- 1The Frame-Corr Laboratory, Department of Clinical Research and Leadership, The George Washington University School of Medicine and Health Sciences, Washington, DC, United States
- 2Department of Biotechnology, Harrisburg University of Science and Technology, Harrisburg, PA, United States
- 3Undergraduate College, Princeton University, Princeton, NJ, United States
Importance: Research is beginning to elucidate the sophisticated mechanisms underlying the microbiota-gut-brain-immune interface, moving from primarily animal models to human studies. Findings support the dynamic relationships between the gut microbiota as an ecosystem (microbiome) within an ecosystem (host) and its intersection with the host immune and nervous systems. Adding this to the effects on epigenetic regulation of gene expression further complicates and strengthens the response. At the heart is inflammation, which manifests in a variety of pathologies including neurodegenerative diseases such as Alzheimer’s disease, Parkinson’s disease, and Multiple Sclerosis (MS).
Observations: Generally, the research to date is limited and has focused on bacteria, likely due to the simplicity and cost-effectiveness of 16s rRNA sequencing, despite its lower resolution and inability to determine functional ability/alterations. However, this omits all other microbiota including fungi, viruses, and phages, which are emerging as key members of the human microbiome. Much of the research has been done in pre-clinical models and/or in small human studies in more developed parts of the world. The relationships observed are promising but cannot be considered reliable or generalizable at this time. Specifically, causal relationships cannot be determined currently. More research has been done in Alzheimer’s disease, followed by Parkinson’s disease, and then little in MS. The data for MS is encouraging despite this.
Conclusions and relevance: While the research is still nascent, the microbiota-gut-brain-immune interface may be a missing link, which has hampered our progress on understanding, let alone preventing, managing, or putting into remission neurodegenerative diseases. Relationships must first be established in humans, as animal models have been shown to poorly translate to complex human physiology and environments, especially when investigating the human gut microbiome and its relationships where animal models are often overly simplistic. Only then can robust research be conducted in humans and using mechanistic model systems.
1 Introduction
While bacteria are the most commonly studied member, the gut microbiome consists of trillions of microbes including fungi, archaea, viruses, phages, and bacteria, which develop early in life and are influenced by genetic and environmental factors, including those that affect brain health (1). We cannot define what a ‘healthy’ gut microbiome is at present; however, low diversity is a common marker of an ‘unhealthy’ gut microbiome, which is often termed ‘dysbiosis.’ Dysbiosis is associated with many disease states, especially those becoming increasingly common in Western societies, likely due to limited exposure to diverse microbiota and inflammatory environmental exposures such as diet (2–4). This includes neuroinflammatory and neurodegenerative diseases, as dysbiosis contributes to gut and brain hyperpermeability, commonly termed ‘leaky gut’ and ‘leaky brain,’ by way of reduced tight junction proteins such as occludins (5). Microbial metabolites produced during dysbiosis are able to induce barrier dysfunction in preclinical models, leading to the passage of abnormal substances across barriers (6). The barrier function of the gut and the brain are an important part of innate immunity, without which the immune system cannot function properly, resulting in chronic inflammation locally and, perhaps eventually, systemically. This is a hallmark of dysregulation of the microbiota-gut-brain-immune interface. Moreover, dysbiosis can occur in any tissue containing a microbiome, including the oral and nasal cavities, lungs, skin, bladder, and vagina (7). While less studied than the gut microbiome, new evidence suggests the resident microbiota in these tissues can also contribute to immunoregulation and therefore a broad spectrum of disease states (7–9). Clues to the importance of microbiomes outside of the gut suggest some involvement in neuropsychiatric and neurodegenerative diseases, such as the presence of oral bacteria in the postmortem brains of persons with Alzheimer’s disease (7). Notably, the extent to which these localized microbiota contribute to disease is in an early stage of exploration; this paper will therefore focus on the more widely-studied gut microbiome. Accordingly, this narrative review will assess the state of the science in the emerging literature behind the microbiota-gut-brain-immune interface and the pathogenesis of neuroinflammatory diseases.
2 The microbiota-gut-brain-immune interface and neuroinflammation
Multidisciplinary research is emerging around the microbiota-gut-brain-immune interface, moving from animal models to human studies. This research is finding that the gut microbiota mediate the relationship between the enteric nervous system (ENS), autonomic nervous system (ANS), central nervous system (CNS) largely through regulation of the immune response and inflammation. The idea that brain function is tied to the gut microbiome and involves epigenetic and immunoregulatory changes is becoming common place in the clinic as well as in research. Further, nervous system epigenetic changes mediated by the gut microbiota show great promise to elucidate the pathogenesis of and novel therapeutics for neurological disorders (10). What is more, intimate and sophisticated relationships between diet, the gut microbiome, and cognition are emerging. Indeed, transdisciplinary perspectives intersecting neuroscience, psychology, and philosophy are further exploring the role of the gut microbiome in perception and cognition, and posit the idea that the microbiome possesses its own proto-cognition independent of, yet interrelated to the rest of the body (11). Interestingly, the hormones ghrelin and leptin (involved in hunger and satiety, respectively) have also been tied to cognition (12). Our microbiome, therefore, may not only affect the quality of our cognition but also how we perceive our internal and external worlds.
While external factors are important contributors to well-being and neuroinflammatory disease by way of epigenetic changes, internal factors (e.g. psyche, lifestyle, age, chronic inflammation, microbiomes) are at least equally important and interact with each other to potentiate a signal, perhaps synergistically. Changes to the epigenetics of the nervous system are typically acquired since neurons do not divide (13); the microbiota and their metabolites influence neurons (14–16). Microbiota-gut-brain-immune interface dysregulation has been associated with neuropathologies commonly linked with inflammation including mild cognitive impairment (MCI), Alzheimer’s disease, Parkinson’s disease, and multiple sclerosis and gastrointestinal (GI) symptoms are common in these disorders or even predate their onset (5, 17–23). For instance, GI symptoms predate the onset of Parkinson’s disease; this and a growing body of research support the theory that Parkinson’s starts in the gut and dysregulates the microbiota-gut-brain-immune interface, resulting in CNS and movement-related symptoms (21). GI symptoms may include nausea, constipation, dysphagia, abnormal salivation and defecatory dysfunction. Further, there is likely a bidirectional relationship, e.g. neuropsychiatric comorbidities are prevalent in inflammatory bowel disease (24). Thus, the microbiota-gut-brain-immune interface is a vital mediator of neuroinflammation likely to affect many facets of brain health including neurodevelopment, cognition, and behavior (20, 23). The most vulnerable aspects of the microbiota-gut-brain-immune interface to these effects involve multi-way physiological communication along the microbiota-gut-brain axis. Direct communication in the microbiota-gut-brain axis occurs predominantly via the vagus nerve while indirect signaling is diverse and complex including the ENS, ANS, CNS, immune system (e.g. glial activation), neuroendocrine system, tryptophan metabolism, and microbial metabolites (e.g. short-chain fatty acids, SCFAs) (18). Additionally, the gut microbiota are crucial to nutrient harvesting and produce some nutrients themselves that are co-factors for epigenetic pathways (25).
The spleen serves a crucial role in facilitating communication within the microbiota-gut-brain-immune interface by acting as a reservoir for various immune factors. Although the precise involvement of the spleen in the gut-brain axis is not completely understood, research indicates a correlation between antibiotic treatment and splenic function. Studies on mice subjected to antibiotic treatment have demonstrated a significant decrease in spleen weight, NK cells, macrophages, and neutrophils compared to control groups. Conversely, there is an observable increase in the percentage of CD8+ T cells within the spleen (26). Moreover, a proposed gut-spleen axis has been identified in patients with asplenia and common variable immune deficiency, wherein the reduction of IgM memory B cells induced by splenectomy may affect secretory IgA production in the gut. Numerous diseases, ranging from traumatic brain injury to conditions like Crohn’s disease, inflammatory bowel disease, septic shock, Alzheimer’s disease, Parkinson’s disease, schizophrenia, and depression, have been linked to the gut–brain–spleen axis. It is suggested that the vagus nerve reflex and systemic circulation serve as potential regulatory routes for these diseases (27).
In the following sections, we discuss the major elements of the microbiome-gut-brain-immune interface. An overview of this relationship can be seen in Figure 1.
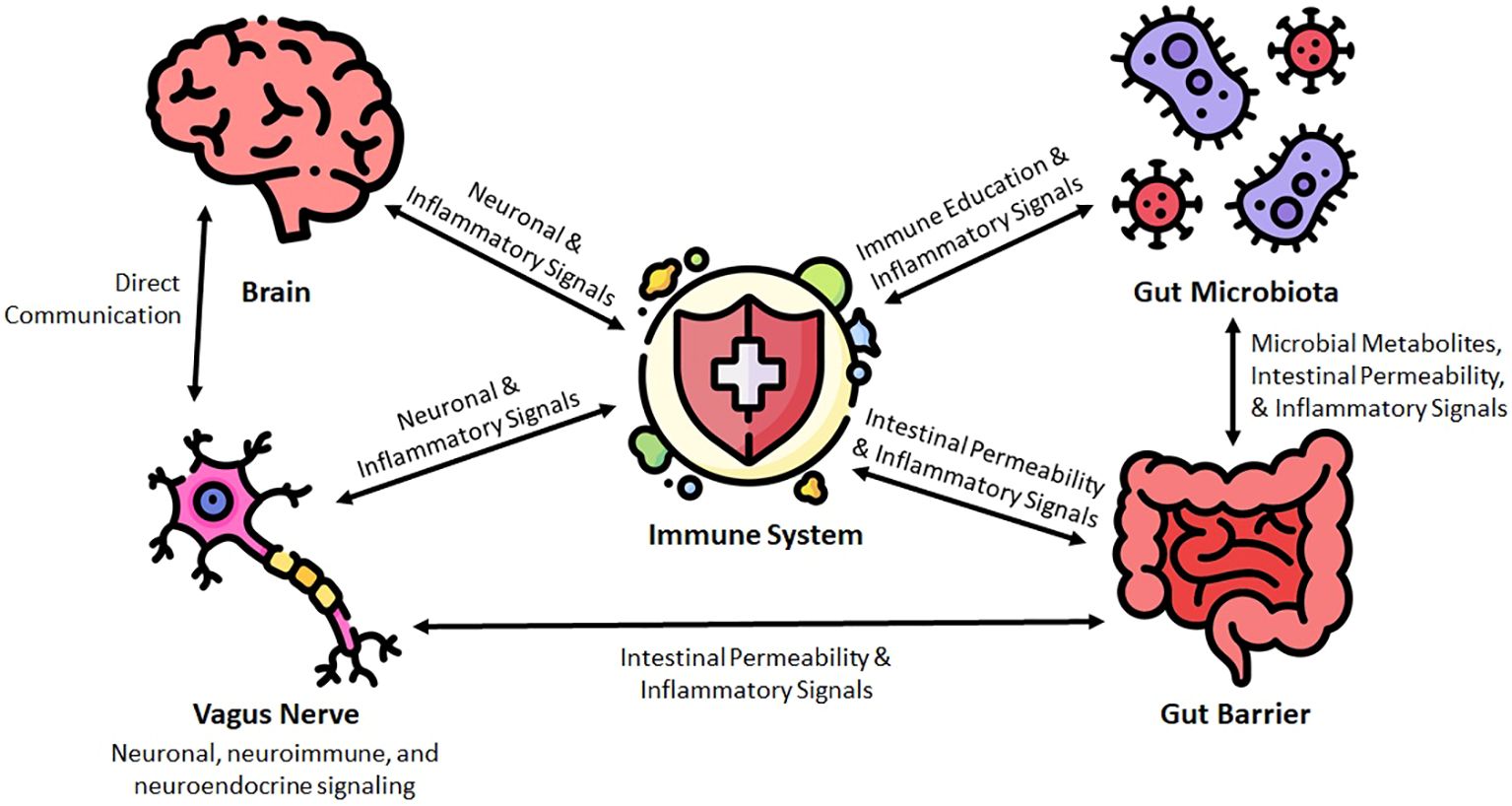
Figure 1 Conceptual framework for the microbiota-gut-brain-immune interface in the pathogenesis of neuroinflammatory diseases.
2.1 Microbiome – gut – brain communication
2.1.1 The vagus nerve
Also known as the tenth cranial nerve or ‘the great wanderer,’ the vagus nerve spans the vast majority of the human body and is the key nerve for interoception (internal sensing), and communication between the body and brain to maintain homeostasis or react accordingly (28–30). This communication is bidirectional and involves neuronal, neuroimmune, and neuroendocrine signaling and reaches beyond the parenchyma to include muscle, mucosa, ENS neurons, and the gut microbiome (the gut microbiota and their metabolites, typically measured with metagenomics and metabolomics) (5, 18, 19, 30–32).
2.1.2 Additional vagal connections
The vagus nerve innervates the gut mucosa including the gut associated lymphoid tissue (GALT). Included in the GALT, the lamina propria are part of the immune system and play a crucial role in immune education, which is why numerous immune cells reside here (20). The proximity of the lamina propria to the vagus nerve, (nascent) immune cells, and the gut microbiome make it central to the microbiota-gut-brain-immune interface. Here, the gut microbiota can influence vagal signaling and/or the immune system and therefore affect brain health (30). For instance, bacterial taxa (i.e. Campylobacter jejuni, Lacticaseibacillus rhamnosus JB-1 a.k.a. Lactobacillus rhamnosus/reuteri JB-1, Limosilactobacillus reuteri a.k.a. Lactobacillus reuteri) have been shown to affect brain, cognition, and behavior via vagal signaling, resulting in positive and negative outcomes (28, 33). Another demonstration of the interplay between the vagus nerve, the brain and the gut microbiota is found in preclinical data obtained from rodent models. Performing subdiaphragmatic vagotomy on rodents treated with cuprizone blocked demylination in the brain and restored the gut microbiota dysbiosis induced by cuprizone (34). This supports the concept that the vagus nerve plays a critical role in the microbiota-gut-brain axis.
Further, the vagus nerve regulates peripheral inflammation and intestinal permeability via the ENS cholinergic anti-inflammatory pathways, and, therefore, plays a key role in the prevention or pathogenesis of so-called ‘leaky gut’ (31). In times of stress or disease, vagal signaling is inhibited (low vagal tone), which hampers the microbiota-gut-brain-immune interface and can results in negative outcomes in the microbiome, gut, brain, and immune system (31). Extra-vagal signaling in the microbiota-gut-brain-immune interface involves microbial metabolites like SCFAs, secondary bile acids, and tryptophan metabolites including the neurotransmitter serotonin a.k.a. 5-hydroxytryptamine (5-HT) and the gut hormones cholecystokinin (CCK), glucagon-like peptide-1 (GLP-1), and peptide YY (PYY), which are propagated via enteroendocrine cells (EECs) (31, 35). However, stress and disease also affect the gut microbiota and their metabolites; therefore, altering extra-vagal signaling as well.
2.1.3 Secondary bile acids
Bile acids are synthesized from cholesterol. Primary bile acids like chenodeoxycholic acid (CDCA) and cholic acid (CA) are produced first (36). After production in the liver, primary bile acids are transported by way of the small intestine to the colon where they are metabolized by the gut microbiota into the secondary bile acids such as lithocholic acid (LCA) and deoxycholic acid (DCA) (36). These secondary bile acids are then transported across the gut barrier where they may travel to the liver or circulate systemically, likely also crossing the blood-brain barrier (36). While the gut microbiota determines the production of secondary bile acids, secondary bile acids also seem to alter the composition of the gut microbiome, indicating a bidirectional relationship (35, 37). In the brain, no less than 20 bile acids have been found, where they likely alter neurological function and promote disease (36, 37). In a healthy, normal state, bile acids appear to be neuroprotective in the brain; however, in a dysbiotic and/or diseased state, this metabolism and regulation is perturbed, degrading or eliminating the neuroprotective effect (36, 37). Bile acids interact with receptors like the farnesoid X receptor (FXR) and G-protein-coupled bile acid receptor 1 (GPBAR1), which are present in many different immune cells, thereby influencing neuroinflammation (38). Secondary bile acids can also exert an indirect influence on neurological function; for example, LCA and DCA can modulate serotonin production by interacting with the enterochromaffin cells (ECCs) of the gut, thus impacting gut-brain axis signaling (39). The dysregulation of secondary bile acids has been correlated with neurodegenerative disorders such as Alzheimer’s disease, Parkinson’s disease, Amyotrophic lateral sclerosis, and Multiple Sclerosis (40, 41) Secondary bile acids are one of many elements of the microbiota-gut-brain-immune interface that appears to play a major role in the pathogenesis of neurodegenerative disease.
2.1.4 Short-chain fatty acids
Many of the bacterial members of the gut microbiome rely on microbiota-accessible carbohydrates (MACs)—fiber and resistant starch—for fuel, producing metabolites in the process. Of these metabolites the most well-known and, perhaps, beneficial class is the SCFAs, which are an energy source for colonocytes and, therefore, support gut barrier function, microbiome balance, and reduce neuroinflammation, likely to within ‘optimal’ ranges (23, 42). Further, SCFAs promote tolerance and homeostasis via regulatory T cells (Tregs) among many other effects on the immune system and are, thus, considered anti-inflammatory (43–45). Communication between the gut microbiota-gut-brain axis is also mediated by SCFAs via receptors on ECCs, a type of EEC that 1) is involved in serotonin production and 2) directly interacts with the vagus nerve (20, 35).
As with most elements of biochemistry and nutrition, there is an optimal range of SCFAs, both above and below which negative health outcomes are seen. In dysbiotic and/or diseased states, SCFAs are produced in too much (e.g. irritable bowel syndrome) or too little (e.g. low MACs diet) quantities. Some of the SCFAs are transported across the gut barrier and into circulation, where they appear to cross the blood-brain barrier, affecting CNS function (46). SCFAs such as butyrate have been shown to promote gene expression via epigenetic regulation (i.e. enhanced chromatin accessibility), which may aid in memory consolidation (46). However, not all SCFAs are created equal. For example, butyrate is particularly neuroprotective via Treg induction while acetate may exacerbate neurodegeneration (23).
2.1.5 Neurotransmitters
Transmitting electrochemical signals between neurons and to effector sites, neurotransmitters can act as hormones, promoting function and health in peripheral tissues including the brain (47, 48). Many neurotransmitters are also produced by gut microbiota including γ-aminobutyric acid (GABA), norepinephrine, epinephrine, dopamine, and acetylcholine (20, 49, 50). While these microbial neurotransmitters clearly play a role in the gut (see Tables 1, 2), in proximal regions, and likely in circulation, it is unclear if all or some of these interact with the CNS in sufficient concentrations to have a meaningful effect; however, they can exert an effect via the ENS including the vagus nerve (51, 52). Further, SCFAs may add to this effect on vagal signaling, as SCFAs play a key role in neurotransmitter metabolism; for example, SCFAs modulate the production of tryptophan by ECCs. Tryptophan is a required pre-cursor to serotonin (20).
Serotonin has been extensively studied for its role in gastrointestinal and brain health and in gut-brain communication (the gut-brain axis) (51). The majority of serotonin (~90%) is stored in ECCs in the gut, produced from tryptophan (47, 53, 54). Tryptophan to serotonin metabolism involves the kynurenine pathway and, therefore, leads to production of quinolic acid (neurotoxic) and kynurenic acid (neuroprotective)—the balance of which is regulated by the gut microbiota and may contribute to neuroinflammation and ultimately neurodegeneration (55). While neurotransmitter-producing gut microbiota are still in the early stages of elucidation, there are a few bacterial taxa of note (see Tables 1, 2).
Throughout the body, the microbiota-gut-brain axis and subsequently the microbiota-gut-brain-immune interface has a profound impact via direct and indirect pathways and is influenced by host genetics, lifestyle, environmental exposures, etc. As many of these are modifiable risk factors, this is an important line of research to support mental and physical health (well-being) and may prove to be crucial for prevention, management, and treatment of neuroinflammatory and neurodegenerative disorders, for which we currently have very few tools at our disposal.
3 Neuroinflammation
Physical and/or psychological stress can also cause inflammation, including chronic neuroinflammation, through dysregulation of the hypothalamic-pituitary-adrenal axis (HPA axis). Inflammation can originate anywhere in the body, typically via inflammatory cascades that include cytokines, chemokines, reactive oxygen species (ROS), and trafficking of immune cells (e.g. T and B cells). Neuroinflammation also involves specialized members of the immune system called glia (microglia and astrocytes) resident in the CNS. A robust, acute immune response is a necessary response to injury or invasion; it is equally important for this inflammation to resolve in a timely manner, avoiding chronic inflammation. This is true of neuroinflammation as well. While acute neuroinflammation is protective, chronic neuroinflammation increases risk of neurodegenerative disorders, the most common of which are Alzheimer’s disease, Parkinson’s disease, prion disease, amyotrophic lateral sclerosis, motor neuron disease, Huntington’s disease, spinal muscular atrophy, and spinocerebellar ataxia (56). Further, local or systemic inflammation can sensitize the immune system, leading to exacerbation of inflammation, including neuroinflammation.
Gut microbiota influence brain function by way of maintenance of homeostasis in innate and adaptive immunity, limiting acute and chronic inflammation in the gut and CNS and, therefore, risk of neurodegenerative disorders even independent of other pathogenesis features like amyloid plaques (23, 55, 57). Neuroinflammation is a key element in the pathogenesis, prevention, and treatment of neurodegenerative disorders; once at a critical point, the epigenetic profile changes dramatically (13). Epigenetic changes due to neuroinflammation may include substantial changes in DNA methylation, histone methylation and acetylation, and non-coding RNA expression (13). Further, the relationship between neuroinflammation and epigenetic changes has long suspected to be bidirectional, as neuroinflammation is also strongly influenced by epigenetic mechanisms. For instance, DNA methylation may be a regulator of activated microglia that drive AD pathology, and presence of neuroinflammatory conditions (e.g. psychiatric disorders) demonstrate altered patterns of DNA methylation (58). The origin of neuroinflammation is often outside of the CNS, commonly certain bacterial taxa in the gut microbiome. For example, Heliobacter pylori, which is present in about half of human gut microbiomes, leads to DNA methyltransferase inhibition, destabilizing the genome in a manner typical of certain disease states (46).
It is important to note the possibility that dysbiosis resulting in altered protein express may also contribute to neuropathology, independent of the inflammatory response. In a mouse model, fecal microbiota transplant (FMT) from aged donors to young adult mice resulted in impaired spatial memory in conjunction with altered protein expression associated with hippocampal synaptic plasticity and neurotransmission with concomitant reduction in SCFA-producing bacteria; yet, gut permeability and cytokines were not affected (59). However, the authors note that cytokines were assessed at the end of the intervention and were unaware if cytokines fluctuated in the early-stage post-FMT.
The role of vascular endothelial growth factor (VEGF) may also serve an underrecognized role in the multifactorial pathophysiology related to alteration of the gut microbiome, protein expression, and inflammation. Of note, in AD mouse models in which alterations in RNA/protein expression and microglia occur with elevated amyloid-beta-peptides in the ENS of the small intestine, some evidence suggests that VEGF mediates neuroprotective and neurodegenerative effects in both the CNS and PNS (60).
3.1 Glia: astrocytes and microglia
These immune cells reside in the nervous system; in the CNS glia are involved in the production, potentiation, and resolution of neuroinflammation. Also known as glial cells or neuroglia, glia support neuronal functions, e.g. synapse formation, neuronal plasticity, neurotransmission, injury response, and protection from neurodegenerative disorders. Glia release cytokines and chemokines that are potential mediators of neurotoxicity (Table 3). The most plentiful glia are astrocytes, which are “master regulators of synapse formation, ion homeostasis, and neurovascular coupling” (13).
In response to changes in their environment, in addition to producing cytokines and chemokines, microglia express antigenic markers, regulate neurotransmitters, and undergo morphological changes (61). Once activated, microglia can cause neuronal damage by producing reactive oxygen species and nitric oxide—both neurotoxic—and cross-reacting with astrocytes to magnify the effect, resulting in loss of neurotrophic functions. Microglia have been proposed to promote neuroinflammation and neurotoxicity; however, recent research suggests that their impact can be context-dependent, contingent upon their polarization phenotype, activation status, and cellular context (62). These effects are modulated through neuron-microglia communication facilitated by various neurotransmitter receptors expressed on microglia. Notably, receptors for neurotransmitters such as glutamate, GABA, norepinephrine, cannabinoid, and acetylcholine play significant roles in mediating these interactions. Microglia may modulate neurotransmitter release, thus, coordinating either positive or negative feedback loops tailored to the needs of the organism. Moreover, these interactions may extend to indirect effects on neighboring microglia, further expressing the role of neuron-microglia communication.
3.2 Chronic neuroinflammation
The relationship between the microbiota-gut-brain-immune interface, microbial metabolites, and glia is a potent regulator of GI and actionable target in neuroinflammatory and neurodegenerative disorders (12). In chronic neuroinflammation, pro-inflammatory cytokines are habitually upregulated and glia are overactive, resulting in damage to neurons, synapse function, cortical tissue, and functional connectivity—commonly observed in neurological disorders (57, 63). The multidirectional relationship of the microbiota-gut-brain-immune interface governs this chronic neuroinflammation, which may start at any point, including moving from the gut into the CNS, which often results in systemic inflammation. Systemic inflammation is a core feature of chronic neuroinflammation. Neuroinflammatory diseases arecharacterized by elevated C-reactive protein (CRP), TNF-α, and IL-1β (23). The overactivation of glia that drives pathogenesis is affected by the gut microbiome and implicated in neuroinflammation and subsequent neurological disorders (e.g. Alzheimer’s and Parkinson’s), as well as gut microbiome composition (dysbiosis) and intestinal inflammation and permeability (‘leaky gut’) (18, 23, 64). Neurodegenerative disorders are likely multifactorial in cause, and a key element of this is the gut microbiota due to their production of neuroactive metabolites (5). In specific, gut microbiota that produce the endotoxin lipopolysaccharides (LPS) may contribute to amyloid deposition and neuroinflammation in Alzheimer’s (65). LPS interacts with the microbiota-gut-brain-immune interface via Toll-like receptor (TLR) 4 and the NF-κB pathway, stimulating an inflammatory cascade, triggering leaky gut, and leading to neuroinflammation (18, 23, 64–66).
Interleukins (ILs) are a category of cytokines: some of which have pro-inflammatory/immune reaction stimulating effects while others have anti-inflammatory/homestasis stimulating effects. Therefore, the change in the concentrations of these cytokines (the cytokine milleau) can have great effects on neuroinflammation. ILs are a promising target for treating neuroinflammation and subsequent neurodegeneration (67). Many of the Preventative and Therapeutic Interventions discussed below alter the cytokine milleau, meaning this is at least in part their method of action.
TLRs are pattern recognition receptors (PRRs) that recognize molecules and patterns of molecular structure, pathogen-associated molecular patterns (PAMPs), from bacteria that are extracellular or have been engulfed into vesicular pathways via phagocytosis. They signal through cytokines such as ILs. TLRs could be used as targets to quench neuroinflammation, according to research from preclinical to clinical trials (68). Futher, small phytocompounds such as curcumin have been shown to target TLRs (68). The dose and other details for such an intervention have yet to be elucidated and show the possiblity of a hormetic response, meaning higher doses are actually detrimental.
No matter the cause(s), dysbiosis fosters a damaging, inflammatory environment via the microbiota-gut-brain-immune interface via LPS and other stimulators of the inflammatory cascade, i.e. pro-inflammatory cytokines and chemokines, T-helper cells, and monocytes (5). Further, dysbiosis contributes to aberrant HPA axis activation that can result in cortisol dysregulation, which exacerbates leaky gut (5). Therefore, dysregulation of the microbiota-gut-brain-immune interface can create a circular inflammatory feedback loop between dysbiosis, leaky gut, and chronic systemic and CNS inflammation.
4 Microbial alterations in neuropathology
The emerging patterns of gut microbiome changes specific to neurological disorders may aid in the development of treatment options for these recalcitrant disorders (described in subsequent sections). This data is largely correlational and focused solely on the composition of the gut microbiome, meaning it is not yet ready for the clinic. Further, some of this compositional work has been done at fairly high order such as phyla/phylum, which is extremely non-specific. While other work is done at the genus level, this is still fairly non-specific with a great deal of diversity of function within a single genus. Keeping this in mind, one must take the research on the composition of the microbiome, especially that at the phyla or genus level, with more than a grain of salt. Functional data on the gut microbiome are beginning to emerge and will grow substantially in the years to come as the cost of advanced technologies such as shotgun metagenomics continue to decline and are adopted more widely. This coupled with more robust study designs including longitudinal studies may lead to groundbreaking therapies and even means of prevention.
Currently, it appears that there are common mechanisms among neurodegenerative disorders like Alzheimer’s disease, Parkinson’s disease, multiple sclerosis, and stroke/traumatic brain injury. These include a dysbiotic gut microbiome, insufficient SCFA production, elevated LPS, and leaky gut that stimulate pro-inflammatory immune, neuroendocrine, and neuroinflammatory pathways. It is likely that the differences between these disorders is due to variation in the microbial alterations of the affected gut microbiomes. This may or may not also be related to baseline microbiome composition and function prior to the onset of neuroinflammation, which are largely determined by environmental exposures including diet and lifestyle in addition to seeding of the gut microbiome during crucial developmental phases as well as host genetics (e.g. propensity for chronic inflammation) (25, 69). These relationships are summarized in Supplementary Table S1.
4.1 Alzheimer’s disease
The hallmarks of Alzheimer’s disease are amyloid-beta and hyperphosphorylated tau protein accumulation with neuronal degeneration, which is thought to develop decades prior to symptoms. The microbiota-gut-brain-immune interface has been implicated in its pathogenesis with human studies finding dysbiosis in the gut microbiomes of those with Alzheimer’s (69–71). For example, the gut microbiomes of those with Alzheimer’s compared to healthy controls has decreased Bacillota (formerly Firmicutes) and Bifidobacterium and increased Bacteroidota (formerly Bacteroidetes), and Escherichia and Shigella (two inflammatory genus) as well as lower abundance of the species Eubacterium rectale (E. rectale is believed to be anti-inflammatory), all of which correlates with increased pro-inflammatory cytokines in Alzheimer’s (72, 73). However, these compositional changes have not been confirmed in cohorts in other countries, such as China, indicating a role for the environment and/or the need for higher resolution data, likely at the species or strain level (74, 75). There is a need for further research, especially that better control for potential confounders, to be able to use this as a screening tool for research or clinically.
4.2 Parkinson’s disease
In Parkinson’s disease, misfolded α-synuclein accumulates in the neuronal cell body with motor impairments at least in part due to progressive dopaminergic neuron damage, resulting in decreased dopamine (5, 76). Of note, digestive symptoms are common in Parkinson’s and typically develop prior to hallmarks of the disease. This points to a key role of the microbiota-gut-brain-immune interface and lends some support for a causative role via temporality à la the Bradford Hill criteria (5, 21, 77). While it is not yet clear that α-synuclein causes neuronal loss (it may be an intermediate step or a symptom), the vagus nerve may transmit pathology to the brainstem, resulting in deposition of α-synuclein (78, 79). In fact, correlations have been established between α-synuclein and composition of the gut microbiome, which have been supported by a recent meta-analysis (76). Specifically, compared to healthy controls, the gut microbiomes of those with Parkinson’s have: depletion of Prevotellaceace that are involved in SCFA production, which leads to leaky gut and endotoxin exposure; depletion of important SCFA producers belonging to the Lachnospiraceae family and of which key players include Blautia, Roseburia, and L-Ruminococcus; increased Enterobacteriaceae, which can raise LPS and eventually lead to neuroinflammation; and enrichment of Lactobacillus, Akkermansia, and Bifidobacterium (5, 76). However, it is unclear if these changes are the cause or consequence of disease at present.
4.3 Multiple sclerosis
Gut dysbiosis appears to promote the pathogenesis of Multiple Sclerosis (MS) via the microbiota-gut-brain-immune interface: leaky gut, leading to immune activation, leading to systemic inflammation, leading to disruption of the blood brain barrier, leading to neuroinflammation, leading to neurodegeneration (52, 80). The impetus for this cascade and the leaky gut that drives it is currently unknow but dysbiosis has been found in MS patients and is mechanistically plausible (52, 80–83). While it is possible that this may actually be a consequence of the disease process rather than its cause, the research to date does not clearly support this concept. Further, the types of MS seem to have their own distinct versions of dysbiosis (52, 80–83). When compared to healthy controls, the gut microbiomes of those with MS have fewer SCFA-producing bacteria, Butyricimonas, Faecalibacterium, Clostridium cluster IV and XIVa, Faecalibacterium prausnitzii, and Blautia species and more Akkermansia muciniphila, Ruthenibacterium lactatiformans, Hungatella hathewayi, Eisenbergiella tayi, and Clostridium perfringens (82–84).
4.4 Overlap in neurodegenerative disorders
In a 2022 systematic review involving 52 studies and 5,496 participants with Alzheimer’s disease, Parkinson’s disease, MS, amyotrophic lateral sclerosis, and stroke, the strongest overlap was seen between Parkinson’s disease and MS with 8 shared genera (85). Interestingly, Parkinson’s also shared 6 genera with stroke. While there was overlap between Alzheimer’s and Parkinson’s the sample size is small, making this an unreliable association at present. Among these CNS disorders, Akkermansia, Faecalibacterium, and Prevotella were most commonly indicated. Again, this work is done at high order (low resolution) and is still mostly correlational and, therefore, may not be causative. However, these overlapping trends may better inform researchers and clinicians about preventative and interventional measures that could be more broadly applicable to neuroinflammatory disorders. Thus, this may represent a research priority for funding agencies. Key relationships for all of these disorders are highlighted in Supplementary Table S1 Observed Functional Relationships with the Gut Microbiota and Neurodegenerative Disorders; of note, only bacterial taxa have been characterized sufficiently at present.
4.5 Preventative and therapeutic interventions
Much of the excitement around the research on the microbiota-gut-brain-immune interface, especially around altering the gut microbiome, is the possibility of preventative and therapeutic interventions. This is especially poignant in neurodegenerative disorders, where there is little to offer in terms of such interventions. Most of the research has been in animal models with limited human studies, however. Translating these findings into the clinic requires further investigation in general and in how best to personalize such interventions to maximize their impact for an individual.
4.6 Nutrition for neuroinflammation
Nutrition is an important, modifiable risk factor that has a major role in the microbiota-gut-brain-immune interface, affecting each aspect of the interface. Gene-diet interactions have been linked to the microbial theory of inflammation, neuroinflammation, and neurodegenerative disorders like dementia (86, 87). Diet induced changes to the gut microbiome are key in the microbiota-gut-brain-immune interface. Gut microbiome changes are associated with shifts in the production of SCFAs and secondary bile acids, which in turn can impact inflammation and the release of neurotransmitters like serotonin (35). Therefore, resolving inflammation, dysbiosis, and leaky gut are likely to prevent and/or manage neurodegenerative disorders. The quantity of MACs present in the diet is linked to the production of SCFAs (88). A diet with adequate calories, rich in MACs promotes health in the microbiota-gut-brain-immune interface, while a high calorie, low MACs diet is associated with cognitive decline (25, 89, 90). SCFAs clearly play a role in this, especially in light of their role as fuel for colonocytes, preventing leaky gut and the inflammatory cascade (25, 89, 90). Polyphenols may also play a key role and have been shown continually and repeatably to be health-promoting elements of a healthy diet (25, 91, 92). Thus, diets low in MACs, high in fat/protein (i.e. Western-style diets) are associated with gut dysbiosis and inflammation (25, 93). While some research on ketogenic diets, which are often low in MACs, has shown promise in reducing neuroinflammation and improving cognitive function in animal models of Alzheimer’s and Parkinson’s diseases, microbiome research poorly translates from animal models, which are overly simplistic. Therefore, the results in humans are often greatly attenuated or even lost due to a much more complex physiology, meaning they are no longer meaningful let alone clinically meaningful. This necessitates research in humans, which is currently lacking for ketogenic diets.
The nutrients and nutraceuticals often recommended for brain health mostly support the gut microbiome and the barrier function of the gut and blood brain barrier (94). However, any individual food is more than the sum of its parts—the concept of the ‘food matrix’ (95–98). Therefore, focusing on nutrients alone is insufficient to promote a healthy diet. Instead, an emphasis on the inclusion of whole, minimally processed foods and limiting ultra-processed foods is necessary and likely better able to support the microbiota-gut-brain-immune interface and prevent the inflammatory cascade and consequently neurodegeneration. Dietary patterns that embody this include the Mediterranean diet and the Mediterranean-DASH Diet for Neurodegenerative Delay (MIND) diet.
The Mediterranean diet is a style of dietary pattern that emphasizes vegetables, fruit, olive oil, and low-moderate alcohol intake (i.e. red wine) and is considered to be health promoting. It is also rich in polyphenols and omega-3 fatty acids, the latter of which is required for the resolution of the inflammatory response via resolvins and are associated with reduced neuroinflammation (99, 100). This dietary pattern has been linked to lower risk of neurodegenerative disorders and cognitive impairment and better global cognition and episodic memory (101). Many of the disorders that are seen to be decreased in those on this type of diet (coronary artery disease, hypertension, diabetes, metabolic syndrome, dyslipidemia) are also risk factors for cognitive impairment and involve the microbiota-gut-brain-immune interface (99).
The MIND diet is a version of the Mediterranean diet with an emphasis on neuroprotection and cardioprotection through anti-inflammatory foods that has been shown to slow cognitive decline with aging (99, 101, 102). It consists of high intake of whole plant foods emphasizing berries and green leafy vegetables, nuts, beans, fish, poultry, and olive oil while limiting animal foods, processed foods, and foods high in saturated fat. The focus on the food matrix likely plays a role in the MIND diet’s effect on the microbiota-gut-brain-immune interface, potentially making it a more comprehensive tool.
Adherence to both the Mediterranean and MIND diets are associated with decreases in all-cause dementia independent of genetic risk and numerous studies support reduction in the risk of Alzheimer’s disease specifically (19, 86, 103). Both the Mediterranean and MIND diets are associated with reduced pathology in Alzheimer’s (104). Evidence to date demonstrates support for the Mediterranean and MIND diets in the prevention of a multitude of disease states, and the MIND diet appears to impart the greatest neuroprotection. However, there are limitations to this research: small cohorts, lacking a gold-standard to measure dietary adherence, potential for reverse causality because of short durations/follow-up (86, 105). In an attempt to account for this, a recent population-based study by de Crom et al. found an association between both diets and reduced dementia risk; however, there is still potential for confounding from lifestyle (105).
4.6.1 Lifestyle modifications
Many modifiable risk factors fall under the category of lifestyle. Stress management, restorative sleep, and other lifestyle factors have been shown to affect epigenetic regulation, the microbiota-gut-brain-immune interface, and neurodegenerative disease risk (106–112). As discussed above, chronic stress can trigger gut dysbiosis and the inflammatory cascade, so it follows that stress management has been linked to improvements in the gut microbiome composition and in stress-related epigenetic regulation (93, 113, 114). Mind-body therapies, e.g. yoga and meditation, are multifaceted interventions with numerous health benefits including stress management. Such mind-body interventions are promising to promote a diverse, non-dysbiotic/eubiotic gut microbiome and to reduce chronic inflammation (115–118). Similarly, restorative sleep is negatively associated with gut dysbiosis and cognitive decline (119, 120).
4.6.2 Physical activity
Effecting both the gut microbiome and epigenetics, physical activity is a potentially powerful modifier of neurodegenerative disorder risk. Physical activity (natural movement and exercise) alters gut composition and function by promoting beneficial gut bacteria and SCFA production; it also supports resolution of inflammation and return to immune homeostasis (121–125). All of these effects are likely to reduce the risk of neurodegenerative disorders. Additionally, physical activity is generally neuroprotective: increasing cerebral blood flow and circulation-related benefits (i.e. oxygenation and nutrient delivery), the production of neurotrophic factors including brain-derived neurotrophic factor (BDNF), and neurotransmitter (e.g. dopamine and serotonin) release that improves mood, cognition, and well-being (126–128). BDNF promotes neuronal growth, survival, and synaptic plasticity, supporting learning and memory (127). This orchestrated interplay of neurotrophic, anti-inflammatory, and metabolic processes work hand in hand with the gut microbiome in conferring neuroprotection associated with physical activity. Epigenetic changes from physical activity also contribute to its neuroprotective role (106, 112, 128–130). Given that yoga is both exercise and a mind-body therapy, both processes are likely contributing to the beneficial effects of yoga on the microbiota-gut-brain-immune interface and therefore reduced risk of neurodegenerative disease.
4.6.3 Probiotics, prebiotics, and fermented foods
Probiotics are, by definition, live microorganisms that confer health benefits when administered in adequate amounts (131). In animal models of neurodegenerative disorders, supplementation with probiotics shows great promise for improving neuroinflammation, cognitive function, gut microbiome composition and function, epigenetic profiles, inflammation, and gut barrier function (132–139). A better understanding of the dysbiosis in neurodegenerative diseases as well as how certain taxa (e.g. keystone species) affect the microbiota-gut-brain-immune interface is necessary to rationally design probiotics that may be preventative or therapeutic for neurodegenerative disorders. At present, the majority of probiotic strains on the market are taken from yogurt, as they are easily granted generally recognized as safe (GRAS) status by the Food and Drug Administration (FDA). This greatly limits the possibility of their efficacy as therapeutics in an ecosystem that is much more diverse than yogurt.
Prebiotics are MACs that stimulate the growth and activity of microbes already present in the gut microbiome, thereby also altering the composition and function of the gut microbiome (140). Prebiotics have been shown to increase beneficial gut bacteria, reduce neuroinflammation, and support cognitive function (134, 137, 139). Given that prebiotics are likely to have a broader effect than the limited types of probiotics currently available, this may be a more promising avenue. However, a healthy diet emphasizing whole foods and limiting ultra-processed foods (e.g. the Mediterranean or MIND diets) can supply sufficient MACs to fuel the gut microbiota. Hence, the use of prebiotics over the emphasis of a healthy diet, which brings many other health-promoting elements, is currently under debate. In those unwilling or unable to adopt a diet rich in MACs, it is possible that prebiotics may support the gut microbiota sufficiently to avoid neurodegenerative disorders or it may be insufficient or missing other key elements from the diet and the food matrix.
The microbiota-gut-brain axis involves bidirectional communication through multiple pathways. These pathways, both direct and indirect, can facilitate epigenetic reprogramming within the microbiota-gut-brain axis, mediated by histone tail modification, DNA methylation, and non-coding RNA. Alterations in the composition of the microbiota can induce epigenetic changes that ultimately influence behavior; for example, Helicobacter pylori in the gut increases CpG-methylation in the promoter region of 06-methylguanine DNA methyltransferase, consequently reducing DNA methyltransferase activity in the gastric mucosa (141). Furthermore, studies have discovered a correlation between the gut microbiome and gene expression within the CNS, particularly in regions controlling the development of mood and neurological disorders; for instance, dietary supplementation of mice with Lactobacillus rhamnosus has been found to modulate the expression and transcription of GABA subunits across various brain regions (141). Interventions involving the supplementation of pre- or probiotics may ameliorate neurobehavioral abnormalities through epigenome alteration, often resulting in phenotypic attenuation.
Fermented foods were traditionally used to extend the storage of perishable food substrates; however, recent studies have highlighted their roles in the introduction of beneficial microbes and molecules to the gut microbiome. The connecting pathways of the microbiota-gut-brain axis have been used to understand the effects of fermented foods on the permeability of the intestinal and blood-brain barrier and their role in the treatment of neuroinflammation and mental health disorders. Various studies have demonstrated a decrease in circulating cytokines, especially IL-6, IL-10, IL-12, and TNF-α, among patients on fermented food diets (142). Consumption of fermented foods has also been shown to reduce corticosterone when exposed to stress; a proposed mechanism of cortisol modulation is via attenuating the response to peripheral immune challenges through a reduction in circulating cytokines and other inflammatory mediators (142). The administration of fermented products has also been shown to improve anxiety and depressive features and improved memory-associated tasks (142).
4.6.4 Other supplements
4.6.4.1 Gut barrier: glutamine and zinc carnosine
Supplements that support gut barrier function include glutamine (143–145) and zinc carnosine a.k.a. polaprezinc, which is important for wound and mucosa (e.g. the gut) healing and likely also beneficial for neuroprotection and reducing neurodegenerative disorder risk (146–149).
4.6.4.2 inflammation
4.6.4.2.1 Omega-3 fatty acids
As mentioned previously, omega-3 fatty acids help to resolve inflammation (anti-inflammatory); thus, supplemental omega-3 may be beneficial for the microbiota-gut-brain-immune interface. In fact, a reduced risk of Alzheimer’s disease and cognitive decline is associated with intake of omega-3, especially docosahexaenoic acid (DHA) (150). However, this effect has not been consistent perhaps due to issues with dose, formulation, rancidity, study design, etc. (151, 152). In Parkinson’s disease, several studies have demonstrated a reduction in dopaminergic neuron degeneration and neuroinflammation with greater intake of omega-3 (151).
4.6.4.2.2 Curcumin
A polyphenol found in turmeric, curcumin’s anti-inflammatory properties have been studied as a potential therapeutic in Alzheimer’s disease with the exploration of several modified formulations include nanotization to improve its bioavailability and pharmacokinetic properties, the major limitation to its therapeutic benefits (153–155). However, given the food matrix/entourage effect, one wonders if the extract is as potent as the whole food (turmeric) and/or if there are synergistic effect in food combinations. For instance, it is well known that black pepper improves the bioavailability and action of turmeric (156–158).
4.6.4.2.3 Resveratrol
Another polyphenol, resveratrol and its sources (i.e. red grapes and wine) have been linked to improved cognitive function and neuroinflammation and are being studies for Alzheimer’s disease and Parkinson’s disease (159–163). However, resveratrol research is still in its early stages and has hit some barriers, including concerns for the need for high doses. Again, the food matrix/entourage effect may be an important component to explore, potentially limiting the need for high dose therapy.
4.6.5 Fecal microbiota transplantation
Fecal Microbiota Transplantation (FMT) is the transfer of fecal matter from a healthy donor into the gut of a recipient after the administration of antibiotics to clear the way (164). While historically thought of as radical and reserved for the life-and-death struggle of recurrent Clostridioides difficile, FMTs are being developed by industry, including two that have been FDA-approved recently (164–167). These standardized FMTs open up the possibility of broader use including for neurodegenerative disorders (168–172). To understand the long-term safety and efficacy of FMT in neurodegenerative disorders and for neuroinflammation, more research is needed.
5 Generalizability of microbiome research findings
In an emerging field like this, some demographic and ethnic groups are underrepresented, limiting the generalizability of its findings (173). Why is this so important for the microbiota-gut-brain-immune interface and the pathogenesis of neurodegenerative disorders? In a 2015 study, gut microbiome compositions were shown to vary significantly by geographic location, meaning the composition of those in the US, are likely different than those in other countries and the findings from US research may not be translatable in other countries (174). This has since been confirmed by many other studies and researchers. The gut microbiome is similar to a finger print in that there is a huge amount of interindividual variability, meaning averages are often not representative as well. Therefore, small sample sizes, especially from groups with limited diversity, are only able to describe the population in the sample—they are not generalizable (175). Despite this, high-income countries (HICs) lead microbiome research output due to well-established research infrastructure and funding availability. Contrastingly, lower-middle-income countries (LMICs) are underrepresented in microbiome research (175). Access is limited to advanced sequencing technologies, funding, and expertise, all of which encumber research efforts in LMICs (173, 176). Further, the emphasis on infectious disease and more immediate concerns to public health likely redirect consideration and resources from more long-term research projects such as the microbiome (176).
In order to truly understand the gut microbiome and the microbiota-gut-brain-immune interface, we must study diverse populations. This will ensure the equitable advancement of personalized medicine and healthcare generally. Diverse populations with distinct lifestyles and dietary patterns reside in LMICs. Microbiome studies in these regions are likely to elucidate population-specific variations in susceptibility to disease, response to therapies, and interaction with the environment. Likely barriers in some of the most underrepresented groups are highlighted subsequently.
5.1 Sub-Saharan Africa
Perhaps the most underrepresented region in gut microbiome research is Sub-Saharan Africa. With its immense genetic, cultural, and environmental diversity, increasing research in this region will greatly advance our understanding of unique microbial interactions and the related implications for human health. Research in this area is limited largely due to barriers such as insufficient research infrastructure, funding scarcity, access to advanced technologies, and ethical issues such as informed consent and sharing samples (176, 177). In addition to addressing these barriers to gut microbiome research, promoting collaborative research in this field within Africa will lead to priceless insights into the diverse human population in this continent as well as a more complete comprehension of the human microbiome globally.
5.2 Latin America
Gut microbiome research remains comparatively limited in Latin America as a region. In contrast, some countries have made advances in this field; however, even these are still lagging behind high-income countries. Major barriers include funding scarcity, insufficient research infrastructure, and inadequate expertise pipelines. Collaboration between Latin American and international researchers can help bridge this gap and foster knowledge exchange to enhance gut microbiome research regionally and globally (178).
5.3 Southeast Asia
Due to the large population in Southeast Asia, this region represents one of the most significant for global health research, generally, and gut microbiome research, specifically. Despite this, this region continues to lag behind in gut microbiome research. Barriers include a dearth of well-established research institutions (insufficient research infrastructure) and funding scarcity. As in Latin America, collaboration may fill some of this gap; however, regional capacity building will also be required (179). Both are necessary to advance gut microbiome research in this region and lead to a wholistic understanding of the gut microbiome globally.
5.4 Middle East
The final key, underrepresented region in gut microbiome region is the Middle East with relatively limited gut microbiome research (180, 181). Barriers in this region include political instability, funding scarcity, and ethical issues including cultural norms and sharing data. Again, international collaboration can overcome some of this with the ultimate need for local capacity building.
5.5 Considerations for improving the generalizability of microbiome research
Capacity building is a key step in addressing the underrepresentation of LMICs in gut microbiome research. To build capacity locally, investments will need to be made to provide training and support to local researchers, equipping them with the latest technology, and developing collaborations between HIC and LMIC institutions. An example of this being success is reported by Maduka et al. in 2017, where African researchers were empowered through bioinformatics training and development of the necessary infrastructure (182).
Another element of support is international partnerships and exchange programs, which can cultivate knowledge exchange and resource sharing. It is common to see large-scale collaborations in HICs (e.g. the Human Microbiome Project), which have facilitated data and resource sharing to advance gut microbiome research with comprehensive datasets and groundbreaking discoveries (141, 183). Some of these HIC projects have promoted international collaboration (i.e. Earth Microbiome Project, American Gut Project, MetaHIT Consortium) and could serve as models for bringing LMICs in as well (184–186).
Additionally, community engagement is indispensable to ensuring appropriate, diverse representation in gut microbiome research. To conduct research in these populations, culturally appropriate approaches must be used, which require respecting cultural practices and beliefs (187). Further, such approaches (e.g. culturally sensitive recruitment strategies and community-based participatory research design) promote diversity, equity, and inclusion in the research and the study population, enhancing the applicability of the findings (188). To assure fair and equitable development of this emerging field, such ethical considerations must be prioritized including as they relate to sharing of data and resources as well as the informed consent process, particularly when vulnerable populations (i.e. those in LICs) are involved.
The generalizability of gut microbiome research can also be improved by the inclusion standardization, longitudinal study designs, and multi-omics analysis. Standardizing methodologies ensures the comparability and reproducibility of gut microbiome research findings (189, 190). To establish guidelines for data generation, processing, and analysis and facilite harmonization across studies, the International Human Microbiome Standards project was established (173, 186); this data will lay the foundation for personalized and innovative methods of prevention, treatment, and management of disease. Longitudinal studies are necessary to understand how the gut microbiome evolves over time and how this relates to health outcomes and/or the pathogenesis of disease. Further, such long-term studies are likely to elucidate unknown relationships between alterations of the gut microbiome and disease development that cannot be studies through observational or cross-sectional studies. Finally, integrating multi-omics data (e.g. genomics, epigenomics, metagenomics, metabolomics) will provide a wholistic understanding of the interactions among the microbiota-gut-brain-immune interface, revealing novel biomarkers and therapeutic targets for numerous disease states. The combination of these will advance personalized healthcare globally.
Author contributions
AW: Conceptualization, Project administration, Writing – original draft, Writing – review & editing. YN: Conceptualization, Project administration, Writing – original draft, Writing – review & editing. NZ: Writing – original draft, Writing – review & editing. AM: Writing – review & editing. LF: Conceptualization, Project administration, Resources, Supervision, Writing – original draft, Writing – review & editing.
Funding
The author(s) declare that no financial support was received for the research, authorship, and/or publication of this article.
Conflict of interest
The authors declare that the research was conducted in the absence of any commercial or financial relationships that could be construed as a potential conflict of interest.
Publisher’s note
All claims expressed in this article are solely those of the authors and do not necessarily represent those of their affiliated organizations, or those of the publisher, the editors and the reviewers. Any product that may be evaluated in this article, or claim that may be made by its manufacturer, is not guaranteed or endorsed by the publisher.
Supplementary material
The Supplementary Material for this article can be found online at: https://www.frontiersin.org/articles/10.3389/fimmu.2024.1365673/full#supplementary-material
Supplementary Table 1 | Observed functional relationship with the gut microbiota.
References
1. Walker RW, Clemente JC, Peter I, Loos RJF. The prenatal gut microbiome: are we colonized with bacteria in utero? Pediatr Obes. (2017) 12:3–17. doi: 10.1111/IJPO.12217
2. Jena PK, Sheng L, Di Lucente J, Jin LW, Maezawa I, Wan YJY. Dysregulated bile acid synthesis and dysbiosis are implicated in Western diet–induced systemic inflammation, microglial activation, and reduced neuroplasticity. FASEB J. (2018) 32:2866–77. doi: 10.1096/fj.201700984RR
3. Więckowska-Gacek A, Mietelska-Porowska A, Wydrych M, Wojda U. Western diet as a trigger of Alzheimer’s disease: From metabolic syndrome and systemic inflammation to neuroinflammation and neurodegeneration. Ageing Res Rev. (2021) 70:101397. doi: 10.1016/J.ARR.2021.101397
4. Mosca A, Leclerc M, Hugot JP. Gut microbiota diversity and human diseases: Should we reintroduce key predators in our ecosystem? Front Microbiol. (2016) 7:455/BIBTEX. doi: 10.3389/fmicb.2016.00455
5. Roy Sarkar S, Banerjee S. Gut microbiota in neurodegenerative disorders. J Neuroimmunol. (2019) 328:98–104. doi: 10.1016/J.JNEUROIM.2019.01.004
6. Aburto MR, Cryan JF. Gastrointestinal and brain barriers: unlocking gates of communication across the microbiota–gut–brain axis. Nat Rev Gastroenterol Hepatol. (2024) 21:222–47. doi: 10.1038/s41575-023-00890-0
7. Hashimoto K. Emerging role of the host microbiome in neuropsychiatric disorders: overview and future directions. Mol Psychiatry. (2023) 28:3625–37. doi: 10.1038/s41380-023-02287-6
8. Ruff WE, Greiling TM, Kriegel MA. Host–microbiota interactions in immune-mediated diseases. Nat Rev Microbiol. (2020) 18:521–38. doi: 10.1038/s41579-020-0367-2
9. Hou K, Wu ZX, Chen XY, Wang JQ, Zhang D, Xiao C, et al. Microbiota in health and diseases. Signal Transduction Targeted Ther. (2022) 7:1–28. doi: 10.1038/s41392-022-00974-4
10. Ullah H, Arbab S, Tian Y, Liu CQ, Chen Y, Qijie L, et al. The gut microbiota–brain axis in neurological disorder. Front Neurosci. (2023) 17:1225875/BIBTEX. doi: 10.3389/fnins.2023.1225875
11. Boem F, Greslehner GP, Konsman JP, Chiu L. Minding the gut: extending embodied cognition and perception to the gut complex. Front Neurosci. (2023) 17:1172783/BIBTEX. doi: 10.3389/fnins.2023.1172783
12. Loh JS, Mak WQ, Tan LKS, Ng CX, Chan HH, Yeow SH, et al. Microbiota–gut–brain axis and its therapeutic applications in neurodegenerative diseases. Signal Transduction Targeted Ther. (2024) 9:1–53. doi: 10.1038/s41392-024-01743-1
13. Giallongo S, Longhitano L, Denaro S, D’Aprile S, Torrisi F, La Spina E, et al. The role of epigenetics in neuroinflammatory-driven diseases. Int J Mol Sci. (2022) 23:15218. doi: 10.3390/IJMS232315218
14. Muller PA, Schneeberger M, Matheis F, Wang P, Kerner Z, Ilanges A, et al. Microbiota modulate sympathetic neurons via a gut–brain circuit. Nat 2020 583:7816. (2020) 583:441–6. doi: 10.1038/s41586-020-2474-7
15. Vicentini FA, Keenan CM, Wallace LE, Woods C, Cavin JB, Flockton AR, et al. Intestinal microbiota shapes gut physiology and regulates enteric neurons and glia. Microbiome. (2021) 9:1–24. doi: 10.1186/s40168-021-01165-z
16. Serger E, Luengo-Gutierrez L, Chadwick JS, Kong G, Zhou L, Crawford G, et al. The gut metabolite indole-3 propionate promotes nerve regeneration and repair. Nature. (2022) 607:585–92. doi: 10.1038/s41586-022-04884-x
17. Li B, He Y, Ma J, Huang P, Du J, Cao L, et al. Mild cognitive impairment has similar alterations as Alzheimer’s disease in gut microbiota. Alzheimer’s Dementia. (2019) 15:1357–66. doi: 10.1016/J.JALZ.2019.07.002
18. Cryan J. The microbiome as a key regulator of stress & Neuroinflammation across the lifespan. Psychoneuroendocrinology. (2021) 131:105571. doi: 10.1016/J.PSYNEUEN.2021.105571
19. Fang P, Kazmi SA, Jameson KG, Hsiao EY. The microbiome as a modifier of neurodegenerative disease risk. Cell Host Microbe. (2020) 28:201–22. doi: 10.1016/J.CHOM.2020.06.008
20. Farooq RK, Alamoudi W, Alhibshi A, Rehman S, Sharma AR, Abdulla FA. Varied composition and underlying mechanisms of gut microbiome in neuroinflammation. Microorganisms. (2022) 10:705. doi: 10.3390/MICROORGANISMS10040705
21. Follmer C. Gut microbiome imbalance and neuroinflammation: impact of COVID-19 on Parkinson’s disease. Movement Disord. (2020) 35:1495–6. doi: 10.1002/MDS.28231
22. Sampson TR, Debelius JW, Thron T, Janssen S, Shastri GG, Ilhan ZE, et al. Gut microbiota regulate motor deficits and neuroinflammation in a model of Parkinson’s disease. Cell. (2016) 167:1469–1480.e12. doi: 10.1016/j.cell.2016.11.018
23. Bostick JW, Schonhoff AM, Mazmanian SK. Gut microbiome-mediated regulation of neuroinflammation. Curr Opin Immunol. (2022) 76:102177. doi: 10.1016/J.COI.2022.102177
24. Masanetz RK, Winkler J, Winner B, Günther C, Süß P. The gut–immune–brain axis: an important route for neuropsychiatric morbidity in inflammatory bowel disease. Int J Mol Sci. (2022) 23:11111. doi: 10.3390/IJMS231911111
25. Frame LA, Costa E, Jackson SA. Current explorations of nutrition and the gut microbiome: A comprehensive evaluation of the review literature. Nutr Rev. (2020) 78:798–812. doi: 10.1093/nutrit/nuz106
26. Wan X, Eguchi A, Sakamoto A, Fujita Y, Yang Y, Qu Y, et al. Impact of broad-spectrum antibiotics on the gut–microbiota–spleen–brain axis. Brain Behav Immun Health. (2023) 27:100573. doi: 10.1016/J.BBIH.2022.100573
27. Wei Y, Wang T, Liao L, Fan X, Chang L, Hashimoto K. Brain-spleen axis in health and diseases: A review and future perspective. Brain Res Bull. (2022) 182:130–40. doi: 10.1016/J.BRAINRESBULL.2022.02.008
28. Chen WG, Schloesser D, Arensdorf AM, Simmons JM, Cui C, Valentino R, et al. The emerging science of interoception: sensing, integrating, interpreting, and regulating signals within the self. Trends Neurosci. (2021) 44:3–16. doi: 10.1016/J.TINS.2020.10.007
29. Goggins E, Mitani S, Tanaka S. Clinical perspectives on vagus nerve stimulation: present and future. Clin Sci. (2022) 136:695–709. doi: 10.1042/CS20210507
30. Fülling C, Dinan TG, Cryan JF. Gut microbe to brain signaling: what happens in Vagus…. Neuron. (2019) 101:998–1002. doi: 10.1016/j.neuron.2019.02.008
31. Bonaz B, Bazin T, Pellissier S. The vagus nerve at the interface of the microbiota-gut-brain axis. Front Neurosci. (2018) 12:49/BIBTEX. doi: 10.3389/fnins.2018.00049
32. Tan C, Yan Q, Ma Y, Fang J, Yang Y. Recognizing the role of the vagus nerve in depression from microbiota-gut brain axis. Front Neurol. (2022) 13:1015175/BIBTEX. doi: 10.3389/fneur.2022.1015175
33. Champagne-Jorgensen K, Mian MF, McVey Neufeld KA, Stanisz AM, Bienenstock J. Membrane vesicles of Lacticaseibacillus rhamnosus JB-1 contain immunomodulatory lipoteichoic acid and are endocytosed by intestinal epithelial cells. Sci Rep. (2021) 11:1–10. doi: 10.1038/s41598-021-93311-8
34. Wang X, Eguchi A, Yang Y, Chang L, Wan X, Shan J, et al. Key role of the gut–microbiota–brain axis via the subdiaphragmatic vagus nerve in demyelination of the cuprizone-treated mouse brain. Neurobiol Dis. (2023) 176:105951. doi: 10.1016/J.NBD.2022.105951
35. Martin CR, Osadchiy V, Kalani A, Mayer EA. The brain-gut-microbiome axis. Cell Mol Gastroenterol Hepatol. (2018) 6:133–48. doi: 10.1016/J.JCMGH.2018.04.003
36. Kiriyama Y, Nochi H. Role of microbiota-modified bile acids in the regulation of intracellular organelles and neurodegenerative diseases. Genes 2023 Vol 14 Page 825. (2023) 14:825. doi: 10.3390/GENES14040825
37. Mulak A. Bile acids as key modulators of the brain-gut-microbiota axis in alzheimer’s disease. J Alzheimer’s Dis. (2021) 84:461–77. doi: 10.3233/JAD-210608
38. Shulpekova Y, Zharkova M, Tkachenko P, Tikhonov I, Stepanov A, Synitsyna A, et al. The role of bile acids in the human body and in the development of diseases. Molecules. (2022) 27:3401. doi: 10.3390/MOLECULES27113401
39. Yano JM, Yu K, Donaldson GP, Shastri GG, Ann P, Ma L, et al. Indigenous bacteria from the gut microbiota regulate host serotonin biosynthesis. Cell. (2015) 161:264–76. doi: 10.1016/j.cell.2015.02.047
40. MahmoudianDehkordi S, Arnold M, Nho K, Ahmad S, Jia W, Xie G, et al. Altered bile acid profile associates with cognitive impairment in Alzheimer’s disease-An emerging role for gut microbiome. Alzheimers Dement. (2019) 15:76–92. doi: 10.1016/J.JALZ.2018.07.217
41. Hurley MJ, Bates R, Macnaughtan J, Schapira AHV. Bile acids and neurological disease. Pharmacol Ther. (2022) 240:108311. doi: 10.1016/J.PHARMTHERA.2022.108311
42. Litvak Y, Byndloss MX, Bäumler AJ. Colonocyte metabolism shapes the gut microbiota. Sci (1979). (2018) 362:eaat9076. doi: 10.1126/science.aat9076
43. Hu M, Alhamwe BA, Santner-nanan B, Miethe S, Harb H, Renz H, et al. Short-chain fatty acids augment differentiation and function of human induced regulatory T cells. Int J Mol Sci. (2022) 23:5740. doi: 10.3390/ijms23105740
44. Kim CH. Control of lymphocyte functions by gut microbiota-derived short-chain fatty acids. Cell Mol Immunol. (2021) 18:1161–71. doi: 10.1038/s41423-020-00625-0
45. Wang J, Zhu N, Su X, Gao Y, Yang R. Gut-microbiota-derived metabolites maintain gut and systemic immune homeostasis. Cells. (2023) 12:793. doi: 10.3390/CELLS12050793
46. Muhammad JS, Siddiqui R, Khan NA. Is there a role of epigenetics in gut microbiome and neurological disorders? APMIS. (2022) 130:778–80. doi: 10.1111/APM.13257
47. Lynch JB, Hsiao EY. Toward understanding links between the microbiome and neurotransmitters. Ann N Y Acad Sci. (2023) 1524:10–6. doi: 10.1111/NYAS.14993
48. Bermúdez-Humarán LG, Salinas E, Ortiz GG, Ramirez-Jirano LJ, Morales JA, Bitzer-Quintero OK. From probiotics to psychobiotics: live beneficial bacteria which act on the brain-gut axis. Nutrients. (2019) 11:890. doi: 10.3390/NU11040890
49. Banerjee A, Pradhan LK, Sahoo PK, Jena KK, Chauhan NR, Chauhan S, et al. Unravelling the potential of gut microbiota in sustaining brain health and their current prospective towards development of neurotherapeutics. Arch Microbiol. (2021) 203:2895–910. doi: 10.1007/S00203-021-02276-9
50. Ferrugem L, Martini G, de Souza C. Influence of the glycemic index of pre-exercise meals in sports performance: A systematic review. Int J Med Rev. (2018) 5(4):151–8. doi: 10.29252/ijmr-050405
51. Margolis KG, Cryan JF, Mayer EA. The microbiota-gut-brain axis: from motility to mood. Gastroenterology. (2021) 160:1486–501. doi: 10.1053/J.GASTRO.2020.10.066
52. Magni G, Riboldi B, Petroni K, Ceruti S. Flavonoids bridging the gut and the brain: Intestinal metabolic fate, and direct or indirect effects of natural supporters against neuroinflammation and neurodegeneration. Biochem Pharmacol. (2022) 205:115257. doi: 10.1016/J.BCP.2022.115257
53. Bakshi A, Tadi P. Biochemistry, serotonin (2022). StatPearls. Available at: https://www.ncbi.nlm.nih.gov/books/NBK560856/ (Accessed November 26, 2023).
54. Guzel T, Mirowska-Guzel D. The role of serotonin neurotransmission in gastrointestinal tract and pharmacotherapy. Molecules. (2022) 27:1680. doi: 10.3390/MOLECULES27051680
55. Goyal D, Ali SA, Singh RK. Emerging role of gut microbiota in modulation of neuroinflammation and neurodegeneration with emphasis on Alzheimer’s disease. Prog Neuropsychopharmacol Biol Psychiatry. (2021) 106:110112. doi: 10.1016/J.PNPBP.2020.110112
56. Lamptey RNL, Chaulagain B, Trivedi R, Gothwal A, Layek B, Singh JA. Review of the common neurodegenerative disorders: current therapeutic approaches and the potential role of nanotherapeutics. Int J Mol Sci. (2022) 23:1851. doi: 10.3390/IJMS23031851
57. Leng F, Hinz R, Gentleman S, Brooks DJ, Edison P. Neuroinflammation, functional connectivity and structural network integrity in the Alzheimer’s spectrum. Alzheimer’s Dementia. (2021) 17:e055970. doi: 10.1002/ALZ.055970
58. Garden GA. Epigenetics and the modulation of neuroinflammation. Neurotherapeutics. (2013) 10:782–8. doi: 10.1007/s13311-013-0207-4
59. D’Amato A, Di Cesare Mannelli L, Lucarini E, Man AL, Le Gall G, Branca JJV, et al. Faecal microbiota transplant from aged donor mice affects spatial learning and memory via modulating hippocampal synaptic plasticity- And neurotransmission-related proteins in young recipients. Microbiome. (2020) 8:1–19. doi: 10.1186/S40168-020-00914-W/FIGURES/10
60. Hecking I, Stegemann LN, Theis V, Vorgerd M, Matschke V, Stahlke S, et al. Neuroprotective effects of VEGF in the enteric nervous system. Int J Mol Sci. (2022) 23:6756. doi: 10.3390/ijms23126756
61. Rea K, Dinan TG, Cryan JF. The microbiome: A key regulator of stress and neuroinflammation. Neurobiol Stress. (2016) 4:23–33. doi: 10.1016/J.YNSTR.2016.03.001
62. Liu H, Leak RK, Hu X. Neurotransmitter receptors on microglia. Stroke Vasc Neurol. (2016) 1:52–8. doi: 10.1136/SVN-2016-000012
63. Passamonti L, Tsvetanov KA, Jones PS, Bevan-Jones WR, Arnold R, Borchert RJ, et al. Neuroinflammation and functional connectivity in alzheimer’s disease: interactive influences on cognitive performance. J Neurosci. (2019) 39:7218–26. doi: 10.1523/JNEUROSCI.2574-18.2019
64. Reyes REN, Zhang Z, Gao L, Asatryan L. Microbiome meets microglia in neuroinflammation and neurological disorders. Neuroimmunol Neuroinflamm. (2020) 7:215–33. doi: 10.20517/2347-8659.2020.13
65. MahmoudianDehkordi S, Arnold M, Nho K, Ahmad S, Jia W, Xie G, et al. Altered bile acid profile associates with cognitive impairment in Alzheimer’s disease—An emerging role for gut microbiome. Alzheimer’s Dementia. (2019) 15:76–92. doi: 10.1016/J.JALZ.2018.07.217
66. Cortes C, Desler C, Mazzoli A, Chen JY, Ferreira VP. The role of properdin and Factor H in disease. Adv Immunol. (2022) 153:1–90. doi: 10.1016/BS.AI.2021.12.001
67. Behzadi P, Sameer AS, Nissar S, Banday MZ, Gajdács M, García-Perdomo HA, et al. The interleukin-1 (IL-1) superfamily cytokines and their single nucleotide polymorphisms (SNPs). J Immunol Res. (2022) 2022:2054431. doi: 10.1155/2022/2054431
68. Mukherjee S, Patra R, Behzadi P, Masotti A, Paolini A, Sarshar M. Toll-like receptor-guided therapeutic intervention of human cancers: molecular and immunological perspectives. Front Immunol. (2023) 14:1244345/BIBTEX. doi: 10.3389/fimmu.2023.1244345
69. Chandra S, Sisodia SS, Vassar RJ. The gut microbiome in Alzheimer’s disease: what we know and what remains to be explored. Mol Neurodegeneration. (2023) 18:1–21. doi: 10.1186/S13024-023-00595-7
70. Ferreiro AL, Choi JH, Ryou J, Newcomer EP, Thompson R, Bollinger RM, et al. Gut microbiome composition may be an indicator of preclinical Alzheimer’s disease. Sci Transl Med. (2023) 15:eabo2984. doi: 10.1126/scitranslmed.abo2984
71. Lu X. Alzheimer’s disease’s tau and amyloid-beta hypothesis - Interplay with the innate immune system, neuroinflammation and gut microbiome. AIP Conf Proc. (2022) 2511:020015. doi: 10.1063/5.0095072/2827487
72. Vogt NM, Kerby RL, Dill-McFarland KA, Harding SJ, Merluzzi AP, Johnson SC, et al. Gut microbiome alterations in Alzheimer’s disease. Sci Rep. (2017) 7:1–11. doi: 10.1038/s41598-017-13601-y
73. Cattaneo A, Cattane N, Galluzzi S, Provasi S, Lopizzo N, Festari C, et al. Association of brain amyloidosis with pro-inflammatory gut bacterial taxa and peripheral inflammation markers in cognitively impaired elderly. Neurobiol Aging. (2017) 49:60–8. doi: 10.1016/J.NEUROBIOLAGING.2016.08.019
74. Zhuang ZQ, Shen LL, Li WW, Fu X, Zeng F, Gui L, et al. Gut microbiota is altered in patients with alzheimer’s disease. J Alzheimer’s Dis. (2018) 63:1337–46. doi: 10.3233/JAD-180176
75. Liu P, Wu L, Peng G, Han Y, Tang R, Ge J, et al. Altered microbiomes distinguish Alzheimer’s disease from amnestic mild cognitive impairment and health in a Chinese cohort. Brain Behav Immun. (2019) 80:633–43. doi: 10.1016/J.BBI.2019.05.008
76. Romano S, Savva GM, Bedarf JR, Charles IG, Hildebrand F, Narbad A. Meta-analysis of the Parkinson’s disease gut microbiome suggests alterations linked to intestinal inflammation. NPJ Parkinson’s Dis. (2021) 7:1–13. doi: 10.1038/s41531-021-00156-z
77. Bradford SA. The environment and disease: association or causation? J R Soc Med. (1965) 58:295–300. doi: 10.1177/003591576505800503
78. Breen DP, Halliday GM, Lang AE. Gut–brain axis and the spread of α-synuclein pathology: Vagal highway or dead end? Movement Disord. (2019) 34:307–16. doi: 10.1002/MDS.27556
79. Keshavarzian A, Engen P, Bonvegna S, Cilia R. The gut microbiome in Parkinson’s disease: A culprit or a bystander? Prog Brain Res. (2020) 252:357–450. doi: 10.1016/BS.PBR.2020.01.004
80. Correale J, Hohlfeld R, Baranzini SE. The role of the gut microbiota in multiple sclerosis. Nat Rev Neurol. (2022) 18:544–58. doi: 10.1038/s41582-022-00697-8
81. Sasmita AO. Modification of the gut microbiome to combat neurodegeneration. Rev Neurosci. (2019) 30:795–805. doi: 10.1515/revneuro-2019-0005
82. Zhou X, Baumann R, Gao X, Mendoza M, Singh S, Katz Sand I, et al. Gut microbiome of multiple sclerosis patients and paired household healthy controls reveal associations with disease risk and course. Cell. (2022) 185:3467–3486.e16. doi: 10.1016/J.CELL.2022.08.021
83. Ma Y, Sannino D, Linden JR, Haigh S, Zhao B, Grigg JB, et al. Epsilon toxin–producing Clostridium perfringens colonize the multiple sclerosis gut microbiome overcoming CNS immune privilege. J Clin Invest. (2023) 133. doi: 10.1172/JCI163239
84. Bronzini M, Maglione A, Rosso R, Matta M, Masuzzo F, Rolla S, et al. Feeding the gut microbiome: impact on multiple sclerosis. Front Immunol. (2023) 14:1176016/BIBTEX. doi: 10.3389/fimmu.2023.1176016
85. Bonnechère B, Amin N, van Duijn C. What are the key gut microbiota involved in neurological diseases? A systematic review. Int J Mol Sci. (2022) 23:13665. doi: 10.3390/ijms232213665
86. Shannon OM, Ranson JM, Gregory S, Macpherson H, Milte C, Lentjes M, et al. Mediterranean diet adherence is associated with lower dementia risk, independent of genetic predisposition: findings from the UK Biobank prospective cohort study. BMC Med. (2023) 21:1–13. doi: 10.1186/s12916-023-02772-3
87. Bello-Corral L, Sánchez-Valdeón L, Casado-Verdejo I, Seco-Calvo JÁ, Antonio Fernández-Fernández J, Nélida Fernández-Martínez M. The influence of nutrition in alzheimer’s disease: neuroinflammation and the microbiome vs. Transmissible Prion Front Neurosci. (2021) 15:677777/BIBTEX. doi: 10.3389/fnins.2021.677777
88. Russell WR, Gratz SW, Duncan SH, Holtrop G, Ince J, Scobbie L, et al. High-protein, reduced-carbohydrate weight-loss diets promote metabolite profiles likely to be detrimental to colonic health. Am J Clin Nutr. (2011) 93:1062–72. doi: 10.3945/AJCN.110.002188
89. Vauzour D, Camprubi-Robles M, Miquel-Kergoat S, Andres-Lacueva C, Bánáti D, Barberger-Gateau P, et al. Nutrition for the ageing brain: Towards evidence for an optimal diet. Ageing Res Rev. (2017) 35:222–40. doi: 10.1016/J.ARR.2016.09.010
90. Hirschberg S, Gisevius B, Duscha A, Haghikia A. Implications of diet and the gut microbiome in neuroinflammatory and neurodegenerative diseases. Int J Mol Sci. (2019) 20:3109. doi: 10.3390/IJMS20123109
91. Ettinger S. Diet, gut microbiome, and cognitive decline. Curr Nutr Rep. (2022) 11:643–52. doi: 10.1007/s13668-022-00435-y
92. Gates EJ, Bernath AK, Klegeris A. Modifying the diet and gut microbiota to prevent and manage neurodegenerative diseases. Rev Neurosci. (2022) 33:767–87. doi: 10.1515/revneuro-2021-0146
93. Foster JA, Rinaman L, Cryan JF. Stress & the gut-brain axis: Regulation by the microbiome. Neurobiol Stress. (2017) 7:124–36. doi: 10.1016/J.YNSTR.2017.03.001
94. Tong J, Satyanarayanan SK, Su H. Nutraceuticals and probiotics in the management of psychiatric and neurological disorders: A focus on microbiota-gut-brain-immune axis. Brain Behav Immun. (2020) 90:403–19. doi: 10.1016/J.BBI.2020.08.027
95. Capuano E, Janssen AEM. Food matrix and macronutrient digestion. Annu Rev Food Sci Technol (2021) 12:193–212. doi: 10.1146/ANNUREV-FOOD-032519-051646
96. Miao M, Hamaker BR. Food matrix effects for modulating starch bioavailability. Annu Rev Food Sci Technol (2021) 12:169–91. doi: 10.1146/ANNUREV-FOOD-070620-013937
97. Zhang J, Wang H, Ai C, Lu R, Chen L, Xiao J, et al. Food matrix-flavonoid interactions and their effect on bioavailability. Crit Rev Food Sci Nutr. (2023) 10:1–22. doi: 10.1080/10408398.2023.2232880
98. Forde CG, Bolhuis D. Interrelations between food form, texture, and matrix influence energy intake and metabolic responses. Curr Nutr Rep. (2022) 11:124–32. doi: 10.1007/s13668-022-00413-4
99. Devranis P, Vassilopoulou E, Tsironis V, Sotiriadis P-M, Chourdakis M, Aivaliotis M, et al. Mediterranean diet, ketogenic diet or MIND diet for aging populations with cognitive decline: A systematic review. Public Health Toxicol. (2022) 2124–32. doi: 10.18332/PHT/149647
100. Grodzicki W, Dziendzikowska K. The role of selected bioactive compounds in the prevention of alzheimer’s disease. Antioxidants. (2020) 9:229. doi: 10.3390/ANTIOX9030229
101. Van Den Brink AC, Brouwer-Brolsma EM, Berendsen AAM, Van De Rest O. The mediterranean, dietary approaches to stop hypertension (DASH), and mediterranean-DASH intervention for neurodegenerative delay (MIND) diets are associated with less cognitive decline and a lower risk of alzheimer’s disease—A review. Adv Nutr. (2019) 10:1040–65. doi: 10.1093/ADVANCES/NMZ054
102. Kheirouri S, Alizadeh M. MIND diet and cognitive performance in older adults: a systematic review. Crit Rev Food Sci Nutr. (2022) 62:8059–77. doi: 10.1080/10408398.2021.1925220
103. Chen H, Dhana K, Huang Y, Huang L, Tao Y, Liu X, et al. Association of the mediterranean dietary approaches to stop hypertension intervention for neurodegenerative delay (MIND) diet with the risk of dementia. JAMA Psychiatry. (2023) 80:630–8. doi: 10.1001/JAMAPSYCHIATRY.2023.0800
104. Agarwal P, Leurgans SE, Agrawal S, Aggarwal NT, Cherian LJ, James BD, et al. Association of mediterranean-DASH intervention for neurodegenerative delay and mediterranean diets with alzheimer disease pathology. Neurology. (2023) 100:e2259–68. doi: 10.1212/WNL.0000000000207176
105. de Crom TOE, Mooldijk SS, Ikram MK, Ikram MA, Voortman T. MIND diet and the risk of dementia: a population-based study. Alzheimers Res Ther. (2022) 14:1–10. doi: 10.1186/s13195-022-00957-1
106. Matusheski NV, Caffrey A, Christensen L, Mezgec S, Surendran S, Hjorth MF, et al. Diets, nutrients, genes and the microbiome: recent advances in personalised nutrition. Br J Nutr. (2021) 126:1489–97. doi: 10.1017/S0007114521000374
107. El-Sayed A, Aleya L, Kamel M. Microbiota and epigenetics: promising therapeutic approaches? Environ Sci pollut Res. (2021) 28:49343–61. doi: 10.1007/s11356-021-15623-6
108. Mehta V, Nagu P, Inbaraj BS, Sharma M, Parashar A, Sridhar K. Epigenetics and gut microbiota crosstalk: A potential factor in pathogenesis of cardiovascular disorders. Bioengineering. (2022) 9:798. doi: 10.3390/BIOENGINEERING9120798
109. Li X, Qi L. Epigenetics in precision nutrition. J Personalized Med. (2022) 12:533. doi: 10.3390/JPM12040533
110. Toledo ARL, Monroy GR, Salazar FE, Lee JY, Jain S, Yadav H, et al. Gut–brain axis as a pathological and therapeutic target for neurodegenerative disorders. Int J Mol Sci. (2022) 23:1184. doi: 10.3390/IJMS23031184
111. Sharma B, Kumar A, Sharma U, Pal D, Prashar S. The potential role of gut microbiota in the pathogenesis of type 2 diabetes mellitus via epigenetics and inflammasome. Endocr Metab Immune Disord Drug Targets. (2022) 22:1331–43. doi: 10.2174/1871530322666220331152809
112. Amanollahi M, Jameie M, Heidari A, Rezaei N. The dialogue between neuroinflammation and adult neurogenesis: mechanisms involved and alterations in neurological diseases. Mol Neurobiol. (2022) 60:923–59. doi: 10.1007/s12035-022-03102-z
113. Kelly JR, Kennedy PJ, Cryan JF, Dinan TG, Clarke G, Hyland NP. Breaking down the barriers: The gut microbiome, intestinal permeability and stress-related psychiatric disorders. Front Cell Neurosci. (2015) 9:392/BIBTEX. doi: 10.3389/fncel.2015.00392
114. Alam R, Abdolmaleky HM, Zhou JR. Microbiome, inflammation, epigenetic alterations, and mental diseases. Am J Med Genet Part B: Neuropsychiatr Genet. (2017) 174:651–60. doi: 10.1002/AJMG.B.32567
115. Yeh AM, Wren A, Golianu B. Mind–body interventions for pediatric inflammatory bowel disease. Children. (2017) 4:22. doi: 10.3390/CHILDREN4040022
116. Yan Q. Neuroimmune imbalances and Yin-Yang dynamics in stress, anxiety, and depression. Methods Mol Biol. (2018) 1781:77–85. doi: 10.1007/978-1-4939-7828-1_5/COVER
117. Breit S, Kupferberg A, Rogler G, Hasler G. Vagus nerve as modulator of the brain-gut axis in psychiatric and inflammatory disorders. Front Psychiatry. (2018) 9:44/BIBTEX. doi: 10.3389/fpsyt.2018.00044
118. Cahn BR, Goodman MS, Peterson CT, Maturi R, Mills PJ. Yoga, meditation and mind-body health: Increased BDNF, cortisol awakening response, and altered inflammatory marker expression after a 3-month yoga and meditation retreat. Front Hum Neurosci. (2017) 11:315/BIBTEX. doi: 10.3389/fnhum.2017.00315
119. Smith RP, Easson C, Lyle SM, Kapoor R, Donnelly CP, Davidson EJ, et al. Gut microbiome diversity is associated with sleep physiology in humans. PloS One. (2019) 14:e0222394. doi: 10.1371/JOURNAL.PONE.0222394
120. Wagner-Skacel J, Dalkner N, Moerkl S, Kreuzer K, Farzi A, Lackner S, et al. Sleep and microbiome in psychiatric diseases. Nutrients. (2020) 12:2198. doi: 10.3390/NU12082198
121. Allen JM, Mailing LJ, Niemiro GM, Moore R, Cook MD, White BA, et al. Exercise alters gut microbiota composition and function in lean and obese humans. Med Sci Sports Exerc. (2018) 50:747–57. doi: 10.1249/MSS.0000000000001495
122. Mailing LJ, Allen JM, Buford TW, Fields CJ, Woods JA. Exercise and the gut microbiome: A review of the evidence, potential mechanisms, and implications for human health. Exerc Sport Sci Rev. (2019) 47:75–85. doi: 10.1249/JES.0000000000000183
123. Ramos C, Gibson GR, Walton GE, Magistro D, Kinnear W, Hunter K. Systematic review of the effects of exercise and physical activity on the gut microbiome of older adults. Nutrients. (2022) 14:674. doi: 10.3390/NU14030674
124. Hughes RL, Holscher HD. Fueling gut microbes: A review of the interaction between diet, exercise, and the gut microbiota in athletes. Adv Nutr. (2021) 12:2190–215. doi: 10.1093/ADVANCES/NMAB077
125. Gubert C, Kong G, Renoir T, Hannan AJ. Exercise, diet and stress as modulators of gut microbiota: Implications for neurodegenerative diseases. Neurobiol Dis. (2020) 134:104621. doi: 10.1016/J.NBD.2019.104621
126. Campos C, Rocha NBF, Lattari E, Paes F, Nardi AE, MaChado S. Exercise-induced neuroprotective effects on neurodegenerative diseases: the key role of trophic factors. Expert Rev Neurother. (2016) 16:723–34. doi: 10.1080/14737175.2016.1179582
127. Palasz E, Wysocka A, Gasiorowska A, Chalimoniuk M, Niewiadomski W, Niewiadomska G. BDNF as a promising therapeutic agent in parkinson’s disease. Int J Mol Sci. (2020) 21:1170. doi: 10.3390/IJMS21031170
128. Sujkowski A, Hong L, Wessells RJ, Todi SV. The protective role of exercise against age-related neurodegeneration. Ageing Res Rev. (2022) 74:101543. doi: 10.1016/J.ARR.2021.101543
129. Ionescu-Tucker A, Cotman CW. Emerging roles of oxidative stress in brain aging and Alzheimer’s disease. Neurobiol Aging. (2021) 107:86–95. doi: 10.1016/J.NEUROBIOLAGING.2021.07.014
130. Mallick R, Duttaroy AK. Epigenetic modification impacting brain functions: Effects of physical activity, micronutrients, caffeine, toxins, and addictive substances. Neurochem Int. (2023) 171:105627. doi: 10.1016/J.NEUINT.2023.105627
131. Hill C, Guarner F, Reid G, Gibson GR, Merenstein DJ, Pot B, et al. The International Scientific Association for Probiotics and Prebiotics consensus statement on the scope and appropriate use of the term probiotic. Nat Rev Gastroenterol Hepatol. (2014) 11:506–14. doi: 10.1038/nrgastro.2014.66
132. Bonfili L, Cecarini V, Berardi S, Scarpona S, Suchodolski JS, Nasuti C, et al. Microbiota modulation counteracts Alzheimer’s disease progression influencing neuronal proteolysis and gut hormones plasma levels. Sci Rep. (2017) 7:1–21. doi: 10.1038/s41598-017-02587-2
133. Kesika P, Suganthy N, Sivamaruthi BS, Chaiyasut C. Role of gut-brain axis, gut microbial composition, and probiotic intervention in Alzheimer’s disease. Life Sci. (2021) 264:118627. doi: 10.1016/J.LFS.2020.118627
134. Chudzik A, Orzyłowska A, Rola R, Stanisz GJ. Probiotics, prebiotics and postbiotics on mitigation of depression symptoms: modulation of the brain–gut–microbiome axis. Biomolecules. (2021) 11:1000. doi: 10.3390/BIOM11071000
135. Cheng LH, Liu YW, Wu CC, Wang S, Tsai YC. Psychobiotics in mental health, neurodegenerative and neurodevelopmental disorders. J Food Drug Anal. (2019) 27:632–48. doi: 10.1016/J.JFDA.2019.01.002
136. Wang Q, Luo Y, Ray Chaudhuri K, Reynolds R, Tan EK, Pettersson S. The role of gut dysbiosis in Parkinson’s disease: mechanistic insights and therapeutic options. Brain. (2021) 144:2571–93. doi: 10.1093/BRAIN/AWAB156
137. Pluta R, Ulamek-Koziol M, Januszewski S, Czuczwar SJ. Gut microbiota and pro/prebiotics in Alzheimer’s disease. Aging. (2020) 12:5539–50. doi: 10.18632/AGING.102930
138. Ghezzi L, Cantoni C, Pinget GV, Zhou Y, Piccio L. Targeting the gut to treat multiple sclerosis. J Clin Invest. (2021) 131:e143774. doi: 10.1172/JCI143774
139. Romanenko M, Kholin V, Koliada A, Vaiserman A. Nutrition, gut microbiota, and alzheimer’s disease. Front Psychiatry. (2021) 12:712673/BIBTEX. doi: 10.3389/fpsyt.2021.712673
140. Gibson GR, Hutkins R, Sanders ME, Prescott SL, Reimer RA, Salminen SJ, et al. Expert consensus document: The International Scientific Association for Probiotics and Prebiotics (ISAPP) consensus statement on the definition and scope of prebiotics. Nat Rev Gastroenterol Hepatol. (2017) 14:491–502. doi: 10.1038/nrgastro.2017.75
141. Louwies T, Johnson AC, Orock A, Yuan T, Greenwood-Van Meerveld B. The microbiota-gut-brain axis: An emerging role for the epigenome. Exp Biol Med (Maywood) (2019) 245:138–45. doi: 10.1177/1535370219891690
142. Balasubramanian R, Schneider E, Gunnigle E, Cotter PD, Cryan JF. Fermented foods: Harnessing their potential to modulate the microbiota-gut-brain axis for mental health. Neurosci Biobehav Rev. (2024) 158:105562. doi: 10.1016/J.NEUBIOREV.2024.105562
143. Perna S, Alalwan TA, Alaali Z, Alnashaba T, Gasparri C, Infantino V, et al. The role of glutamine in the complex interaction between gut microbiota and health: A narrative review. Int J Mol Sci. (2019) 20:5232. doi: 10.3390/IJMS20205232
144. Arribas-López E, Zand N, Ojo O, Snowden MJ, Kochhar T. The effect of amino acids on wound healing: A systematic review and meta-analysis on arginine and glutamine. Nutrients. (2021) 13:2498. doi: 10.3390/NU13082498
145. Khoshbin K, Camilleri M. Effects of dietary components on intestinal permeability in health and disease. Am J Physiol Gastrointest Liver Physiol. (2020) 319:G589–608. doi: 10.1152/ajpgi.00245.2020
146. Kawahara M, Tanaka KI, Kato-Negishi M. Zinc, carnosine, and neurodegenerative diseases. Nutrients. (2018) 10:147. doi: 10.3390/NU10020147
147. Tang W, Liu H, Ooi TC, Rajab NF, Cao H, Sharif R. Zinc carnosine: Frontiers advances of supplement for cancer therapy. Biomed Pharmacother. (2022) 151:113157. doi: 10.1016/J.BIOPHA.2022.113157
148. Efthymakis K, Neri M. The role of Zinc L-Carnosine in the prevention and treatment of gastrointestinal mucosal disease in humans: a review. Clin Res Hepatol Gastroenterol. (2022) 46:101954. doi: 10.1016/J.CLINRE.2022.101954
149. Hewlings S, Kalman D. A review of zinc-L-carnosine and its positive effects on oral mucositis, taste disorders, and gastrointestinal disorders. Nutrients. (2020) 12:665. doi: 10.3390/NU12030665
150. Araya-Quintanilla F, Gutiérrez-Espinoza H, Sánchez-Montoya U, Muñoz-Yañez MJ, Baeza-Vergara A, Petersen-Yanjarí M, et al. Efectividad de la suplementación de ácidos grasos omega-3 en pacientes con enfermedad de Alzheimer: revisión sistemática con metaanálisis. Neurología. (2020) 35:105–14. doi: 10.1016/J.NRL.2017.07.009
151. Sweeney TE, Gaine SP, Michos ED. Eicosapentaenoic acid vs. docosahexaenoic acid for the prevention of cardiovascular disease. Curr Opin Endocrinol Diabetes Obes. (2023) 30:87–93. doi: 10.1097/MED.0000000000000796
152. Hands JM, Anderson ML, Cooperman T, Frame LA. A multi-year rancidity analysis of 72 marine and microalgal oil omega-3 supplements. J Diet Suppl. (2023) 2:195–206. doi: 10.1080/19390211.2023.2252064
153. Chainoglou E, Hadjipavlou-Litina D. Curcumin in health and diseases: alzheimer’s disease and curcumin analogues, derivatives, and hybrids. Int J Mol Sci. (2020) 21:1975. doi: 10.3390/IJMS21061975
154. Shabbir U, Rubab M, Tyagi A, Oh DH. Curcumin and its derivatives as theranostic agents in alzheimer’s disease: the implication of nanotechnology. Int J Mol Sci. (2020) 22:196. doi: 10.3390/IJMS22010196
155. Ege D. Action mechanisms of curcumin in alzheimer’s disease and its brain targeted delivery. Materials. (2021) 14:3332. doi: 10.3390/MA14123332
156. Han HK. The effects of black pepper on the intestinal absorption and hepatic metabolism of drugs. Expert Opin Drug Metab Toxicol. (2011) 7:721–9. doi: 10.1517/17425255.2011.570332
157. Shoba G, Joy D, Joseph T, Majeed M, Rajendran R, Srinivas PSSR. Influence of piperine on the pharmacokinetics of curcumin in animals and human volunteers. Planta Med. (1998) 64:353–6. doi: 10.1055/s-2006-957450
158. Smilkov K, Ackova DG, Cvetkovski A, Ruskovska T, Vidovic B, Atalay M. Piperine: old spice and new nutraceutical? Curr Pharm Des. (2019) 25:1729–39. doi: 10.2174/1381612825666190701150803
159. Moraes DS, Moreira DC, Andrade JMO, Santos SHS. Sirtuins, brain and cognition: A review of resveratrol effects. IBRO Rep. (2020) 9:46–51. doi: 10.1016/J.IBROR.2020.06.004
160. Moussa C, Hebron M, Huang X, Ahn J, Rissman RA, Aisen PS, et al. Resveratrol regulates neuro-inflammation and induces adaptive immunity in Alzheimer’s disease. J Neuroinflamm. (2017) 14:1–10. doi: 10.1186/s12974-016-0779-0
161. Rao YL, Ganaraja B, Joy T, Pai MM, Ullal SD, Murlimanju BV. Neuroprotective effects of resveratrol in Alzheimer’s disease. Front Biosci - Elite. (2020) 12:139–49. doi: 10.2741/E863/PDF
162. Griñán-Ferré C, Bellver-Sanchis A, Izquierdo V, Corpas R, Roig-Soriano J, Chillón M, et al. The pleiotropic neuroprotective effects of resveratrol in cognitive decline and Alzheimer’s disease pathology: From antioxidant to epigenetic therapy. Ageing Res Rev. (2021) 67:101271. doi: 10.1016/J.ARR.2021.101271
163. Arbo BD, André-Miral C, Nasre-Nasser RG, Schimith LE, Santos MG, Costa-Silva D, et al. Resveratrol derivatives as potential treatments for alzheimer’s and parkinson’s disease. Front Aging Neurosci. (2020) 12:103/BIBTEX. doi: 10.3389/fnagi.2020.00103
164. Walter J, Shanahan F. Fecal microbiota-based treatment for recurrent Clostridioides difficile infection. Cell. (2023) 186:1087. doi: 10.1016/j.cell.2023.02.034
165. Wortelboer K, Nieuwdorp M, Herrema H. Fecal microbiota transplantation beyond Clostridioides difficile infections. EBioMedicine. (2019) 44:716–29. doi: 10.1016/J.EBIOM.2019.05.066
166. Live fecal microbiota (Rebyota) for prevention of CDI recurrence. The Medical Letter Inc. Available at: https://secure.medicalletter.org/TML-article-1671b (Accessed December 5, 2023).
167. Jain N, Umar TP, Fahner AF, Gibietis V. Advancing therapeutics for recurrent clostridioides difficile infections: an overview of vowst’s FDA approval and implications. Gut Microbes. (2023) 15:2232137. doi: 10.1080/19490976.2023.2232137
168. Rostagno AA, Ghiso J, Matheson J-AT, Damian Holsinger RM. The role of fecal microbiota transplantation in the treatment of neurodegenerative diseases: A review. Int J Mol Sci. (2023) 24:1001. doi: 10.3390/IJMS24021001
169. Vendrik KEW, Ooijevaar RE, de Jong PRC, Laman JD, van Oosten BW, van Hilten JJ, et al. Fecal microbiota transplantation in neurological disorders. Front Cell Infect Microbiol. (2020) 10:98/BIBTEX. doi: 10.3389/fcimb.2020.00098
170. Kang Y, Kang X, Zhang H, Liu Q, Yang H, Fan W. Gut microbiota and parkinson’s disease: implications for faecal microbiota transplantation therapy. ASN Neuro. (2021) 13:17590914211016217. doi: 10.1177/17590914211016217
171. Jena R, Jain R, Muralidharan S, Yanamala VL, Zubair Z, Kantamaneni K, et al. Role of gastrointestinal dysbiosis and fecal transplantation in parkinson’s disease. Cureus. (2021) 13:e19035. doi: 10.7759/CUREUS.19035
172. Nassar ST, Tasha T, Desai A, Bajgain A, Ali A, Dutta C, et al. Fecal microbiota transplantation role in the treatment of alzheimer’s disease: A systematic review. Cureus. (2022) 14:e29968. doi: 10.7759/CUREUS.29968
173. Porras AM, Brito IL. The internationalization of human microbiome research. Curr Opin Microbiol. (2019) 50:50–5. doi: 10.1016/J.MIB.2019.09.012
174. Rampelli S, Schnorr SL, Consolandi C, Turroni S, Severgnini M, Peano C, et al. Metagenome sequencing of the hadza hunter-gatherer gut microbiota. Curr Biol. (2015) 25:1682–93. doi: 10.1016/j.cub.2015.04.055
175. Shanahan F, Ghosh TS, O’Toole PW. Human microbiome variance is underestimated. Curr Opin Microbiol. (2023) 73:102288. doi: 10.1016/J.MIB.2023.102288
176. Allali I, Abotsi RE, Tow LA, Thabane L, Zar HJ, Mulder NM, et al. Human microbiota research in Africa: a systematic review reveals gaps and priorities for future research. Microbiome. (2021) 9:1–54. doi: 10.1186/S40168-021-01195-7
177. Brewster R, Tamburini FB, Asiimwe E, Oduaran O, Hazelhurst S, Bhatt AS. Surveying gut microbiome research in africans: toward improved diversity and representation. Trends Microbiol. (2019) 27:824–35. doi: 10.1016/j.tim.2019.05.006
178. Magne F, O’Ryan ML, Vidal R, Farfan M. The human gut microbiome of Latin America populations: A landscape to be discovered. Curr Opin Infect Dis. (2016) 29:528–37. doi: 10.1097/QCO.0000000000000300
179. Gounot JS, Chia M, Bertrand D, Saw WY, Ravikrishnan A, Low A, et al. Genome-centric analysis of short and long read metagenomes reveals uncharacterized microbiome diversity in Southeast Asians. Nat Commun. (2022) 13:1–11. doi: 10.1038/s41467-022-33782-z
180. Al Bataineh MT, Dash NR, Lassen PB, Banimfreg BH, Nada AM, Belda E, et al. Revealing links between gut microbiome and its fungal community in Type 2 Diabetes Mellitus among Emirati subjects: A pilot study. Sci Rep 2020 10:1. (2020) 10:1–11. doi: 10.1038/s41598-020-66598-2
181. Moossavi S. The necessity for an Iranian gut microbiome initiative. Middle East J Dig Dis. (2014) 6:109. Available at: https://pubmed.ncbi.nlm.nih.gov/24872871.
182. Maduka O, Akpan G, Maleghemi S. Using android and open data kit technology in data management for research in resource-limited settings in the Niger delta region of Nigeria: cross-sectional household survey. JMIR Mhealth Uhealth. (2017) 5:e171. doi: 10.2196/mhealth.7827
183. Qin J, Li R, Raes J, Arumugam M, Burgdorf KS, Manichanh C, et al. A human gut microbial gene catalogue established by metagenomic sequencing. Nature. (2010) 464:59–65. doi: 10.1038/nature08821
184. Gilbert JA, Jansson JK, Knight R. The Earth Microbiome project: Successes and aspirations. BMC Biol. (2014) 12:1–4. doi: 10.1186/s12915-014-0069-1
185. Gilbert JA, Quinn RA, Debelius J, Xu ZZ, Morton J, Garg N, et al. Microbiome-wide association studies link dynamic microbial consortia to disease. Nature. (2016) 535:94–103. doi: 10.1038/nature18850
186. Segata N, Waldron L, Ballarini A, Narasimhan V, Jousson O, Huttenhower C. Metagenomic microbial community profiling using unique clade-specific marker genes. Nat Methods. (2012) 9:811–4. doi: 10.1038/nmeth.2066
187. Wallerstein N, Duran B. Community-based participatory research contributions to intervention research: The intersection of science and practice to improve health equity. Am J Public Health. (2010) 100:S40–6. doi: 10.2105/AJPH.2009.184036
188. Shalowitz MU, Isacco A, Barquin N, Clark-Kauffman E, Delger P, Nelson D, et al. Community-based participatory research: A review of the literature with strategies for community engagement. J Dev Behav Pediatr. (2009) 30:350–61. doi: 10.1097/DBP.0B013E3181B0EF14
189. Stulberg E, Fravel D, Proctor LM, Murray DM, LoTempio J, Chrisey L, et al. An assessment of US microbiome research. Nat Microbiol. (2016) 1:1–7. doi: 10.1038/nmicrobiol.2015.15
Keywords: human gastrointestinal microbiome, gut-brain axis, neuroimmunomodulation, enteric nervous system, neurogenic inflammation, neurodegenerative diseases, neuropathology, neuroinflammatory disease
Citation: Warren A, Nyavor Y, Zarabian N, Mahoney A and Frame LA (2024) The microbiota-gut-brain-immune interface in the pathogenesis of neuroinflammatory diseases: a narrative review of the emerging literature. Front. Immunol. 15:1365673. doi: 10.3389/fimmu.2024.1365673
Received: 04 January 2024; Accepted: 29 April 2024;
Published: 16 May 2024.
Edited by:
Servaas Antonie Morré, VU Medical Center, NetherlandsReviewed by:
Claudio Nicoletti, University of Siena, ItalyPayam Behzadi, Islamic Azad University, Iran
Kenji Hashimoto, Chiba University, Japan
Panida Sittipo, Burapha University, Thailand
Copyright © 2024 Warren, Nyavor, Zarabian, Mahoney and Frame. This is an open-access article distributed under the terms of the Creative Commons Attribution License (CC BY). The use, distribution or reproduction in other forums is permitted, provided the original author(s) and the copyright owner(s) are credited and that the original publication in this journal is cited, in accordance with accepted academic practice. No use, distribution or reproduction is permitted which does not comply with these terms.
*Correspondence: Leigh A. Frame, leighframe@gwu.edu
†These authors have contributed equally to this work