Symbiont composition and coral genotype determines massive coral species performance under end-of-century climate scenarios
- 1Mote Marine Laboratory, International Center for Coral Reef Research and Restoration, Summerland Key, FL, United States
- 2Cooperative Institute for Marine and Atmospheric Studies, University of Miami, Miami, FL, United States
- 3National Oceanographic and Atmospheric Administration (NOAA) Atlantic Oceanographic and Meteorological Laboratory, Ocean Chemistry and Ecosystems Division, Miami, FL, United States
- 4National Coral Reef Institute, Nova Southeastern University, Dania Beach, FL, United States
- 5Mote Marine Laboratory, Sarasota, FL, United States
The recent decline of coral health and substantial loss of coral cover along Florida’s Coral Reef (FCR) results from local stressors such as degraded water quality and disease outbreaks in addition to anthropogenically driven global stressors including ocean warming and acidification. Intervention strategies intended for the restoration of degraded reef habitats need a better understanding of the influence of ocean warming and acidification on coral health to target coral species and individual genotypes that may be more resistant or resilient to such stressors. Here, we examined a suite of physiological traits (coral host and algal symbiont) in response to experimentally elevated water temperatures and pCO2 levels, both separately and in concert, using threatened reef-building corals Pseudodiploria clivosa and Orbicella faveolata reared within a land-based coral nursery. After two months of exposure, responses differed by coral species, where P. clivosa showed declined physiology in response to combined ocean warming and acidification stress and ocean warming alone, whereas O. faveolata showed a positive response under ocean acidification. Responses to temperature could be associated with the algal symbionts harbored, as P. clivosa was dominated by the thermally sensitive Breviolum, and O. faveolata was dominated by the thermally tolerant Durusdinium. Additionally, corals were raised in well-sourced seawater that was naturally high in pCO2, which could have led to corals acclimating to acidified conditions. Of the three P. clivosa genets tested, we determined a top-performing genotype under the combined warming and acidification treatment. O. faveolata, however, displayed high genet variation by treatment and phenotypic trait, making genotype performance rankings challenging to discern. The evidence provided in this study demonstrates that high phenotypic variation in nursery-reared corals contributes to variable warming-acidification responses, suggesting that high-standing genetic variation in nursery-reared corals could support diverse coral restoration population outcomes along FCR.
Introduction
In recent decades, climate change has exerted a combination of acute and chronic stressors on coral reefs, threatening the function and integrity of such productive and valuable ecosystems (Hughes et al., 2017; Hughes et al., 2018b). Ocean warming (OW) and ocean acidification (OA) associated with anthropogenic CO2 emissions and other coastal processes contribute to the declining health of scleractinian corals, notably causing thermally induced coral bleaching events (the dissociation between the coral host and photosymbiotic algae; Jokiel and Coles, 1990; Baker et al., 2008;) and reductions in coral calcification rates (Anthony et al., 2008; Schoepf et al., 2013). Mass bleaching events have become increasingly common in the tropics (Heron et al., 2016; Hughes et al., 2018a), driving the loss of coral cover from bleaching-related mortality (Hughes et al., 2017; Hughes et al., 2018b). Changes in oceanic pCO2 are also disturbing the carbonate budgets of coral reefs. A recent assessment of the long-term persistence of reefs (Cornwall et al., 2021) forecasted coral net carbonate production under representative concentration pathways (RCP) 2.6, 4.5, and 8.5 and found declines in accretion by 76, 149, and 156%, respectively, by 2100. Moreover, the Intergovernmental Panel on Climate Change (IPCC) revealed that +1.5°C warming above pre-industrial levels will result in a 70-90% decline of coral cover on most reefs (Frieler et al., 2013; Schleussner et al., 2016), placing reef ecosystems at greater risk in the coming decades under multiple stressors.
Within the wider Caribbean, there has been a steady decline in live coral cover since the 1980s, attributed to seven mass coral bleaching and disease events (Aronson and Precht, 2001; Gardner et al., 2003; Manzello, 2015; Precht et al., 2016). Along Florida’s Coral Reef (FCR), recent surveys indicate living coral cover constitutes only 5% of the benthic substrate (Ruzicka et al., 2013). Many regions of FCR and the coral species within this region have been increasingly susceptible to disease outbreaks (Precht et al., 2016; Muller et al., 2020), bleaching events (Manzello, 2015), as well as ocean and coastal acidification processes (Muehllehner et al., 2016; Enochs et al., 2019; Meléndez et al., 2020), resulting in a more homogeneous coral assemblage with the loss of reef-building species (Burman et al., 2012). According to the International Union for the Conservation of Nature’s Red List, Orbicella annularis and Orbicella faveolata are identified as endangered and Dendrogyra cylindricus, Acropora cervicornis, and Acropora palmata are critically endangered Caribbean coral species (Neely et al., 2021; https://www.iucnredlist.org). The decline of key taxa coupled with increases in octocoral and sponges in reef communities along FCR (Ruzicka et al., 2013) could be associated with recent net dissolution rates (Muehllehner et al., 2016), net CO2 sinks (Moses et al., 2009), and decreased aragonite saturation states of FCR (Gledhill et al., 2008).
Despite predictions of coral reef community responses to climate change stressors, the fate of individual reef habitats is contingent on individual coral species and genotype-specific responses, and only tolerant or resilient coral genotypes may survive to contribute to future generations (Libro and Vollmer, 2016; Muller et al., 2018). In addition, high genetic and phenotypic diversity within metapopulations contributes to the adaptive potential under rapidly increasing environmental change (Matz et al., 2018). Research has shown variation in phenotypic responses of the coral holobiont to elevated temperatures and pCO2 when acting independently and in combination, depending on coral species and genotype (Comeau et al., 2013; Okazaki et al., 2017; Schoepf et al., 2017). Studies incorporating ocean acidification scenarios alone have revealed contrasting patterns of coral growth and health, where some coral species have reduced calcification rates (Jokiel et al., 2008; Krief et al., 2010; Comeau et al., 2013; Schoepf et al., 2013) and dramatically increased bleaching susceptibility under high irradiance (Anthony et al., 2008). Alternatively, other coral species remain unaffected (Reynaud et al., 2003; Krief et al., 2010; Edmunds et al., 2012; Comeau et al., 2013; Hoadley et al., 2015; Schoepf et al., 2017) as they can maintain intracellular pH and aragonite saturation in the subcalicoblastic medium (Venn et al., 2013; Schoepf et al., 2017), and decreased pH has been shown to stimulate endosymbiont physiology (Hoadley et al., 2015; Noonan and Fabricius, 2016). However, the influence of temperature appears to have a greater negative impact on coral growth and acid-base regulation (Helmle et al., 2011; Gibbin et al., 2015), and sustained thermal stress can induce coral bleaching and mortality. The interaction of both OW and OA is more realistic as it is unlikely corals are exposed to an individual stressor alone. OAOW appears to exert more physiological stress on some coral species than others (Anthony et al., 2008; Edmunds et al., 2012; Schoepf et al., 2013; Hoadley et al., 2015; Noonan and Fabricius, 2016; Okazaki et al., 2017). As these two stressors exert different timescales and effects on coral holobiont physiology and likely have synergistic or additive effects on coral health (Muller et al., 2021), it is critical to investigate coral responses to both temperature and acidification.
To facilitate recovery of and buffer ecosystem function and structure of degraded reef communities along the FCR, coral restoration programs have been implemented and expanded upon over the past 20 years (Jaap et al., 2006; Lirman and Schopmeyer, 2016). The development of ecological restoration and coral propagation, by incorporating the ‘coral gardening’ method (Rinkevich, 1995) and further refinements to maximize coral survivorship and productivity (Johnson et al., 2011), coral nurseries have the potential to buffer ecosystem structure by providing habitat and rugosity. Coral restoration and propagation projects have achieved ecologically meaningful scales - 10,000s of corals are being grown within nurseries and outplanted onto degraded reefs each year (Lirman and Schopmeyer, 2016). However, outplanting of nursery corals throughout the Caribbean and particularly in Florida, initially focused on acroporid corals per their conservation status and the implementation of species recovery plans (National Marine Fisheries Service, 2015) in addition to their fast growth and contribution to reef structure, yet high mortality rates occur a few years after outplanting (Ware et al., 2020; van Woesik et al., 2021). For massive species, outplanting has been bottlenecked due to stony coral tissue loss disease (Aeby et al., 2019), size- and tissue-selection predation risk (Rivas et al., 2021), and slow growth despite advances in microfragmentation (Forsman et al., 2015; Page et al., 2018). The success of outplanted corals reared from in situ and ex situ nurseries often have mixed results due to variable performance of outplants (Lirman et al., 2014), as some reef sites have unfavorable water quality (Muehllehner et al., 2016) or high levels of coral predators (Schopmeyer and Lirman, 2015).
Despite local challenges associated with coral outplanting success, it is imperative to understand the responses of nursery-reared coral to the major threats owing to coral reef decline - OW and OA - and investigate which coral species and/or individual genotypes are resistant to these stressors to aid in effective ecological restoration of FCR. Although massive coral species outplanting projects are lagging that of acroporids (Forsman et al., 2015; Page et al., 2018; Aeby et al., 2019; Rivas et al., 2021), this provides a window of opportunity to screen the vast biomass of restoration broodstock to understand phenotypic response and resistance to environmental stressors persistent along FCR that are likely to increase in frequency and severity (Manzello, 2015). Since 2016, Mote Marine Laboratory’s (MML) Coral Health and Disease Program has conducted long-term experimental exposures using end-of-century ocean warming and acidification scenarios on their nursery broodstock coral species to understand physiological responses to such stressors, which ultimately influences long-term survival after outplanting at restoration reef sites. For this study, we investigated the response of two nursery massive coral species - Pseudodiploria clivosa and Orbicella faveolata - to OW and OA independently and in combination and characterized whether genotypic variation in phenotypic traits conferred more tolerant individuals to evaluate the phenotypic diversity and adaptive capacity under end-of-century climate conditions.
Methods
Coral collection, experimental system and design
Orbicella faveolata and Pseudodiploria clivosa coral fragments (~4 cm2 ramet adhered to ceramic plugs) were sourced from Mote Marine Laboratory’s (MML) ex-situ nursery at the Elizabeth Moore International Center for Coral Reef Research and Restoration (IC2R3) facility in Summerland Key, Florida (24.6617° N, -81.4554° W) on 3 May 2018. Corals were originally collected opportunistically from MML’s Key West dockside nursery between 2010-2017 and then entered MML’s microfragmentation land-based production pipeline (Page et al., 2018). Colonies were genotyped via 2bRAD or microsatellites in 2018 (unpublished data) to confirm genetic differences. Twenty-four ramets from 10 genotypes of O. faveolata (n=240) and 24 ramets from 3 genotypes of P. clivosa (n=72) were transferred into a shallow flow-through raceway (2.54 m x 1.02 m x 0.30 m) in MML’s Climate and Acidification Ocean Simulator (CAOS) system. Following the transfer, corals were acclimated from the ex situ system’s well-derived seawater to CAOS’s nearshore-derived seawater over a 17-day period. During this time, raceways were covered with an overhead shade cloth from 1000-1400 each day to minimize light stress as CAOS has ~200-300 Photosynthetically Active Radiation (PAR; µmol photon m-2 s-1) greater than in the ex situ nursery.
Oceanic nearshore-derived seawater was filtered prior to entering CAOS and then held in two 3785 L mixing tanks. Temperature and pH within each mixing tank were independently controlled using Walchem Series W900 controllers (Iwaki America, Inc., Holliston, MA). One mixing tank contained ambient seawater pH targeting present-day ocean conditions (418.94 ppm pCO2; Pörtner et al., 2019), and the other contained end-of-century IPCC RCP 8.5 projections of 1200 ppm pCO2. High pCO2 was achieved by injecting pure CO2 into the mixing tank from an automatically controlled solenoid valve and venturi system connected to the networked Walchem W900. The mixing tanks used during the experiment were rotated every two weeks among a total of six mixing tanks.
After the acclimation to nearshore-derived seawater, corals were then transferred into 24, 16 L flow-through experimental aquaria distributed across two raceways (Figure S1) for 3 days in controlled aquarium conditions in CAOS system. Each experimental tank contained one ramet per genotype of each species on an egg crate rack, a submersible pump (99 GPH; Kedsum), and a plexiglass lid (Figure S1). Seawater was independently pumped into each experimental tank at a rate of 18 L hr-1. All coral replicates were kept at control temperatures and pH for baseline physiological measurements and on 31 May 2018, incremental increases in temperature and pCO2 began. The elevated temperature raceway was raised from 27°C to 31.5°C (+0.5°C per day) over 10 days and pCO2 of inflow water was raised in each pCO2 treatment tank gradually (+200 ppm pCO2 at three-day intervals) by administering pCO2 dosed seawater (e.g., pH change 8.0 to 7.7). Raceways were maintained at either ambient/control (27 ± 0.2°C) or elevated temperatures (31.5 ± 0.2°C) using a recirculating seawater bath. The control temperature of 27°C represents the mean Florida Keys coral reef temperatures for May and the elevated temperature of 31.5°C represents +1°C over the bleaching threshold for the region (Manzello, 2015). The targeted elevated pCO2 conditions were selected to represent end-of-century IPCC RCP 8.5 projections for acidification under business-as-usual CO2 emissions (IPCC, 2022). To achieve a fully factorial design among conditions, half of the tanks in each raceway were randomly assigned the high pCO2 treatment and half were assigned the ambient pCO2 treatment (Table 1), resulting in four temperature-pCO2 treatments, control (C: 27°C, 500 ppm pCO2), ocean acidification (OA: 27°C, 1100 ppm pCO2), ocean warming (OW: 31.5°C, 500 ppm pCO2), and ocean acidification with warming (OAOW: 31.5°C, 1100 ppm pCO2). Water quality (temperature, salinity, dissolved oxygen [DO], and pHTotal) was measured daily (0900-1000 GMT-5) in each experimental tank using a multi-parameter handheld (YSI ProDSS, Yellow Springs, OH) and a pH sensor (Seven2Go S8, Mettler-Toledo, Greifensee, Switzerland). Husbandry, consisting of removing algae from tanks and ceramic plugs, was completed weekly and corals were not fed during the experiment. Light levels (PARmax) were collected weekly (LI-1500 Light Sensor Logger with a LI-192 planar underwater quantum sensor; Lincoln, NE). Light levels in the aquaria averaged 385 ± 146 μmol photons m-2 s-1 for the duration of the experiment.
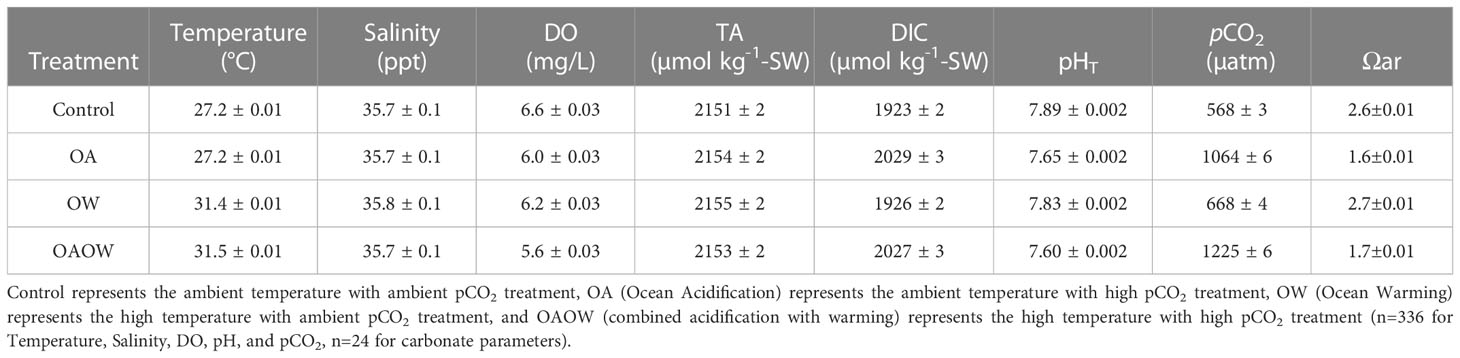
Table 1 Summary of mean ± SE water quality parameters from the experimental tanks for all treatments (DO [Dissolved Oxygen], TA [Total Alkalinity], DIC [Dissolved Inorganic Carbon], pHT [total scale], pCO2 [partial pressure of CO2], Ωar [Saturation state of seawater with respect to aragonite]).
Carbonate chemistry analysis
Seawater samples were collected weekly in 125 mL amber bottles from the mixing tanks and 12 random aquaria (n = 3 aquaria per treatment) at 0900 (GMT -5) and temperature and salinity measurements were collected from each sampled tank. Samples were stored in the dark at 4°C and analyzed for total alkalinity (TA) and dissolved inorganic carbon (DIC) following Dickson et al. (2007) within 24 hr of collection. For DIC, water samples were first filtered to 0.2 μM prior to analysis. TA was measured using a Metrohm 905 Titrator with an 800 Dosino and DIC was measured using an Apollo SciTech AS-C3 and then corrected with certified reference materials (CRMs 170,174,175, and 176) provided by A.G. Dickson (Scripps Institution of Oceanography, Oceanic Carbon Dioxide Quality Control, La Jolla, CA). DIC, TA, temperature, and salinity were used in CO2SYS to calculate pCO2, pHT, and ΩAR. If samples were not analyzed within 24 hours, they were fixed with saturated mercuric chloride (HgCl2).
Non-destructive physiological trait measurements
Physiological measurements of buoyant weight, coral tissue surface area, and photochemical efficiency of each coral fragment were completed before (T=0), at one month (T=1; 7th-12th of June 2018) and following two months of exposure (T=2; 6th-10th of August 2018). From the 14th to the 23rd of August 2018, respiration, photosynthesis, and calcification rates were measured (Muller et al., 2021). After measurements were completed, half of the corals were individually placed in sterile sampling bags (Whirl Pak) filled with ~10 mL of RNAlater® (Thermo Fisher Scientific) and stored at -80°C; the other half were saved for a disease exposure experiment (Eaton et al. in prep).
Buoyant weight of coral ramets followed Davies (1989), where corals were placed on a submerged weighted platform suspended under a precision balance (VWR-503B, VWR, Radnor, PA) atop 45 L aquarium tanks filled with 35 L of respective treatment seawater. One coral replicate per treatment tank was weighed twice to determine balance accuracy (0.001 ± 0.008 g). Temperature and salinity within weighing tanks were recorded hourly to calculate the density of seawater. Dry weight was calculated using the equation in Jokiel et al. (1978) and growth rate (Δgd-1) was calculated as the change in the dry weight between the initial and final time point divided by the initial weight, then normalized to the number of days between measurements.
At each time point, all coral ramets were photographed with a Nikon (Coolpix AW130) digital camera mounted to an overhead rig at a fixed distance with a ruler in the frame for scale. Total surface area (cm2) was measured by tracing around the live tissue using ImageJ software (National Institute of Health, Bethesda, MD, USA) followed by the methods described in (Abramoff et al., 2004), and values were used to normalize coral traits (i.e., chlorophyll, protein).
Dark-adapted photosynthetic efficiencies of Photosystem II (PSII) were measured for each coral fragment using an IMAGING-PAM M-series chlorophyll fluorometer (Walz, Germany). Fluorometry measurements were made on the center of each fragment following 30 min of dark adaptation. Fv/Fm, the maximum quantum yield of PSII, and ETRmax (maximum electron transport rate) were collected from fluorescence measurements. Settings were measuring light = 3, gain = 3, and damping = 1.
Net photosynthesis (Pnet) rates and respiration (R) rates were measured in closed temperature-controlled incubation chambers (300 mL) using a fiber-optic oxygen meter (Firesting O2, PyroScience, Aachen, Germany) connected to Pyro Oxygen Logger software (PyroScience, Denmark). Oxygen sensors were calibrated prior to measurements in 100% air-saturated water. One chamber per treatment per day contained only treatment seawater to correct for background microbial respiration and instrument drift. Photosynthesis rates were measured at approximately 190 ± 18 µmol photons m-2 (mean ± SD) for 60 min using a standard overhead fluorescent light strip at a fixed distance above the chambers. Respiration rates were measured in complete darkness for 60 min. Oxygen evolution rates (µmol O2 hr–1) for both photosynthesis and respiration rates were standardized to surface area and then used to calculate P:R.
Net calcification rates of corals were determined using the Total Alkalinity (AT) anomaly technique (Chisholm and Gattuso, 1991), where seawater samples for each coral were collected from each chamber before and after 60 min of light and 60 min of dark incubations to calculate the change in total alkalinity. Samples were stored in the dark at 4°C and analyzed within 24 hr of collection; if samples were not analyzed within 24 hr they were fixed with saturated mercuric chloride (HgCl2). AT was measured using a modification of the open-cell titration method (Dickson et al., 2007) on an automated titrator (Metrohm 905 Titrando) with 0.05N HCl in 0.6M NaCl for ~40g seawater samples. Calcification rates were calculated from the difference between AT measured at the beginning and the end of each incubation period corrected for blank values from the seawater-only incubation according to the equation described by (Schneider and Erez, 2006). Calcification rates (µmol CaCO3 h-1) were normalized to surface area.
Destructive physiological trait measurements
Concentrations of soluble host protein, algal symbiont density, and chlorophyll a (chl a) were quantified from half of the corals that were preserved in RNAlater. Coral tissue was removed from the skeleton using an airbrush (Paasche, Kenosha, Wisconsin) with filtered seawater (0.2 µm; FSW) and collected into a sterile 50 mL tube (Falcon®). The tissue slurry was homogenized (VWR® 200 Homogenizer) and then centrifuged at 4500 rpm for 5 min at 4°C. An aliquot (200 uL) was taken from the supernatant for host protein concentration determination and measured spectrophotometrically at 595 nm on a Synergy H1 microplate reader (BioTek Instruments, Inc., Winooski, VT) using a colorimetric protein assay (Bradford, 1976) and bovine serum albumin as the standard. The remaining supernatant was removed from the pelleted tissue, which was then resuspended in 1 mL FSW to standardize the volume of all samples. A 90 uL aliquot was fixed with 10% v/v Formalin and then stored at 4°C for symbiont cell counts. Counts were performed in triplicate using a hemocytometer (Neubauer improved, Superior Marienfeld, Germany) on a compound microscope (Amscope, Irvine, CA). The remaining slurry (910 uL) was centrifuged at 4500 rpm for 5 min at 4°C and the supernatant was discarded. Then, the algal pellet was resuspended in 1 mL 90% cold acetone with ~200 uL 0.5 mm glass beads (Biospec Products, Bartlesville, OK), bead-beaten at maximum speed on a vortex fitted with a horizontal microtube holder (Scientific Industries, Inc., Bohemia, NY) for 5 min to disrupt cellular material, and placed in darkness at 4°C for 24 hr to extract chlorophyll. Chlorophyll absorbance at 630, 663, and 750 nm was measured on a microplate reader and concentration calculations followed (Ritchie, 2006). Host protein, algal symbiont density, and chlorophyll a were all standardized to surface area (cm2).
Symbiodiniaceae genotyping
Samples for Symbiodiniaceae genotype identification were collected from three ex-situ nursery replicates of each coral genotype used in the experiment. Samples were collected by scraping the surface of each replicate coral (approximately 3 - 4 polyps) with a sterile razor blade and preserved in RNAlater (ThermoFisher Scientific, Waltham, MA). DNA was extracted using a DNeasy PowerSoil Kit (QIAGEN, Germantown, MD) with modifications to the manufacturer’s protocol (Rosales et al., 2020). From the PowerBead tube, 200 µL of PowerBead solution was removed before coral samples were added to the tube. Then, 200 µL of phenol:chloroform:isoamyl alcohol (PCA; 7.8 pH) and 60 µL of Solution C1 were added, and tubes were vortexed for 10 min before the original protocol was resumed. DNA concentrations and quality were measured using a NanoDrop 2000™ Microvolume UV-Vis Spectrophotometer (ThermoFisher Scientific, Waltham, MA, USA) prior to sequencing the forward and reverse ITS2 region with MR DNA laboratory (www.mrdnalab.com, Shallowater, TX, USA). This gene region was amplified using the forward primer (symbiITS1) sequence GAATTGCAGAACTCCGTG and the reverse primer (symbiITS2) sequence GGATCCATATGCTTAAGTTCAGCGGGT. Pooled samples were sequenced on Illumina’s MiSeq platform (paired-end 300 bp) on the 17th of April 2019 with a sequencing depth of 20K. Resulting raw paired-end reads were demultiplexed and then submitted to SymPortal (Hume et al., 2019) for analysis using default parameters.
Statistical analysis
Fixed effects of genotype and treatment with the random effect of tank were tested on overall coral physiology (growth rate, Fv/Fm, chl a, symbiont density, host protein, P:R, and light calcification) using a permutational multivariate analysis of variance (PERMANOVA) with the vegan package (Oksanen et al., 2013) of R v1.4.1106 (R Core Team, 2018). Three outlier samples from each coral species were removed from analyses prior to standardization and conducting PERMANOVAs using Euclidean distance calculations to generate p values. Significant effects of treatment and genotype were further examined using the pairwiseAdonis package (Martinez Arbizu, 2009) with Bonferroni adjusted p values. We were unable to compute pairwise comparisons for the interacting main effects of temperature and genotype and analyzed interactions further among individual response variables.
Individual phenotype responses were examined using linear mixed-effects models (LME) in the lmerTest package (Kuznetsova et al., 2017) with genotype and treatment as fixed effects and tank replicate as a random effect to account for potential tank effects. Non-destructive response variables measured throughout the experiment (growth rate and Fv/Fm) had the fixed effect of time added to each model. Assumption checking of normality and homogeneity of variance were performed on residuals of each model and response variable transformations occurred when necessary. Post hoc comparisons on significant main effects using Tukey’s honestly significant difference (HSD) were conducted using the emmeans package (Lenth et al., 2020). Pearson’s correlations using cor.test (stats package) were conducted on growth rate and end Fv/Fm values to examine potential tradeoffs between growth and photochemical efficiency for each treatment within each species. All figures were created in ggplot2 (Wickham, 2016) and post-processed in Illustrator.
Symbiodiniaceae abundance counts for each Defining Intragenomic Variant (DIV; Hume et al., 2019) within each coral species were analyzed using the MCMC.OTU package (Green et al., 2014). First, any DIVs that represented less than 0.1% of total sequence counts were removed. Then Poisson-lognormal generalized linear mixed model analyses of multivariate count data were conducted using MCMC to determine any change in relative proportions of individual DIVs across genotype (fixed) and replicate (random) within each species. PERMANOVAs were used to test for differences in Symbiodiniaceae ITS2 type profiles across the fixed factor of genotype in the adonis function, with 9,999 permutations of residuals from Bray-Curtis dissimilarities. Significant effects of genotype were assessed further with the package pairwiseAdonis using false discovery rate (FDR) corrected p-values. All raw data and code for analyses and Figures are available in the electronic notebook associated with this publication on the GitHub repository: https://github.com/courtneyklepac/Mote_2018OFAV-PCLI_End-of-Century-OAOW-Physiology.
Results
Treatment conditions
The physical and chemical conditions of the four treatments were maintained throughout the two-month exposure experiment (Table 1). pCO2 levels were different for all treatments, where the OAOW treatment was significantly higher than the OA treatment (Tukey p < 0.001; Figure S2), and the OW treatment had higher pCO2 than the Control (Tukey p < 0.001), which is expected under increased temperature releasing more CO2 into the atmosphere, resulting in a reduction of pH due to less available H+ and HCO3- (Hunter, 1998). In addition, temperatures in both the OW and OAOW were greater than in the OA and Control treatment (Tukey p < 0.001), and the OAOW treatment was slightly warmer than the OW treatment (p = 0.047).
Physiological response to treatments
For the physiological traits of P. clivosa, there was a difference in overall host and algal symbiont physiology among treatments, where both OW and OAOW differed from the Control and OA treatments (all comparisons adj. p < 0.01; Figure 1). All response variables contributed equally to differences across treatments for this coral species. For O. faveolata, the holobiont physiology varied by treatment, genotype, and the interaction of the two (PERMANOVA p < 0.001, p < 0.001, p = 0.04, respectively; Figure 1). There was a difference in overall physiology between the OA treatment and Control, OW, and OAOW treatments (adj. p < 0.01), with chlorophyll a (chl a [µg cm-2]), symbiont cell density, and host protein as the main contributing responses in the OA treatment. After adjusting p-values for multiple comparisons between genotypes across treatment, genotype 8 was different from genotypes 1 and 126 (p = 0.04), genotype 3 was different from 11 (p = 0.04), and genotype 61 was different from genotype 126 (p = 0.04).
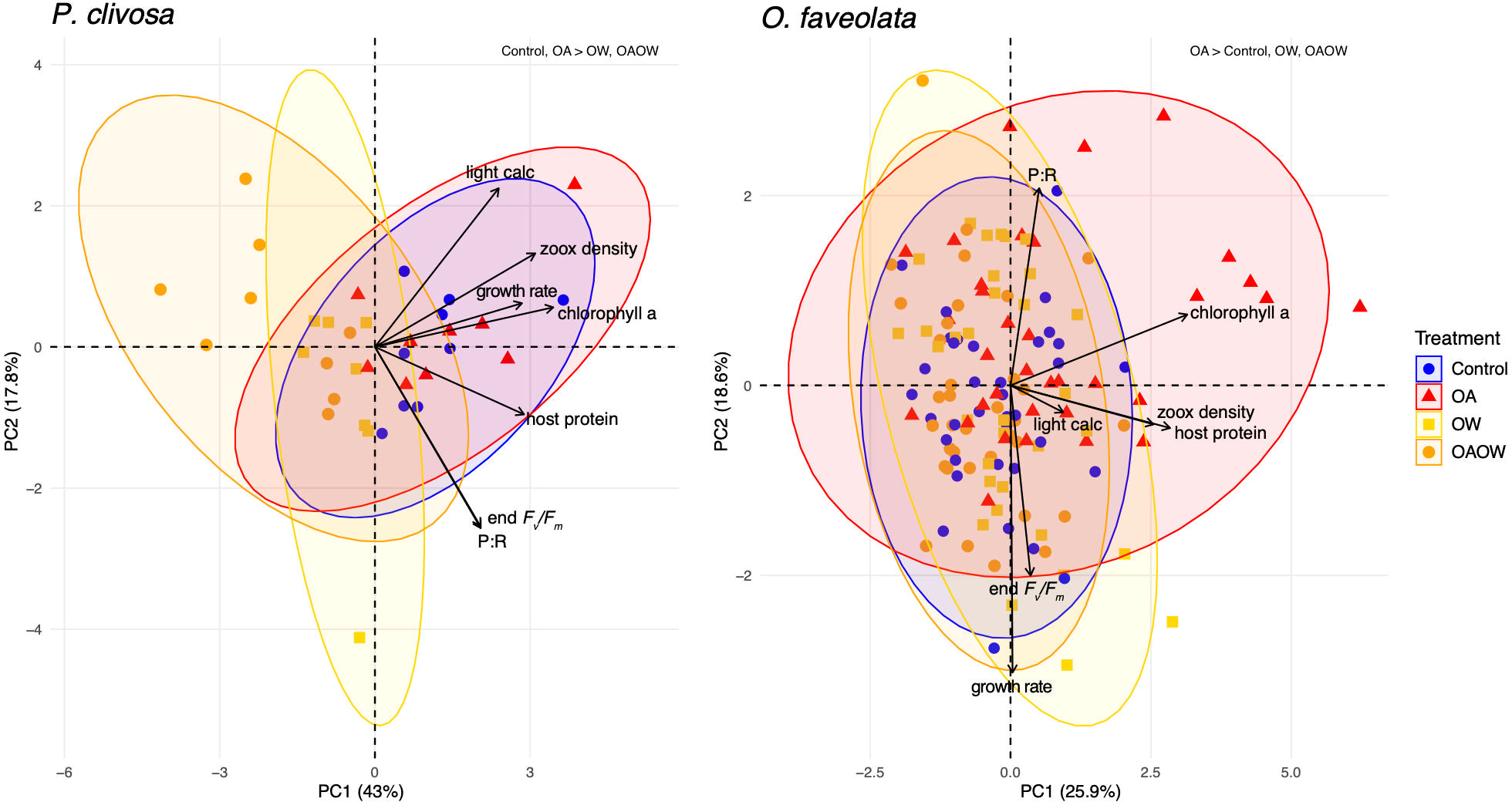
Figure 1 Principal component analysis (PCA) biplot of physiological trait data for 69 P. clivosa (left) and 115 O. faveolata (right) samples. Data point symbols are colored and shaped by treatment. Solid black vectors are trait loadings. PERMANOVA results are displayed in the top right corner of each plot and display differences among treatments.
Associations between growth and Fv/Fm following two-month exposure to treatments
Non-destructive measurements made throughout the experiment revealed effects of a treatment and genotype interaction for growth rate (Δg d-1) and mixed effects of genotype, treatment, and time for Fv/Fm for both coral species. In P. clivosa, genotype 17 had the lowest growth under OA (p < 0.01; Figure S3A) and grew slower than genotype 12 under OW (p < 0.01). In the OAOW treatment, genotypes 17 and 3 had lower growth than genotype 12 (p < 0.01, p = 0.02, respectively). For O. faveolata, genotypes 2, 8, and 27 had the lowest growth in the Control treatment, genotypes 2 and 8 had the lowest growth in the OA treatment, genotypes 8 and 27 had the lowest growth in the OW treatment, and genotypes 27 and 125 had the lowest growth in the OAOW treatment (Figure S3B).
For Fv/Fm there was a significant effect of time for both species (p < 0.01; Figure S4), where pre-exposure measurements had significantly greater Fv/Fm values than the mid and final timepoints across all treatments, whereas the mid- and end-point measurements did not differ, except for O. faveolata OA, OW, and OAOW, where end Fv/Fm values were lower than mid-point values (p < 0.001, p < 0.01, and p < 0.0001, respectively). In P. clivosa, all genotypes had similar Fv/Fm values across all treatments until the final timepoint, where genotype 17 had higher Fv/Fm values than genotype 3 and 12 (p < 0.01 for both) under OAOW and Fv/Fm values were greater for genotype 17 than 3 under OW (p < 0.01; Figure S4A). In O. faveolata, mid-point OAOW Fv/Fm values were higher than Control (p < 0.001) and OA (p < 0.01), and OW was higher than Control (p < 0.01). By the end of the experiment, OW Fv/Fm values were greater than the OA treatment (p = 0.03), and genotypes 2 and 126 had the lowest Fv/Fm values in both the OW and OAOW treatments (Figure S4B).
By the end of the experiment, genotypes with the slowest growth rates typically had greater Fv/Fm values, so we explored the potential for tradeoffs in these two phenotypes for both species across all treatments. For P. clivosa, although no significant Pearson correlations were found across treatments, there was a trend towards a positive and negative correlation between growth and Fv/Fm under OW and OAOW treatments, respectively (Figure 2A), yet this didn’t result in any significant correlations for each genotype within these treatments. There was a significant positive correlation for O. faveolata under OA treatment (p < 0.001, R2 = 0.44; Figure 3A). Interestingly, there was a strong negative relationship between growth and Fv/Fm for genotype OF126 under Control conditions (p < 0.05, R2 = -0.86).
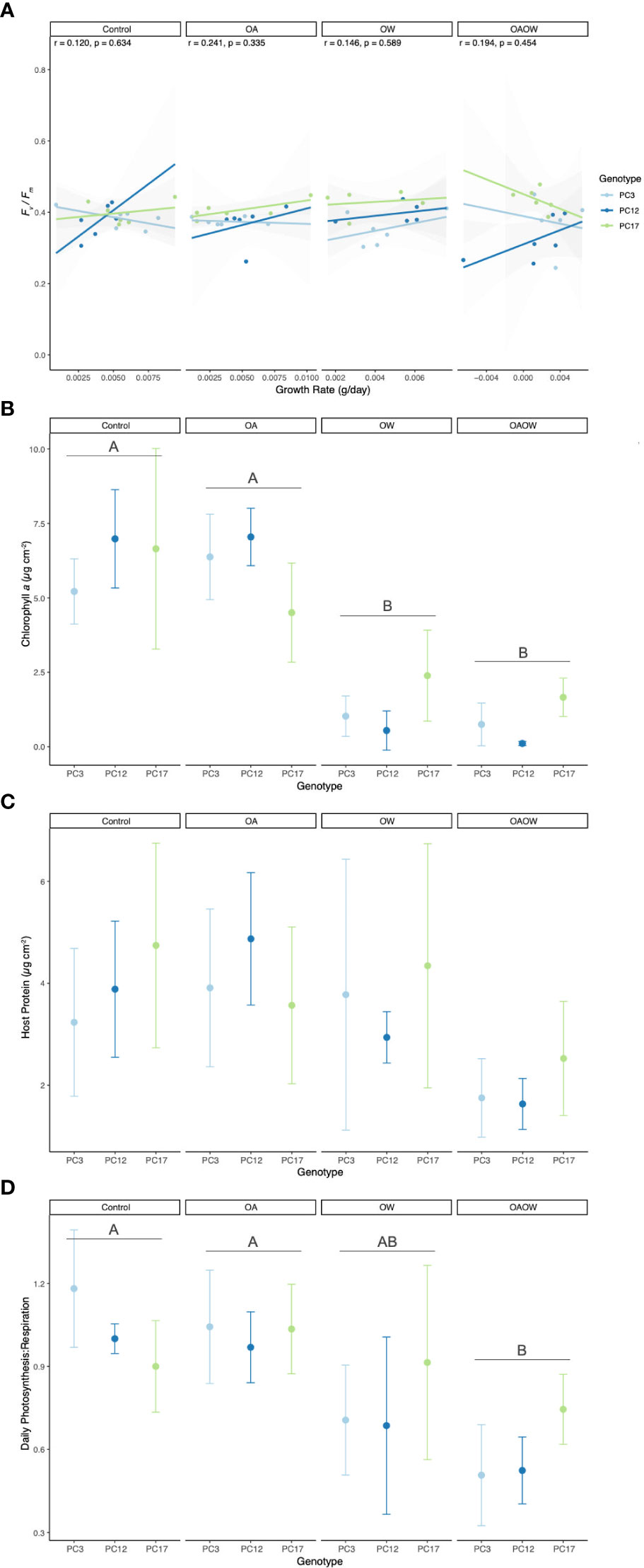
Figure 2 Physiological trait measurements of (A) growth rate correlated with end Fv/Fm, (B) chlorophyll a, (C) host soluble protein, and (D) daily net Photosynthesis : Respiration (P:R) ratio for each genotype of P. clivosa, faceted by treatment (Control, OA, OW, OAOW). Pearson correlation coefficients and p-values are within each treatment for panel (A). Significant pairwise comparisons among treatment are indicated by capital letters in each treatment facet. Points are colored by genotype and represent mean ± 95% confidence intervals (n=5-6 per treatment).
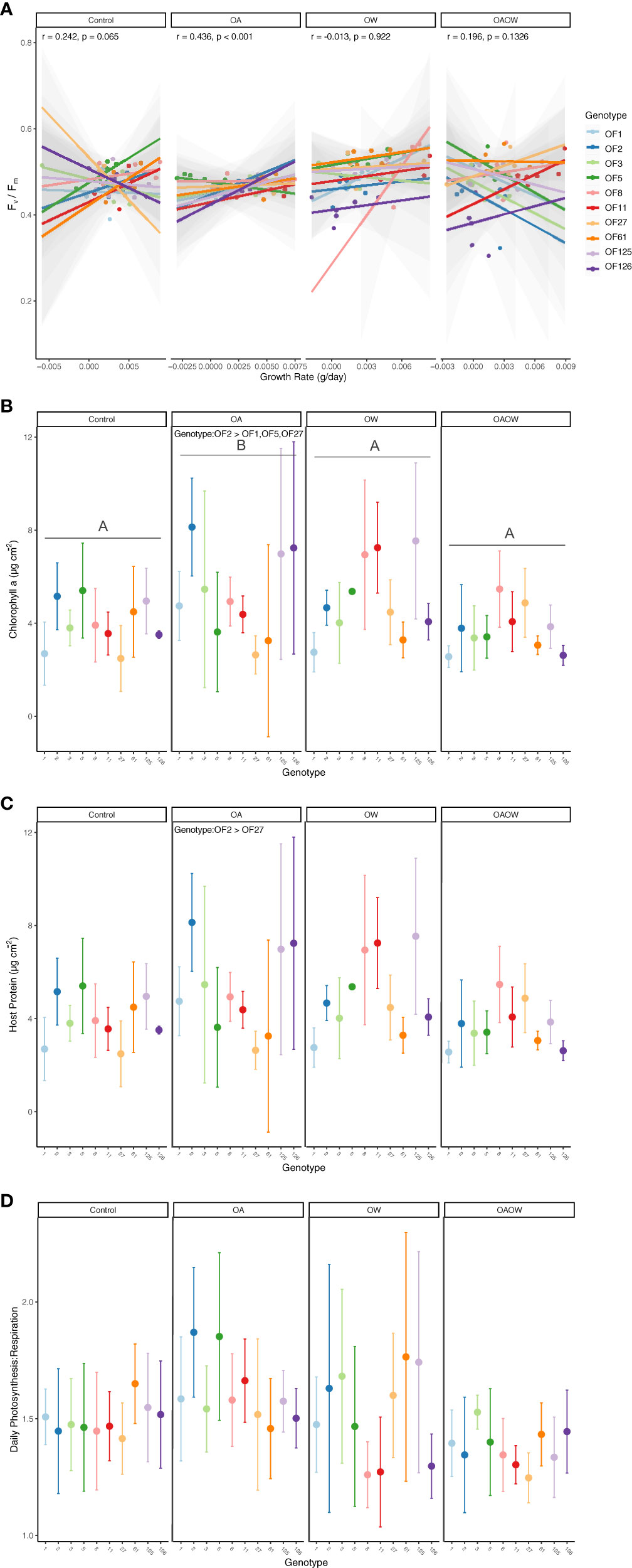
Figure 3 Physiological trait measurements of (A) growth rate correlated with end Fv/Fm, (B) chlorophyll a, (C) host soluble protein, and (D) daily net Photosynthesis: Respiration (P:R) ratio for each genotype of O. faveolata, faceted by treatment (Control, OA, OW, OAOW). Pearson correlation coefficients and p-values are within each treatment for panel (A). Significant pairwise comparisons among treatment are indicated by capital letters in each treatment facet and Tukey HSD significant pairwise comparisons among genotype are specified within treatment, where applicable. Points are colored by genotype and represent mean ± 95% confidence intervals (n=5-6 per treatment).
Phenotypic traits in response to treatment and/or genotype
Differences among treatments were detected in chl a concentration (µg cm-2), symbiont density (cells cm-2), P:R, and light calcification (µmol CaCO3 cm-2 h-1) in P. clivosa. Both OA and OAOW treatments had an overall negative effect on the concentration of chl a than both the Control and OA treatments (p < 0.01; Figure 2B). Mean (± SD) chl a values for OW were 1.32 ± 1.15, OAOW was 0.84 ± 0.80, OA was 5.98 ± 1.56, and Control was 6.28 ± 1.91 µg cm-2. Similar to chl a, square root transformed symbiont cellular density was lower in both OW and OAOW treatments in comparison to OA and Control (OW vs. OA p < 0.01; OAOW vs. OA p < 0.01; OW vs. Control p < 0.01; OAOW vs. Control p < 0.01; Figure S5A). Within the OAOW treatment, genotype 12 had 96% lower symbiont density than genotype 17 (p = 0.03). Host protein concentrations remained unchanged across all treatments and genotypes (Treatment p = 0.06, Genotype p = 0.48; Figure 2C). For daily P:R, values in the OAOW treatment were 42% lower than Control and OA values (p < 0.01; Figure 2D). Light calcification in the OW and OAOW treatments were 64% and 58% lower than in comparison to the OA treatment (p = 0.04 and 0.07, respectively; Figure S6A).
For O. faveolata, chl a and host protein concentrations varied by genotype (p < 0.01 for both) and treatment (p < 0.01 and p = 0.05, respectively; Figures 3B, C). Fragments in the OA treatment had 37% more chl a than in the Control, and OAOW treatments (p< 0.01 for both; Figure 3B) and 33% more than in the OW treatment (p = 0.01). Overall, genotype 2 had greater chl a values than genotypes 1, 27, 125, and 126 (p < 0.01, p = 0.001, p < 0.01, p < 0.05, respectively). For host protein concentrations within the OA treatment, genotype 2 had 68% more host protein than genotype 27 (p = 0.02) but overall, genotypes 2, 8, and 125 had greater protein concentrations than genotype 1 (p = 0.03, 0.04, 0.01, respectively). There were no differences in daily P:R and symbiont density across treatment and genotype (Figures 3D, S5B). There was an effect of genotype for light calcification where genotype 5 had greater overall values than genotype 1 (p < 0.01; Figure S6B).
Performance of genotypes across traits
Heatmaps of scaled phenotypic traits (end growth rate, Fv/Fm, chl a, daily P:R, and light calcification) reveal the overall relative performance of each genotype under the end-of-century (OAOW) treatment (Figure 4). By summing the averaged trait values for each genotype, a total value results and can be used to qualitatively rank each genotype as a better or worse physiological performer. For P. clivosa under OAOW, genotype 17 would be considered the highest performer under future environmental stress - displayed by all red tiles for each phenotype - followed by genotype 3 and then 12. For O. faveolata, genotype rankings are complicated by similar phenotypic responses to most treatments, but genotype 3 has the highest total score, the best performer followed by 8, and the ‘worst’ performers would be 27 and 11. Across the remaining treatments, there was sufficient genotypic variation for the ‘best’ and ‘worst’ performers (Figure S7). Heatmaps of Control, OA, and OW treatments illustrate the complexity of each genotype or trait investigated, where certain genotypes perform better than others under one treatment or trait, but not another, and there are incongruencies of rankings between metrics that characterize similar phenotypes (i.e., photophysiology: end Fv/Fm and chl a).
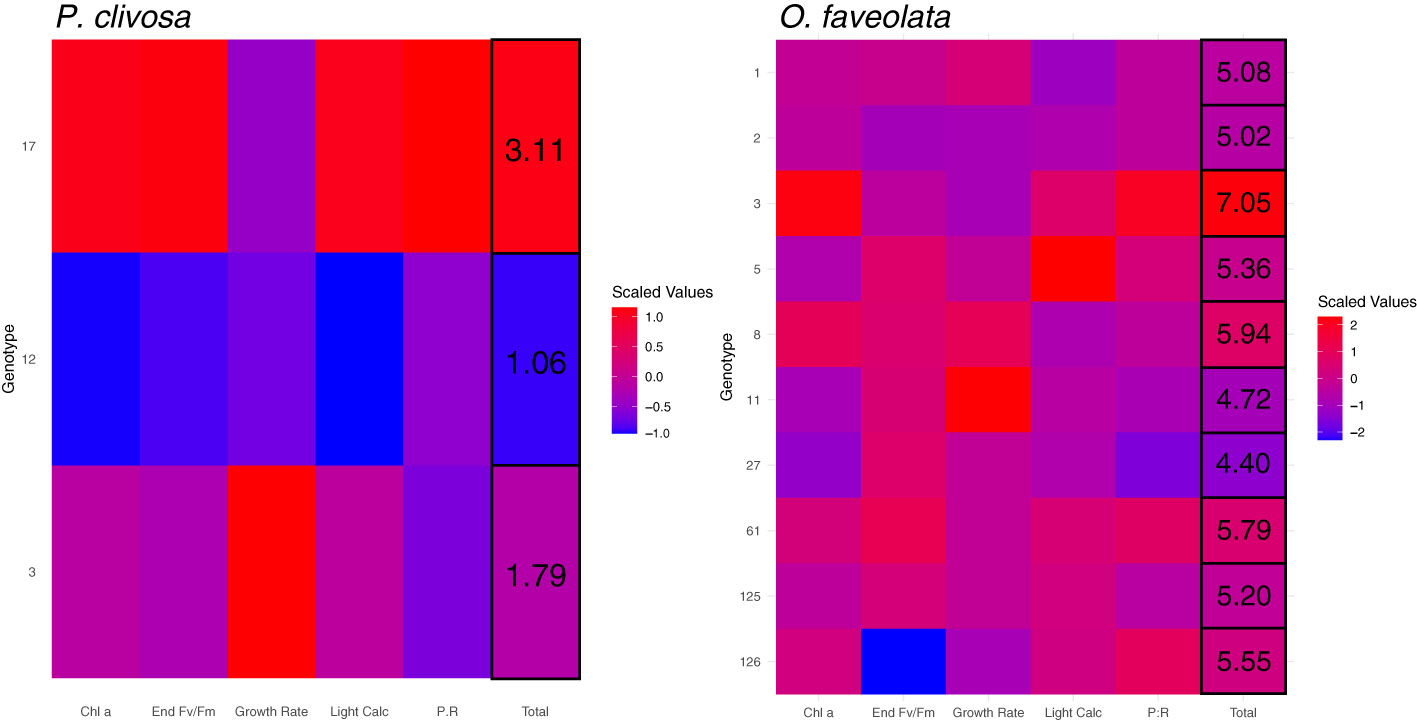
Figure 4 Heatmaps of scaled mean physiological trait values (x-axis) for each genotype (y-axis) of P. clivosa (left) and O. faveolata (right) under the OAOW treatment, where red indicates higher values (= higher performance) and blue are lower values (= lower performance). Values in the ‘Total’ column correspond to the summed average trait values for each genotype.
Symbiont composition
P. clivosa corals sourced from Mote’s land-based nursery contained 84-94% ITS2 type Breviolum B1 symbionts, followed by 1-11% ITS2 type Durusdinium D1 symbiont communities (Figure S8), yet neither MCMC.OTU nor PERMANOVA results indicated a significant difference in symbiont composition between genotypes. In contrast, O. faveolata corals contained 50-97% ITS2 type Durusdinium D1, 0.2-36% ITS2 type Breviolum B1 symbionts, and 0-19% ITS2 type Cladocopium C3 symbionts. Two O. faveolata genotypes - OF8 and OF126 - contained the highest proportion of Breviolum B1 (36% and 22%, respectively) than Durusdinium D1, and OF126 was the only genotype to contain Cladocopium C3 (Figure S8). Four of the nine Symbiodiniaceae ITS2 type profiles identified through SymPortal had significantly different proportions across genotypes of O. faveolata. For Breviolum ITS2 type profile B1-B1ao-B1x-B14e-B1ai-B1am-B1j-B14b, genotypes OF5 and OF126 had a greater abundance than OF3, OF27, and OF125 (MCMC p < 0.05; Figure S9). Genotypes OF5, OF8, OF61, and OF126 had greater proportions of ITS2 type profile B1-B1au-B1by-B1bw-B14h-B1dm than OF11 (MCMC p < 0.05), and genotypes OF1, OF2, OF3, OF11, OF27, and OF125 had greater proportions of B1-B1g-B1br-B1ao-B1x-B1dq than OF61 and OF126. One Cladocopium ITS2 type profile, C3-C21-C3an, was more abundant in OF126 than OF2 and OF125 (MCMC p < 0.05; Figure S8).
Discussion
Restoration coral species differ in response to climate change stressors
Coral restoration practitioners have established successful outplant and monitoring methods, with the ability to put out massive biomass of restoration broodstock (Lirman and Schopmeyer, 2016), but are challenged with ensuring the long-term survival of outplanted corals subject to environmental disturbances and ecological dynamics exacerbated by global climate change. Here, we examined restoration coral broodstock responses to ocean warming (OW), ocean acidification (OA), and the combined effect of both (OAOW) to understand the overall effects of climate change stressors and which, if any, genotypes are more resistant to these stressors. Both massive coral species, Orbicella faveolata and Pseudodiploria clivosa, had contrasting responses to treatments, which could be explained by the Symbiodiniaceae genus harbored (Sampayo et al., 2008; Hoadley et al., 2019) and species-specific differences in physiological responses (Abrego et al., 2008; Smith et al., 2013; Gibbin et al., 2015; Klepac and Barshis, 2020). Genotype-specific differences in phenotypes differed by species and across treatments, highlighting the challenges with ascertaining resilience values (i.e., ability to survive disturbances) for each coral species to inform best restoration practices.
Nursery P. clivosa coral phenotypes were negatively affected by the OW and OAOW treatments, indicating possible susceptibility to ocean warming scenarios and the combined effects of OA and OW predicted for the next decades. Reduced symbiont photophysiology (chlorophyll and Fv/Fm) affects coral holobiont traits such as metabolism (P:R) and calcification (Fitt et al., 2000), where increased respiration and reduced light calcification affect the long-term growth and reproductive potential of corals (Albright, 2011). P. clivosa genotypes were dominated by symbionts belonging to the genus Breviolum which could explain this species’ sensitivity to thermal stress in comparison to the symbiont genus Durusdinium (Russnak et al., 2021). Interestingly, genotype PC17 contained greater proportions of Durusdinium D1 (10.75%) than genotype PC12 (Durusdinium 0.5%; Figure S8), had greater Fv/Fm, but lower growth, indicating the potential tradeoffs with harboring this taxon of Symbiodiniaceae (Cunning et al., 2015).
In contrast to P. clivosa, nursery O. faveolata displayed similar responses between control, OW, and OAOW treatments and an apparent benefit under OA conditions, specifically within photophysiological responses of the algal endosymbiont. Corals can mitigate the acidification of the surrounding seawater by maintaining elevated pH levels and aragonite saturation states in the subcalicoblastic medium and carbon concentrating mechanisms to maintain high dissolved inorganic carbon levels to promote photosynthesis (Davy et al., 2012; Barott et al., 2015; Putnam et al., 2017). As a result, symbionts consume excess CO2 for photosynthesis, potentially contributing to or cascading into increased photochemical apparatuses and efficiency. In addition to increased phenotypic responses to OA, O. faveolata was not significantly affected by ocean warming or ocean warming and acidification treatments. Nursery-reared O. faveolata in the present study contained predominantly Durusdinium trenchii and many studies have shown reduced bleaching responses in corals harboring this symbiont (Silverstein et al., 2017; Cunning et al., 2018), conferring a bleaching threshold 1-2°C higher than other types of Symbiodiniaceae (Silverstein et al., 2017). Interestingly, genotypes OF126 and OF8 contained mixed assemblages of Durusdinium, Breviolum, and Cladocopium, but it was genotypes OF1, OF27, OF125, and OF126 with the lowest overall chlorophyll content. Moreover, the growth of genotypes dominated by Durusdinium ITS2 types was not reduced under OW and OAOW conditions and appears to be decoupled from photophysiological responses under those treatments as well (Figure 3A; Edmunds, 2017; but see Pettay et al., 2015). These inconsistencies underlying reduced physiology under climate change stressors highlight the potential roles of microbial community composition (Grottoli et al., 2018) or other factors, such as host genetics or host buffering, not accounted for herein. Regardless, future research investigating thermal bleaching responses of nursery-grown O. faveolata could incorporate even higher temperatures than used in the present study to elucidate this species’ thermal tolerance and identify genotypic-level differences in heat stress response.
Nursery corals are acclimatized to ocean acidification conditions
Ocean acidification threatens coral calcifying processes such as acid-base regulation, growth, and physical integrity (Venn et al., 2013; Putnam et al., 2017). Few studies have investigated the impacts of ocean acidification on coral health (Chan and Connolly, 2013), yet much research has not demonstrated a direct negative effect of ocean acidification on coral growth per se (Venn et al., 2013; Kavousi et al., 2015; Schoepf et al., 2017; Page et al., 2021; ; but see Okazaki et al., 2017). Here, we demonstrate that the effects of OA on P. clivosa were like that of control treatments, and for O. faveolata, OA was beneficial for coral health metrics such as chlorophyll a content, symbiont density, and host protein concentration. Corals have shown steady-state maintenance of higher internal pH and aragonite saturation at the subcalicoblastic medium under short- (24 hr) and long-term (1 year) acidification conditions (Venn et al., 2013), and could be the mechanistic response of O. faveolata and P. clivosa exposed for two months herein. Similar to Schoepf et al. (2017) and Page et al. (2021), corals used in this study resided in raceways containing well water that exposed corals to seasonally variable and lower pH levels (pH ~7.7; Table S1). Therefore, it is highly likely that the high pCO2 treatment was not more stressful than control conditions and acclimation to pH of similar magnitudes could sustain coral growth under ocean acidification conditions (Schoepf et al., 2017). In contrast, wild-collected O. faveolata and P. clivosa demonstrated decreased calcification rates after two months of experimentally increased temperature and pCO2 (Okazaki et al., 2017). Corals that originated from Lower FCR sites in Okazaki et al. (2017) were adapted to present-day pCO2 regimes and subsequently may be more affected by increased pCO2, despite being subject to similar experimental exposure periods herein. More evidence is needed to further explore whether the contrasting responses to experimental OA conditions between this study and Okazaki et al. (2017) can be attributed to environmental history (nursery aquaria versus wild reef) or underlying physiological differences.
Combined ocean acidification and warming negatively affect coral health
From an ecosystem-wide perspective, it is projected that all future CO2 emission scenarios (RCP 2.6 - 8.5) will reduce global calcium carbonate production and accretion through changes in calcification and bioerosion (Cornwall et al., 2021). However, this is predominantly driven by declining coral health and mortality following coral bleaching events and marine heatwaves, where the bare skeleton is susceptible to microbial biofilms and metabolic activity driving skeletal decay alongside dissolution of the carbonate skeleton (Leggat et al., 2019). Here, we demonstrated the potential direct and negative effects of combined ocean warming and acidification are more pronounced for P. clivosa compared with O. faveolata, where an increasingly negative effect of high temperatures, and more so of combined increased temperatures and pCO2, was apparent for all coral physiological responses (Figure 2). Other studies have found species-specific responses to combined ocean warming and acidification, where physiological stress was more severe for some species (Anthony et al., 2008; Schoepf et al., 2013; Hoadley et al., 2015; Kavousi et al., 2015) but not for others (Edmunds et al., 2012; Schoepf et al., 2013; Hoadley et al., 2015; Kavousi et al., 2015; Noonan and Fabricius, 2016), similar to our contrasting results between nursery-reared O. faveolata and P. clivosa. However, both wild O. faveolata and P. clivosa demonstrated decreased calcification rates under increased temperatures and pCO2 (Okazaki et al., 2017), indicating the possible detriment to coral health of Florida’s Coral Reef under future climate stressors, especially if restoration corals quickly acclimatize to their restoration site environment. The synergy of both climate change stressors coupled with species-specific responses will ultimately impact future coral reef composition (Okazaki et al., 2017) and the long-term success of coral restoration.
Characterization of genotypic performance
In addition to examining nursery-reared coral physiological responses to projected climate change stressors, we also sought to characterize any genotype differences to inform restoration practitioners about which genotypes would likely have inherently greater resistance to environmental stress. Information from resistance screenings serves to inform reproduction and restoration pipelines on which genotypes to continue using for direct outplanting, selective breeding, and/or whether susceptible genotypes should be phased out of production. We found substantial variation among genotypes and across phenotypes within treatments, challenging our interpretation of genotype-specific ranking of performance. For instance, the scaled phenotype heatmaps for each treatment reveal inconsistent patterns for each genotype, where each genotype’s phenotype has a different score depending on the treatment (see Figure S7). However, some genotype scorings were reflective of a physiological advantage (albeit not across the full suite of traits) when reviewing the total values as putative “rankings” in the end-of-century heatmaps (Figure 4). In this case, genotype OF3 could be considered the likely ‘winner’ for O. faveolata, and a potential tradeoff could exist for P. clivosa genotype 17 under end-of-century conditions although it is the top performer. Caution should be exerted from extrapolating performance rankings of P. clivosa as we only had sufficient biomass to examine three genotypes. This small sample size likely inflated the identification of top performers, and the inclusion of additional genotypes would naturally increase the amount of phenotypic variation in response to experimental stress (Kavousi et al., 2015). We did find possible ‘losers’ for O. faveolata, where OF11 and OF27 had the lowest chlorophyll a content and growth (light calcification). Moreover, related traits such as photophysiology (chlorophyll a, Fv/Fm, symbiont density) did not reveal similar responses among genotypes but could instead reflect differences in the timing of biological and subsequent physiological responses to stress (Fitt et al., 2000). Although we demonstrated substantial variation within both coral species and are unable to definitively identify which genotypes are most resistant to climate change stressors, the standing phenotypic variation in O. faveolata could potentially serve as standing genetic variation for environmental selection of beneficial traits across restoration sites (Dixon et al., 2015; Drury et al., 2016; Matz et al., 2018) as well as increasing genetic diversity via selective breeding (Dixon et al., 2015; Quigley et al., 2020).
High phenotypic variation under different treatments across multiple genotypes in the two coral species could also result from exposure levels that were not stressful enough to elicit genotypic differences in response to each treatment. The nursery corals used in this study were grown in raceways with well-sourced seawater, where pH fluctuated naturally by season. In addition to naturally low rearing pH compared to experimental control and OA pH levels, the ocean warming treatment temperature of 31.5°C did not elicit a large physiological bleaching response in O. faveolata. This is likely due to O. faveolata associating with the thermally tolerant symbiont Durusdinium, which is becoming more prevalent in many Caribbean coral species (Manzello et al., 2019; Pettay et al., 2015). The OW treatment temperature was based on the finding that number of days ≥31.5 °C correlated with mass bleaching events in 1997, 1998, and 2005 (Manzello, 2015). However, from the mid-1990s to present day, the average number of days with temperatures ≥31.5°C per year has increased by 2670% in the Florida Keys (Manzello, 2015), so future studies using Durusdinium-associated coral species should incorporate higher temperature treatments or a gradient of temperatures (Voolstra et al., 2020; Evensen et al., 2021), to glean genotypic-differences in stress tolerance thresholds. Moreover, it is imperative to continue physiological assessments of stress responses in restoration corals after they have been outplanted to better understand environmental and genotypic effects on tolerance, ultimately influencing long-term restoration survival and success.
Data availability statement
The datasets presented in this study can be found in online repositories. The names of the repository/repositories and accession number(s) can be found below: https://github.com/courtneyklepac/Mote_2018OFAV-PCLI_End-of-Century-OAOW-Physiology.
Author contributions
EM and EH designed the study, KRE and LA ran experiments; LA and EH ran carbonate chemistry analyses; LA, KRE, and EH ran non-destructive physiological analyses; CGP and CK performed destructive physiological analyses; CK did statistical analysis; CK and CGP wrote the draft. All authors contributed to the article and approved the submitted version.
Funding
Funding for this study was provided by the Florida Fish and Wildlife Conservation Commission (FWC) contract #17176 awarded to Mote Marine Laboratory.
Acknowledgments
We would like to acknowledge Zachary Craig and Joey Mandara for providing the corals for experimentation. We would also like to acknowledge Kyle Knoblock and Brian Richard for providing technical support with Mote’s CAOS system throughout the experiment. We would like to thank participating interns Kari Imhof, Claire Moreland-Ochoa, Curtie Leipold, Ellie Jones, Alle Brown-Law, and Shauna Wright.
Conflict of interest
The authors declare that the research was conducted in the absence of any commercial or financial relationships that could be construed as a potential conflict of interest.
Publisher’s note
All claims expressed in this article are solely those of the authors and do not necessarily represent those of their affiliated organizations, or those of the publisher, the editors and the reviewers. Any product that may be evaluated in this article, or claim that may be made by its manufacturer, is not guaranteed or endorsed by the publisher.
Supplementary material
The Supplementary Material for this article can be found online at: https://www.frontiersin.org/articles/10.3389/fmars.2023.1026426/full#supplementary-material
References
Abramoff M. D., Magalhães P. J., Ram S. J. (2004). Image processing with ImageJ. Biophotonics Int. 11, 36–42.
Abrego D., Ulstrup K. E., Willis B. L., van Oppen M. J. H. (2008). Species-specific interactions between algal endosymbionts and coral hosts define their bleaching response to heat and light stress. Proc. Biol. Sci. 275, 2273–2282. doi: 10.1098/rspb.2008.0180
Aeby G. S., Ushijima B., Campbell J. E., Jones S., Williams G. J., Meyer J. L., et al. (2019). Pathogenesis of a tissue loss disease affecting multiple species of corals along the Florida reef tract. Front. Mar. Sci. 6. doi: 10.3389/fmars.2019.00678
Albright R. (2011). Reviewing the effects of ocean acidification on sexual reproduction and early life history stages of reef-building corals. J. Mar. Biol. 2011, e473615. doi: 10.1155/2011/473615
Anthony K. R. N., Kline D. I., Diaz-Pulido G., Dove S., Hoegh-Guldberg O. (2008). Ocean acidification causes bleaching and productivity loss in coral reef builders. Proc. Natl. Acad. Sci. 105, 17442–17446. doi: 10.1073/pnas.0804478105
Aronson R. B., Precht W. F. (2001). “White-band disease and the changing face of Caribbean coral reefs,” in The ecology and etiology of newly emerging marine diseases developments in hydrobiology. (Dordrecht: Springer Netherlands), 25–38. doi: 10.1007/978-94-017-3284-0_2
Baker A. C., Glynn P. W., Riegl B. (2008). Climate change and coral reef bleaching: An ecological assessment of long-term impacts, recovery trends and future outlook. Estuar. Coast. Shelf Sci. 80, 435–471. doi: 10.1016/j.ecss.2008.09.003
Barott K. L., Venn A. A., Perez S. O., Tambutté S., Tresguerres M. (2015). Coral host cells acidify symbiotic algal microenvironment to promote photosynthesis. Proc. Natl. Acad. Sci. 112, 607–612. doi: 10.1073/pnas.1413483112
Bradford M. M. (1976). A rapid and sensitive method for the quantitation of microgram quantities of protein utilizing the principle of protein-dye binding. Anal. Biochem. 72, 248–254. doi: 10.1016/0003-2697(76)90527-3
Burman S. G., Aronson R. B., Woesik R.v. (2012). Biotic homogenization of coral assemblages along the Florida reef tract. Mar. Ecol. Prog. Ser. 467, 89–96. doi: 10.3354/meps09950
Chan N. C. S., Connolly S. R. (2013). Sensitivity of coral calcification to ocean acidification: A meta-analysis. Glob. Change Biol. 19, 282–290. doi: 10.1111/gcb.12011
Chisholm J. R. M., Gattuso J.-P. (1991). Validation of the alkalinity anomaly technique for investigating calcification of photosynthesis in coral reef communities. Limnol. Oceanogr. 36, 1232–1239. doi: 10.4319/lo.1991.36.6.1232
Comeau S., Edmunds P. J., Spindel N. B., Carpenter R. C. (2013). The responses of eight coral reef calcifiers to increasing partial pressure of CO2 do not exhibit a tipping point. Limnol. Oceanogr. 58, 388–398. doi: 10.4319/lo.2013.58.1.0388
Core Team R. (2013). R: A language and environment for statistical computing (Vienna: R Found. Stat. Comput).
Cornwall C. E., Comeau S., Kornder N. A., Perry C. T., Hooidonk R., DeCarlo T. M., et al. (2021). Global declines in coral reef calcium carbonate production under ocean acidification and warming. Proc. Natl. Acad. Sci. 118 (21), e2015265118. doi: 10.1073/pnas.2015265118
Cunning R., Gillette P., Capo T., Galvez K., Baker A. C. (2015). Growth tradeoffs associated with thermotolerant symbionts in the coral Pocillopora damicornis are lost in warmer oceans. Coral Reefs 34, 155–160. doi: 10.1007/s00338-014-1216-4
Cunning R., Silverstein R. N., Baker A. C. (2018). Symbiont shuffling linked to differential photochemical dynamics of Symbiodinium in three Caribbean reef corals. Coral Reefs 37, 145–152. doi: 10.1007/s00338-017-1640-3
Davies S. P. (1989). Short-term growth measurements of corals using an accurate buoyant weighing technique. Mar. Biol. 101, 389–395. doi: 10.1007/BF00428135
Davy S. K., Allemand D., Weis V. M. (2012). Cell biology of cnidarian-dinoflagellate symbiosis. Microbiol. Mol. Biol. Rev. MMBR 76, 229–261. doi: 10.1128/MMBR.05014-11
Dickson A. G., Sabine C. L., Christian J. R. (2007) Guide to best practices for ocean CO2 measurements (North Pacific Marine Science Organization). Available at: https://repository.oceanbestpractices.org/handle/11329/249 (Accessed 5, 2022).
Dixon G. B., Davies S. W., Aglyamova G. V., Meyer E., Bay L. K., Matz M. V. (2015). Genomic determinants of coral heat tolerance across latitudes. Science 348 (6242), 1460–1462. doi: 10.1126/science.1261224
Drury C., Dale K. E., Panlilio J. M., Miller S. V., Lirman D., Larson E. A., et al. (2016). Genomic variation among populations of threatened coral: Acropora cervicornis. BMC Genomics 17, 286. doi: 10.1186/s12864-016-2583-8
Edmunds P. J. (2017). Intraspecific variation in growth rate is a poor predictor of fitness for reef corals. Ecology 98, 2191–2200. doi: 10.1002/ecy.1912
Edmunds P. J., Brown D., Moriarty V. (2012). Interactive effects of ocean acidification and temperature on two scleractinian corals from moorea, French Polynesia. Glob. Change Biol. 18, 2173–2183. doi: 10.1111/j.1365-2486.2012.02695.x
Enochs I. C., Manzello D. P., Jones P. R., Stamates S. J., Carsey T. P. (2019). Seasonal carbonate chemistry dynamics on southeast Florida coral reefs: Localized acidification hotspots from navigational inlets. Front. Mar. Sci. 6. doi: 10.3389/fmars.2019.00160
Evensen N. R., Fine M., Perna G., Voolstra C. R., Barshis D. J. (2021). Remarkably high and consistent tolerance of a red Sea coral to acute and chronic thermal stress exposures. Limnol. Oceanogr. 66, 1718–1729. doi: 10.1002/lno.11715
Fitt W. K., McFarland F. K., Warner M. E., Chilcoat G. C. (2000). Seasonal patterns of tissue biomass and densities of symbiotic dinoflagellates in reef corals and relation to coral bleaching. Limnol. Oceanogr. 45, 677–685. doi: 10.4319/lo.2000.45.3.0677
Forsman Z. H., Page C. A., Toonen R. J., Vaughan D. (2015). Growing coral larger and faster: micro-colony-fusion as a strategy for accelerating coral cover. PeerJ 3, e1313. doi: 10.7717/peerj.1313
Frieler K., Meinshausen M., Golly A., Mengel M., Lebek K., Donner S. D., et al. (2013). Limiting global warming to 2°C is unlikely to save most coral reefs. Nat. Clim. Change 3, 165–170. doi: 10.1038/nclimate1674
Gardner T. A., Côté I. M., Gill J. A., Grant A., Watkinson A. R. (2003). Long-term region-wide declines in Caribbean corals. Science 301, 958–960. doi: 10.1126/science.1086050
Gibbin E. M., Putnam H. M., Gates R. D., Nitschke M. R., Davy S. K. (2015). Species-specific differences in thermal tolerance may define susceptibility to intracellular acidosis in reef corals. Mar. Biol. 162, 717–723. doi: 10.1007/s00227-015-2617-9
Gledhill D. K., Wanninkhof R., Millero F. J., Eakin M. (2008). Ocean acidification of the greater Caribbean region 1996–2006. J. Geophys. Res. Oceans 113 (C10). doi: 10.1029/2007JC004629
Green E., Davies S. W., Matz M. V., Medina M. (2014). Next-generation sequencing reveals cryptic Symbiodinium diversity within Orbicella faveolata and Orbicella franksi at the flower garden banks, gulf of Mexico. PeerJ Inc 2, e386. doi: 10.7287/peerj.preprints.246v1
Grottoli A. G., Martins P. D., Wilkins M. J., Johnston M. D., Warner M. E., Cai W.-J., et al. (2018). Coral physiology and microbiome dynamics under combined warming and ocean acidification. PLos One 13, e0191156. doi: 10.1371/journal.pone.0191156
Helmle K. P., Dodge R. E., Swart P. K., Gledhill D. K., Eakin C. M. (2011). Growth rates of Florida corals from 1937 to 1996 and their response to climate change. Nat. Commun. 2, 215. doi: 10.1038/ncomms1222
Heron S. F., Maynard J. A., van Hooidonk R., Eakin C. M. (2016). Warming trends and bleaching stress of the world’s coral reefs 1985–2012. Sci. Rep. 6, 38402. doi: 10.1038/srep38402
Hoadley K. D., Lewis A. M., Wham D. C., Pettay D. T., Grasso C., Smith R., et al. (2019). Host–symbiont combinations dictate the photo-physiological response of reef-building corals to thermal stress. Sci. Rep. 9 (1), 1–15. doi: 10.1038/s41598-019-46412-4
Hoadley K. D., Pettay D. T., Grottoli A. G., Cai W.-J., Melman T. F., Schoepf V., et al. (2015). Physiological response to elevated temperature and pCO2 varies across four pacific coral species: Understanding the unique host+ symbiont response. Sci. Rep. 5, 1–15.
Hughes T. P., Anderson K. D., Connolly S. R., Heron S. F., Kerry J. T., Lough J. M., et al. (2018a). Spatial and temporal patterns of mass bleaching of corals in the anthropocene. Science 359, 80–83. doi: 10.1126/science.aan8048
Hughes T. P., Kerry J. T., Álvarez-Noriega M., Álvarez-Romero J. G., Anderson K. D., Baird A. H., et al. (2017). Global warming and recurrent mass bleaching of corals. Nature 543, 373–377. doi: 10.1038/nature21707
Hughes T. P., Kerry J. T., Baird A. H., Connolly S. R., Dietzel A., Eakin C. M., et al. (2018b). Global warming transforms coral reef assemblages. Nature 556, 492–496. doi: 10.1038/s41586-018-0041-2
Hume B. C., Smith E. G., Ziegler M., Warrington H. J., Burt J. A., LaJeunesse T. C., et al. (2019). SymPortal: A novel analytical framework and platform for coral algal symbiont next-generation sequencing ITS2 profiling. Mol. Ecol. Resour. 19 (4), 1063–1080. doi: 10.1111/1755-0998.13004
Hunter K. A. (1998). The temperature dependence of pH in surface seawater. Deep Sea Res. Part I: Oceanogr. Res. Pap. 45 (11), 1919–1930. doi: 10.1016/S0967-0637(98)00047-8
IPCC (2022). The ocean and cryosphere in a changing climate: Special report of the intergovernmental panel on climate change. 1st ed (Cambridge University Press). doi: 10.1017/9781009157964
IUCN (2020) The IUCN red list of threatened species. version 2020-1. Available at: https://www.iucnredlist.orghttps://www.google.com/search?q=IUCN+(2020)+The+IUCN+Red+List+of+Threatened+Species.+Version+20201.+https%3A%2F%2F+www.iucnredlist.org&oq=IUCN+(2020)+The+IUCN+Red+List+of+Threatened+Species.+Version+20201.+https%3A%2F%2F+www.iucnredlist.org&aqs=chrome.69i57.386j0j7&sourceid=chrome&ie=UTF-8 (Accessed 5, 2022).
Jaap W. C., Hudson J. H., Dodge R. E., Gilliam D., Shaul R. (2006). Coral reef restoration with case studies from Florida. Conserv. Biol. Ser.-Camb.- 13, 478.
Johnson M. E., Lustic C., Bartels E., Al E. (2011) Caribbean Acropora restoration guide : best practices for propagation and population enhancement (Arlington, VA: The Nature Conservancy). Available at: https://dspace.mote.org:8443/xmlui/handle/2075/2910 (Accessed 5, 2022).
Jokiel P. L., Coles S. L. (1990). Response of Hawaiian and other indo-pacific reef corals to elevated temperature. Coral Reefs 8, 155–162. doi: 10.1007/BF00265006
Jokiel P. L., Maragos J. E., Franzisket L. (1978). Coral Reefs: Research Methods (Paris, France: UNESCO) 529–541.
Jokiel P. L., Rodgers K. S., Kuffner I. B., Andersson A. J., Cox E. F., Mackenzie F. T. (2008). Ocean acidification and calcifying reef organisms: a mesocosm investigation. Coral Reefs 27, 473–483. doi: 10.1007/s00338-008-0380-9
Kavousi J., Reimer J. D., Tanaka Y., Nakamura T. (2015). Colony-specific investigations reveal highly variable responses among individual corals to ocean acidification and warming. Mar. Environ. Res. 109, 9–20. doi: 10.1016/j.marenvres.2015.05.004
Klepac C. N., Barshis D. J. (2020). Reduced thermal tolerance of massive coral species in a highly variable environment. Proc. R. Soc B Biol. Sci. 287, 20201379. doi: 10.1098/rspb.2020.1379
Krief S., Hendy E. J., Fine M., Yam R., Meibom A., Foster G. L., et al. (2010). Physiological and isotopic responses of scleractinian corals to ocean acidification. Geochim. Cosmochim. Acta 74, 4988–5001. doi: 10.1016/j.gca.2010.05.023
Kuznetsova A., Brockhoff P. B., Christensen R. H. B. (2017). lmerTest package: tests in linear mixed effect models. J. Stat. Softw 82, 1–26.
Lenth R., Singmann H., Love J., Buerkner P., Herve M. (2020). emmeans: Estimated Marginal Means. R package version 1.4.8.
Lirman D., Schopmeyer S. (2016). Ecological solutions to reef degradation: optimizing coral reef restoration in the Caribbean and Western Atlantic. PeerJ 4, e2597. doi: 10.7717/peerj.2597
Lirman D., Schopmeyer S., Galvan V., Drury C., Baker A. C., Baums I. B. (2014). Growth dynamics of the threatened Caribbean staghorn coral Acropora cervicornis: Influence of host genotype, symbiont identity, colony size, and environmental setting. PLos One 9, e107253. doi: 10.1371/journal.pone.0107253
Leggat W. P., Camp E. F., Suggett D. J., Heron S. F., Fordyce A. J., Gardner S., et al. (2019). Rapid coral decay is associated with marine heatwave mortality events on reefs. Curr. Biol. 29 (16), 2723–2730. doi: 10.1016/j.cub.2019.06.077
Libro S., Vollmer S. V. (2016). Genetic signature of resistance to white band disease in the Caribbean staghorn coral acropora cervicornis. PloS One 11 (1), e0146636. doi: 10.1371/journal.pone.0146636
Manzello D. P. (2015). Rapid recent warming of coral reefs in the Florida keys. Sci. Rep. 5, 16762. doi: 10.1038/srep16762
Manzello D. P., Matz M. V., Enochs I. C., Valentino L., Carlton R. D., Kolodziej G., et al. (2019). Role of host genetics and heat-tolerant algal symbionts in sustaining populations of the endangered coral orbicella faveolata in the Florida keys with ocean warming. Glob Chang Biol. 25 (3), 1016–1031. doi: 10.1111/gcb.14545
Martinez Arbizu P. (2019). “R package ver. 0.3,” in pairwiseAdonis: pairwise multilevel comparison using adonis.
Matz M. V., Treml E. A., Aglyamova G. V., Bay L. K. (2018). Potential and limits for rapid genetic adaptation to warming in a great barrier reef coral. PLos Genet. 14, e1007220. doi: 10.1371/journal.pgen.1007220
Meléndez M., Salisbury J., Gledhill D., Langdon C., Morell J. M., Manzello D., et al. (2020). Seasonal variations of carbonate chemistry at two Western Atlantic coral reefs. J. Geophys. Res. Oceans 125, e2020JC016108. doi: 10.1029/2020JC016108
Moses C. S., Andréfouët S., Kranenburg C. J., Muller-Karger F. E. (2009). Regional estimates of reef carbonate dynamics and productivity using landsat 7 ETM+, and potential impacts from ocean acidification. Mar. Ecol. Prog. Ser. 380, 103–115. doi: 10.3354/meps07920
Muehllehner N., Langdon C., Venti A., Kadko D. (2016). Dynamics of carbonate chemistry, production, and calcification of the Florida reef tract, 2009–2010): Evidence for seasonal dissolution. Glob. Biogeochem. Cycles 30, 661–688. doi: 10.1002/2015GB005327
Muller E. M., Bartels E., Baums I. B. (2018). Bleaching causes loss of disease resistance within the threatened coral species acropora cervicornis (Elife 7). doi: 10.7554/eLife.35066
Muller E. M., Dungan A. M., Million W. C., Eaton K. R., Petrik C., Bartels E., et al. (2021). Heritable variation and lack of tradeoffs suggest adaptive capacity in Acropora cervicornis despite negative synergism under climate change scenarios. Proc. R. Soc B Biol. Sci. 288, 20210923. doi: 10.1098/rspb.2021.0923
Muller E. M., Sartor C., Alcaraz N. I., van Woesik R. (2020). Spatial epidemiology of the stony-Coral-Tissue-Loss disease in Florida. Front. Mar. Sci. 7. doi: 10.3389/fmars.2020.00163
National Marine Fisheries Service (2015). “Prepared by the acropora recovery team for the national marine fisheries service,” in Recovery plan for elkhorn (Acropora palmata) and staghorn (A. cervicornis) corals, vol. 167. (MD: Silver Spring).
Neely K. L., Lewis C. L., Lunz K. S., Kabay L. (2021). Rapid population decline of the pillar coral Dendrogyra cylindrus along the Florida reef tract. Front. Mar. Sci. 8. doi: 10.3389/fmars.2021.656515
Noonan S. H. C., Fabricius K. E. (2016). Ocean acidification affects productivity but not the severity of thermal bleaching in some tropical corals. ICES J. Mar. Sci. 73, 715–726. doi: 10.1093/icesjms/fsv127
Okazaki R. R., Towle E. K., van Hooidonk R., Mor C., Winter R. N., Piggot A. M., et al. (2017). Species-specific responses to climate change and community composition determine future calcification rates of Florida keys reefs. Glob. Change Biol. 23, 1023–1035. doi: 10.1111/gcb.13481
Oksanen J., Blanchet F. G., Kindt R., Legendre P., Minchin P. R., O’hara R. B., et al. (2013). Community ecology package (R package version 2), pp.321–pp.326.
Page H. N., Hewett C., Tompkins H., Hall E. R. (2021). Ocean acidification and direct interactions affect coral, macroalga, and sponge growth in the Florida keys. J. Mar. Sci. Eng. 9, 739. doi: 10.3390/jmse9070739
Page C. A., Muller E. M., Vaughan D. E. (2018). Microfragmenting for the successful restoration of slow growing massive corals. Ecol. Eng. 123, 86–94. doi: 10.1016/j.ecoleng.2018.08.017
Pettay D. T., Wham D. C., Smith R. T., Iglesias-Prieto R., LaJeunesse T. C. (2015). Microbial invasion of the Caribbean by an indo-pacific coral zooxanthella. Proc. Natl. Acad. Sci. 112, 7513–7518. doi: 10.1073/pnas.1502283112
Pörtner H. O., Roberts D. C., Masson-Delmotte V., Zhai P., Tignor M., Poloczanska E., et al. (2019). IPCC special report on the ocean and cryosphere in a changing climate Vol. 1 (Geneva, Switzerland: IPCC Intergovernmental Panel on Climate Change).
Precht W. F., Gintert B. E., Robbart M. L., Fura R., van Woesik R. (2016). Unprecedented disease-related coral mortality in southeastern Florida. Sci. Rep. 6, 31374. doi: 10.1038/srep31374
Putnam H. M., Barott K. L., Ainsworth T. D., Gates R. D. (2017). The vulnerability and resilience of reef-building corals. Curr. Biol. 27, R528–R540. doi: 10.1016/j.cub.2017.04.047
Quigley K. M., Bay L. K., van Oppen M. J. H. (2020). Genome-wide SNP analysis reveals an increase in adaptive genetic variation through selective breeding of coral. Mol. Ecol. 29, 2176–2188. doi: 10.1111/mec.15482
Reynaud S., Leclercq N., Romaine-Lioud S., Ferrier-Pagés C., Jaubert J., Gattuso J.-P. (2003). Interacting effects of CO2 partial pressure and temperature on photosynthesis and calcification in a scleractinian coral. Glob. Change Biol. 9, 1660–1668. doi: 10.1046/j.1365-2486.2003.00678.x
Rinkevich B. (1995). Restoration strategies for coral reefs damaged by recreational activities: The use of sexual and asexual recruits. Restor. Ecol. 3, 241–251. doi: 10.1111/j.1526-100X.1995.tb00091.x
Ritchie R. J. (2006). Consistent sets of spectrophotometric chlorophyll equations for acetone, methanol and ethanol solvents. Photosynth. Res. 89, 27–41. doi: 10.1007/s11120-006-9065-9
Rivas N., Hesley D., Kaufman M., Unsworth J., D’Alessandro M., Lirman D. (2021). Developing best practices for restoring massive corals and mitigating predation impacts: influences of physical protection, colony size, and genotype on outplant mortality. Coral Reefs 40, 1227–1241. doi: 10.1007/s00338-021-02127-5
Rosales S. M., Clark A. S., Huebner L. K., Ruzicka R. R., Muller E. M. (2020). Rhodobacterales and rhizobiales are associated with stony coral tissue loss disease and its suspected sources of transmission. Front. Microbiol. 11. doi: 10.3389/fmicb.2020.00681
Russnak V., Rodriguez-Lanetty M., Karsten U. (2021). Photophysiological tolerance and thermal plasticity of genetically different symbiodiniaceae endosymbiont species of cnidaria. Front. Mar. Sci. 8. doi: 10.3389/fmars.2021
Ruzicka R. R., Colella M. A., Porter J. W., Morrison J. M., Kidney J. A., Brinkhuis V., et al. (2013). Temporal changes in benthic assemblages on Florida keys reefs 11 years after the 1997/1998 El niño. Mar. Ecol. Prog. Ser. 489, 125–141. doi: 10.3354/meps10427
Sampayo E. M., Ridgway T., Bongaerts P., Hoegh-Guldberg O. (2008). Bleaching susceptibility and mortality of corals are determined by fine-scale differences in symbiont type. Proc. Natl. Acad. Sci. 105, 10444–10449. doi: 10.1073/pnas.0708049105
Schleussner C. F., Lissner T. K., Fischer E. M., Wohland J., Perrette M., Golly A., et al. (2016). Differential climate impacts for policy-relevant limits to global warming: the case of 1.5 c and 2 c. Earth system dynamics 7 (2), 327–351. doi: 10.5194/esd-7-327-2016
Schneider K., Erez J. (2006). The effect of carbonate chemistry on calcification and photosynthesis in the hermatypic coral Acropora eurystoma. Limnol. Oceanogr. 51, 1284–1293. doi: 10.4319/lo.2006.51.3.1284
Schoepf V., Grottoli A. G., Warner M. E., Cai W.-J., Melman T. F., Hoadley K. D., et al. (2013). Coral energy reserves and calcification in a high-CO2 world at two temperatures. PLos One 8, e75049. doi: 10.1371/journal.pone.0075049
Schoepf V., Jury C. P., Toonen R. J., McCulloch M. T. (2017). Coral calcification mechanisms facilitate adaptive responses to ocean acidification. Proc. R. Soc B Biol. Sci. 284, 20172117. doi: 10.1098/rspb.2017.2117
Schopmeyer S. A., Lirman D. (2015). Occupation dynamics and impacts of damselfish territoriality on recovering populations of the threatened staghorn coral, Acropora cervicornis. PLos One 10, e0141302. doi: 10.1371/journal.pone.0141302
Silverstein R. N., Cunning R., Baker A. C. (2017). Tenacious d: Symbiodinium in clade d remain in reef corals at both high and low temperature extremes despite impairment. J. Exp. Biol. 220, 1192–1196. doi: 10.1242/jeb.148239
Smith T. B., Brandt M. E., Calnan J. M., Nemeth R. S., Blondeau J., Kadison E., et al. (2013). Convergent mortality responses of Caribbean coral species to seawater warming. Ecosphere 4, art87. doi: 10.1890/ES13-00107.1
US Department of Commerce, N. O. and A. A (2015) CoRIS featured publication: Recovery plan for elkhorn coral (Acropora palmata) and staghorn coral (A. cervicornis). Available at: https://www.coris.noaa.gov/activities/elkhorn_recovery_plan/ (Accessed 5, 2022).
van Woesik R., Banister R. B., Bartels E., Gilliam D. S., Goergen E. A., Lustic C., et al. (2021). Differential survival of nursery-reared Acropora cervicornis outplants along the Florida reef tract. Restor. Ecol. 29, e13302. doi: 10.1111/rec.13302
Venn A. A., Tambutté E., Holcomb M., Laurent J., Allemand D., Tambutté S. (2013). Impact of seawater acidification on pH at the tissue–skeleton interface and calcification in reef corals. Proc. Natl. Acad. Sci. 110, 1634–1639. doi: 10.1073/pnas.1216153110
Voolstra C. R., Buitrago-López C., Perna G., Cárdenas A., Hume B. C. C., Rädecker N., et al. (2020). Standardized short-term acute heat stress assays resolve historical differences in coral thermotolerance across microhabitat reef sites. Glob. Change Biol. 26, 4328–4343. doi: 10.1111/gcb.15148
Ware M., Garfield E. N., Nedimyer K., Levy J., Kaufman L., Precht W., et al. (2020). Survivorship and growth in staghorn coral (Acropora cervicornis) outplanting projects in the Florida keys national marine sanctuary. PLos One 15, e0231817. doi: 10.1371/journal.pone.0231817
Keywords: coral restoration, coral bleaching, ocean acidification (OA), climate change, Florida’s Coral Reef, Orbicella faveolata, Pseudodiploria clivosa, massive corals
Citation: Klepac CN, Eaton KR, Petrik CG, Arick LN, Hall ER and Muller EM (2023) Symbiont composition and coral genotype determines massive coral species performance under end-of-century climate scenarios. Front. Mar. Sci. 10:1026426. doi: 10.3389/fmars.2023.1026426
Received: 23 August 2022; Accepted: 19 January 2023;
Published: 14 February 2023.
Edited by:
Kefu Yu, Guangxi University, ChinaReviewed by:
Xiangcheng Yuan, South China Sea Institute of Oceanology (CAS), ChinaXiaopeng Yu, Guangxi University, China
Copyright © 2023 Klepac, Eaton, Petrik, Arick, Hall and Muller. This is an open-access article distributed under the terms of the Creative Commons Attribution License (CC BY). The use, distribution or reproduction in other forums is permitted, provided the original author(s) and the copyright owner(s) are credited and that the original publication in this journal is cited, in accordance with accepted academic practice. No use, distribution or reproduction is permitted which does not comply with these terms.
*Correspondence: Courtney N. Klepac, cklepac@mote.org
†ORCID: Courtney Nicole Klepac, orcid.org/0000-0002-3935-8275