- 1The Affiliated Lihuili Hospital, Ningbo University, Ningbo, China
- 2Health Science Center, Ningbo University, Ningbo, China
Head and neck squamous cell carcinoma (HNSCC) rank among the most prevalent types of head and neck cancer globally. Unfortunately, a significant number of patients receive their diagnoses at advanced stages, limiting the effectiveness of available treatments. The tumor microenvironment (TME) is a pivotal player in HNSCC development, with macrophages holding a central role. Macrophages demonstrate diverse functions within the TME, both inhibiting and facilitating cancer progression. M1 macrophages are characterized by their phagocytic and immune activities, while M2 macrophages tend to promote inflammation and immunosuppression. Striking a balance between these different polarization states is essential for maintaining overall health, yet in the context of tumors, M2 macrophages typically prevail. Recent efforts have been directed at controlling the polarization states of macrophages, paving the way for novel approaches to cancer treatment. Various drugs and immunotherapies, including innovative treatments based on macrophages like engineering macrophages and CAR-M cell therapy, have been developed. This article provides an overview of the roles played by macrophages in HNSCC, explores potential therapeutic targets and strategies, and presents fresh perspectives on the future of HNSCC treatment.
1 Introduction
Head and neck squamous cell carcinoma (HNSCC) is the most prevalent form of head and neck cancer and ranks as the seventh most common cancer worldwide (1). Regrettably, most instances are detected at advanced stages, often involving locally advanced (LA) conditions or distant metastasis (DM). Despite the availability of various treatment options, such as surgery, radiation therapy (RT), chemotherapy (CT), and immunotherapy (IT), a significant portion (40-60%) of LA tumors eventually experience relapse or local progression. Palliative CT for metastatic and recurrent (R/M) HNSCC tumors also presents a grim prognosis (2). The tumor microenvironment (TME) refers to the immediate surroundings of HNSCC tumors during their growth or mutation, exhibiting complexity. On one hand, alterations such as cytokine production and extracellular matrix changes occur within the TME, alongside immune surveillance which identifies and attacks tumor cells, thereby inhibiting tumor growth. On the other hand, tumor cells can interact with surrounding tissues to modify nutrient supply, generate cytokines, and suppress immune responses within the TME, thus promoting their own survival and development (3, 4).
Most patients diagnosed as HNSCC often present with locally advanced disease, requiring multimodal treatments, including immunotherapy (5, 6). Immunotherapy involves the specific recognition and targeting of cancer cells by immune cells within the TME. The TME contains various immune cells such as macrophages, effector T cells, natural killer cells, and dendritic cells (7). Among them, macrophages constitute the largest and most critical group of innate immune cells in the TME (8) (Figure 1A). Macrophages originate from bone marrow hematopoietic stem cells and embryonic yolk sac tissue (9, 10). Under the influence of different microenvironmental stimuli, macrophages exhibit heterogeneity and plasticity, allowing them to adapt their characteristics in highly specialized ways to perceive and respond to their environment. While the specific phenotypes are hard to categorize, they can be simplified into two extremes with entirely different molecular phenotypes and functional characteristics: IFN-γ/lipopolysaccharide (LPS)-induced M1 macrophages and IL-4/IL-10/IL-13-induced M2 macrophages (7).
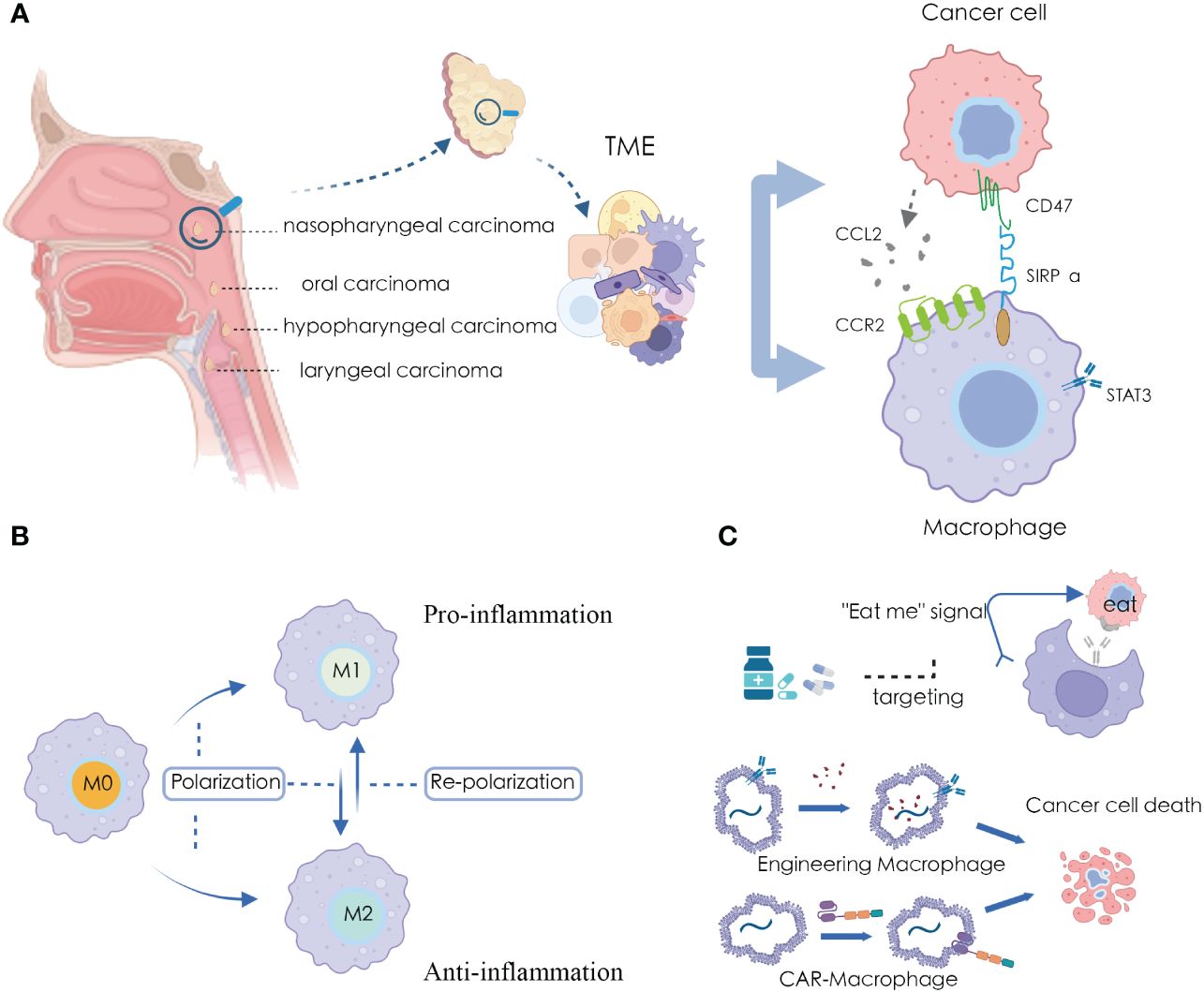
Figure 1 Macrophages and applications in HNSCC microenvironment (A) Significant crosstalk between macrophages and tumor cells in HNSCC TME; (B) The plasticity of macrophage polarization states; (C) Targeting and harnessing macrophages in disease treatment and immunotherapy.
Furthermore, tumor-associated macrophages (TAMs) refer to a type of macrophages that appear in the TME that exhibit characteristics of both M1 and M2 macrophages under different signals and stimuli (11, 12). Among these, in the TME, typical M1 activators include LPS, IL-1β, and IFN-γ, which activate macrophages to produce inflammatory factors. Conversely, M2 polarization factors such as IL-4, IL-13, IL-10, and TGF-β promote macrophage polarization towards the M2 type, exhibiting characteristics of anti-inflammatory and immune suppression (13). In HNSCC, TAMs typically display M2 macrophage features, contributing to the establishment of an immune-suppressive TME, thereby promoting tumor escape and growth (12, 14). The balance between macrophage M1 and M2 subtypes is crucial for maintaining a stable state of health in the human body. When this balance is disrupted, it can lead to disease states (15). Metabolic adaptation supports the heterogeneity of tumor-associated macrophage activities and functions, maintaining their polarization in specific environments (16, 17). In particular, in terms of energy supply, M1 TAMs primarily rely on glycolysis. The two interruptions in their TCA cycle lead to the accumulation of itaconate and succinate, resulting in the stabilization of HIF1α. This further activates the transcription of glycolytic genes, thereby maintaining the glycolytic metabolism of M1 cells (18). Conversely, M2 cells are more dependent on oxidative phosphorylation, with their TCA cycle intact and providing substrates for the electron transport chain complexes (18). Additionally, both subtypes have specific metabolic pathways that regulate lipid and amino acid metabolism, influencing their responses (19, 20).
Due to the high plasticity of macrophages, they play a significant role in various pathological processes, further exploring macrophages is considered as an alternative approach for cancer therapy (21). Recent studies have indicated that promoting M1 macrophage polarization and inhibiting M2 macrophage polarization can exert anti-tumor effects, while promoting M2 macrophage polarization and inhibiting M1 macrophage polarization can have anti-inflammatory activity (22) (Figure 1B). Several drugs have been discovered that can modulate the polarization state of macrophages for disease treatment (23, 24), and many immunotherapeutic approaches have been developed using macrophages as carriers or tools (25, 26) (Figure 1C).
This review commences by examining the roles fulfilled by macrophages within the microenvironment of HNSCC. Additionally, explain the diverse roles and conditions they play during the development of head and neck tumors. Furthermore, we elaborate on essential therapeutic targets and the most recent related treatment methodologies, with the intent of presenting novel perspectives for forthcoming research and therapeutic strategies targeting macrophages in HNSCC.
2 Macrophage involvement in the HNSCC microenvironment
2.1 Phagocytosis and secretory regulation
In the tumor microenvironment, macrophages exhibit a dual role in HNSCC. On one hand, they can suppress cancer progression through immune actions, while on the other hand, they play a pro-cancer role by affecting several features of HNSCC, such as immune evasion, promoting invasion and metastasis, participating in angiogenesis, and influencing cancer cell proliferation (27–30).
Phagocytosis is one of the most innate capabilities of macrophages. They can actively engulf abnormal cells within the body, including cancer cells. During this process, macrophages extend pseudopodia to envelop cancer cells in vesicles formed by the cell membrane, creating a structure called a phagosome. Subsequently, this phagosome is internalized within the macrophage (31). This process helps reduce the number of tumor cells, alleviate the tumor burden, and contribute to controlling tumor growth. Currently, in cancer therapy research and development, a new generation of anti-cancer therapies has emerged based on harnessing and enhancing the phagocytic ability of macrophages, such as CAR-engineered cell therapies known as CAR-macrophages (32), which may potentially reshape the landscape of HNSCC treatment in the future.
Macrophages also regulate immune responses and tumor progression by modulating their polarization states through secretory regulation, wherein they secrete cytokines (33). For example, the secretion of certain cytokines may induce macrophages to polarize towards the M1 phenotype, exhibiting characteristics that promote immune responses and anti-tumor effects, such as TNF-α, IL-6, and IL-12 (34). Conversely, other factors may lead to macrophage polarization towards the M2 phenotype, showing features of immune suppression and promoting tumor growth, such as TGF-β and IL-10 (34). Additionally, chemokines such as CXCL9, CXCL10, and CXCL11 can attract T cells and other immune cells, thereby promoting macrophage polarization towards the M1 phenotype (35). The expression levels and activities of these factors are crucial for regulating the polarization status of macrophages, thereby influencing their functions and roles within the tumor microenvironment.
In HNSCC, TAMs typically exhibit an M2-polarized state, promoting immune evasion and growth of the tumor (12). In the early stages of tumors, TAMs release nitric oxide (NO) and reactive oxygen intermediates (ROI), causing DNA damage and genetic instability (36). Afterward, they actively participate in regulating the HNSCC microenvironment through direct secretion. These macrophages can secrete factors that enhance cell migration, including epidermal growth factor, cysteine cathepsins, and matrix metalloproteinases. Through the action of these matrix-degrading enzymes, they facilitate the movement of tumor cells (37). TAMs can also suppress T cell cytotoxicity by secreting IL-10, promote regulatory T cells, leading to immune evasion and tumor proliferation (38). Moreover, they can produce factors that promote the growth of blood vessels within the tumor, such as VEGF-A, VEGF-C, and adrenomedullin, thereby supplying oxygen for tumor development (39).
2.2 Exosomes
Extracellular vesicles, also known as exosomes, serve as vesicles originating from tumor cells, immune cells, and various other cell types. They play a role in promoting tumor proliferation, invasion, migration, modulating tumor immunology, fostering angiogenesis, and reprogramming the tumor microenvironment (40). In the progression of head and neck cancer, exosomes serve as a vital means of communication between macrophages and cancer cells. Previous studies have revealed that M1 macrophages secrete exosomes, inhibiting the proliferation, migration, and invasion abilities of head and neck cancer. These exosomes can also induce apoptosis in cancer cells, and HOXA transcripts at the distal tip (HOTTIP), as a tumorigenic specific lncRNA, is a critical molecule in these exosomes, showing the same functionality when overexpressed (41).
Additionally, in oral squamous cell carcinoma, cancer cells secrete exosomes, particularly CMTM6, which induce polarization of M2 macrophages via the ERK1/2 signaling pathway, thereby promoting malignant progression of the tumor. During this process, CMTM6 also enhances the expression of PD-L1, thereby driving tumor migration and invasion (42).
Exosomes extracted from other HNSCC cell lines, including JHU011, SNU1076, and SCC-VII, can significantly induce polarization of M2 macrophages. Exosomes carrying PD-L1 and stimulating HNSCC promote the activation of regulatory T cells (aTregs), further strengthening the positive feedback loop between aTregs and M2 macrophages, ultimately leading to immune escape in tumors (43). The crosstalk mediated by exosomes between macrophages plays a significant role in the complex pathophysiology of head and neck cancer.
2.3 Macrophage polarization identification
Traditionally, in HNSCC surgical specimens, TAMs are typically detected using specific antibodies like CD68, CD80, and CD163. CD80+ corresponds to M1 type, while CD163+ corresponds to M2 type (44).
Recently, there has been a shift towards using the mutually exclusive gene expression of CXCL9 and SPP1, along with their ratio, as key features and standards for assessing the polarization level of macrophages within the TME. Bill et al. conducted sequencing and clinical data analysis on 52 patients with head and neck squamous cell carcinoma, revealing that the CXCL9:SPP1 expression ratio, termed CShi or CSlow, is associated with inhibition of certain pro-tumor and anti-tumor effects in head and neck tumors. For instance, CShi tumors are more prone to immune cell infiltration, promoting anti-tumor immunity (45). They propose that evaluating the CXCL9:SPP1 ratio in macrophages could serve as a comprehensive indicator for several critical aspects, such as the presence of anti-tumor immune cells in cancer, gene expression profiles of different tumor-infiltrating cell types, control or progression of communication networks influencing tumors, and the effectiveness of immunotherapy (45). This valuable insight holds profound implications for prospective studies aimed at formulating personalized treatment strategies and prognostic evaluations.
3 Diverse macrophage polarization and its implications in the progression of HNSCC
3.1 Polarization
Macrophage polarization refers to the distinct activation status of macrophages at a specific moment. This state is determined by their variable expression of surface receptors, secretion patterns, and functional roles. In cancer researches, macrophages typically exhibit an M1 pro-inflammatory profile in the early phases, but they transition to an anti-inflammatory M2 profile in later stages. Macrophage polarization is dynamic, reflecting their adaptability, and it can change in response to a variety of signals from other cells, tissues, and pathogens (46).
In the mice model of oral cancer precursor lesions exposed to nicotine smoke, the degree of M2 polarization at the disease site increased with exposure. Simultaneously, metabolic levels of compounds such as L-nicotine, D-glutamate, arachidic acid, and L-arginine also rose. Some of the mice with heightened M2 polarization even directly developed oral cancer. During this process, there was a decrease in pro-inflammatory factors (iNOS and TNF-α) that induce M1 polarization, resulting in reduced monocyte recruitment to replace them. The polarization shifted towards M2, leading to a significant increase in M2 functional factors like Arg-1 and IL-10. Further, this sustained M2 polarization is indicative of an ongoing immune response, facilitating heightened he activity of Th2 cells and instigating an immunogenic reaction (47).
In head and neck tumors, the Warburg effect manifests as excessive lactate formation, enabling cancer cells to adapt their metabolism to meet the oxygen requirements and the substantial nucleotide, amino acid, and lipid needs for cell proliferation (48). The end product, lactate, is found at higher concentrations in head and neck cancer compared to normal tissue, creating an active environment to promote cancer progression. Notably, the lactate produced by tumor cells has multifaceted effects. It can promote tumor progression by activating pro-inflammatory pathways like IL-23/IL-17 (49), while also inducing monocytes to polarize towards the M2 phenotype, thereby serving as a mediator of immunosuppression to further drive cancer progression (50, 51). Indeed, low pH can reduce the expression of iNOS, CCL2, and IL-6 in M1 macrophages, but increase the expression of M2 macrophage markers in the TME (52). Correspondingly, lactate can promote the M2-like phenotype by activating G-protein-coupled receptor 132 (GPR132) in macrophages, and genetic deletion of Gpr132 in macrophages reduces the M2-like features of tumor-associated macrophages and decreases lung metastasis in a mouse breast cancer model (53). Additionally, lactate can induce the expression of the enzyme ARG1 which indicates lactate can transform macrophages into immunosuppressive macrophages and promote M2-macrophage polarization in mice (54). The intratumoral lactate levels in human HNSCC are associated with the polarization of M2-like macrophages in the TME as well. When lactate levels in the tumor are low, more macrophages accumulate at the tumor site. Conversely, under conditions of high lactate concentration, monocyte migration is inhibited, preventing effective macrophage infiltration into lactate-rich tumors, but it promotes their polarization towards the M2 phenotype. However, unlike in mice models, both lactate and M2-polarization levels are not associated with the expression of ARG1 in human macrophages (55).
Moreover, in the interaction between cancer cells and macrophages in HNSCC, cancer cells release Apelin peptide, which promotes the polarization of M2 macrophages. Inhibiting the release of Apelin peptide by cancer cells leads to an increase in pro-inflammatory responses in co-cultured macrophages, resulting in a significant upregulation of genes like IL-1β, IL-6, and TNF-α, along with a marked reduction in anti-inflammatory cytokine levels. In the Apelin (+) group, pro-inflammatory factors are decreased, while anti-inflammatory factors are heightened (56).Tumor-derived extracellular vesicles expressing TGF-β also play a significant role in the crosstalk between tumor cells and macrophages in the HNSCC TME. These extracellular vesicles induce polarization and chemotaxis of human macrophages and also reprogram the function of primary human macrophages. This reprogramming results in increased secretion of pro-angiogenic factors, including Angiopoietin-2, MMP-9, PD-ECGF, and TIMP-1, and a shift toward a pro-angiogenic phenotype. Upon injection into mice with oral cancer induced by 4-nitroquinoline 1-oxide (4-NQO), these vesicles promote tumor angiogenesis, enhance infiltration of M2-like macrophages, and accelerate tumor progression (28).
The mechanisms through which M2-polarized cells regulate the progression of head and neck cancer are intricate. TAMs enhance the stemness of head and neck cancer cells by activating the PI3K-4EBP1 pathway. Additionally, TAMs interact with head and neck cancer cells through the CD44-VCAM-1 pathway, ultimately boosting the invasive capabilities of cancer cells (57). Furthermore, it has been demonstrated that M2 polarization can increase the expression of PD-L2 in TAMs, leading to immune evasion and tumor progression through the PD-1 signaling pathway (58).
3.2 Re-polarization
In general, M1 macrophages provide immune protection by releasing pro-inflammatory cytokines, whereas M2 macrophages exhibit anti-inflammatory properties that aid in tissue remodeling and tumor advancement (59). In cancer research, the differentiation of macrophages into M1 type from the alternative M2 type, a process known as macrophage repolarization, is a promising approach in contemporary cancer immunotherapy. Repolarizing TAMs from M2-to-M1 is considered a prospective therapeutic strategy.
To reprogram TAMs without altering the M1/M2 polarization balance within healthy organs, Xiao et al. developed a micelle nano-therapy. They released M2-targeted antagonists after exposure to the acidic tumor microenvironment, co-delivering inhibitors like STAT6 to effectively achieve M2-to-M1 repolarization, thereby inhibiting tumor growth and metastasis (60). Additionally, Wu et al. have coupled targeted drugs with tumor-specific STING agonists, finding that within the tumor microenvironment, M2 repolarizes towards M1 (61). Furthermore, statins have been found to inhibit proliferation of recurrent/metastatic HNSCC cells, enhance T cell cytotoxicity against tumor cells, and promote M2-to-M1 macrophage repolarization (62). Statins, known for their tolerability and affordability, may further enhance responses to PD-L1 checkpoint blockade and other HNSCC immunotherapies, although this potential remains to be fully explored.
Simultaneously, macrophage repolarization often broadly refers to macrophages polarizing towards different functional directions. In the previously mentioned exosomes, M1 exosomes and HOTTIP induced M1 repolarization within the tumor microenvironment, encompassing macrophage repolarization.
4 Main targets for macrophage targeting in HNSCC
4.1 STAT3
Signal transducer and activator of transcription 3 (STAT3) is frequently overactivated in various human cancers, serving as a crucial signaling node in tumor cells and the cellular components of the TME, especially in tumor-infiltrating immune cells (63). Radiation therapy, a commonly used treatment for HNSCC, aims to utilize high-energy radiation to selectively kill or control the growth of cancer cells, reducing tumor size or eliminating the tumor altogether (64). It is often employed as a treatment option for patients who are not suitable candidates for surgery.
In the circulatory system of HNSCC patients undergoing radiotherapy, there is an accumulation of therapy-resistant bone marrow cells, which affects the efficacy of radiation therapy (65). Moreira et al. found that targeting STAT3 in TAMs can enhance the therapeutic effects of radiation therapy for HNSCC. They employed the CpG-STAT3ASO strategy to target STAT3 in HNSCC-related macrophages in conjunction with TLR9 triggering. This approach can overcome radiation resistance in tumors of both HPV-positive and HPV-negative mice. The combined treatment results in reduced residual M2 macrophages in the tumor and the recruitment of activated M1 macrophages to the tumor-draining lymph nodes (TDLNs).
A single-cell transcriptomic study of oral squamous cell carcinoma has revealed an enrichment of the IL-6/JAK2/STAT3 axis in the tumor microenvironment, particularly in cell populations like macrophages, in samples induced by chemotherapy and other treatments (66). Additionally, the phosphorylation level of STAT3 can modulate the response of regulatory T cells (Tregs) to radiation therapy in head and neck cancer (67). These findings indicate that STAT3 could serve as a significant combinatorial therapeutic target to enhance the efficacy of radiotherapy and chemotherapy in head and neck cancer.
Targeting STAT3 in current research offers several advantages, including improving immune dysregulation in the tumor microenvironment, reducing endogenous proliferation of tumor cells, and enhancing the anti-tumor effects of tumor-infiltrating immune cells, among others (68, 69). As a potential target for cancer treatment, the current drug development efforts against STAT3 involve direct inhibition using peptides, small molecules, and decoy oligonucleotides (70–73), or indirect inhibition through blocking upstream signaling pathways such as the IL-6 and JAK2 pathways (74, 75).
4.2 CCL2/CCR2
CC-chemokine receptor 2 (CCR2) is primarily expressed in monocytes and macrophages and has a strong pro-inflammatory function (76). This has led to the development of CCR2 antagonists aimed at inhibiting unnecessary immune responses in inflammation and autoimmune diseases. Paradoxically, in the tumor microenvironment, CCR2-expressing monocytes and macrophages can strongly suppress immune responses (77). In recent years, researchers have explored strategies using CCR2 antagonists to selectively attract suppressive monocytes and macrophages into the tumor, with the goal of altering the tumor microenvironment and enhancing the immune system’s ability to combat cancer (78).
While the mice model of HNSCC treated with radiotherapy, there showed an increase in the production of the chemotactic factor CCL2 in tumor cells, leading to the accumulation of CCR2-dependent TNF-α-producing monocytes/macrophages and CCR2+ Tregs (79). CCL2/CCR2 could potentially serve as clinical candidates for radioimmunotherapy to counteract the radio-protective effects of macrophages and Treg cells. Currently, synthetic inhibitors of CCL2, Bindarit(Bnd) (80) and Carlumab(CNTO 888) (81), as well as CCR2 antagonists RS-50439 and MLN1202, have been developed for targeted disruption of CCL2/CCR2 signaling to intervene in the progression of various tumor types (82–85).
4.3 NRF2
NRF2, encoded by the NFE2L2 gene, plays a crucial role in maintaining cellular redox homeostasis, regulating immune responses, and detoxifying drugs (86, 87). Activation of NRF2 can lead to metabolic reprogramming, enhancing tumor proliferation, suppressing various forms of stress, and promoting immune evasion (88).
NRF2 is upregulated in HNSCC, and its expression levels are positively correlated with malignancy (89, 90). Carcinogens such as nicotine and arecoline can trigger c-myc-driven NRF2 activation in HNSCC cells, reprogramming the pentose phosphate pathway metabolism in the tumor microenvironment (90). In this metabolic pathway, glucose-6-phosphate dehydrogenase (G6PD) and transketolase (TKT) are key downstream effectors driven by NRF2, contributing to the progression of head and neck squamous cell carcinoma.
Mutations in the NRF2-encoding gene NFE2L2 can result in radiation resistance in HNSCC. Notably, in HNSCC patients undergoing radio chemotherapy, NFE2L2 mutations are significantly linked to a heightened risk of local treatment failure. In immunocompetent mice, tumors carrying NFE2L2 mutations displayed increased resistance to radiation compared to tumors with the wild-type NRF2. However, this discrepancy was less pronounced in immunodeficient mice. NFE2L2 enhances radiation resistance by diminishing the presence of M1-polarized macrophages (91).
Previously, researchers have attempted to inhibit NRF2 by studying the natural inhibitory protein Kelch-like ECH-associated protein 1 (KEAP1) that targets NRF2 (92, 93). Additionally, in order to discover new NRF2 inhibitors for targeted therapy, Singh et al. conducted a quantitative high-throughput screening in the small molecule library MLSMR and identified ML385 as a probe molecule that binds to NRF2 and inhibits its downstream target gene expression (94).
4.4 CD47
CD47 is a widely expressed cell surface protein that acts as a ligand for signal regulatory protein alpha (SIRPα) on macrophages, which, in turn, inhibits phagocytosis (95). Previous studies in various preclinical models have demonstrated that blocking the CD47-SIRPα pathway can enhance phagocytic functions, demonstrating significant anti-tumor efficacy across multiple tumor types (96, 97).
Regarding a macrophage-mediated anti-tumor immunotherapy strategy based on gene-edited nanoparticles: the first step involves blocking the CD47-SIRPα pathway, and the second step is to repolarize tumor-associated macrophages (98). Additionally, Ni and colleagues discovered in preclinical models using the IBI188 drug to block the CD47-SIRPα pathway that angiogenesis can, to some extent, limit the effectiveness of anti-CD47 antibodies against tumors. Combining anti-angiogenesis therapies with CD47 blockade can achieve higher therapeutic efficacy (99).
Recently, Lee et al. conducted macrophage phagocytosis experiments on the HN31R head and neck cancer cell line and found that the downregulation of Tristetraprolin (TTP) can induce sustained overexpression of CD47, which, in turn, inhibits the phagocytosis of head and neck cancer cells (100). Furthermore, when CD47 was expressed in vitro in HNSCC cell lines, both M1 and M2 macrophages exhibited a certain degree of phagocytic potential (101). However, under conditions where CD47 was inhibited, the phagocytic ability of M1 enhanced, while M2 did not (101). In summary, CD47-positive oral squamous cell carcinoma cells primarily inhibit M1 phagocytosis, leading to immune evasion.
Currently, several antibodies targeting CD47 have entered clinical trials, such as Hu5F9-G4, TTI-621, and others, for the treatment of both solid tumors and hematologic malignancies (102, 103).
4.5 TGF-β
Transforming growth factor-β (TGF-β) is a widely recognized immunosuppressive factor, playing a role in restraining excessive inflammatory responses (104–106). Additionally, TGF-β triggers macrophage M2 polarization, contributing to the alleviation of inflammation mediated by macrophages (105, 107).
PD-1 blockade therapy in the treatment of HNSCC has demonstrated a significant extension of survival in recurrent/metastatic (R/M) patients, coupled with favorable safety profiles (108, 109). However, numerous challenges persist, with a substantial portion of cancer patients exhibiting suboptimal responses to PD-1 monotherapy (110). The crucial role of TGF-β in the delicate balance between immunity and tolerance among non-responsive patients to PD-1 monotherapy has been identified (111, 112). TGF-β modulates the cancer immune cycle by altering T cell proliferation, activation, differentiation, and impeding the activity of dendritic cells and natural killer cells (113). Combining anti-TGF-β with anti-PD-1 therapy has proven effective in overcoming resistance in immune rejection models (114, 115). Subsequently, Yi et al. developed the bispecific antibody (BsAb) YM101 which targeting both TGF-β and PD-L1 (116). They observed potent anti-tumor activity of this drug in immune-inflammatory and immunosuppressive models of diverse tumors (117). Additionally, a TGF-β/PD-L1 specific antibody, the drug BiTP, has been developed and demonstrated promising anti-tumor efficacy in both in vitro and in vivo experiments (118). Simultaneously, Matos et al. have recently engineered a Polyoxazoline-Based nano-vaccine carrying a TGF-β expression regulator in combination with a PD-1 inhibitor. This combination exhibits synergistic anti-tumor effects and holds significant potential in improving the immunotherapeutic outcomes for solid cancer patients (119).
4.6 Other targets
The regulation of macrophage proliferation, differentiation, and survival hinges on the control of CSF1R and its ligands. A multitude of preclinical investigations have underscored that the inhibition of CSF1R leads to a decreased density of TAMs, resulting in the inhibition of tumor growth and heightened sensitivity to chemotherapy (120, 121). Besides, elevated expression of CSF1R leads to increased lactate levels in HNSCC, reduces the presence of tumor-infiltrating macrophages, and promotes the induction of M2-like macrophage polarization within the tumor (55). The drugs targeting CSF1/CSF1R, such as PLX3397 and HMPL-012, have been proven to be effective in other tumors, including tenosynovial giant cell tumor and neuroendocrine tumors (122, 123).
Moreover, high expression of RACK1 in oral squamous cell carcinoma (OSCC) is associated with increased infiltration of M2 macrophages (124). OSCC cells that overexpress RACK1 promote M2-like macrophage polarization through the regulation of NF-kappa B, leading to an increase in the proportion of M2-like macrophages in xenograft mouse models (27). The corresponding targeted drug is M435-1279, a critical ubiquitin-conjugating enzyme E2T (UBE2T) inhibitor that catalyzes the proteasomal degradation of RACK1, which also has certain prospects for future applications (125). Those typical targets, pathways, and associated drugs of macrophages in the progression HNSCC are shown in Table 1.
5 Applications of macrophages in the treatment of HNSCC
5.1 Conventional immunotherapy targeting
Macrophages are an important target in current checkpoint blockade immunotherapy, suppressing adaptive immune responses by expressing inhibitory counter-receptors such as PD-L1 and PD-L2. Certain chemotherapy drugs, like anthracyclines, induce the release of tumor antigens and co-stimulatory molecules, a process referred to as immunogenic cell death, engaging macrophages in a productive cancer immune cycle (126). There are also other cell-depleting therapies aimed at targeting macrophages (127). Specific strategies focused on macrophages have partly entered clinical assessment, including monocyte-derived macrophages used for cellular therapy, either through targeted recruitment and differentiation or functional reprogramming via activation or inhibition of checkpoint receptors.
In the treatment of recurrent/metastatic HNSCC patients, checkpoint inhibitors have demonstrated their effectiveness (128). However, the majority of patients do not benefit from these drugs (129). To enhance the efficacy of checkpoint inhibitors, Sato-Kaneko F et al. have established HNSCC models and employed a combination of TLR agonists and PD-1 blockade (130). They found that this approach could activate TAMs, induce tumor-specific adaptive immunity, and thus inhibit primary tumor growth and prevent metastasis. Notably, treatment with TLR7 agonists increased the M1/M2 ratio and promoted the generation of tumor-specific immune factors.
To enhance the immunotherapeutic efficacy in HNSCC, Wu et al. developed an injectable nano-composite hydrogel (131). This hydrogel is created by incorporating imiquimod-encapsulated CaCO3 nanoparticles (RC) and a cancer cell membrane (CCM)-coated mesoporous silica nanoparticle within a polymer framework (PLGA-PEG-PLGA). These components include a peptide-based protein hydrolysis targeting chimera (PROTAC) against BMI1 paclitaxel (PepM@PacC). The injectable hydrogel can selectively manipulate tumor-associated macrophages, further activating T cell immune responses.
5.2 Engineering macrophages
In response to the phagocytic and pro-inflammatory actions of M1 macrophages on tumor cells, engineered macrophages targeting cancer cells as carriers for anti-tumor therapy have been developed to modulate the tumor microenvironment (132).
For example, controlled-release biomimetic or macrophage membrane-coated nanoparticles have been developed for cancer therapy to respond to the TME (21). Rao et al. engineered cell membrane-coated magnetic nanoparticles (gCM-MNs) to enhance the affinity between the genetically overexpressed SIRPα variant on gCM shells and CD47, effectively blocking the CD47-SIRPα pathway and preserving macrophage’s ability to phagocytose cancer cells (133). Meanwhile, these magnetic nanoparticles promote M2-to-M1 repolarization in the tumor microenvironment of B16F10 melanoma mice model and the triple negative breast cancer 4T1 mice model, blocking the process of tumor cells secreting colony-stimulating factors to polarize tumor-associated macrophages into tumor-promoting M2 macrophages. This synergistically enhances macrophage phagocytosis of cancer cells and triggers anti-tumor T-cell immunity. This method effectively activates macrophages for anti-tumor immunotherapy. In addition, macrophage membrane-coated nanoparticles (cskc-PPiP/PTX@Ma) developed by Zhang et al. show enhanced therapeutic effects, homing to tumor sites and gradually controlling drug release in response to the acidic pH changes in the tumor microenvironment, releasing the hydrophobic anti-cancer drug paclitaxel to kill cancer cells. Testing the administration capability and therapeutic effects of this formulation in an orthotopic breast cancer-bearing mice model, this combination of a biomimetic cell membrane and a cascade-responsive polymeric nanoparticle yielded significant results (134).
Furthermore, Rayamajhi and colleagues developed hybrid exosomes (HE) with a size smaller than 200nm by hybridizing exosomes extracted from mouse macrophages with synthetic liposomes (135). They loaded a water-soluble doxorubicin into these HE, increasing the toxicity of drug-loaded HE to cancer cells and enabling drug release in the acidic tumor microenvironment. These macrophage-derived mimetic exosome vesicles effectively deliver bioactive molecules to recipient cells, making them suitable for drug delivery and therapy in cancer.
5.3 CAR-macrophage
Chimeric antigen receptor (CAR)-T cell therapy is an early cell-based immunotherapy designed to prevent tumor cells from evading recognition by T cell receptors. This method has been successfully used to treat hematologic malignancies, but its effectiveness in solid tumors remains limited (136). In the tumor microenvironment, macrophages, as the most abundant innate immune cells, can infiltrate solid tumor tissues and interact with almost all other cell types (137). Therefore, researchers are attempting to use CAR-modified macrophages (CAR-M) to combat solid tumors.
The first-generation CAR-M cells primarily utilize the characteristics of macrophages, focusing on their phagocytic function (138, 139). In contrast, second-generation CAR-M cells, in addition to retaining the features of the first generation, also aim to improve the presentation of tumor-associated antigens and T cell activation. In this scenario, Klichinsky et al. design murine or human macrophages through chimeric vectors and then obtain the drug after in vitro expansion, concentration, and purification (140). Currently, third-generation CAR-M cells are being designed by reprogramming CAR-M cells in vivo using non-viral vectors (141). There has been an approach to fuse nanobiotechnology with CAR-M cells, using nanocarriers to deliver the encoded CAR and interferon-gamma genes to macrophages in vivo, with the aim of further enhancing anti-tumor efficacy by repolarizing M2-polarized macrophages into M1 macrophages (142).
The progress of CAR-M therapy in HNSCC is currently quite limited. However, with the continuous iteration of CAR-M technology and the advancement of macrophages in head and neck squamous cell carcinoma, this field holds tremendous potential for application.
6 Discussion and prospects
Macrophages exhibit a high degree of plasticity in response to various microenvironments within normal human tissues, inflammatory stimuli, and tumor tissues. This functional diversity results in various characteristics within macrophages, making their categorization challenging. Currently, macrophages are broadly categorized into two phenotypes, M1 and M2, which are associated with pro-inflammatory and anti-inflammatory properties, respectively. Tumor-associated macrophages represent the complex interplay of various cell types within the TME and can exhibit M1 or M2 characteristics under the influence of different TME stimuli. Typically, M1-like TAMs that promote an inflammatory response against tumor cells often exhibit anti-cancer effects, while M2-like TAMs tend to support tumorigenesis (143).
Head and neck squamous cell carcinoma, being an invasive malignant tumor, is characterized by high incidence and low survival rates. Treatment options for HNSCC are limited, typically involving local surgery, radiation, and chemotherapy (144). The development of immunotherapy has harnessed the collaborative role of the TME in HNSCC progression. By understanding the processes of tumor cell evolution and immune evasion (Figure 2), immunotherapy demonstrates effective anti-cancer properties through the manipulation of self-immunogenicity or the expression of immune inhibitory mediators, ultimately enhancing the survival rates of HNSCC patients. However, it’s worth noting that fewer than 20% of patients exhibit sustained responses to these treatments (145).
Within the HNSCC microenvironment, TAMs, being the most abundant group of innate immune cells, play a role in mediating immunosuppressive effects on adaptive immune cells in the TME. The polarization state of TAMs can be influenced by various signals like nicotine, Apelin peptide, and lactate. This polarization state has a strong connection with the development and immune evasion in head and neck cancer, although it doesn’t necessarily impede immune responses. In the context of head and neck cancer, the M2 polarization of macrophages can impact tumor stemness, invasiveness, and the mechanisms of immune evasion. Consequently, inhibiting M2 polarization and promoting M2-to-M1 repolarization have emerged as crucial strategies that leverage the remarkable plasticity of macrophages in anti-cancer efforts. Building upon this foundational theory, more effective immunotherapeutic approaches have been further explored, including the engineering of macrophages and the utilization of CAR-M technology to eliminate HNSCC cells.
Targeting TAMs and HNSCC remains an ongoing and challenging endeavor in progress. Macrophages play a crucial dual role in different anticancer modalities, as they are actively involved not only in chemotherapy, radiation therapy, and immune checkpoint blockade (ICB) immunotherapy, as mentioned above, but also in anti-angiogenesis and hormone therapy. For instance, in one study, it was found that metformin reduces the accumulation of M2-TAMs in the tumor microenvironment, impeding M2-like macrophage-induced angiogenesis promotion. On the other hand, melatonin indirectly inhibits tumor angiogenesis by increasing the accumulation of M1-TAMs (146). Consequently, developing more precise targeted treatment strategies and exploring the potential of macrophage-based therapies are all research directions for further improving HNSCC survival rates and refining the approaches to HNSCC treatment in the future.
This review presents a comprehensive overview of the immune-regulatory roles played by macrophages in HNSCC. It delves into the diverse polarization states of macrophages within the tumor microenvironment and explores potential therapeutic strategies for repolarization. Recent years have witnessed significant progress in research targeting critical macrophage-related factors, along with substantial advancements and refinements in macrophage-based therapies for head and neck cancer. These developments aim to boost the efficacy of immunotherapy for HNSCC. Through this contribution, our objective is to advance macrophage-related therapeutic strategies for HNSCC, revealing more effective potential treatment methods in this evolving era.
Author contributions
CL: Writing – review & editing, Writing – original draft. YC: Writing – review & editing, Visualization, Validation, Investigation. YZ: Writing – review & editing, Visualization, Validation. SG: Writing – review & editing, Supervision, Funding acquisition. YH: Writing – review & editing, Methodology. JH: Writing – review & editing, Methodology. ZS: Writing – review & editing, Supervision, Project administration, Funding acquisition.
Funding
The author(s) declare financial support was received for the research, authorship, and/or publication of this article. This research project is supported by Ningbo Top Medical and Health Research Program (Grant No.2023030514); Ningbo Clinical Research Center for Otolaryngology Head and Neck Disease (Grant No.2022L005); Ningbo medical and health brand discipline (Grant No.PPXK2018-02); Zhejiang Provincial Natural Science Foundation of China (Grant No. LY19H160014, LQ21H130001); Ningbo “Technology Innovation 2025” Major Special Project (Grant No. 2020Z097); Ningbo Natural Science Foundation (Grant No. 2023J030, 2021J290), all of which are in China.
Acknowledgments
Figures were created with biorender.com.
Conflict of interest
The authors declare that the research was conducted in the absence of any commercial or financial relationships that could be construed as a potential conflict of interest.
Publisher’s note
All claims expressed in this article are solely those of the authors and do not necessarily represent those of their affiliated organizations, or those of the publisher, the editors and the reviewers. Any product that may be evaluated in this article, or claim that may be made by its manufacturer, is not guaranteed or endorsed by the publisher.
References
1. Mody MD, Rocco JW, Yom SS, Haddad RI, Saba NF. Head and neck cancer. Lancet. (2021) 398:2289–99. doi: 10.1016/S0140-6736(21)01550-6
2. Johnson DE, Burtness B, Leemans CR, Lui VWY, Bauman JE, Grandis JR. Head and neck squamous cell carcinoma. Nat Rev Dis Primers. (2020) 6:92. doi: 10.1038/s41572-020-00224-3
3. Joyce JA, Fearon DT. T cell exclusion, immune privilege, and the tumor microenvironment. Science. (2015) 348:74–80. doi: 10.1126/science.aaa6204
4. Chen SMY, Krinsky AL, Woolaver RA, Wang X, Chen Z, Wang JH. Tumor immune microenvironment in head and neck cancers. Mol Carcinog. (2020) 59:766–74. doi: 10.1002/mc.23162
5. Bhat AA, Yousuf P, Wani NA, Rizwan A, Chauhan SS, Siddiqi MA, et al. Tumor microenvironment: an evil nexus promoting aggressive head and neck squamous cell carcinoma and avenue for targeted therapy. Signal Transduct Target Ther. (2021) 6:12. doi: 10.1038/s41392-020-00419-w
6. Bhatia A, Burtness B. Treating head and neck cancer in the age of immunotherapy: A 2023 update. Drugs. (2023) 83:217–48. doi: 10.1007/s40265-023-01835-2
7. Peltanova B, Raudenska M, Masarik M. Effect of tumor microenvironment on pathogenesis of the head and neck squamous cell carcinoma: a systematic review. Mol Cancer. (2019) 18:63. doi: 10.1186/s12943-019-0983-5
8. Tong N, He Z, Ma Y, Wang Z, Huang Z, Cao H, et al. Tumor associated macrophages, as the dominant immune cells, are an indispensable target for immunologically cold tumor-glioma therapy? Front Cell Dev Biol. (2021) 9:706286. doi: 10.3389/fcell.2021.706286
9. Guilliams M, Mildner A, Yona S. Developmental and functional heterogeneity of monocytes. Immunity. (2018) 49:595–613. doi: 10.1016/j.immuni.2018.10.005
10. Kimm MA, Klenk C, Alunni-Fabbroni M, Kastle S, Stechele M, Ricke J, et al. Tumor-associated macrophages-implications for molecular oncology and imaging. Biomedicines. (2021) 9. doi: 10.3390/biomedicines9040374
11. Dallavalasa S, Beeraka NM, Basavaraju CG, Tulimilli SV, Sadhu SP, Rajesh K, et al. The role of tumor associated macrophages (TAMs) in cancer progression, chemoresistance, angiogenesis and metastasis - current status. Curr Med Chem. (2021) 28:8203–36. doi: 10.2174/1875533XMTE20ODIe4
12. Li B, Ren M, Zhou X, Han Q, Cheng L, et al. Targeting tumor-associated macrophages in head and neck squamous cell carcinoma. Oral Oncol. (2020) 106:104723. doi: 10.1016/j.oraloncology.2020.104723
13. Shapouri-Moghaddam A, Mohammadian S, Vazini H, Taghadosi M, Esmaeili SA, Mardani F, et al. Macrophage plasticity, polarization, and function in health and disease. J Cell Physiol. (2018) 233:6425–40. doi: 10.1002/jcp.26429
14. Liu Z, Rui T, Lin Z, Xie S, Zhou B, Fu M, et al. Tumor-associated macrophages promote metastasis of oral squamous cell carcinoma via CCL13 regulated by stress granule. Cancers (Basel). (2022) 14. doi: 10.3390/cancers14205081
15. Mills CD. M1 and M2 macrophages: oracles of health and disease. Crit Rev Immunol. (2012) 32:463–88. doi: 10.1615/CritRevImmunol.v32.i6
16. Viola A, Munari F, Sanchez-Rodriguez R, Scolaro T, Castegna A. The metabolic signature of macrophage responses. Front Immunol. (2019) 10:1462. doi: 10.3389/fimmu.2019.01462
17. Liu Y, Xu R, Gu H, Zhang E, Qu J, Cao W, et al. Metabolic reprogramming in macrophage responses. biomark Res. (2021) 9:1. doi: 10.1186/s40364-020-00251-y
18. Li M, Yang Y, Xiong L, Jiang P, Wang J, Li C. Metabolism, metabolites, and macrophages in cancer. J Hematol Oncol. (2023) 16:80. doi: 10.1186/s13045-023-01478-6
19. Liu PS, Chen YT, Li X, Hsueh PC, Tzeng SF, Chen H, et al. CD40 signal rewires fatty acid and glutamine metabolism for stimulating macrophage anti-tumorigenic functions. Nat Immunol. (2023) 24:452–62. doi: 10.1038/s41590-023-01430-3
20. Zhu L, Zhao Q, Yang T, Ding W, Zhao Y. Cellular metabolism and macrophage functional polarization. Int Rev Immunol. (2015) 34:82–100. doi: 10.3109/08830185.2014.969421
21. Mantovani A, Allavena P, Marchesi F, Garlanda C. Macrophages as tools and targets in cancer therapy. Nat Rev Drug Discovery. (2022) 21:799–820. doi: 10.1038/s41573-022-00520-5
22. Bashir S, Sharma Y, Elahi A, Khan F. Macrophage polarization: the link between inflammation and related diseases. Inflammation Res. (2016) 65:1–11. doi: 10.1007/s00011-015-0874-1
23. Wang L, Lu Q, Gao W, Yu S. Recent advancement on development of drug-induced macrophage polarization in control of human diseases. Life Sci. (2021) 284:119914. doi: 10.1016/j.lfs.2021.119914
24. Li C, Xu X, Wei S, Jiang P, Xue L, Wang J. Tumor-associated macrophages: potential therapeutic strategies and future prospects in cancer. J Immunother Cancer. (2021) 9. doi: 10.1136/jitc-2020-001341
25. Pan K, Farrukh H, Chittepu V, Xu H, Pan CX, Zhu Z. CAR race to cancer immunotherapy: from CAR T, CAR NK to CAR macrophage therapy. J Exp Clin Cancer Res. (2022) 41:119. doi: 10.1186/s13046-022-02327-z
26. Kumari N, Choi SH. Tumor-associated macrophages in cancer: recent advancements in cancer nanoimmunotherapies. J Exp Clin Cancer Res. (2022) 41:68. doi: 10.1186/s13046-022-02272-x
27. Dan H, Liu S, Liu J, Liu D, Yin F, Wei Z, et al. RACK1 promotes cancer progression by increasing the M2/M1 macrophage ratio via the NF-kappaB pathway in oral squamous cell carcinoma. Mol Oncol. (2020) 14:795–807. doi: 10.1002/1878-0261.12644
28. Ludwig N, Yerneni SS, Azambuja JH, Pietrowska M, Widlak P, Hinck CS, et al. TGFbeta(+) small extracellular vesicles from head and neck squamous cell carcinoma cells reprogram macrophages towards a pro-angiogenic phenotype. J Extracell Vesicles. (2022) 11:e12294. doi: 10.1002/jev2.12294
29. Liss C, Fekete MJ, Hasina R, Lam CD, Lingen MW. Paracrine angiogenic loop between head-and-neck squamous-cell carcinomas and macrophages. Int J Cancer. (2001) 93:781–5. doi: 10.1002/(ISSN)1097-0215
30. Watermann C, Pasternack H, Idel C, Ribbat-Idel J, Bragelmann J, Kuppler P, et al. Recurrent HNSCC harbor an immunosuppressive tumor immune microenvironment suggesting successful tumor immune evasion. Clin Cancer Res. (2021) 27:632–44. doi: 10.1158/1078-0432.CCR-20-0197
31. Mylvaganam S, Freeman SA, Grinstein S. The cytoskeleton in phagocytosis and macropinocytosis. Curr Biol. (2021) 31:R619–32. doi: 10.1016/j.cub.2021.01.036
32. Moradinasab S, Pourbagheri-Sigaroodi A, Ghaffari SH, Bashash D. Targeting macrophage-mediated tumor cell phagocytosis: An overview of phagocytosis checkpoints blockade, nanomedicine intervention, and engineered CAR-macrophage therapy. Int Immunopharmacol. (2022) 103:108499. doi: 10.1016/j.intimp.2021.108499
33. Zhang X, Goncalves R, Mosser DM. The isolation and characterization of murine macrophages. Curr Protoc Immunol. (2008) 83(1):14.1.1–14.1.14. doi: 10.1002/0471142735.im1401s83
34. Atri C, Guerfali FZ, Laouini D. Role of human macrophage polarization in inflammation during infectious diseases. Int J Mol Sci. (2018) 19. doi: 10.3390/ijms19061801
35. Korbecki J, Kojder K, Kapczuk P, Kupnicka P, Gawronska-Szklarz B, Gutowska I, et al. The effect of hypoxia on the expression of CXC chemokines and CXC chemokine receptors-A review of literature. Int J Mol Sci. (2021) 22. doi: 10.3390/ijms22020843
36. Mantovani A, Marchesi F, Malesci A, Laghi L, Allavena P, et al. Tumour-associated macrophages as treatment targets in oncology. Nat Rev Clin Oncol. (2017) 14:399–416. doi: 10.1038/nrclinonc.2016.217
37. Gocheva V, Wang HW, Gadea BB, Shree T, Hunter KE, Garfall AL, et al. IL-4 induces cathepsin protease activity in tumor-associated macrophages to promote cancer growth and invasion. Genes Dev. (2010) 24:241–55. doi: 10.1101/gad.1874010
38. van Dalen FJ, van Stevendaal M, Fennemann FL, Verdoes M, Ilina O. Molecular repolarisation of tumour-associated macrophages. Molecules. (2018) 24. doi: 10.3390/molecules24010009
39. Gudgeon J, Marin-Rubio JL, Trost M. The role of macrophage scavenger receptor 1 (MSR1) in inflammatory disorders and cancer. Front Immunol. (2022) 13:1012002. doi: 10.3389/fimmu.2022.1012002
40. Qu X, Li JW, Chan J, Meehan K. Extracellular vesicles in head and neck cancer: A potential new trend in diagnosis, prognosis, and treatment. Int J Mol Sci. (2020) 21. doi: 10.3390/ijms21218260
41. Jiang H, Zhou L, Shen N, Ning X, Wu D, Jiang K. M1 macrophage-derived exosomes and their key molecule lncRNA HOTTIP suppress head and neck squamous cell carcinoma progression by upregulating the TLR5/NF-kappaB pathway. Cell Death Dis. (2022) 13:183. doi: 10.1038/s41419-022-04640-z
42. Pang X, Wang SS, Zhang M, Jiang J, Fan HY, Wu JS, et al. OSCC cell-secreted exosomal CMTM6 induced M2-like macrophages polarization via ERK1/2 signaling pathway. Cancer Immunol Immunother. (2021) 70:1015–29. doi: 10.1007/s00262-020-02741-2
43. Wei F, Fang R, Lyu K, Liao J, Long Y, Yang J, et al. Exosomal PD-L1 derived from head and neck squamous cell carcinoma promotes immune evasion by activating the positive feedback loop of activated regulatory T cell-M2 macrophage. Oral Oncol. (2023) 145:106532. doi: 10.1016/j.oraloncology.2023.106532
44. Troiano G, Caponio VCA, Adipietro I, Tepedino M, Santoro R, Laino L, et al. Prognostic significance of CD68(+) and CD163(+) tumor associated macrophages in head and neck squamous cell carcinoma: A systematic review and meta-analysis. Oral Oncol. (2019) 93:66–75. doi: 10.1016/j.oraloncology.2019.04.019
45. Bill R, Wirapati P, Messemaker M, Roh W, Zitti B, Duval F, et al. CXCL9:SPP1 macrophage polarity identifies a network of cellular programs that control human cancers. Science. (2023) 381:515–24. doi: 10.1126/science.ade2292
46. Mehla K, Singh PK. Metabolic regulation of macrophage polarization in cancer. Trends Cancer. (2019) 5:822–34. doi: 10.1016/j.trecan.2019.10.007
47. Zhu Y, Zhang S, Sun J, Wang T, Liu Q, Wu G, et al. Cigarette smoke promotes oral leukoplakia via regulating glutamine metabolism and M2 polarization of macrophage. Int J Oral Sci. (2021) 13:25. doi: 10.1038/s41368-021-00128-2
48. Zheng Y, Lin C, Chu Y, Gu S, Deng H, Shen Z. Spatial metabolomics in head and neck tumors: a review. Front Oncol. (2023) 13:1213273. doi: 10.3389/fonc.2023.1213273
49. Shime H, Yabu M, Akazawa T, Kodama K, Matsumoto M, Seya T, et al. Tumor-secreted lactic acid promotes IL-23/IL-17 proinflammatory pathway. J Immunol. (2008) 180:7175–83. doi: 10.4049/jimmunol.180.11.7175
50. Ye H, Zhou Q, Zheng S, Li G, Lin Q, Wei L, et al. Tumor-associated macrophages promote progression and the Warburg effect via CCL18/NF-kB/VCAM-1 pathway in pancreatic ductal adenocarcinoma. Cell Death Dis. (2018) 9:453. doi: 10.1038/s41419-018-0486-0
51. Hirschhaeuser F, Sattler UG, Mueller-Klieser W. Lactate: a metabolic key player in cancer. Cancer Res. (2011) 71:6921–5. doi: 10.1158/0008-5472.CAN-11-1457
52. Xie D, Zhu S, Bai L. Lactic acid in tumor microenvironments causes dysfunction of NKT cells by interfering with mTOR signaling. Sci China Life Sci. (2016) 59:1290–6. doi: 10.1007/s11427-016-0348-7
53. Chen P, Zuo H, Xiong H, Kolar MJ, Chu Q, Saghatelian A, et al. Gpr132 sensing of lactate mediates tumor-macrophage interplay to promote breast cancer metastasis. Proc Natl Acad Sci U.S.A. (2017) 114:580–5. doi: 10.1073/pnas.1614035114
54. Ohashi T, Akazawa T, Aoki M, Kuze B, Mizuta K, Ito Y, et al. Dichloroacetate improves immune dysfunction caused by tumor-secreted lactic acid and increases antitumor immunoreactivity. Int J Cancer. (2013) 133:1107–18. doi: 10.1002/ijc.28114
55. Ohashi T, Aoki M, Tomita H, Akazawa T, Sato K, Kuze B, et al. M2-like macrophage polarization in high lactic acid-producing head and neck cancer. Cancer Sci. (2017) 108:1128–34. doi: 10.1111/cas.13244
56. Celik FS, Gunes CE, Yavuz E, Kurar E. Apelin triggers macrophage polarization to M2 type in head and neck cancer. Immunobiology. (2023) 228:152353. doi: 10.1016/j.imbio.2023.152353
57. Gomez KE, Wu F, Keysar SB, Morton JJ, Miller B, Chimed TS, et al. Cancer cell CD44 mediates macrophage/monocyte-driven regulation of head and neck cancer stem cells. Cancer Res. (2020) 80:4185–98. doi: 10.1158/0008-5472.CAN-20-1079
58. Yang H, Zhang Q, Xu M, Wang L, Chen X, Feng Y, et al. CCL2-CCR2 axis recruits tumor associated macrophages to induce immune evasion through PD-1 signaling in esophageal carcinogenesis. Mol Cancer. (2020) 19:41. doi: 10.1186/s12943-020-01165-x
59. Anderson NR, Minutolo NG, Gill S, Klichinsky M. Macrophage-based approaches for cancer immunotherapy. Cancer Res. (2021) 81:1201–8. doi: 10.1158/0008-5472.CAN-20-2990
60. Xiao H, Guo Y, Li B, Li X, Wang Y, Han S, et al. M2-like tumor-associated macrophage-targeted codelivery of STAT6 inhibitor and IKKbeta siRNA induces M2-to-M1 repolarization for cancer immunotherapy with low immune side effects. ACS Cent Sci. (2020) 6:1208–22. doi: 10.1021/acscentsci.9b01235
61. Wu YT, Fang Y, Wei Q, Shi H, Tan H, Deng Y, et al. Tumor-targeted delivery of a STING agonist improvescancer immunotherapy. Proc Natl Acad Sci U.S.A. (2022) 119:e2214278119. doi: 10.1073/pnas.2214278119
62. Kansal V, Burnham AJ, Kinney BLC, Saba NF, Paulos C, Lesinski GB, et al. Statin drugs enhance responses to immune checkpoint blockade in head and neck cancer models. J Immunother Cancer. (2023) 11. doi: 10.1136/jitc-2022-005940
63. Zou S, Tong Q, Liu B, Huang W, Tian Y, Fu X. Targeting STAT3 in cancer immunotherapy. Mol Cancer. (2020) 19:145. doi: 10.1186/s12943-020-01258-7
64. Porceddu SV, Daniels C, Yom SS, Liu H, Waldron J, Gregoire V, et al. Head and neck cancer international group (HNCIG) consensus guidelines for the delivery of postoperative radiation therapy in complex cutaneous squamous cell carcinoma of the head and neck (cSCCHN). Int J Radiat Oncol Biol Phys. (2020) 107:641–51. doi: 10.1016/j.ijrobp.2020.03.024
65. Moreira D, Sampath S, Won H, White SV, Su YL, Alcantara M, et al. Myeloid cell-targeted STAT3 inhibition sensitizes head and neck cancers to radiotherapy and T cell-mediated immunity. J Clin Invest. (2021) 131. doi: 10.1172/JCI137001
66. Song H, Lou C, Ma J, Gong Q, Tian Z, You Y, et al. Single-cell transcriptome analysis reveals changes of tumor immune microenvironment in oral squamous cell carcinoma after chemotherapy. Front Cell Dev Biol. (2022) 10:914120. doi: 10.3389/fcell.2022.914120
67. Oweida AJ, Darragh L, Phan A, Binder D, Bhatia S, Mueller A, et al. STAT3 modulation of regulatory T cells in response to radiation therapy in head and neck cancer. J Natl Cancer Inst. (2019) 111:1339–49. doi: 10.1093/jnci/djz036
68. Ding L, Wang Q, Martincuks A, Kearns MJ, Jiang T, Lin Z, et al. STING agonism overcomes STAT3-mediated immunosuppression and adaptive resistance to PARP inhibition in ovarian cancer. J Immunother Cancer. (2023) 11. doi: 10.1136/jitc-2022-005627
69. Pal GP, Betzel C, Jany KD, Saenger W. Crystallization of the bifunctional proteinase/amylase inhibitor PKI-3 and of its complex with proteinase K. FEBS Lett. (1986) 197:111–4. doi: 10.1016/0014-5793(86)80308-8
70. Liu Z, Wang H, Guan L, Lai C, Yu W, Lai M. LL1, a novel and highly selective STAT3 inhibitor, displays anti-colorectal cancer activities in vitro and in vivo. Br J Pharmacol. (2020) 177:298–313. doi: 10.1111/bph.14863
71. Martin JD, Cabral H, Stylianopoulos T, Jain RK. Improving cancer immunotherapy using nanomedicines: progress, opportunities and challenges. Nat Rev Clin Oncol. (2020) 17:251–66. doi: 10.1038/s41571-019-0308-z
72. Bai L, Zhou H, Xu R, Zhao Y, Chinnaswamy K, McEachern D, et al. A potent and selective small-molecule degrader of STAT3 achieves complete tumor regression in vivo. Cancer Cell. (2019) 36:498–511 e17. doi: 10.1016/j.ccell.2019.10.002
73. Ouyang S, Li H, Lou L, Huang Q, Zhang Z, Mo J, et al. Inhibition of STAT3-ferroptosis negative regulatory axis suppresses tumor growth and alleviates chemoresistance in gastric cancer. Redox Biol. (2022) 52:102317. doi: 10.1016/j.redox.2022.102317
74. Yoon S, Wu X, Armstrong B, Habib N, Rossi JJ. An RNA Aptamer Targeting the Receptor Tyrosine Kinase PDGFRalpha Induces Anti-tumor Effects through STAT3 and p53 in Glioblastoma. Mol Ther Nucleic Acids. (2019) 14:131–41. doi: 10.1016/j.omtn.2018.11.012
75. Jin Y, Kang Y, Wang M, Wu B, Su B, Yin H, et al. Targeting polarized phenotype of microglia via IL6/JAK2/STAT3 signaling to reduce NSCLC brain metastasis. Signal Transduct Target Ther. (2022) 7:52. doi: 10.1038/s41392-022-00872-9
76. Shi C, Pamer EG. Monocyte recruitment during infection and inflammation. Nat Rev Immunol. (2011) 11:762–74. doi: 10.1038/nri3070
77. Ozga AJ, Chow MT, Luster AD. Chemokines and the immune response to cancer. Immunity. (2021) 54:859–74. doi: 10.1016/j.immuni.2021.01.012
78. Fei L, Ren X, Yu H, Zhan Y. Targeting the CCL2/CCR2 axis in cancer immunotherapy: one stone, three birds? Front Immunol. (2021) 12:771210. doi: 10.3389/fimmu.2021.771210
79. Mondini M, Loyher PL, Hamon P, Gerbe de Thore M, Laviron M, Berthelot K, et al. CCR2-dependent recruitment of tregs and monocytes following radiotherapy is associated with TNFalpha-mediated resistance. Cancer Immunol Res. (2019) 7:376–87. doi: 10.1158/2326-6066.CIR-18-0633
80. Ge S, Shrestha B, Paul D, Keating C, Cone R, Guglielmotti A, et al. The CCL2 synthesis inhibitor bindarit targets cells of the neurovascular unit, and suppresses experimental autoimmune encephalomyelitis. J Neuroinflamm. (2012) 9:171. doi: 10.1186/1742-2094-9-171
81. Brana I, Calles A, LoRusso PM, Yee LK, Puchalski TA, Seetharam S, et al. Carlumab, an anti-C-C chemokine ligand 2 monoclonal antibody, in combination with four chemotherapy regimens for the treatment of patients with solid tumors: an open-label, multicenter phase 1b study. Target Oncol. (2015) 10:111–23. doi: 10.1007/s11523-014-0320-2
82. Xu H, Zhang S, Sathe AA, Jin Z, Guan J, Sun W, et al. CCR2(+) macrophages promote orthodontic tooth movement and alveolar bone remodeling. Front Immunol. (2022) 13:835986. doi: 10.3389/fimmu.2022.835986
83. Xu M, Wang Y, Xia R, Wei Y, Wei X. Role of the CCL2-CCR2 signalling axis in cancer: Mechanisms and therapeutic targeting. Cell Prolif. (2021) 54:e13115. doi: 10.1111/cpr.13115
84. Gilbert J, Lekstrom-Himes J, Donaldson D, Lee Y, Hu M, Xu J, et al. Effect of CC chemokine receptor 2 CCR2 blockade on serum C-reactive protein in individuals at atherosclerotic risk and with a single nucleotide polymorphism of the monocyte chemoattractant protein-1 promoter region. Am J Cardiol. (2011) 107:906–11. doi: 10.1016/j.amjcard.2010.11.005
85. Raghu H, Lepus CM, Wang Q, Wong HH, Lingampalli N, Oliviero F, et al. CCL2/CCR2, but not CCL5/CCR5, mediates monocyte recruitment, inflammation and cartilage destruction in osteoarthritis. Ann Rheum Dis. (2017) 76:914–22. doi: 10.1136/annrheumdis-2016-210426
86. Kobayashi EH, Suzuki T, Funayama R, Nagashima T, Hayashi M, Sekine H, et al. Nrf2 suppresses macrophage inflammatory response by blocking proinflammatory cytokine transcription. Nat Commun. (2016) 7:11624. doi: 10.1038/ncomms11624
87. Mitsuishi Y, Taguchi K, Kawatani Y, Shibata T, Nukiwa T, Aburatani H, et al. Nrf2 redirects glucose and glutamine into anabolic pathways in metabolic reprogramming. Cancer Cell. (2012) 22:66–79. doi: 10.1016/j.ccr.2012.05.016
88. Pillai R, Hayashi M, Zavitsanou AM, Papagiannakopoulos T. NRF2: KEAPing tumors protected. Cancer Discovery. (2022) 12:625–43. doi: 10.1158/2159-8290.CD-21-0922
89. Yu X, Liu Y, Pan K, Sun P, Li J, Li L, et al. Breast cancer susceptibility gene 1 regulates oxidative damage via nuclear factor erythroid 2-related factor 2 in oral cancer cells. Arch Oral Biol. (2022) 139:105447. doi: 10.1016/j.archoralbio.2022.105447
90. Tang YC, Hsiao JR, Jiang SS, Chang JY, Chu PY, Liu KJ, et al. c-MYC-directed NRF2 drives Malignant progression of head and neck cancer via glucose-6-phosphate dehydrogenase and transketolase activation. Theranostics. (2021) 11:5232–47. doi: 10.7150/thno.53417
91. Kohno M, Kamachi T, Fukui K. Intermolecular binding between bulk water and dissolved gases in earth's magnetic field. PloS One. (2022) 17:e0267391. doi: 10.1371/journal.pone.0267391
92. Baird L, Yamamoto M. The molecular mechanisms regulating the KEAP1-NRF2 pathway. Mol Cell Biol. (2020) 40. doi: 10.1128/MCB.00099-20
93. Ulasov AV, Rosenkranz AA, Georgiev GP, Sobolev AS. Nrf2/Keap1/ARE signaling: Towards specific regulation. Life Sci. (2022) 291:120111. doi: 10.1016/j.lfs.2021.120111
94. Singh A, Venkannagari S, Oh KH, Zhang YQ, Rohde JM, Liu L, et al. Small molecule inhibitor of NRF2 selectively intervenes therapeutic resistance in KEAP1-deficient NSCLC tumors. ACS Chem Biol. (2016) 11:3214–25. doi: 10.1021/acschembio.6b00651
95. Takizawa H, Manz MG. Macrophage tolerance: CD47-SIRP-alpha-mediated signals matter. Nat Immunol. (2007) 8:1287–9. doi: 10.1038/ni1207-1287
96. Li Y, Zhang M, Wang X, Liu W, Wang H, Yang YG. Vaccination with CD47 deficient tumor cells elicits an antitumor immune response in mice. Nat Commun. (2020) 11:581. doi: 10.1038/s41467-019-14102-4
97. Vonderheide RH. CD47 blockade as another immune checkpoint therapy for cancer. Nat Med. (2015) 21:1122–3. doi: 10.1038/nm.3965
98. Yang H, Shao R, Huang H, Wang X, Rong Z, Lin Y. Engineering macrophages to phagocytose cancer cells by blocking the CD47/SIRPa axis. Cancer Med. (2019) 8:4245–53. doi: 10.1002/cam4.2332
99. Ni H, Cao L, Wu Z, Wang L, Zhou S, Guo X, et al. Combined strategies for effective cancer immunotherapy with a novel anti-CD47 monoclonal antibody. Cancer Immunol Immunother. (2022) 71:353–63. doi: 10.1007/s00262-021-02989-2
100. Lee WH, Kim SH, An JH, Kim TK, Cha HJ, Chang HW, et al. Tristetraprolin regulates phagocytosis through interaction with CD47 in head and neck cancer. Exp Ther Med. (2022) 24:541. doi: 10.3892/etm
101. Sakakura K, Takahashi H, Kaira K, Toyoda M, Murata T, Ohnishi H, et al. Relationship between tumor-associated macrophage subsets and CD47 expression in squamous cell carcinoma of the head and neck in the tumor microenvironment. Lab Invest. (2016) 96:994–1003. doi: 10.1038/labinvest.2016.70
102. Sikic BI, Lakhani N, Patnaik A, Shah SA, Chandana SR, Rasco D, et al. First-in-human, first-in-class phase I trial of the anti-CD47 antibody hu5F9-G4 in patients with advanced cancers. J Clin Oncol. (2019) 37:946–53. doi: 10.1200/JCO.18.02018
103. Petrova PS, Viller NN, Wong M, Pang X, Lin GH, Dodge K, et al. TTI-621 (SIRPalphaFc): A CD47-blocking innate immune checkpoint inhibitor with broad antitumor activity and minimal erythrocyte binding. Clin Cancer Res. (2017) 23:1068–79. doi: 10.1158/1078-0432.CCR-16-1700
104. de Araujo Farias V, Carrillo-Galvez AB, Martin F, Anderson P. TGF-beta and mesenchymal stromal cells in regenerative medicine, autoimmunity and cancer. Cytokine Growth Factor Rev. (2018) 43:25–37. doi: 10.1016/j.cytogfr.2018.06.002
105. Batlle E, Massague J. Transforming growth factor-beta signaling in immunity and cancer. Immunity. (2019) 50:924–40. doi: 10.1016/j.immuni.2019.03.024
106. Kelly A, Houston SA, Sherwood E, Casulli J, Travis MA. Regulation of innate and adaptive immunity by TGFbeta. Adv Immunol. (2017) 134:137–233. doi: 10.1016/bs.ai.2017.01.001
107. Liu F, Qiu H, Xue M, Zhang S, Zhang X, Xu J, et al. MSC-secreted TGF-beta regulates lipopolysaccharide-stimulated macrophage M2-like polarization via the Akt/FoxO1 pathway. Stem Cell Res Ther. (2019) 10:345. doi: 10.1186/s13287-019-1447-y
108. Chen Y, Ding X, Bai X, Zhou Z, Liu Y, Zhang X, et al. The current advances and future directions of PD-1/PD-L1 blockade in head and neck squamous cell carcinoma (HNSCC) in the era of immunotherapy. Int Immunopharmacol. (2023) 120:110329. doi: 10.1016/j.intimp.2023.110329
109. Cramer JD, Burtness B, Ferris RL. Immunotherapy for head and neck cancer: Recent advances and future directions. Oral Oncol. (2019) 99:104460. doi: 10.1016/j.oraloncology.2019.104460
110. Sun JY, Zhang D, Wu S, Xu M, Zhou X, Lu XJ, et al. Resistance to PD-1/PD-L1 blockade cancer immunotherapy: mechanisms, predictive factors, and future perspectives. biomark Res. (2020) 8:35. doi: 10.1186/s40364-020-00212-5
111. Chen DS, Mellman I. Elements of cancer immunity and the cancer-immune set point. Nature. (2017) 541:321–30. doi: 10.1038/nature21349
112. Yi M, Niu M, Wu Y, Ge H, Jiao D, Zhu S, et al. Combination of oral STING agonist MSA-2 and anti-TGF-beta/PD-L1 bispecific antibody YM101: a novel immune cocktail therapy for non-inflamed tumors. J Hematol Oncol. (2022) 15:142. doi: 10.1186/s13045-022-01363-8
113. Bai X, Yi M, Jiao Y, Chu Q, Wu K. Blocking TGF-beta signaling to enhance the efficacy of immune checkpoint inhibitor. Onco Targets Ther. (2019) 12:9527–38. doi: 10.2147/OTT
114. Mariathasan S, Turley SJ, Nickles D, Castiglioni A, Yuen K, Wang Y, et al. TGFbeta attenuates tumour response to PD-L1 blockade by contributing to exclusion of T cells. Nature. (2018) 554:544–8. doi: 10.1038/nature25501
115. Zhu S, Zhang T, Zheng L, Liu H, Song W, Liu D, et al. Combination strategies to maximize the benefits of cancer immunotherapy. J Hematol Oncol. (2021) 14:156. doi: 10.1186/s13045-021-01164-5
116. Yi M, Zhang J, Li A, Niu M, Yan Y, Jiao Y, et al. The construction, expression, and enhanced anti-tumor activity of YM101: a bispecific antibody simultaneously targeting TGF-beta and PD-L1. J Hematol Oncol. (2021) 14:27. doi: 10.1186/s13045-021-01045-x
117. Yi M, Niu M, Zhang J, Li S, Zhu S, Yan Y, et al. Combine and conquer: manganese synergizing anti-TGF-beta/PD-L1 bispecific antibody YM101 to overcome immunotherapy resistance in non-inflamed cancers. J Hematol Oncol. (2021) 14:146. doi: 10.1186/s13045-021-01155-6
118. Yi M, Wu Y, Niu M, Zhu S, Zhang J, Yan Y, et al. Anti-TGF-beta/PD-L1 bispecific antibody promotes T cell infiltration and exhibits enhanced antitumor activity in triple-negative breast cancer. J Immunother Cancer. (2022) 10. doi: 10.1136/jitc-2022-005543
119. Matos AI, Peres C, Carreira B, Moura LIF, Acurcio RC, Vogel T, et al. Polyoxazoline-based nanovaccine synergizes with tumor-associated macrophage targeting and anti-PD-1 immunotherapy against solid tumors. Adv Sci (Weinh). (2023) 10:e2300299. doi: 10.1002/advs.202300299
120. Beltraminelli T, De Palma M. Biology and therapeutic targeting of tumour-associated macrophages. J Pathol. (2020) 250:573–92. doi: 10.1002/path.5403
121. Cassetta L, Pollard JW. Targeting macrophages: therapeutic approaches in cancer. Nat Rev Drug Discovery. (2018) 17:887–904. doi: 10.1038/nrd.2018.169
122. Lamb YN. Pexidartinib: first approval. Drugs. (2019) 79:1805–12. doi: 10.1007/s40265-019-01210-0
123. Xu J, Shen L, Zhou Z, Li J, Bai C, Chi Y, et al. Surufatinib in advanced extrapancreatic neuroendocrine tumours (SANET-ep): a randomised, double-blind, placebo-controlled, phase 3 study. Lancet Oncol. (2020) 21:1500–12. doi: 10.1016/S1470-2045(20)30496-4
124. Liu S, Liu J, Wang J, Cheng J, Zeng X, Ji N, et al. RACK1 is an organ-specific prognostic predictor in OSCC. Oral Oncol. (2018) 76:22–6. doi: 10.1016/j.oraloncology.2017.10.025
125. Yu Z, Jiang X, Qin L, Deng H, Wang J, Ren W, et al. A novel UBE2T inhibitor suppresses Wnt/beta-catenin signaling hyperactivation and gastric cancer progression by blocking RACK1 ubiquitination. Oncogene. (2021) 40:1027–42. doi: 10.1038/s41388-020-01572-w
126. Galluzzi L, Humeau J, Buque A, Zitvogel L, Kroemer G. Immunostimulation with chemotherapy in the era of immune checkpoint inhibitors. Nat Rev Clin Oncol. (2020) 17:725–41. doi: 10.1038/s41571-020-0413-z
127. Lopez-Yrigoyen M, Cassetta L, Pollard JW. Macrophage targeting in cancer. Ann N Y Acad Sci. (2021) 1499:18–41. doi: 10.1111/nyas.14377
128. Szturz P, Vermorken JB. Immunotherapy in head and neck cancer: aiming at EXTREME precision. BMC Med. (2017) 15:110. doi: 10.1186/s12916-017-0879-4
129. Echarri MJ, Lopez-Martin A, Hitt R. Targeted therapy in locally advanced and recurrent/metastatic head and neck squamous cell carcinoma (LA-R/M HNSCC). Cancers (Basel). (2016) 8. doi: 10.3390/cancers8030027
130. Sato-Kaneko F, Yao S, Ahmadi A, Zhang SS, Hosoya T, Kaneda MM, et al. Combination immunotherapy with TLR agonists and checkpoint inhibitors suppresses head and neck cancer. JCI Insight. (2017) 2. doi: 10.1172/jci.insight.93397
131. Wu Y, Chang X, Yang G, Chen L, Wu Q, Gao J, et al. A physiologically responsive nanocomposite hydrogel for treatment of head and neck squamous cell carcinoma via proteolysis-targeting chimeras enhanced immunotherapy. Adv Mater. (2023) 35:e2210787. doi: 10.1002/adma.202210787
132. Xia Y, Rao L, Yao H, Wang Z, Ning P, Chen X. Engineering macrophages for cancer immunotherapy and drug delivery. Adv Mater. (2020) 32:e2002054. doi: 10.1002/adma.202002054
133. Rao L, Zhao SK, Wen C, Tian R, Lin L, Cai B, et al. Activating macrophage-mediated cancer immunotherapy by genetically edited nanoparticles. Adv Mater. (2020) 32:e2004853. doi: 10.1002/adma.202004853
134. Zhang Y, Cai K, Li C, Guo Q, Chen Q, He X, et al. Macrophage-membrane-coated nanoparticles for tumor-targeted chemotherapy. Nano Lett. (2018) 18:1908–15. doi: 10.1021/acs.nanolett.7b05263
135. Rayamajhi S, Nguyen TDT, Marasini R, Aryal S. Macrophage-derived exosome-mimetic hybrid vesicles for tumor targeted drug delivery. Acta Biomater. (2019) 94:482–94. doi: 10.1016/j.actbio.2019.05.054
136. Jin A, Feng J, Wei G, Wu W, Yang L, Xu H, et al. CD19/CD22 chimeric antigen receptor T-cell therapy for refractory acute B-cell lymphoblastic leukemia with FLT3-ITD mutations. Bone Marrow Transplant. (2020) 55:717–21. doi: 10.1038/s41409-020-0807-7
137. Guilliams M, Thierry GR, Bonnardel J, Bajenoff M. Establishment and maintenance of the macrophage niche. Immunity. (2020) 52:434–51. doi: 10.1016/j.immuni.2020.02.015
138. Morrissey MA, Williamson AP, Steinbach AM, Roberts EW, Kern N, Headley MB, et al. Chimeric antigen receptors that trigger phagocytosis. Elife. (2018) 7. doi: 10.7554/eLife.36688
139. Zhang W, Liu L, Su H, Liu Q, Shen J, Dai H, et al. Chimeric antigen receptor macrophage therapy for breast tumours mediated by targeting the tumour extracellular matrix. Br J Cancer. (2019) 121:837–45. doi: 10.1038/s41416-019-0578-3
140. Klichinsky M, Ruella M, Shestova O, Lu XM, Best A, Zeeman M, et al. Human chimeric antigen receptor macrophages for cancer immunotherapy. Nat Biotechnol. (2020) 38:947–53. doi: 10.1038/s41587-020-0462-y
141. Wang S, Yang Y, Ma P, Zha Y, Zhang J, Lei A, et al. CAR-macrophage: An extensive immune enhancer to fight cancer. EBioMedicine. (2022) 76:103873. doi: 10.1016/j.ebiom.2022.103873
142. Kang M, Lee SH, Kwon M, Byun J, Kim D, Kim C, et al. Nanocomplex-mediated in vivo programming to chimeric antigen receptor-M1 macrophages for cancer therapy. Adv Mater. (2021) 33:e2103258. doi: 10.1002/adma.202103258
143. Kerneur C, Cano CE, Olive D. Major pathways involved in macrophage polarization in cancer. Front Immunol. (2022) 13:1026954. doi: 10.3389/fimmu.2022.1026954
144. Elmusrati A, Wang J, Wang CY. Tumor microenvironment and immune evasion in head and neck squamous cell carcinoma. Int J Oral Sci. (2021) 13:24. doi: 10.1038/s41368-021-00131-7
145. Ruffin AT, Li H, Vujanovic L, Zandberg DP, Ferris RL, Bruno TC. Improving head and neck cancer therapies by immunomodulation of the tumour microenvironment. Nat Rev Cancer. (2023) 23:173–88. doi: 10.1038/s41568-022-00531-9
146. Florido J, Martinez-Ruiz L, Rodriguez-Santana C, Lopez-Rodriguez A, Hidalgo-Gutierrez A, Cottet-Rousselle C, et al. Melatonin drives apoptosis in head and neck cancer by increasing mitochondrial ROS generated via reverse electron transport. J Pineal Res. (2022) 73:e12824. doi: 10.1111/jpi.12824
Keywords: head and neck squamous cell carcinoma, macrophages, tumor microenvironment, immunotherapies, engineering macrophages, CAR-M cell therapy
Citation: Lin C, Chu Y, Zheng Y, Gu S, Hu Y, He J and Shen Z (2024) Macrophages: plastic participants in the diagnosis and treatment of head and neck squamous cell carcinoma. Front. Immunol. 15:1337129. doi: 10.3389/fimmu.2024.1337129
Received: 12 November 2023; Accepted: 18 March 2024;
Published: 08 April 2024.
Edited by:
George S. Karagiannis, Albert Einstein College of Medicine, United StatesCopyright © 2024 Lin, Chu, Zheng, Gu, Hu, He and Shen. This is an open-access article distributed under the terms of the Creative Commons Attribution License (CC BY). The use, distribution or reproduction in other forums is permitted, provided the original author(s) and the copyright owner(s) are credited and that the original publication in this journal is cited, in accordance with accepted academic practice. No use, distribution or reproduction is permitted which does not comply with these terms.
*Correspondence: Zhisen Shen, c3pzNzIxNkAxNjMuY29t