- 1Department of Chemistry, University of Virginia, Charlottesville, VA, United States
- 2Department of Chemistry, Lehigh University, Bethlehem, PA, United States
Cytotoxic T lymphocytes are the primary effector immune cells responsible for protection against cancer, as they target peptide neoantigens presented through the major histocompatibility complex (MHC) on cancer cells, leading to cell death. Targeting peptide-MHC (pMHC) complex offers a promising strategy for immunotherapy due to their specificity and effectiveness against cancer. In this work, we exploit the acidic tumor micro-environment to selectively deliver antigenic peptides to cancer using pH(low) insertion peptides (pHLIP). We demonstrated the delivery of MHC binding peptides directly to the cytoplasm of melanoma cells resulted in the presentation of antigenic peptides on MHC, and activation of T cells. This work highlights the potential of pHLIP as a vehicle for the targeted delivery of antigenic peptides and its presentation via MHC-bound complexes on cancer cell surface for activation of T cells with implications for enhancing anti-cancer immunotherapy.
Introduction
The unmet need for effective and innovative treatments against cancer has underscored the importance of targeted delivery of therapeutic agents for cancer therapy (1). Cytotoxic effects associated with conventional cancer treatments have significantly limited their overall effectiveness (2). In addition, off-target effects also pose challenges in terms of dosing and administration by causing unwanted side effects to normal cells (3). Balancing the therapeutic benefits with the risk of toxicity is critical in minimizing damage to healthy tissue and to improve existing treatments. To overcome these limitations, there is an increasing demand for innovative cancer treatment strategies that can overcome the cytotoxicity-associated drawbacks and improve pharmacological properties.
An effective method to mitigate off-target impact involves linking therapeutic agents to well-defined carriers to precisely target cancer cells. Current advances in targeted cancer treatment primarily leverage the overexpression of specific biomarkers on cancer cell surfaces to administer high doses of therapeutic compounds more precisely. An important consideration in designing these types of therapeutics is the specific covalent attachment used to link the therapeutic payload (4). Numerous covalent linkers, including peptides, disulfides, and thioethers, have been employed to conjugate the therapeutic payload to the antibody (5–9). Additionally, other delivery systems such as nanoparticles, liposomes, and dendrimers have been engineered to target overexpressed biomarkers such as epidermal growth factor receptors, folate receptors, surface glycoproteins, and transferrin receptors to effectively target cancer (10–15). While targeting overexpressed biomarkers has been successful in increasing the effective concentration of therapeutic compounds in cells, the same biomarkers expressed at low levels on healthy cells have contributed to significant levels of off-target toxicity, highlighting the need to develop alternative strategies (16).
Alternatively, a strategy to enhance targeted delivery toward tumors involves exploiting the acidic microenvironment that is characteristic of solid tumors. Most tumors display rapid growth levels, which demands increased energy production via glycolysis. This metabolic shift results in the production of lactic acid as a byproduct and the expulsion of protons from the cancer cells into the extracellular space, a phenomenon known as the Warburg effect (17). As a result, the extracellular space surrounding cancer cells typically has an acidic pH ranging from 6.7 to 7.1 as opposed to a healthy pH range of 7.35 to 7.45. Interestingly, the environment closest to the cell surface is even more acidic; the pH can reach 6.1, making them an ideal target for pH(low) insertion peptides (pHLIPs) (18). The distinctive feature of pHLIP centers on its ability to specifically target the acidic microenvironment by undergoing a pH-dependent rearrangement. This pH-dependent change leads to the insertion of its C-terminus across the cell membrane, forming a transmembrane α-helix. (19, 20) Importantly, pHLIP can effectively target tumors, transport cargo, and facilitate the translocation of various payloads into the cytosol without needing cell receptor interactions or membrane pore formation (21–26). Previous studies have successfully harnessed pHLIP to deliver various drug molecules (including peptides) into solid tumors and metastatic sites in animal models (27–32).
A promising payload for targeted tumor therapies involves antigenic peptides for presentation on the major histocompatibility complex (MHC). MHC molecules can present antigenic peptides to cytotoxic T lymphocytes, initiating an immune response (33). Specifically, antigenic peptides presented on MHC class I molecules can be recognized by cytotoxic CD8+ T cell receptors (TCRs), triggering the release of perforin and granzyme B, ultimately leading to target cell death (34, 35). In the context of cancer, cancer cells often display unique peptide-MHC complexes due to various alterations in their proteome, such as protein mutations, aberrant post-translational modifications, and other cellular processes (36–38). These unique peptides, called neoantigens, are absent in healthy cells and enable the immune system to selectively target and eliminate cancer cells with high efficiency, particularly in cancers with a high mutational burden (39). However, targeting neoantigens in cancers with a low mutational burden or heterogenous neoantigen expression has shown limited success (40). Additionally, the negative selection of self-reactive T cells can prevent neoantigens from having strong anti-cancer activity (41). Therefore, delivering highly antigenic peptides that are orthogonal to endogenous neoantigens and prompting their presentation on MHC molecules represents a potential strategy for enhancing treatment outcomes.
Prior work by Irvine and colleagues highlighted the benefit of delivering antigenic peptides in a non-specific manner by using cell-penetrating peptides. This approach led to the improved display of antigenic peptides on MHC and the development of T cells specifically targeting the desired epitope (42). An advantage of cytosolic delivery of antigenic peptides is that it bypasses endosomal processing of peptides, which can lead to significant degradation of MHC-binding peptide epitopes before they can be presented on MHC (43). Additionally, recent clinical trials have demonstrated the effectiveness of delivering cancer-specific neoantigens for display on MHC in use for cancer therapy (44).
Building upon these findings, we posed that pHLIP could deliver antigenic peptides to cancer cells and this would offer a novel approach to selectively activate the immune system against cancer cells. Here, we showed that pHLIP conjugated to the model antigen SIINFEKL selectively translocated through the membrane of cells in low pH environments and becomes displayed on MHC (Figure 1). The peptide epitope SIINFEKL (OVA) comes from ovalbumin and there are no known human equivalents, thus making it an orthogonal antigenic peptide with high affinity towards MHC molecules. Notably, melanoma cells in acidic environments showed enhanced MHC display of the target epitope and increased recognition and activation by CD8+ T cells. These findings highlight the potential of pHLIP-mediated delivery of immunomodulatory agents.
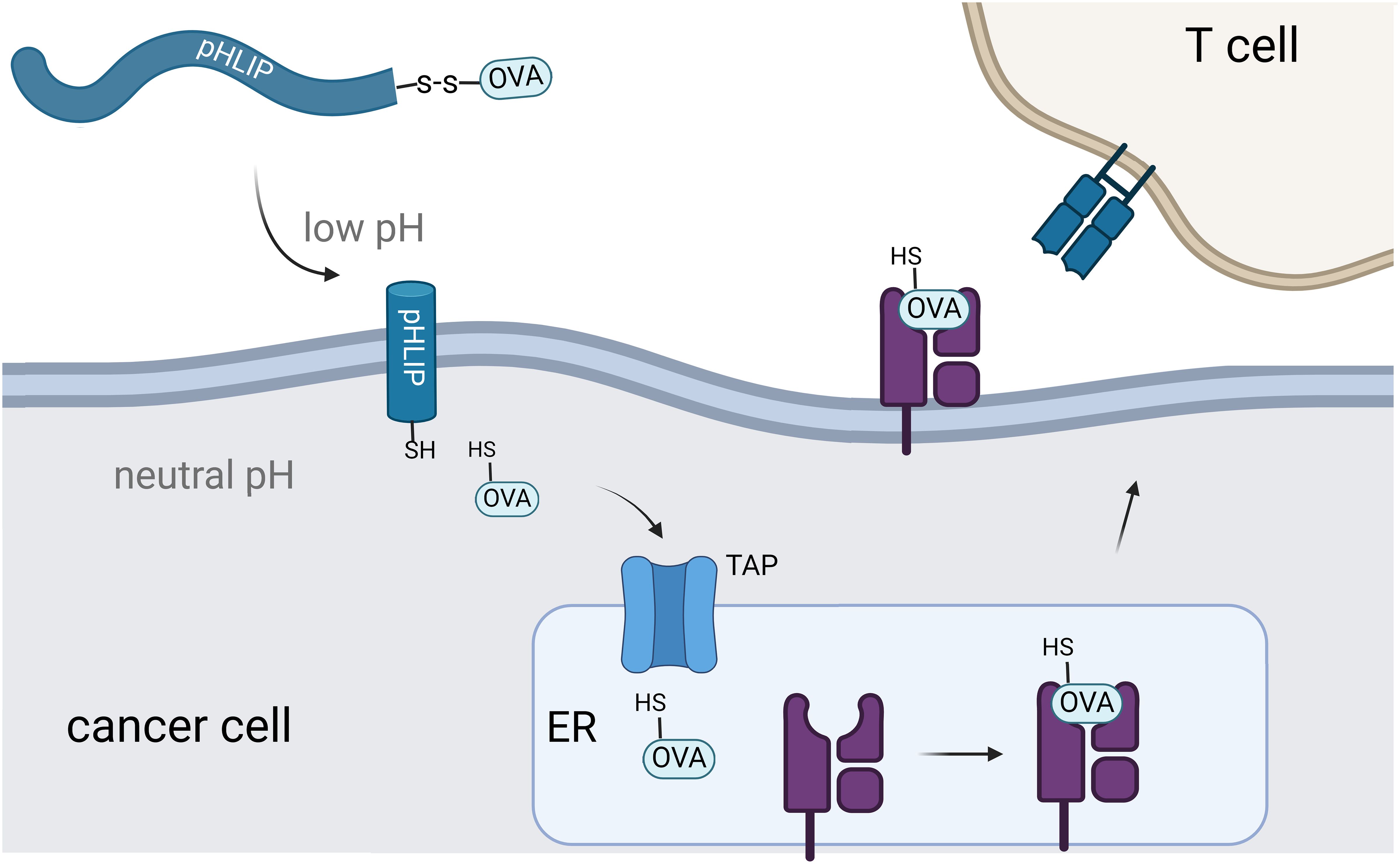
Figure 1 Schematic representation of pHLIP-mediated translocation of antigenic peptides for display on MHC. Intracellular delivery of the OVA peptide results in transportation to the endoplasmic reticulum for eventual display on MHC.
Results and discussion
We sought to link the OVA peptide to pHLIP via a chemical handle that would selectively uncouple upon arrival in the cytosolic space. While there are a number of strategies that would be compatible with our system, we selected to connect OVA to pHLIP via a disulfide bond. The selection of OVA was based on its considerable precedence as a model MHC binding antigen, and the rationale behind employing disulfide conjugation was to facilitate the release of the peptide in the reducing cytosolic environment to enable its entry into the antigen presentation pathway. To accomplish this strategy, it would be necessary to introduce a thiol group into the sequence of OVA. Our initial goal was to identify a site on OVA where adding a cysteine would have minimal impact on MHC binding and maintain recognition by OVA-specific TCRs, considering that structural alterations on peptide sequences can significantly influence both parameters (45, 46).
To assess changes in peptide affinity to MHC complexes (including thiol-modified OVA peptides), we used the RMA-S stabilization assay. The RMA-S cell line, which lacks the transporter associated with antigen processing (TAP), can be used to isolate the effect of peptide affinity because it lacks the ability to intracellularly process peptides for presentation (47, 48). Under low-temperature conditions (22-26°C), RMA-S cells present low-affinity pMHC complex on their cell surface. Increasing the temperature to 37°C causes the low-affinity pMHC complex to dissociate and become internalized and degraded. By introducing peptides with high affinity to bind to the MHC molecules, the pMHC complex remains stable on the cell surface at 37°C. The quantification of the peptide-MHC binding affinity, specifically to the H-2Kb haplotype on RMA-S cells, is determined using flow cytometry with a fluorescent anti-H-2Kb antibody.
Cysteine-containing OVA peptides were synthesized using a standard solid-phase peptide synthesis (SPPS) approach. Cysteine residues were introduced in sites within the OVA peptide that we projected would minimally impact binding to MHC molecules. These included the addition of cysteine to the termini of the sequence and replacement of residues for cysteine (Supplementary Table S1). Subsequently, RMA-S cells were incubated with the synthesized peptides at 26°C, allowing for the exchange of existing low-affinity pMHC complexes. Afterwards, the cells were then warmed to 37°C and treated with an APC-conjugated anti-mouse H-2Kb antibody. As expected, RMA-S cells treated with unmodified OVA peptide displayed high levels of MHC presentation on the cell surface (Figure 2A). The peptide SNFVSAGI (cntPEP) was used as a negative control as it has been reported to not appreciably bind to MHC (46, 49). Satisfyingly, the introduction of cysteine was well tolerated in most of the cysteine-modified OVA peptides; in particular, the cysteine introduction was better tolerated when the cysteine residues were located near the N-terminus. Nonetheless, all the cysteine-modified OVA peptides demonstrated substantial stabilization of the pMHC complex.
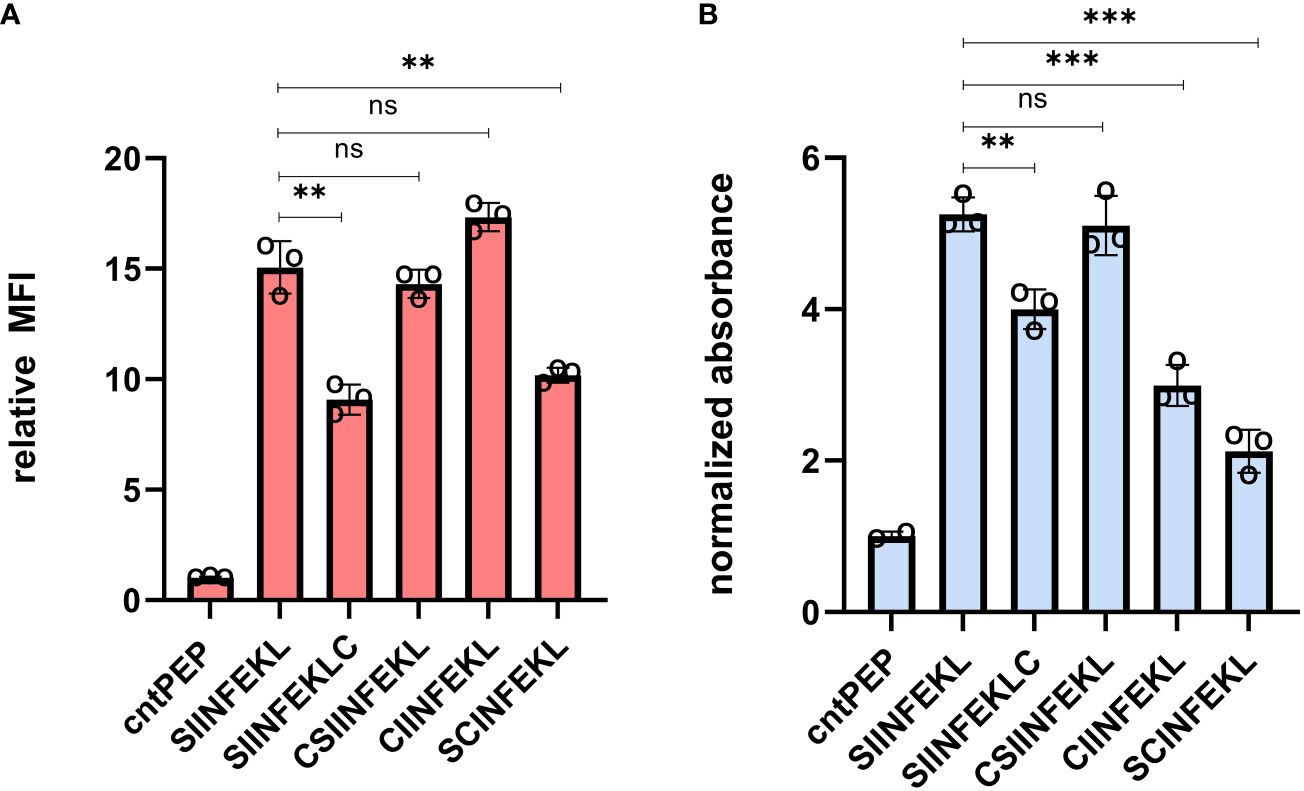
Figure 2 (A) Flow cytometry analysis of RMA-S cells. RMA-S cells were incubated with 20 μM peptide for 6 hours and pMHC complex display was quantified via APC conjugated anti-mouse H-2Kb antibodies. MFI is mean fluorescence intensity of the level of fluorescence relative to the control peptide. (B) RMA-S cells were incubated with 20 μM peptide and co-cultured with B3Z T cells for 8 hours at an effector-to-target ratio of 1:1. β-galactosidase expression was analyzed by measuring the hydrolysis of the colorimetric reagent CPRG on a plate reader at 570 nm and the data presented has been normalized to the absorbance from cntPEP. Data are represented as mean ± SD of biological replicates (n=3). P-values were determined by a two-tailed t-test (**p < 0.01, ***p < 0.001, ns, not significant).
Presentation of a cysteine-modified OVA peptide on MHC is critical for the success of our strategy, However, we appreciated that the cysteine modification could also alter the recognition of T cells by the OVA-specific TCR. TCRs can display altered binding affinity towards modified peptide sequences, prompting us to empirically assess how the position of cysteine might impact T cell activation. Previous findings, conducted by us and others, have indicated that changes to the OVA structure can potentially abolish TCR binding and subsequent T cell activation (46, 50). Additionally, we introduced cysteines in places reported to have the least disruptive effects to minimize any unfavorable interactions with SIINFEKL-specific TCRs (51).
We used the effector cells, B3Z T cells, to evaluate T cell activation of target cells displaying cysteine modified OVA peptides. These cells have OVA-specific TCRs and express the enzyme β-galactosidase under the control of an IL-2 inducible promoter upon activation of target cells. The hydrolysis of chlorophenol red-β-D-galactopyranoside (CPRG) by β-galactosidase leads to a measurable color change, reflecting the levels of T cell activation. RMA-S cells were incubated with the peptides at 26°C before being co-cultured with B3Z T cells at 37°C. In this assay, β-galactosidase expression was quantified by measuring the hydrolysis of CPRG at 570 nm. All cysteine-containing OVA peptides demonstrated the capability to activate T cells to varying levels (Figure 2B). However, amongst the peptides tested, CSIINFEKL (CysOVA) exhibited both high MHC binding and TCR activation levels, comparable to those of the wild-type OVA peptide. Therefore, we proceeded with CysOVA for conjugation to pHLIP.
Next, pHLIP was synthesized using SPPS and it included a corresponding cysteine added on its C-terminus. The disulfide conjugation between CysOVA and pHLIP was performed in solution, and the resulting conjugate (pHLIP-CysOVA) was purified via reverse phase high performance liquid chromatography (RP-HPLC). To determine the secondary structures of pHLIP-OVA in the presence of a lipid bilayer at neutral and acidic pH, we utilized far-ultraviolet circular dichroism (CD) spectroscopy. pHLIP-OVA was incubated in the presence of 200 nM 1-palmitoyl-2-oleoyl-sn-glycero-3-phosphocholine (POPC) liposomes at pH 7.4 or pH 5.0. As shown in Figure 3A, a pH-dependent conformational shift from an unstructured random coil (pH 7.4) to an α-helix (pH 5.0) characteristic of pHLIP’s behavior was observed. Tryptophan (Trp) fluorescence spectroscopy was also employed to validate the pH-dependent membrane insertion propensity of pHLIP-OVA. Fluorescence emission from the two Trp residues in the sequence of pHLIP is sensitive to environment polarity and thus reports on lipid membrane insertion. When the pH was lowered from pH 7.4 to pH 5.0, we observed a λmax blue shift indicating the Trp residues have transitioned to the hydrophobic environment of the lipid bilayer (Figure 3B). Together with the CD spectra, these results indicate that conjugation to CysOVA does not significantly impact the ability of pHLIP to insert across lipid membranes. This result agrees with the many previous studies showing that pHLIP can translocate a wide variety of C-terminally linked peptide cargoes across lipid bilayers (21, 24, 26, 32, 52).
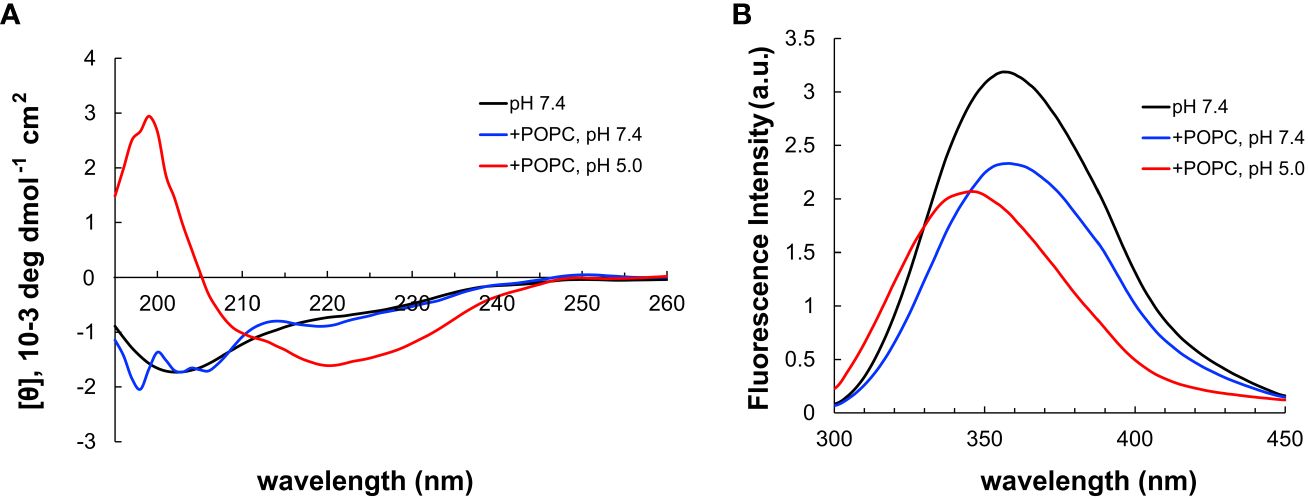
Figure 3 (A) CD spectrum of pHLIP-CysOVA in the presence of POPC vesicles at pH 7.4 or 5.0. (B) Tryptophan fluorescence spectra of pHLIP-CysOVA (20 μM) in the presence of POPC vesicles at pH 7.4 or 5.0.
Given that the length of peptides that bind to MHC class I molecules are in the range of 8-12 amino acids, we anticipated that the disulfide bond would have to be uncoupled to generate CysOVA before proper MHC binding. Therefore, we set out to investigate whether pHLIP-CysOVA needs to be reduced for enhanced antigen presentation on MHC. For this, we used the RMA-S stabilization assay to assess the relative affinity of the peptide to MHC before and after disulfide reduction at physiological pH. In this assay, RMA-S cells were incubated in the presence or absence of β-mercaptoethanol (BME) to cleave the disulfide linkage to assess changes in MHC binding affinity. Our results showed that cleaving the disulfide bond to generate CysOVA stabilized the peptide-MHC complex on RMA-S cells and significantly improved antigen presentation on the cell surface (Figure 4A). We observed cytotoxic effects of the RMA-S cells with higher concentrations of BME. Therefore, we believe that there may be incomplete reduction of the pHLIP-CysOVA in the presence of BME. There is also the possibility that CysOVA produces an in situ adduct with BME, which would change the stabilization levels. Overall, these results indicate that pHLIP-CysOVA must be reduced into CysOVA for optimal display on MHC.
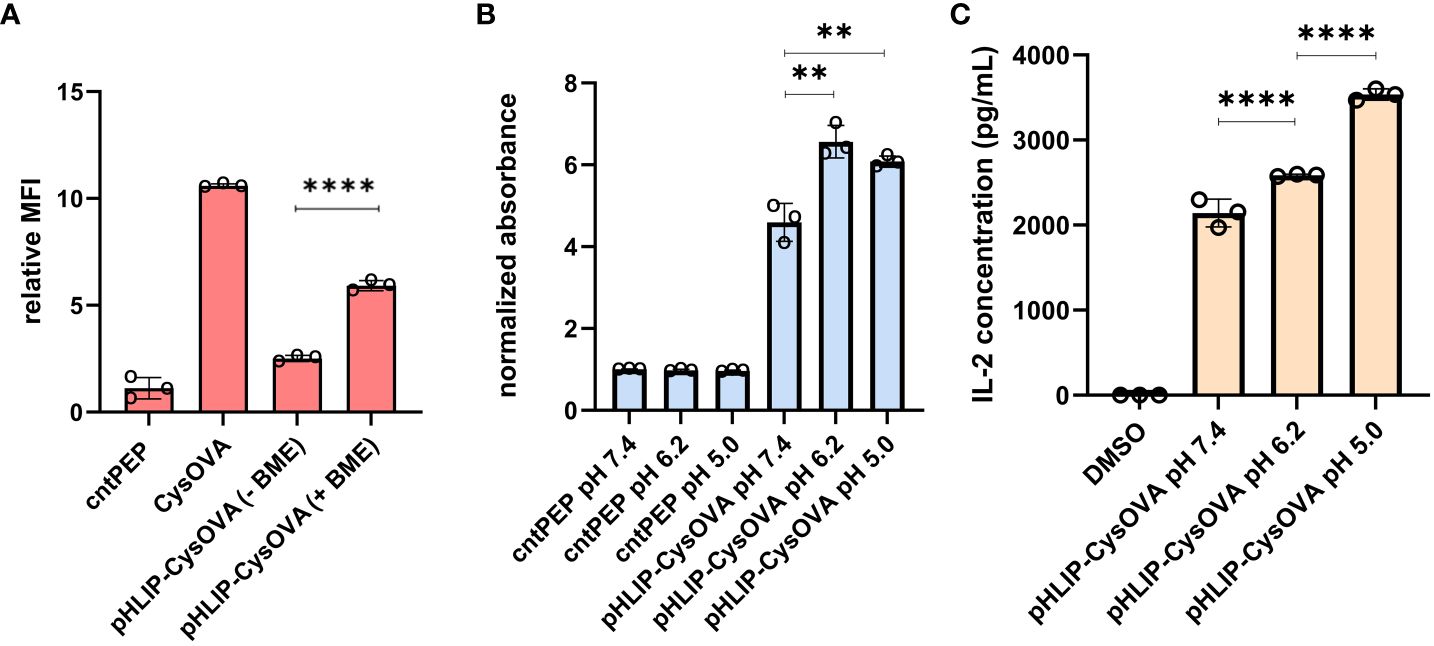
Figure 4 (A) RMA-S cells at physiological pH were incubated with 2.5 μM pHLIP-CysOVA for 6 hours in the presence or absence of BME. Cells were then labeled with anti-mouse H-2Kb antibody and analyzed via flow cytometry. MFI is mean fluorescence intensity of the level of fluorescence relative to the control peptide (B) 2.5 μM pHLIP-CysOVA was incubated with B16 cells for 5 mins before adjusting to the indicated pH for 10 mins. Cells were washed and co-cultured with B3Z T cells for 8 hours before lysing and measuring β-galactosidase activity via the colorimetric reagent CPRG on a plate reader at 570 nm and the data presented has been normalized to the absorbance from cntPEP. (C) B16 cells were treated with pHLIP-CysOVA for 5 mins before adjusting to pH 5 for 10 mins. Subsequently, these cells were washed and co-cultured with B3Z T cells overnight, with secreted IL-2 levels measured through ELISA. Data are represented as mean ± SD of biological replicates (n= 3). P-values were determined by a two-tailed t-test (**p<0.01, ****p < 0.0001).
To evaluate the efficacy of pHLIP-CysOVA in enabling antigen presentation on MHC in cells within acidic microenvironments, DC2.4 dendritic cells were subjected to incubation with pHLIP-CysOVA under neutral and acidic conditions. Cells were treated with pHLIP-CysOVA at either pH 7.4 or 5.0 for 10 mins at 37°C. Subsequently, cells were washed and were incubated with APC-conjugated 25-D1.16 antibodies (specific to SIINFEKL bound to H-2Kb) to quantify antigen presentation on MHC. Our data demonstrated a significant increase in antigen presentation on MHC in cells treated at low pH, indicating successful translocation of pHLIP-CysOVA across the membrane and entry into the antigen presentation pathway (Supplementary Figure S1).
Finally, to demonstrate that treatment at low pH enables selective immune activation of pHLIP-CysOVA in a clinically relevant model, B16 melanoma cells were employed. B16 cells were incubated with pHLIP-CysOVA at either physiological or low pH for 10 min. Following this incubation, the cells were washed and co-cultured with effector cells (B3Z T cells). Once again, treatment at lower pH conditions significantly enhanced T cell activations against the target B16 cells (Figure 4B). Satisfyingly, pHLIP-CysOVA also shows improved T cell activation against the melanoma model at a more physiologically relevant pH of 6.2 (Figure 4B). Noteworthy, cells treated with CysOVA peptide alone did not show any pH dependent T cell activation (Supplementary Figure S2). Additionally, ELISA results confirmed that pHLIP-CysOVA treatment at low pH induced significant T cell activation through elevated IL-2 cytokine secretion from B3Z T cells (Figure 4C). Taken together, these findings demonstrate selective activation of T cells in response to pHLIP-CysOVA treatment within acidic environments.
Conclusion
In this study, we have described a targeted approach to deliver antigenic peptides specifically to cancer cells, thereby aiding their presentation on MHC molecules to enhance immune activation. Our method used pHLIP to deliver CysOVA directly to the cytosol, allowing for the cytoplasmic delivery of exogenously derived peptides. Traditionally, antigen-presenting cells would be required to translocate exogenous peptides into the cytosol for display on MHC class I through a process called cross-presentation (53). By avoiding this step, we can deliver peptides to non-antigen presenting cells.
A key advantage of our approach is the versatility of pHLIP, which has previously demonstrated the ability to translocate a wide range of molecules, including hydrophilic peptides, across cell membranes (26). We envision that our strategy can be adapted to deliver various potential neoantigens, thereby broadening its applicability and therapeutic potential. Additionally, it was recently reported that MHC peptides tagged with distinct covalent small-molecule inhibitors can be targeted by immune cells for immunotherapy applications (54). Consequently, we anticipate that pHLIP can serve as a delivery vehicle for chemically modified peptides, potentially used alongside antibodies or CAR-T cells to enable precision immunotherapy against cancer (55).
However, developing a robust T cell response towards pMHC complexes is hindered by the variety of ways in which cancer evades immune recognition. One widely reported mechanism for immune escape is the downregulation of MHC which impairs their ability to display antigenic peptides (56, 57). Indeed, non-responders to immune checkpoint inhibition often possess cancer mutations that decrease the presentation of peptides on the cell surface via MHC (58). Furthermore, the acidic cancer microenvironment is known to diminish T cells activity against tumors (59–61). Another means by which CD8+ T cells become less effective against tumors is through the immunosuppressive activity of regulatory CD4+ T cells (62). Therefore, effective immunotherapy strategies will likely require a multifactorial approach in order to overcome these limitations related to immune evasion.
Data availability statement
The original contributions presented in the study are included in the article/Supplementary Materials. Further inquiries can be directed to the corresponding author.
Author contributions
JK: Conceptualization, Data curation, Formal analysis, Investigation, Methodology, Visualization, Writing – original draft, Writing – review & editing. EA: Data curation, Formal analysis, Investigation, Methodology, Visualization, Writing – original draft. SN: Writing – review & editing, Investigation. DT: Conceptualization, Formal analysis, Funding acquisition, Investigation, Methodology, Supervision, Writing – original draft, Writing – review & editing. MP: Conceptualization, Formal analysis, Funding acquisition, Investigation, Methodology, Supervision, Visualization, Writing – original draft, Writing – review & editing.
Funding
The author(s) declare financial support was received for the research, authorship, and/or publication of this article. This study was supported by the NIH grant R21 CA259800.
Conflict of interest
The authors declare that the research was conducted in the absence of any commercial or financial relationships that could be construed as a potential conflict of interest.
Publisher’s note
All claims expressed in this article are solely those of the authors and do not necessarily represent those of their affiliated organizations, or those of the publisher, the editors and the reviewers. Any product that may be evaluated in this article, or claim that may be made by its manufacturer, is not guaranteed or endorsed by the publisher.
Supplementary material
The Supplementary Material for this article can be found online at: https://www.frontiersin.org/articles/10.3389/fimmu.2024.1337973/full#supplementary-material
References
1. Manzari MT, Shamay Y, Kiguchi H, Rosen N, Scaltriti M, Heller DA. Targeted drug delivery strategies for precision medicines. Nat Rev Mater. (2021) 6:351–70. doi: 10.1038/s41578-020-00269-6
2. Rothenberg ML, Carbone DP, Johnson DH. Improving the evaluation of new cancer treatments: challenges and opportunities. Nat Rev Cancer. (2003) 3:303–9. doi: 10.1038/nrc1047
3. Zhong L, Li Y, Xiong L, Wang W, Wu M, Yuan T, et al. Small molecules in targeted cancer therapy: advances, challenges, and future perspectives. Signal Transduct Target Ther. (2021) 6:201. doi: 10.1038/s41392-021-00572-w
4. Chari RV. Targeted cancer therapy: conferring specificity to cytotoxic drugs. Acc Chem Res. (2008) 41:98–107. doi: 10.1021/ar700108g
5. Walles M, Connor A, Hainzl D. ADME and safety aspects of non-cleavable linkers in drug discovery and development. Curr Top Med Chem. (2017) 17:3463–75. doi: 10.2174/1568026618666180118153502
6. Pettinato MC. Introduction to antibody-drug conjugates. Antibod (Basel). (2021) 10. doi: 10.3390/antib10040042
7. Ford CH, Newman CE, Johnson JR, Woodhouse CS, Reeder TA, Rowland GF, et al. Localization and toxicity study of a vindesine-anti-CEA conjugate in patients with advanced cancer. Br J Cancer. (1983) 47:35–42. doi: 10.1038/bjc.1983.4
8. Tsuchikama K, An Z. Antibody-drug conjugates: recent advances in conjugation and linker chemistries. Protein Cell. (2018) 9:33–46. doi: 10.1007/s13238-016-0323-0
9. Erickson HK, Park PU, Widdison WC, Kovtun YV, Garrett LM, Hoffman K, et al. Antibody-maytansinoid conjugates are activated in targeted cancer cells by lysosomal degradation and linker-dependent intracellular processing. Cancer Res. (2006) 66:4426–33. doi: 10.1158/0008-5472.CAN-05-4489
10. Rosenblum D, Joshi N, Tao W, Karp JM, Peer D. Progress and challenges towards targeted delivery of cancer therapeutics. Nat Commun. (2018) 9:1410. doi: 10.1038/s41467-018-03705-y
11. Palmerston Mendes L, Pan J, Torchilin VP. Dendrimers as nanocarriers for nucleic acid and drug delivery in cancer therapy. Molecules. (2017) 22. doi: 10.3390/molecules22091401
12. Master AM, Sen Gupta A. EGF receptor-targeted nanocarriers for enhanced cancer treatment. Nanomedicine (Lond). (2012) 7:1895–906. doi: 10.2217/nnm.12.160
13. Ebrahimnejad P, Sodagar Taleghani A, Asare-Addo K, Nokhodchi A. An updated review of folate-functionalized nanocarriers: A promising ligand in cancer. Drug Discovery Today. (2022) 27:471–89. doi: 10.1016/j.drudis.2021.11.011
14. Bloise N, Okkeh M, Restivo E, Della Pina C, Visai L. Targeting the "Sweet side" of tumor with glycan-binding molecules conjugated-nanoparticles: implications in cancer therapy and diagnosis. Nanomater (Basel). (2021) 11. doi: 10.3390/nano11020289
15. Ramalho MJ, Loureiro JA, Coelho MAN, Pereira MC. Transferrin receptor-targeted nanocarriers: overcoming barriers to treat glioblastoma. Pharmaceutics. (2022) 14. doi: 10.3390/pharmaceutics14020279
16. Akhtar MJ, Ahamed M, Alhadlaq HA, Alrokayan SA, Kumar S. Targeted anticancer therapy: overexpressed receptors and nanotechnology. Clin Chim Acta. (2014) 436:78–92. doi: 10.1016/j.cca.2014.05.004
17. Boedtkjer E, Pedersen SF. The acidic tumor microenvironment as a driver of cancer. Annu Rev Physiol. (2020) 82:103–26. doi: 10.1146/annurev-physiol-021119-034627
18. Anderson M, Moshnikova A, Engelman DM, Reshetnyak YK, Andreev OA. Probe for the measurement of cell surface pH in vivo and ex vivo. Proc Natl Acad Sci U.S.A. (2016) 113:8177–81. doi: 10.1073/pnas.1608247113
19. Reshetnyak YK, Andreev OA, Segala M, Markin VS, Engelman DM. Energetics of peptide (pHLIP) binding to and folding across a lipid bilayer membrane. Proc Natl Acad Sci U.S.A. (2008) 105:15340–5. doi: 10.1073/pnas.0804746105
20. Reshetnyak YK, Segala M, Andreev OA, Engelman DM. A monomeric membrane peptide that lives in three worlds: in solution, attached to, and inserted across lipid bilayers. Biophys J. (2007) 93:2363–72. doi: 10.1529/biophysj.107.109967
21. Thevenin D, An M, Engelman DM. pHLIP-mediated translocation of membrane-impermeable molecules into cells. Chem Biol. (2009) 16:754–62. doi: 10.1016/j.chembiol.2009.06.006
22. An M, Wijesinghe D, Andreev OA, Reshetnyak YK, Engelman DM. pH-(low)-insertion-peptide (pHLIP) translocation of membrane impermeable phalloidin toxin inhibits cancer cell proliferation. Proc Natl Acad Sci U.S.A. (2010) 107:20246–50. doi: 10.1073/pnas.1014403107
23. Burns KE, Robinson MK, Thevenin D. Inhibition of cancer cell proliferation and breast tumor targeting of pHLIP-monomethyl auristatin E conjugates. Mol Pharm. (2015) 12:1250–8. doi: 10.1021/mp500779k
24. Burns KE, Thevenin D. Down-regulation of PAR1 activity with a pHLIP-based allosteric antagonist induces cancer cell death. Biochem J. (2015) 472:287–95. doi: 10.1042/BJ20150876
25. Onyango JO, Chung MS, Eng CH, Klees LM, Langenbacher R, Yao L, et al. Noncanonical amino acids to improve the pH response of pHLIP insertion at tumor acidity. Angew Chem Int Ed Engl. (2015) 54:3658–63. doi: 10.1002/anie.201409770
26. Burns KE, McCleerey TP, Thevenin D. pH-selective cytotoxicity of pHLIP-antimicrobial peptide conjugates. Sci Rep. (2016) 6:28465. doi: 10.1038/srep28465
27. Cheng CJ, Bahal R, Babar IA, Pincus Z, Barrera F, Liu C, et al. MicroRNA silencing for cancer therapy targeted to the tumor microenvironment. Nature. (2015) 518:107–10. doi: 10.1038/nature13905
28. Reshetnyak YK, Yao L, Zheng S, Kuznetsov S, Engelman DM, Andreev OA. Measuring tumor aggressiveness and targeting metastatic lesions with fluorescent pHLIP. Mol Imaging Biol. (2011) 13:1146–56. doi: 10.1007/s11307-010-0457-z
29. Adochite RC, Moshnikova A, Carlin SD, Guerrieri RA, Andreev OA, Lewis JS, et al. Targeting breast tumors with pH (low) insertion peptides. Mol Pharm. (2014) 11:2896–905. doi: 10.1021/mp5002526
30. Karabadzhak AG, Weerakkody D, Wijesinghe D, Thakur MS, Engelman DM, Andreev OA, et al. Modulation of the pHLIP transmembrane helix insertion pathway. Biophys J. (2012) 102:1846–55. doi: 10.1016/j.bpj.2012.03.021
31. Burns KE, Hensley H, Robinson MK, Thevenin D. Therapeutic efficacy of a family of pHLIP-MMAF conjugates in cancer cells and mouse models. Mol Pharm. (2017) 14:415–22. doi: 10.1021/acs.molpharmaceut.6b00847
32. Gerhart J, Thévenin AF, Bloch E, King KE, Thévenin D. Inhibiting epidermal growth factor receptor dimerization and signaling through targeted delivery of a juxtamembrane domain peptide mimic. ACS Chem Biol. (2018) 13:2623–32. doi: 10.1021/acschembio.8b00555
33. Neefjes J, Jongsma ML, Paul P, Bakke O. Towards a systems understanding of MHC class I and MHC class II antigen presentation. Nat Rev Immunol. (2011) 11:823–36. doi: 10.1038/nri3084
34. Huppa JB, Davis MM. T-cell-antigen recognition and the immunological synapse. Nat Rev Immunol. (2003) 3:973–83. doi: 10.1038/nri1245
35. Gorentla BK, Zhong XP. T cell Receptor Signal Transduction in T lymphocytes. J Clin Cell Immunol. (2012) 2012:5. doi: 10.4172/2155-9899.S12-005
36. Xie N, Shen G, Gao W, Huang Z, Huang C, Fu L. Neoantigens: promising targets for cancer therapy. Signal Transduct Target Ther. (2023) 8:9. doi: 10.1038/s41392-022-01270-x
37. Engelhard VH, Obeng RC, Cummings KL, Petroni GR, Ambakhutwala AL, Chianese-Bullock KA, et al. MHC-restricted phosphopeptide antigens: preclinical validation and first-in-humans clinical trial in participants with high-risk melanoma. J Immunother Cancer. (2020) 8. doi: 10.1136/jitc-2019-000262
38. Ouspenskaia T, Law T, Clauser KR, Klaeger S, Sarkizova S, Aguet F, et al. Unannotated proteins expand the MHC-I-restricted immunopeptidome in cancer. Nat Biotechnol. (2022) 40:209–17. doi: 10.1038/s41587-021-01021-3
39. Pearlman AH, Hwang MS, Konig MF, Hsiue EH, Douglass J, DiNapoli SR, et al. Targeting public neoantigens for cancer immunotherapy. Nat Cancer. (2021) 2:487–97. doi: 10.1038/s43018-021-00210-y
40. Lang F, Schrors B, Lower M, Tureci O, Sahin U. Identification of neoantigens for individualized therapeutic cancer vaccines. Nat Rev Drug Discovery. (2022) 21:261–82. doi: 10.1038/s41573-021-00387-y
41. Miller JFAP, Basten A. Mechanisms of tolerance to self. Curr Opin Immunol. (1996) 8:815–21. doi: 10.1016/S0952-7915(96)80010-0
42. Backlund CM, Holden RL, Moynihan KD, Garafola D, Farquhar C, Mehta NK, et al. Cell-penetrating peptides enhance peptide vaccine accumulation and persistence in lymph nodes to drive immunogenicity. Proc Natl Acad Sci U.S.A. (2022) 119:e2204078119. doi: 10.1073/pnas.2204078119
43. Embgenbroich M, Burgdorf S. Current concepts of antigen cross-presentation. Front Immunol. (2018) 9:1643. doi: 10.3389/fimmu.2018.01643
44. Niemi JVL, Sokolov AV, Schioth HB. Neoantigen vaccines; clinical trials, classes, indications, adjuvants and combinatorial treatments. Cancers (Basel). (2022) 14. doi: 10.3390/cancers14205163
45. Pawlak JB, Hos BJ, van de Graaff MJ, Megantari OA, Meeuwenoord N, Overkleeft HS, et al. The optimization of bioorthogonal epitope ligation within MHC-I complexes. ACS Chem Biol. (2016) 11:3172–8. doi: 10.1021/acschembio.6b00498
46. Kelly JJ, Dalesandro BE, Liu Z, Chordia MD, Ongwae GM, Pires MM. Measurement of accumulation of antibiotics to staphylococcus aureus in phagosomes of live macrophages. bioRxiv. (2023). doi: 10.1101/2023.02.13.528196
47. Ljunggren HG, Stam NJ, Ohlen C, Neefjes JJ, Hoglund P, Heemels MT, et al. Empty MHC class I molecules come out in the cold. Nature. (1990) 346:476–80. doi: 10.1038/346476a0
48. Schumacher TN, Heemels MT, Neefjes JJ, Kast WM, Melief CJ, Ploegh HL. Direct binding of peptide to empty MHC class I molecules on intact cells and. vitro Cell. (1990) 62:563–7. doi: 10.1016/0092-8674(90)90020-F
49. Dubey P, Hendrickson RC, Meredith SC, Siegel CT, Shabanowitz J, Skipper JC, et al. The immunodominant antigen of an ultraviolet-induced regressor tumor is generated by a somatic point mutation in the DEAD box helicase p68. J Exp Med. (1997) 185:695–705. doi: 10.1084/jem.185.4.695
50. van der Gracht AMF, de Geus MAR, Camps MGM, Ruckwardt TJ, Sarris AJC, Bremmers J, et al. Chemical Control over T-Cell Activation in Vivo Using Deprotection of trans-Cyclooctene-Modified Epitopes. ACS Chem Biol. (2018) 13:1569–76. doi: 10.1021/acschembio.8b00155
51. Messaoudi I, LeMaoult J, Nikolic-Zugic J. The mode of ligand recognition by two peptide:MHC class I-specific monoclonal antibodies. J Immunol. (1999) 163:3286–94. doi: 10.4049/jimmunol.163.6.3286
52. Svoronos AA, Bahal R, Pereira MC, Barrera FN, Deacon JC, Bosenberg M, et al. Tumor-targeted, cytoplasmic delivery of large, polar molecules using a pH-low insertion peptide. Mol Pharmaceut. (2020) 17:461–71. doi: 10.1021/acs.molpharmaceut.9b00883
53. Joffre OP, Segura E, Savina A, Amigorena S. Cross-presentation by dendritic cells. Nat Rev Immunol. (2012) 12:557–69. doi: 10.1038/nri3254
54. Zhang Z, Rohweder PJ, Ongpipattanakul C, Basu K, Bohn MF, Dugan EJ, et al. A covalent inhibitor of K-Ras(G12C) induces MHC class I presentation of haptenated peptide neoepitopes targetable by immunotherapy. Cancer Cell. (2022) 40:1060–1069 e1067. doi: 10.1016/j.ccell.2022.07.005
55. Zhang AQ, Hostetler A, Chen LE, Mukkamala V, Abraham W, Padilla LT, et al. Universal redirection of CAR T cells against solid tumors via membrane-inserted ligands for the CAR. Nat BioMed Eng. (2023) 7:113–1128. doi: 10.1038/s41551-023-01048-8
56. Dersh D, Holly J, Yewdell JW. A few good peptides: MHC class I-based cancer immunosurveillance and immunoevasion. Nat Rev Immunol. (2021) 21:116–28. doi: 10.1038/s41577-020-0390-6
57. Zhao B, Hou S, Ricciardi RP. Chromatin repression by COUP-TFII and HDAC dominates activation by NF-kappaB in regulating major histocompatibility complex class I transcription in adenovirus tumorigenic cells. Virology. (2003) 306:68–76. doi: 10.1016/S0042-6822(02)00079-X
58. Sade-Feldman M, Jiao YJ, Chen JH, Rooney MS, Barzily-Rokni M, Eliane JP, et al. Resistance to checkpoint blockade therapy through inactivation of antigen presentation. Nat Commun. (2017) 8:1136. doi: 10.1038/s41467-017-01062-w
59. Tannock IF, Rotin D. Acid pH in tumors and its potential for therapeutic exploitation. Cancer Res. (1989) 49:4373–84.
60. Lardner A. The effects of extracellular pH on immune function. J Leukoc Biol. (2001) 69:522–30. doi: 10.1189/jlb.69.4.522
61. Calcinotto A, Filipazzi P, Grioni M, Iero M, De Milito A, Ricupito A, et al. Modulation of microenvironment acidity reverses anergy in human and murine tumor-infiltrating T lymphocytes. Cancer Res. (2012) 72:2746–56. doi: 10.1158/0008-5472.CAN-11-1272
Keywords: acidosis, cancer, immunotherapy, MHC, surface target
Citation: Kelly JJ, Ankrom ET, Newkirk SE, Thévenin D and Pires MM (2024) Targeted acidosis mediated delivery of antigenic MHC-binding peptides. Front. Immunol. 15:1337973. doi: 10.3389/fimmu.2024.1337973
Received: 14 November 2023; Accepted: 27 March 2024;
Published: 11 April 2024.
Edited by:
Remy Bosselut, National Cancer Institute (NIH), United StatesReviewed by:
Lance Hellman, Nevada State College, United StatesSophia Sarafova, Davidson College, United States
Copyright © 2024 Kelly, Ankrom, Newkirk, Thévenin and Pires. This is an open-access article distributed under the terms of the Creative Commons Attribution License (CC BY). The use, distribution or reproduction in other forums is permitted, provided the original author(s) and the copyright owner(s) are credited and that the original publication in this journal is cited, in accordance with accepted academic practice. No use, distribution or reproduction is permitted which does not comply with these terms.
*Correspondence: Marcos M. Pires, bXBpcmVzQHZpcmdpbmlhLmVkdQ==