoglycan-dependent pathway and a PGRP-LC/IMD/MEKK1/MKK3/p38/ATF2 cascade or the uracil-dependent PLCß pathway (45).
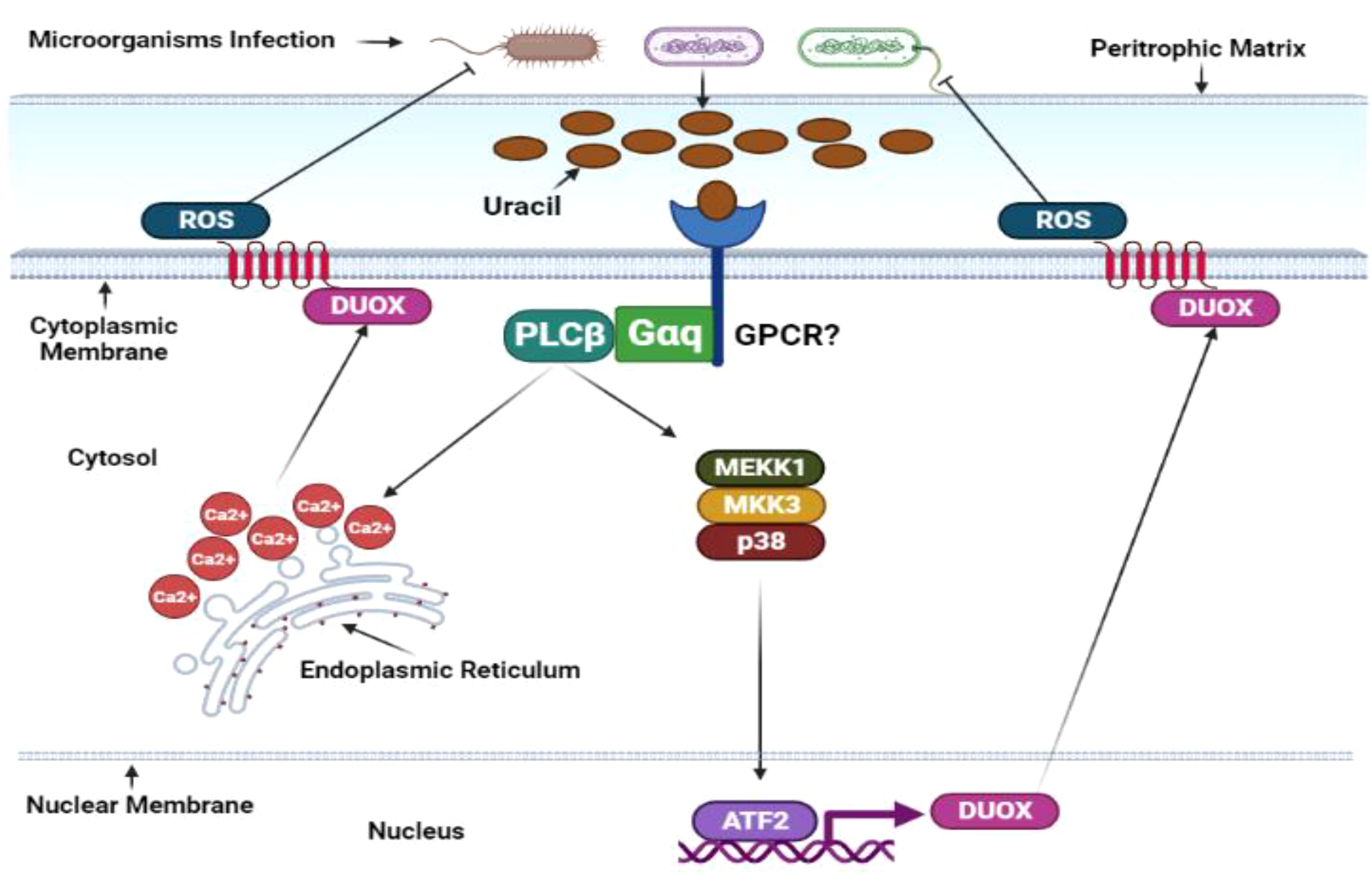
Figure 5 Duox-ROS defense mechanisms in D. melanogaster. The immune response in the insect gut produces reactive oxygen species. Similar to the IMD pathway, ROS is activated under basal conditions in response to gut microbiota and ingested microorganisms. However, this response is significantly amplified upon microbial infection. Microbially derived uracil triggers the activation of the adaptor molecules guanine-nucleotide-binding protein q subunit-α (Gαq) and phospholipase Cβ (PLCβ). These molecules stimulate inositol-3-phosphate synthesis, releasing intracellular calcium and transcribing Duox, the gene responsible for encoding oxidase enzymes. Duox transcription can also be induced by the p38 mitogen-activated protein kinase pathway and transcription factor Atf2. Under basal conditions, the activity of the Duox pathway is tightly regulated. The transcription of Duox is downregulated by MAPK phosphatase 3 (Mkp3), which is induced by PLCβ and Calcineurin B (CanB) in the absence of microbial infections. The specific activities of G protein-coupled receptors (GPCRs) in this pathway remain to be determined and require further investigation. This illustration has been adopted from N. Buchon et al (146).
Drosophila gut cells’ intricacy includes the ability to discern commensal bacteria from their pathogenic counterparts by detecting uracil’s metabolic signature and secretion from pathogens during the stationary growth phase. Intriguingly, diverse phenotypic activities manifest among genetically similar strains of Drosophila gut epithelia. For example, while the Lactobacillus brevis strain EW exhibits colitogenic behavior, another L. brevis strain is a harmonious commensal bacterium. Uracil, notably integral to this intricate system, induces gut epithelium cell renewal through the DUOX signaling pathway and activates the JAK-STAT pathway via cytokine Unpaired-3 expression, orchestrating gut homeostasis (148). Uracil is formidably intricate, as it activates the Hedgehog (Hh) signaling pathway and spurs the cell adhesion molecule Cadherin 99C (Cad99C) into action. The resulting endosome formations are pivotal for activating PLCß/PKC/Ca2+-dependent DUOX machinery (149, 150). Furthermore, studies have unveiled the complex dance between lipogenesis induction, DUOX activity blockade, and ATG1-dependent lipophagy-induced lipolysis, a process indispensable for DUOX activation. TRAF3-AMPK/WTS-ATG1 pathway components deftly modulate infection-induced lipolysis, diminishing DUOX activation and any lingering D. melanogaster gut infections (151). Amidst intricacies, the Mesh protein regulates DUOX expression and gut bacteria proliferation by forming an Arrestin-mediated MAPK JNK/ERK phosphorylation cascade (152). In the grand tapestry of gut biology, DUOX-dependent reactive oxygen species are invaluable as they dictate the delicate balance between gut cell repair, pathogenic bacteria eradication, and host survival during infection (153).
4.5 JNK/FOXO signaling pathway in D. melanogaster
Within the realm of cellular intricacies, the c-Jun N-terminal kinases, esteemed members of the mitogen-activated protein kinase family, stand as guardians of tissue homeostasis, diligently overseeing gut functionality, cell damage repair, the delicate dance between cell survival and death, the orchestrated responses to DNA damage, and regulate inflammatory cytokines. While JNK1, JNK2, and JNK3 genes are responsible for JNK protein production in most organisms, a solitary basket (bsk)-encoded JNK gene and the two Hemipterous (hep) and DJun/DFos JNK kinases activate this multifaceted pathway in D. melanogaster. When the JNK signaling pathway diverges from the imd pathway, a distinct cascade at the dTAK1 stage begins (Figure 6). Traversing D. melanogaster’s embryonic stage, the JNK pathway relies on the slipper (slpr) for activation, setting transcription factors AP-1 into motion while simultaneously stimulating the Forkhead Box O transcription factor FOXO, thus maintaining gut homeostasis (155). Inquiries into dietary influences have revealed that high-sugar edibles incite gut stem cell differentiation, showcasing the JNK pathway’s complexity and prominence (156). Another study unveiled that activating the JNK/FOXO pathway stimulates dPrxV, essential for the subsequent pathogenic onslaught within D. melanogaster’s gut (157).
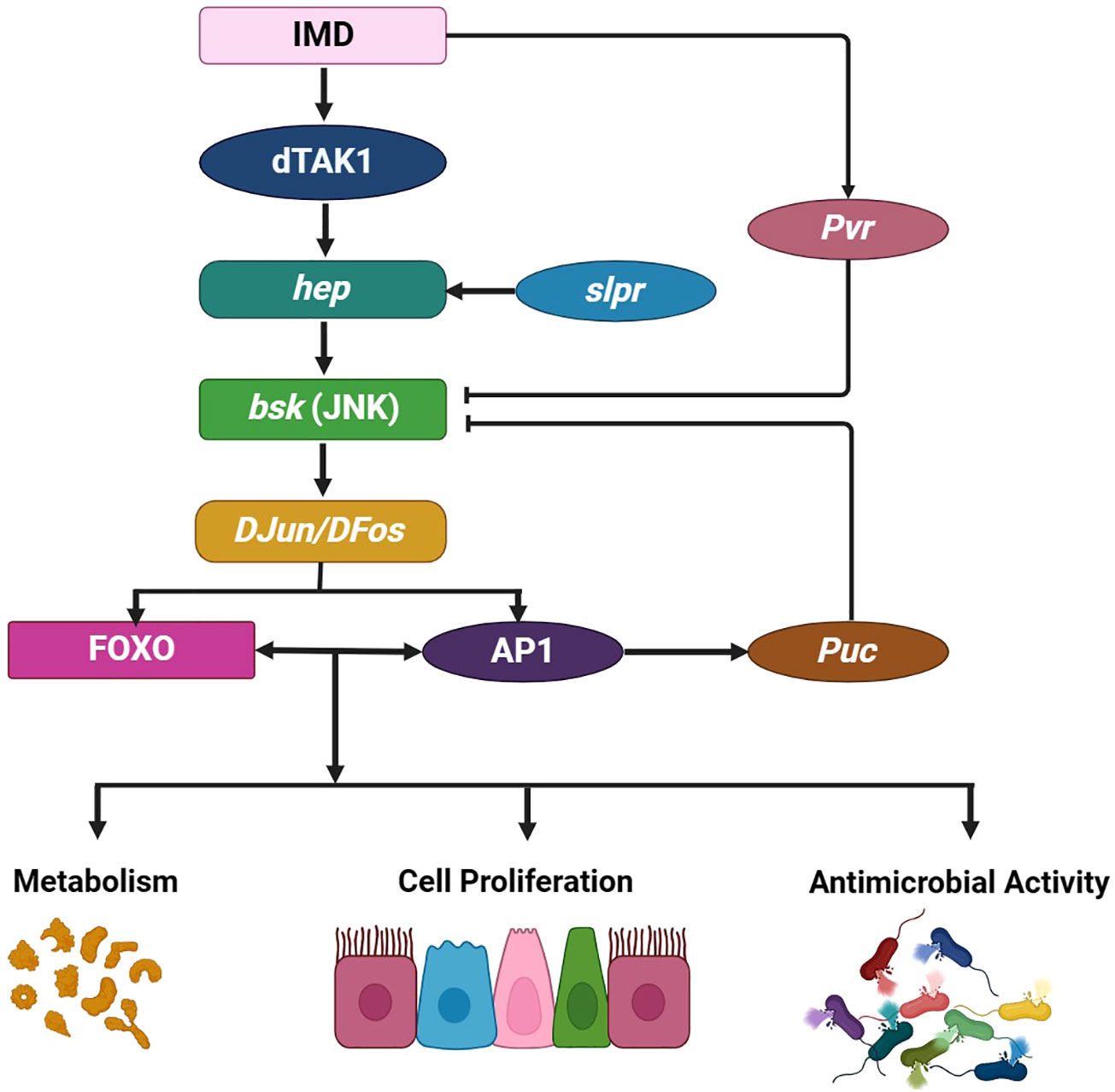
Figure 6 JNK pathway in D. melanogaster. The JNK pathway branches off from the Immune deficiency pathway at dTAK1, comprising the single gene basket (bsk) and two JNK kinases, Hemipterous (hep) and DJun/DFos. A broad range of intrinsic and extrinsic factors can activate this pathway. For example, the slipper (slpr) triggers JNK activation during embryonic dorsal closure, prompting various tissue- and context-specific cellular responses. The JNK signaling pathway is pivotal for regulating and promoting several crucial physiological processes that influence gut immunity and homeostasis, including antimicrobial responses regulated by the transcription factor Relish. This process also involves cytoprotection, which induces protective gene expressions, such as hsp68, gstD1, fer1HCH, and mtnA, in response to oxidative damage. Furthermore, the JNK pathway influences metabolism and controls inflammation-induced apoptosis, wound healing, and cell proliferation, which are regulated by Upd3. Negative JNK pathway regulation occurs through Puckered (Puc) and Pvr activity. This illustration has been adopted from G. Tafesh-Edwards and I. Eleftherianos et al (154).
4.6 Other signaling pathways in D. melanogaster
Safeguarding against viral invasion in D. melanogaster’s gut epithelia is entrusted to the ERK signaling pathway. This intricate defense mechanism unfolds through two signals. First, the peptidoglycan recognition secretion factor Pvf2 emanates from the gut microbiota A. pomorum. The virus sets off a signaling cascade, triggering kinase Cdk9 release, which is indispensable for Pvf2 production (158). Further revelations have verified that the p38 pathway-mediated heat-shock factor is pivotal for safeguarding the gut against pathogenic assault by managing the JNK pathway (159).
5 T. molitor’s gut immune response
The pathways in T. molitor’s gut immune response are each directed by a diverse array of signaling molecules released by the gut and its commensal inhabitants. These immune signaling pathways vary by the specific invading microorganisms, unveiling a fascinating interplay between host and pathogen.
5.1 Toll signaling pathway
The Toll signaling pathway begins when GNBP3 recognizes fungal ß-glucan and Lys-type PGN, namely PGRP-SA and GNBP1, from gram-positive bacteria. These extracellular PRRs activate a serine cascade, culminating in extracellular cytokine Spätzle induction and its binding with the Toll receptor ligand. Within this intricate network, the trimeric complex formed by adaptor protein MyD88, Tube, and the kinase Pelle invoke a series of phosphorylation events. Pelle phosphorylates Cactus, an inhibitory protein. Meanwhile, the transcription factors Dorsal and Dorsal-related immunity factor (Dif) within the cytoplasm traverse into the nucleus, where they generate an array of antimicrobial peptides (Figure 7) (161). Remarkably, the Toll signaling pathway’s activation is not initiated through direct contact with the microorganisms but rather upon detecting the cytokine-like polypeptide Spätzle, evidencing Semmelweis and Osiris genes’ influence in gram-positive-dependent Toll activation (94).
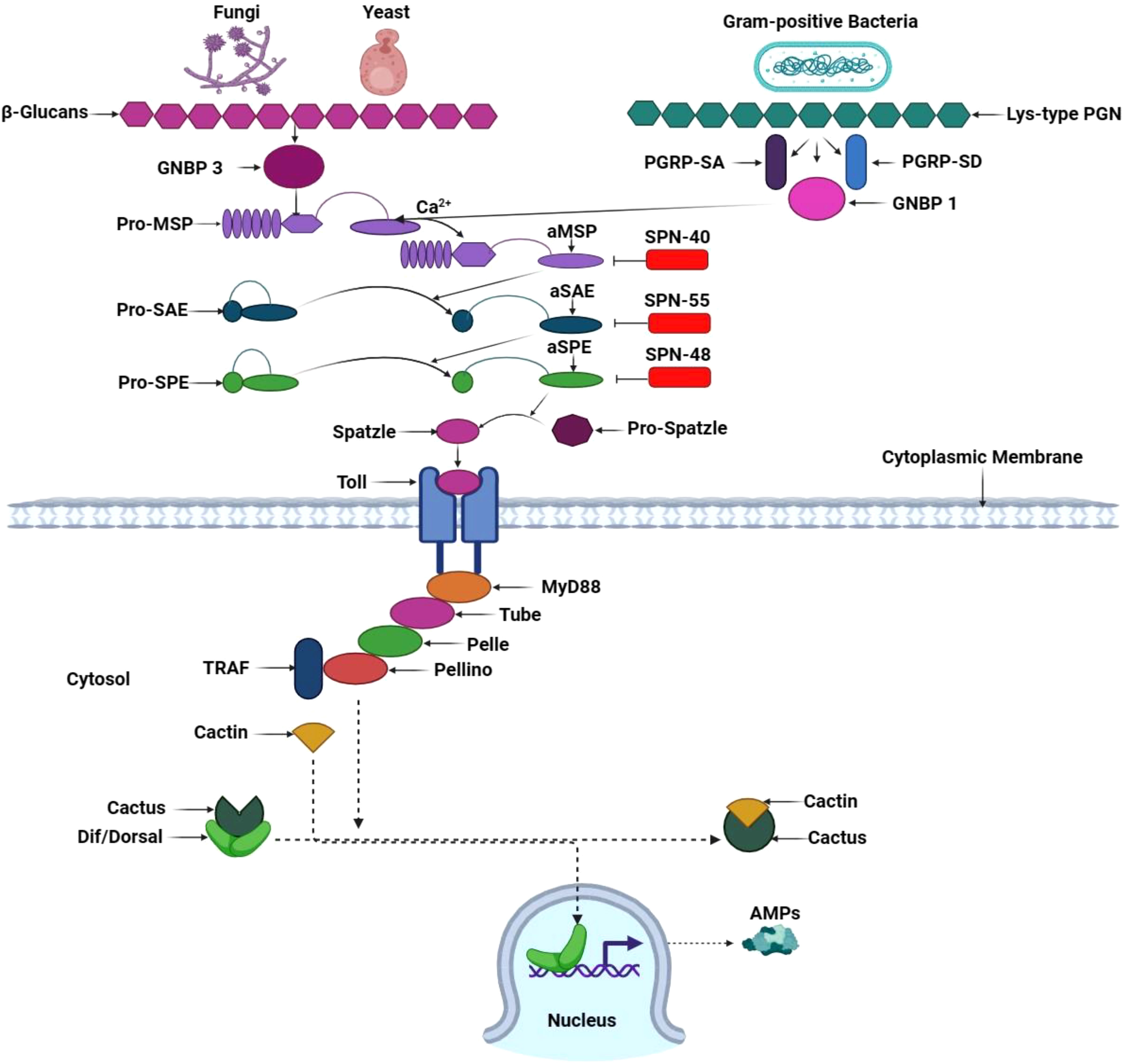
Figure 7 Toll signaling pathway in T. molitor. Circulating pathogen recognition receptor GNBP3 recognizes the fungal cell walls’ β-1,3 glucan component and activates the Toll pathway in T. molitor. Simultaneously, GNBP1 and the peptidoglycan recognition proteins PGRP-SA and PGRP-SD recognize Gram-positive bacteria’s lyse-type peptidoglycan (PGN). In Tm, PGN/β-1,3-glucan exposure binds Lys-type PGN to TmPGRP-SA, thereby recruiting TmGNBP1 and Tm modular serine protease (MSP) zymogen. In the presence of calcium ions (Ca2+), the PGN/TmPGRP-SA/TmGNBP1 complex induces the activation of the TmMSP zymogen, resulting in the activation of TmMSP. This initiates a proteolytic cascade involving three serine proteases: MSP, Spz processing enzyme (SPE), and SPE-activating enzyme (SAE). The activated SPE cleaves pro-Spz, leading to the generation of processed Spz. A specific three-serpin complex (SPN40, 55, and 48) combines with serine proteases to prevent pro-Spz processing and phenoloxidase-mediated melanin synthesis. Next, downstream signaling through the Toll pathway is activated when mature Spz binds to its receptor, triggering the TmMyD88, TmTube, TmPelle, TmPellino, and TmTumor necrosis factor receptor-associated factor (TRAF) association in the cytosol. TmCactin binds to Cactus, resulting in the release of Dorsal-related immunity factor (Dif) and Dorsal from Cactus. This process translocates Dif and Dorsal into the nucleus, where they promote antimicrobial peptide gene transcription. This illustration has been adopted from M. A. M. Kojour et al and K. Ryu et al (94, 160).
Regarding T. molitor’s gut immunity, TmPGRP-SA mRNA levels are upregulated in response to E. coli and C. albicans invasion. This upregulation triggers antimicrobial peptide transcription, including TmTenecin-2, -4; TmDefensin-2; TmColeoptericin-1, -2; and TmAttacin-1a, 1b, and -2 (139). The cystine knot protein TmSpz-like is expressed upon E. coli invasion, generating numerous AMPs to protect the gut epithelia (162). Through a study on Coleoptericin gene expression in T. molitor’s gut immunity among E. coli, S. aureus, and C. albicans; it was discovered that the infection of E. coli tantalized the genes TmCole B and TmCole C of Coleoptericin, while fungi did not share the same allure (163). In addition, TmToll-2 expression was elevated in young larvae gut after E. coli, S. aureus, and C. albicans invasion (164). C. albicans infection in young T. molitor larvae induced TmToll-3 within the gut; however, AMP gene transcription factors were downregulated by E. coli infection (165).
6 Insect gut post-infection recovery
The potent production and proliferation of specialized daughter cells from intestinal stem cells are crucial in gut cell self-renewal following infection (136). ISC proliferation generates two distinct daughter cell types: ISC with self-renewal capacity and enteroblast cells capable of differentiating into enterocytes or enteroendocrine cells. Controlling ISC proliferation is paramount during gut epithelial damage from pathogens or toxic substances. Rapid ISC proliferation excessively accumulates cells, exhibiting pathogenic behavior. Conversely, if ISC proliferation is slowed, damaged cells cannot be adequately replaced, resulting in compromised gut integrity and ultimately leading to the demise of the host (166).
Moreover, a multitude of signaling pathways, namely JNK, JAK/Stat, Hippo, EGFR, Wg, Hh, and Dpp/BMP, are intricately involved in the complex process of gut recovery in insect (136). The regulatory molecule Myc influences downstream events within the Wingless, EGFR, Jak-Stat, and Hippo pathways, effectively proliferating intestinal stem cells (Figure 8). Conversely, in conditions of cellular stress within enterocytes the Upd3 ligands of the Jak-Stat pathway exhibit the ability to activate the JNK pathway, whereas the Keren ligands of the EGFR pathway can induce the activation of the Hippo pathway. When enteroblasts are stressed, Upd2 ligand production activates the Hedgehog pathway, and EBs activate Wingless ligands to stimulate the JNK pathway. Consequently, every cell within the gut possesses the remarkable capacity to regulate ISC proliferation; thus, each type is crucial in restorative processes following gut infection (166).
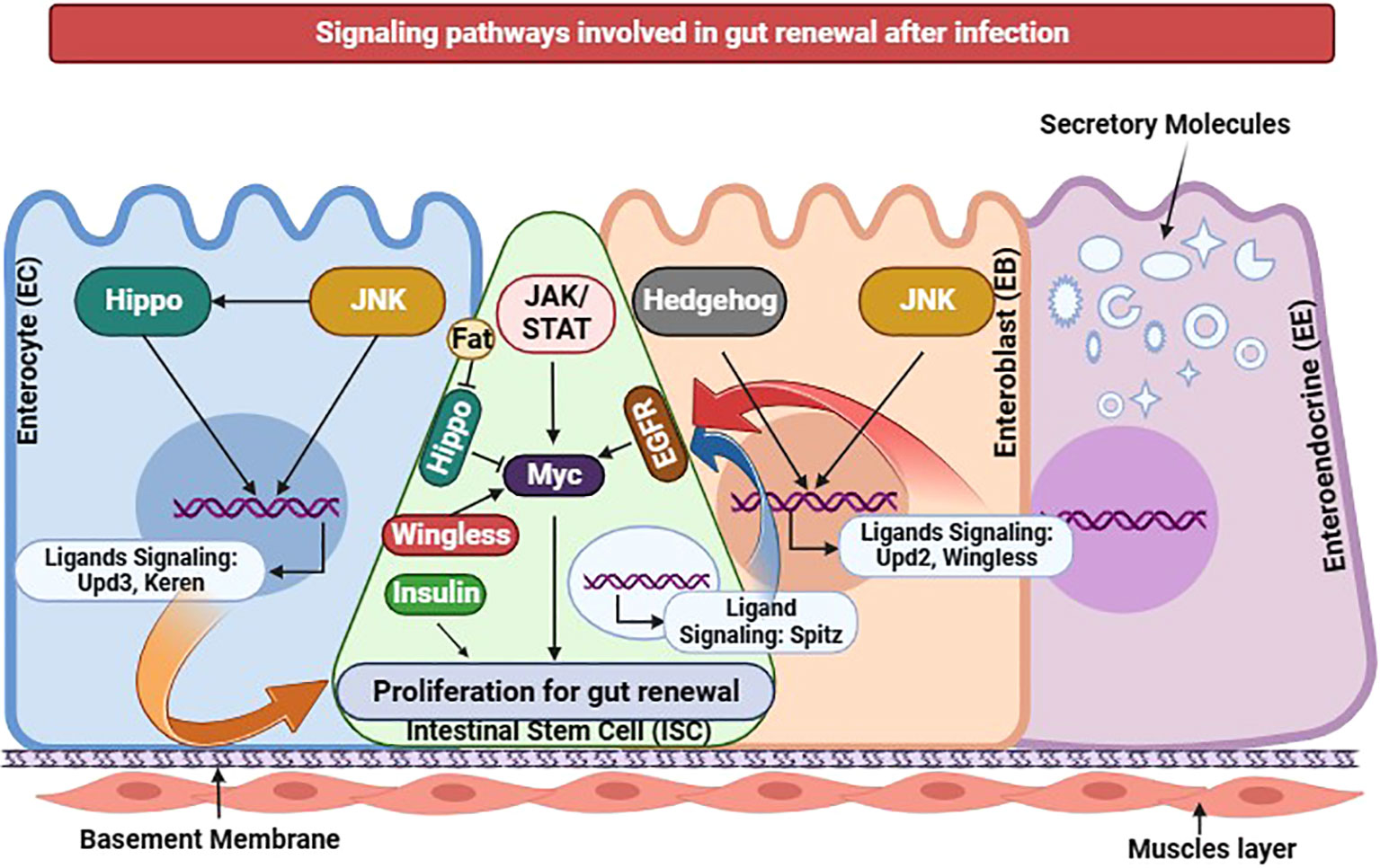
Figure 8 Insect gut renewal through different signaling pathways during infection in D. melanogaster. Various signaling pathways have been recently identified as regulators for intestinal stem cell proliferation. Jak/Stat, EGFR, Hippo, JNK, or Wingless pathway activation in ISCs alone can induce ISC proliferation. The transcription factor Myc functions as a downstream effector shared by Jak/Stat, EGFR, Hippo, and Wingless pathways in promoting ISC proliferation. Additionally, proper insulin receptor signaling in ISCs is necessary for its proliferation. In response to cellular stress, Enterocytes exhibit changes in gene expression regulated by the JNK and Hippo pathways, resulting in the induction of Jak/Stat pathway ligands, particularly Upd3, and EGFR pathway ligands, particularly Keren. Ligands of the EGFR pathway namely Vein and Spitz are expressed in visceral muscles and progenitors, respectively. Under stressed conditions, Enteroblasts produce Upd2 through activation of the Hedgehog pathway. EBs also express the Wingless ligand, regulated by the JNK pathway. The activity of the Hippo pathway in ISCs may be modulated by the intercellular interaction between two atypical cadherins: Fat in ISCs and Dachsous in ECs. Collectively, the diverse cellular components within the gut epithelium possess the ability to regulate ISC proliferation, functioning as intricate sensors that respond to environmental damage and initiate the gut renewal program. This illustration has been adopted from J.-H. Lee et al (167).
Nonetheless, researchers are actively investigating the interplay between intestinal renewal and immune pathways. While the imd pathway does not directly induce intestinal stem cell division, it does modulate commensal microorganisms within the gut to ensure homeostasis (168). Conversely, reactive oxygen species molecules, including SDS, DSS, and bleomycin, can stimulate ISC proliferation through the DUOX pathway. Similarly, ROS molecules can activate the Jak-Stat pathway by inducing redox-sensitive protein tyrosine phosphatases, the JNK pathway through thioredoxin, and the Wnt pathway via nucleoredoxin (166). One scientific study demonstrated that translational blockage triggered by P. entomophila infection subsequently released pore-forming toxins, and the host response producing reactive oxygen species can hinder immune response and impair gut repair. This impairment transpires through GCN2 kinase activation and rapamycin pathway inhibition (169). In response to apoptosis, pathogenic infections, and JNK-mediated stress signaling, enterocytes produce various cytokines, such as Upd, Upd2, and Upd3. These cytokines activate Jak/Stat signaling in ISC, promoting their proliferation and stimulating Delta/Notch signaling to facilitate gut tissue repair (170). In addition, the epidermal growth factor receptor (EGFR) pathway is initiated by Spitz, Keren, and Vein EGF ligands. Combined with the JAK/STAT pathway, this effectively triggers intestinal stem cell proliferation, facilitating proper infected gut tissue renewal from pathogen-induced damage (171).
Another investigation uncovered EGFR/MAPK pathway induction by the Insulin/Pi3K/TOR signaling cascade, serving as a crucial mechanism for gut renewal under stressful conditions by promoting the growth of EBs and ECs. The E2f1 transcription factor is essential for this induction; the Ras/Raf signaling pathway actively upregulates E2f1 levels and ensures proper transcriptional induction and fine-tuning of the EGFR/RAS/MAPK pathway activity, ultimately driving efficient gut regeneration (172). Moreover, during pathogenic infections within the gut, the YKi pro-growth transcription factor activates the Hippo pathway, influencing ISC differentiation and contributing to gut defense and repair (173). In a comprehensive gene expression study, 1833 genes were identified as responsible for ISC expression, 233 genes governed enterocyte function, 433 genes regulated enteroendocrine cell activity, and 2646 genes influenced Enteroblast behavior. Either directly or indirectly, these genes are vital for intricate gut tissue damage repair (174).
7 Conclusions and prospects
Despite these insights and explanations, numerous questions pertaining to insect gut immunity defense mechanisms of gut immunity in insects remain unanswered. Notably, inquiries arise regarding the mechanisms by which the immune system controls the composition and abundance of gut microorganisms. Additionally, the intricate relationship between gut microbiota and host behavior and physiological activities warrants further exploration. Furthermore, elucidating the precise mechanisms underlying gut microbiota dysbiosis remains a critical area of investigation. The differential activation of the Toll pathway in gut immunity between D. melanogaster and T. molitor raises intriguing questions, necessitating an examination of the underlying reasons for this discrepancy study. Moreover, unraveling the exact mechanisms that trigger the activation of intestinal renewal pathways in T. molitor presents an intriguing avenue for future research. Furthermore, understanding the intricate mechanisms underlying gut immunity during both pathogenic and non-pathogenic viral infections in T. molitor is of paramount importance. Exploration of the stages of infection, the duration required for gut tissue recovery following pathogen incorporation, and the precise pathogenic dose or number of infectious doses required for infection in D. melanogaster and T. molitor are crucial aspects that demand further investigation.
In the context of considering the utilization of T. molitor as a viable food source for human consumption, it is important to investigate the intricate interplay between the pathogens harbored within the T. molitor gut and the commensal microorganisms residing within the human intestinal ecosystem. A critical inquiry arises as to whether the commensal microorganisms present in T. molitor confer any advantageous attributes upon their introduction into the human intestinal milieu. Furthermore, during gut infections, T. molitor elicits the production of antimicrobial peptides as a defense mechanism against invading pathogens. An intriguing avenue for exploration emerges regarding the potential implications of these AMPs on human intestinal immunity. Could these AMPs be harnessed as a means to fortify human immune responses, potentially through targeted injections or other innovative modalities? Moreover, as T. molitor is ingested as a food source, various metabolites derived from diverse metabolic pathways are generated. It is imperative to ascertain the potential repercussions of these intermediate products on the integrity and functionality of the human intestinal tract. Meticulous investigations encompassing these concerns are promising for unraveling the intricacies underlying insect gut immune defense. Furthermore, such endeavors bear the potential for far-reaching applications spanning a vast array of disciplines.
Author contributions
YH: Conceptualization, Funding acquisition, Supervision, Writing – original draft, Writing – review & editing. SA: Conceptualization, Investigation, Visualization, Writing – original draft, Writing – review & editing. MA: Conceptualization, Validation, Writing – review & editing.
Funding
The author(s) declare financial support was received for the research, authorship, and/or publication of this article. This research was supported by “Regional Innovation Strategy (RIS)” through the National Research Foundation of Korea (NRF) funded by the Ministry of Education (MOE) (2021RIS-002), and also by the Basic Science Research Program through the National Research Foundation of Korea (NRF) funded by the Ministry of Science, ICT and Future Planning (Grant No. 2022R1A2C1013108).
Conflict of interest
The authors declare that the research was conducted in the absence of any commercial or financial relationships that could be construed as a potential conflict of interest.
Publisher’s note
All claims expressed in this article are solely those of the authors and do not necessarily represent those of their affiliated organizations, or those of the publisher, the editors and the reviewers. Any product that may be evaluated in this article, or claim that may be made by its manufacturer, is not guaranteed or endorsed by the publisher.
Glossary
References
1. Miguel-Aliaga I, Jasper H, Lemaitre B. Anatomy and physiology of the digestive tract of drosophila melanogaster. Genetics (2018) 210:357–96. doi: 10.1534/genetics.118.300224
2. Zeng T, Jaffar S, Xu Y, Qi Y. The intestinal immune defense system in insects. Int J Mol Sci (2022). doi: 10.3390/ijms232315132
3. Kuraishi T, Binggeli O, Opota O, Buchon N, Lemaitre B. Genetic evidence for a protective role of the peritrophic matrix against intestinal bacterial infection in Drosophila melanogaster. Proc Natl Acad Sci United States America (2011) 108:15966–71. doi: 10.1073/pnas.1105994108
4. Syed ZA, Härd T, Uv A, van Dijk-Härd IF. A potential role for Drosophila mucins in development and physiology. PloS One (2008) 3:e3041. doi: 10.1371/journal.pone.0003041
5. Simões ML, Gonçalves L, Silveira H. Hemozoin activates the innate immune system and reduces Plasmodium berghei infection in Anopheles Gambiae. Parasites Vectors (2015) 8:12. doi: 10.1186/s13071-014-0619-y
6. Jang S, Mergaert P, Ohbayashi T, Ishigami K, Shigenobu S, Itoh H, et al. Dual oxidase enables insect gut symbiosis by mediating respiratory network formation. Proc Natl Acad Sci United States America (2021) 118. doi: 10.1073/pnas.2020922118
7. Lu A, Zhang Q, Zhang J, Yang B, Wu K, Xie W, et al. Insect prophenoloxidase: the view beyond immunity. Front Physiol (2014) 5:252. doi: 10.3389/fphys.2014.00252
8. Bang IS. JAK/STAT signaling in insect innate immunity. Entomological Res (2019) 49:339–53. doi: 10.1111/1748-5967.12384
9. Zhang X, Zhang F, Lu X. Diversity and functional roles of the gut microbiota in lepidopteran insects. Microorganisms (2022) 10. doi: 10.3390/microorganisms10061234
10. Duplais C, Sarou-Kanian V, Massiot D, Hassan A, Perrone B, Estevez Y, et al. Gut bacteria are essential for normal cuticle development in herbivorous turtle ants. Nat Commun (2021) 12:676. doi: 10.1038/s41467-021-21065-y
11. Bai S, Yao Z, Raza MF, Cai Z, Zhang H. Regulatory mechanisms of microbial homeostasis in insect gut. Insect Sci (2021) 28:286–301. doi: 10.1111/1744-7917.12868
12. Napoleão TH, Albuquerque LP, Santos ND, Nova IC, Lima TA, Paiva PM, et al. Insect midgut structures and molecules as targets of plant-derived protease inhibitors and lectins. Pest Manage Sci (2019) 75:1212–22. doi: 10.1002/ps.5233
13. Ma E, Zhu Y, Liu Z, Wei T, Wang P, Cheng G. Interaction of viruses with the insect intestine. Annu Rev Virol (2021) 8:115–31. doi: 10.1146/annurev-virology-091919-100543
14. Smith CC, Srygley RB, Healy F, Swaminath K, Mueller UG. Spatial structure of the mormon cricket gut microbiome and its predicted contribution to nutrition and immune function. Front Microbiol (2017) 8:801. doi: 10.3389/fmicb.2017.00801
15. Subta P, Yodsuwan P, Yongsawas R, In-On A, Warrit N, Panha S, et al. Bacterial communities in three parts of intestinal tracts of carpenter bees (Xylocopa tenuiscapa). Insects (2020). doi: 10.3390/insects11080497
16. Chew YM, Lye S, Md. Salleh M, Yahya A. 16S rRNA metagenomic analysis of the symbiotic community structures of bacteria in foregut, midgut, and hindgut of the wood-feeding termite Bulbitermes sp. Symbiosis (2018) 76:187–97. doi: 10.1007/s13199-018-0544-5
17. Sharma P, Sharma S, Maurya RK, Das De T, Thomas T, Lata S, et al. Salivary glands harbor more diverse microbial communities than gut in Anopheles culicifacies. Parasites Vectors (2014) 7:235. doi: 10.1186/1756-3305-7-235
18. Lanan MC, Rodrigues PA, Agellon A, Jansma P, Wheeler DE. A bacterial filter protects and structures the gut microbiome of an insect. ISME J (2016) 10:1866–76. doi: 10.1038/ismej.2015.264
19. Abou El Asrar R, Cools D, Vanden Broeck J. Role of peptide hormones in insect gut physiology. Curr Opin Insect Sci (2020) 41:71–8. doi: 10.1016/j.cois.2020.07.004
20. Singh SR, Zeng X, Zheng Z, Hou SX. The adult Drosophila gastric and stomach organs are maintained by a multipotent stem cell pool at the foregut/midgut junction in the cardia (proventriculus). Cell Cycle (Georgetown Tex.) (2011) 10:1109–20. doi: 10.4161/cc.10.7.14830
21. Roelfstra L, Vlimant M, Betschart B, Pfister K, Diehl PA. Light and electron microscopy studies of the midgut and salivary glands of second and third instars of the horse stomach bot, Gasterophilus intestinalis. Med veterinary entomology (2010) 24:236–49. doi: 10.1111/j.1365-2915.2010.00881.x
22. Bonelli M, Bruno D, Caccia S, Sgambetterra G, Cappellozza S, Jucker C, et al. Structural and functional characterization of hermetia illucens larval midgut. Front Physiol (2019) 10:204. doi: 10.3389/fphys.2019.00204
23. Buchon N, Osman D, David FP, Fang HY, Boquete JP, Deplancke B, et al. Morphological and molecular characterization of adult midgut compartmentalization in Drosophila. Cell Rep (2013) 3:1725–38. doi: 10.1016/j.celrep.2013.04.001
24. Tokuda G, Watanabe H, Hojo M, Fujita A, Makiya H, Miyagi M, et al. Cellulolytic environment in the midgut of the wood-feeding higher termite Nasutitermes takasagoensis. J Insect Physiol (2012) 58:147–54. doi: 10.1016/j.jinsphys.2011.10.012
25. Shi X, Liu X, Silver K, Zhu KY, Zhang J. Lethal giant larvae gene is required for normal nymphal development and midgut morphogenesis in Locusta migratoria. Insect Sci (2022) 29:1017–29. doi: 10.1111/1744-7917.12996
26. Jiang H, Edgar BA. Intestinal stem cells in the adult Drosophila midgut. Exp Cell Res (2011) 317:2780–8. doi: 10.1016/j.yexcr.2011.07.020
27. Shao Q, Yang B, Xu Q, Li X, Lu Z, Wang C, et al. Hindgut innate immunity and regulation of fecal microbiota through melanization in insects. J Biol Chem (2012) 287:14270–9. doi: 10.1074/jbc.M112.354548
28. Kuraishi T, Hori A, Kurata S. Host-microbe interactions in the gut of Drosophila melanogaster. Front Physiol (2013) 4:375. doi: 10.3389/fphys.2013.00375
29. Merzendorfer H, Kelkenberg M, Muthukrishnan S. Peritrophic matrices. In: Extracellular composite matrices in arthropods (2016). p. 255–324. doi: 10.1007/978-3-319-40740-1_8pp
30. Rodgers FH, Gendrin M, Wyer CAS, Christophides GK. Microbiota-induced peritrophic matrix regulates midgut homeostasis and prevents systemic infection of malaria vector mosquitoes. PloS Pathog (2017) 13:e1006391. doi: 10.1371/journal.ppat.1006391
31. Talyuli OAC, Oliveira JHM, Bottino-Rojas V, Silveira GO, Alvarenga PH, Barletta ABF, et al. The Aedes aEgypti peritrophic matrix controls arbovirus vector competence through HPx1, a heme-induced peroxidase. PloS Pathog (2023) 19:e1011149. doi: 10.1371/journal.ppat.1011149
32. Pigeyre L, Schatz M, Ravallec M, Gasmi L, Nègre N, Clouet C, et al. Interaction of a densovirus with glycans of the peritrophic matrix mediates oral infection of the lepidopteran pest spodoptera frugiperda. Viruses (2019) 11. doi: 10.3390/v11090870
33. Hegedus DD, Toprak U, Erlandson M. Peritrophic matrix formation. J Insect Physiol (2019) 117:103898. doi: 10.1016/j.jinsphys.2019.103898
34. Wu K, Yang B, Huang W, Dobens L, Song H, Ling E. Gut immunity in Lepidopteran insects. Dev Comp Immunol (2016) 64:65–74. doi: 10.1016/j.dci.2016.02.010
35. Dias RO, Cardoso C, Pimentel AC, Damasceno TF, Ferreira C, Terra WR. The roles of mucus-forming mucins, peritrophins and peritrophins with mucin domains in the insect midgut. Insect Mol Biol (2018) 27:46–60. doi: 10.1111/imb.12340
36. Syed ZA, Zhang L, Ten Hagen KG. In vivo models of mucin biosynthesis and function. Advanced Drug delivery Rev (2022) 184:114182. doi: 10.1016/j.addr.2022.114182
37. Moriyama M, Hayashi T, Fukatsu T. A mucin protein predominantly expressed in the female-specific symbiotic organ of the stinkbug Plautia stali. Sci Rep (2022) 12:7782. doi: 10.1038/s41598-022-11895-1
38. Fuzita FJ, Palmisano G, Pimenta DC, Terra WR, Ferreira C. A proteomic approach to identify digestive enzymes, their exocytic and microapocrine secretory routes and their compartmentalization in the midgut of Spodoptera frugiperda. Comp Biochem Physiol Biochem Mol Biol (2022) 257:110670. doi: 10.1016/j.cbpb.2021.110670
39. Terra WR, Barroso IG, Dias RO, Ferreira C. Molecular physiology of insect midgut. (2019). pp. 117–63. doi: 10.1016/bs.aiip.2019.01.004pp.
40. Jasper H. Intestinal stem cell aging: origins and interventions. Annu Rev Physiol (2020) 82:203–26. doi: 10.1146/annurev-physiol-021119-034359
41. Funk MC, Zhou J, Boutros M. Ageing, metabolism and the intestine. EMBO Rep (2020) 21:e50047. doi: 10.15252/embr.202050047
42. Liu X, Hodgson JJ, Buchon N. Drosophila as a model for homeostatic, antibacterial, and antiviral mechanisms in the gut. PloS Pathog (2017) 13:e1006277. doi: 10.1371/journal.ppat.1006277
43. Buchon N, Broderick NA, Lemaitre B. Gut homeostasis in a microbial world: insights from Drosophila melanogaster. Nat Rev Microbiol (2013) 11:615–26. doi: 10.1038/nrmicro3074
44. Capo F, Wilson A, Di Cara F. The intestine of drosophila melanogaster: an emerging versatile model system to study intestinal epithelial homeostasis and host-microbial interactions in humans. Microorganisms (2019). doi: 10.3390/microorganisms7090336
45. Lee J-H, Lee K-A, Lee W-J. Microbiota, gut physiology, and insect immunity. In: Insect immunity (2017). p. 111–38. doi: 10.1016/bs.aiip.2016.11.001pp
46. Erkosar B, Leulier F. Transient adult microbiota, gut homeostasis and longevity: novel insights from the Drosophila model. FEBS Lett (2014) 588:4250–7. doi: 10.1016/j.febslet.2014.06.041
47. Zhu C, Guan F, Wang C, Jin LH. The protective effects of Rhodiola crenulata extracts on Drosophila melanogaster gut immunity induced by bacteria and SDS toxicity. Phytotherapy research: PTR (2014) 28:1861–6. doi: 10.1002/ptr.5215
48. Solomon GM, Dodangoda H, McCarthy-Walker T, Ntim-Gyakari R, Newell PD. The microbiota of Drosophila suzukii influences the larval development of Drosophila melanogaster. PeerJ (2019) 7:e8097. doi: 10.7717/peerj.8097
50. Newell PD, Douglas AE. Interspecies interactions determine the impact of the gut microbiota on nutrient allocation in Drosophila melanogaster. Appl Environ Microbiol (2014) 80:788–96. doi: 10.1128/aem.02742-13
51. Yamauchi T, Oi A, Kosakamoto H, Akuzawa-Tokita Y, Murakami T, Mori H, et al. Gut bacterial species distinctively impact host purine metabolites during aging in drosophila. iScience (2020) 23:101477. doi: 10.1016/j.isci.2020.101477
52. Huang JH, Douglas AE. Consumption of dietary sugar by gut bacteria determines Drosophila lipid content. Biol Lett (2015) 11:20150469. doi: 10.1098/rsbl.2015.0469
53. Dobson AJ, Chaston JM, Newell PD, Donahue L, Hermann SL, Sannino DR, et al. Host genetic determinants of microbiota-dependent nutrition revealed by genome-wide analysis of Drosophila melanogaster. Nat Commun (2015) 6:6312. doi: 10.1038/ncomms7312
54. Henry Y, Tarapacki P, Colinet H. Larval density affects phenotype and surrounding bacterial community without altering gut microbiota in Drosophila melanogaster. FEMS Microbiol Ecol (2020) 96. doi: 10.1093/femsec/fiaa055
55. Dodge R, Jones EW, Zhu H, Obadia B, Martinez DJ, Wang C, et al. A symbiotic physical niche in Drosophila melanogaster regulates stable association of a multi-species gut microbiota. Nat Commun (2023) 14:1557. doi: 10.1038/s41467-023-36942-x
56. Blum JE, Fischer CN, Miles J, Handelsman J. Frequent replenishment sustains the beneficial microbiome of Drosophila melanogaster. mBio (2013) 4:e00860–00813. doi: 10.1128/mBio.00860-13
57. Labachyan KE, Kiani D, Sevrioukov EA, Schriner SE, Jafari M. The impact of Rhodiola rosea on the gut microbial community of Drosophila melanogaster. Gut Pathog (2018) 10:12. doi: 10.1186/s13099-018-0239-8
58. Ma D, Storelli G, Mitchell M, Leulier F. Studying host-microbiota mutualism in Drosophila: Harnessing the power of gnotobiotic flies. Biomed J (2015) 38:285–93. doi: 10.4103/2319-4170.158620
59. Lee JH, Lee KA, Lee WJ. Drosophila as a model system for deciphering the ‘host physiology-nutrition-microbiome’ axis. Curr Opin Insect Sci (2020) 41:112–9. doi: 10.1016/j.cois.2020.09.005
60. Wong AC, Wang QP, Morimoto J, Senior AM, Lihoreau M, Neely GG, et al. Gut microbiota modifies olfactory-guided microbial preferences and foraging decisions in drosophila. Curr biology: CB (2017) 27:2397–2404.e2394. doi: 10.1016/j.cub.2017.07.022
61. Silva V, Palacios-Muñoz A, Okray Z, Adair KL, Waddell S, Douglas AE, et al. The impact of the gut microbiome on memory and sleep in Drosophila. J Exp Biol (2021) 224. doi: 10.1242/jeb.233619
62. Jia Y, Jin S, Hu K, Geng L, Han C, Kang R, et al. Gut microbiome modulates Drosophila aggression through octopamine signaling. Nat Commun (2021) 12:2698. doi: 10.1038/s41467-021-23041-y
63. Douglas AE. Drosophila and its gut microbes: a model for drug-microbiome interactions. Drug Discovery Today Dis Models (2018) 28:43–9. doi: 10.1016/j.ddmod.2019.08.004
64. Grenier T, Leulier F. How commensal microbes shape the physiology of Drosophila melanogaster. Curr Opin Insect Sci (2020) 41:92–9. doi: 10.1016/j.cois.2020.08.002
65. Lesperance DN, Broderick NA. Microbiomes as modulators of Drosophila melanogaster homeostasis and disease. Curr Opin Insect Sci (2020) 39:84–90. doi: 10.1016/j.cois.2020.03.003
66. Fast D, Kostiuk B, Foley E, Pukatzki S. Commensal pathogen competition impacts host viability. Proc Natl Acad Sci United States America (2018) 115:7099–104. doi: 10.1073/pnas.1802165115
68. Su W, Liu J, Bai P, Ma B, Liu W. Pathogenic fungi-induced susceptibility is mitigated by mutual Lactobacillus plantarum in the Drosophila melanogaster model. BMC Microbiol (2019) 19:302. doi: 10.1186/s12866-019-1686-1
69. Mistry R, Kounatidis I, Ligoxygakis P. Interaction between familial transmission and a constitutively active immune system shapes gut microbiota in drosophila melanogaster. Genetics (2017) 206:889–904. doi: 10.1534/genetics.116.190215
70. Marra A, Hanson MA, Kondo S, Erkosar B, Lemaitre B. Drosophila antimicrobial peptides and lysozymes regulate gut microbiota composition and abundance. mBio (2021) 12:e0082421. doi: 10.1128/mBio.00824-21
71. Jang S, Kikuchi Y. Impact of the insect gut microbiota on ecology, evolution, and industry. Curr Opin Insect Sci (2020) 41:33–9. doi: 10.1016/j.cois.2020.06.004
72. Li L, Xie B, Dong C, Wang M, Liu H. Can closed artificial ecosystem have an impact on insect microbial community? A case study of yellow mealworm (Tenebrio molitor L.). Ecol Eng (2016) 86:183–9. doi: 10.1016/j.ecoleng.2015.09.015
73. Wang Y, Zhang Y. Investigation of Gut-Associated Bacteria inTenebrio molitor(Coleoptera: Tenebrionidae) Larvae Using Culture-Dependent and DGGE Methods. Ann Entomological Soc America (2015) 108:941–9. doi: 10.1093/aesa/sav079
75. An R, Liu C, Wang J, Jia P. Recent advances in degradation of polymer plastics by insects inhabiting microorganisms. Polymers (2023). doi: 10.3390/polym15051307
76. Brandon AM, Garcia AM, Khlystov NA, Wu WM, Criddle CS. Enhanced bioavailability and microbial biodegradation of polystyrene in an enrichment derived from the gut microbiome of tenebrio molitor (Mealworm larvae). Environ Sci Technol (2021) 55:2027–36. doi: 10.1021/acs.est.0c04952
77. Available at: https://ssm.com/abstract=4146181.
78. Wang X, Tang T. Effects of polystyrene diet on the growth and development of tenebrio molitor. Toxics (2022) 10. doi: 10.3390/toxics10100608
79. Zhong Z, Nong W, Xie Y, Hui JHL, Chu LM. Long-term effect of plastic feeding on growth and transcriptomic response of mealworms (Tenebrio molitor L.). Chemosphere (2022) 287:132063. doi: 10.1016/j.chemosphere.2021.132063
80. Lou Y, Li Y, Lu B, Liu Q, Yang SS, Liu B, et al. Response of the yellow mealworm (Tenebrio molitor) gut microbiome to diet shifts during polystyrene and polyethylene biodegradation. J hazardous materials (2021) 416:126222. doi: 10.1016/j.jhazmat.2021.126222
81. Tsochatzis E, Berggreen IE, Tedeschi F, Ntrallou K, Gika H, Corredig M. Gut Microbiome and Degradation Product Formation during Biodegradation of Expanded Polystyrene by Mealworm Larvae under Different Feeding Strategies. Molecules (Basel Switzerland) (2021) 26. doi: 10.3390/molecules26247568
82. Orts JM, Parrado J, Pascual JA, Orts A, Cuartero J, Tejada M, et al. Polyurethane Foam Residue Biodegradation through the Tenebrio molitor Digestive Tract: Microbial Communities and Enzymatic Activity. Polymers (2022). doi: 10.3390/polym15010204
83. Ballen-Segura MA, López-Ramírez NA, Peña-Pascagaza PM. Tenebrio molitor and its gut bacteria growth in polystyrene (PS) presence as the sole source carbon. Universitas Scientiarum (2020) 25:37–53. doi: 10.11144/Javeriana.SC25-1.tmai
84. Peng BY, Sun Y, Xiao S, Chen J, Zhou X, Wu WM, et al. Influence of polymer size on polystyrene biodegradation in mealworms (Tenebrio molitor): responses of depolymerization pattern, gut microbiome, and metabolome to polymers with low to ultrahigh molecular weight. Environ Sci Technol (2022) 56:17310–20. doi: 10.1021/acs.est.2c06260
85. Available at: https://ssm.com/abstract=440977.
86. Przemieniecki SW, Kosewska A, Ciesielski S, Kosewska O. Changes in the gut microbiome and enzymatic profile of Tenebrio molitor larvae biodegrading cellulose, polyethylene and polystyrene waste. Environ pollut (Barking Essex: 1987) (2020) 256:113265. doi: 10.1016/j.envpol.2019.113265
87. Dahal S, Jensen AB, Lecocq A. Effect of Probiotics on Tenebrio molitor Larval Development and Resistance against the Fungal Pathogen Metarhizium brunneum. Insects (2022) 13. doi: 10.3390/insects13121114
88. Lecocq A, Natsopoulou ME, Berggreen IE, Eilenberg J, Heckmann LHL, Nielsen HV, et al. Probiotic properties of an indigenous Pediococcus pentosaceus strain on Tenebrio molitor larval growth and survival. J Insects as Food Feed (2021) 7:975–86. doi: 10.3920/jiff2020.0156
89. Carvalho NM, Teixeira F, Silva S, Madureira AR, Pintado ME. Potential prebiotic activity of Tenebrio molitor insect flour using an optimized. Vitro gut microbiota Model Food Funct (2019) 10:3909–22. doi: 10.1039/c8fo01536h
90. Poveda J, Jiménez-Gómez A, Saati-Santamaría Z, Usategui-Martín R, Rivas R, García-Fraile P. Mealworm frass as a potential biofertilizer and abiotic stress tolerance-inductor in plants. Appl Soil Ecol (2019) 142:110–22. doi: 10.1016/j.apsoil.2019.04.016
91. Slowik AR, Herren P, Bessette E, Lim FS, Hernández-Pelegrín L, Savio C. Harmful and beneficial symbionts of Tenebrio molitor and their implications for disease management. J Insects as Food Feed (2023). doi: 10.3920/jiff2022.0171
92. Fredensborg BL, Fossdal Í Kálvalíð I, Johannesen TB, Stensvold CR, Nielsen HV, Kapel CMO. Parasites modulate the gut-microbiome in insects: A proof-of-concept study. PloS One (2020) 15:e0227561. doi: 10.1371/journal.pone.0227561
93. Vigneron A, Jehan C, Rigaud T, Moret Y. Immune defenses of a beneficial pest: the mealworm beetle, tenebrio molitor. Front Physiol (2019) 10:138. doi: 10.3389/fphys.2019.00138
94. Ali Mohammadie Kojour M, Han YS, Jo YH. An overview of insect innate immunity. Entomological Res (2020) 50:282–91. doi: 10.1111/1748-5967.12437
95. Lu Y, Su F, Li Q, Zhang J, Li Y, Tang T, et al. Pattern recognition receptors in Drosophila immune responses. Dev Comp Immunol (2020) 102:103468.
96. El Chamy L, Matt N, Ntwasa M, Reichhart JM. The multilayered innate immune defense of the gut. Biomed J (2015) 38:276–84. doi: 10.4103/2319-4170.158621
97. Myllymaki H, Valanne S, Ramet M. The Drosophila imd signaling pathway. J Immunol (2014) 192:3455–62. doi: 10.4049/jimmunol.1303309
98. Zhai Z, Boquete JP, Lemaitre B. Cell-Specific Imd-NF-κB Responses Enable Simultaneous Antibacterial Immunity and Intestinal Epithelial Cell Shedding upon Bacterial Infection. Immunity (2018) 48:897–910.e897. doi: 10.1016/j.immuni.2018.04.010
99. Kamareddine L, Robins WP, Berkey CD, Mekalanos JJ, Watnick PI. The Drosophila Immune Deficiency Pathway Modulates Enteroendocrine Function and Host Metabolism. Cell Metab (2018) 28:449–462.e445. doi: 10.1016/j.cmet.2018.05.026
100. Bosco-Drayon V, Poidevin M, Boneca IG, Narbonne-Reveau K, Royet J, Charroux B. Peptidoglycan sensing by the receptor PGRP-LE in the Drosophila gut induces immune responses to infectious bacteria and tolerance to microbiota. Cell Host Microbe (2012) 12:153–65. doi: 10.1016/j.chom.2012.06.002
101. Wen Y, He Z, Xu T, Jiao Y, Liu X, Wang YF, et al. Ingestion of killed bacteria activates antimicrobial peptide genes in Drosophila melanogaster and protects flies from septic infection. Dev Comp Immunol (2019) 95:10–8. doi: 10.1016/j.dci.2019.02.001
102. Ferrandon D. The complementary facets of epithelial host defenses in the genetic model organism Drosophila melanogaster: from resistance to resilience. Curr Opin Immunol (2013) 25:59–70. doi: 10.1016/j.coi.2012.11.008
103. Limmer S, Haller S, Drenkard E, Lee J, Yu S, Kocks C, et al. Pseudomonas aeruginosa RhlR is required to neutralize the cellular immune response in a Drosophila melanogaster oral infection model. Proc Natl Acad Sci United States America (2011) 108:17378–83. doi: 10.1073/pnas.1114907108
104. Hori A, Kurata S, Kuraishi T. Unexpected role of the IMD pathway in Drosophila gut defense against Staphylococcus aureus. Biochem Biophys Res Commun (2018) 495:395–400. doi: 10.1016/j.bbrc.2017.11.004
105. Watnick PI, Jugder BE. Microbial Control of Intestinal Homeostasis via Enteroendocrine Cell Innate Immune Signaling. Trends Microbiol (2020) 28:141–9. doi: 10.1016/j.tim.2019.09.005
106. Jugder BE, Kamareddine L, Watnick PI. Microbiota-derived acetate activates intestinal innate immunity via the Tip60 histone acetyltransferase complex. Immunity (2021) 54:1683–1697.e1683. doi: 10.1016/j.immuni.2021.05.017
107. Kosakamoto H, Yamauchi T, Akuzawa-Tokita Y, Nishimura K, Soga T, Murakami T, et al. Local Necrotic Cells Trigger Systemic Immune Activation via Gut Microbiome Dysbiosis in Drosophila. Cell Rep (2020) 32:107938. doi: 10.1016/j.celrep.2020.107938
108. Wang Z, Hang S, Purdy AE, Watnick PI. Mutations in the IMD pathway and mustard counter Vibrio cholerae suppression of intestinal stem cell division in Drosophila. mBio (2013) 4:e00337–00313. doi: 10.1128/mBio.00337-13
109. Jang HA, Kojour MAM, Patnaik BB, Han YS, Jo YH. Current status of immune deficiency pathway in Tenebrio molitor innate immunity. Front Immunol (2022) 13:906192.
110. Kleino A, Silverman N. The Drosophila IMD pathway in the activation of the humoral immune response. Dev Comp Immunol (2014) 42:25–35.
111. Ali Mohammadie Kojour M, Edosa TT, Jang HA, Keshavarz M, Jo YH, Han YS. Critical roles of spätzle5 in antimicrobial peptide production against Escherichia coli in Tenebrio molitor malpighian tubules. Front Immunol (2021) 12:760475.
112. Chowdhury M, Li C-F, He Z, Lu Y, Liu X-S, Wang Y-F, et al. Toll family members bind multiple Spätzle proteins and activate antimicrobial peptide gene expression in Drosophila. J Biol Chem (2019) 294:10172–81.
113. Edosa TT, Jo YH, Keshavarz M, Bae YM, Kim DH, Lee YS, et al. Tm Spz4 plays an important role in regulating the production of antimicrobial peptides in response to Escherichia coli and Candida albicans infections. Int J Mol Sci (2020) 21:1878.
114. Edosa TT, Jo YH, Keshavarz M, Bae YM, Kim DH, Lee YS, et al. Tm Spz6 is essential for regulating the immune response to Escherichia coli and Staphylococcus aureus infection in Tenebrio molitor. Insects (2020) 11:105.
115. Edosa TT, Jo YH, Keshavarz M, Kim IS, Han YS. Biosurfactants Induce Antimicrobial Peptide Production through the Activation of Tm Spatzles in Tenebrio molitor. Int J Mol Sci (2020) 21:6090.
116. Jang HA, Patnaik BB, Ali Mohammadie Kojour M, Kim BB, Bae YM, Park KB, et al. Tm Spz-like plays a fundamental role in response to E. coli but not S. aureus or C. albican infection in Tenebrio molitor via regulation of antimicrobial peptide production. Int J Mol Sci (2021) 22:10888.
117. Kambris Z, Brun S, Jang I-H, Nam H-J, Romeo Y, Takahashi K, et al. Drosophila immunity: a large-scale in vivo RNAi screen identifies five serine proteases required for Toll activation. Curr Biol (2006) 16:808–13.
118. Ali Mohammadie Kojour M, Jang HA, Lee YS, Jo YH, Han YS. Immunological Roles of TmToll-2 in Response to Escherichia coli Systemic Infection in Tenebrio molitor. Int J Mol Sci (2022) 23:14490.
119. Ali Mohammadie Kojour M, Jang HA, Lee YS, Jo YH, Han YS. Innate Immune Response of TmToll-3 Following Systemic Microbial Infection in Tenebrio molitor. Int J Mol Sci (2023) 24:6751.
120. Tanji T, Hu X, Weber AN, Ip YT. Toll and IMD pathways synergistically activate an innate immune response in Drosophila melanogaster. Mol Cell Biol (2007) 27:4578–88.
121. Yagi Y, Nishida Y, Ip YT. Functional analysis of Toll-related genes in Drosophila. Development Growth differentiation (2010) 52:771–83.
122. Jo YH, Kim YJ, Park KB, Seong JH, Kim SG, Park S, et al. TmCactin plays an important role in Gram-negative and-positive bacterial infection by regulating expression of 7 AMP genes in Tenebrio molitor. Sci Rep (2017) 7:46459.
123. Patnaik BB, Patnaik HH, Seo GW, Jo YH, Lee YS, Lee BL, et al. Gene structure, cDNA characterization and RNAi-based functional analysis of a myeloid differentiation factor 88 homolog in Tenebrio molitor larvae exposed to Staphylococcus aureus infection. Dev Comp Immunol (2014) 46:208–21.
124. Keshavarz M, Jo YH, Park KB, Ko HJ, Edosa TT, Lee YS, et al. Tm DorX2 positively regulates antimicrobial peptides in Tenebrio molitor gut, fat body, and hemocytes in response to bacterial and fungal infection. Sci Rep (2019) 9:16878.
125. Combe BE, Defaye A, Bozonnet N, Puthier D, Royet J, Leulier F. Drosophila microbiota modulates host metabolic gene expression via IMD/NF-κB signaling. PloS One (2014) 9:e94729.
126. Tapadia MG, Verma P. Immune response and anti-microbial peptides expression in Malpighian tubules of Drosophila melanogaster is under developmental regulation. PloS One (2012) 7:e40714.
127. Ko HJ, Am Jang H, Park KB, Kim CE, Patnaik BB, Lee YS, et al. IKKβ regulates antimicrobial innate immune responses in the yellow mealworm, Tenebrio molitor. Dev Comp Immunol (2023) 104761.
128. Schmidt RL, Trejo TR, Plummer TB, Platt JL, Tang AH. Infection-induced proteolysis of PGRP-LC controls the IMD activation and melanization cascades in Drosophila. FASEB J (2008) 22:918–29.
129. Wen Y, He Z, Xu T, Jiao Y, Liu X, Wang Y-F, et al. Ingestion of killed bacteria activates antimicrobial peptide genes in Drosophila melanogaster and protects flies from septic infection. Dev Comp Immunol (2019) 95:10–8.
130. Jang HA, Park KB, Kim BB, Ali Mohammadie Kojour M, Bae YM, Baliarsingh S, et al. In silico identification and expression analyses of Defensin genes in the mealworm beetle Tenebrio molitor. Entomological Res (2020) 50:575–85.
131. Keshavarz M, Jo YH, Edosa TT, Bae YM, Han YS. Tm PGRP-SA regulates antimicrobial response to bacteria and fungi in the fat body and gut of Tenebrio molitor. Int J Mol Sci (2020) 21:2113.
132. Kambris Z, Hoffmann JA, Imler J-L, Capovilla M. Tissue and stage-specific expression of the Tolls in Drosophila embryos. Gene Expression Patterns (2002) 2:311–7.
133. Park S, Jo YH, Park KB, Ko HJ, Kim CE, Bae YM, et al. TmToll-7 plays a crucial role in innate immune responses against Gram-negative bacteria by regulating 5 AMP genes in Tenebrio molitor. Front Immunol (2019) 10:310.
134. Yang YT, Lee MR, Lee SJ, Kim S, Nai YS, Kim JS. Tenebrio molitor Gram-negative-binding protein 3 (TmGNBP3) is essential for inducing downstream antifungal Tenecin 1 gene expression against infection with Beauveria bassiana JEF-007. Insect Sci (2018) 25:969–77.
135. Ali Mohammadie Kojour M, Edosa TT, Jang HA, Keshavarz M, Jo YH, Han YS. Critical Roles of Spätzle5 in Antimicrobial Peptide Production Against Escherichia coli in Tenebrio molitor Malpighian Tubules. Front Immunol (2021) 12:760475. doi: 10.3389/fimmu.2021.760475
136. Ko HJ, Jo YH, Patnaik BB, Park KB, Kim CE, Keshavarz M, et al. IKKγ/NEMO Is Required to Confer Antimicrobial Innate Immune Responses in the Yellow Mealworm, Tenebrio Molitor. Int J Mol Sci 202021. doi: 10.3390/ijms21186734
137. Jang HA, Kojour MAM, Patnaik BB, Han YS, Jo YH. Current Status of Immune Deficiency Pathway in Tenebrio molitor Innate Immunity. Front Immunol (2022) 13:906192. doi: 10.3389/fimmu.2022.906192
138. Jo YH, Patnaik BB, Hwang J, Park KB, Ko HJ, Kim CE, et al. Regulation of the expression of nine antimicrobial peptide genes by TmIMD confers resistance against Gram-negative bacteria. Sci Rep (2019) 9:10138. doi: 10.1038/s41598-019-46222-8
139. Keshavarz M, Jo YH, Edosa TT, Bae YM, Han YS. TmPGRP-SA regulates Antimicrobial Response to Bacteria and Fungi in the Fat Body and Gut of Tenebrio molitor. Int J Mol Sci (2020) 21. doi: 10.3390/ijms21062113
140. Keshavarz M, Jo YH, Edosa TT, Han YS. Tenebrio molitor PGRP-LE Plays a Critical Role in Gut Antimicrobial Peptide Production in Response to Escherichia coli. Front Physiol (2020) 11:320. doi: 10.3389/fphys.2020.00320
141. Keshavarz M, Jo YH, Patnaik BB, Park KB, Ko HJ, Kim CE, et al. TmRelish is required for regulating the antimicrobial responses to Escherichia coli and Staphylococcus aureus in Tenebrio molitor. Sci Rep (2020) 10:4258. doi: 10.1038/s41598-020-61157-1
142. Lemaitre B, Miguel-Aliaga I. The digestive tract of Drosophila melanogaster. Annu Rev Genet (2013) 47:377–404. doi: 10.1146/annurev-genet-111212-133343
143. Myllymaki H, Ramet M. JAK/STAT pathway in Drosophila immunity. Scand J Immunol (2014) 79:377–85. doi: 10.1111/sji.12170
144. Kietz C, Meinander A. Drosophila caspases as guardians of host-microbe interactions. Cell Death Differentiation (2023) 30:227–36.
145. Liu X, Nagy P, Bonfini A, Houtz P, Bing XL, Yang X, et al. Microbes affect gut epithelial cell composition through immune-dependent regulation of intestinal stem cell differentiation. Cell Rep (2022) 38:110572. doi: 10.1016/j.celrep.2022.110572
146. Buchon N, Broderick NA, Lemaitre B. Gut homeostasis in a microbial world: insights from Drosophila melanogaster. Nat Rev Microbiol (2013) 11:615–26.
147. Charroux B, Royet J. Drosophila immune response: From systemic antimicrobial peptide production in fat body cells to local defense in the intestinal tract. Fly (Austin) (2010) 4:40–7. doi: 10.4161/fly.4.1.10810
148. You H, Lee WJ, Lee WJ. Homeostasis between gut-associated microorganisms and the immune system in Drosophila. Curr Opin Immunol (2014) 30:48–53. doi: 10.1016/j.coi.2014.06.006
149. Ha EM, Lee KA, Park SH, Kim SH, Nam HJ, Lee HY, et al. Regulation of DUOX by the Galphaq-phospholipase Cbeta-Ca2+ pathway in Drosophila gut immunity. Dev Cell (2009) 16:386–97. doi: 10.1016/j.devcel.2008.12.015
150. Lee KA, Kim B, Bhin J, Kim DH, You H, Kim EK, et al. Bacterial uracil modulates Drosophila DUOX-dependent gut immunity via Hedgehog-induced signaling endosomes. Cell Host Microbe (2015) 17:191–204. doi: 10.1016/j.chom.2014.12.012
151. Lee KA, Cho KC, Kim B, Jang IH, Nam K, Kwon YE, et al. Inflammation-Modulated Metabolic Reprogramming Is Required for DUOX-Dependent Gut Immunity in Drosophila. Cell Host Microbe (2018) 23:338–352.e335. doi: 10.1016/j.chom.2018.01.011
152. Xiao X, Yang L, Pang X, Zhang R, Zhu Y, Wang P, et al. Mesh-Duox pathway regulates homeostasis in the insect gut. Nat Microbiol (2017) 2:17020. doi: 10.1038/nmicrobiol.2017.20
153. Lee KA, Kim SH, Kim EK, Ha EM, You H, Kim B, et al. Bacterial-derived uracil as a modulator of mucosal immunity and gut-microbe homeostasis in Drosophila. Cell (2013) 153:797–811. doi: 10.1016/j.cell.2013.04.009
154. Tafesh-Edwards G, Eleftherianos I. JNK signaling in Drosophila immunity and homeostasis. Immunol Lett (2020) 226:7–11.
155. Tafesh-Edwards G, Eleftherianos I. JNK signaling in Drosophila immunity and homeostasis. Immunol Lett (2020) 226:7–11. doi: 10.1016/j.imlet.2020.06.017
156. Zhang X, Jin Q, Jin LH. High sugar diet disrupts gut homeostasis though JNK and STAT pathways in Drosophila. Biochem Biophys Res Commun (2017) 487:910–6. doi: 10.1016/j.bbrc.2017.04.156
157. Ahn HM, Lee KS, Lee DS, Yu K. JNK/FOXO mediated PeroxiredoxinV expression regulates redox homeostasis during Drosophila melanogaster gut infection. Dev Comp Immunol (2012) 38:466–73. doi: 10.1016/j.dci.2012.07.002
158. Sansone CL, Cohen J, Yasunaga A, Xu J, Osborn G, Subramanian H, et al. Microbiota-Dependent Priming of Antiviral Intestinal Immunity in Drosophila. Cell Host Microbe (2015) 18:571–81. doi: 10.1016/j.chom.2015.10.010
159. Chen J, Xie C, Tian L, Hong L, Wu X, Han J. Participation of the p38 pathway in Drosophila host defense against pathogenic bacteria and fungi. Proc Natl Acad Sci United States America (2010) 107:20774–9. doi: 10.1073/pnas.1009223107
160. Ryu K, Park J, Kurokawa K, Matsushita M, Lee B. The molecular activation and regulation mechanisms of proteolytic Toll signaling cascade in insect innate immunity. Invertebrate Survival J (2010) 7:181–91.
161. Kietz C, Meinander A. Drosophila caspases as guardians of host-microbe interactions. Cell Death differentiation (2023) 30:227–36. doi: 10.1038/s41418-022-01038-4
162. Jang HA, Patnaik BB, Ali Mohammadie Kojour M, Kim BB, Bae YM, Park KB, et al. TmSpz-like Plays a Fundamental Role in Response to E. coli but Not S. aureus or C. albican Infection in Tenebrio molitor via Regulation of Antimicrobial Peptide Production. Int J Mol Sci (2021) 22. doi: 10.3390/ijms221910888
163. Jang HA, Park KB, Kim BB, Ali Mohammadie Kojour M, Bae YM, Baliarsingh S, et al. Bacterial but not fungal challenge up-regulates the transcription of Coleoptericin genes in Tenebrio molitor. Entomological Res (2020) 50:440–9. doi: 10.1111/1748-5967.12465
164. Ali Mohammadie Kojour M, Jang HA, Lee YS, Jo YH, Han YS. Immunological Roles of TmToll-2 in Response to Escherichia coli Systemic Infection in Tenebrio molitor. Int J Mol Sci (2022) 23. doi: 10.3390/ijms232214490
165. Ali Mohammadie Kojour M, Jang HA, Lee YS, Jo YH, Han YS. Innate Immune Response of TmToll-3 Following Systemic Microbial Infection in Tenebrio molitor. Int J Mol Sci 202324. doi: 10.3390/ijms24076751
166. Nászai M, Carroll LR, Cordero JB. Intestinal stem cell proliferation and epithelial homeostasis in the adult Drosophila midgut. Insect Biochem Mol Biol (2015) 67:9–14. doi: 10.1016/j.ibmb.2015.05.016
167. Lee J-H, Lee K-A, Lee W-J. Microbiota, gut physiology, and insect immunity. Adv Insect Physiol (2017) 52:111–38.
168. Buchon N, Broderick NA, Chakrabarti S, Lemaitre B. Invasive and indigenous microbiota impact intestinal stem cell activity through multiple pathways in Drosophila. Genes Dev (2009) 23:2333–44. doi: 10.1101/gad.1827009
169. Chakrabarti S, Liehl P, Buchon N, Lemaitre B. Infection-induced host translational blockage inhibits immune responses and epithelial renewal in the Drosophila gut. Cell Host Microbe (2012) 12:60–70. doi: 10.1016/j.chom.2012.06.001
170. Jiang H, Patel PH, Kohlmaier A, Grenley MO, McEwen DG, Edgar BA. Cytokine/Jak/Stat signaling mediates regeneration and homeostasis in the Drosophila midgut. Cell (2009) 137:1343–55. doi: 10.1016/j.cell.2009.05.014
171. Buchon N, Broderick NA, Kuraishi T, Lemaitre B. Drosophila EGFR pathway coordinates stem cell proliferation and gut remodeling following infection. BMC Biol (2010) 8:152. doi: 10.1186/1741-7007-8-152
172. Xiang J, Bandura J, Zhang P, Jin Y, Reuter H, Edgar BA. EGFR-dependent TOR-independent endocycles support Drosophila gut epithelial regeneration. Nat Commun (2017) 8:15125. doi: 10.1038/ncomms15125
173. Shaw RL, Kohlmaier A, Polesello C, Veelken C, Edgar BA, Tapon N. The Hippo pathway regulates intestinal stem cell proliferation during Drosophila adult midgut regeneration. Development (2010) 137:4147–58. doi: 10.1242/dev.052506
Keywords: gut immunity, gut compartments, cellular composition, microbiota, immune signaling pathways, Drosophila melanogaster, Tenebrio molitor, antimicrobial peptides
Citation: Khan SA, Kojour MAM and Han YS (2023) Recent trends in insect gut immunity. Front. Immunol. 14:1272143. doi: 10.3389/fimmu.2023.1272143
Received: 03 August 2023; Accepted: 29 November 2023;
Published: 18 December 2023.
Edited by:
Qiuning Liu, Yancheng Teachers University, ChinaReviewed by:
Chan-Hee Kim, Pukyong National University, Republic of KoreaYuemei Dong, Johns Hopkins University, United States
Copyright © 2023 Khan, Kojour and Han. This is an open-access article distributed under the terms of the Creative Commons Attribution License (CC BY). The use, distribution or reproduction in other forums is permitted, provided the original author(s) and the copyright owner(s) are credited and that the original publication in this journal is cited, in accordance with accepted academic practice. No use, distribution or reproduction is permitted which does not comply with these terms.
*Correspondence: Yeon Soo Han, aGFueXNAY2hvbm5hbS5hYy5rcg==