- 1Department of Biotechnology and Life Sciences, University of Insubria, Varese, Italy
- 2Nutrigenomics and Fish Growth Endocrinology, Institute of Aquaculture Torre de la Sal (IATS-CSIC), Castellón, Spain
- 3Fish Pathology, Institute of Aquaculture Torre de la Sal (IATS-CSIC), Castellón, Spain
- 4Centro Sperimentale del Latte S.r.l., Zelo Buon Persico, Italy
- 5Sacco S.r.l., Cadorago, Italy
- 6VRM S.r.l. Naturalleva, Cologna Veneta, Italy
The present research tested the effects of dietary nisin-producing Lactococcus lactis on growth performance, feed utilization, intestinal morphology, transcriptional response, and microbiota in gilthead sea bream (Sparus aurata). A feeding trial was conducted with fish weighting 70–90 g. Fish were tagged with passive, integrated transponders and distributed in nine 500 L tanks with 40 fish each. Fish were fed for 12 weeks with either a control (diet A) or experimental diets (diets B and C) in triplicate (3 tanks/diet). Extruded pellets of diets B and C were supplemented with a low (2 × 109 CFU/kg) and a high (5 × 109 CFU/kg) dose of probiotic, respectively. No significant differences were found between groups for the feed conversion ratio or specific growth rates. However, the final body weight of fish fed diet C was significantly higher than the control group with intermediate values for fish fed diet B. Histological analysis conducted using a semi-quantitative scoring system showed that probiotic did not alter the morphology of the intestine and did not trigger inflammation. With regard to the transcriptomic response, a customized PCR array layout was designed to simultaneously profile a panel of 44 selected genes. Significant differences in the expression of key genes involved in innate and acquired immunity were detected between fish fed probiotic and control diets. To analyze the microbiota associated to the feeds and the gut autochthonous microbial communities, we used the Illumina MiSeq platform for sequencing the 16S rRNA gene and a metagenomics pipeline based on VSEARCH and RDP databases. The analysis of gut microbiota revealed a lack of colonization of the probiotic in the host’s intestinal mucosa. However, probiotic did modulate the fish gut microbiota, confirming that colonization is not always necessary to induce host modification. In fact, diets B and C were enriched with Actinomycetales, as compared to diet A, which instead showed a higher percentage of Pseudomonas, Sphyngomonas, and Lactobacillus genera. These results were confirmed by the clear separation of gut bacterial community of fish fed with the probiotic from the bacterial community of control fish group in the beta-diversity and PLS-DA (supervised partial least-squares discriminant analysis) analyses.
Introduction
The definition of “Probiotics” has changed many times during this century. However, according to (Food and Agriculture Organization of the United [FAO] and World Health Organisation [WHO], 2001) probiotics are “live microorganisms that confer a health benefit on the host when administered in adequate amounts.” The most commonly used probiotics are bacteria belonging to Lactobacillus, Bifidobacterium, Bacillus, and Enterococcus genera (European Medicines Agency [EMA], and European Food Safety Authority [EFSA], 2017; EFSA FEEDAP [EFSA Panel on Additives and Products or Substances used in Animal Feed] et al., 2018), but some fungal genera have also been reported as novel probiotics.
In the last 25–30 years, the use of probiotics in animal production has increased (Chaucheyras-Durand and Durand, 2010; Ezema, 2013). Indeed, several publications have reported numerous beneficial effects associated with the supplementation of live yeast or bacteria (mostly Lactobacillus) in the diet of terrestrial animals, including amelioration of resistance to pathogens, improvement in growth parameters (in swine and poultry), increase in productivity and quality of eggs in laying hens, and enhancement of milk production in cattle (Gallazzi et al., 2008; Shabani et al., 2012; Puphan et al., 2015; Uyeno et al., 2015; De Cesare et al., 2017; Wang et al., 2017; Dowarah et al., 2018; Forte et al., 2018).
In aquaculture, a great number of bacterial species are currently used as probiotics (for a review, please see Newaj-Fyzul et al., 2014). These microorganisms can be administered as multi-species (multi-strain) or single-species (single-strain) (Food and Agriculture Organization of the United [FAO], 2016) and provided either as a suspension in water, or added to the feed. However, use in feed is considered the best option; therefore, this approach is employed most frequently (Nayak, 2010; Jahangiri and Esteban, 2018). In the European Union (EU), probiotic strains, must obtain a market authorization by the EFSA (European Safety Food Authority)1, which grants a QPS (Qualified Presumption of Safety) status. The QPS is based on reasonable evidence. No microorganism belonging to a QPS status group needs to undergo a full safety assessment, but microorganisms that pose a safety concern to humans, animals, or environment are not considered suitable for QPS status and must undergo a full safety assessment. The QPS assessment requires: (1) the identity of the strain to be conclusively established, and (2) absence of resistance to antibiotics (for bacteria) or antimycotics (for yeasts) used in human and veterinary medicine (EFSA Panel on Biological Hazards (BIOHAZ) et al., 2020).
The increase in the use of probiotics in aquaculture is mostly related to the need to decrease or even avoid the use of antibiotics, increasing at the same time the sustainability of the aquaculture industry. The negative effects of antibiotics overuse include the accumulation of residue in the aquatic environment, particularly in the marine sediments where antibiotics can persist for months, favoring the selection of multi-antibiotic-resistant bacterial strains. Indeed, there is an increasing risk that antibiotic-resistant bacteria, initially derived from food-producing animals, could render the latest generation of antibiotics virtually ineffective for humans (Cabello, 2006; World Health Organisation [WHO] et al., 2006). Another negative outcome of antibiotics being used as growth promoters in cultured fish is the reduction of biodiversity and quantity of indigenous gut microbiota, which can impair fish immune responses (Borch et al., 2015).
For these reasons, the use of antibiotics as growth promoters in animal production has been fully banned in the EU since 2006 (Casewell et al., 2003; European Parliament and the Council of the European Union, 2003, 2019; European Medicines Agency [EMA], and European Food Safety Authority [EFSA], 2017) and many research efforts have been undertaken to replace them with probiotics for animal health management (Ezema, 2013).
Several studies have demonstrated that probiotics can reduce pathogenic bacteria due to direct competition-colonizing dynamics, through which microorganisms can partition spatial niche habitats in the intestinal mucosa (Balcázar et al., 2007b; Sugimura et al., 2011). Probiotics can also produce inhibitory molecules, such as bacteriocins, siderophores, enzymes, and hydrogen peroxide, or inhibit pathogenic bacteria by decreasing the intestinal pH through the release of organic acids (Ringø, 2008; Zhou X. et al., 2010; Ustyugova et al., 2012; Perez et al., 2014; Dahiya et al., 2020).
In addition, probiotics enhance the host immune system by generating systemic and/or local responses (Balcázar et al., 2006b; Salinas et al., 2008) that include activation of various antioxidant pathways and an increase in several innate immune parameters, such as phagocytosis, lysozyme levels, respiratory burst peroxidase and antiprotease activity, cytokine production, and white blood cell count (Nayak, 2010; Lazado and Caipang, 2014; Newaj-Fyzul et al., 2014; Simó-Mirabet et al., 2017).
In cultured fish, probiotics improve fish growth and feed conversion rates, too, due to an increase in feed digestibility and absorption of nutrients (Dimitroglou et al., 2011; Martínez Cruz et al., 2012). These effects stem from the capacity of probiotics to secrete enzymes, such as proteases, amylases, and lipases that hydrolyze molecules, which the fish intestine cannot otherwise digest (Balcázar et al., 2006b; Abd El-Rhman et al., 2009). Furthermore, the use of probiotics can restore the eubiotic state of the intestinal microbiota after antibiotic treatment or a pathogenic insult or can help maintain gut microbiota homeostasis, even in larval stages, when vaccination is difficult (Abdelhamid et al., 2009; Borch et al., 2015).
Hence, positive effects of different probiotics have been reported in several fish species, such as Nile tilapia (Oreochromis niloticus) (Ridha and Azad, 2012), common carp (Cyprinus carpio) (Feng et al., 2019), African catfish (Clarias gariepinus) (Al-Dohail et al., 2009), olive flounder (Paralichthys olivaceus) (Heo et al., 2013), Asian sea bass (Lates calcarifer) (Ringø, 2008; Lin et al., 2017), red drum (Sciaenops ocellatus) (Zhou Q.C. et al., 2010), European sea bass (Dicentrarchus labrax) (Carnevali et al., 2006; Mahdhi, 2012), common dentex (Dentex dentex) (Hidalgo et al., 2006), gilthead sea bream (Sparus aurata) (Suzer et al., 2008; Varela et al., 2010), rainbow trout (Oncorhynchus mykiss) (Merrifield et al., 2010), and abalone (Haliotis midae) (Macey and Coyne, 2005), and in crustaceans, such as white shrimp (Litopenaeus vannamei) (Lin et al., 2004).
According to the above findings, the aim of the present research was to evaluate the effects of the lactic acid bacteria Lactococcus lactis subsp. lactis SL242, used as feed additive, on growth performance, feed utilization, intestinal morphology, transcriptional response, and microbiota in gilthead sea bream (Sparus aurata).
The probiotic strain L. lactis subsp. lactis SL242 was selected due to important characteristics of Lc. lactis in general and SL242 in particular. Lc. lactis are mesophilic lactic acid bacteria that are present in the intestinal microbiota of fish (Tarnecki et al., 2017; Ringø et al., 2020) and can adapt to the water temperature of many reared fish species. Lactococci are proteolytic bacteria (Samaržija et al., 2001) that are potentially useful for improving the digestion of proteins contained in fish feed. The proteolytic system of lactococci includes a cell wall-associated proteinase and an extracellular peptidase (Samaržija et al., 2001). Furthermore, SL242 produces the antibiotic nisin A (Malvisi et al., 2016), which can inhibit or kill vegetative cells and bacterial spores (European Safety Food Authority [EFSA], 2005). Due to its antibacterial activity, nisin is of great interest in aquaculture. Nisin-susceptible bacterial species are found among Bacillus, Clostridium, Listeria, Staphylococcus, Streptococcus, and Vibrio genera (European Safety Food Authority [EFSA], 2005; Malvisi et al., 2016; Hamid et al., 2020), including known aquatic pathogens, such as V. parahaemolyticus, and V. alginotlyticus (Hamid et al., 2020). Lc. lactis probiotics have also shown inhibitory action against Yersinia rukeri and Aeromonas salmonicida, which can affect fish growth (Balcázar et al., 2007a, 2006b). Furthermore, Lc. lactis probiotic has been effective against Aeromonas hydrophila in Oreochromis niloticus (Zhou X. et al., 2010).
Materials and Methods
Ethics Statement
Procedures for fish manipulation and tissue collection were carried out according to the Spanish (Royal Decree RD53/2013) and the current EU legislation (2010/63/EU) for handling of experimental fish. All procedures were approved by the Ethics and Animal Welfare Committees of Institute of Aquaculture Torre de la Sal (IATS-CSIC, Castellón, Spain) (Permit number 824/2019) and “Generalitat Valenciana” (permit number 2019/VSC/PEA/0197).
Animals
On June 2019, juveniles of gilthead sea bream were purchased from a Mediterranean hatchery (Piscimar, Burriana, Spain) and adapted for more than 2 months to the indoor experimental facilities of IATS-CSIC, under natural photoperiod and temperature conditions (40°5′N; 0°10′E). Seawater was pumped ashore (open system); oxygen content of water effluents was always above 85% saturation, and unionized ammonia remained below 0.02 mg/L. During the acclimation and experimental period, water temperature increased from 20–22°C in June to 28°C in August, decreasing thereafter from 24–25°C in mid-September to 13–16°C in December.
Diets
Extruded pellets of a control (diet A) and two experimental diets (diets B and C) were manufactured by VRM Srl Naturalleva (Verona, Italy), mimicking commercial fish feed formulations with traditional vegetable proteins and oils as the main replacers of fishmeal and fish oil, respectively (Table 1). The mash of each diet was extruded using a single-screw extruder (X-165, Wenger United States). To ensure product stability, the probiotic was homogenized with the dietary oil and included by vacuum coating (La Meccanica vacuum coater, Italy) during the post-extrusion process. During the vacuum process, only dry basal extruded pellets of diets B and C were supplemented with 2.5 and 6.2 g/100 kg of L. lactis subsp. lactis SL242, corresponding to a probiotic dosage of 2 × 109 CFU/kg (low dose) and 5 × 109 CFU/Kg (high dose), respectively. Sacco S.r.l [Cadorago (Co), Italy] provided the probiotic strain.
The two doses were chosen on the basis of our experience and literature data (Villamil et al., 2002; Adel et al., 2017) in order to verify the most effective one. They are also in line with dosages that could be used commercially in a cost-effective manner.
The final feeds were stored in a refrigerated room (6–7°C) for the entire duration of the feeding trial. A preliminary stability study of SL242 in the feed supplemented with probiotic was conducted for 12 weeks (the duration of the experiment), at 6°C. At the end of this period, the average loss of viability determined by plate count resulted about 50%, consistent with our expectations. Although further improvement may be warranted for a commercial probiotic product, at this stage of the process, the observed stability is considered acceptable.
Feeding Trial
In September 2019, fish weighing 70–90 g were randomly distributed in nine 500 L tanks to establish triplicate groups of 40 fish each (initial rearing density, 6.6–6.7 kg/m3). All fish were tagged with PIT (passive integrated transponders) (ID-100A 1.25 Nano Transponder, Trovan) in the dorsal skeletal muscle. Fish were individually weighed and measured at initial, intermediate, and final sampling points (every 4 weeks), by using a FR-200 Fish Reader W (Trovan, Madrid, Spain) for data capture and pre-processing.
The trial lasted 12 weeks (October 2019–December 2019). Fish were hand-fed once daily (12 a.m.), 5–6 days per week to visual satiety with either control or experimental diets for the entire duration of the trial. Feed intake and mortalities (<1%) were recorded daily and normal fish behavior was assessed routinely by camera monitoring.
Sample Collection
At the end of the feeding trial, four fish per replicate (12 fish/diet) were anesthetized with 0.1 g/L of tricaine-methasulfonate (MS-222, Sigma-Aldrich) and then sacrificed by severing the spinal cord. The intestine (excluding the pyloric ceca) of each fish was dissected out, weighed, and measured aseptically to calculate the intestine weight index (IWI) and intestine length index (ILI). Then, anterior (AI) and posterior (PI) intestine tissue portions (∼0.4 cm) were put either into RNAlater, or in 10% neutral buffered formalin for subsequent molecular (AI) and histological (AI, PI) analyses. The remaining part of AI was opened and washed with sterile Hank’s balanced salt solution before collecting the autochthonous intestinal bacteria by scraping intestinal mucosa with the blunt end of a clean scalpel. Then, mucus samples were transferred to a sterile Eppendorf tube and stored in ice until subsequent (within 2 h) DNA extraction for microbiota analysis.
To characterize feed-associated bacterial communities, two samples of 200 mg each from each feed were taken at the end of the trial and used for bacterial DNA extraction and sequencing.
Histological Analysis
Fixed samples of AI and PI were dehydrated in ethanol solutions with gradually increasing concentrations and then, embedded in paraffin. Sections of 5 μm were obtained with a microtome (Leica RM2245) and stained with hematoxylin and eosin (H&E), following standard histological protocols. The sections were examined under a stereomicroscope Eurotek Tecno NB50T (Orma Srl, Milan, Italy) and photographed with a digital camera Eurotek CMOS MDH5 (Orma Srl, Milan, Italy). Based on previous studies (Knudsen et al., 2007; Uran et al., 2008; Urán et al., 2009; Khojasteh, 2012), the semi-quantitative scoring system focused on five different gut morphological parameters (mucosal folds, connective tissue, lamina propria of simple folds, and supranuclear vacuoles). Histological alterations of each morphological parameter were classified using a score value ranging from one (normal condition) to five (severe alteration). The final values, obtained by the sum of score values for each parameter, were then used to classify the severity of the morphological damage by using a class-based scoring system: Class I (values ≤ 10)—normal tissue structure with slight histological alterations; Class II (values 11–15)—moderate histological alterations; and Class III (values > 15)—severe histological alterations of the organ.
Gene Expression Analysis
Total RNA from AI was extracted using a MagMax-96 total RNA isolation kit (Life Technologies, Carlsbad, CA, United States). The RNA yield was higher than 3.5 μg with absorbance measures (A260/280) of 1.9–2.1. cDNA was synthesized with the High-Capacity cDNA Archive Kit (Applied Biosystems, Foster City, CA, United States), using random decamers and 500 ng of total RNA in a final volume of 100 μL. Reverse transcription (RT) reactions were incubated 10 min at 25°C and 2 h at 37°C. Negative control reactions were run without the enzyme. As reported previously (Estensoro et al., 2016), a customized PCR array layout was designed to simultaneously profile a panel of 44 selected genes, including markers of epithelial integrity (11), nutrient transport (4), mucins (3), cytokines (9), immunoglobulins (2), cell markers and chemokines (7), and pattern recognition receptors (8) (Table 2). qPCR reactions were performed using an iCycler IQ Real-Time Detection System (Bio-Rad, Hercules, CA, United States). Diluted RT reactions (×6) were used for qPCR assays in a 25 μL volume in combination with a SYBR Green Master Mix (Bio-Rad, Hercules, CA, United States) and specific primers at a final concentration of 0.9 μM (Supplementary Table 1). The program used for PCR amplification included an initial denaturation step at 95°C for 3 min, followed by 40 cycles of denaturation for 15 s at 95°C and annealing/extension for 60 s at 60°C. All the pipetting operations were executed by means of an EpMotion 5070 Liquid Handling Robot (Eppendorf, Hamburg, Germany) to improve data reproducibility. The efficiency of PCRs (>92%) was checked, and the specificity of reactions was verified by analyzing the melting curves (ramping rates of 0.5°C/10 s over a temperature range of 55–95°C), and linearity of serial dilutions of RT reactions (r2 > 0.98). Fluorescence data acquired during the extension phase were normalized by the delta-delta CT method (Livak and Schmittgen, 2001), using beta-actin as housekeeping gene due to its stability in different experimental conditions (average CT between experimental groups varied less than 0.2).
Bacterial DNA Extraction
The bacterial DNA was extracted from feeds (2 samples/feed) and from intestinal samples (7–10 fish/dietary group). Intestinal mucus samples (200 μl) were treated with 250 μg/ml of lysozyme (Sigma) for 15 min at 37°C. Then, DNA was extracted using the High Pure PCR Template Preparation Kit (Roche) following the manufacturer’s instructions. DNA concentration, quality, and purity were measured using a NanoDrop 2000c (Thermo Fisher Scientific) and agarose gel electrophoresis (1% w/v in Tris-EDTA buffer). Samples were stored at −20°C until sequencing. The same procedure was used to extract DNA from the control and experimental feeds (previously ground to a fine powder) to evaluate the concentration of the probiotic supplement.
Illumina MiSeq Sequencing and Bioinformatic Analysis
The V3-V4 region of the 16S rRNA gene (reference nucleotide interval 341–805 nt) was sequenced using the Illumina MiSeq system (2 × 300 paired-end run) at the Genomics Unit from the Madrid Science Park Foundation (FPCM, Spain). The details on the PCR and sequencing of amplicons have been described elsewhere (Piazzon et al., 2019). Raw sequence data were uploaded to the NCBI (National Center for Biotechnology Information) and Sequence Read Archive (SRA) under NCBI BIOPROJECT ID: PRJNA679278; NCBI BIOSAMPLE ID: SAMN16828235-61; and SRA ACCESSION: SRR13081673-99. Raw forward and reverse reads were quality filtered using FastQC2, and pre-processed using Prinseq (Rahlwes et al., 2019). Terminal N bases were trimmed at both ends and sequences with >5% of total N bases were discarded. Reads that were <150 bp long with a Phred quality score <28 in both of the sequence ends and with a Phred average quality score <26 were excluded. Then, forward and reverse reads were merged using fastq-join (Aronesty, 2013).
Bacterial taxonomy was assigned using the Ribosomal Database Project (RDP) release 11 as a reference database (Cole et al., 2014). Reads were aligned with a custom-made pipeline using VSEARCH and BLAST (Altschul et al., 1990; Rognes et al., 2016). Alignment was performed establishing high stringency filters (≥90% sequence identity, ≥90% query coverage). Taxonomic assignment results were filtered and data were summarized in an Operational Taxonomic Units (OTUs) table. Sample depths were normalized by total sum scaling and then made proportional to the total sequencing depth, following previously described recommendations (McKnight et al., 2019). Species richness estimates and alpha diversity indexes were calculated using the R package Phyloseq (Mcmurdie and Holmes, 2013). Rarefaction curves were obtained by plotting the number of observed taxonomic assignations in an OTU table against the number of sequences in each sample using the R package phyloseq.
Inferred Metagenome and Pathway Analysis
Piphillin was used to normalize the amplicon data by 16S rRNA gene copy number and to infer the metagenomics content (Iwai et al., 2016). This analysis was performed with the OTUs significantly driving the separation by probiotic in the PLS-DA analysis (described in the section “Statistics”). For the analysis, a sequence identity cut-off of 97% was implemented, and the inferred metagenomics functions were assigned using the Kyoto Encyclopedia of Genes and Genomes database (KEGG, Oct 2018 Release). Raw KEGG pathway output from Piphillin was analyzed with the R Bioconductor package DESeq2 using default parameters, after flooring fractional counts to the nearest integer (Love et al., 2014; Bledsoe et al., 2016; Piazzon et al., 2019). Comparisons were also performed between different diets to evaluate possible pathway differences across diets.
Statistics
Data on growth and gene expression were analyzed by one-way ANOVA using SigmaPlot v14 (Systat Software Inc., San Jose, CA, United States). Normality of the data was verified by Shapiro-Wilk test, and Dunn’s post hoc test was used for multiple comparisons between groups. For analysis of qualitative histological data, we conducted the non-parametric Kruskall-Wallis test, followed by Dunn’s test for the multiple comparisons. GraphPad Prism8 (GraphPad Software, Inc., La Jolla, CA, United States) was used for both analyses. Microbiota species richness, alpha diversity indexes, and phylum abundance between experimental groups were determined by Kruskal-Wallis test followed by Dunn’s post hoc test. Beta diversity was tested with permutational multivariate analysis of variance (PERMANOVA), using the non-parametric method adonis from the R package Vegan with 10,000 random permutations. To further study microbiota differences between dietary groups, supervised partial least-squares discriminant analysis (PLS-DA) and hierarchical clustering of samples were sequentially applied using EZinfo v3.0 (Umetrics, Umea, Sweden) and hclust function (gplots R package), respectively. Hotelling’s T2 statistic was calculated by employing the multivariate software package, whereby points above the 95% confidence limit for T2 were considered as outliers and discarded. Values of normalized counts of OTUs present in 3 or more samples were included in the analyses, and the significant contribution to the group separation was determined by the minimum variable importance in the projection (VIP) values (Wold et al., 2001; Li et al., 2012), which renders an accurate clustering using the average linkage method and Euclidean distance feasible. The quality of the PLS-DA model was evaluated by the parameters R2Y (cum) and Q2 (cum), which indicate the fit and prediction ability, respectively. To assess whether the supervised model was being overfitted, a validation test consisting on 600 random permutations was performed using SIMCA-P + (v11.0, Umetrics).
Results
Growth Performance
Data on growth performance, feed intake, and feed conversion ratio (FCR) are reported in Table 3. All fish grew efficiently during the first 30 days of the trial (FCR = 1.27–1.28), reaching an overall FCR of 1.55–1.60 at the end of trial. The decrease in the length of the day and temperature from October to December should be noted.
No statistically significant differences were found between groups for the condition factor and specific growth rates (SGR), although the highest SGR tended to be achieved in fish fed diet C (high dose of probiotic). Indeed, the final body weight of these animals was higher than in the control group (diet A) (P < 0.05) with intermediate values for fish fed diet B (low dose of probiotic). Thus, total weight gain varied from 97% in fish fed diet A to 106% in fish fed diet C.
Histological and Biometric Scoring
Histological analysis of gilthead sea bream intestine was performed according to the aforementioned morphological criteria. The intestinal scoring data are reported in Table 4. The AI (Figures 1A–C) and PI (Figures 1D–F) portions were not affected by probiotic administration. Although the mucosal folds of the PI were significantly different (P < 0.05) between groups fed diets A and B, the total scores, calculated for each group, fall within an evaluation of Class I. In particular, the simple and complex folds appeared thin and regularly branched, lamina propria and connective tissue appeared normally proportioned and supranuclear vacuoles were numerous and well-distributed. Regarding the index of intestine length (ILI) (Table 4), diet B showed a significantly lower ILI than the control group (diet A) (P < 0.05), but no differences were observed between the other groups. No differences in the intestine weight index (IWI) were observed between groups.
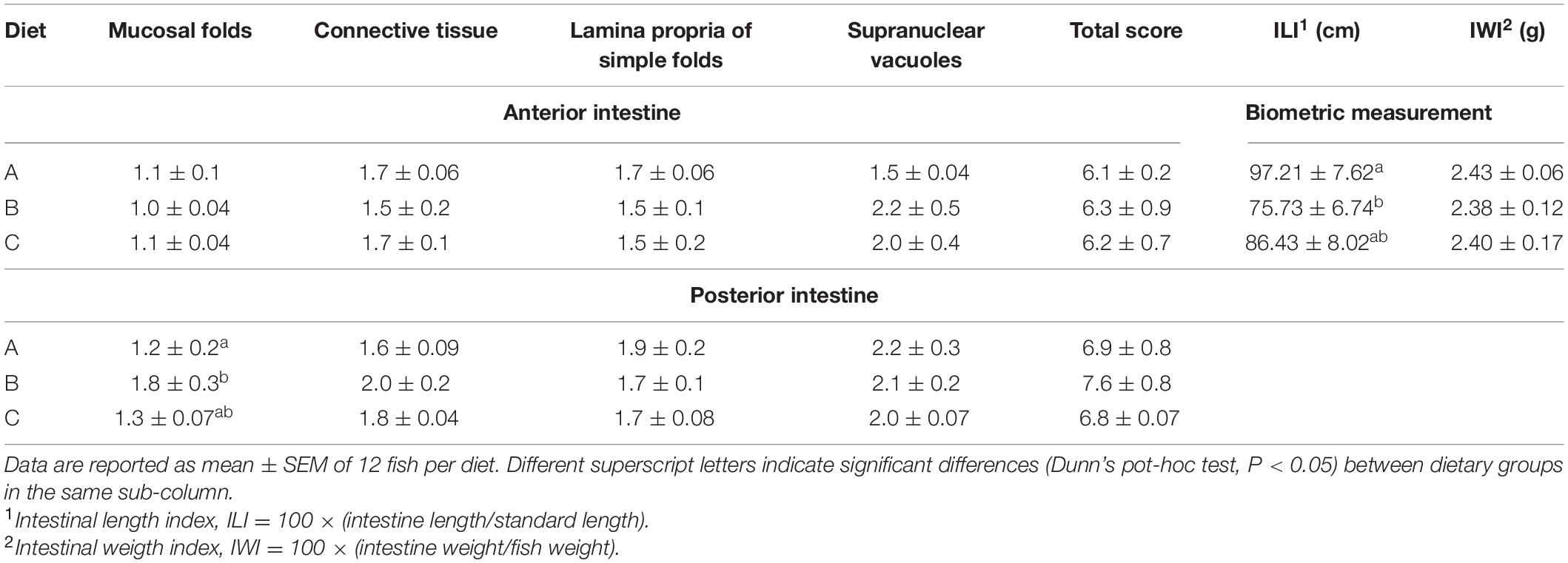
Table 4. Histological scoring (for anterior and posterior intestine) and biometric measurement [intestinal length index (ILI) and intestinal weight index (IWI)] of gilthead sea bream (Sparus aurata) juveniles fed the control (A) and experimental (B and C) diets.
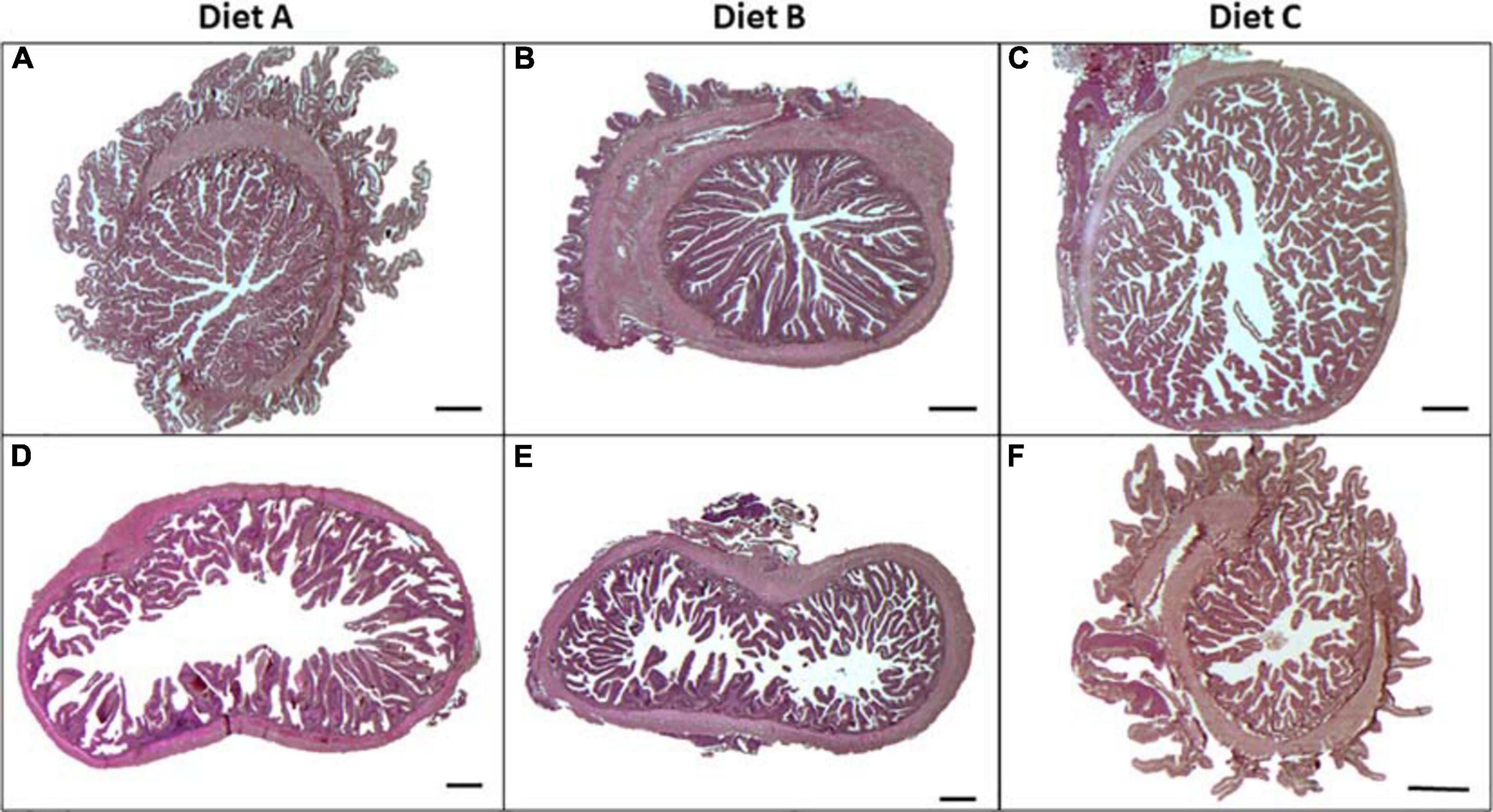
Figure 1. Light microscope images obtained from anterior (A–C) and posterior (D–F) intestine of gilthead sea bream juveniles (Sparus aurata) fed with diets A, B, and C, stained with hematoxylin and eosin (H&E). Scale bar = 500 μm.
Gene Expression Profiling
All genes included in the PCR-array were found at detectable levels with the highest expression level for markers of nutrient transport (alpi, fabp1, and fabp2), epithelial integrity (cx32.2), mucus production (muc2, muc13) and pattern recognition receptors (fcl) (Supplementary Table 2). Regarding the probiotic effect, statistically significant changes were found in the expression patterns of 5 out of 44 genes (P < 0.05) (Figure 2). In particular, expression of interleukin 10 (il10), interleukin (il12), and toll-like receptor 2 (tlr2) was upregulated in fish fed diet C (high probiotic dose) with intermediate values (not statistically different from the control group) in fish fed diet B (low probiotic dose). In contrast, the highest values of toll-like receptor 5 (tlr5) and galectin-8 (lgals8) were seen in fish fed diet B, whereas intermediate values were found in fish fed diet C. The probiotic treatment altered other markers (desmoplakin, dsp; interleukin 34, il34; C-C chemokine receptor 3, ccr3; and macrophage mannose receptor 1, mrc1) to a lesser extent, with an overall enhancement of gene expression that was especially evident in fish fed diet C (P < 0.1).
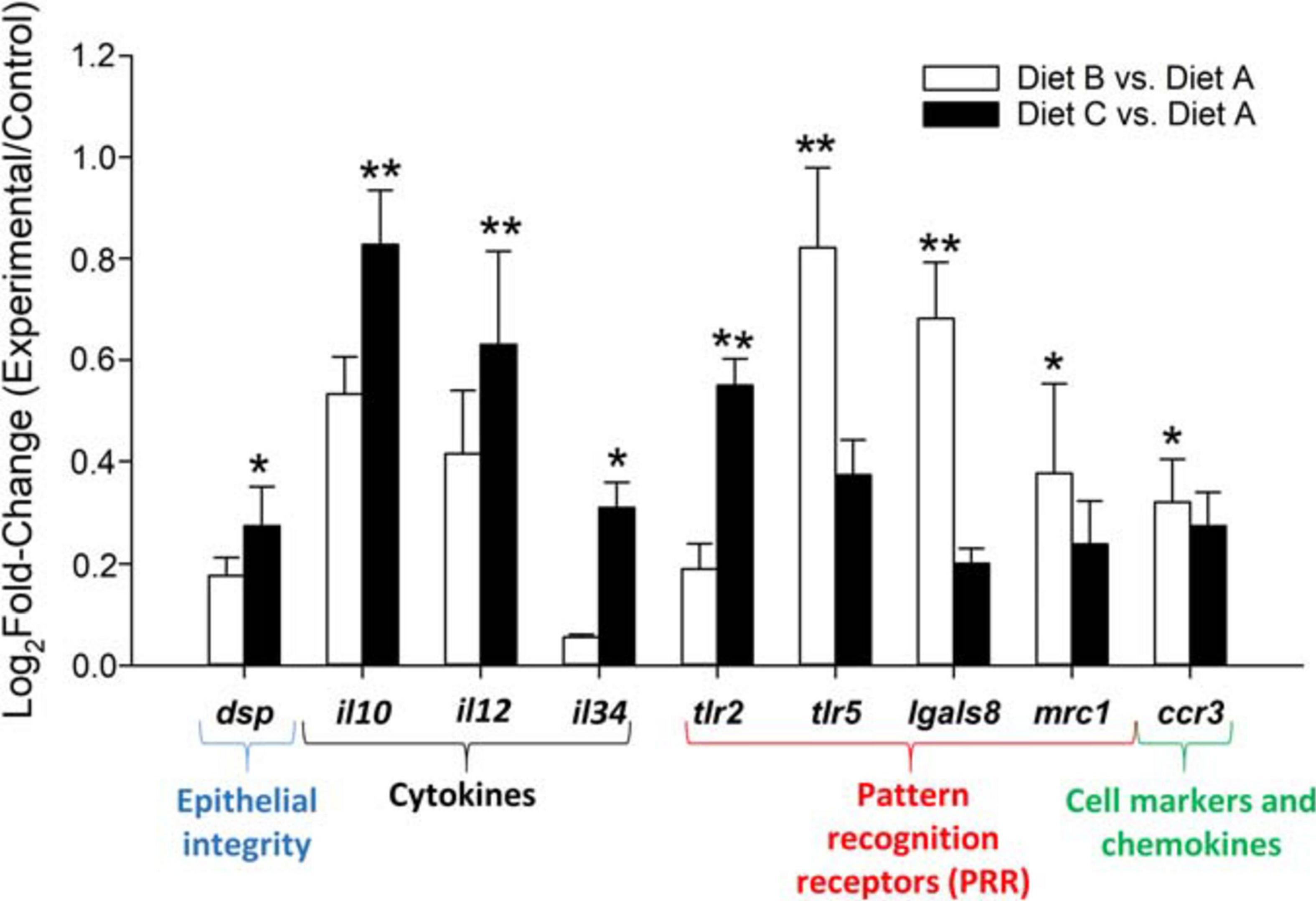
Figure 2. Fold change of differentially expressed genes (Dunn’s post hoc test; **P < 0.05; *P < 0.1) in the anterior intestine of fish fed experimental diets (diets B and C) relative to the control diet (A). Data are the mean + SEM of 9–12 fish per diet. White columns (fish fed diet B). Black columns fish fed diet C.
Characterization of Feed-Associated Bacterial Communities
At the end of the trial, the normalized counts of L. lactis subsp. lactis resulted 8–11 in diet A (<0.0001% total bacterial counts); 30,204 in diet B (2.5% total counts); and 61,828 (5.4% total counts) in diet C (Figure 3). By excluding Cyanobacteria/Chloroplast (>90% total counts), Firmicutes and Proteobacteria proved to be the most highly represented bacterial phyla in the three feeds, whereas the rest of the bacterial population consisted of Bacteriodetes and Fusobacteria phyla (Supplementary Figure 1A). However, the percentage of Firmicutes varied considerably between feeds, with higher values in feed B (4.2%) and C (7.8%) than in the control feed, in which Firmicutes represented only 2% of the total counts. Thus, by recalculating the relative bacterial abundances after excluding Cyanobacteria/Chloroplast, the percentage of Firmicutes rose from 34% in the control diet A to 70% in diet B and 79% in diet C (Supplementary Figure 1B). Then, by specifically analyzing the relative abundance of the probiotic L. lactis subsp. lactis in comparison to the most representative genera within the phylum Firmicutes, the percentage of L. lactis subsp. lactis was close to 0% in the control diet, whereas in B and C diets, it was significantly higher, reaching values of 64 and 71%, respectively (Supplementary Figure 1C).
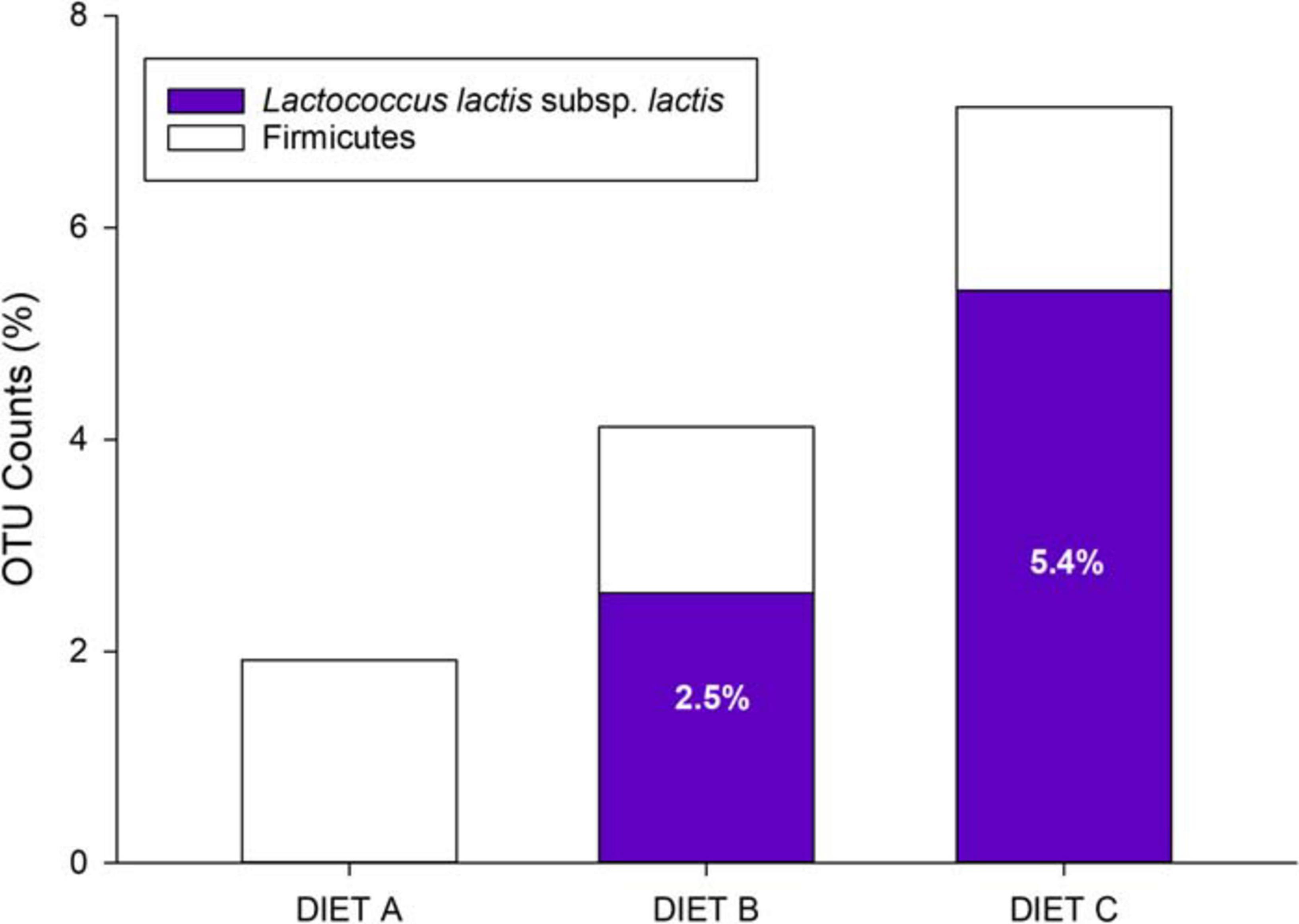
Figure 3. Relative abundance of Firmicutes phylum and Lactococcus lactis subsp. lactis in control (Diet A) and experimental diets (Diets B and C).
Alpha Diversity and Gut Microbiota Composition
Illumina sequencing of AI-adherent bacteria yielded 3,677,860 high-quality and merged reads, with an average value of 136,217 reads per sample (Supplementary Table 3). When annotated, the reads were assigned to 1,313 OTUs at 97% identity threshold. Rarefaction analysis showed curves that approximated saturation (horizontal asymptote); thus, a good coverage of the bacterial community was achieved and the number of sequences for analysis was considered appropriate (Supplementary Figure 2). Indeed, up to 85% of the OTUs were classified at the level of species and more than 90% at the level of genus (94.1%), family (96%), order (97%), class (97.2%), and phylum (99%).
As shown in Table 5, the richness estimator (ACE) indicated a higher OTU richness in fish fed diet B than in fish fed diet A or diet C. At the same time, alpha diversity estimators (Shannon and Simpson) disclosed a reduced evenness in fish fed diet C, which indicates that abundant OTUs predominated over the others in this group of fish.
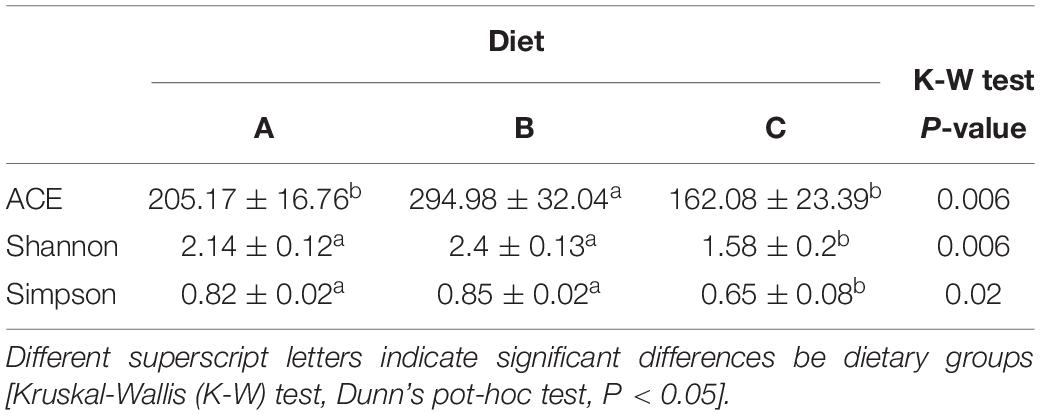
Table 5. Species richness estimate (ACE) and diversity indexes (Shannon and Simpson) of the adherent microbial communities in the anterior intestine of fish fed diet A (10), diet B (10), and diet C (7).
Changes in bacterial composition were also found at the phylum level (Figure 4). Proteobacteria was the most abundant phylum in the three groups, ranging from 55.9% in fish fed diet C to 55.7% in fish fed diet A, and 50.1% in the diet B fed group. The second-most abundant phylum was Firmicutes, representing the 26.6% of the OTU counts in fish fed diet A, decreasing progressively with the probiotic supplementation in fish fed diet B (26.2%) and diet C (5.6%). The same trend was shown by the phylum Bacteroidetes, ranging from 2.7% in fish fed diet A to 1.3% in fish fed diet B and 0.1% in fish fed diet C. The phylum Actinobacteria increased from 9.3% in fish fed diet A to 16.3% in fish fed diet B but decreased to its minimum level in group C (3.2%). Finally, Spirochetes appeared in a significant proportion (32%) only in fish fed with diet C, being practically absent in the other groups (<3%).
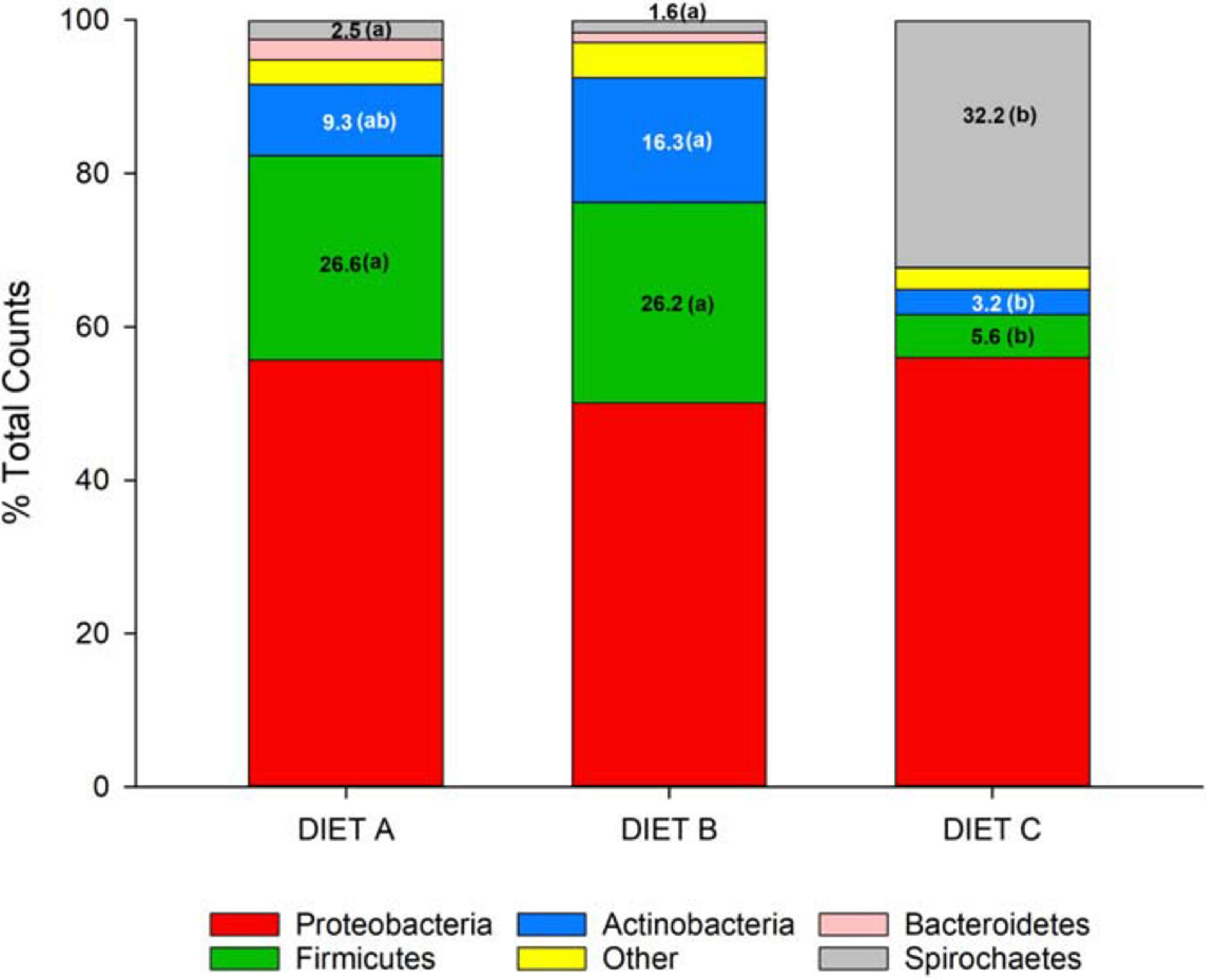
Figure 4. Stacked bar chart representing the relative abundance (%) of bacterial phyla in fish fed control (A) and experimental (B and C) diets. The Kruskal–Wallis test (Dunn’s post hoc test, P < 0.05) showed significant differences between groups for the phyla Firmicutes, Actinobacteria and Spirochetes. The differences are indicated by different letters in parenthesis that correspond to pairwise comparisons within each phylum between groups.
Beta Diversity, Discriminant Analysis, and Inferred Pathways
No significant differences in beta diversity were found when experimental groups were computed independently (PERMANOVA, P = 0.34, F = 1.031, R2 = 0.04). In contrast, when B and C groups were computed together, beta diversity became statistically significant (PERMANOVA, P = 0.032, F = 1.8789, R2 = 0.099). Taking this analysis further, a PLS-DA model was constructed with a 99% to the total variance explained (Figure 5). During the statistical processing to construct the model, two fish from the Diet A group and one fish from the Diet C group appeared as outliers and were excluded from the model. This approach displayed a clear separation of control fish and fish fed probiotic diets (B + C group) along component 1 (84.52%) with a higher individual variability within fish fed diet B than in those fed diet C. This PLS-DA model was successfully validated with a permutation test (pCV ANOVA = 0.015) discarding the possibility of over-fitting of the supervised model (Supplementary Figure 3).
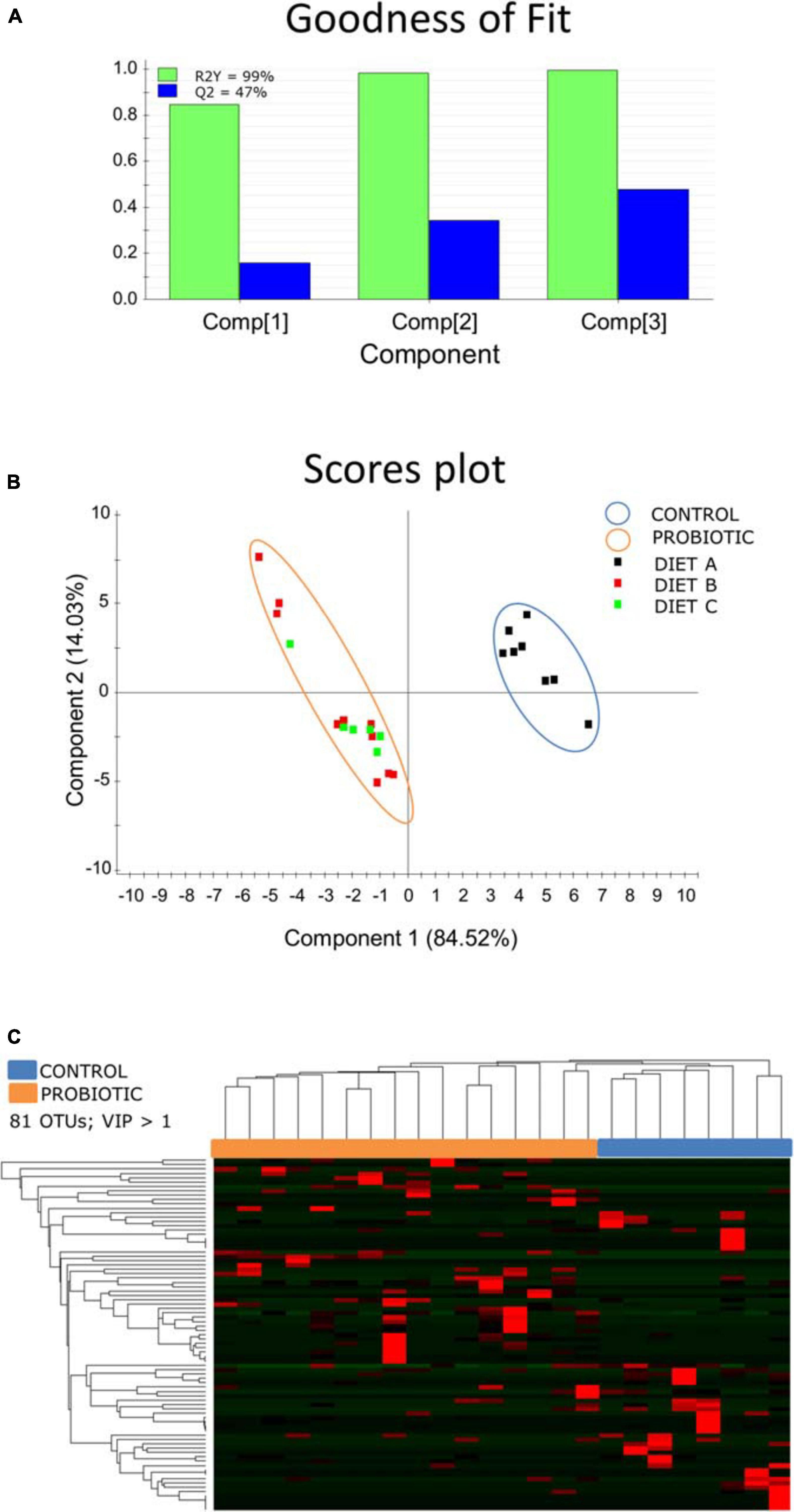
Figure 5. (A) Graphical representation of the goodness-of-fit of the PLS-DA model. (B) Two-dimensional PLS-DA score plot representing the distribution of the samples between the first two components in the model. (C) Heatmap showing the abundance distribution (z-score) of the OTUs identified to be driving the separation between fish fed probiotic diets (B + C; orange) and fish fed diet A (blue).
Differences between control fish and the probiotic-fed merged groups were driven by 81 OTUs (VIP > 1), mainly belonging to the phyla Proteobacteria, Spirochetes, and Firmicutes. A detailed list of the VIPs can be found in Supplementary Table 3. The inferred metagenomic analysis using DESeq2 disclosed nine differentially abundant pathways across groups (Figure 6). Pathways related to protein digestion and absorption, as well as renin secretion were over-represented in the probiotic fed fish groups, whereas the control group showed a relative preponderance of pathways related to shigellosis, proteasome and autophagy.
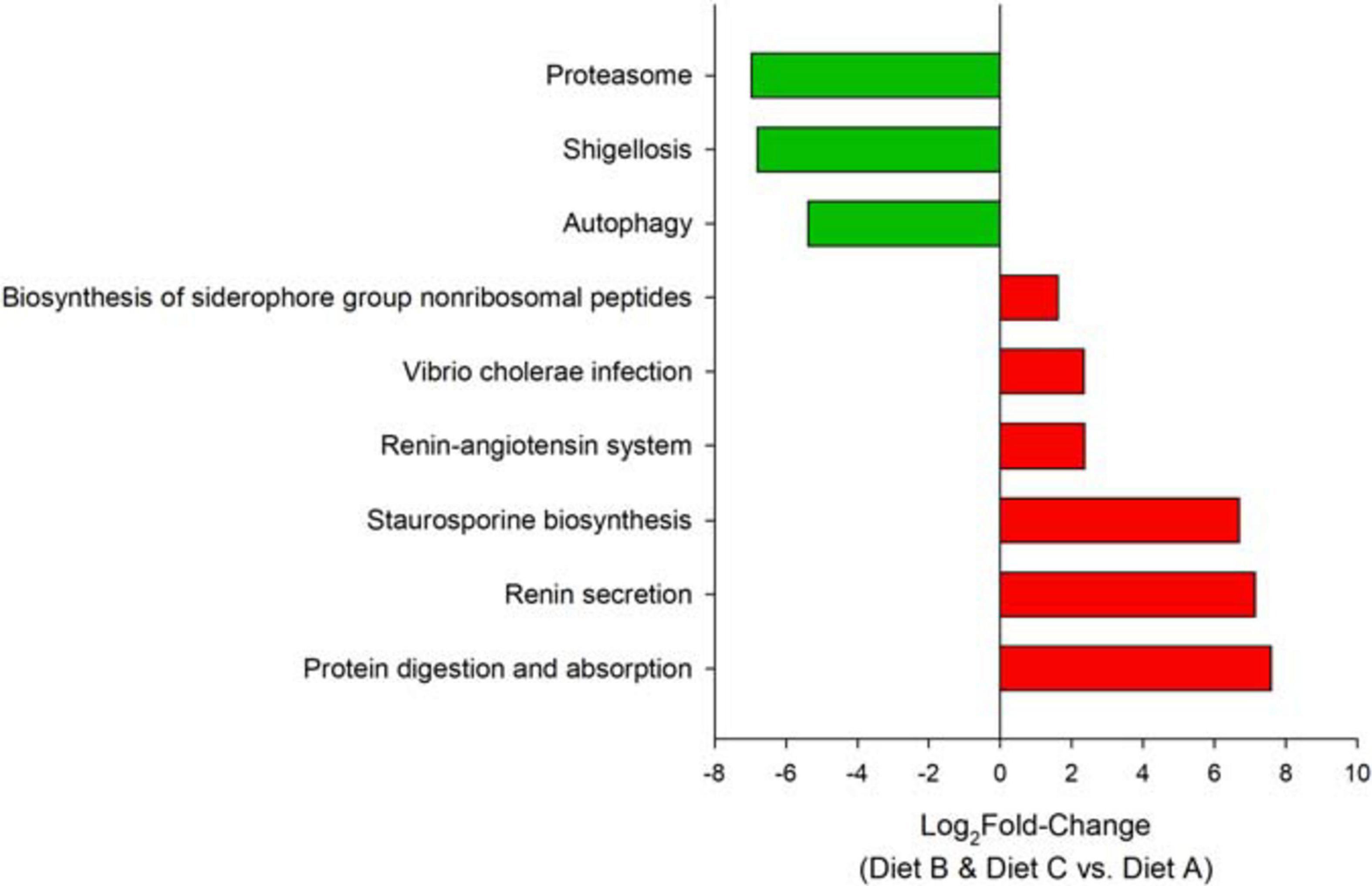
Figure 6. Bar plots depicting the changes in metabolic capacities in the comparison between fish fed probiotic diets (B + C group) and fish fed the control diet A. Bars show the log2-fold change in the metabolic pathway.
Discussion
In aquaculture the use of probiotics is significantly increasing and a growing number of studies are demonstrating their positive effects in the most economically important fish species (Merrifield et al., 2010; Varela et al., 2010; Mahdhi, 2012; Ridha and Azad, 2012; Chauhan and Singh, 2019).
As mentioned previously, one of the most interesting effects of probiotics is the increase in the animals’ growth performance (Sun et al., 2012; Nguyen et al., 2017; Won et al., 2020). In the present study, gilthead sea bream fed diets C and B, supplemented with high and low doses of L. lactis subsp. lactis, respectively, reached a higher final biomass than control fish fed with diet A, and differences in biomass gain were statistically significant between groups C and A. Although differences between fish groups arose at the December sampling, most of the weight gain was attained during September–October, as this period still corresponds to the active fish feeding behavior at IATS-CSIC latitude. This result highlights, albeit slightly, the beneficial action of the probiotic, suggesting a more efficient digestion and utilization of nutrients in gilthead sea bream fed probiotics. Indeed, although no significant differences were detected in FCR and SGR between dietary groups, the lowest FCR (1.60 ± 0.03) and the highest SGR (0.85 ± 0.02) were registered in fish fed diet C. Similar results were obtained in gilthead sea bream by Suzer et al. (2008) and Varela et al. (2010), using Lactobacillus spp. and Shewanella putrefaciens Pdp11, respectively. Positive results in fish growth performance, using L. lactis as probiotic, were also obtained in other cultured fish species, such as common carp, European sea bass, tilapia, and olive flounder (Balcázar et al., 2006a; Carnevali et al., 2006; Heo et al., 2013; Xia et al., 2018; Feng et al., 2019).
Histological analysis was conducted using a semi-quantitative scoring system. The parameters taken into account for the AI and PI morphological evaluation were related to the mucosal folds that represent the intestinal absorptive surface area, and to the associated connective tissue (Dimitroglou et al., 2011; Khojasteh, 2012; Puphan et al., 2015). Our results confirmed that probiotic did not alter the morphology of the gut and did not trigger intestinal inflammation. Indeed, no structural modifications were detected in fish fed with diets supplemented with probiotic (diets B and C), in comparison to the control group fed diet A. In line with our results, other studies have shown that probiotics improve gut morphology, leading to an increase in intestinal absorption capacity (Batista et al., 2016; Won et al., 2020). In contrast, Cerezuela et al. (2012; 2013) reported several negative effects related to the administration of probiotics in gilthead sea bream. In particular, those authors showed that both Tetraselmis chuii and Bacillus subtilis induced intestinal inflammation with numerous signs of edema in the mucosal folds. Therefore, more in-depth histological analyses are needed to better understand the effects of different probiotic strains on the adsorptive surface area in fish intestine and, in particular, on the villi length and density.
Numerous studies that have investigated the effects of probiotics on the piscine immune system have reported an enhanced immune response, thus improving survival rates and resistance to a pathogenic attack (Nayak, 2010; Lazado and Caipang, 2014). Different probiotic strains stimulate the immune system in fish, but the effect appears to be species-specific. L. lactis supplementation increased the concentration of several pro- and anti-inflammatory cytokines (Tnfα, Il1β, Il6, Il12, Il10 and Tgfβ) in common carp serum (Feng et al., 2019) and upregulated the expression of tnfα, ifnγ, hsp70, and il1β genes in the intestine of tilapia (Xia et al., 2018; Won et al., 2020). Conversely, L. lactis did not induce any differences in the abundance of cytokines and pattern recognition receptors (PRRs) transcripts in intestine or head kidney of trout (Pérez-Sánchez et al., 2011). In gilthead sea bream, the anti-inflammatory action of a Bacillus-based probiotic induced decreased expression of lgals8 and cd4 transcripts in anterior intestine, lower amounts of circulating IgM and cortisol, a lower respiratory burst activity of blood leukocytes, and lower numbers of eosinophilic granulocytes (in particular, mast cells) in the intestinal submucosa (Simó-Mirabet et al., 2017). Herein, significant differences in the expression of key genes involved in innate and acquired immunity (interleukins and PRRs) were detected between fish fed probiotic and control diets. Among the mechanisms induced by probiotics, it has been postulated that the activation of immunity derives from the interaction of the host with the probiotic microbial associated molecular patterns (MAMPs) (Yang et al., 2014). The direct effect of MAMPs was recently demonstrated by feeding grouper (Epinephelus coioides) with MAMPs isolated from the probiotic Bacillus pumilus SE5. Indeed, an activation of intestinal immunity via up-regulation of TLR signaling pathways was observed (Yang et al., 2019). Thus, the observed activation of the immune system in the present study is likely taking place by direct induction of gilthead sea bream PRRs by components on the cell wall of the probiotic, such as peptidoglycan or lipoteichoic acid, which are in fact TLR2 agonists (Dammermann et al., 2013).
The density, composition and function of intestinal microbiota of fish, including gilthead sea bream, are shaped by numerous factors, such as diet, sex, developmental stage, and rearing conditions (Piazzon et al., 2017, 2019; Rimoldi et al., 2020), as well as multiple endogenous host-microbe interactions, such as the host’s genetic background (Piazzon et al., 2020), and possible intestinal disorders or intestinal diseases (Bakke-Mckellep et al., 2007; Green et al., 2013). Furthermore, microbiota vary taxonomically and functionally in different sections of the GIT of fish (Kokou et al., 2020). There is also a distinction between the allochthonous, i.e., free-living, transient microbiota associated with the digesta (feces), and autochthonous communities that colonize the mucosal surface of the digestive tract and make up the core community (Merrifield et al., 2010; Ringø et al., 2016; Nguyen et al., 2017; Egerton et al., 2018).
Taxonomically, gut bacteria are classified according to phyla, classes, orders, families, genera, and species. The “core” intestinal microbiota, which can often persist in spite of changing factors is constituted by Proteobacteria, Firmicutes, and Actinobacteria phyla in both freshwater and marine fish species (Silva et al., 2011; Kormas et al., 2014; Ghanbari et al., 2015; Piazzon et al., 2019). These taxa are largely considered important players in nutritional provisioning, immune defense, and metabolic homeostasis (Estruch et al., 2015; Givens et al., 2015; Rimoldi et al., 2019; Terova et al., 2019).
Accordingly, in the present experiment, gilthead sea bream were fed with three different feeds and at the end of the experiment, the microbiota of these feeds was analyzed. Data revealed that Firmicutes and Proteobacteria were the bacterial phyla represented most, followed in descending order by Bacteriodetes and Fusobacteria. Then, by analyzing specifically the relative abundance of the probiotic L. lactis subsp. lactis compared to the most representative genera of Firmicutes phylum, we found that the percentage of L. lactis subsp. lactis was close to 0% in diet A (control), whereas in diets B and C, it was definitely high, reaching values of 64 and 71%, respectively. This results is in agreement with the supplementation of a low and a high dose of probiotic to diets B and C, respectively.
With regard to the gut microbiota, gilthead sea bream fed diet C showed a significant increase in bacteria belonging to the Spirochetes phylum, which were practically absent in the gut of fish fed diets B and A (<3%). In the same fish group, a decrease in Actinobacteria, Bacteroidetes, and Firmicutes phyla was recorded. The Firmicutes phylum is composed of more than 200 different genera, such as Lactobacillus, Bacillus, Enterococcus, Ruminococcus, and Clostridium. Lactic acid bacteria (LAB) include, among others, Streptococcus sp., Lactobacillus sp., Leuconostoc sp. and Carnobacterium sp., which are considered as beneficial microorganisms that contribute to an healthy status of the fish intestine (Kim et al., 2012; Terova et al., 2019). It is known that commensal Firmicutes and Bacteroidetes are the major producers of short chain fatty acids, such as butyrate, acetate, and propionate that are the end products of fiber fermentations.
While is difficult to assess from genomic data alone the physiological effect on the host of the microbiota changes we found, it is worth noting that Firmicutes/Bacteroidetes ratio in the gut has been directly related to lean body mass in both human and animals (Magne et al., 2020). Indeed, the ratio of Firmicutes vs. Bacteroidetes was increased in obese individuals as compared to lean ones. Actually, gilthead sea bream fed with diets containing probiotic showed a higher Firmicutes/Bacteroidetes ratio than control fish and this could be correlated to their better growth performances. Likewise in mice, the reduced amount of Bacteroidetes was a direct consequence of probiotic supplementation (Grazul et al., 2016). In addition, gilthead sea bream fed diet C, showing the best FCR and SGR values, had the highest percentage of Spirochetes. In swine, the Spirochaetaceae bacterial family was shown to correlate positively with the host weight (Unno et al., 2015). The gut microbiome of the feeding group C was also characterized by a Proteobacteria/Firmicutes ratio five times higher than in the other groups. This result is not surprising because Lc. lactis subsp. lactis SL242 produces the antibiotic nisin, displaying strong activity against Gram-positive bacteria (Li et al., 2018), and a vast majority of Firmicutes are Gram-positive.
The analysis of gut-adherent (autochthonous) microbiota did not reveal significant differences between fish groups in relation to L. lactis, suggesting a lack of colonization of the probiotic in the host’s intestinal mucosa. This was not a surprising result since it is known that probiotics generally do not colonize the digestive tract i.e., they do not become established permanently or for a long-term (weeks, months, or years) in the intestinal tract (Marco, 2019). Thus, the ingested bacteria can be beneficial while they are in the gut, but they do not have a lasting effect and continued probiotic consumption is needed for sustained impact. Thus, instead of colonizing, the new bacteria may temporarily complement resident microbial communities, forming part of a transient (allochthonous) microbiome in fish without displacing the native gut microbiota, but instead altering digestive tract function by producing active metabolites that modulate the activity of the gut microbiota, or by stimulating the intestinal epithelium directly (Marco, 2019). Hence, in the present trial, although the probiotic did not colonize the host’s intestinal mucosa, it did modulate the fish gut microbiota, confirming that colonization is not always necessary to induce host modification. Indeed, diets B and C were enriched with Actinomycetales, as compared to diet A, which instead showed a higher percentage of Pseudomonas, Sphyngomonas, and Lactobacillus genera. These results were confirmed by the clear separation of bacterial community of fish fed with the probiotic from the bacterial community of control fish group (diet A) in the beta-diversity and PLS-DA analyses. Furthermore, the KEGG pathway analysis underlined such differences, highlighting several pathways potentially affected by the diet. Particularly interesting were those related to protein absorption and digestion.
In the present study, the analysis of gut microbial communities revealed significant differences between fish groups in term of species richness and diversity. Among alpha diversity indices, fish fed with diet B showed the highest level of richness estimator ACE and biodiversity, in comparison to the other two fish groups. In contrast, dietary group C, although achieving the best growth performances, showed the lowest gut bacterial diversity.
A reduction in bacterial diversity is usually considered an adverse outcome, since this could lead to less competition for opportunistic or invading pathogens due to a functionally unbalanced ecosystem (Cerezuela et al., 2013; Li et al., 2014; Rimoldi et al., 2020). However, while an increase in intestinal microbial biodiversity following prebiotics (dietary compounds that induce the growth or activity of gut microbiota) administration has been frequently described, the data currently available on the effects of probiotics in fish are more controversial. For instance, in line with our results, the species richness and diversity indexes decreased in gilthead sea bream in response to dietary administration of the probiotic Bacillus subtilis, either alone or in combination with prebiotics or microalgae (Cerezuela et al., 2012, 2013). In contrast, in line with what we found in fish fed diet B, lactic acid bacteria supplementation was associated with an increase in bacterial diversity in the intestinal mucus of Atlantic salmon (Gupta et al., 2019). In addition, probiotics, such as lactic acid bacteria, are known to produce several antimicrobial compounds capable of suppressing the growth of other microorganisms, which can alter the gut microbiota in terms of both composition and biodiversity (Collado et al., 2007).
Conclusion
According to analysis of gut-adherent (autochthonous) microbiota, the probiotic L. lactis subsp. lactis did not colonize in the host’s intestinal mucosa. However, the probiotic did modulate the fish gut microbiota, confirming that colonization is not always necessary to induce host modification. Indeed, gut microbiota of fish fed diets B (low dose of probiotic) and C (high dose) were clearly separated from the bacterial community of control fish in the beta-diversity and PLS-DA analyses. Furthermore, the KEGG pathway analysis underlined such differences, highlighting several pathways potentially affected by the diet. Particularly interesting were those related to protein absorption and digestion.
With regard to fish growth performance, there were no significant differences between groups for the FCR and SGR. The only difference was the final body weight of fish fed diet C (high dose of probiotics) that resulted higher than the control group.
Dietary probiotic administration did not alter the morphology of the intestine and did not trigger inflammation.
Researches such as these highlight the interaction between fish diet and their microbiota and suggest that manipulating diet to tune the gut microbiome may be a promising intervention, together with well-designed probiotics.
Data Availability Statement
The datasets presented in this study can be found in online repositories. The names of the repository/repositories and accession number(s) can be found below: NCBI (accession: SAMN16828235–SAMN16828261 and PRJNA679278).
Ethics Statement
The animal study was reviewed and approved by the Ethics and Animal Welfare Committees of Institute of Aquaculture Torre de la Sal (IATS-CSIC, Castellón, Spain) (Permit number 824/2019) and “Generalitat Valenciana” (Permit number 2019/VSC/PEA/0197).
Author Contributions
FM, FN-C, and MP: experimental investigation, methodology, data curation, formal analysis, and writing—review and editing. SR: methodology, formal analysis, and writing—review and editing. JC-G: methodology, data curation, formal analysis, and writing—review and editing. AG: conceptualization and writing—review and editing. IM: writing—review and editing. FB: experimental investigation and methodology. JP-S: conceptualization, experimental investigation, data curation, and writing—review and editing. GT: conceptualization, data curation, and writing—review and editing. All authors read and approved the final manuscript.
Funding
This work was supported by the EU Horizon 2020 Research Innovation Program under the TNA program (project AE130011) at IATS-CSIC research infrastructure within AQUAEXCEL2020 project (652831). This work was also co-funded by the EU Horizon 2020 AquaIMPACT project (Genomic and nutritional innovations for genetically superior farmed fish to improve efficiency in European aquaculture; 818367). This output reflects only the author’s view and the European Union cannot be held responsible for any use that may be made of the information contained herein. MP was funded by a Ram n y Cajal Postdoctoral Research Fellowship [RYC2018-024049-I/AEI/10.13039/501100011033 co-funded by the European Social Fund (ESF) & ACOND/2020 Generalitat Valenciana].
Conflict of Interest
AG, IM, and FB are employed by the companies Centro Sperimentale del Latte S.r.l., Sacco S.r.l., and VRM S.r.l, respectively.
The remaining authors declare that the research was conducted in the absence of any commercial or financial relationships that could be construed as a potential conflict of interest.
Acknowledgments
Special thanks to Micaela Antonini at the Aquaculture Unit of the University of Insubria for her assistance with the histological platform. FM is a Ph.D. student of the “Dottorato in Scienze della Vita e Biotecnologie” at the “Università degli Studi dell’Insubria,” Varese, Italy.
Supplementary Material
The Supplementary Material for this article can be found online at: https://www.frontiersin.org/articles/10.3389/fmars.2021.659519/full#supplementary-material
Supplementary Figure 1 | (A) Relative abundance of phyla in control (Diet A) and experimental diets (Diets B and C). (B) Relative abundance of phyla excluding Cyanobacteria/Chloroplast in control and experimental diets. (C) Relative abundance of Firmicutes genera, including Lactococcus lactis subsp. lactis in control and experimental diets.
Supplementary Figure 2 | Rarefaction curves obtained from the sequencing data of the different groups included in this study (Diets A, B, C).
Supplementary Figure 3 | Validation plot of the PLS-DA model between control (Diet A) and experimental (Diets B and C) groups, consisting of 600 random permutations.
Supplementary Table 1 | Primers for qPCR gene expression. Colors in letters of column Gene show different gene functions included in the array as follows: red: Epithelial integrity; dark blue: Nutrient transport; green: Mucus production; Orange: Cytokines; purple: Immunoglobulins; black: Cell markers and chemokines; light blue: Pattern recognition receptors.
Supplementary Table 2 | Relative gene expression of anterior intestine in fish fed control (A) and experimental (B,C) diets. Data are the mean ± SEM of 9–12 fish. All data values were referred to the expression level of hes1-b of control fish with an arbitrary assigned value of 1. 1P-value results from ANOVA. Bold font indicates statistically significant differences between groups (P < 0.05).
Supplementary Table 3 | Sequencing and taxonomy assignment results.
Supplementary Table 4 | OTUs driving the separation between fish fed probiotic diets (B + C) and fish fed control diet A in the PLS-DA model (VIP > 1).
Footnotes
References
Abd El-Rhman, A. M., Khattab, Y. A. E., and Shalaby, A. M. E. (2009). Micrococcus luteus and Pseudomonas species as probiotics for promoting the growth performance and health of Nile tilapia, Oreochromis niloticus. Fish Shellfish Immunol. 27, 175–180. doi: 10.1016/j.fsi.2009.03.020
Abdelhamid, A. M., Mehrim, A. I., El-Barbary, M. I., Ibrahim, S. M., and El-Wahab, A. I. A. (2009). Evaluation of a New Egyptian Probiotic by African Catfish Fingerlings. J. Environ. Sci. Technol. 2, 133–145. doi: 10.3923/jest.2009.133.145
Adel, M., El-Sayed, A.-F. M., Yeganeh, S., Dadar, M., and Giri, S. S. (2017). Effect of Potential Probiotic Lactococcus lactis Subsp. lactis on Growth Performance, Intestinal Microbiota, Digestive Enzyme Activities, and Disease Resistance of Litopenaeus vannamei. Probiotics Antimicrob. Proteins 9, 150–156.
Al-Dohail, M. A., Hashim, R., and Aliyu-Paiko, M. (2009). Effects of the probiotic, Lactobacillus acidophilus, on the growth performance, haematology parameters and immunoglobulin concentration in African Catfish (Clarias gariepinus, Burchell 1822) fingerling. Aquac. Res. 40, 1642–1652. doi: 10.1111/j.1365-2109.2009.02265.x
Altschul, S. F., Gish, W., Miller, W., Myers, E. W., and Lipman, D. J. (1990). Basic local alignment search tool. J. Mol. Biol. 215, 403–410.
Bakke-Mckellep, A. M., Penn, M. H., Salas, P. M., Refstie, S., Sperstad, S., Landsverk, T., et al. (2007). Effects of dietary soyabean meal, inulin and oxytetracycline on intestinal microbiota and epithelial cell stress, apoptosis and proliferation in the teleost Atlantic salmon (Salmo salar L.). Br. J. Nutr. 97, 699–713. doi: 10.1017/S0007114507381397
Balcázar, J. L., de Blas, I., Ruiz-Zarzuela, I., Cunningham, D., Vendrell, D., and Múzquiz, J. L. (2006a). The role of probiotics in aquaculture. Vet. Microbiol. 114, 173–186. doi: 10.1016/j.vetmic.2006.01.009
Balcázar, J. L., de Blas, I., Ruiz-Zarzuela, I., Vendrell, D., Calvo, A. C., Márquez, I., et al. (2007a). Changes in intestinal microbiota and humoral immune response following probiotic administration in brown trout (Salmo trutta). Br. J. Nutr. 97, 522–527. doi: 10.1017/S0007114507432986
Balcázar, J. L., Vendrell, D., de Blas, I., Ruiz-Zarzuela, I., Gironés, O., and Muzquiz, J. L. (2006b). Immune modulation by probiotic strains: quantification of phagocytosis of Aeromonas salmonicida by leukocytes isolated from gut of rainbow trout (Oncorhynchus mykiss) using a radiolabelling assay. Comp. Immunol. Microbiol. Infect. Dis. 29, 335–343. doi: 10.1016/j.cimid.2006.09.004
Balcázar, J. L., Vendrell, D., de Blas, I., Ruiz-Zarzuela, I., Gironés, O., and Múzquiz, J. L. (2007b). In vitro competitive adhesion and production of antagonistic compounds by lactic acid bacteria against fish pathogens. Vet. Microbiol. 122, 373–380. doi: 10.1016/j.vetmic.2007.01.023
Batista, S., Medina, A., Pires, M. A., Moriñigo, M. A., Sansuwan, K., Fernandes, J. M. O., et al. (2016). Innate immune response, intestinal morphology and microbiota changes in Senegalese sole fed plant protein diets with probiotics or autolysed yeast. Appl. Microbiol. Biotechnol. 100, 7223–7238.
Bledsoe, J. W., Peterson, B. C., Swanson, K. S., and Small, B. C. (2016). Ontogenetic characterization of the intestinal microbiota of channel Catfish through 16S rRNA gene sequencing reveals insights on temporal shifts and the influence of environmental microbes. PLoS One 11:e0166379. doi: 10.1371/journal.pone.0166379
Borch, K., Pederson, I. E., and Hogmo, R. O. (2015). The use of probiotics in fish feed for intensive aquaculture to promote healthy guts. Adv. Aquac. Fish. Manag. 3, 264–273.
Cabello, F. C. (2006). Heavy use of prophylactic antibiotics in aquaculture: a growing problem for human and animal health and for the environment. Environ. Microbiol. 8, 1137–1144. doi: 10.1111/j.1462-2920.2006.01054.x
Carnevali, O., de Vivo, L., Sulpizio, R., Gioacchini, G., Olivotto, I., Silvi, S., et al. (2006). Growth improvement by probiotic in European sea bass juveniles (Dicentrarchus labrax, L.), with particular attention to IGF-1, myostatin and cortisol gene expression. Aquaculture 258, 430–438. doi: 10.1016/j.aquaculture.2006.04.025
Casewell, M., Friis, C., Marco, E., McMullin, P., and Phillips, I. (2003). The European ban on growth-promoting antibiotics and emerging consequences for human and animal health. J. Antimicrob. Chemother. 52, 159–161. doi: 10.1093/jac/dkg313
Cerezuela, R., Fumanal, M., Tapia-paniagua, S. T., Meseguer, J., Moriñigo, Á. M., and Esteban, Á. M. (2012). Histological alterations and microbial ecology of the intestine in gilthead seabream (Sparus aurata L.) fed dietary probiotics and microalgae. Cell Tissue Res. 350, 477–489.
Cerezuela, R., Fumanal, M., Tapia-paniagua, S. T., Meseguer, J., Moriñigo, Á. M., and Esteban, Á. M. (2013). Changes in intestinal morphology and microbiota caused by dietary administration of inulin and Bacillus subtilis in gilthead sea bream (Sparus aurata L.) specimens. Fish Shellfish Immunol. 34, 1063–1070. doi: 10.1016/j.fsi.2013.01.015
Chaucheyras-Durand, F., and Durand, H. (2010). Probiotics in animal nutrition and health. Benef. Microbes 1, 3–9. doi: 10.3920/BM2008.1002
Chauhan, A., and Singh, R. (2019). Probiotics in aquaculture: a promising emerging alternative approach. Symbiosis 77, 99–113.
Cole, J. R., Wang, Q., Fish, J. A., Chai, B., McGarrell, D. M., Sun, Y., et al. (2014). Ribosomal database project: data and tools for high throughput rRNA analysis. Nucleic Acids Res. 42, D633–D642. doi: 10.1093/nar/gkt1244
Collado, M. C., Meriluoto, J., and Salminen, S. (2007). Role of commercial probiotic strains against human pathogen adhesion to intestinal mucus. Lett. Appl. Microbiol. 45, 454–460. doi: 10.1111/j.1472-765X.2007.02212.x
Dahiya, T., Ravikant, Singh, G., Singh, R., and Singh, S. (2020). Applications and possible modes of action of probiotics in aquaculture. Int. J. Agric. Sci. 12, 9753–9755.
Dammermann, W., Wollenberg, L., Bentzien, F., Lohse, A., and Luth, S. (2013). Toll like receptor 2 agonists lipoteichoic acid and peptidoglycan are able to enhance antigen specific IFNgamma release in whole blood during recall antigen responses. J. Immunol. Methods 396, 107–115. doi: 10.1016/j.jim.2013.08.004
De Cesare, A., Sirri, F., Manfreda, G., Moniaci, P., Giardini, A., Zampiga, M., et al. (2017). Effect of dietary supplementation with Lactobacillus acidophilus D2/CSL (CECT 4529) on caecum microbioma and productive performance in broiler chickens. PLoS One 12:e0176309. doi: 10.1371/journal.pone.0176309
Dimitroglou, A., Merrifield, D. L., Carnevali, O., Picchietti, S., Avella, M., Daniels, C., et al. (2011). Microbial manipulations to improve fish health and production - A Mediterranean perspective. Fish Shellfish Immunol. 30, 1–16. doi: 10.1016/j.fsi.2010.08.009
Dowarah, R., Verma, A. K., Agarwal, N., Singh, P., and Singh, B. R. (2018). Selection and characterization of probiotic lactic acid bacteria and its impact on growth, nutrient digestibility, health and antioxidant status in weaned piglets. PLoS One 13:e0192978.
EFSA FEEDAP [EFSA Panel on Additives and Products or Substances used in Animal Feed], Rychen, G., Aquilina, G., Azimonti, G., Bampidis, V., Bastos, M. L., et al. (2018). Guidance on the characterisation of microorganisms used as feed additives or as production organisms. EFSA J. 16:5206. doi: 10.2903/j.efsa.2018.5206
EFSA Panel on Biological Hazards (BIOHAZ), Koutsoumanis, K., Allende, A., Alvarez-Ordóñez, A., Bolton, D., Bover-Cid, S., et al. (2020). Update of the list of QPS-recommended biological agents intentionally added to food or feed as notified to EFSA 12: suitability of taxonomic units notified to EFSA until March 2020. EFSA J. 18, 1–41. doi: 10.2903/j.efsa.2020.6174
Egerton, S., Culloty, S., Whooley, J., Stanton, C., and Ross, R. P. (2018). The gut microbiota of marine fish. Front. Microbiol. 9:873. doi: 10.3389/fmicb.2018.00873
Estensoro, I., Ballester-Lozano, G., Benedito-Palos, L., Grammes, F., Martos-Sitcha, J. A., Mydland, L.-T., et al. (2016). Dietary butyrate helps to restore the intestinal status of a marine Teleost (Sparus aurata) fed extreme diets low in fish meal and fish oil. PLoS One 11:e0166564. doi: 10.1371/journal.pone.0166564
Estruch, G., Collado, M. C., Peñaranda, D. S., Tomás Vidal, A., Jover Cerdá, M., Pérez Martínez, G., et al. (2015). Impact of fishmeal replacement in diets for gilthead sea bream (Sparus aurata) on the gastrointestinal microbiota determined by pyrosequencing the 16S rRNA gene. PLoS One 10:0136389. doi: 10.1371/journal.pone.0136389
European Medicines Agency [EMA], and European Food Safety Authority [EFSA] (2017). EMA, and EFSA Joint Scientific Opinion on measures to reduce the need to use antimicrobial agents in animal husbandry in the European Union, and the resulting impacts on food safety (RONAFA). EFSA J. 15:4666. doi: 10.2903/j.efsa.2017.4666
European Parliament and the Council of the European Union (2003). Regulation (EC) No 1831/2003. Off. J. Eur. Union 4, 29–43.
European Parliament and the Council of the European Union (2019). Regulation (EU) 2019/6 of the European Parliament and of the Council of 11 December 2018 on veterinary medicinal products and repealing Directive 2001/82/EC. Off. J. Eur. Union L4/43, 43–167.
European Safety Food Authority [EFSA] (2005). Opinion of the scientific panel on food additives, flavourings, processing aids and materials in contact with food on a request from the commission related to the use of nisin (E 234) as a food additive. EFSA J. 314, 1–16. doi: 10.2903/j.efsa.2004.35
Ezema, C. (2013). Probiotics in animal production: a review. J. Vet. Med. Anim. Heal. 5, 308–316. doi: 10.5897/JVMAH2013.0201
Feng, J., Chang, X., Zhang, Y., Yan, X., Zhang, J., and Nie, G. (2019). Effects of Lactococcus lactis from Cyprinus carpio L. as probiotics on growth performance, innate immune response and disease resistance against Aeromonas hydrophila. Fish Shellfish Immunol. 93, 73–81. doi: 10.1016/j.fsi.2019.07.028
Food and Agriculture Organization of the United [FAO] (2016). Probiotics in Animal Nutrition. Rome: FAO.
Food and Agriculture Organization of the United [FAO], and World Health Organisation [WHO] (2001). Probiotics in Food. Report of a Joint FAO/WHO Expert Consultation on Evaluation of Health and Nutritional Properties of Probiotics in Food Including Powder Milk with Live Lactic acid Bacteria. Cordoba: FAO.
Forte, C., Manuali, E., Abbate, Y., Papa, P., Vieceli, L., Tentellini, M., et al. (2018). Dietary Lactobacillus acidophilus positively influences growth performance, gut morphology, and gut microbiology in rurally reared chickens. Poult. Sci. 97, 930–936. doi: 10.3382/ps/pex396
Gallazzi, D., Giardini, A., Mangiagalli, M. G., Marelli, S., Ferrazzi, V., Orsi, C., et al. (2008). Effects of Lactobacillus acidophilus D2/CSL on laying hen performance. Ital. J. Anim. Sci. 7, 27–37. doi: 10.4081/ijas.2008.27
Ghanbari, M., Kneifel, W., and Domig, K. J. (2015). A new view of the fish gut microbiome: advances from next-generation sequencing. Aquaculture 448, 464–475. doi: 10.1016/j.aquaculture.2015.06.033
Givens, C. E., Ransom, B., Bano, N., and Hollibaugh, J. T. (2015). Comparison of the gut microbiomes of 12 bony fish and 3 shark species. Mar. Ecol. Prog. Ser. 518, 209–223. doi: 10.3354/meps11034
Grazul, H., Kanda, L. L., and Gondek, D. (2016). Impact of probiotic supplements on microbiome diversity following antibiotic treatment of mice. Gut Microbes 7, 101–114. doi: 10.1080/19490976.2016.1138197
Green, T. J., Smullen, R., and Barnes, A. C. (2013). Dietary soybean protein concentrate-induced intestinal disorder in marine farmed Atlantic salmon, Salmo salar is associated with alterations in gut microbiota. Vet. Microbiol. 166, 286–292. doi: 10.1016/j.vetmic.2013.05.009
Gupta, S., Feckaninová, A., Lokesh, J., Koscova, J., Sorensen, M., Fernandes, J., et al. (2019). Lactobacillus dominate in the intestine of atlantic salmon fed dietary probiotics. Front. Microbiol. 9:3247. doi: 10.3389/fmicb.2018.03247
Hamid, A. H. T., Zahli, K. I. A., and Alotaibi, M. (2020). Lactococcus lactis strains from intestinal organ of black tips shark carcharhinus limbatus producing nisin-like bacteriocin active against shrimp and fish pathogens (Vibrio parahaemolyticus and Vibrio alginolyticus). J. Microbiol. Biotechnol. Food Sci. 354–360.
Heo, W. S., Kim, Y. R., Kim, E. Y., Bai, S. C., and Kong, I. S. (2013). Effects of dietary probiotic, Lactococcus lactis subsp. lactis I2, supplementation on the growth and immune response of olive flounder (Paralichthys olivaceus). Aquaculture 376–379, 20–24. doi: 10.1016/j.aquaculture.2012.11.009
Hidalgo, M. C., Skalli, A., Abellán, E., Arizcun, M., and Cardenete, G. (2006). Dietary intake of probiotics and maslinic acid in juvenile dentex (Dentex dentex L.): effects on growth performance, survival and liver proteolytic activities. Aquac. Nutr. 12, 256–266. doi: 10.1111/j.1365-2095.2006.00408.x
Iwai, S., Weinmaier, T., Schmidt, B. L., Albertson, D. G., Poloso, N. J., Dabbagh, K., et al. (2016). Piphillin: improved prediction of metagenomic content by direct inference from human microbiomes. PLoS One 11:e0166104. doi: 10.1371/journal.pone.0166104
Jahangiri, L., and Esteban, M. Á. (2018). Administration of probiotics in the water in finfish aquaculture systems: a review. Fishes 3:33. doi: 10.3390/fishes303003
Khojasteh, B. M. S. (2012). The morphology of the post-gastric alimentary canal in teleost fishes: a brief review. Int. J. Aquat. Sci. 3, 71–88.
Kim, S., Bhatnagar, I., and Kang, K. (2012). Development of marine probiotics: prospects and approach. Adv. Food Nutr. Res. 65, 353–362.
Knudsen, D., Urán, P., Arnous, A., Koppe, W., and Frøkiaer, H. (2007). Saponin-containing subfractions of soybean molasses induce enteritis in the distal intestine of Atlantic salmon. J. Agric. Food Chem. 55, 2261–2267. doi: 10.1021/jf0626967
Kokou, F., Sasson, G., Mizrahi, I., and Cnaani, A. (2020). Antibiotic effect and microbiome persistence vary along the European seabass gut. Sci. Rep. 10:10003.
Kormas, K. A., Meziti, A., Mente, E., and Frentzos, A. (2014). Dietary differences are reflected on the gut prokaryotic community structure of wild and commercially reared sea bream (Sparus aurata). Microbiologyopen 3, 718–728. doi: 10.1002/mbo3.202
Lazado, C. C., and Caipang, C. M. A. (2014). Mucosal immunity and probiotics in fish. Fish Shellfish Immunol. 39, 78–89.
Li, H., Ma, M. L., Luo, S., Zhang, R. M., Han, P., and Hu, W. (2012). Metabolic responses to ethanol in Saccharomyces cerevisiae using a gas chromatography tandem mass spectrometry-based metabolomics approach. Int. J. Biochem. Cell Biol. 44, 1087–1096. doi: 10.1016/j.biocel.2012.03.017
Li, J., Ni, J., Li, J., Wang, C., Li, X., Wu, S., et al. (2014). Comparative study on gastrointestinal microbiota of eight fish species with different feeding habits. J. Appl. Microbiol. 117, 1750–1760. doi: 10.1111/jam.12663
Li, Q., Montalban-lopez, M., and Kuipers, P. O. (2018). Increasing the antimicrobial activity of nisin-based lantibiotics against gram-negative pathogens. Appl. Environ. Microbiol. 84:e00052.
Lin, H. L., Shiu, Y. L., Chiu, C. S., Huang, S. L., and Liu, C. H. (2017). Screening probiotic candidates for a mixture of probiotics to enhance the growth performance, immunity, and disease resistance of Asian seabass, Lates calcarifer (Bloch), against Aeromonas hydrophila. Fish Shellfish Immunol. 60, 474–482. doi: 10.1016/j.fsi.2016.11.026
Lin, H. Z., Guo, Z., Yang, Y., Zheng, W., and Li, Z. J. (2004). Effect of dietary probiotics on apparent digestibility coefficients of nutrients of white shrimp Litopenaeus vannamei Boone. Aquac. Res. 35, 1441–1447. doi: 10.1111/j.1365-2109.2004.01169.x
Livak, K. J., and Schmittgen, T. D. (2001). Analysis of relative gene expression data using real-time quantitative PCR and the 2-ΔΔCT method. Methods 25, 402–408. doi: 10.1006/meth.2001.1262
Love, M. I., Huber, W., and Anders, S. (2014). Moderated estimation of fold change and dispersion for RNA-seq data with DESeq2. Genome Biol. 15:550.
Macey, B. M., and Coyne, V. E. (2005). Improved growth rate and disease resistance in farmed Haliotis midae through probiotic treatment. Aquaculture 245, 249–261. doi: 10.1016/j.aquaculture.2004.11.031
Magne, F., Gotteland, M., Gauthier, L., Zazueta, A., Pesoa, S., Navarrete, P., et al. (2020). The firmicutes/bacteroidetes ratio: a relevant marker of gut dysbiosis in obese patients? Nutrients 12:1474. doi: 10.3390/nu12051474
Mahdhi, A. (2012). Probiotic properties of Brevibacillus brevis and its influence on sea bass (Dicentrarchus labrax) larval rearing. Afr. J. Microbiol. Res. 6, 6487–6495. doi: 10.5897/AJMR12.1201
Malvisi, M., Stuknyte, M., Magro, G., Minozzi, G., Giardini, A., De Noncty, I., et al. (2016). Antibacterial activity and immunomodulatory effects on a bovine mammary epithelial cell line exerted by nisin A-producing Lactococcus lactis strains. J. Dairy Sci. 99, 2288–2296.
Marco, M. L. (2019). Is Probiotic Colonization Essential? - International Scientific Association for Probiotics and Prebiotics (ISAPP). Available online at: https://isappscience.org/is-probiotic-colonization-essential/ (accessed January 22, 2021).
Martínez Cruz, P., Ibáñez, A. L., Monroy Hermosillo, O. A., and Ramírez Saad, H. C. (2012). Use of probiotics in aquaculture. ISRN Microbiol. 2012:916845. doi: 10.5402/2012/916845
McKnight, D. T., Huerlimann, R., Bower, D. S., Schwarzkopf, L., Alford, R. A., and Zenger, K. R. (2019). Methods for normalizing microbiome data: an ecological perspective. Methods Ecol. Evol. 10, 389–400. doi: 10.1111/2041-210X.13115
Mcmurdie, P. J., and Holmes, S. (2013). phyloseq: an R package for reproducible interactive analysis and graphics of microbiome census data. PLoS One 8:e0061217. doi: 10.1371/journal.pone.0061217
Merrifield, D. L., Bradley, G., Baker, R. T. M., and Davies, S. J. (2010). Probiotic applications for rainbow trout (Oncorhynchus mykiss Walbaum) II. Effects on growth performance, feed utilization, intestinal microbiota and related health criteria postantibiotic treatment. Aquac. Nutr. 16, 496–503. doi: 10.1111/j.1365-2095.2009.00688.x
Nayak, S. K. (2010). Probiotics and immunity: a fish perspective. Fish Shellfish Immunol. 29, 2–14. doi: 10.1016/j.fsi.2010.02.017
Newaj-Fyzul, A., Al-Harbi, A. H., and Austin, B. (2014). Review: developments in the use of probiotics for disease control in aquaculture. Aquaculture 431, 1–11. doi: 10.1016/j.aquaculture.2013.08.026
Nguyen, T. L., Park, C.-I., and Kim, D.-H. (2017). Improved growth rate and disease resistance in olive flounder, Paralichthys olivaceus, by probiotic Lactococcus lactis WFLU12 isolated from wild marine fish. Aquaculture 471, 113–120. doi: 10.1016/j.aquaculture.2017.01.008
Perez, R. H., Zendo, T., and Sonomoto, K. (2014). Novel bacteriocins from lactic acid bacteria (LAB): various structures and applications. Microb. Cell Fact. 13(Suppl. 1):S3.
Pérez-Sánchez, T., Balcázar, J. L., Merrifield, D. L., Carnevali, O., Gioacchini, G., de Blas, I., et al. (2011). Expression of immune-related genes in rainbow trout (Oncorhynchus mykiss) induced by probiotic bacteria during Lactococcus garvieae infection. Fish Shellfish Immunol. 31, 196–201. doi: 10.1016/j.fsi.2011.05.005
Piazzon, M. C., Calduch-giner, J. A., Fouz, B., Estensoro, I., Simó-Mirabet, P., Puyalto, M., et al. (2017). Under control: how a dietary additive can restore the gut microbiome and proteomic profile, and improve disease resilience in a marine teleostean fish fed vegetable diets. Microbiome 5:164.
Piazzon, M. C., Naya-català, F., Perera, E., Palenzuela, O., Sitjà-bobadilla, A., and Pérez-Sánchez, J. (2020). Genetic selection for growth drives differences in intestinal microbiota composition and parasite disease resistance in gilthead sea bream. Microbiome 8:168. doi: 10.1186/s40168-020-00922-w
Piazzon, M. C., Naya-català, F., Simó-Mirabet, P., Picard-sánchez, A., Roig, F. J., Calduch-giner, J. A., et al. (2019). Sex, age, and bacteria: how the intestinal microbiota is modulated in a protandrous hermaphrodite fish. Front. Microbiol. 10:2512. doi: 10.3389/fmicb.2019.02512
Puphan, K., Sornplang, P., Uriyapongson, S., and Navanukraw, C. (2015). Screening of lactic acid bacteria as potential probiotics in beef cattle. Pak. J. Nutr. 14, 474–479. doi: 10.3923/pjn.2015.474.479
Rahlwes, K. C., Sparks, I. L., and Morita, Y. S. (2019). Cell Walls and membranes of actinobacteria. Subcell Biochem. 92, 417–469.
Ridha, M. T., and Azad, I. S. (2012). Preliminary evaluation of growth performance and immune response of Nile tilapia Oreochromis niloticus supplemented with two putative probiotic bacteria. Aquac. Res. 43, 843–852. doi: 10.1111/j.1365-2109.2011.02899.x
Rimoldi, S., Gini, E., Iannini, F., Gasco, L., and Terova, G. (2019). The effects of dietary insect meal from Hermetia illucens prepupae on autochthonous gut microbiota of rainbow trout (Oncorhynchus mykiss). Animals 9:143. doi: 10.3390/ani9040143
Rimoldi, S., Gini, E., Koch, J. F. A., Iannini, F., Brambilla, F., and Terova, G. (2020). Effects of hydrolyzed fish protein and autolyzed yeast as substitutes of fishmeal in the gilthead sea bream (Sparus aurata) diet, on fish intestinal microbiome. BMC Vet. Res. 16:118. doi: 10.1186/s12917-020-02335-1
Ringø, E. (2008). The ability of carnobacteria isolated from fish intestine to inhibit growth of fish pathogenic bacteria: a screening study. Aquac. Res. 39, 171–180. doi: 10.1111/j.1365-2109.2007.01876.x
Ringø, E., Van Doan, H., Lee, S. H., Soltani, M., Hoseinifar, S. H., Harikrishnan, R., et al. (2020). Probiotics, lactic acid bacteria and bacilli: interesting supplementation for aquaculture. J. Appl. Microbiol. 129, 116–136. doi: 10.1111/jam.14628
Ringø, E., Zhou, Z., Vecino, J. L. G., Wadsworth, S., Romero, J., Krogdahl, Å., et al. (2016). Effect of dietary components on the gut microbiota of aquatic animals. A never-ending story? Aquac. Nutr. 22, 219–282. doi: 10.1111/anu.12346
Rognes, T., Flouri, T., Nichols, B., Quince, C., and Mahé, F. (2016). VSEARCH: a versatile open source tool for metagenomics. PeerJ 4:e2584. doi: 10.7717/peerj.2584
Salinas, I., Abelli, L., Bertoni, F., Picchietti, S., Roque, A., Furones, D., et al. (2008). Monospecies and multispecies probiotic formulations produce different systemic and local immunostimulatory effects in the gilthead seabream (Sparus aurata L.). Fish Shellfish Immunol. 25, 114–123. doi: 10.1016/j.fsi.2008.03.011
Samaržija, D., Antunac, N., and Havranek, L. J. (2001). Taxonomy, physiology and growth of Lactococcus lactis: a review. Mljekarstvo 51, 35–48.
Shabani, R., Nosrati, M., Javandel, F., Ahmad, A., Gothbi, A., and Kioumarsi, H. (2012). The effect of probiotics on growth performance of broilers. Ann. Biol. Res. 3, 5450–5452.
Silva, F. C. D. P., Nicoli, J. R., Zambonino-Infante, J. L., Kaushik, S., and Gatesoupe, F.-J. (2011). Infuence of the diet on the microbial diversity of faecal and gastrointestinal contents in gilthead sea bream(Sparus aurata) and intestinal contents in goldfish (Carassius auratus). FEMS Microbiol. Ecol. 78, 285–296. doi: 10.1111/j.1574-6941.2011.01155.x
Simó-Mirabet, P., Piazzon, M. C., Calduch-Giner, J. A., Ortiz, Á., Puyalto, M., Sitjà-bobadilla, A., et al. (2017). Sodium salt medium-chain fatty acids and Bacillus -based probiotic strategies to improve growth and intestinal health of gilthead sea bream (Sparus aurata). PeerJ 5:e4001. doi: 10.7717/peerj.4001
Sugimura, Y., Hagi, T., and Hoshino, T. (2011). Correlation between in vitro mucus adhesion and the in vivo colonization ability of lactic acid bacteria: screening of new candidate carp probiotics. Biosci. Biotechnol. Biochem. 75, 511–515. doi: 10.1271/bbb.100732
Sun, Y. Z., Yang, H. L., Ma, R. L., Song, K., and Li, J. S. (2012). Effect of Lactococcus lactis and Enterococcus faecium on growth performance, digestive enzymes and immune response of grouper Epinephelus coioides. Aquac. Nutr. 18, 281–289. doi: 10.1111/j.1365-2095.2011.00894.x
Suzer, C., Çoban, D., Kamaci, H. O., Saka, Ş, Firat, K., Otgucuoǧlu, Ö., et al. (2008). Lactobacillus spp. bacteria as probiotics in gilthead sea bream (Sparus aurata, L.) larvae: effects on growth performance and digestive enzyme activities. Aquaculture 280, 140–145. doi: 10.1016/j.aquaculture.2008.04.020
Tarnecki, A. M., Burgos, F. A., Ray, C. L., and Arias, C. R. (2017). Fish intestinal microbiome: diversity and symbiosis unravelled by metagenomics. J. Appl. Microbiol. 123, 2–17. doi: 10.1111/jam.13415
Terova, G., Rimoldi, S., Ascione, C., Gini, E., Ceccotti, C., and Gasco, L. (2019). Rainbow trout (Oncorhynchus mykiss) gut microbiota is modulated by insect meal from Hermetia illucens prepupae in the diet. Rev. Fish Biol. Fish. 29, 465–486. doi: 10.1007/s11160-019-09558-y
Unno, T., Kim, J., Guevarra, R. B., and Nguyen, S. G. (2015). Effects of antibiotic growth promoter and characterization of ecological succession in swine gut microbiota. J. Microbiol. Biotechnol. 25, 431–438. doi: 10.4014/jmb.1408.08063
Uran, P. A., Schrama, J. W., Rombout, J. H. W. M., Obach, A., Jensen, L., Koppe, W., et al. (2008). Soybean meal-induced enteritis in Atlantic salmon(Salmo salar L.) at different temperatures. Aquac. Nutr. 14, 324–330. doi: 10.1111/j.1365-2095.2007.00534.x
Urán, P. A., Schrama, J. W., Rombout, J. H. W. M., Taverne-Thiele, J. J., Obach, A., Koppe, W., et al. (2009). Time-related changes of the intestinal morphology of Atlantic salmon, Salmo salar L., at two different soybean meal inclusion levels. J. Fish Dis. 32, 733–744. doi: 10.1111/j.1365-2761.2009.01049.x
Ustyugova, E. A., Timofeeva, A. V., Stoyanova, L. G., Netrusov, A. I., and Katrukha, G. S. (2012). Characteristics and Identification of Bacteriocins Produced by Lactococcus lactis subsp. lactis 194-K. Appl. Biochem. Microbiol. 48, 557–563. doi: 10.1134/S0003683812060105
Uyeno, Y., Shigemori, S., and Shimosato, T. (2015). Effect of probiotics/prebiotics on cattle health and productivity. Microbes Environ. 30, 126–132. doi: 10.1264/jsme2.ME14176
Varela, J. L., Ruiz-Jarabo, I., Vargas-Chacoff, L., Arijo, S., León-Rubio, J. M., García-Millán, I., et al. (2010). Dietary administration of probiotic Pdp11 promotes growth and improves stress tolerance to high stocking density in gilthead seabream Sparus auratus. Aquaculture 309, 265–271. doi: 10.1016/j.aquaculture.2010.09.029
Villamil, L., Tafalla, C., Figueras, A., and Novoa, B. (2002). Evaluation of immunomodulatory effects of lactic acid bacteria in turbot (Scophthalmus maximus). Clin. Diagn. Lab. Immunol. 9, 1318–1323. doi: 10.1128/CDLI.9.6.1318-1323.2002
Wang, Y., Sun, J., Zhong, H., Li, N., Xu, H., Zhu, Q., et al. (2017). Effect of probiotics on the meat flavour and gut microbiota of chicken. Sci. Rep. 7:6400. doi: 10.1038/s41598-017-06677-z
Wold, S., Sjöström, M., and Eriksson, L. (2001). PLS-regression: a basic tool of chemometrics. Chemom. Intell. Lab. Syst. 58, 109–130.
Won, S., Hamidoghli, A., Choi, W., Park, Y., Jang, W. J., Kong, I.-S., et al. (2020). Effects of Bacillus subtilis wb60 and Lactococcus lactis on growth, immune responses, histology and gene expression in Nile Tilapia, Oreochromis niloticus. Microorganisms 8:67. doi: 10.3390/microorganisms8010067
World Health Organisation [WHO], Food and Agriculture Organization of the United Nations [FAO], and World Organisation for Animal Health [OIE] (2006). Antimicrobial Use in Aquaculture and Antimicrobial Resistance. Geneva: WHO.
Xia, Y., Lu, M., Chen, G., Cao, J., Gao, F., Wang, M., et al. (2018). Effects of dietary Lactobacillus rhamnosus JCM1136 and Lactococcus lactis subsp. lactis JCM5805 on the growth, intestinal microbiota, morphology, immune response and disease resistance of juvenile Nile tilapia, Oreochromis niloticus. Fish Shellfish Immunol. 76, 368–379. doi: 10.1016/j.fsi.2018.03.020
Yang, H. L., Sun, Y. Z., Hu, X., Ye, J., Lu, K. L., Hu, L. H., et al. (2019). Bacillus pumilus SE5 originated PG and LTA tuned the intestinal TLRs/MyD88 signaling and microbiota in grouper (Epinephelus coioides). Fish Shellfish Immunol. 88, 266–271. doi: 10.1016/j.fsi.2019.03.005
Yang, H. L., Xia, H. Q., Ye, J. D., Zou, W. C., and Sun, Y. Z. (2014). Probiotic Bacillus pumilus SE5 shapes the intestinal microbiota and mucosal immunity in grouper Epinephelus coioides. Dis. Aquat. Org. 111, 119–127. doi: 10.3354/dao02772
Zhou, Q.-C., Buentello, J. A., and Gatlin, D. M. III (2010). Effects of dietary prebiotics on growth performance, immune response and intestinal morphology of red drum (Sciaenops ocellatus). Aquaculture 309, 253–257. doi: 10.1016/j.aquaculture.2010.09.003
Keywords: aquaculture, gilthead sea bream, probiotic, Lactococcus lactis, gut microbiota, transcriptomic
Citation: Moroni F, Naya-Català F, Piazzon MC, Rimoldi S, Calduch-Giner J, Giardini A, Martínez I, Brambilla F, Pérez-Sánchez J and Terova G (2021) The Effects of Nisin-Producing Lactococcus lactis Strain Used as Probiotic on Gilthead Sea Bream (Sparus aurata) Growth, Gut Microbiota, and Transcriptional Response. Front. Mar. Sci. 8:659519. doi: 10.3389/fmars.2021.659519
Received: 27 January 2021; Accepted: 22 March 2021;
Published: 12 April 2021.
Edited by:
Fotini Kokou, Wageningen University and Research, NetherlandsReviewed by:
Carlo C. Lazado, Norwegian Institute of Food, Fisheries and Aquaculture Research (Nofima), NorwayYun-Zhang Sun, Jimei University, China
Copyright © 2021 Moroni, Naya-Català, Piazzon, Rimoldi, Calduch-Giner, Giardini, Martínez, Brambilla, Pérez-Sánchez and Terova. This is an open-access article distributed under the terms of the Creative Commons Attribution License (CC BY). The use, distribution or reproduction in other forums is permitted, provided the original author(s) and the copyright owner(s) are credited and that the original publication in this journal is cited, in accordance with accepted academic practice. No use, distribution or reproduction is permitted which does not comply with these terms.
*Correspondence: Genciana Terova, Z2VuY2lhbmEudGVyb3ZhQHVuaW5zdWJyaWEuaXQ=
†These authors have contributed equally to this work