Culturing reef-building corals on a laboratory dish: a simple experimental platform for stony corals
- 1Institute of Marine Environment and Ecology, National Taiwan Ocean University, Keelung, Taiwan
- 2Center of Excellence for the Oceans, National Taiwan Ocean University, Keelung, Taiwan
- 3Biodiversity Research Center, Academia Sinica, Taipei, Taiwan
- 4Institute of Earth Sciences, Academia Sinica, Taipei, Taiwan
Development and maintenance of coral reef ecosystems rely on daily micro-biological activities of healthy corals. Although a comprehensive understanding of coral biological properties, as well as factors negatively affecting coral growth, are essential to conserve existing corals, the current lack of a tractable culture and experimental platform has delayed acquisition of such knowledge. Here we show a highly versatile culture system, “coral-on-a-laboratory dish” (CLD), allowing long-term culturing of various corals in plastic/glass Petri dishes with maintenance of their biological properties. Under optimized conditions, coral microcolonies (~5 mm x 5 mm fragments) of Pocillopora damicornis were cultured for several months in dishes with high survivorship and characteristic growth. These microcolonies maintained their biological properties, such as reproduction, skeleton formation, coral-algal symbiosis, ingestion, and digestion. Thus far, CLD has been used to maintain at least 4 other coral species from 4 other families, including the Acroporidae, the Pocilloporidae, the Poritidae, and the Merulinidae for more than 2 months with 100% survivorship. CLD is applicable to a variety of biophysiological studies, including coral-algal symbiosis and impact assessment of marine pollutants, e.g., a sunscreen substance, oxybenzone, and an organic biocide, Irgarol 1051, at cellular-level resolution. The CLD platform, which allows easy and inexpensive coral maintenance in a laboratory incubator, represents a technological breakthrough that will greatly advance coral studies.
1 Introduction
Stony corals are the keystone animals that create coral reef ecosystems, which support the highest marine biodiversity on the planet. The three-dimensional structures produced by corals function as nurseries for various reef organisms, and sustain fisheries and tourism in tropical and subtropical regions (Odum and Odum, 1955; Halpern et al., 2012). In recent years, however, coral reefs have steadily declined worldwide mainly due to anthropogenic impacts, diseases, and predator damage (Hoegh-Guldberg, 1999; Hughes et al., 2017; Hughes et al., 2018), and they are predicted to decline further with continuing climate change (Hoegh-Guldberg et al., 2017). With coral reefs in decline, fundamental knowledge and technologies have been developed worldwide to protect existing coral reefs and to attempt to restore damaged coral reefs (Selig and Bruno, 2010; Young et al., 2012; Leal et al., 2014; Barton et al., 2015; Omori, 2019).
Formation and development of coral reefs are supported by coral biological activities that occur at microscale levels. For examples, asexual reproduction and skeletogenesis underlie coral growth and reef development. Coral health is maintained primarily through symbiotic relationships with zooxanthellae and host coral cells, and the breakdown of this relationship, known as “coral bleaching,” not only results in coral mortality, but also causes disrupts the balance of coral reef ecosystems (Venn et al., 2006; Hughes et al., 2018). Thus, more detailed biological knowledge at the micro-scale level is a prerequisite to assess and predict impacts of global or local climate changes on existing corals. Moreover, there is growing public concern regarding the impact of personal care products such as sunscreens, fungicides and other agricultural chemicals, and other marine pollutants in recent years (Danovaro et al., 2008; Sheikh et al., 2009; van Dam et al., 2011; Fel et al., 2019; Watkins and Sallach, 2021). Efforts to identify substances that negatively impact coral survival and to reduce or replace them with non-toxic alternatives are important for management and protection of existing corals.
Despite their importance, knowledge of cellular and molecular biology of corals is largely inadequate. A major limiting factor is the lack of a simple, inexpensive system to maintain corals in the laboratory, i.e., poor accessibility to experimental samples. Generally, culturing corals is regarded as difficult, and long-term indoor culture systems require aquaria, sophisticated LED lights, and expensive circulatory filtration equipment to maintain water quality (Craggs et al., 2017). If corals could be cultured easily and inexpensively in limited space, e.g., in incubators, like other cnidarian model species, e.g., sea anemones and Hydra (Darling et al., 2005; Bossert and Galliot, 2012), access to experimental samples could be improved and research could be accelerated. The other major limiting factor is the lack of a tractable experimental platform that would allow observation and status assessment of living corals at the cellular level. Such a platform to observe effects of factors of interest on corals in real time at the microscopic level under well-defined culture conditions would make it possible not only to understand effects of environmental factors on corals, but also to screen substances that positively/negatively affect corals at large scale.
To date, several experimental platforms using live corals have been established. These platforms, using primary/juvenile polyps, are one of the major methodologies. Because early coral polyps can be maintained relatively easily in culture dishes or well plates, cellular-level microscopic observations are possible and have proven useful in studies of cellular and molecular mechanisms of symbiosis and skeletogenesis (Yuyama and Higuchi, 2014; Wolfowicz et al., 2016; Ohno et al., 2017). However, a major drawback is the limited time available for conducting the experiment, as early polyps are mainly prepared from fertilized eggs of wild corals during the reproductive season. Recently, a novel experimental platform, coral-on-a-chip, was developed (Shapiro et al., 2016). An isolated coral polyp from adult colony was maintained for a short period of time in a small microwell fabricated on a microscope slide equipped with a microfluidic system. Combined with live-imaging using confocal microscopy, detailed changes occurring in tissues of living corals, e.g., calcification and bleaching, could be observed in real time (Shapiro et al., 2016). Although it is a powerful platform, there are still some drawbacks, such as the limited number of species (a single genus) to which it can be applied, low survivorship of isolated polyps caused by the current isolation method using high-salinity stress (Shikina et al., unpublished data), and the fact that it can only be performed in a research environment with relatively expensive, specialized equipment.
To address these issues, we sought to establish a tractable experimental platform that can be applied to a wide range of coral species, and that can be easily applied in any laboratory for both culturing and microscopic observation of corals at the same time. Further, this platform needed to enable long-term culturing of corals while maintaining high survival rates and a wide range of biological properties. To achieve these ends, the present study attempted to culture corals in 9-cm Petri dishes, to establish a simple platform, coral-on-a-laboratory dish (CLD). As the main experimental organism, we used Pocillopora damicornis, which is distributed worldwide and for which transcriptomic and genomic information are available (Traylor-Knowles et al., 2011; Cunning et al., 2018). Small coral fragments, namely microcolonies (about 5 mm x 5 mm) were collected from adult colonies, superglued onto Petri dishes, and maintained using commercially available artificial seawater in a laboratory incubator equipped with LED lights (Figure 1A). Here, we show the establishment of a simple, but powerful and effective platform, demonstrating its strength and potential applications to biophysiological and ecotoxicological studies of corals.
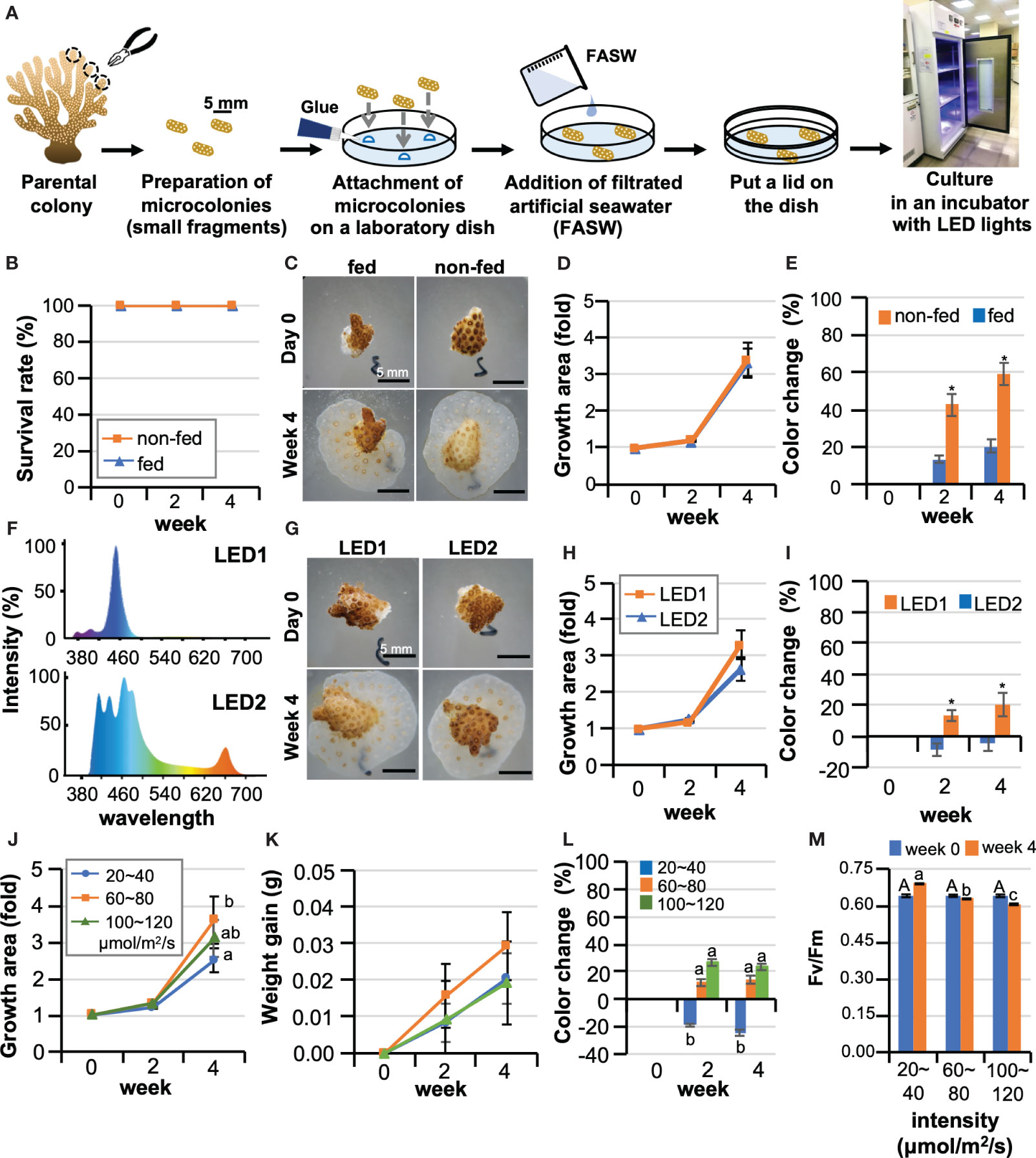
Figure 1 Optimization of culture conditions for maintaining corals in laboratory dishes. (A) Schematic diagram of the procedure for starting a coral microcolony culture in a laboratory dish. (B–E) Effects of Artemia feeding on Pocillopora damicornis microcolonies. Data are expressed as the mean ± SE (n = 3 replicates for each group). (B) Representative micrographs of microcolonies of a group fed Artemia (fed) and a group not fed (non-fed) at 0 days and 4 weeks of culture. (C) Survival rate, (D) Changes in the area covered by microcolonies, (E) Changes in the color of microcolonies *P < 0.05. (F–I) Results of microcolony culture under two types of LED light with different light spectra. Data are expressed as the mean ± SE (n = 3 replicates for each group). (F) Light spectra of two types of LED light (LED1 and LED2) used in the experiment. (G) Representative pictures of microcolonies cultured under LED1 or LED2 at 0 days and 4 weeks of culture. (H) Changes in the area covered by microcolonies, (I) Changes in the color of microcolonies. *P < 0.05. (J–M) The effect of light intensity on P. damicornis microcolonies. Data are expressed as the mean ± SE (n = 3 replicates for each group). (J) Changes in areas covered by microcolonies. (K) Changes in masses of microcolonies. (L) Changes in the color of microcolonies. (M) Maximum quantum yield (Fv/Fm) of zooxanthellae in microcolonies. Groups with different letters are significantly different (P < 0.05).
2 Materials and methods
2.1 Coral sampling
Collection of all coral species used in this study, i.e., Pocilloporidae: P. damicornis and Stylophora pistillata, Acroporidae: Acropora tumida, Poritidae: Porites lobata, and Merulinidae: Favites pentagona, was conducted at Pitouchiao Park (25°07’N, 120°54’E) in northern Taiwan with permission of the Fisheries and Fishing Port Affairs Management Office of the New Taipei city government (Issue number: 1063334179). For each species, 4~5 large coral colonies (approximately 30 cm in diameter) were selected, and small fragments (5~8 cm in length/diameter for each colonies) were collected by scuba divers with a chisel and hammer. All fragments were transported to and cultured in an outdoor pond (40 m in length × 25 m in width × 3 m in depth) at the Marine Resources Recovery Park, New Taipei City before use.
2.2 Preparation of microcolonies and culture in laboratory dishes
Microcolonies (approximately 5-8 mm in length on each side) were prepared by cutting the apical parts of fragments with a stainless-steel nipper. Dissected microcolonies were pooled in a dish and washed twice with 0.22 μm filtered artificial seawater (FASW) (filter, Stericup Quick Release, Millepore, MA, USA; artificial seawater, Coral Pro Salt, Red Sea Aquatics Ltd, Hong Kong) to remove small skeletal debris and mucus. Three microcolonies were randomly selected, and the amputation sites (skeleton) were affixed to bottoms of plastic (9-cm diameter, Jet Bio-Filtration Co., Ltd. Guanzhou, China) or glass culture dishes (9-cm diameter, ANUMBRA, Germany) with underwater superglue (ISTA, Taiwan). After adding 60 mL of FASW per dish, dishes were covered with lids and sealed with Parafilm M (Amcor, United States) to prevent evaporation of seawater. Microcolonies were then cultured under LED light (Xmini, Illumagic, Taiwan; 12 L: 12 D cycle) in a plant incubator (SS-980, Tominaga, Taipei, Taiwan; 190 cm in height × 86 cm in width × 82 cm in depth). The temperature was set to 23 ± 0.1°C based on the average annual water temperature of the sampling site. For feeding, HUFA-enriched Artemia nauplii were provided as the only food source. Artemia were added directly to the dishes (500 Artemia/dish once every three days). Feeding time was set as 3 h. After feeding, all seawater in the culture dishes was replaced with 60 mL of FASW.
2.3 Optimization of culture conditions
Effects of Artemia feeding (fed or non-fed), light spectrum (2 types of LED lights. LED1:ReefLED 90, Red Sea Aquatics Ltd.,; and LED2: Illumagic Blaze X Mini LED Aquarium Lighting, Taiwan), and light intensity (3 levels: 20-40, 60-80, 100-120 μmol/m2/s, use LED2) were investigated. Test periods were 4-6 weeks. In all groups, 3 dishes (3 microcolonies/dish, total 9 microcolonies) were prepared from one colony per experiment. Experiments were performed at least 3 times (triplicate) with different colonies. To evaluate coral status non-destructively, all microcolonies in culture dishes were photographed weekly with a digital camera (RX100II, Sony, Tokyo, Japan) under a stereo microscope (EZ4, Leica Microsystems, Mannheim, Germany). Survival (%), abnormality (%, for more detail, see Figure S1), growth (area fold change), newly produced polyp number, polyp morphology (tentacle number), polyp expansion status (degree rating, for more detail, see Table S1; Figure S1), and color change (Red Green Blue [RGB] values) were quantified by comparing and analyzing photographs taken at the beginning of the culture and at various times thereafter. Image analysis was performed with Image J software (Wayne Rasband, National Institutes of Health, Bethesda, MD, USA; https://imagej.nih.gov/ij). For color analysis, RGB values (three values) from a single microcolony were added, and the color change was shown relative to the value on the first day of culture. Positive values indicate whitening, while negative values indicate darker colors. To measure changes in microcolony mass, seawater in culture dishes was discarded and any remaining water removed using a cloth. Masses of culture dishes containing coral microcolonies were determined using an electronic balance and masses of culture dishes were subtracted from the total to obtain masses of coral fragments. This simplified measurement method was employed because the purpose of this measurement was to provide a rough measurement of the increase in coral volume during the culture period. Negligible amounts of seawater on coral surfaces were ignored. The maximum quantum yield (Fv/Fm) of zooxanthellae in microcolonies was assessed with a Junior-PAM portable chlorophyll fluorometer (Heinz Walz GmbH, Effeltrich, Germany) according to the manufacturer’s instruction. Samples were dark-acclimated for 30 min prior to measurement of Fv/Fm.
2.4 Monitoring changes in seawater chemistry in culture dishes
With CLD, seawater in culture dishes is replaced with fresh FASW every 3 days. In other words, microcolonies are maintained for 3 days in a closed-dish environment. To examine changes in seawater chemistry in the dishes during the culture period, all 60 mL of seawater were collected at 2:00 pm (6 h after turning on the lights) from each dish after 3 days of culture, and salinity, pH, total alkalinity (TA), Ca2+ concentrations, dissolved oxygen (DO), ammonia, NO2, NO3, and PO4 were measured using a multiparameter waterproof meter (HI98194, HANNA instruments, Inc. RI), a multiparameter benchtop photometer, and a pH meter (HI83300, HANNA), according to the manufacturer’s instructions. Three timepoints during weeks 2-4 of culture with actively growing microcolonies were selected for measurements: (i) days 14-17 of culture, (ii) days 18-21 of culture, and (iii) days 22-25 of culture. Culturing seawater was collected from 3 culture dishes at each timepoint (n=3 dishes, derived from 3 colonies) and used for measurements (total, 9 seawater samples). As a control group, three culture dishes with no microcolonies were filled with fresh FASW at the same time and incubated in the same culture environment for 3 days, and then changes in water quality were measured.
2.5 Skeletogenesis
Calcein AM (Sigma-Aldrich), a fluorescent label that binds calcium, was introduced into cultures for 4 h at a final concentration of 20 μM at 28 days of culture. After coral tissues were removed with 10% sodium hypochlorite, the remaining skeletons on the dishes were washed with distilled water and observed under a fluorescence stereomicroscope (SZX16; Olympus, Tokyo, Japan). Ultrastructure of skeletons was also observed with a scanning electron microscope (JSM-7160F, Joel, Tokyo, Japan).
2.6 Histological analysis and immunohistochemistry
Microcolonies were fixed in Zinc Formal-Fixx (Thermo Shandon, Pittsburgh, PA) diluted 1:5 in FASW. After decalcification with 5% formic acid, samples were preserved in 70% ethanol before use. For histological analysis, a portion of each sample was embedded in paraplast plus (Sherwood Medical, St. Louis, USA) and cut into 4-μm serial sections. Hydrated sections were stained with Hematoxylin and Eosin Y (Thermo Scientific Shandon). To determine numbers of zooxanthellae in polyps, 10 to 15 sections (approximately 20% of the total sections/polyp) were randomly photographed for each polyp. Tentacle areas and the number of zooxanthellae in the tentacles were determined using Image J software, and the average concentration of zooxanthellae/10,000 μm2 was estimated for each polyp. Immunohistochemistry to detect germline cells was performed according to the methodology of our previous report (Shikina et al., 2012). For the primary antibody reaction, hydrated sections were incubated with a polyclonal antibody against Eapiwi (Shikina et al., 2015) diluted 1:4,000 in phosphate buffered saline with Tween-20 (PBST) and 5% skim milk for 16 hr at 4°C. After several washes with PBST, sections were incubated with a biotinylated goat anti-rabbit IgG antibody (Vector Laboratories, Burlingame, CA) diluted 1:4,000 in PBST with 5% skim milk for 1 h for the secondary antibody reaction. Sections were then incubated in standard avidin‐biotinylated‐ peroxidase complex solution (ABC kit, Vector Laboratories) for 30 min. Immunoreactivity (brown color) was visualized using 3,3′‐diaminobenzidine (DAB, Sigma‐Aldrich). Sections were then counter-stained with Haematoxylin (blue-purple color), and observed under an IX71SF1 microscope (Olympus).
2.7 Induction of bleaching
Bleaching was induced by menthol treatment (final concentration: 0.19 mM) according to a previous report (Wang et al., 2012). Bleaching status was monitored by observing the chlorophyll autofluorescence of zooxanthellae (excitation at 559 nm) under a fluorescentce microscope. Histological analysis was also performed to further confirm bleaching status according to methods described above. Bleached microcolonies were maintained by feeding Artemia (500 Artemia/dish) and/or liquid-type coral food (Product name: Reef Energy, Red Sea Aquatics Ltd.) once every three days. After feeding, all seawater in the culture dishes was replaced with fresh FASW.
2.8 Zooxanthella enrichment, labeling with fluorescent dye, and infection
P. damicornis fragments (1 cm x 1 cm in size) were immersed in 0.43 U/mL trypsin (Worthington Biochemicals, Freehold, NJ) in FASW for 2 h at room temperature (Chiu et al., 2019). Dissociated cells were collected, washed with FASW, and resuspended in FASW. Subsequently, a Percoll gradient was used to enrich zooxanthellae. Briefly, Percoll solutions (Cytiva, Sweden) with different concentrations, i.e., 30%, 50%, 60%, and 70%, were prepared by mixing Percoll with FASW containing 0.1% bovine serum albumin (BSA). BSA was added to increase cell recovery. The Percoll gradient was created in a 15-mL tube, and the dissociated coral cell suspension was placed on the top layer. After centrifugation at 1,000 rpm for 10 min, the fraction with enriched zooxanthellae was collected in a 15-mL tube and washed three times with FASW containing 0.1% BSA. Then, cell membranes of zooxanthellae were labeled with PKH26 fluorescent dye (Sigma-Aldrich, USA) according to the manufacture’s protocol. For zooxanthella infection, PKH26-labeled zooxanthellae (1×106 cells) were resuspended in 50 μL of FASW, and the zooxanthellae suspension was injected onto bleached microcolonies that were maintained in culture dishes. After 3 and 24 h, the presence of the exogenous zooxanthellae was observed under a fluorescence stereomicroscope (SZX16; Olympus).
2.9 Self-non-self-recognition
Two microcolonies prepared from identical or different P. damicornis colonies were cultured attaching to each other. The microcolonies were fixed to bottoms of plastic dishes (Jet Bio-Filteration Co., Ltd.) with underwater superglue (ISTA). Reactions occurring between the two microcolonies were observed and photographed under a stereo microscope (EZ4, Leica) at 0, 7, 14, 21, and 28 days of culture. The experiment was designed based on previous studies (Jokiel and Bigger, 1994; Nozawa and Loya, 2005; Puill-Stephan et al., 2012). Artemia feeding and water replacement were carried out once every three days according to the method described above. Experiments were performed 3 times using 3 different colonies combinations, and coral conditions were evaluated using the health indices described above.
2.10 Effects of benzophenone-3 and Irgarol 1051 on microcolonies
Microcolonies were treated with BP3 and Irgarol 1051 (Sigma-Aldrich) at concentrations of 0, 2, 4, 6, 8, 10 µg/mL, and 0, 1, 10 µg/mL, respectively, according to previous reports (Danovaro et al., 2008; Hirayama et al., 2017; Fel et al., 2019). Vehicle (dimethyl sulfoxide or acetone) was added to the control group. Pocillopora damicornis microcolonies were cultured in the CLD platform for 1-2 weeks, and microcolonies were photographed at different times. Survival, abnormality rate, growth, bleaching levels, and maximum quantum yield (Fv/Fm) of zooxanthellae were determined as described above.
2.11 Food intake ability
A cloning cylinder (10 mm x 10 mm, Sigma-Aldrich) was put into Petri dishes surrounding the cultured microcolony, and Artemia were added inside the cylinder at a concentration of 100 individuals/microcolony. Microcolonies were allowed to feed for 3 h, and then the remaining Artemia in the cylinder were collected and counted. The number of Artemia consumed by a microcolony was determined as the difference between the initial and final number of Artemia. Then, the polyp number of the microcolony was determined, and the number of ingested Artemia/hour/polyp was calculated and compared among conditions.
2.12 Statistics
All data are presented as means ± standard error (SE). Statistical differences between two groups were determined using Student’s t-test. For comparisons among more than three groups, statistical significance was determined using one-way ANOVA followed by Tukey’s test using a statistical significance level of P<0.05. All analyses were performed using the Statistical Package for the Social Sciences (SPSS) software.
3 Results
3.1 Maintaining corals in a laboratory dish and optimizing culture conditions
We first attempted to develop indexes to assess the health status of corals in culture. Generally, healthy corals grow while maintaining their tissue structural integrity and their symbiotic relationship with zooxanthellae. Additionally, they not only reproduce asexually to increase polyp numbers, but also grow via skeletogenesis. We then developed a non-destructive coral health index to evaluate and compare the status of corals in culture by quantifying various coral characteristics (Table S1; Figure S1).
To optimize culture conditions, first, effects of feeding on microcolonies were investigated. We compared survivorship, growth rates, and color changes during a 4-week culture period between a group fed Artemia twice a week (fed) and a group not fed (non-fed). For the culture system, type1 LED lights, which have a bluish spectrum (intensity: 20-40 μmol/m2/s) were used. Seawater was replaced every three days, and dishes were covered with a lid and sealed with parafilm to prevent seawater evaporation. As a result, although microcolonies were cultured in a static, closed environment, the survival rate was 100% in both groups (Figure 1B). Microcolonies settled on surfaces of the culture dishes and expanded their tissues, also producing new polyps by asexual reproduction in both groups (Figure 1C). The growth rate (change in area) was 3.3-fold, with no significant difference between the groups (Figure 1D). However, differences were observed in coral color, with the non-fed group showing significant bleaching, while feeding mitigated bleaching (Figure 1E).
Next, we cultured microcolonies under two types of LED (intensity: 20-40 μmol/m2/s) with different spectra. LED1 has a mainly blue spectrum, while LED2 has red and green wavelengths as well as blue (Figure 1F). The survival rate of both groups was 100%, and a slightly higher coral growth was observed under LED 1 than under LED 2 (Figures 1G, H). However, slight coral bleaching was observed under LED1, but not under LED2 (Figures 1G, I). To further optimize lighting conditions, the effect of light intensity was investigated. Considering future applications to the study of coral-algal symbiosis, LED2, which caused less bleaching, was employed in this experiment. Microcolonies were cultured at 20-40, 60-80, or 100-120 μmol/m2/s for 4 weeks, and survival rates of all groups were 100%. The highest growth was observed in the 60-80 μmol/m2/s group (Figures 1J, K). In contrast, in the 20-40 μmol/m2/s group, no bleaching was observed, and coral color became even darker than at culture initiation (Figure 1L). Slight bleaching was observed in other groups (Figure 1L). Maximum quantum yield (Fv/Fm) was highest in the 20-40 μmol/m2/s group during the 4-week culture period (Figure 1M). Given the results of the above experiments, with future applications in mind, we developed the following culture protocol for P. damicornis microcolonies using the CLD platform (Table S2).
3.2 Long-term culture and propagation of microcolonies using the CLD platform
Subsequently, we examined whether long-term culture and growth of microcolonies using the CLD platform were feasible. All P. damicornis microcolonies exhibited 100% survival and grew for 3 months without tissue abnormalities (Figures S2A–C). They all settled on the dish surface during the one month of culture, and gradually expanded their tissues, increasing in polyp numbers and mass (Figures S2D–F). Tentacle numbers in newly produced polyps were the same as those of original polyps (Figure S2G). Distinct differences were found in growth status of the colonies. During the 3-month culture period, the fastest growing colony, colony 2, produced about 80 new polyps with an apparent mass increase, and its area increased about 12 times. In contrast, the slowest growing colony, colony 3, produced only about 30 new polyps, and its area increased about 7 times (Figure S2E, F, H). Although there were occasional microcolonies that bleached slightly, zooxanthellae in microcolonies retained their photosynthetic activity over the 3-month culture period (Figure S2I).
We examined whether fragmentation of microcolonies grown in vitro and reseeding are possible. From microcolonies cultured for 5 months (Figures 2A–C), newly generated parts were fragmented to an appropriate size with tweezers on the culture dishes, and seeded into new culture dishes (Figure 2D). All fragments survived and grew for at least 6 weeks without tissue abnormalities or bleaching (Figures 2E–G). Zooxanthellae in the fragments retained their photosynthetic activity throughout the culture period (Figure 2H).
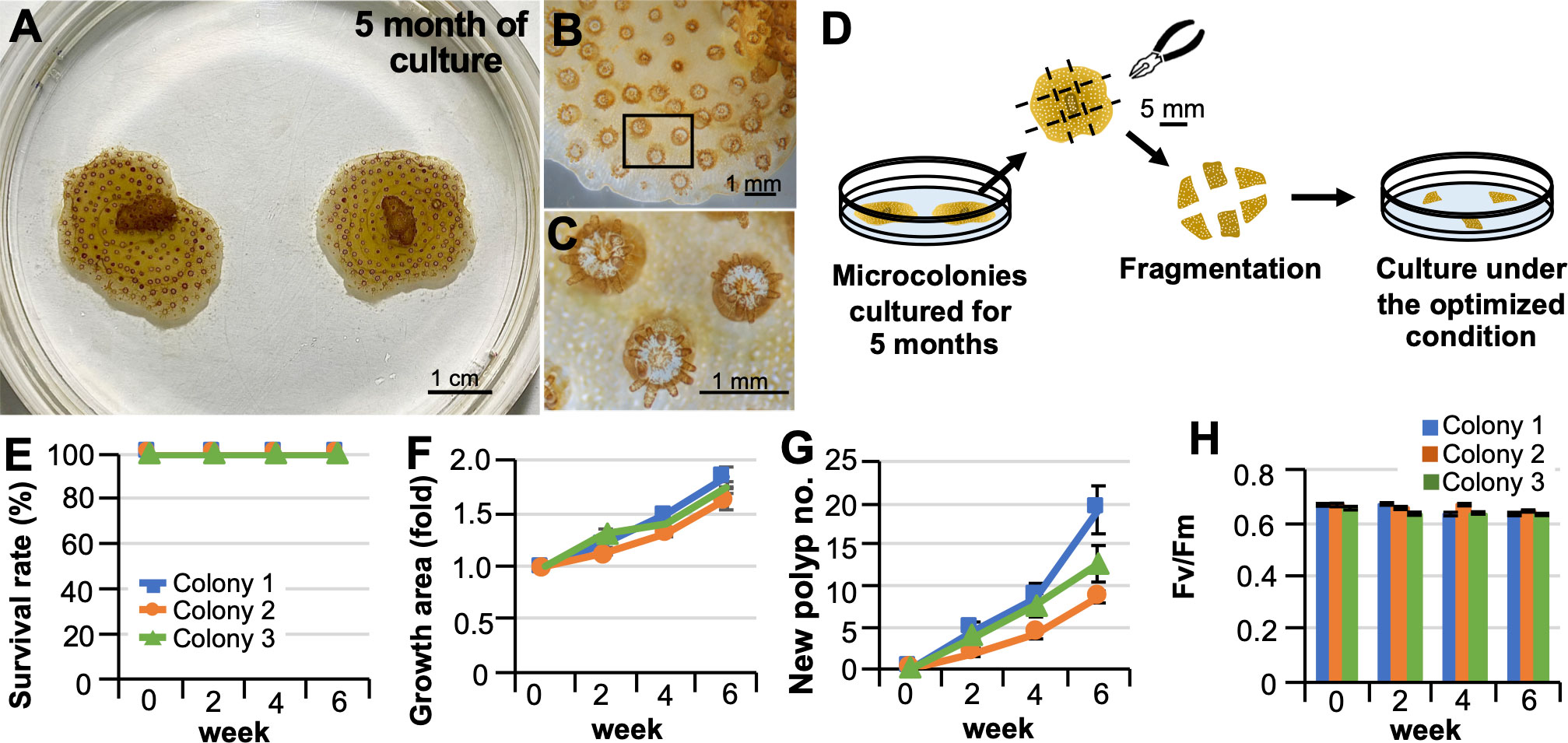
Figure 2 Fragmentation of microcolonies grown in vitro and their re-seeding. (A) Representative pictures of microcolonies cultured in laboratory dishes for 5 months under optimized conditions. (B) Representative micrograph of part of the grown area in vitro. (C) A higher magnification view of the inset shown in (B) Note that polyps produced in vitro can extend their tentacles. Zooxanthellae (brown) can be observed within their tentacles. (D) Schematic diagram of the procedure for fragmentation of microcolonies grown in vitro and their culture in a laboratory dish. (E–H) Results of the culture after 6 weeks. Results show the average of triplicate experiments performed using 3 colonies. (E) Survival rate. (F) Changes in areas covered by microcolonies. (G) Changes in the color of microcolonies. (H) Maximum quantum yield (Fv/Fm) of zooxanthellae in microcolonies.
3.3 Monitoring changes in seawater chemistry
With the CLD platform, seawater in culture dishes is replaced every 3 days. Salinity of seawater in the culture dishes of P. damicornis microcolonies showed no significant changes, and levels of ammonia, NO2, NO3, and PO4 were very low or almost undetectable after 3 days of culture. DO increased slightly. However, the pH decreased from 8.3 to 7.4 and levels of Ca2+ and total alkalinity also decreased significantly (Table 1).
3.4 Observation and characterization of biological properties of microcolonies in culture
Microcolonies exhibited asexual reproduction and continuously increased their polyp numbers. Microscopic observation of the budding process at 1 month of culture revealed small aggregations of zooxanthellae, from which new polyps with tentacles appeared in an average of 6-8 days (Figure 3A; A1-A4). In cultures, releases of planula larvae were occasionally observed in microcolonies cultured for more than 16 days (Figure 3B). Released planulae were morphologically normal (Figure 3C) and retained their settlement and metamorphosis abilities (Figure 3D). Immunohistochemical analysis demonstrated the presence of spermaries (male germ cell clusters) in microcolonies cultured for 1 month (Figure 3E). Microcolonies were also observed to actively expand tissues on the dish surface via skeletogenesis (Figure 3F). Skeletogenesis in culture could also be visualized by calcein incorporation (Figure 3G). Microscopic observation of skeletons formed in culture demonstrated the presence of skeletal elements such as sclerosepta and coenosteal surface spines. Desmocyte attachment scars, which are necessary for polyp anchoring, were also observed by electron microscopy (Figures 3H, I). Additionally, microcolonies in cultures fed actively. When Artemia were added to cultures, polyps used their tentacles to prey on them (Figure 3J). The time between capture and incorporation into the body was 30-60 s, and incorporated Artemia were digested in 5-6 h (Figures 3K–M). Furthermore, polyps had the ability to sense external stimuli, e.g., vibrations and strong water currents, and they contracted in response to these stimuli within seconds (Figures 3N–Q).
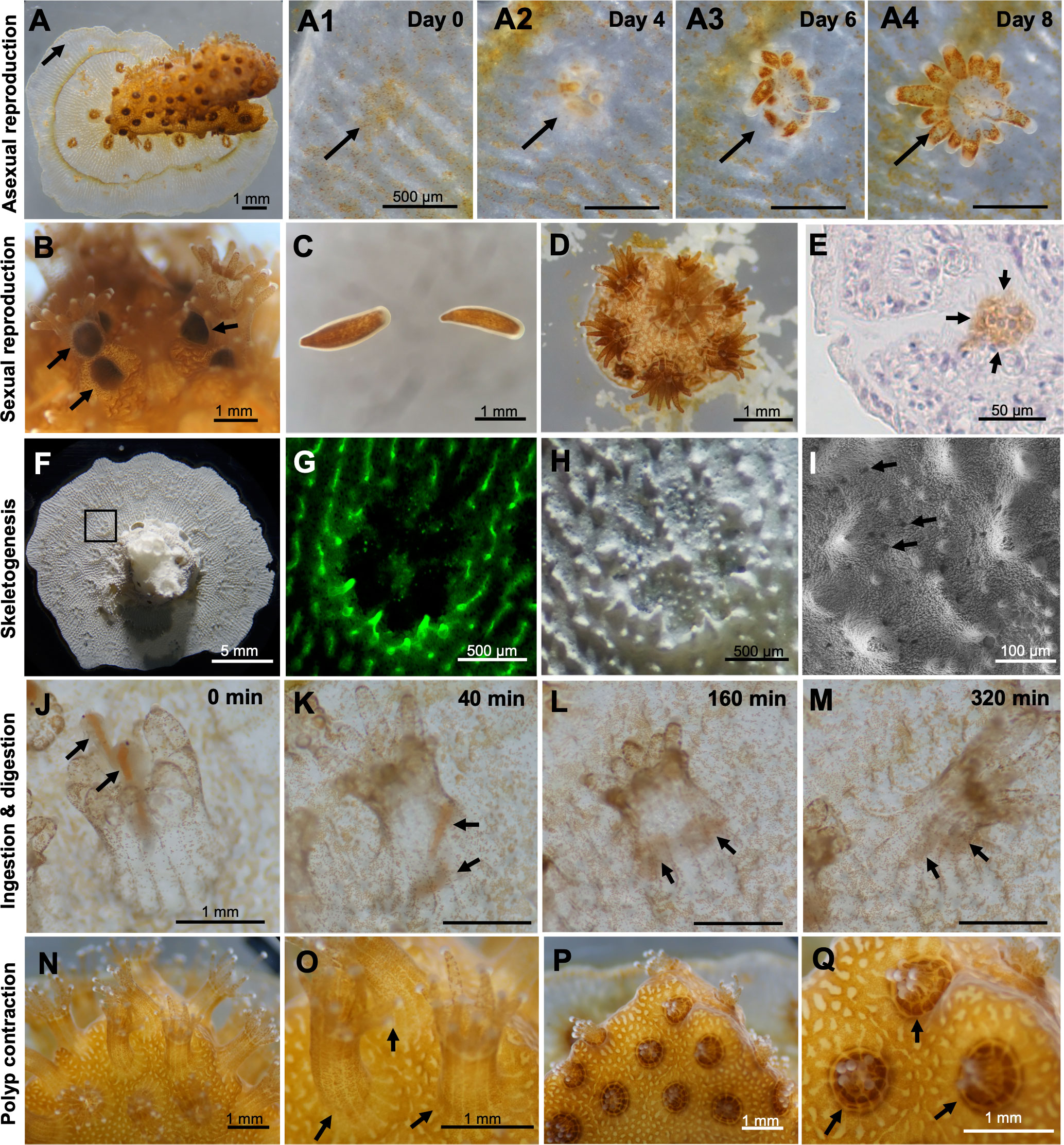
Figure 3 Observation and characterization of biological properties of microcolonies in culture. (A) A microcolony cultured in CLD for 1 month. A1-A4. Time series observations of the budding process. Arrows indicate budding sites or a newly forming polyp. (B) Brooding of planula larvae by some polyps in a microcolony cultured in CLD for 16 days. The microcolonies were prepared from the P. damicornis colony fragments collected in the field during the reproductive season (May-June) in the northern Taiwan. (C) Released planulae. (D) A small new colony formed by a released planula in vitro. (E) Immunohistochemical demonstration of the presence of a spermary in the microcolony cultured in CLD for 1 month. Arrows indicate a male germ cell cluster (brown color). Cell nuclei were stained with hematoxylin (blue-purple color). (F) Skeleton of a microcolony cultured for 1 month. (G) Calcein incorporation into the skeleton. (H) Microscopy of the skeleton formed in vitro. (I) Electron microscopy of the skeleton formed in vitro. Desmocyte attachment scars, which are necessary for polyp anchoring, are observed. (J–M) Time-series observations of predation and digestion of Artemia by P. damicornis polyp. Arrows indicate Artemia. (N–Q) Polyp contraction in response to vibration.(N, O) Microcolonies before stimulation. (P, Q) Microcolonies after stimulation.
3.5 Culturing other stony corals using the CLD platform
We examined the possibility of cultivating microcolonies of other stony corals from different families using the CLD platform. Three small-polyp species (polyps 1-2 mm in diameter, Acroporidae: Acropora tumida, Pocilloporidae: Stylophora pistillata, Poritidae: Porites lobata), and one larger-polyp species (polyps 5-10 mm in diameter, Merulinidae: Favites pentagona), were selected. These corals were cultured for more than 2 months with 100% survival rates and apparent growth (Table 2; Figure S3).
3.6 Potential application of CLD to biophysiological and ecotoxicological studies
In view of its application to biophysiological studies, first, experiments regarding coral-algal symbiosis study were conducted. Menthol treatment (Figure 4A) induced apparent bleaching of all microcolonies. Survival rates of menthol-treated microcolonies were approximately 80% at 2 weeks (Figure 2B). The number of symbiotic algae and the maximum quantum yield of zooxanthellae (Fv/Fm) in bleached microcolonies was significantly decreased (Figure 4C, D). Notably, infection of bleached microcolonies with labeled zooxanthellae was successful (Figures 4E–I). Microscopy revealed that labeled exogenous zooxanthellae were first distributed on the mesenterial filament 3 h after infection (Figures 4J, K). Then, they were distributed in the gastrodermis of tentacles (Figures 4L–N) and body wall (Figures S4A, B) 24 h after infection, as were endogenous zooxanthellae. The percentage of microcolonies with labeled exogenous zooxanthellae in tentacles or body walls 24 h after infection was about 60%. In the control group infected with unlabeled zooxanthellae, infection could not be confirmed due to an inability to distinguish between residual and exogenous zooxanthellae (Figures S4C, D).
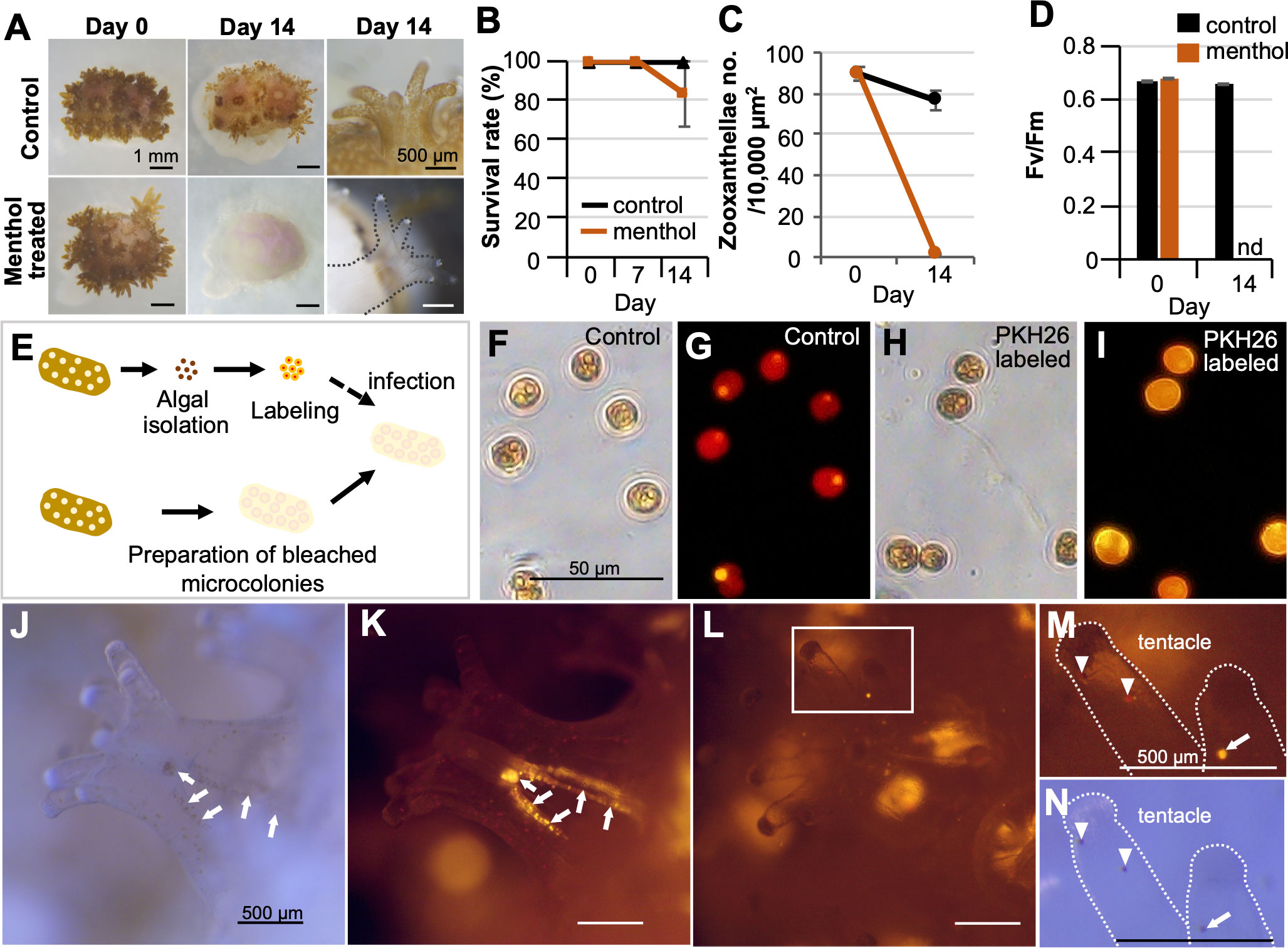
Figure 4 Potential application of CLD to coral-algal symbiosis studies. (A) Induction of bleaching by menthol treatment. The top panel shows the control Pocillopora damicornis microcolony and the lower panel shows the menthol-treated microcolony. (B) Survival rate during the 14-day culture period. (C) Number of zooxanthellae in control and menthol-treated microcolonies as estimated by histological analysis. (D) Maximum quantum yield (Fv/Fm) of zooxanthellae in control and menthol-treated microcolonies. nd, not detected. Data in (B–D) are expressed as the mean ± SE (n = 3 replicates for each group). (E) Schematic diagram showing the infection procedure of labeled zooxanthellae to bleached microcolonies. (F, G) Brightfield and fluorescence views of isolated zooxanthellae with no labeling (control). (H, I) Brightfield and fluorescence views of zooxanthellae with PKH26 fluorescence. (J–M) Brightfield and fluorescence views of the bleached microcolony that received PKH26-labeled zooxanthellae. (J, K) 3 h after infection. (L–N) 24 h after infection. Note that labeled exogenous zooxanthellae were distributed on the mesenterial filament 3 h after infection (arrows), and then distributed in the gastrodermis of tentacles (arrowhead) 24 h after infection. Arrowhead, endogenous zooxanthellae.
Subsequently, we examined whether the CLD platform can be used in studies of self-non-self-recognition mechanisms in corals. Microcolonies prepared from identical or different colonies were cultured attaching to each other, and reactions occurring between two microcolonies were examined. Fusion reactions occurred between identical colonies (Figures 5A–D) and rejection reactions between non-identical colonies (Figures 5E–H). No mortality or abnormalities were observed in any microcolonies during the experiment.
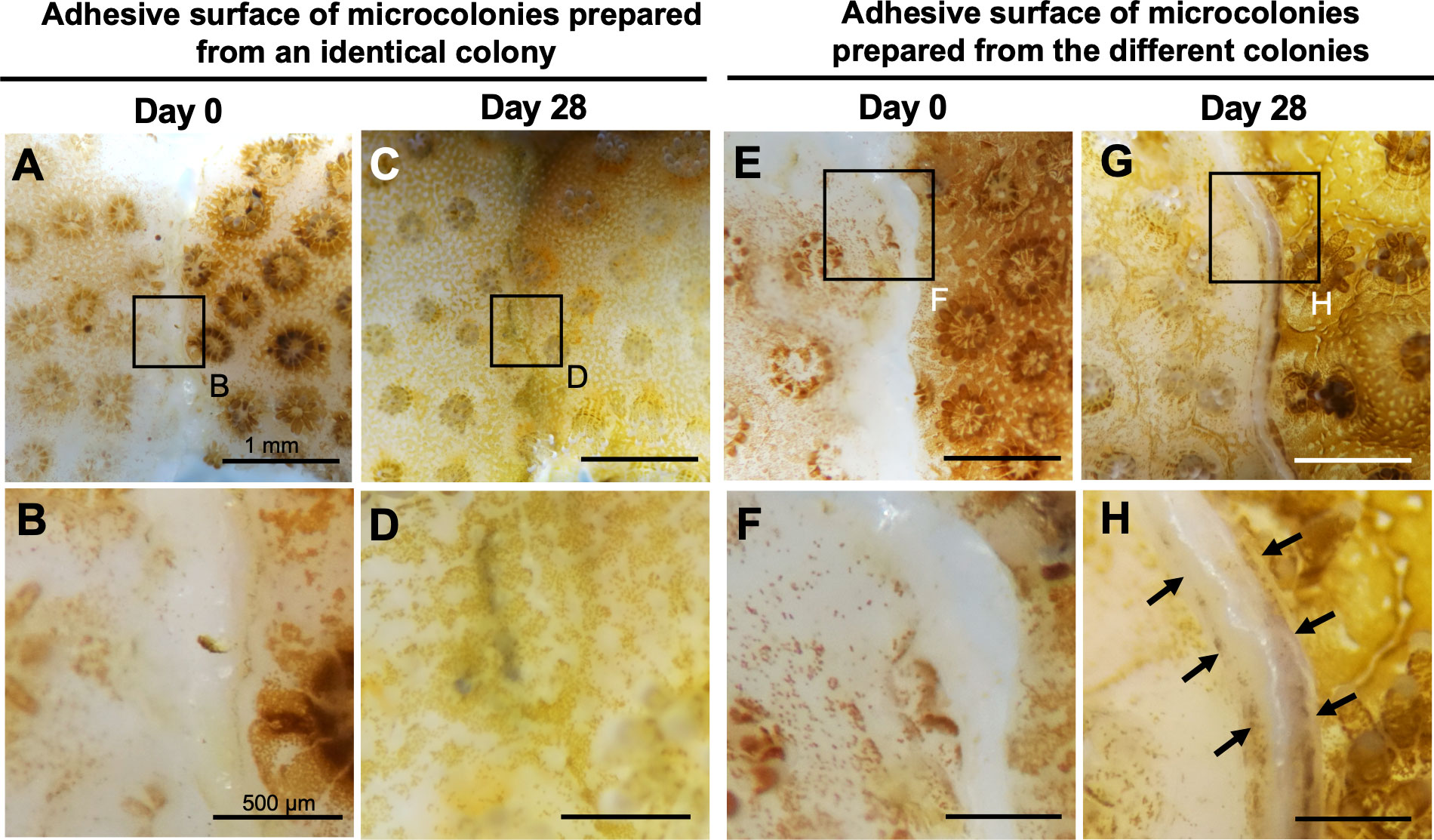
Figure 5 Potential application of CLD to self-non-self recognition mechanisms in corals. (A–D) Micrographs of the adhesive surface of two microcolonies prepared from the same parental colony. (E–H) Micrographs of the adhesive surface of two microcolonies prepared from two different parental colonies. Note that fusion reactions occurred between identical colonies and rejection reactions (arrows) occurred between non-identical colonies. Micrographs were taken at 0 and 28 days of culture. Boxed regions in (A, C, E, G) indicate the source of the higher magnification views (B, D, F, H respectively).
With a view to ecotoxicological applications, effects of benzophenone-3 (BP3, also known as oxybenzone, a sunscreen substance) and Irgarol 1051 (an organic biocide in marine antifouling paints) were evaluated on corals using the CLD platform. Concentrations of BP3 ≥4 μg/mL caused abnormalities in tissues, such as loss of the coenosarc at 0-2 days of culture, and eventually led to polyp detachment and mortality (Figures 6A–C, also see Figure S1). Interestingly, no obvious coral bleaching nor a decrease in the maximum quantum yield of zooxanthellae was observed (data not shown). In contrast, microcolonies treated with Irgarol 1051 showed distinct bleaching at concentrations of 1 μg/mL and 10 μg/mL at 7 days of culture with decreases in the maximum quantum yield of zooxanthellae (Figures 6D–F). There were no other readily noticeable external abnormalities, and polyps displayed approximately 80% survivorship at concentrations of 10 μg/mL for the two-week experimental period (Figure 6G). However, polyps of bleached microcolonies showed a tendency toward reduced feeding ability (Figure 6H).
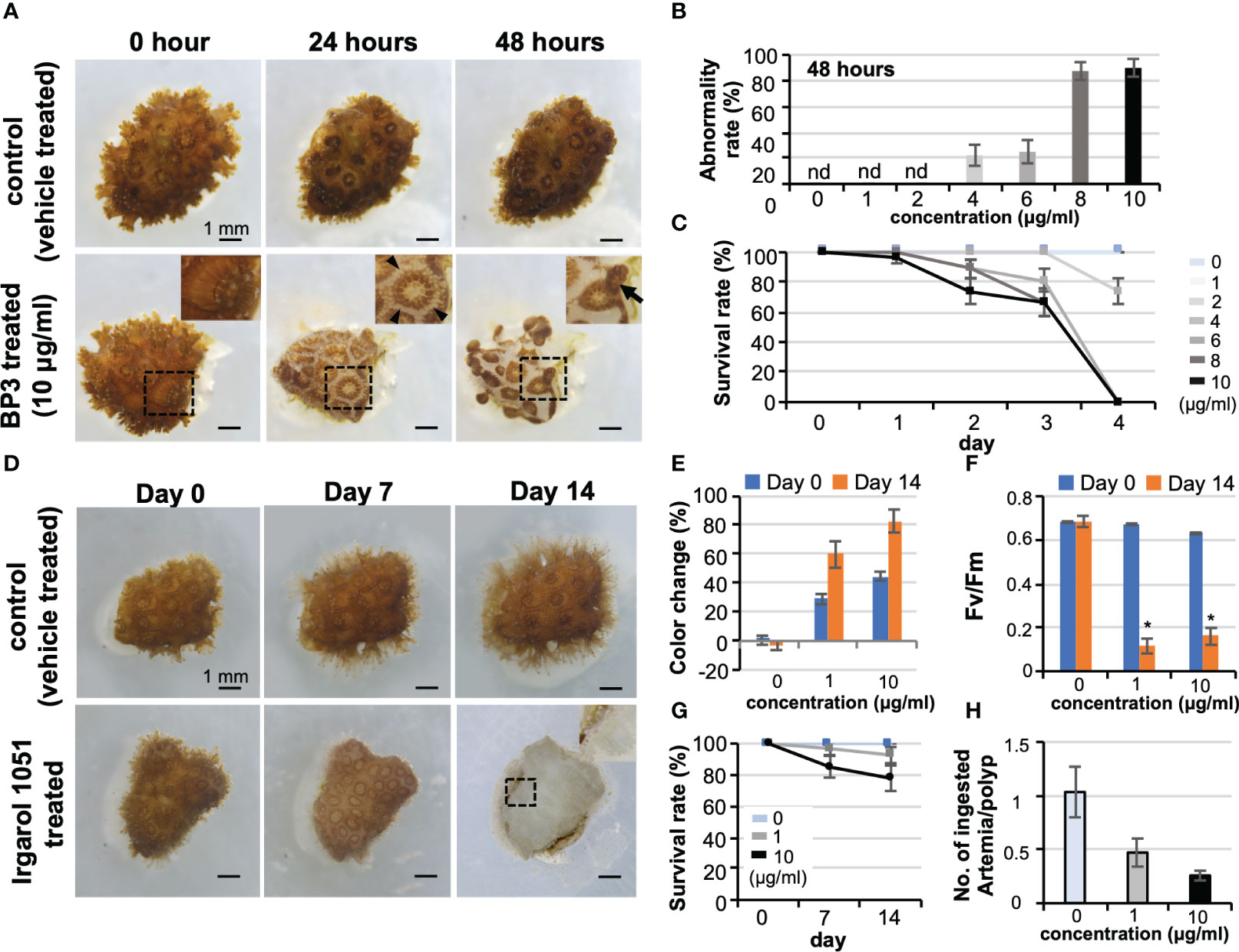
Figure 6 Potential application of CLD to ecotoxicological studies. (A) Effects of benzophenone-3 (BP3, a sunscreen substance) on Pocillopora damicornis microcolonies. The top panel shows the control microcolony (vehicle treated) and the lower panel shows the microcolony treated with 10 μg/mL BP3 (BP3 treated). The BP3-treated microcolony shows tissue abnormalities such as loss of coenosarc at 24 h of culture and detachment and mortality at 48 h of culture. Boxed regions indicate the source of the higher magnification views. Arrowheads indicate the loss of coenosarc and arrows indicate tissue detachment. (B) Abnormality rate of microcolonies at 48 h of culture. (C) Survival rate. Data are expressed as the mean ± SE (n = 3 replicates for each group). (D) Effects of Irgarol 1051 (an organic biocide in marine antifouling paints) on P. damicornis microcolonies. The top panel shows the control microcolony (vehicle-treated) and the lower panel shows the microcolony treated with 10 μg/mL Irgarol 1051 (Irgarol 1051 treated). The Irgarol 1051-treated microcolony shows distinct bleaching during 14 days of treatment. Boxed region, which shows an expanded polyp, indicates the source of the higher magnification view. (E–H) Results of Irgarol 1051 treatment for 14 days. Data are expressed as the mean ± SE (n = 3 replicates for each group). (E) Change in the color of microcolonies, (F) Maximum quantum yield (Fv/Fm) of zooxanthellae in the control and Irgarol 1051-treated microcolonies. *P < 0.05. (G) Survival rate. (H) Effects of Irgarol 1051 treatment on the feeding ability of P. damicornis microcolonies. nd, not detected.
4 Discussion
The present study successfully established techniques for stable long-term culture of P. damicornis microcolonies in Petri dishes while maintaining its biological properties. We have also shown that CLD is a useful experimental platform for conducting a variety of studies, such as coral-algal symbiosis, coral self-non-self-recognition, and impact assessment of environmental pollutants. Furthermore, the platform is able to maintain at least 4 other coral species from 4 other families for more than 2 months with 100% survivorship. These results demonstrate that the established platform is highly versatile, and has great potential for application to a variety of biophysiological and ecotoxicological studies of corals at cellular-level resolution. In general, stony corals are considered difficult to culture in the laboratory and their maintenance has required expensive equipment. Therefore, the CLD platform, which allows for easy and inexpensive coral maintenance, represents a technological breakthrough, enabling a variety of coral studies.
CLD has three important features as a study platform. First, small laboratory dishes and commercially available artificial seawater are used as the main materials. These items are inexpensive, readily accessible, and allow for microscopic observation of the experimental subject. Second, coral microcolonies (or nubbins) were used as the experimental samples. A number of genetically identical microcolonies can be easily be prepared from a single parental colony (Shafir et al., 2003; Shafir et al., 2006). Indeed, the preparation of coral microcolonies is easy and fast, and can be performed in the laboratory without special treatments or expensive equipment (Shafir et al., 2003; Shafir et al., 2006). Unlike embryos and larvae that can be obtained only during the coral reproductive season (Ohno et al., 2017), microcolonies can be prepared regardless of the season. Moreover, unlike isolated coral polyps from adult colonies using high salinity stress (Shapiro et al., 2016), microcolonies not only have high survivorship in CLD, but also retain polyp-polyp connections. Microcolonies also maintain polyp structure, tissue integrity, and a wide range of biological properties which are lost in the conventional coral cell/tissue culture studies (Rinkevich, 2005; Domart-Coulon and Ostrander, 2015). Third, aquaria/tanks and specialized devices are not used for coral maintenance in CLD. In previous studies, a system has been used in which coral microcolonies were grown on coverslip glued to the bottom of a Petri dish, and observed under a microscope for calcification processes (lateral skeleton preparative assay, Muscatine et al., 1997; Raz-Bahat et al., 2006). However, in that system, Petri dishes with corals are maintained in an indoor flowing aquarium (Raz-Bahat et al., 2006). A special device for maintaining coral microcolonies, a flow-through coral chamber, has recently been developed and migration of dinoflagellates has been studied (Bockel and Rinkevich, 2019). CLD, by contrast, does not require expensive aquaria/tanks system or specialized devices, and consists only of materials that can be easily prepared in any laboratory. Thus, it can be introduced into many laboratories with comparative ease. Assuming that each dish is equivalent to an independent tank, CLD will allow us to perform multiple biological experiments at once at a cellular level and low cost. A common incubator (about 300 L) could have 50 or more dishes in it at the same time. The CLD platform is small and requires only a small amount of seawater, so it may be useful for experiments in which small amounts of test chemicals are added. Establishment of techniques for gene functional analysis such as gene knockdown by RNA interference (Wilson and Doudna, 2013) and genome editing (Wang et al., 2016) will make CLD a more powerful platform for studying biological characteristics of corals at the molecular, cellular, and organismal levels.
Another important achievement of this study is the establishment of non-destructive coral health indexes. Conventionally, presence/absence of bleaching and photosynthesis ability of zooxanthellae (Fv/Fm) have been used as health indicators (Warner et al., 1999). In addition, the present study focused on and quantified intrinsic biological properties that can be analyzed based mainly on image analysis and direct counts, i.e., survivorship, abnormalities in tissue structural integrity, polyp number increment, size change, weight change, and the number of Artemia consumed per polyp. Using this more comprehensive health index on the CLD platform, status of living corals can be more reliably evaluated.
Our data demonstrate that CLD can be useful not only for studying coral physiology, but also ecotoxicology, to aid coral conservation. Currently, to identify cellular and molecular mechanisms underlying symbiotic partnerships between cnidarians and zooxanthellae, the symbiotic sea anemone Exaiptasia diaphana is used as a surrogate model system for corals because of the ease of rearing and manipulation of symbiotic algae (Belda-Baillie et al., 2002; Weis et al., 2008; Matthews et al., 2016). However, since corals and sea anemones are assumed to have diverged between 520 to 490 million years ago (Shinzato et al., 2011), symbiosis in corals and zooxanthellae may be regulated in a slightly different manner than that in sea anemones. By using CLD, cellular and molecular mechanisms underlying establishment, maintenance, and breakdown of symbiosis (bleaching) between corals and zooxanthellae could be better studied in the future. Additionally, CLD may also be useful to identify substances harmful to corals and to study their defense mechanisms. Chemical pollutants are currently becoming significant threats to coral reefs, especially in areas with high population density and tourist activities (van Dam et al., 2011). The negative impacts of personal care products such as sunscreens, and agricultural chemicals such as fungicides on corals have been demonstrated (Markey et al., 2007; Danovaro et al., 2008; Fel et al., 2019). High-throughput screening using CLD would allow more efficient identification of substances toxic to corals. Ultimately, data acquired with CLD will facilitate development of products with no negative impact on corals.
Seawater pH in the culture dishes decreased from 8.3 to 7.4 after 3 days of culture. Levels of calcium ion and total alkalinity also significantly decreased. These changes may be due to coral metabolism, coral-associated bacteria, or coral skeletogenesis in dishes (Tambutté et al., 1995; Langdon et al., 2010). Previously, acidified seawater was shown not only affects skeletogenesis, but also causes loss of the coenosarc (Fine and Tchernov, 2007; Kvitt et al., 2015). However, in CLD, microcolonies grow continuously with active skeletogenesis and no loss of coenosarc or subsequent polyp bailout events. This suggests that microcolonies in CLD are acclimating to transiently acidified conditions and that this change in seawater pH in culture does not have a significant impact. Further investigation of this phenomenon at molecular and cellular levels will provide insight into the mechanism of acclimation to changing environmental pH.
The presence of male germ clusters was confirmed by immunostaining in P. damicornis microcolonies that were maintained in culture for one month. Although it is unclear at this stage whether this cluster was already present at the start of the culture or whether it formed during the culture period, the results suggest that germ cell survival was maintained for at least one month on the CLD platform. By optimizing food type, feeding frequency, light conditions, and culture temperature, it may be possible to induce coral germ cell proliferation and differentiation in culture in the future.
When microcolonies prepared from the same colony were cultured in the CLD platform in an adherent state, tissues of the two microcolonies fused and the boundary became indistinct. On the other hand, when microcolonies prepared from different colonies were cultured together in an adherent state, a distinct border with skeleton was formed between them. Similar histocompatibility pattern have been reported in previous studies which mainly used aquarium systems (Jokiel and Bigger, 1994; Nozawa and Loya, 2005; Puill-Stephan et al., 2012). These results suggest that the CLD platform can be used to study self-non-self-recognition mechanisms in corals at the molecular and cellular levels. By comparing transcriptomic differences between the two conditions, it will be possible to identify genes participating in self- and non-self-recognition. Use of microcolonies of different species in experiments would also allow for studies on molecular mechanisms of defense and interspecific competition.
We demonstrated that increased numbers of coral polyps are achievable using newly produced coral polyps in laboratory dishes. Currently, coral polyps have been cultured for more than 6 months without noticeable problems, and polyp numbers are continually increasing. By increasing polyps in vitro and continuing with further passages, an experimental strain of corals could be established in the future. Further understanding fundamental differences in properties of corals in vitro and those in the wild is important to determine the potential and limitations of CLD. Multi-omics approaches (Pinu et al., 2019) may clarify differences in the future.
A variety of techniques for maintaining and propagating corals in laboratory dishes was established in this study. However, it is obvious that methodologies developed are not yet perfect and that required culture conditions may differ among species. In the future, further optimization of conditions such as culture temperature, light intensity, frequency of seawater change, and feeding frequency for each species will improve coral viability in culture and will accelerate their growth.
In summary, the present study established a platform enabling long-term culture of stony corals, the keystone organisms of coral reef ecosystems, in Petri dishes while maintaining their wide range of biological properties. This highly tractable platform is potentially applicable to a variety of coral studies ranging from physiology to ecotoxicology. CLD is expected to greatly accelerate coral research.
Data availability statement
The original contributions presented in the study are included in the article/Supplementary Material. Further inquiries can be directed to the corresponding author.
Ethics statement
All experiments were carried out in accordance with principles and procedures approved by the Institutional Animal Care and Use Committee, National Taiwan Ocean University.
Author contributions
SS conceived and designed the experiments. T-CL, Y-EC, Y-LC, Y-CC, Y-LC, NW, S-LT, YI, and SS performed the experiments. T-CL, Y-EC, Y-LC, Y-CC, Y-LC, NW, and SS analyzed the data. SS wrote the manuscript. All authors contributed to the article and approved the submitted article.
Funding
This research was supported by a grant from the Ministry of Science and Technology, Taiwan (111-2311-B-019-002) (to S.S.).
Acknowledgments
The authors gratefully acknowledge Ms. Yi-Chun Yu, Fisheries and Fishing Port Affairs Management Office, New Taipei City Government for permits for use and daily maintenance of coral aquaculture facilities.
Conflict of interest
The authors declare that the research was conducted in the absence of any commercial or financial relationships that could be construed as a potential conflict of interest.
Publisher’s note
All claims expressed in this article are solely those of the authors and do not necessarily represent those of their affiliated organizations, or those of the publisher, the editors and the reviewers. Any product that may be evaluated in this article, or claim that may be made by its manufacturer, is not guaranteed or endorsed by the publisher.
Supplementary material
The Supplementary Material for this article can be found online at: https://www.frontiersin.org/articles/10.3389/fmars.2023.1149495/full#supplementary-material
Supplementary Figure 1 | Representative micrographs of normal and abnormal Pocillopora damicornis microcolonies. A and B. A normal microcolony. Tentacles are extended, and no tissue detachment or loss is observed. C and D. An abnormal microcolony. Tentacles are not extended, and partial tissue loss (arrows) is observed. E and F. An abnormal microcolony. Tissue detachment (arrows) is observed.
Supplementary Figure 2 | Long-term culture of Pocillopora damicornis microcolonies in CLD. A and B. Representative pictures of microcolonies before (Day 0, A) and after (3 months of culture, B) culturing in a laboratory dish. C-I. Results of the culture for 3 months. Data are expressed as the mean ± SE (n = 3 replicates for each group). C. Survival rate. D. Settlement rate. E. Newly produced polyp number. F. Increased weight. G. Tentacle numbers of polyps originally present in the microcolonies (original) and that of a newly produced polyp in vitro. H. Changes in the area covered by microcolonies. I. Maximum quantum yield (Fv/Fm) of zooxanthellae in microcolonies.
Supplementary Figure 3 | Culturing microcolonies of other stony coral species using the CLD platform. Left panel. Representative micrographs of microcolonies of Acropora tumida (Family Acroporidae), Stylophora pistillata (Family Pocilloporidae), Porites lobata (Family Poritidae), and Favites pentagona (Family Merulinidae) at 0 days of culture. Right panel. Micrographs of the same microcolonies after 2 months of culture. Settlement on the culture dish surface and an increased number of polyps by asexual reproduction are observed.
Supplementary Figure 4 | A and B. Brightfield and fluorescence views of a polyp in the bleached microcolony that received PKH26-labeled zooxanthellae. ten, tentacle; bw, body wall of the polyp; Arrowhead, PKH26-labeled exogenous zooxanthellae. C and D. Brightfield and fluorescentce views of a polyp in the bleached microcolony that received non-labeled zooxanthellae (control). Photographs were taken at 24 h after infection.
References
Barton J. A., Willis B. L., Kate S., Hutson K. S. (2015). Coral propagation: a review of techniques for ornamental trade and reef restoration. Rev. Aquac. 9 (3), 238–256. doi: 10.1111/raq.12135
Belda-Baillie C. A., Baillie B. K., Maruyama T. (2002). Specificity of a model cnidarian–dinoflagellate symbiosis. Biol. Bull. 202 (1), 74–85. doi: 10.2307/1543224
Bockel T., Rinkevich B. (2019). Rapid recruitment of symbiotic algae into developing scleractinian coral tissues. J. Mar. Sci. Eng. 7 (9), 306. doi: 10.3390/jmse7090306
Bossert P., Galliot B. (2012). How to use hydra as a model system to teach biology in the classroom. Int. J. Dev. Biol. 56 (6-8), 637–652. doi: 10.1387/ijdb.123523pb
Chiu Y. L., Shikina S., Chang C. F. (2019). Testicular somatic cells in the stony coral euphyllia ancora express an endogenous green fluorescent protein. Mol. Rep. Dev. 86 (7), 798–811. doi: 10.1002/mrd.23157
Craggs J., Guest J. R., Davis M., Simmons J., Dashti E., Sweet M. (2017). Inducing broadcast coral spawning ex situ: closed system mesocosm design and husbandry protocol. Ecol. Evol. 7 (24), 11066–11078. doi: 10.1002/ece3.3538
Cunning R., Bay R. A., Gillette P., Baker A. C., Traylor-Knowles N. (2018). Comparative analysis of the Pocillopora damicornis genome highlights role of immune system in coral evolution. Sci. Rep. 8 (1), 16134. doi: 10.1038/s41598-018-34459-8
Danovaro R., Bongiorni L., Corinaldesi C., Giovannelli D., Damiani E., Astolfi P., et al. (2008). Sunscreens cause coral bleaching by promoting viral infections. Environ. Health Perspect. 116 (4), 441–447. doi: 10.1289/ehp.10966
Darling J. A., Reitzel A. R., Burton P. M., Mazza M. E., Ryan J. F., Sullivan J. C., et al. (2005). Rising starlet: the starlet sea anemone, Nematostella vectensis. BioEssays 27 (2), 211–221. doi: 10.1002/bies.20181
Domart-Coulon I., Ostrander G. K. (2015). Coral cell and tissue culture methods. Dis. Coral Ch 37, 489–505. doi: 10.1002/9781118828502.ch37
Fel J. P., Lacherez C., Bensetra A., Sakina M., Béraud E., Léonard M., et al. (2019). Photochemical response of the scleractinian coral Stylophora pistillata to some sunscreen ingredients. Coral Reefs 38, 109–122. doi: 10.1007/s00338-018-01759-4
Fine M., Tchernov D. (2007). Scleractinian coral species survive and recover from decalcification. Science 315 (5820), 1811. doi: 10.1126/science.1137094
Halpern B. S., Longo C., Hardy D., McLeod K. L., Samhouri J. F., Katona S. K., et al. (2012). An index to assess the health and benefits of the global ocean. Nature 488 (7413), 615–620. doi: 10.1038/nature11397
Hirayama K., Takayama K., Haruta S., Ishibashi H., Takeuchi I. (2017). Effect of low concentrations of irgarol 1051 on RGB (R, red; G, green; b, blue) colour values of the hard-coral Acropora tenuis. Mar. pollut. Bull. 124 (2), 678–686. doi: 10.1016/j.marpolbul.2017.05.027
Hoegh-Guldberg O. (1999). Coral bleaching, climate change and the future of the world’s coral reefs. Mar. Freshw. Res. 50 (8), 839–866. doi: 10.1071/MF99078
Hoegh-Guldberg O., Poloczanska E. S., Skirving W., Dove S. (2017). Coral reef ecosystems under climate change and ocean acidification. Front. Mar. Sci. 4. doi: 10.3389/fmars.2017.00158
Hughes T. P., Anderson K. D., Connolly S. R., Heron S. F., Kerry J. T., Lough J. M., et al. (2018). Spatial and temporal patterns of mass bleaching of corals in the anthropocene. Science 359 (6371), 80–83. doi: 10.1126/science.aan8048
Hughes T. P., Barnes M. L., Bellwood D. R., Cinner J. E., Cumming G. S., Jackson J. B. C., et al. (2017). Coral reefs in the anthropocene. Nature 546 (7656), 82–90. doi: 10.1038/nature22901
Jokiel P. L., Bigger C. H. (1994). Aspects of histocompatibility and regeneration in the solitary reef coral Fungia scutaria. Biol. Bull. 186 (1), 72–80. doi: 10.2307/1542037
Kvitt H., Kramarsky-Winter E., Maor-Landaw K., Zandbank K., Kushmaro A., Rosenfeld H., et al. (2015). Breakdown of coral colonial form under reduced pH conditions is initiated in polyps and mediated through apoptosis. Proc. Natl. Acad. Sci. U.S.A. 112 (7), 2082–2086. doi: 10.1073/pnas.1419621112
Langdon C., Gattuso J. P., Andersson A. (2010). “Measurements of calcification and dissolution of benthic organisms and communities,” in Guide to best practices for ocean acidification research and data reporting (Luxembourg: Publications Office of the European Union).
Leal M. C., Ferrier-Pagès C., Petersen D., Osinga R. (2014). Coral aquaculture: applying scientific knowledge to ex situ production. Rev. Aquac. 8 (2), 136–153. doi: 10.1111/raq.12087
Markey K. L., Baird A. H., Humphrey C., Negri A. P. (2007). Insecticides and a fungicide affect multiple coral life stages. Mar. Ecol. Prog. Ser. 330, 127–137. doi: 10.3354/meps330127
Matthews J. L., Sproles A. E., Oakley C. A., Grossman A. R., Weis V. M., Davy S. K. (2016). Menthol-induced bleaching rapidly and effectively provides experimental aposymbiotic sea anemones (Aiptasia sp.) for symbiosis investigations. J. Exp. Biol. 219 (Pt 3), 306–310. doi: 10.1242/jeb.128934
Muscatine L., Tambutte E., Allemand D. (1997). Morphology of coral desmocytes, cells that anchor the calicoblastic epithelium to the skeleton. Coral Reefs. 16 (4), 205–213. doi: 10.1007/s003380050075
Nozawa Y., Loya Y. (2005). Genetic relationship and maturity state of the allorecognition system affect contact reactions in juvenile Seriatopora corals. Mar. Ecol. Prog. Ser. 286, 115–123. doi: 10.3354/meps286115
Odum H. T., Odum E. P. (1955). Trophic structure and productivity of a windward coral reef community on eniwetok atoll. Ecol. Monog. 25 (3), 291–320. doi: 10.2307/1943285
Ohno Y., Iguchi A., Shinzato C., Inoue M., Suzuki A., Sakai K., et al. (2017). An aposymbiotic primary coral polyp counteracts acidification by active pH regulation. Sci. Rep. 7, 40324. doi: 10.1038/srep40324
Omori M. (2019). Coral restoration research and technical developments: what we have learned so far. Mar. Biol. Res. 15 (7), 377–409. doi: 10.1080/17451000.2019.1662050
Pinu F. R., Beale D. J., Paten A. M., Kouremenos K., Swarup S., Schirra H. J., et al. (2019). Systems biology and multi-omics integration: viewpoints from the metabolomics research community. Metabolites 9 (4), 76. doi: 10.3390/metabo9040076
Puill-Stephan E., Willis B. L., Abrego D., Raina J. B., van Oppen M. J. H. (2012). Allorecognition maturation in the broadcast-spawning coral Acropora millepora. Coral Reefs 31 (4), 1019–1028. doi: 10.1007/s00338-012-0912-1
Raz-Bahat M., Erez J., Rinkevich B. (2006). In vivo light-microscopic documentation for primary calcification processes in the hermatypic coral Stylophora pistillata. Cell Tissue Res. 325 (2), 361–368. doi: 10.1007/s00441-006-0182-8
Rinkevich B. (2005). Marine invertebrate cell cultures: new millennium trends. Mar. Biotechnol. 7 (5), 429–439. doi: 10.1007/s10126-004-0108-y
Selig E. R., Bruno J. F. (2010). A global analysis of the effectiveness of marine protected areas in preventing coral loss. PloS One 5 (2), e9278. doi: 10.1371/journal.pone.0009278
Shafir S., Rijn J. V., Rinkevich B. (2003). The use of coral nubbins in coral reef ecotoxicology testing. Biomol Eng. 20 (4-6), 401–406. doi: 10.1016/s1389-0344(03)00062-5
Shafir S., Rijn J. V., Rinkevich B. (2006). Coral nubbins as source material for coral biological research: a prospectus. Aquaculture 259 (1-4), 444–448. doi: 10.1016/j.aquaculture.2006.05.026
Shapiro O. H., Kramarsky-Winter E., Gavish A. R., Stocker R., Vardi A. (2016). A coral-on-a-chip microfluidic platform enabling live-imaging microscopy of reef-building corals. Nat. Commun. 7, 10860. doi: 10.1038/ncomms10860
Sheikh M. A., Fujimura H., Imo T. S., Miyagi T., Oomori T. (2009). Contamination and impacts of new antifouling biocide irgarol-1051 on subtropical coral reef waters. Int. J. Environ. Sci. Technol. 6 (3), 353–358. doi: 10.1007/BF03326073
Shikina S., Chen C. J., Liou J. Y., Shao Z. F., Chung Y. J., Lee Y. H., Chang c. F. (2012). Germ cell development in the scleractinian coral Euphyllia ancora (Cnidaria, anthozoa). PloS One. 7 (7), e41569. doi: 10.1371/journal.pone.0041569
Shikina S., Chung Y. L., Wang H. M., Chiu Y. L., Shao Z. F., Lee Y. H., et al. (2015). Immunohistochemical localization of early germ cells in a stony coral, Euphyllia ancora: potential implications for a germline stem cell system in coral gametogenesis. Coral Reefs. 34 (2), 639–653. doi: 10.1007/s00338-015-1270-6
Shinzato C., Shoguchi E., Kawashima T., Hamada M., Hisata K., Tanaka M., et al. (2011). Using the Acropora digitifera genome to understand coral responses to environmental change. Nature 476 (7360), 320–323. doi: 10.1038/nature10249
Tambutté E., Allemand D., Bourge I., Gattuso J. P., Jaubert J. (1995). An improved 45Ca protocol for investigating physiological mechanisms in coral calcification. Mar. Biol. 122 (3), 453–459. doi: 10.1007/BF00350879
Traylor-Knowles N., Granger B. R., Lubinski T. J., Parikh J. R., Garamszegi S., Xia Y., et al. (2011). Production of a reference transcriptome and transcriptomic database (PocilloporaBase) for the cauliflower coral, Pocillopora damicornis. BMC Genomics 12, 585. doi: 10.1186/1471-2164-12-585
van Dam J. W., Negri A. P., Uthicke S., Mueller J. F. (2011). “Chemical pollution on coral reefs: exposure and ecological effects,” in Ecological impacts of toxic chemicals (Amsterdam: Bentham Science Publishers), 187–211. doi: 10.2174/978160805121211101010187
Venn A. A., Wilson M. A., Trapido-Rosenthal H. G., Keely B. J., Douglas A. E. (2006). The impact of coral bleaching on the pigment profile of the symbiotic alga, Symbiodinium. Plant Cell Environ. 29 (12), 2133–2142. doi: 10.1111/j.1365-3040.2006.001587.x
Wang J. T., Chen Y. Y., Tew K. S., Meng P. J., Chen C. A. (2012). Physiological and biochemical performances of menthol-induced aposymbiotic corals. PloS One 7 (9), e46406. doi: 10.1371/journal.pone.0046406
Wang H., La Russa M., Qi L. S. (2016). CRISPR/Cas9 in genome editing and beyond. Annu. Rev. Biochem. 85, 227–264. doi: 10.1146/annurev-biochem-060815-014607
Warner M. E., Fitt W. K., Schmidt G. W. (1999). Damage to photosystem II in symbiotic dinoflagellates: a determinant of coral bleaching. Proc. Natl. Acad. Sci. U.S.A. 96 (14), 8007–8012. doi: 10.1073/pnas.96.14.8007
Watkins Y. S. D., Sallach J. B. (2021). Investigating the exposure and impact of chemical UV filters on coral reef ecosystems: review and research gap prioritization. Integr. Environ. Assess. Manage. 17 (5), 967–981. doi: 10.1002/ieam.4411
Weis V. M., Davy S. K., Hoegh-Guldberg O., Rodriguez-Lanetty M., Pringle J. R. (2008). Cell biology in model systems as the key to understanding corals. Trends Ecol. Evol. 23 (7), 369–376. doi: 10.1016/j.tree.2008.03.004
Wilson R. C., Doudna J. A. (2013). Molecular mechanisms of RNA interference. Annu. Rev. Biophys. 42, 217–239. doi: 10.1146/annurev-biophys-083012-130404
Wolfowicz I., Baumgarten S., Voss P., Hambleton E.A., Voolstra C.R., Hatta M., et al. (2016). Aiptasia sp. larvae as a model to reveal mechanisms of symbiont selection in cnidarians. Sci. Rep. 6, 32366. doi: 10.1038/srep32366
Young C. N., Schopmeyer S. A., Lirman D. (2012). A review of reef restoration and coral propagation using the threatened genus Acropora in the Caribbean and western Atlantic. Bull. Mar. Sci. 88 (4), 1075–1098. doi: 10.5343/bms.2011.1143
Keywords: stony corals, culture, laboratory dish, Petri dish, microcolony
Citation: Shikina S, Lin T-C, Chu Y-L, Cheng Y-C, Chang Y-E, Wada N, Tang S-L, Iizuka Y and Chiu Y-L (2023) Culturing reef-building corals on a laboratory dish: a simple experimental platform for stony corals. Front. Mar. Sci. 10:1149495. doi: 10.3389/fmars.2023.1149495
Received: 22 January 2023; Accepted: 28 June 2023;
Published: 13 July 2023.
Edited by:
Hyun Woo Kim, Pukyong National University, Republic of KoreaReviewed by:
Neviaty Zamani, IPB University, IndonesiaDaniel Aagren Nielsen, University of Technology Sydney, Australia
Ikuko Yuyama, Yamaguchi University, Japan
Copyright © 2023 Shikina, Lin, Chu, Cheng, Chang, Wada, Tang, Iizuka and Chiu. This is an open-access article distributed under the terms of the Creative Commons Attribution License (CC BY). The use, distribution or reproduction in other forums is permitted, provided the original author(s) and the copyright owner(s) are credited and that the original publication in this journal is cited, in accordance with accepted academic practice. No use, distribution or reproduction is permitted which does not comply with these terms.
*Correspondence: Shinya Shikina, shikina@mail.ntou.edu.tw