- 1MARE-Marine and Environmental Sciences Centre, Agência Regional para o Desenvolvimento da Investigação, Tecnologia e Inovação (ARDITI), Funchal, Portugal
- 2GEOMAR, Marine Ecology Department, Helmholtz Centre for Ocean Research Kiel, Kiel, Germany
- 3MARE-Marine and Environmental Sciences Centre, Faculdade de Ciências da Universidade de Lisboa, Lisbon, Portugal
- 4Smithsonian Environmental Research Center, Edgewater, MD, United States
Macroalgal forests play a key role in shallow temperate rocky reefs worldwide, supporting communities with high productivity and providing several ecosystem services. Sea urchin grazing has been increasingly influencing spatial and temporal variation in algae distributions and it has become the main cause for the loss of these habitats in many coastal areas, causing a phase shift from macroalgae habitats to barren grounds. The low productive barrens often establish as alternative stable states and only a major reduction in sea urchin density can trigger the recovery of macroalgal forests. The present study aims to assess if the 2018 disease outbreak, responsible for a strong reduction in the sea urchin Diadema africanum densities in Madeira Island, was able to trigger a reverse shift from barren grounds into macroalgae-dominated state. By assessing the diversity and abundance of benthic sessile organisms, macroinvertebrates and fishes before, during and after that particular mass mortality event, we evaluate changes in benthic assemblages and relate them to variations in grazer and herbivore densities. Our results revealed a clear shift from barren state to a macroalgae habitat, with barrens characterized by bare substrate, sessile invertebrate and Crustose Coralline Algae (CCA) disappearing after the mortality event. Overall variations in benthic assemblages was best explained by four taxa (among grazers and herbivores species). However, it was the 2018 demise of D. africanum and its density reduction that most contributed to the reverse shift from a long stable barren state to a richer benthic assemblage with higher abundance of macroalgae. Despite this recent increase in macroalgae dominated habitats, their stability and persistence in Madeira Island is fragile, since it was triggered by an unpredictable disease outbreak and depends on how D. africanum populations will recover. With no control mechanisms, local urchin populations can easily reach the tipping point needed to promote a new shift into barren states. New conservation measures and active restoration are likely required to maintain and promote the local stability of macroalgal forests.
Introduction
Coastal habitats represent one of the most productive and valued ecosystems of our planet (Costanza et al., 1997) but they are also seriously threatened by multiple factors and compounding pressures such as habitat loss and degradation, pollution, overexploitation, species introductions and climate change (see Solan and Whiteley, 2016 and references therein). Shallow marine coastal habitats are often dominated by macroalgae, where canopy and erect algae forests support biodiverse communities with high productivity (Graham, 2004; Ling, 2008; Christie et al., 2009; Tamaki et al., 2009). Macroalgae communities play a central role in the shallow temperate benthic ecosystems, representing the primary producers in the coastal zone as an important source of carbon sequestration (Krause-Jensen and Duarte, 2016) and providing valuable ecosystem services in shallow coastal systems (Muguerza et al., 2017; Ware et al., 2019; Edwards et al., 2020). In macroalgal forests, local herbivore populations are often able to drive fluctuations in spatial and temporal distribution of algal communities (Stachowicz et al., 2007; Schmitz, 2008; Owen-Smith, 2014), determine algal abundance and species composition (Van Alstyne, 1989), influence algal growth rates and reproductive output (Shurin et al., 2002; Steneck et al., 2002). For this reason, major increases in local populations of key grazers and herbivores can lead to the replacement of productive macroalgae forests with impoverished barrens dominated by encrusting organisms (Pinna et al., 2020 and references therein). These phase-shifts often occur with variation in sea urchin grazing intensity (Melis et al., 2019) as their feeding activity can play a major role in the stability, biodiversity, production and functioning of these ecosystems (Duffy and Hay, 1990; Poore et al., 2009; Korpinen et al., 2010; Kraufvelin, 2017). Sea urchins can thrive at very high population densities (Lawrence, 1975; Alves et al., 2001; Gizzi et al., 2020), possess limited mobility and a powerful excavating mouth providing them the ability to regulate the distribution, abundance, and diversity of benthic marine algae communities, influencing the establishment, spread and persistence of algal species (e.g., Steneck, 2013; Filbee-Dexter and Scheibling, 2014; Friedlander et al., 2017; Melis et al., 2019). Numerous studies have unequivocally demonstrated an inverse relationship between urchin density and algal biomass as well as their role in promoting a shift of habitats with complex macroalgae forests into urchin barrens (e.g., Filbee-Dexter and Scheibling, 2014; Ling et al., 2015; Hernández, 2017; Melis et al., 2019; Pinna et al., 2020).
With several natural factors influencing the intensity of urchin grazing (e.g., population density, food availability, and predation pressure), anthropogenic pressures such as overfishing (and consequent reduction in urchin predators) and global warming (and consequent changes in recruitment, physiology and distribution) can favor the proliferation of sea urchins and the spread of barren grounds worldwide (Filbee-Dexter and Scheibling, 2014; Ling et al., 2015; Melis et al., 2019; Pinna et al., 2020). The geographically widespread increase of barren grounds (Feehan et al., 2012; Filbee-Dexter and Scheibling, 2014; Perreault et al., 2014) and the persistence of this alternate state in some areas where the causes for sea urchin proliferation have been removed, give cause for concern (see Filbee-Dexter and Scheibling, 2014 and references therein). The occurrence of hysteresis mechanisms promote a self-perpetuating positive feedback that maintain the barrens even if the initial conditions are restored, reducing habitat complexity and ecosystem services (Scheffer et al., 2001; Filbee-Dexter and Scheibling, 2014). This loss in complexity and services leads to a crucial need in better understanding the mechanisms involved in forward and reverse shifts between algae dominated and barren states. Previous studies have consistently demonstrated that the threshold (i.e., urchin density or biomass) for a forward shift into barren state to occur is much higher than that of a reverse shift (Filbee-Dexter and Wernberg, 2018; Guarnieri et al., 2020). This non-linearity in triggering thresholds implies that once the barren ground is formed, a major reduction in urchin density is required for a reversal of a barren state into an erect algae dominated one (Ling et al., 2010; Trowbridge et al., 2011; Piazzi and Ceccherelli, 2019; Guarnieri et al., 2020). In some circumstances, multiple phase shifts between macroalgae forests and urchin barrens can go back and forward, driven by various factors such as fisheries targeting urchins or their predators, storm events, temperature increases or urchin diseases (Filbee-Dexter and Scheibling, 2014 and references therein). In contrast, they have consistently required a major decrease in urchin populations to achieve a tipping point and reverse into an algae-dominated state (Scheibling et al., 2013). This non-linear nature of phase shifts can be particularly relevant in coastal marine habitats that are especially vulnerable or under pressure from other stressors such as coastal development, pollution and overfishing. Forward shifts can be easily triggered whereas state reversal toward habitats with higher complexity and diversity may be hampered and barrens may become alternative stable states (Claisse et al., 2013; Filbee-Dexter and Scheibling, 2014; Cabanillas-Terán et al., 2015; Ling et al., 2015).
In the northeastern Atlantic archipelagos of Madeira and Canary Islands (Macaronesian region), populations of the long-spined sea urchin Diadema africanum with average densities of 10 individuals/m2 have been responsible for the persistence of vast urchin barrens (Alves et al., 2001; Hernández et al., 2013). Several ecological and physical processes are involved in the structure and abundance of local D. africanum populations, including water turbulence (Alves et al., 2001), environmental parameters (e.g., nutrient and temperature; Hernández et al., 2006a, b), abundance of top-predators (Tuya et al., 2004b), substrate complexity (Tuya et al., 2004a) and mass mortality events (Clemente et al., 2014; Gizzi et al., 2020), but evidence suggests a general stability in the barren state of shallow coastal habitats across multiple islands of these archipelagos.
In Madeira Island, shallow rocky reef bottoms appear to have been the stage for a phase shift from algae dominated bottoms (Augier, 1985) toward D. africanum barrens (Alves et al., 2001; Hernández et al., 2008b). In 2009, a D. africanum mass-mortality event was first registered off the coasts of Madeira (Clemente et al., 2014) and resulted in a reduction of 65% of sea urchins abundance (Hernández et al., 2013; Clemente et al., 2014). At that time, the lack of long term monitoring and historical data series have hampered dedicated studies on how it may have triggered an increase of algae cover and a phase shift from barren to algae dominated bottoms during the following months. In the summer of 2018 (early August, late September), a second mass mortality event was recorded in Madeira, with a major drop of approximately 90% in population density (described in detail in Gizzi et al., 2020). A disease outbreak that had been initially detected in the Canary Islands during February 2018 (Hernández et al., 2020), took more than 4 months to reach Madeira (where mortality was first detected in late July) but disrupted the local population of D. africanum across the entire archipelago.
Like many oceanic islands, Madeira Island is particularly vulnerable due to its offshore location, high level of endemisms and limited spatial extent suitable for macroalgal forests (Nunn et al., 1999; Mata et al., 2013). For this reason, it is of crucial importance that efforts are made to restore the macroalgal forests once present in the archipelago and thus protect local marine biodiversity.
There is typically a strong inverse correlation between D. africanum urchin density and erect algae abundance (Alves et al., 2001; Hernández et al., 2008b) due to the grazing pressure associated with urchin populations (Brito et al., 2004; Tuya et al., 2004a; Hernández et al., 2008b). The 2018 disease outbreak and urchin mass-mortality (Gizzi et al., 2020) provided an unique opportunity to leverage census data from annual monitoring surveys along the south coast of Madeira Island (2017–2019) to assess if D. africanum mortality had reduced grazing pressure for long enough to allow an increase in algal cover.
In this context, the present study aims to assess whether the 2018 abrupt reduction in D. africanum densities (Gizzi et al., 2020) triggered areas that were previously barren to transform into algae dominated areas by correlating urchin densities with the occurrence of distinct benthic assemblages. Additionally we discuss the role that periodic natural events and disease outbreaks may have in controlling D. africanum populations in Madeira and allow reverse phase-shifts and recovery of macro-algae communities. Finally, we suggest active conservation and habitat restoration practices that may apply toward preserving macroalgal forests in the region.
Materials and Methods
Study Area
Madeira is a Portuguese archipelago located in the warm-temperate waters of the Northeast Atlantic Ocean within the Macaronesia area, together with the Azores, the Canary Islands and Cabo Verde, and is located about 650 km west of Morocco at its closest point to the mainland (Figure 1). The archipelago consists of two main populated islands, Madeira and Porto Santo, and five uninhabited islands (Desertas and Selvagens Islands). This study was conducted in the main island of Madeira, where the coastline and shallow waters (0–12 m) are dominated by rocky shores and reefs (Canning-Clode et al., 2008; Schäfer et al., 2020). The archipelago is influenced by north currents (Portuguese, Azorean and Canary currents; Caldeira et al., 2002), resulting in oligotrophic waters with high salinity, high temperature and low-nutrient regime waters (Johnson and Stevens, 2000; New et al., 2001). In the summers of 2017, 2018, and 2019, benthos and associated motile fauna were monitored in 10 sites along the south coast of the island (Figure 1). Sites were selected to include surveys along the entire south coast; whereas no sites were selected along the north coast due to difficulties in systematically access these sites due to strong prevailing winds and swells from the north quadrant, especially in the early summer months (Caldeira et al., 2002). Surveys targeted shallow rocky bottoms from the extreme western tip (Ponta do Pargo), to the easternmost part of the island (Quinta do Lorde), covering a range of different constraints (e.g., coastal development, population density, fishing, coastal runoff). With the exception of Ponta do Pargo, most sites are fairly protected from the prevailing north winds and currents with no major differences in salinity or temperature regimes (Caldeira et al., 2002).
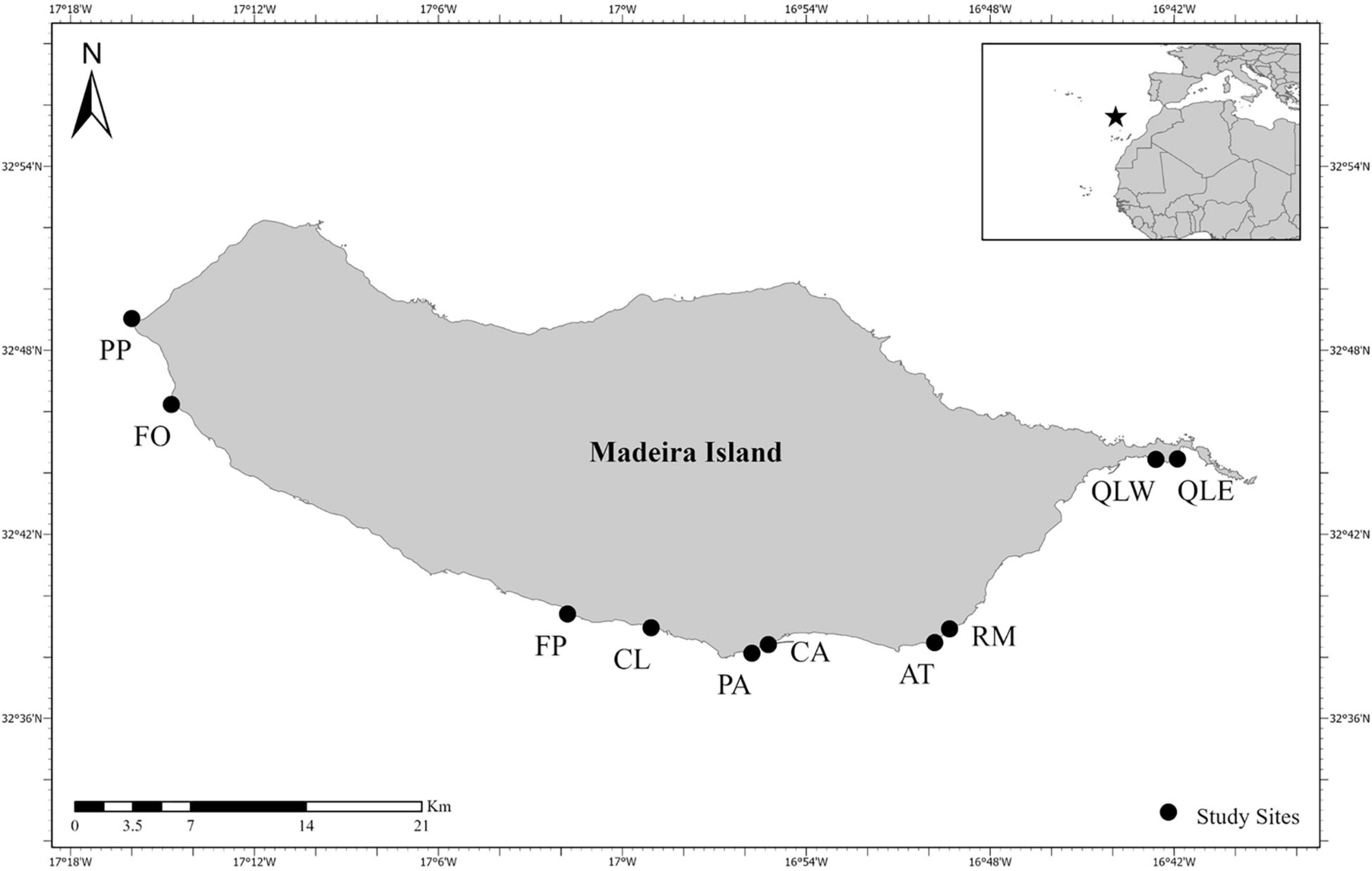
Figure 1. Study area (Madeira Island, Portugal) and study sites represented by dots. PP, Ponta do Pargo; FO, Fajã da Ovelha; FP, Fajã dos Padres; CL, Câmara de Lobos; PA, Palms; CA, Carlton; AT, Atalaia; RM, Reis Magos; QLW, Quinta do Lorde West; QLE, Quinta do Lorde East.
Benthic Community Distributions and Associated Motile Fauna
Changes in benthic communities subsequent to the 2018 D. africanum mortality event (Gizzi et al., 2020) were assessed by: (i) identifying existing distinct assemblages; (ii) inspecting their occurrence in each summer (2017, 2018, and 2019), and (iii) assessing the influence of D. africanum density reduction in the occurrence of each unique assemblage.
Data on the abundance and density of sessile organisms, fish and macroinvertebrates were compiled from Underwater Visual Census (UVC) yearly surveys, performed by scientific divers along the south coast of Madeira Island (Figure 1). For each study site three transects were performed at 9–11 m depth. Benthic sessile diversity and relative abundance was assessed using 10 m point-intersect transects with 100 points (10 cm each), whereas fish and conspicuous macro-invertebrates diversity and abundance were recorded by counting individuals along a 50 × 4 m transect. Both the benthos and motile transects had the same starting point, georeferenced with a GPS equipped buoy, and with perpendicular orientation to the depth gradient.
During benthic surveys, sessile organisms under each intersection point were identified in situ to the highest taxonomic resolution possible and macro-photographs were collected when identification was uncertain or unknown. Imagery was later inspected to resolve uncertainties. All point-intersect data were grouped into nine categories, adapted from Friedlander et al. (2017, 2018): Crustose Coralline Algae (CCA), encrusting algae (non-CCA), filamentous microalgae (i.e., diatoms and cyanobacteria), turf algae (<3 cm in height), erect algae, canopy algae, sessile invertebrates, motile invertebrates and bare substrate (Supplementary Table 1).
Motile organisms abundance data were collected by two divers counting all fishes from 0 to 2 m above the rocky reef and all conspicuous macroinvertebrates (including D. africanum individuals) exposed over rocky reefs and in holes and crevices. Identified taxa were later grouped into the following five functional groups (based on their feeding guild): grazer, herbivore, omnivore, invertivore and macro carnivore. For subsequent analysis, motile data were pruned to select herbivores and grazers (as these are the organisms that can directly affect macro-algae abundance and promote phase shifts; e.g., Filbee-Dexter and Scheibling, 2014; Filbee-Dexter and Wernberg, 2018; Supplementary Table 2). Finally, all sessile and motile data and metadata were compiled and labeled for analysis.
Statistical Analysis
To identify and assess changes in the occurrence of significantly different sessile assemblages, the relative abundance of major sessile functional groups in each transect (see above) was standardized (to exclude all remaining uncertainties) and square-rooted (to minimize weight of dominant taxa in similarity based analysis). A Bray-Curtis similarity matrix was constructed and a cluster analysis with a Similarity Profile (SIMPROF) routine with 9999 permutations was used to visualize (dis)similarity in benthic structure between transects and identify significantly different groups of samples based solely on taxa group composition and data ordination (Clarke et al., 2008). SIMPROF generated groups (SFG) composition and occurrence over the 3 year period was inspected by plotting a shade plot, while a one-way Analysis of Similarity (ANOSIM) was used to test differences between years and confirm significant differences occurred after the 2018 D. africanum mortality event. The contributions of sessile taxonomic categories to the SIMPROF generated grouping were assessed with a Similarity Percentage routine (SIMPER), illustrating the typical composition of each assemblage.
Having established significant differences in sessile data over the 3 year period, a Distance-based Linear Model (DistLM) with the Akaike information criterion (AICc) was constructed to establish if and which herbivores and grazers were most contributing to the shape of the sessile assemblages identified with SIMPROF. Density data on all herbivore and grazer taxa was included as trial predictors, selecting BEST procedure to generate the top 10 best solutions to explain sessile data ordination. Lastly, Principal Coordinates Ordination (PCO) and non-metric Multi-Dimensional Scaling (nMDS) plots of the sessile Bray-Curtis similarity matrix were constructed with vector and bubble overlays to illustrate how variations in DistLM selected motile taxa related to the sessile functional groups ordination and grouping. Data analyses were performed and figures generated in Primer v7 (Clarke and Gorley, 2015) with PERMANOVA + add-on (Anderson et al., 2008).
Results
Inspection of the abundance of sessile functional groups (Figure 2) occurring in the summers of 2017, 2018, and 2019 suggests a shift from a barren state to a macroalgae habitat, with a general decrease in relative abundance of bare substrate, sessile invertebrates and CCA and an increase in turf, erect and canopy forming algae (Figure 2). The general decreasing trend in the abundance of benthos categories and sessile functional groups that are typically dominant in barren states, were accompanied by a yearly decrease in the density of individuals from grazer taxa (Figure 3). Based on a hierarchical cluster analysis of Bray-Curtis similarity matrix with SIMPROF routine, three significantly different groups of sessile organisms were identified (Figure 4 and Supplementary Table 3): a typical barren state assemblage (SFG = a), an intermediate state with no clear dominance of any functional groups (SFG = b) and a macroalgae dominated assemblage (SFG = c). The identification of these three distinct assemblages is independent of any factor or pre-deterministic grouping (e.g., year, site or location), as it is generated by permutation and profiling Bray-Curtis similarity among samples (Clarke et al., 2008, 2014). While samples with typical barren state assemblage, where bare substrate is more common and CCA, sessile invertebrates and filamentous microalgae dominated living cover (SFG = a) occurred in 2017 and 2018 (n = 15 and n = 8, respectively), 2019 was characterized by the absence of this assemblage (Figure 4). Remaining assemblages characterized by higher algal cover (SFG = b and c) were identified in samples from all years. However, with the disappearance of barren grounds (SFG = a), they become increasingly more common over time (Figure 4). This post-mortality change in the frequency and occurrence of the three identified assemblages was further assessed by testing years as a factor in a one-way ANOSIM. The only significant differences in sessile functional groups’ ordination were those between 2019 and remaining years (Table 1), supporting the hypothesis that D. africanum density reduction in 2018 has enabled a reverse phase shift.
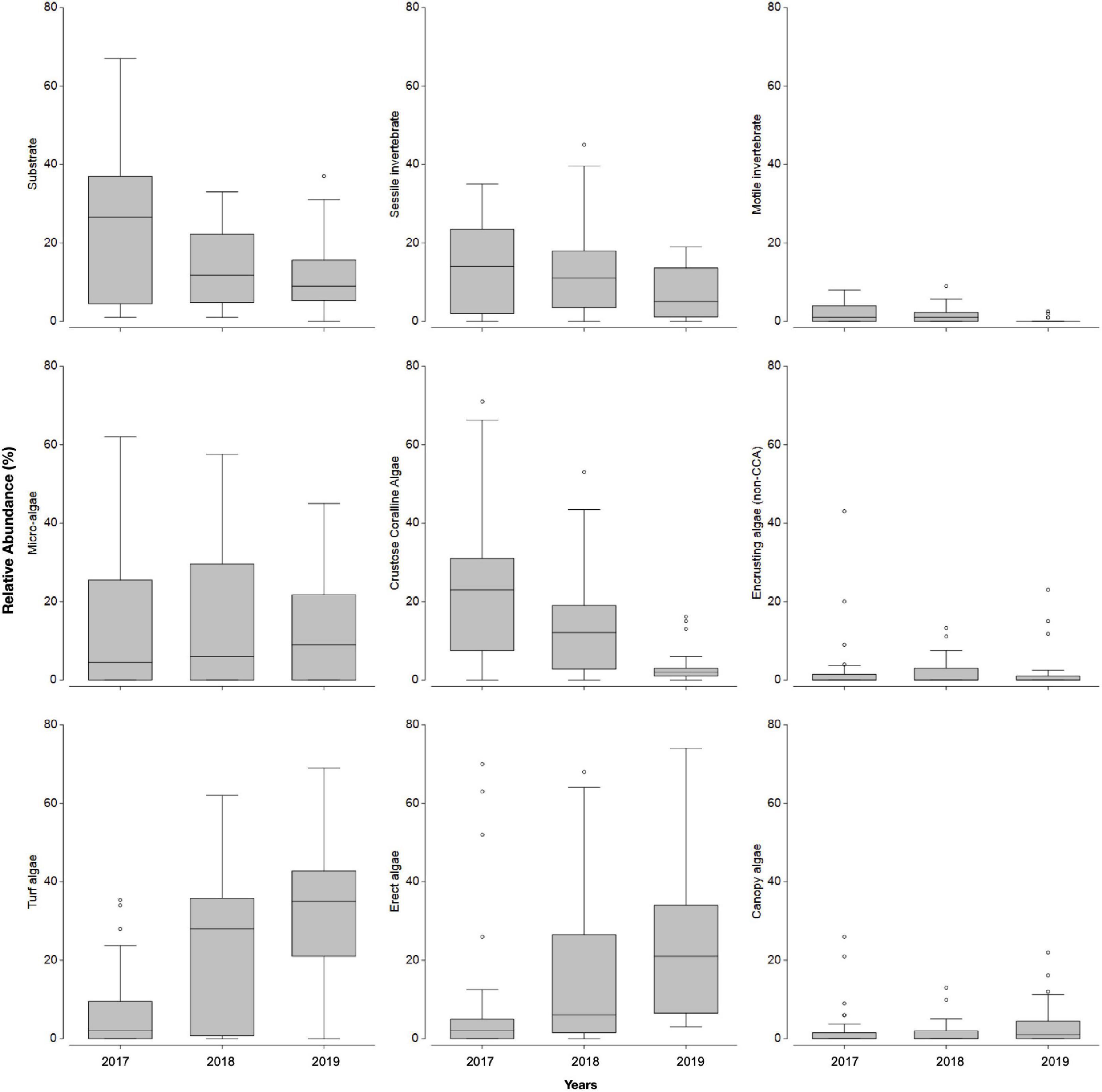
Figure 2. Sessile functional group average relative abundance (%) in each of the three considered years (2017, 2018, 2019); boxplot with first quartile, median and third quartile, minimum and maximum as whiskers and points as outliers.
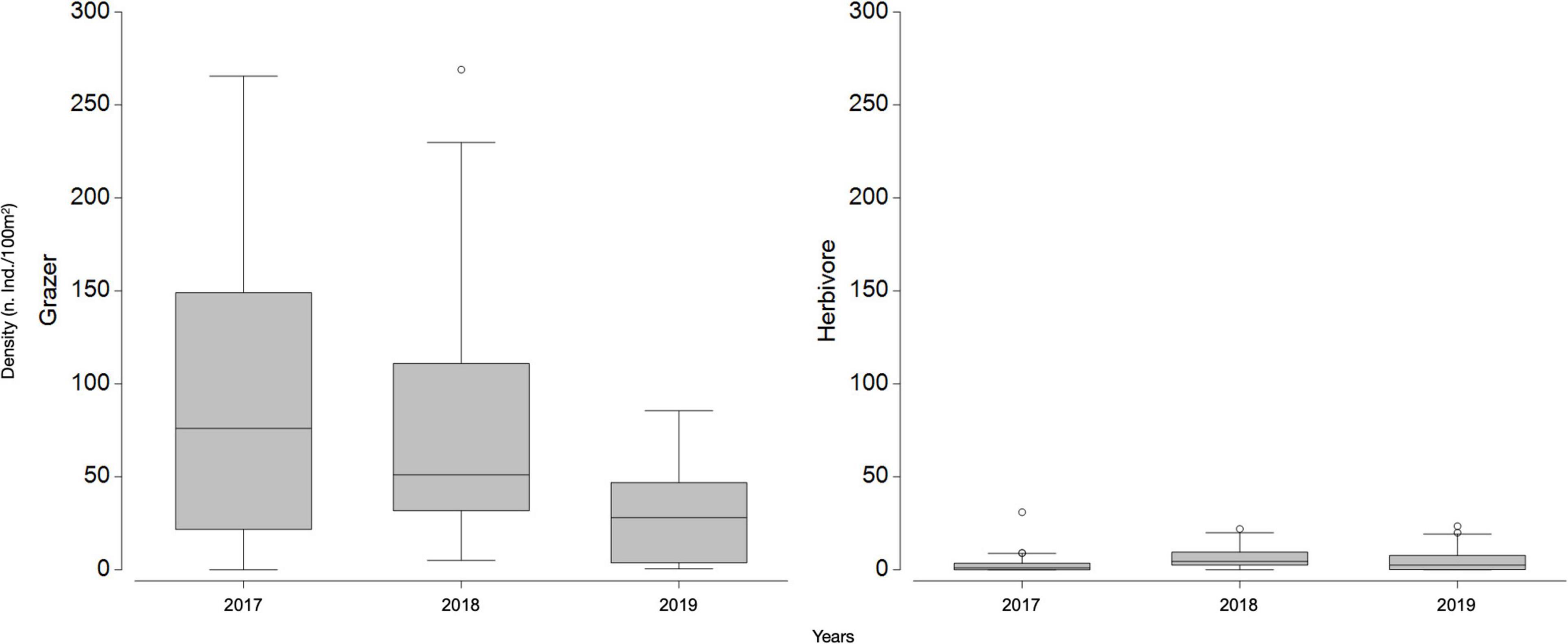
Figure 3. Grazer and herbivore densities (individuals/100 m2) in each of the three considered years (2017, 2018, 2019); boxplot with first quartile, median and third quartile, minimum and maximum as whiskers and points as outliers.
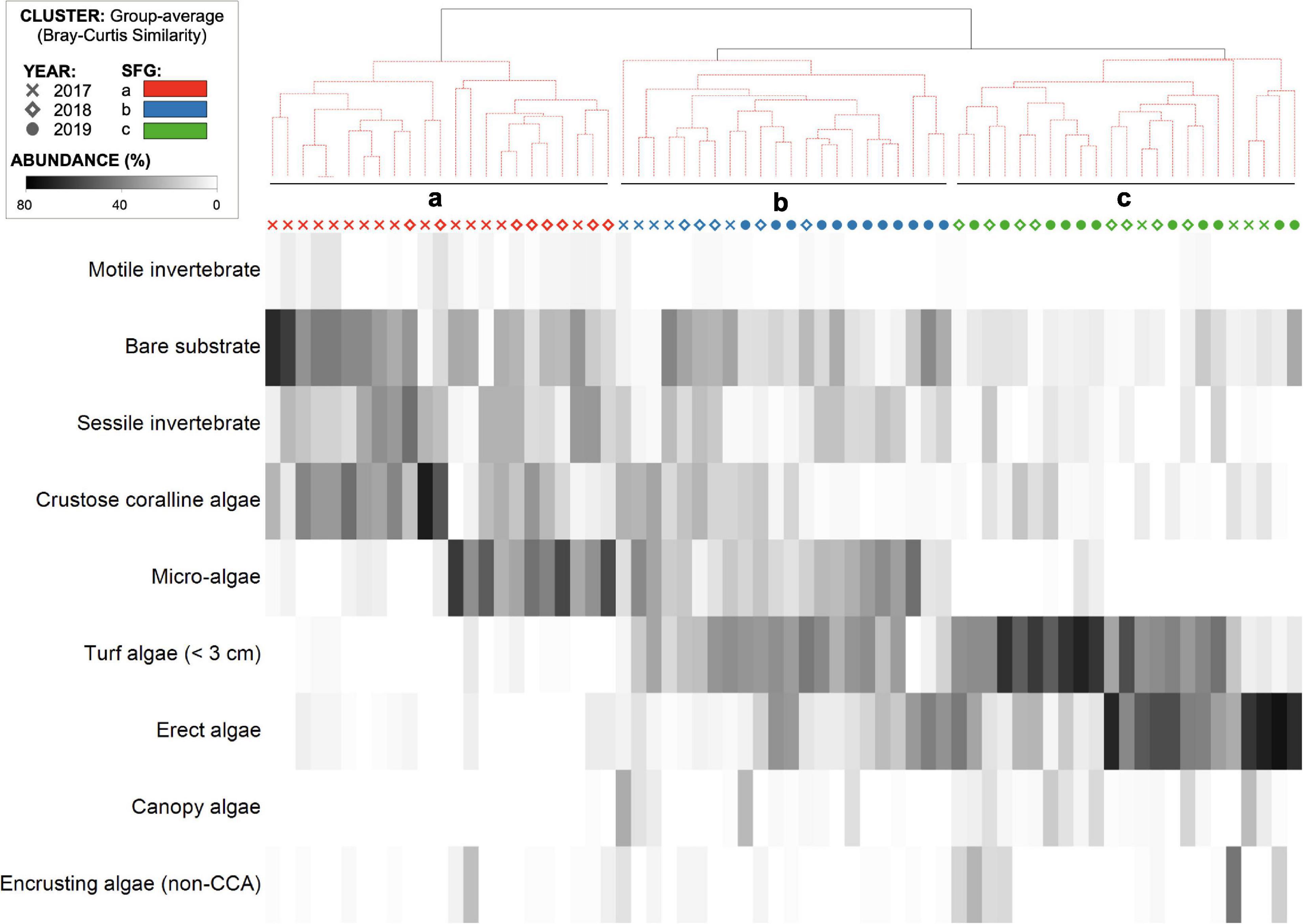
Figure 4. Hierarchical cluster analysis (top) of Bray-Curtis similarities in benthic transect samples for each of the three considered years (2017, 2018, 2019; shapes) with SIMPROF-routine generated groups (SFG = a, b, and c; colors) and sessile functional groups relative density (individuals/100 m2; shade plot).
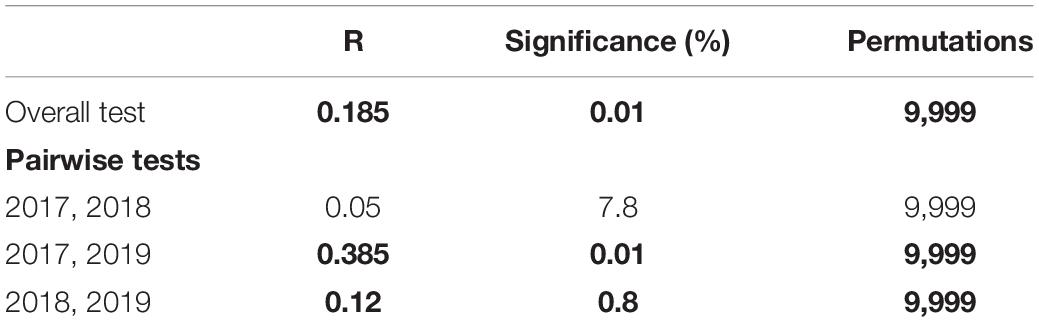
Table 1. One-way ANOSIM of year as a significant factor in the ordination of Bray-Curtis similarities between benthic transect samples (n = 68) with pairwise tests (significant differences in bold).
A Distance-based Linear Model, using herbivore and grazer taxa densities as possible predictors, supported that the reduction in D. africanum density following the 2018 mass mortality event had a major role in the absence of typical urchin barren state in 2019 (Tables 2, 3). The model that best explained the variation in benthic assemblages included four taxa: two grazer sea urchins D. africanum and Paracentrotus lividus, one herbivore crab Percnon gibbesi and the grazer fish Ophioblennius atlanticus. The four-axis model explained over 41% of the variability in sessile assemblages and benthic sample ordination (Table 4) with axis 1 being responsible for more than 88% of the fitted model. With D. africanum density variation having stronger correlation with axis 1 than any of the other taxa, it has a greater weight in explaining sessile sample ordination. This greater influence and stronger correlation of D. africanum densities, and sessile assemblages and sample ordination can be further illustrated by inspecting a Principal Coordinate Ordination plot with vector overlays displaying correlations with the four selected grazer and herbivore taxa (Figure 5). Beside the stronger correlation, fluctuations and variation range of D. africanum density was also considerably greater than those observed in the remaining three taxa (Supplementary Figure 1).
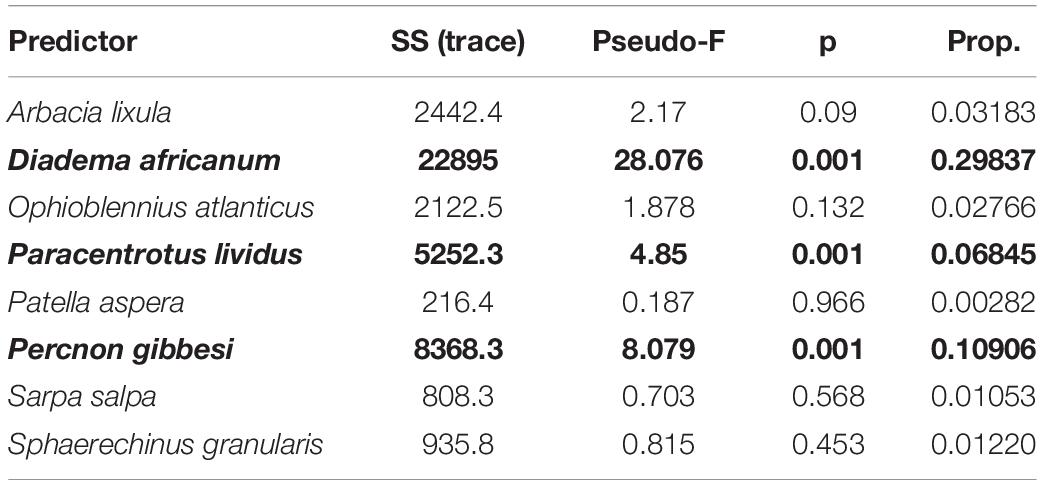
Table 2. Marginal tests for distance-based linear modeling to predict Bray-Curtis similarities between benthic transect samples (n = 68) with herbivore and grazer taxa densities as predictors (significant predictors in bold; SS, Sum of Squares; Prop., proportion).
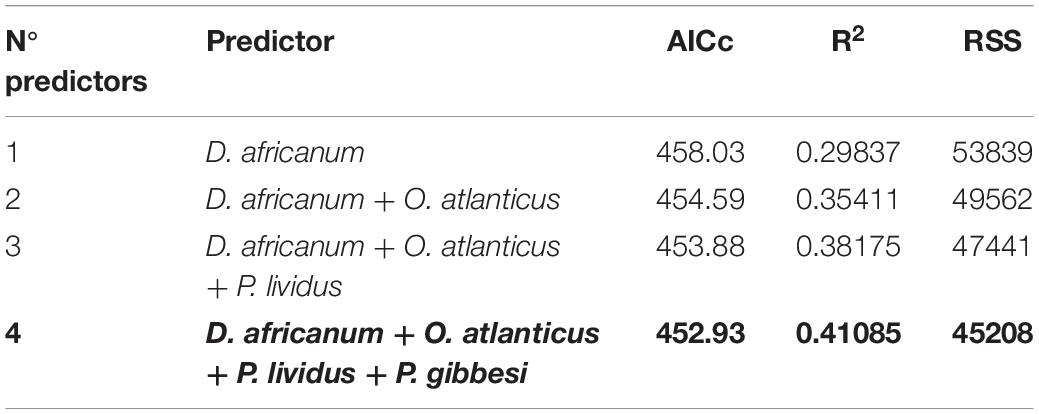
Table 3. DistLM top solutions predicting Bray-Curtis similarities between benthic transect samples (n = 68) with herbivore and grazer taxa densities as predictors (overall best solution in bold; AICc, Akaike information criterion; RSS, R Sum of Squares).
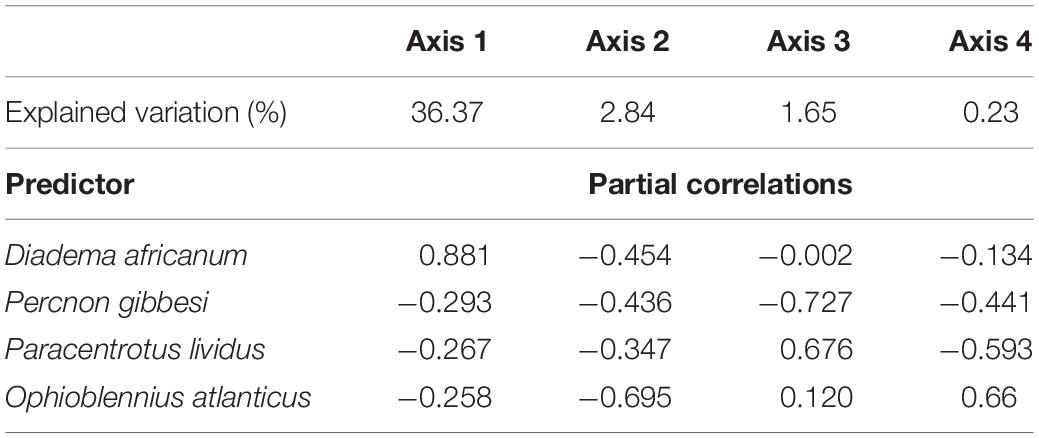
Table 4. Variation in benthic transect explained by each coordinate axis of the best DistLM solution (%) and correlations with orthonormal variables (predictor taxa).
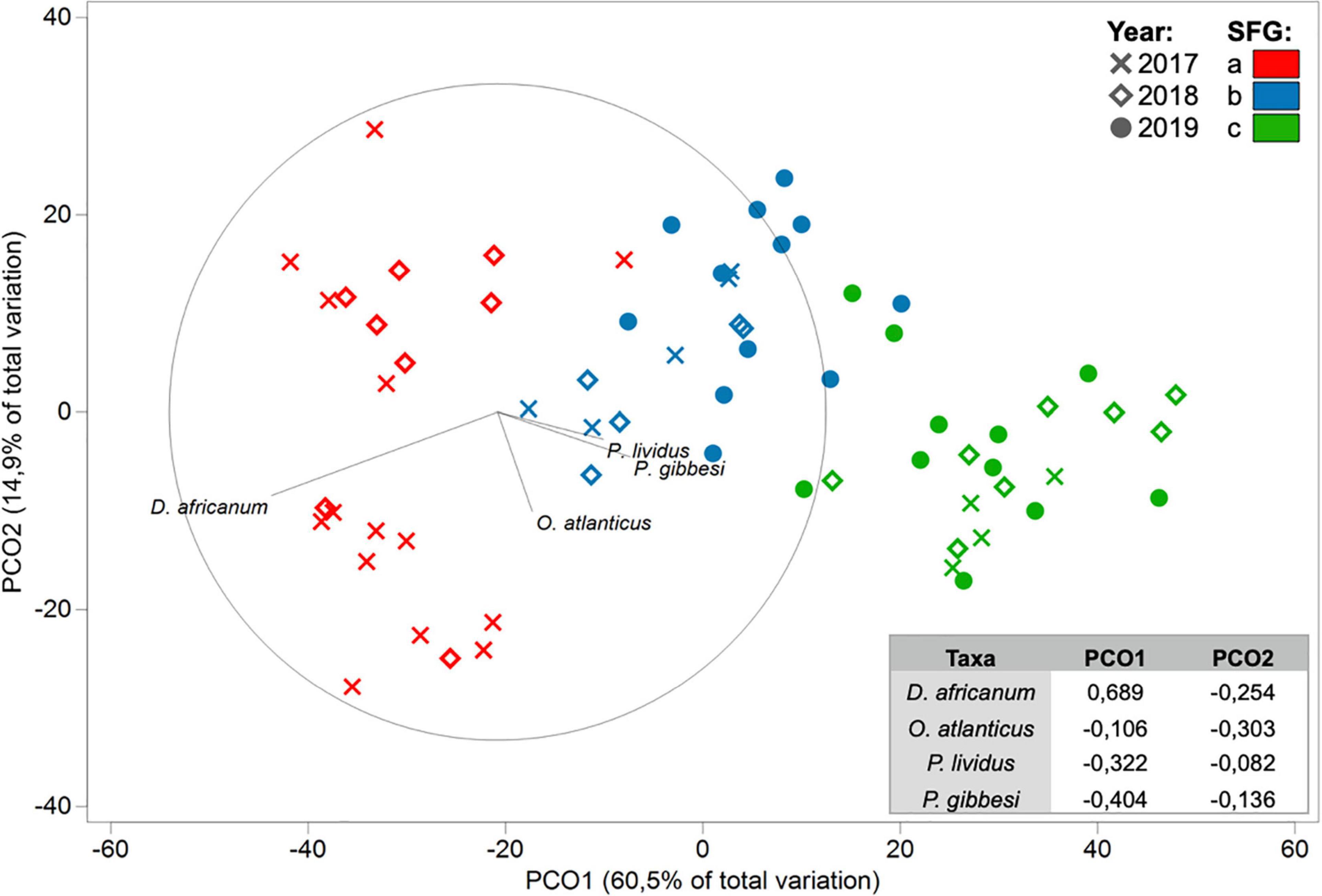
Figure 5. Principal Coordinate Ordination (PCO) plot of benthic transect samples for each of the three considered years (2017, 2018, 2019; shapes) with SIMPROF-routine generated Functional Groups (SFG = a, b, and c; colors) and correlations between the four DistLM selected taxa (vectors) and PCO axes (bottom-right table).
The distribution of identified sessile assemblages (SFG = a, b, c) during annual surveys (Figure 6) also illustrates a clear reverse shift from barren state (SFG = a) to intermediate or algae dominated state (SFG = b, c) in sites where it had been previously identified. Similarly, some locations where an intermediate state (SFG = b) occurred in 2017 and 2018 shifted to an algae dominated state (SFG = c) in the following year. An inspection of matching D. africanum densities further supports the role of the urchin grazing pressure in the shaping of benthic assemblages (Figure 6). In addition, despite spatial variation in urchin densities, the 2018 disease outbreak had a clear impact in D. africanum population along the entire south coast of the island, confirming the severity and extent of the mortality event described in Gizzi et al. (2020).
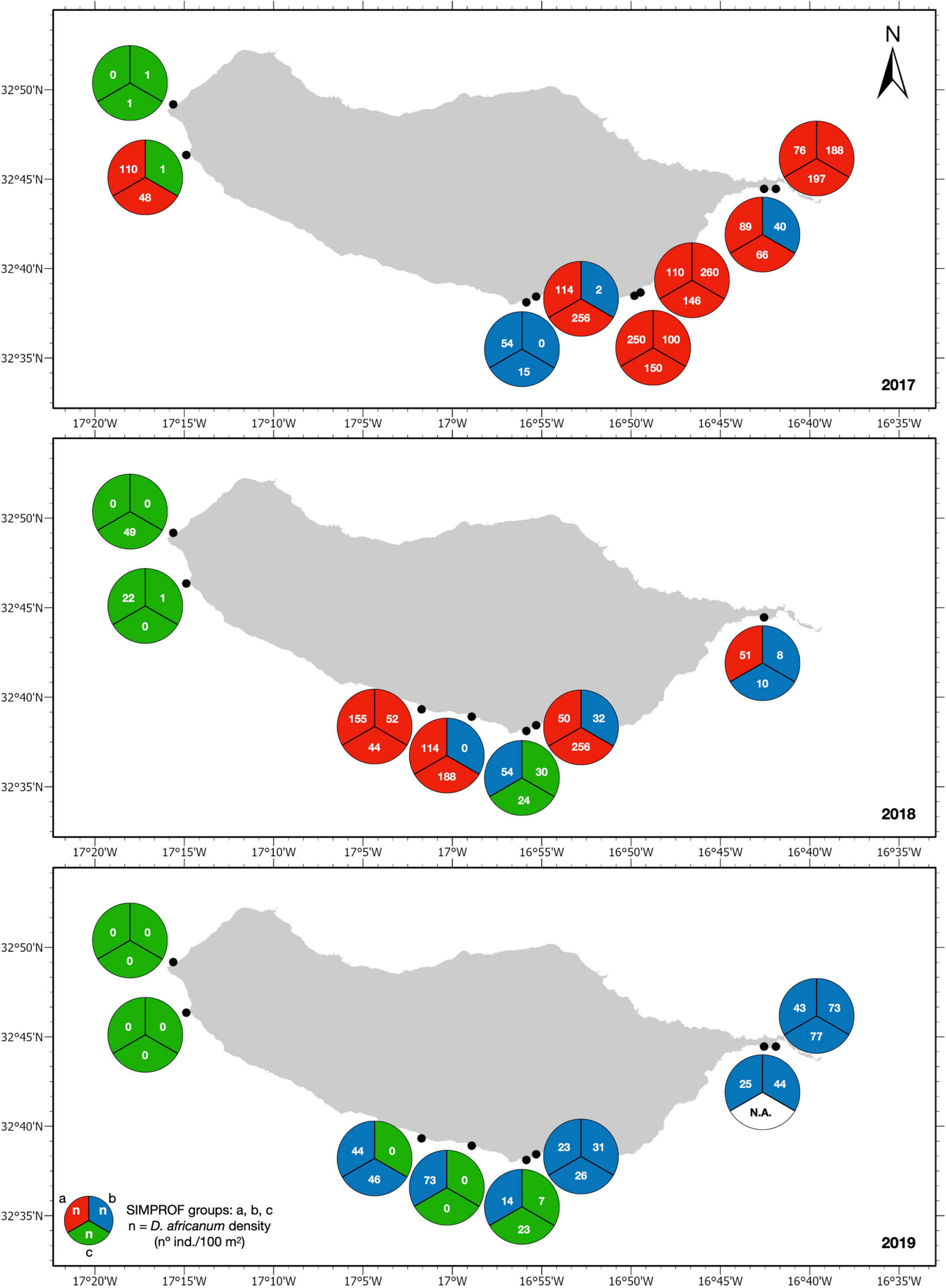
Figure 6. Identified assemblages (SFG = a, b, c; colors) and D. africanum density (individuals/100 m2) in each transect (pie chart slices) for sites surveyed in 2017 (top), 2018 (middle), and 2019 (bottom).
By inspecting D. africanum densities found in each assemblage (Figures 6, 7) it is also clear that the urchin density was considerably higher in barren areas (SFG = a) where values are typically above 50 individuals per 100 m2. Opposingly, intermediate and algae dominated assemblage (SFG = b and c) were typically below this value (Figures 6, 7), suggesting that 50 ind./100 m2 can be empirically perceived as the threshold (i.e., maximum density) to reduce grazing pressure and trigger reverse phase shift from a barren state.
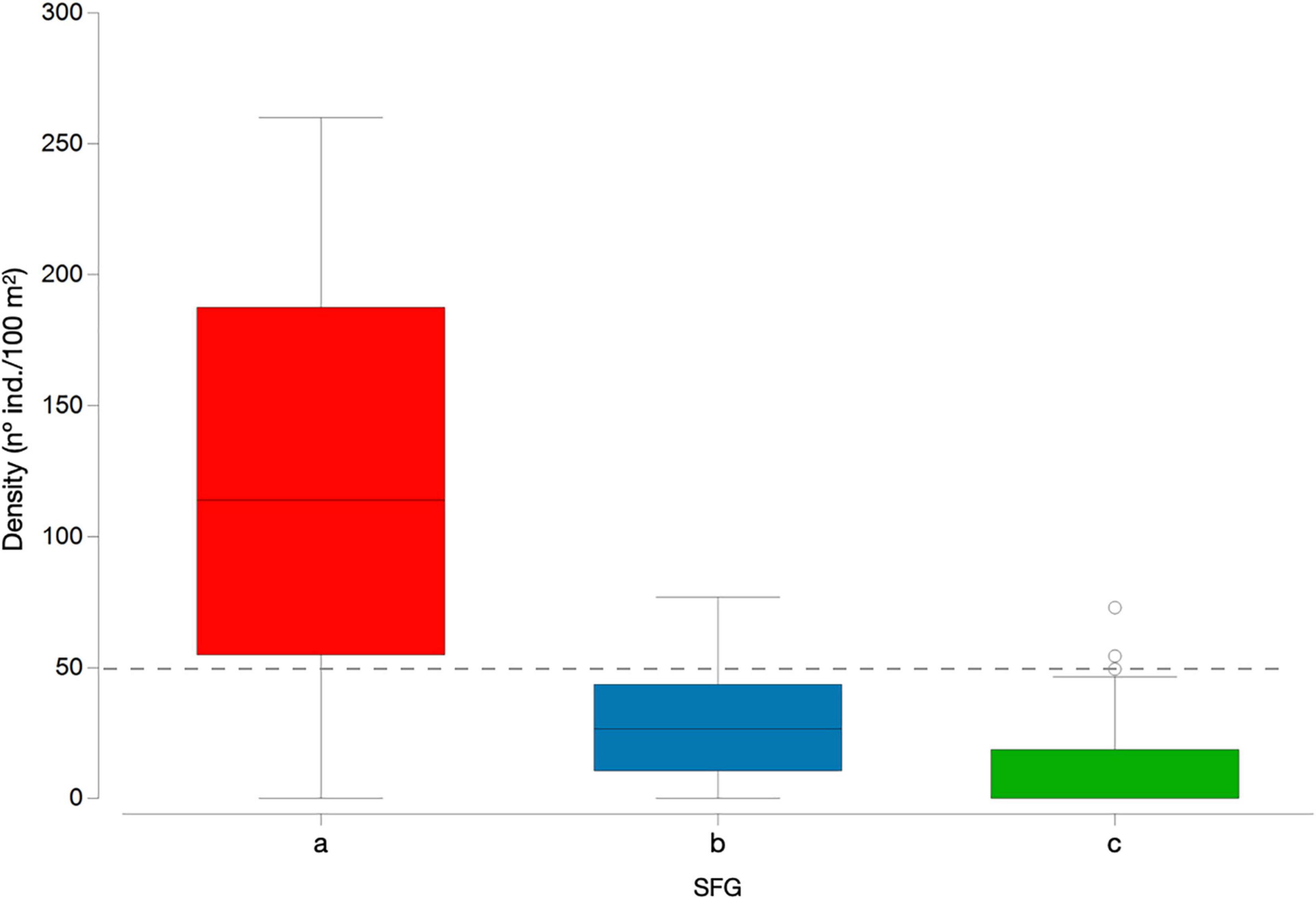
Figure 7. Diadema africanum density (individuals/100 m2) in each of the three identified assemblages (SFG = a, b, c); boxplot with first quartile, median and third quartile, minimum and maximum as whiskers and points as outliers.
Discussion
Worldwide, temperate rocky reef ecosystems with macro-algae forests host numerous diverse communities of marine organisms. However, kelp and other algae rich biotopes have been increasingly under pressure by compounding stressors such as climate change, eutrophication, coastal development, and pest species proliferation (Steneck et al., 2002; Koch et al., 2012; Borja et al., 2013; Ling et al., 2015). The uncontrolled proliferation of keystone gazers, such as sea urchins, has been reported to be an increasing problem for kelp forests and other algae rich habitats (Feehan et al., 2012; Cabanillas-Terán et al., 2015; Ling et al., 2015). In fact, temperate shallow benthic communities often present one of two ecological states: macroalgae forests with high densities of urchin predators, or; barrens where urchins overgraze algae and predators are typically scarce (reviewed in Lawrence, 1975; Steneck et al., 2002; Konar and Estes, 2003; Ling et al., 2015). In north Atlantic coasts, cyclical alternation between kelp beds and urchin barren seems to occur every 10–20 years, thanks to recurrent sea urchin mass mortality events (Scheibling et al., 2013). The role of macroalgae can be especially relevant in oceanic islands, where productivity can be low and shallow complex habitats scarce. Consequently, local populations of sea urchins (or other keystone herbivores or grazers) can have a particularly nefarious effect on local biodiversity when they become a threat to algae-dominated habitats.
In the northeast Atlantic archipelagos of Madeira and Canary Islands, previous studies describe D. africanum as a keystone species, exhibiting a key role in controlling fleshy macroalgae on rocky bottoms and promoting a barren state, through intense grazing activity (Alves et al., 2001; Brito et al., 2004; Tuya et al., 2004b; Hernández et al., 2007). The genera Halopteris, Lobophora, and Dyctiota have been identified as the preferred algae, followed by Padina and Cystoseira (Tuya et al., 2001). However, when the habitat is in the barren state, D. africanum shifts from specialist to generalist feeding, and survives eating all available algae (Tuya et al., 2004b), including CCA, microbial films, drift algae and even consuming animals (Hernández et al., 2007).
In Madeira, the southeast coast has been characterized by urchin barrens as an alternative stable state for more than 20 years (Augier, 1985; Alves et al., 2001). In theory, reverse phase shifts may occur after a major reduction in urchin population (Filbee-Dexter and Scheibling, 2014; Ling et al., 2015), however, the lack of long term datasets has hampered the ability to assess if and how mortality events and reductions in D. africanum densities may locally facilitate the recovery of macroalgae. In 2018, thanks to a yearly monitoring program of Madeira coastal habitats, it was possible to detect an ongoing D. africanum mortality event (Gizzi et al., 2020). Mass mortality events represent a natural removal experiment that eliminates many of the artifacts associated with experimental manipulations and are useful to study the effect of natural control mechanisms, sea urchin removal and consequences to algal abundance and composition. The present study investigates the variability and distribution of distinct benthic assemblages before (2017), during (2018) and after the mortality event (2019) and explores the relation between algal composition and variations in herbivore and grazer densities.
Our findings support that the reduction of D. africanum density following the 2018 mortality, was strongly correlated with shifts in benthic community structure and increase in algal cover (Figures 2–6). A non-deterministic analysis of the entire pool of samples identified three significantly different assemblages of benthic functional-groups (Figure 4). There is spatial variation in the distribution of the identified assemblages (SFG = a, b and c) in each year, however the frequency of transects with barren state assemblage (SFG = a) reduces from most common, in 2017, to absent in 2019 (Figure 6). Many factors are likely to contribute to the spatial variability in benthic assemblages and D. africanum densities (e.g., fishing, urban and coastal development, turbidity, exposure). However, an inspection and analysis of our data clearly demonstrates that locations that had a barren state assemblage prior to the disease outbreak have shifted to intermediate or algae dominated states (Figures 4, 6). Similarly, some areas also shifted from intermediate to an algae dominated state, suggesting an overall increase in macroalgal cover. These changes over time and the overall influence of D. africanum mortality in sample ordination was further confirmed by ANOSIM, with 2019 benthic data being significantly different from remaining years. Results from ANOSIM (Table 1) also support that reverse shifts (from barren to algae dominated states) can take several months to occur (Ling et al., 2015), explaining why, despite the reduction in barren state areas, no significant differences were found between 2017 and 2018 samples.
The use of a Distance-based Linear Model (Anderson et al., 2008; Clarke et al., 2014) assisted in understanding how herbivore and grazer taxa were correlated with the benthic sample ordination and grouping (SFG), with variations in benthic community structure being best explained in a model with contributions of D. africanum, and three additional taxa (Tables 2–4). The higher contribution of D. africanum (Table 2) and the stronger correlation with benthic data ordination (Figure 5) can be easily explained by the greater variance in density (Supplementary Figure 1) compounded by his voracious appetite and a mean daily algal consumption of 0.5–0.7 gDW/ind./day (Tuya et al., 2004a). This is in agreement with findings in the neighboring Canary Islands, where D. africanum is able to maintain barren rocky reefs (Tuya et al., 2004a). The characteristics of this species, including the high level of daily consumption, the ability to change its diet depending on habitat, the capacity to live at high population densities and the lack of predators, makes D. africanum determinant in shaping rocky reef communities and the stability of barren states in Madeira.
Overall, the strong correlation between D. africanum density and benthic data ordination (Tables 2, 4 and Figure 5) coupled with the significant differences in benthic data (Table 1 and Figure 4) and the reductions in the urchin numbers and barren areas following the 2018 disease outbreak (Figures 2, 3, 6) support that the mass mortality event has indeed triggered a reverse shift and increase in macroalgae cover. The severe reduction in the numbers of D. africanum appear to have reached a tipping point that enabled the recovery of algal cover within 12 months. A close inspection of urchin densities in the three distinct assemblages (SFG = a, b and c) can assist in identifying the density tipping point for a reverse shift to occur (Figure 7). With the lower quartile in barren state (SFG = a) above 50 individuals per 100 m2 and both intermediate and algae dominated states (SFG = b and c) upper quartile below this density, it is possible to empirically identify the triggering threshold to allow a reverse shift from a stable barren state at 0.5 ind./m2. This critical tipping point is considerably lower than the 2 ind./m2 density threshold of urchin barren grounds suggested for the Canary Islands (Clemente and Hernández, 2008), but is in agreement with findings in other locations, where barrens formation occur at higher densities than reverse shifts (Ling et al., 2009, 2015).
Despite the 2018 disease outbreak and D. africanum mass mortality in the archipelago, a recovery of mature local urchin populations may indeed promote a regression in algal cover and easily return coastal habitats to a stable barren state (Filbee-Dexter and Scheibling, 2014; Ling et al., 2015). Urchin populations recovery can require years to pass before urchins emerge from crypsis to graze (Ling et al., 2009; Ling and Johnson, 2009; Ling, 2013). However, the 6-month recovery density values in local D. africanum populations reported in Gizzi et al. (2020), suggest that the algae dominated state may not be stable for long. If urchin densities reach the forward-shift critical threshold and grazing intensity is restored, barren formation can occur in a short period of time (Ling et al., 2015). As such, the stability of macroalgal forest habitats in Madeira will be partly dependent on control mechanisms keeping local D. africanum populations below the tipping point required for a forward shift. Even if assuming the 2 ind./m2 density threshold reported in barren grounds of Canary Islands (Clemente and Hernández, 2008), local populations of D. africanum are expected to easily reach this density in many areas of the island.
Predation is one of the main controlling mechanisms in the recovery of sea urchin populations (e.g., Halpern et al., 2006; Clemente et al., 2011; Medrano et al., 2019). The intensive fishing activity in Madeira (Martínez-Escauriaza et al., 2020a, b), has not only reduced local fish stocks, but also the number of sea urchin predators (Friedlander et al., 2017). In fact, previous studies in the archipelagos of Madeira and Canary Islands had already reported that local reductions in predator numbers, such as Balistes capriscus, Bodianus scrofa, Canthidermis sufflamen, and Sparisoma cretense, likely contributed to the proliferation of D. africanum and promoted urchin barrens (Tuya et al., 2004b, 2005; Hernández et al., 2008a, b; Clemente et al., 2010, 2011; Sangil et al., 2011; Friedlander et al., 2017). With a low number of natural predators due to its long spines, the reduction of predation pressure associated with fishing activities can disproportionately favor D. africanum proliferation and consequently result in a damaging effect on local macroalgae forests. Spatial variation in fishing efforts will likely have a major influence in predatory pressure and the recovery of D. africanum, but adequate management and marine spatial planning can contribute to reduce chances of new barren formations and contribute to the conservation of macroalgae dominated habitats.
In view of the ecological importance of macroalgal forests and that erect macroalgae provides nursery areas and shelter to numerous organisms in the eastern Atlantic (Hernández et al., 2008a; Sangil et al., 2014; Friedlander et al., 2017), it is of the utmost importance to take action toward the conservation and even restoration of this important habitat (Rice and Smith, 2017; Verdura et al., 2018; Melis et al., 2019). Despite the occurrence of disease outbreaks as natural control mechanisms of urchin populations, the unpredictable frequency and possible impacts on other marine species (Boudouresque and Verlaque, 2007; Rogers-Bennett, 2007; Jurgens et al., 2015; Gizzi et al., 2020; Hernández et al., 2020) makes them unreliable controlling agents for future conservation efforts of macroalgae forests. Effective management of local coastal habitats that aim to enhance marine biodiversity need to prioritize measures toward the conservation and restoration of macroalgal forests (Melis et al., 2019; Pinna et al., 2020). The present study illustrates that a severe reduction in urchin population can trigger the return of macroalgae dominated states, but to assure its stability urgent action will be required to assure that: (i) urchin densities stay below the tipping point that initiates phase shift toward barren state, and (ii) macroalgae fronds are not facing catastrophic collapse from other pressure sources such as eutrophication, sedimentation and siltation, contamination and physical damage from activities such as marine construction or dredging (Filbee-Dexter and Scheibling, 2014; Schäfer et al., 2020).
General measures for macroalgae conservation include: improvements in local fishery management policies to account for recreational fishing activities and reduce chances of overfishing; closely monitor wastewater treatment plants and other potential sources of marine pollution; regulate and enforce strict measures to reduce the impact of coastal and urban development, and; establish long-term monitoring programs to assess the status of marine coastal habitats. Management programs that target urchin control are likely the most reliable way to assure low urchin population densities and the stability and restoration of local macroalgal forests. However, the establishment of marine protected areas can simultaneously favor urchin predator pressure (by imposing fishing restrictions; Medrano et al., 2019) and reduce chances of damage to algae populations from other sources (by banning human activities that may have negative impacts), making it a preferable approach in promoting habitat and biodiversity conservation. Finally, targeted pilot-studies should explore the feasibility and success of D. africanum selective harvesting and other culling methods as proactive restoration initiatives to maintain and promote the stability of local macroalgae forests.
Data Availability Statement
The raw data supporting the conclusions of this article will be made available by the authors, without undue reservation.
Ethics Statement
Ethical review and approval was not required for the animal study because Scientific divers just counted all the macro invertebrates and fishes presented along underwater transects.
Author Contributions
FG and JM: conceptualization, analysis, and writing—original draft. FG, JM, RS, SS, NC, FH, and IG: fieldwork. JM and SA: visualization. JM, IG, and JC-C: resources and funding acquisition. FG, JM, RS, SS, NC, SA, SC, AB-I, FH, IG, and JC-C: writing—review and editing. All authors contributed to the article and approved the submitted version.
Funding
FG was supported by a post-doctoral research fellowship granted by ARDITI in the framework of project RAGES (ARDITI-RAGES-2019-001). JM and IG were supported by a post-doctoral research fellowship by Agência Regional para o Desenvolvimento da Investigação, Tecnologia e Inovação (ARDITI–M1420-09-5369-FSE-000002). RS was supported by a research fellowship in the framework of project PLASMAR+ (MAC2/1.1a/347). SS was supported through a federal state scholarship by the Kiel University, Germany and is currently funded by an ARDITI research fellowship in the scope of the H2020 project GoJelly. NC was funded by a doctoral grant (SFRH/BD/146881/2019) awarded by Fundação para a Ciência e Tecnologia (FCT). SA was supported by the CleanAtlantic INTERREG (Atlantic Area) project. AB and SC were financially supported by doctoral fellowships by Agência Regional para o Desenvolvimento da Investigação, Tecnologia e Inovação (ARDITI–M1420-09-5369-FSE-000002). JC-C was funded by national funds through FCT—Fundação para a Ciência e a Tecnologia, I.P., under the Scientific Employment Stimulus–Institutional Call–(CEECINST/00098/2018). Activities within this study were partially supported by projects MIMAR (MAC/4.6.d/066) and Project Observatório Oceânico da Madeira-OOM (M1420-01-0145-FEDER-000001). Finally, this study also had the support of Fundação para a Ciência e Tecnologia (FCT), Portugal, through the strategic project (UIDB/04292/2020) granted to MARE UI&I. This is contribution 74 from the Smithsonian’s MarineGEO Network.
Conflict of Interest
The authors declare that the research was conducted in the absence of any commercial or financial relationships that could be construed as a potential conflict of interest.
Acknowledgments
We thank the Captain and 2017–2018 crews of the research vessel NRP Auriga (Instituto Hidrográfico/Marinha Portuguesa) and local dive operators Atalaia Diving Center, Madeira Diving Center, Azul Diving, Madeira Divepoint and Scuba Madeira for their logistic support.
Supplementary Material
The Supplementary Material for this article can be found online at: https://www.frontiersin.org/articles/10.3389/fmars.2021.645578/full#supplementary-material
References
Alves, F. M. A., Chicharo, L. M., Serrão, E., and Abreu, A. D. (2001). Algal cover and sea urchin spatial distribution at Madeira Island (NE Atlantic). Sci. Mar. 65, 383–392. doi: 10.3989/scimar.2001.65n4383
Anderson, M. J., Gorley, R. N., and Clarke, K. R. (2008). PERMANOVA+ for PRIMER: Guide to Software and Statistical Methods. Plymouth: PRIMER-E.
Augier, H. (1985). Première contribution a l’étude et à la cartographie des biocénoses marines benthiques de l’île de Madère. Bol. Mus. Mun. Funchal. 37, 86–129.
Borja, Á, Fontán, A., and Muxika, I. (2013). Interactions between climatic variables and human pressures upon a macroalgae population: implications for management. Ocean Coast. Manag. 76, 85–95. doi: 10.1016/j.ocecoaman.2013.02.023
Boudouresque, C., and Verlaque, M. (2007). “Chapter 13 Ecology of Paracentrotus lividus,” in Edible Sea Urchins: Biology and Ecology, ed. J. M. Lawrence (Amsterdam: Elsevier), 243–285. doi: 10.1016/S0167-9309(07)80077-9
Brito, A., Hernández, J. C., Falcón, J. M., García, N., González-Lorenzo, G., Gil-Rodríguez, M. C., et al. (2004). El Erizo de lima (Diadema antillarum) una especie clave en los fondos rocosos litorales de Canarias. Makaronesia 6, 68–86.
Cabanillas-Terán, N., Martín, J. A., Rodríguez-Barreras, R., and Luque, Á (2015). Size-density strategy displayed by Diadema africanum linked with the stability of urchin-barrens in the Canary Islands. J. Mar. Biol. Assoc. U. K. 95, 145–151. doi: 10.1017/S0025315414001246
Caldeira, R. M. A., Groom, S., Miller, P., Pilgrim, D., and Nezlin, N. P. (2002). Sea-surface signatures of the island mass effect phenomena around Madeira Island, Northeast Atlantic. Remote Sens. Environ. 80, 336–360. doi: 10.1016/S0034-4257(01)00316-9
Canning-Clode, J., Kaufmann, M., Molis, M., Wahl, M., and Lenz, M. (2008). Influence of disturbance and nutrient enrichment on early successional fouling communities in an oligotrophic marine system. Mar. Ecol. 29, 115–124. doi: 10.1111/j.1439-0485.2007.00210.x
Christie, H., Norderhaug, K. M., and Fredriksen, S. (2009). Macrophytes as habitat for fauna. Mar. Ecol. Prog. Ser. 396, 221–233. doi: 10.3354/meps08351
Claisse, J. T., Williams, J. P., Ford, T., Pondella, D. J., Meux, B., and Protopapadakis, L. (2013). Kelp forest habitat restoration has the potential to increase sea urchin gonad biomass. Ecosphere 4, 1–19. doi: 10.1890/ES12-00408.1
Clarke, K. R., and Gorley, R. N. (2015). Getting STARTED with PRIMER v7. Plymouth: PRIMER-E. Plymouth Mar. Lab., 20.
Clarke, K. R., Gorley, R. N., Somerfield, P. J., and Warwick, R. M. (2014). Change in Marine Communities: An Approach to Statistical Analysis and Interpretation, 3rd Edn. Plymouth: PRIMER-E Ltd.
Clarke, K. R., Somerfield, P. J., and Gorley, R. N. (2008). Testing of null hypotheses in exploratory community analyses: similarity profiles and biota-environment linkage. J. Exp. Mar. Bio. Ecol. 366, 56–69. doi: 10.1016/j.jembe.2008.07.009
Clemente, S., and Hernández, J. C. (2008). Influence of wave exposure and habitat complexity in determining spatial variation of the sea urchin Diadema aff. antillarum (Echinoidea: Diadematidae) populations and macro algal cover (Canary Islands-Eastern Atlantic Ocean). Rev. Biol. Trop. 56, 229–254. doi: 10.15517/RBT.V56I3.27088
Clemente, S., Hernández, J. C., Rodríguez, A., and Brito, A. (2010). Identifying keystone predators and the importance of preserving functional diversity in sublittoral rocky-bottom areas. Mar. Ecol. Prog. Ser. 413, 55–67. doi: 10.3354/meps08700
Clemente, S., Lorenzo-Morales, J., Mendoza, J. C., López, C., Sangil, C., Alves, F., et al. (2014). Sea urchin Diadema africanum mass mortality in the subtropical eastern Atlantic: role of waterborne bacteria in a warming ocean. Mar. Ecol. Prog. Ser. 506, 1–14. doi: 10.3354/meps10829
Clemente, S., Rodríguez, A., Brito, A., Ramos, A., Monterroso, Ó, and Hernández, J. C. (2011). On the occurrence of the hydrocoral Millepora (Hydrozoa: Milleporidae) in the subtropical eastern Atlantic (Canary Islands): is the colonization related to climatic events? Coral Reefs 30, 237–240. doi: 10.1007/s00338-010-0681-7
Costanza, R., D’Arge, R., de Groot, R., Farber, S., Grasso, M., Hannon, B., et al. (1997). The value of the world’s ecosystem services and natural capital. Nature 387, 253–260. doi: 10.1038/387253a0
Duffy, J. E., and Hay, M. E. (1990). Seaweed adaptations to herbivory. Bioscience 40, 368–375. doi: 10.2307/1311214
Edwards, M., Konar, B., Kim, J.-H., Gabara, S., Sullaway, G., McHugh, T., et al. (2020). Marine deforestation leads to widespread loss of ecosystem function. PLoS One 15:e0226173. doi: 10.1371/journal.pone.0226173
Feehan, C., Scheibling, R. E., and Lauzon-Guay, J. S. (2012). Aggregative feeding behavior in sea urchins leads to destructive grazing in a Nova Scotian kelp bed. Mar. Ecol. Prog. Ser. 444, 69–83. doi: 10.3354/meps09441
Filbee-Dexter, K., and Scheibling, R. E. (2014). Sea urchin barrens as alternative stable states of collapsed kelp ecosystems. Mar. Ecol. Prog. Ser. 495, 1–25. doi: 10.3354/meps10573
Filbee-Dexter, K., and Wernberg, T. (2018). Rise of turfs: a new battlefront for globally declining kelp forests. Bioscience 68, 64–76. doi: 10.1093/biosci/bix147
Friedlander, A. M., Ballesteros, E., Clemente, S., Gonçalves, E. J., Estep, A., Rose, P., et al. (2017). Contrasts in the marine ecosystem of two Macaronesian islands: a comparison between the remote Selvagens Reserve and Madeira Island. PLoS One 12:e0187935. doi: 10.1371/journal.pone.0187935
Friedlander, A. M., Ballesteros, E., Clemente, S., Gonçalves, E. J., Estep, A., Rose, P., et al. (2018). Data from: contrasts in the marine ecosystem of two Macaronesian islands: a comparison between the remote Selvagens Reserve and Madeira Island. Dryad Digital Repository doi: 10.5061/dryad.322q2
Gizzi, F., Jiménez, J., Schäfer, S., Castro, N., Costa, S., Lourenço, S., et al. (2020). Before and after a disease outbreak: tracking a keystone species recovery from a mass mortality event. Mar. Environ. Res. 156:104905. doi: 10.1016/j.marenvres.2020.104905
Graham, M. H. (2004). Effects of local deforestation on the diversity and structure of southern California giant kelp forest food webs. Ecosystems 7, 341–357. doi: 10.1007/s10021-003-0245-6
Guarnieri, G., Bevilacqua, S., Figueras, N., Tamburello, L., and Fraschetti, S. (2020). Large-Scale Sea urchin culling drives the reduction of subtidal barren grounds in the mediterranean sea. Front. Mar. Sci. 7:519. doi: 10.3389/fmars.2020.00519
Halpern, B. S., Cottenie, K., and Broitman, B. R. (2006). Strong top-down control in southern california kelp forest ecosystems. Science 312, 1230–1232. doi: 10.1126/science.1128613
Hernández, J. C. (2017). Influencia humana en las fluctuaciones poblacionales de erizos de mar. Rev. Biol. Trop. 65, S23–S34. doi: 10.15517/RBT.V65I1-1.31663
Hernández, J. C., Brito, A., Cubero, E., García, N., Girard, D., González-Lorenzo, G., et al. (2006a). Temporal patterns of larval settlement of Diadema antillarum (Echinodermata: Echinoidea) in the Canary Islands using an experimental larval collector. Bull. Mar. Sci. 78, 271–279.
Hernández, J. C., Brito, A., García, N., Gil-Rodríguez, M. C., Herrera, G., Cruz-Reyes, A., et al. (2006b). Spatial and seasonal variation of the gonad index of Diadema antillarum (Echinodermata: Echinoidea) in the Canary Islands. Sci. Mar. 70, 689–698. doi: 10.3989/scimar.2006.70n4689
Hernández, J. C., Clemente, S., Sangil, C., and Brito, A. (2008a). Actual status of the sea urchin Diadema aff. antillarum populations and macroalgal cover in marine protected areas compared to a highly fished area (Canary Islands-eastern Atlantic Ocean). Aquat. Conserv. Mar. Freshw. Ecosyst. 18, 1091–1108. doi: 10.1002/aqc.903
Hernández, J. C., Clemente, S., Sangil, C., and Brito, A. (2008b). The key role of the sea urchin Diadema aff. antillarum in controlling macroalgae assemblages throughout the Canary Islands (eastern subtropical Atlantic): an spatio-temporal approach. Mar. Environ. Res. 66, 259–270. doi: 10.1016/j.marenvres.2008.03.002
Hernández, J. C., Gil-Rodríguez, M. C., Herrera-López, G., and Brito, A. (2007). Diet of the key herbivore Diadema antillarum in two contrasting habitats in the Canary Islands (Eastern Atlantic). Vieraea 35, 109–120.
Hernández, J. C., Sangil, C., and Clemente, S. (2013). “Sea urchins, natural events and benthic ecosystems functioning in the Canary Islands,” in Climate Change Perspectives From the Atlantic: Past, Present and Future, eds J. M. Fernández-Palacios, L. Nascimento, J. C. Hernández, S. Clemente, A. González, and J. P. Díaz-González (Spain: Universidad de La Laguna), 487–512.
Hernández, J. C., Sangil, C., and Lorenzo-Morales, J. (2020). Uncommon southwest swells trigger sea urchin disease outbreaks in Eastern Atlantic archipelagos. Ecol. Evol. 10, 7963–7970. doi: 10.1002/ece3.6260
Johnson, J., and Stevens, I. (2000). A fine resolution model of the eastern North Atlantic between the Azores, the Canary Islands and the Gibraltar Strait. Deep Sea Res. Part I Oceanogr. Res. Pap. 47, 875–899.
Jurgens, L. J., Rogers-Bennett, L., Raimondi, P. T., Schiebelhut, L. M., Dawson, M. N., Grosberg, R. K., et al. (2015). Patterns of mass mortality among rocky shore invertebrates across 100 km of northeastern Pacific coastline. PLoS One 10:e0126280. doi: 10.1371/journal.pone.0126280
Koch, M., Bowes, G., Ross, C., and Zhang, X.-H. (2012). Climate change and ocean acidification effects on seagrasses and marine macroalgae. Glob. Change Biol. 19, 103–132. doi: 10.1111/j.1365-2486.2012.02791.x
Konar, B., and Estes, J. A. (2003). The stability of boundary regions between kelp beds and deforested areas. Ecology 84, 174–185.
Korpinen, S., Jormalainen, V., and Pettay, E. (2010). Nutrient availability modifies species abundance and community structure of Fucus-associated littoral benthic fauna. Mar. Environ. Res. 70, 283–292. doi: 10.1016/j.marenvres.2010.05.010
Kraufvelin, P. (2017). Macroalgal grazing by the green sea urchin: born to consume resources. Mar. Biol. 164, 1–3. doi: 10.1007/s00227-017-3161-6
Krause-Jensen, D., and Duarte, C. M. (2016). Substantial role of macroalgae in marine carbon sequestration. Nat. Geosci. 9, 737–742. doi: 10.1038/ngeo2790
Lawrence, J. M. (1975). On the relationship between marine plants and sea urchins. Oceanogr. Mar. Biol. Ann. Rev. 13, 213–286.
Ling, S. D. (2008). Range expansion of a habitat-modifying species leads to loss of taxonomic diversity: a new and impoverished reef state. Oecologia 156, 883–894. doi: 10.1007/s00442-008-1043-9
Ling, S. D. (2013). “Pushing boundaries of range and resilience: a review of range-extension by a barrens-forming sea urchin,” in Climate Change Perspectives From the Atlantic: Past, Present and Future, eds J. M. Fernández-Palacios, L. Nascimento, J. C. Hernández, S. Clemente, A. González, and J. P. Díaz-González (Spain: Universidad de La Laguna), 411–442.
Ling, S. D., Ibbott, S., and Sanderson, J. C. (2010). Recovery of canopy-forming macroalgae following removal of the enigmatic grazing sea urchin Heliocidaris erythrogramma. J. Exp. Mar. Bio. Ecol. 395, 135–146. doi: 10.1016/j.jembe.2010.08.027
Ling, S. D., and Johnson, C. R. (2009). Population dynamics of an ecologically important range-extender: kelp beds versus sea urchin barrens. Mar. Ecol. Prog. Ser. 374, 113–125. doi: 10.3354/meps07729
Ling, S. D., Johnson, C. R., Frusher, S. D., and Ridgway, K. R. (2009). Overfishing reduces resilience of kelp beds to climate-driven catastrophic phase shift. Proc. Natl. Acad. Sci. U.S.A. 106, 22341–22345. doi: 10.1073/pnas.0907529106
Ling, S. D., Scheibling, R. E., Rassweiler, A., Johnson, C. R., Shears, N., Connell, S. D., et al. (2015). Global regime shift dynamics of catastrophic sea urchin overgrazing. Philos. Trans. R. Soc. B Biol. Sci. 370, 1–10. doi: 10.1098/rstb.2013.0269
Martínez-Escauriaza, R., Hermida, M., Villasante, S., Gouveia, L., Gouveia, N., and Pita, P. (2020a). Importance of recreational shore angling in the archipelago of Madeira, Portugal (northeast Atlantic). Sci. Mar. 84, 331–341. doi: 10.3989/scimar.05046.30A
Martínez-Escauriaza, R., Vieira, C., Gouveia, L., Gouveia, N., and Hermida, M. (2020b). Characterization and evolution of spearfishing in Madeira archipelago. Eastern Atlantic. Aquat. Living Resour. 33:12. doi: 10.1051/alr/2020015
Mata, J., Fonseca, P. F., Prada, S., Rodrigues, D., Martins, S., Ramalho, R., et al. (2013). “O Arquipélago da Madeira,” in Geologia de Portugal, Volume II Geologia Meso-Cenozóica de Portugal, eds R. Dias, A. Araújo, P. Terrinha, and J. C. Kullberg (Lisboa: Escolar Editora), 691–746.
Medrano, A., Linares, C., Aspillaga, E., Capdevila, P., Montero-Serra, I., Pagès-Escolà, M., et al. (2019). No-take marine reserves control the recovery of sea urchin populations after mass mortality events. Mar. Environ. Res. 145, 147–154. doi: 10.1016/j.marenvres.2019.02.013
Melis, R., Ceccherelli, G., Piazzi, L., and Rustici, M. (2019). Macroalgal forests and sea urchin barrens: structural complexity loss, fisheries exploitation and catastrophic regime shifts. Ecol. Complex. 37, 32–37. doi: 10.1016/j.ecocom.2018.12.005
Muguerza, N., Díez, I., Quintano, E., Bustamante, M., and Gorostiaga, J. M. (2017). Structural impoverishment of the subtidal vegetation of southeastern Bay of Biscay from 1991 to 2013 in the context of climate change. J. Sea Res. 130, 166–179. doi: 10.1016/j.seares.2017.06.006
New, A. L., Jia, Y., Coulibaly, M., and Dengg, J. (2001). On the role of the azores current in the ventilation of the north atlantic ocean. Prog. Oceanogr. 48, 163–194. doi: 10.1016/S0079-6611(01)00004-0
Nunn, P. D., Veitayaki, J., Ram-Bidesi, V., and Vunisea, A. (1999). Coastal issues for oceanic islands: implications for human futures. Nat. Resour. Forum 23, 195–207. doi: 10.1111/j.1477-8947.1999.tb00909.x
Owen-Smith, N. (2014).). Spatial ecology of large herbivore populations. Ecography 37, 416–430. doi: 10.1111/j.1600-0587.2013.00613.x
Perreault, M.-C., Borgeaud, I. A., and Gaymer, C. F. (2014). Impact of grazing by the sea urchin Tetrapygus niger on the kelp Lessonia trabeculata in Northern Chile. J. Exp. Mar. Bio. Ecol. 453, 22–27. doi: 10.1016/j.jembe.2013.12.021
Piazzi, L., and Ceccherelli, G. (2019). Effect of sea urchin human harvest in promoting canopy forming algae restoration. Estuar. Coast. Shelf Sci. 219, 273–277. doi: 10.1016/j.ecss.2019.02.028
Pinna, S., Piazzi, L., Ceccherelli, G., Castelli, A., Costa, G., Curini-Galletti, M., et al. (2020). Macroalgal forest vs sea urchin barren: patterns of macro-zoobenthic diversity in a large-scale Mediterranean study. Mar. Environ. Res. 159:104955. doi: 10.1016/j.marenvres.2020.104955
Poore, A. G. B., Campbell, A. H., and Steinberg, P. D. (2009). Natural densities of mesograzers fail to limit growth of macroalgae or their epiphytes in a temperate algal bed. J. Ecol. 97, 164–175. doi: 10.1111/j.1365-2745.2008.01457.x
Rice, J., and Smith, A. D. M. (2017). “Ecosystem-based management: opportunities and challenges for application in the ocean forest,” in Marine Animal Forests, eds S. Rossi, L. Bramanti, A. Gori, and C. Orejas (Cham: Springer), 965–988. doi: 10.1007/978-3-319-21012-4_26
Rogers-Bennett, L. (2007). “Chapter 19 the ecology of Strongylocentrotus franciscanus and Strongylocentrotus purpuratus,” in Edible Sea Urchins: Biology and Ecology, ed. J. M. Lawrence (Amsterdam: Elsevier), 393–425. doi: 10.1016/S0167-9309(07)80083-4
Sangil, C., Sansón, M., and Afonso-Carrillo, J. (2011). Spatial variation patterns of subtidal seaweed assemblages along a subtropical oceanic archipelago: thermal gradient vs herbivore pressure. Estuar. Coast. Shelf Sci. 94, 322–333. doi: 10.1016/j.ecss.2011.07.004
Sangil, C., Sansón, M., Clemente, S., Afonso-Carrillo, J., and Hernández, J. C. (2014). Contrasting the species abundance, species density and diversity of seaweed assemblages in alternative states: urchin density as a driver of biotic homogenization. J. Sea Res. 85, 92–103. doi: 10.1016/j.seares.2013.10.009
Schäfer, S., Monteiro, J., Castro, N., Gizzi, F., Henriques, F., Ramalhosa, P., et al. (2020). Lost and found: a new hope for the seagrass Cymodocea nodosa in the marine ecosystem of a subtropical Atlantic Island. Reg. Stud. Mar. Sci. 41:101575. doi: 10.1016/j.rsma.2020.101575
Scheffer, M., Carpenter, S., Foley, J. A., Folke, C., and Walker, B. (2001). Catastrophic shifts in ecosystems. Nature 413, 591–596. doi: 10.1038/35098000
Scheibling, R. E., Feehan, C. J., and Lauzon-Guay, J.-S. (2013). “Climate change, disease and the dynamics of a kelp-bed ecosystem in Nova Scotia,” in Climate Change Perspectives From the Atlantic: Past, Present and Future, eds J. M. Fernández-Palacios, L. Nascimento, J. C. Hernández, S. Clemente, A. González, and J. P. Díaz-González (Spain: Universidad de La Laguna), 361–387.
Schmitz, O. J. (2008). Herbivory from individuals to ecosystems. Annu. Rev. Ecol. Evol. Syst. 39, 133–152. doi: 10.1146/annurev.ecolsys.39.110707.173418
Shurin, J. B., Borer, E. T., Seabloom, E. W., Anderson, K., Blanchette, C. A., Broitman, B., et al. (2002). A cross-ecosystem comparison of the strength of trophic cascades. Ecol. Lett. 5, 785–791. doi: 10.1046/j.1461-0248.2002.00381.x
Solan, M., and Whiteley, N. (2016). Stressors in the Marine Environment: Physiological and Ecological Responses; Societal Implications. Oxford: Oxford University Press.
Stachowicz, J. J., Bruno, J. F., and Duffy, J. E. (2007). Understanding the effects of marine biodiversity on communities and ecosystems. Annu. Rev. Ecol. Evol. Syst. 38, 739–766. doi: 10.1146/annurev.ecolsys.38.091206.095659
Steneck, R. S. (2013). “Chapter 14 sea urchins as drivers of shallow benthic marine community structure,” in Sea Urchins: Biology and Ecology, ed. J. M. Lawrence (Amsterdam: Elsevier), 195–212. doi: 10.1016/B978-0-12-396491-5.00014-9
Steneck, R. S., Graham, M. H., Bourque, B. J., Corbett, D., Erlandson, J. M., Estes, J. A., et al. (2002). Kelp forest ecosystems: biodiversity, stability, resilience and future. Environ. Conserv. 29, 436–459. doi: 10.1017/S0376892902000322
Tamaki, H., Kusaka, K., Fukuda, M., Arai, S., and Muraoka, D. (2009). Undaria pinnatifida habitat loss in relation to sea urchin grazing and water flow conditions, and their restoration effort in Ogatsu Bay. Japan. J. Water Environ. Technol. 7, 201–213. doi: 10.2965/jwet.2009.201
Trowbridge, C. D., Little, C., Pilling, G. M., Stirling, P., and Miles, A. (2011). Decadal-scale changes in the shallow subtidal benthos of an Irish marine reserve. Bot. Mar. 54, 497–506. doi: 10.1515/BOT.2011.057
Tuya, F., Boyra, A., Sanchez-Jerez, P., Barbera, C., and Haroun, R. (2004a). Can one species determine the structure of the benthic community on a temperate rocky reef? The case of the long-spined sea-urchin Diadema antillarum (Echinodermata: Echinoidea) in the eastern Atlantic. Hydrobiologia 519, 211–214. doi: 10.1023/B:HYDR.0000026599.57603.bf
Tuya, F., Boyra, A., Sanchez-Jerez, P., Barbera, C., and Haroun, R. J. (2004b). Relationships between rocky-reef fish assemblages, the sea urchin Diadema antillarum and macroalgae throughout the Canarian Archipelago. Mar. Ecol. Prog. Ser. 278, 157–169. doi: 10.3354/meps278157
Tuya, F., Martín, J. A., Reuss, G. M., and Luque, A. (2001). Food preferences of the sea urchin Diadema antillarum in Gran Canaria (Canary Islands, central-east Atlantic Ocean). J. Mar. Biol. Assoc. U. K. 81, 845–849.
Tuya, F., Sanchez-Jerez, P., and Haroun, R. J. (2005). Influence of fishing and functional group of algae on sea urchin control of algal communities in the eastern Atlantic. Mar. Ecol. Prog. Ser. 287, 255–260. doi: 10.3354/meps287255
Van Alstyne, K. L. (1989). Adventitious branching as a herbivore-induced defense in the intertidal brown alga Fucus distichus. Mar. Ecol. Prog. Ser. Oldend. 56, 169–176.
Verdura, J., Sales, M., Ballesteros, E., Cefalì, M. E., and Cebrian, E. (2018). Restoration of a canopy-forming alga based on recruitment enhancement: methods and long-term success assessment. Front. Plant. Sci. 9:1832. doi: 10.3389/fpls.2018.01832
Keywords: sea urchin, Diadema africanum, alternative stable state, phase-shift, microalgae restoration, tipping point, rocky reef, Madeira Island
Citation: Gizzi F, Monteiro JG, Silva R, Schäfer S, Castro N, Almeida S, Chebaane S, Bernal-Ibáñez A, Henriques F, Gestoso I and Canning-Clode J (2021) Disease Outbreak in a Keystone Grazer Population Brings Hope to the Recovery of Macroalgal Forests in a Barren Dominated Island. Front. Mar. Sci. 8:645578. doi: 10.3389/fmars.2021.645578
Received: 23 December 2020; Accepted: 14 May 2021;
Published: 08 June 2021.
Edited by:
Charitha Bandula Pattiaratchi, University of Western Australia, AustraliaReviewed by:
Yining Chen, Second Institute of Oceanography, Ministry of Natural Resources, ChinaNuno Vaz Álvaro, Instituto de Investigação e Tecnologia da Agronomia e Meio Ambiente (IITAA), Portugal
Copyright © 2021 Gizzi, Monteiro, Silva, Schäfer, Castro, Almeida, Chebaane, Bernal-Ibáñez, Henriques, Gestoso and Canning-Clode. This is an open-access article distributed under the terms of the Creative Commons Attribution License (CC BY). The use, distribution or reproduction in other forums is permitted, provided the original author(s) and the copyright owner(s) are credited and that the original publication in this journal is cited, in accordance with accepted academic practice. No use, distribution or reproduction is permitted which does not comply with these terms.
*Correspondence: Francesca Gizzi, ZnJhbmNlc2NhLmdpenppQG1hcmUtY2VudHJlLnB0; João Gama Monteiro, am9hby5tb250ZWlyb0BtYXJlLWNlbnRyZS5wdA==
†These authors have contributed equally to this work and share first authorship