- Instituto de Lactología Industrial, Facultad de Ingeniería Química, Universidad Nacional del Litoral, Santa Fe, Argentina
Probiotic bacteria, according to the definition adopted by the World Health Organization in 2002, are live microorganisms, which when administered in adequate amounts confer a health benefit to the host. Recent studies show that the same probiotic strain produced and/or preserved under different storage conditions, may present different responses regarding their susceptibility to the adverse conditions of the gastrointestinal tract, its capacity to adhere to the intestinal epithelium, or its immunomodulating capacity, the functionality being affected without changes in cell viability. This could imply that the control of cell viability is not always enough to guarantee the functionality (probiotic capacity) of a strain. Therefore, a new challenge arises for food technologists and microbiologists when it comes to designing and monitoring probiotic food: to be able to monitor the functionality of a probiotic microorganism throughout all the stages the strain goes through from the moment it is produced and included in the food vehicle, until the moment of consumption. Conventional methodological tools or others still to be developed must be used. The application of cell membrane functionality markers, the use of tests of resistance to intestinal barriers, the study of surface properties and the application of in vivo models come together as complementary tools to assess the actual capacity of a probiotic organism in a specific food, to exert functional effects regardless of the number of viable cells present at the moment of consumption.
Probiotic Microorganisms Used in Food
The intestinal microbiota comprises about 95% of the total cells in the human body, and it contains approximately 1011–1012 CFU/g of intestinal content. This diffuse “organ” which is not encoded in our DNA, is rapidly acquired after birth and is carried with us all throughout our lives, experiencing changes in its composition and its activities which respond not only to endogenous factors (e.g., age, stress), but also to exogenous factors (e.g., diet, medical treatments). The presence of the intestinal microbiota is of essential importance for the development of the mucosal immune system and the maintenance of its activity, and also for the numerous barrier and biochemical activities that it performs. As for the species and strains comprising the intestinal microbiota, there is a wide variability among individuals, depending on their age, diet, immunological status, stress factors, and intrinsic characteristics of the individual not yet totally known (Isolauri et al., 2004). The main genera found in the intestinal microbiota include Bacteroides, Eubacterium, Ruminococcus, Clostridium, and Bifidobacterium, and as the subdominant microbiota, Escherichia, Veillonella, Staphylococcus, Proteus, Streptococcus, and Lactobacillus have been reported (Tannock, 2003).
It is possible to classify the components of the intestinal microbiota according to the effects they exert on the host’s health: bacteria with potentially pathogenic effects, bacteria presenting a mixture of pathogenic and beneficial activities, or bacteria with strictly beneficial effects (Gibson et al., 2003). Bifidobacterium and Lactobacillus belong to this last group, and are the genera most frequently chosen for the isolation and characterization of probiotic bacteria.
Among the numerous definitions of probiotics, the one with the prevailing international scientific consensus is the one adopted in 2002 by the Joint Committee of the World Health Organization (WHO) and the Food and Agriculture Organization (FAO). It was established that probiotics are “live microorganisms which when administered in adequate amounts confer a health benefit on the host.” (WHO/FAO, 2002). The majority of the probiotic strains available were isolated and characterized from the following species: Lactobacillus casei, L. paracasei, L. rhamnosus, L. acidophilus, L. gasseri, L. johnsonii, L. plantarum, L. reuteri, L. crispatus, L. fermentum, Bifidobacterium bifidum, B. adolescentis, B. lactis, B. breve, B. infantis, B. longum, Saccharomyces boulardii, S. cerevisiae, and Enterococcus faecium. Certain species of the genera Bifidobacterium and Lactobacillus, common inhabitants of the intestinal microbiota, are traditionally regarded as microorganisms beneficial to health, due to the fact they exclusively promote healthy effects in their natural niche. Furthermore, some of these strains show an adequate tolerance to gastrointestinal barriers and to technological parameters applied in the production of food. These facts determine that the main commercial strains used for the manufacture of functional foods belong to these genera.
Microbiological Control of Probiotics in Food
The microbiological enumeration of probiotic bacteria in fermented foods represents a real challenge to the industry, due to the simultaneous presence of probiotics and the acidifying starter bacteria used for the fermentation of the food. Even though there is an increasingly marked tendency to apply advanced techniques such as FISH, flow cytometry (Ben Amor et al., 2007), and real-time quantitative PCR (Friedrich and Lenke, 2006) for the selective and/or differential count of probiotic bacteria in fermented milks, routine controls, mainly at an industrial level, are carried out by traditional plate counts techniques, which are simple and easy to implement in any quality control laboratory. Probiotic bacteria (mainly bifidobacteria and species of the L. casei and L. acidophilus groups; Klein et al., 1998) and the starter bacteria used in the elaboration of fermented milks (Streptococcus thermophilus and Lactobacillus delbrueckii subsp. bulgaricus) or cheese (S. thermophilus, L. helveticus, Lactococcus lactis, among other species), are microorganisms phylogenetically very closely related and with very similar nutritional requirements. Therefore, this factor becomes one of the biggest challenges for the differentiation of their colonies on the surface of agar plates, since their metabolic responses are very similar. A wide variety of culture media has been proposed in the last 20 years for the microbiological control of probiotics (Vinderola et al., 2009).
However, the problem of the standardization of the microbiological count of probiotics in food is very far from being solved, since it is not possible to call upon a single and official protocol valid for all the strains presently used in probiotic foods. This is mainly due to the strain-specific response to the different culture media. Some aspects to take into account for the development or adoption of a culture medium for evaluation of probiotics in food, are the following:
• Probiotic microorganisms, mainly those derived from the genera Lactobacillus and Bifidobacterium, are closely related in terms of metabolism to lactic acid starter bacteria. From a nutritional point of view, it is difficult to favor the growth of the former while trying to inhibit the development of the latter.
• In general, probiotics are added to food as adjunct cultures in concentrations of 107–108 CFU/g or ml, without participating in the fermentative process and without practically modifying their concentration during the elaboration of the product. As for starter bacteria, they can reach, after the fermentative process, a concentration of between 108 and 109 CFU/g or ml. If the development of starter bacteria on the surface of the culture medium is not adequately inhibited, they may not allow the development of or hinder the differential count of probiotic bacteria.
• In contrast to analyses for groups of microorganisms such as coliforms, psychrotrophs, Salmonella, or Escherichia coli, for which there are commercially available culture media and standardized protocols for their count, probiotics do not constitute a homogeneous group. Therefore, it has not been possible so far to design a single culture medium for all the existing probiotic strains, suitable at the same time, for all the food matrices used as vehicles for probiotics.
• Considering that most of the species of probiotic bacteria belong to the Lactobacillus and Bifidobacterium genera and many of them have an intestinal origin, it is advisable to use biological or chemical inhibitors (mineral salts, bile salts, organic acids, for example) to change a general medium into a selective medium for probiotic bacteria. However, it must be verified first, by pure culture studies and in the presence of the food matrix to be used, that the recovery of viable bacteria is as close as possible to 100%.
• In products containing more than one probiotic species, the level of difficulty for carrying out a selective/differential count increases, especially if they belong to the same genera. It is more likely to be able to adequately distinguish diverse species of Lactobacillus than of Bifidobacterium, always in a strain-dependent way. So far, it is impossible to differentiate probiotic strains belonging to the same species through the plate count technique, which constitutes an important limitation when it comes to designing a multi-probiotic food using strains with different beneficial effects. At the same time, the effect of the simultaneous presence of strains must be always assessed since beneficial effects are not always additive (Timmerman et al., 2004).
• When a selective or differential culture medium is designed or adopted for the count of probiotics in a particular food, it is necessary to prove first the individual capacity of each of the (probiotic and starter) strains to grow on that medium, so as to ensure the complete inhibition of the starter bacteria and the total recovery of the probiotic microorganisms. In case this is not possible, an adequate differential capacity must be guaranteed for an accurate count of each strain. These simple steps are very often not determined, hindering then the count of probiotics once the food is microbiologically formulated and manufactured.
Probiotic Bacteria: Factors Influencing Their Functionality
The definition of probiotics recognizes the capacity of live microorganisms to exert a beneficial effect on the consumer’s health. From the moment they are incorporated into a food, the microbiological control of their cell viability is routinely used for the monitoring of their functionality. For this reason, the scientific criteria (Champagne et al., 1997; Ross et al., 2005) or legislations in force (Amagase, 2008; Degnan, 2008; Saxelin, 2008) in some countries, require a particular concentration of viable bacteria to guarantee the functional quality of the food and to call it, then, a probiotic food. However, in the last years and, on the basis of different reports, there has been evidence of certain changes in functionality, i.e., in the qualitative and quantitative magnitude of the beneficial effect of a strain, without levels of viable cells being modified. Briefly, changes in the effect induced without changes in cell counts: same amount, different effects. In this context, the microbiological count would only partially reflect the functional capacity of the strain. Therefore, it appears necessary to know, manage and control the microbiological variables that may alter the functionality of a strain in a particular food matrix, without having an impact on the number of viable cells. Throughout the following sections, some aspects of the currently available information on the factors which may provoke changes in the in vivo response of a probiotic, without affecting cell viability, will be presented.
Functionality of Probiotics and Culture Production
The first step in the production of a probiotic culture for its large scale implementation entails its development in a culture medium suitable for biomass production. In this sense, the variables involved during the growth of the strain (pH of the medium, type, and concentration of carbohydrates, growth phase at harvesting, cell physiological state, etc.) may significantly affect both its resistance to biological barriers (gastric acidity and bile salts) and its capacity to interact with immune cells, thus conditioning its functionality.
Bifidobacterium animalis subsp. lactis INL1, a strain isolated from human breast milk (Zacarías et al., 2011), was grown in MRS broth in a biofermentor at 37°C. The culture reached the stationary phase more rapidly when grown at pH 6.5, in comparison to a culture grown at pH 5.0. However, when the strain was subjected to a gastric resistance test (pH 2.0, 0.3% pepsin, 37°C, 90 min), a 4 log orders of cell death was observed for the culture grown at pH 6.5, while the viability loss was negligible during the gastric resistance test performed on the culture grown at pH 5.0. Moreover, scanning electron microscopy studies performed on the cultures, showed the production of extracellular compounds (exopolysaccharide type) when the strain was grown at pH 6.5, but not at pH 5.0. These compounds might exert an in vivo response different from that exerted by the culture grown at pH 5 which displayed no extracellular compounds, a fact that must be still studied.
In relation to the culture medium pH, Saarela et al. (2009) studied the resistance to gastric acidity and to bile salts of a L. rhamnosus strain grown at pH 5.0 and 5.8 and further freeze-dried in polydextrose and sucrose. The authors observed that the culture obtained at pH 5.0 presented a higher resistance to gastric acidity than the one grown at pH 5.8, though these differences were only observed in the cultures freeze-dried in polydextrose but not in the cultures freeze-dried in sucrose as cryoprotectant. In this study, it has been unveiled not only the importance of the pH of the culture medium used for the production of biomass in gastric resistance but also the influence of the cryoprotectant on the viability and gastric resistance of the cultures produced. For both pH values tested and the two cryoprotectants used, the L. rhamnosus culture showed the same retention of viability when preserved at 37°C for 2 weeks, while gastric acid resistance and resistance to bile salts were significantly affected; this demonstrates the utility of these tests as monitors for cell functionality.
The growth phase in which the cell harvesting is carried out during biomass propagation, is another variable determining cell functionality. In our laboratory we observed that the gastric resistance of B. animalis subsp. lactis INL1 strain after 22 h of culture (late stationary growth phase) in an anaerobic biofermentor at 37°C, presented a gastric resistance significantly higher than the same culture grown for 12 h (early stationary growth phase). Sashihara et al. (2007) showed that the capacity of a L. gasseri strain to induce the synthesis of the pro-inflammatory cytokine IL-12 when co-cultured with mice splenocytes, depended on the time at which the culture was harvested (growth phase); the capacity to induce maximum IL-12 production was when the strain reached the late stationary growth phase.
The physiological status of a strain is another variable involved in its functional properties. So as to exemplify this fact, Lepercq et al. (2004) assessed the capacity of a B. animalis strain to deconjugate, in vivo, bile salts during its transit through the pig intestine. In this case, the activity of bile salts hydrolase turned out to be higher when pigs received viable cells instead of heat-inactivated cells. Additionally, bile salts hydrolase activity was higher in cells harvested in the exponential growth phase compared to cells harvested in stationary phase. More recently, it was reported (Kimoto-Nira et al., 2008) that the immunomodulatory activity (capacity to induce IL-12 in cell lines), of a L. lactis strain, changed according to the physiological cell status (viable or dead cells) and, at the same time, according to the culture medium used for its growth (M17 or MRS with added glucose). Likewise, different cell wall compositions, at the sugar’s level and in the content of fatty acids of cells cultured in both media, were verified, thus determining variations in the immunomodulatory activity, and hence in the functionality of the strain.
Functionality of Probiotics and Technological Processes
High pressure homogenization (HPH) constitutes a novel technology that implies the application of high pressures to liquids in order to inactivate food pathogens or to modify the rheological properties of the product, without decreasing the nutritional value of the product compared to the application of heat treatments (Paquin, 1999; Patrignani et al., 2009). The application of mild HPH treatments directly to the cells (up to 500 bars, depending on the strain) does not cause cell death but might induce permanent changes of functional attributes such as cell surface hydrophobicity and gastric resistance. It has been hypothesized that strains presenting a high value of cell surface hydrophobicity may have a better capacity to interact with immune cells such as macrophages (Ofek and Doyle, 1994) and may possess a better adhesion to the intestinal epithelium (Kotzamanidis et al., 2010). When a HPH treatment of 500 bars was applied to a strain of L. paracasei suspended in buffer, its gastric resistance (assessed at pH 2.0, 37°C, 0.3% porcine pepsin for 90 min) significantly increased. The strain also experienced a significant increase in its cell hydrophobicity. However, when the HPH treatment was applied to cells suspended in 10% skim milk, the effectiveness of the treatment on increasing gastric resistance was less significant, probably due to dissipation of the HPH effect among milk proteins and cell surface proteins. It is known that the exposure to sublethal levels of a stress factor (HPH treatment, in this case) may induce resistance to higher levels of the same stress factor or to others (gastric acidity, for example), a phenomenon called “cross-resistance” (O’Driscoll et al., 1996; Burns et al., 2008).
Another important selection criterion for probiotic bacteria has to do with the capacity of adhesion to mucosal surfaces, since it allows triggering of some of their functional attributes such as immunomodulation. Therefore, adhesion capacity, which is determined by the superficial structures of cells, may suffer modifications during the production process of probiotic cells, affecting as a result, cell functionality without affecting cell viability. In the review by Tuomola et al. (2001) there is a mention of variations in the adhesion capacity of commercial probiotic strains during the manufacture of probiotic food. As for L. rhamnosus GG, Elo et al. (1991) observed mild variations in the levels of adhesion of the strain isolated at different points of its production process, after analyzing different batches of products. It was also verified that an important loss in the adhesion capacity to cell lines occurred when the strain was subcultured on a weekly basis for a long period of time in MRS broth. For instance, a strain of L. acidophilus isolated in two different years from the same commercial product displayed a significantly different adhesion capacity between the two isolation times, in both models studied (Caco-2 cells and intestinal mucus).Thus, it can be inferred that the factors related to the production process of the food matrix used as vehicle for probiotics may modify the adhesion of a probiotic strain together with its functional attributes with changes not being noticed by quality control cell counts.
Functionality of Probiotics and Shelf Life
Foods containing probiotic bacteria act as their vehicles or carriers until cells reach the intestinal tract. The food itself may have an important influence on the functional attributes of the probiotic strain, since it is incorporated in food during its passage through the different biological barriers of the gastrointestinal tract. In this way, the physicochemical attributes of food (fat and protein content, type of proteins, sugars, pH, etc.), certain food ingredients (flavoring agents, thickeners, sweeteners, stabilizers, etc.) and added functional ingredients (bioactive components) to which probiotic bacteria are exposed, may affect their performance in this complex matrix, modifying their functionality and efficacy. Consequently, a critical point in the formulation of probiotic foods is the optimization of all these variables so as to improve the probiotic capacity of a designated strain or, at least, so as not to negatively modify it with respect to when it was characterized as a pure culture outside a food matrix. In particular, dairy products (fermented milks, cheese, ice cream, etc.), due to their physicochemical and functional attributes, are considered the ideal vehicles for probiotic bacteria. The way in which the food matrix influences the functionality of probiotics, has been reviewed recently by Ranadheera et al. (2010).
In a recent study conducted by our group, the strain of breast milk origin, B. animalis subsp. lactis INL1, was cultured in a biofermentor at pH 5.0, harvested and freeze-dried in 10% lactose as protectant. The freeze-dried culture was added to different commercial liquid food matrices. Inoculated samples were cold-stored to assess the influence of the matrix on the gastric resistance of the strain along storage. Within 4 weeks at 5°C, there were no changes in the levels of viable cells in any of the products studied. However, when the products containing the strain were subjected to a simulated gastric digestion (pH 2.0, 0.3% pepsin, 37°C, 90 min), there were no changes in the resistance to acidity of the banana–apple juice and the vanilla flavored milk. However, in the case of the multi-fruit juice and the apple–peach puree, a cell death of about 1 logarithmic cycle was observed after the gastric digestion, while in the case of the apple–banana mix puree and the orange juice, the decay of cell viabilities were 2 and 3 log orders, respectively. These results show the importance of an adequate selection of a proper food matrix for probiotic strains, considering functional variabilities, and beyond merely monitoring the levels of viable cells. In relation to these results, our group recently showed (Vinderola et al., 2011) that different L. casei strains in fermented milks of different flavors, which maintained adequate levels of viable cells during refrigerated storage, experienced changes in gastric resistance depending on the temperature of storage (5 or 12°C, an usual temperature on supermarket shelves), during the storage period, and on the flavor of the fermented milk. These factors resulted in an increase or decrease of gastric resistance, as a result of the possible simultaneous presence of sublethal stress factors that might induce gastric resistance (such as lactic acidity) as well as the presence of factors having inhibitory potential, such as chemical agents used in the formulation of commercial dairy products. These results indicate that the same probiotic strain can present different behaviors in terms of gastric resistance according to the flavor of the fermented milk to which is added, stressing the need for adequate viability and functionality controls when it comes to developing a new probiotic food, even when it may be similar to other products already existing in the market, as in the case of fermented milks of different flavors.
Recently, Wang et al. (2009) assessed the resistance of a L. casei strain to the gastrointestinal tract (at pH 2.0 and 2.5). L. casei was cultured in bovine milk and soy milk and stored at 4°C for 28 days. When the resistance of the strain to biological barriers (simulated gastric and intestinal juice) was assessed in both products, a protective effect of the food was observed (in relation to a control culture in MRS broth), which was much more significant in the case of bovine milk compared to soy milk. As expected, the gastric resistance was higher at pH 2.5 than at pH 2.0. These results stress the importance of the food matrix to guarantee not only the survival capacity but also the functional properties of probiotic bacteria until the moment they are consumed.
Functionality of Probiotics and Biological Barriers
As mentioned in the previous sections of this chapter, numerous studies have been carried out with the aim of identifying and studying the microbiological and technological factors that can modify the viability and functionality of probiotic bacteria in food. Nevertheless, until now, less attention has been paid to the analysis of the physiological factors of the host and the eating habits that can affect the functionality of probiotics, apart from the studies of resistance to physiological barriers, such as gastric acidity and bile salts. One aspect that has been little explored is the effect of the simultaneous consumption of different probiotics on the host since, a priori, an addition of effects should not be expected. While in some cases the addition of multiple strains to a food results in additive or potentiating effects, among other things, the relation of cost/benefit does not always justify such addition and, may even lead to the occurrence of undesirable effects (Timmerman et al., 2004). Another important aspect to consider is the period of administration of the probiotic. The continuous consumption of an immunomodulatory strain could induce mucosal immune profiles that can change with time of exposure. This fact might then modify the functional properties of the probiotic during continuous intake, due to the regulatory capacity of the immune system associated to the intestinal mucosa (Coombes and Maloy, 2007). For example, de Moreno de LeBlanc et al. (2008) showed in mice that the continuous long-term administration of a fermented milk containing a L. casei probiotic strain, caused a significant increase in the number of cells producing IgA in the intestine by day 7 of feeding; after the IgA peak a decrease in such response was observed when the consumption continued. These results may suggest that a cyclical consumption (alternation of administration/withdrawal cycles) of the probiotic, would induce an average response in time, higher than that obtained with a continuous consumption. In another study, the short-term administration to mice of the cell-free fraction of the fermented milk Kefir, induced a proliferation of cells of the innate immunity which produce the pro-inflammatory cytokine TNF alpha. On the other hand, a longer period of administration resulted in an increase in the production of the regulatory cytokine IL-10 (Vinderola et al., 2006). These changes in the profile of induced cytokines in the gut during the long-term administration of bioactive food, would indicate possible changes in the functionality of the food depending on the length of the administration period, shifting from a pro-inflammatory profile without tissue damage (effective, perhaps, against intestinal pathogens) to a regulatory profile (potentially effective against intestinal inflammatory diseases). These hypotheses need to be studied in depth and validated in human studies. Finally, the in vivo effect of biological barriers, such as bile salts, on the functionality of probiotic strains and on their capacity of interacting with the intestine, should also be elucidated. In recent studies by our group, the adaptation of a non-intestinal strain of L. delbrueckii subsp. lactis to bile salts allowed the development of a bile-resistant derivative, resistant to physiological concentrations of bile salts (0.5% w/v). However, the adaptation to bile diminished the autoaggregation capacity of the strain (Figure 1) as well as its hydrophobicity and adhesion capacity in vitro and in vivo (Burns et al., 2008; 2010). These changes in the cell surface attributes induced by bile salts, a powerful natural detergent (Begley et al., 2005), impacted on cell functionality: the bile-resistant derivative was less effective in enhancing the gut mucosal defenses mediated by IgA (Burns et al., 2011).
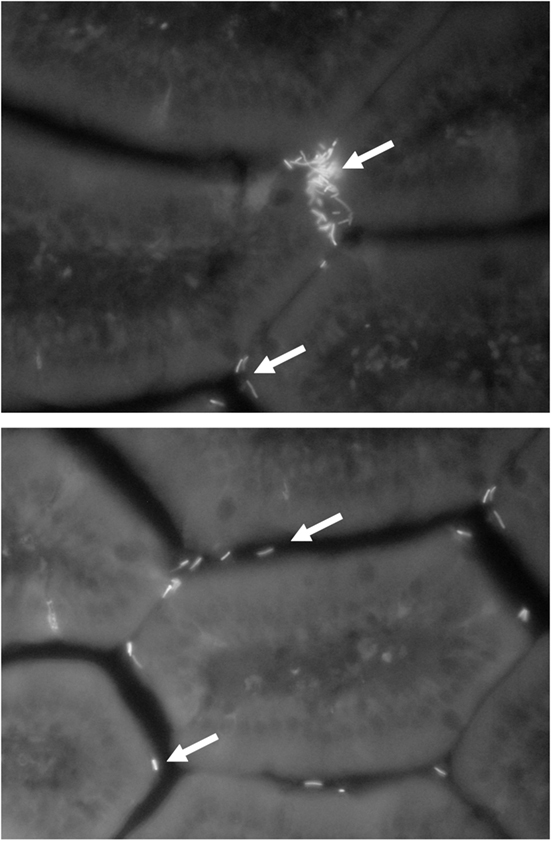
Figure 1. FITC-labeled (fluorescent) L. delbrueckii subsp. lactis 200 (above) and its non-autoaggregative bile-resistant derivative L. delbrueckii subsp. lactis 200+ (below) in the small intestine lamina propria after 30 min of their oral administration to mice. Magnification: 40×. Arrows indicate lactobacilli cells.
Conclusion
The current commercial expansion in the use of probiotic bacteria in functional foods must be accompanied by new knowledge and exploration of new concepts, together with the study in depth of how these bacteria may be influenced by different factors inherent to technological processes, the physicochemical environment of food, and the gastrointestinal transit. In this sense, the traditional concept of control of cell viability as a tool for determining the probiotic value of the food must be complemented by an assessment of the functionality of the strain included in a particular food. Only in that way will it be possible to ensure that the food product is really functional, beyond the functional value that has been reported for the probiotic strain alone. In the current chapter, some of the different factors that can modify the functionality of a strain during its industrial propagation, its inclusion into a technological process for food manufacture and its exposure to the product environment, as well as to biological barriers, were discussed. Thus, determining the in vivo functionality of food must be requested as a procedure that allows commercializing “proven” probiotic products, not potential ones.
Conflict of Interest Statement
The authors declare that the research was conducted in the absence of any commercial or financial relationships that could be construed as a potential conflict of interest.
References
Amagase, H. (2008). Current marketplace for probiotics: a Japanese perspective. Clin. Infect. Dis. 46, S73–S75.
Begley, M., Cormac, C. G. M., and Hill, C. (2005). The interaction between bacteria and bile. FEMS Microbiol. Rev. 29, 625–651.
Ben Amor, K., Vaughan, E. E., and de Vos, W. M. (2007). Advanced molecular tools for the identification of lactic acid bacteria. J. Nutr. 137(3 Suppl. 2), 741S–747S.
Burns, P., Sánchez, B., Vinderola, G., Ruas-Madiedo, P., Ruiz, L., Margolles, A., Reinheimer, J., and de los Reyes-Gavilán, C. G. (2010). Inside the adaptation process of Lactobacillus delbrueckii subsp. lactis to bile. Int. J. Food Microbiol. 142, 132–141.
Burns, P., Vinderola, G., and Reinheimer, J. (2011). Impact of bile salt adaptation of L. delbrueckii subsp. lactis 200 on its interaction capacity with the gut. Res. Microbiol. (sent).
Burns, P., Vinderola, G., Binetti, A., Quiberoni, A., de los Reyes-Gavilán, C. G., and Reinheimer, J. (2008). Bile-resistant derivatives obtained from non-intestinal dairy lactobacilli. Int. Dairy J. 18, 377–385.
Champagne, C. P., Roy, D., and Lafond, A. (1997). Selective enumeration of Lactobacillus casei in yoghurt-type fermented milks based on a 15°C incubation temperature. Biotechnol. Technol. 11, 567–569.
Coombes, J. L., and Maloy, K. J. (2007). Control of intestinal homeostasis by regulatory T cells and dendritic cells. Semin. Immunol. 19, 116–126.
de Moreno de LeBlanc, A., Chaves, S., Carmuega, E., Weill, R., Antóine, J., and Perdigón, G. (2008). Effect of long-term continuous consumption of fermented milk containing probiotic bacteria on mucosal immunity and the activity of peritoneal macrophages. Immunobiology 213, 97–108.
Degnan, F. H. (2008). The US Food and Drug Administration and probiotics: regulatory categorization. Clin. Infect. Dis. 46, S133–S136.
Elo, S., Saxelin, M., and Salminen, S. (1991). Attachment of Lactobacillus casei strain GG to human colon carcinoma cell line Caco-2: comparison with other dairy strains. Lett. Appl. Microbiol. 13, 154–156.
Friedrich, U., and Lenke, J. (2006). Improved enumeration of lactic acid bacteria in mesophilic dairy starter cultures by using multiplex quantitative real-time PCR and flow cytometry-fluorescence in situ hybridization. Appl. Environ. Microbiol. 72, 4163–4171.
Gibson, G. R., Rastall, R. A., and Fuller, R. (2003). “The health benefits of probiotics and prebiotics,” in Gut, Nutrition, Immunity and Health, eds R. Fuller and G. Perdigón (Oxford: Blackwell Publishing), 52–76.
Isolauri, E., Salminen, S., and Ouwehand, A. C. (2004). Microbial-gut interactions in health and disease. Probiotics. Best Pract. Res. Clin. Gastroenterol. 18, 299–313.
Kimoto-Nira, H., Suzuki, C., Kobayashi, M., and Mizumachi, K. (2008). Different growth media alter the induction of interleukin 12 by a Lactococcus lactis strain. J. Food Prot. 71, 2124–2128.
Klein, G., Pack, A., Bonaparte, C., and Reuter, G. (1998). Taxonomy and physiology of probiotic lactic acid bacteria. Int. J. Food Microbiol. 41, 103–125.
Kotzamanidis, C., Kourelis, A., Litopoulou-Tzanetaki, E., Tzanetakis, N., and Yiangou, M. (2010). Evaluation of adhesion capacity, cell surface traits and immunomodulatory activity of presumptive probiotic Lactobacillus strains. Int. J. Food Microbiol. 140, 154–163.
Lepercq, P., Relano, P., Cayuela, C., and Juste, C. (2004). Bifidobacterium animalis strain DN-173 010 hydrolyses bile salts in the gastrointestinal tract of pigs. Scand. J. Gastroenterol. 39, 1266–1271.
O’Driscoll, B., Gahan, C. G. M., and Hill, C. (1996). Adaptive acid tolerance response in Listeria monocytogenes: isolation of an acid-tolerant mutant which demonstrates increased virulence. Appl. Environ. Microbiol. 62, 1693–1698.
Ofek, I., and Doyle, J. D. (1994). Bacterial Adhesion to Cells and Tissues. New York: Chapman and Hall.
Paquin, P. (1999). Technological properties of high pressure homogenizers: the effect of fat globules, milk proteins, and polysaccharides. Int. Dairy J. 9, 329–335.
Patrignani, F., Burns, P., Serrazanetti, D., Vinderola, C. G., Reinheimer, J. A., Lanciotti, R., and Guerzoni, M. E. (2009). Suitability of high pressure-homogenized milk for the production of probiotic fermented milk containing Lactobacillus paracasei and Lactobacillus acidophilus. J. Dairy Res. 76, 74–82.
Ranadheera, R. D. C. S., Baines, S. K., and Adams, M. C. (2010). Importance of food in probiotic efficacy. Food Res. Int. 43, 1–7.
Ross, R. P., Desmond, C., Fitzgerald, G. F., and Stanton, C. (2005). Overcoming the technological hurdles in the development of probiotic foods. J. Appl. Microbiol. 98, 1410–1417.
Saarela, M. H., Alakomi, H. L., Puhakka, A., and Mätto, J. (2009). Effect of the fermentation pH on the storage stability of Lactobacillus rhamnosus preparations and suitability of in vitro analyses of cell physiological functions to predict it. J. Appl. Microbiol. 106, 1204–1212.
Sashihara, T., Sueki, N., Furuich, K., and Ikegami, S. (2007). Effect of growth conditions of Lactobacillus gasseri OLL2809 on the immunostimulatory activity for production of interleukin-12 (p70) by murine splenocytes. Int. J. Food Microbiol. 120, 274–281.
Saxelin, M. (2008). Probiotic formulations and applications, the current probiotics market, and changes in the marketplace: a European perspective. Clin. Infect. Dis. 46, S76–S79.
Tannock, G. W. (2003). “The intestinal microflora,” in Gut, Nutrition, Immunity and Health, eds R. Fuller and G. Perdigón (Oxford: Blackwell Publishing), 1–23.
Timmerman, H. M., Koning, C. J. M., Mulder, L., Rombouts, F. M., and Beynen, A. C. (2004). Monostrain, multistrain and multispecies probiotics – a comparison of functionality and efficacy. Int. J. Food Microbiol. 96, 219–233.
Tuomola, E., Ross, C., Playne, M., Isolauri, E., and Salminen, S. (2001). Quality assurance criteria for probiotic bacteria1. Am. J. Clin. Nutr. 73, 393S–398S.
Vinderola, G., Céspedes, M., Mateolli, D., Cárdenas, P., Lescano, M., Aimaretti, N., and Reinheimer, J. (2011). Changes in gastric resistance of Lactobacillus casei in flavoured commercial fermented milks during refrigerated storage. Int. J. Dairy Technol. 64, 269–275.
Vinderola, G., de los Reyes-Gavilán, C., and Reinheimer, J. (2009). “Probiotics and prebiotics in fermented dairy products,” in Contemporary Food Engineering, eds C. P. Ribeiro and M. L. Passos (New York: CRC Press and Taylor & Francis Group), 601–634.
Vinderola, G., Perdigón, G., Duarte, J., Thangavel, D., Farnworth, E., and Matar, C. (2006). Effects of kefir fractions on innate immunity. Immunobiology 211, 149–156.
Wang, J., Guo, Z., Zhang, Q., Yan, L., Chen, W., Liu, X. M., and Zhang, H. P. (2009). Fermentation characteristics and transit tolerance of probiotic Lactobacillus casei Zhang in soymilk and bovine milk during storage. J. Dairy Sci. 92, 2468–2476.
WHO/FAO. (2002). Guidelines for the Evaluation of Probiotics in Food. London. Available at: ftp://ftp. fao. org/es/esn/food/wgreport2. pdf
Keywords: probiotics, viability, functionality
Citation: Vinderola G Binetti A Burns P and Reinheimer J (2011) Cell viability and functionality of probiotic bacteria in dairy products. Front. Microbio. 2:70. doi: 10.3389/fmicb.2011.00070
Received: 29 December 2010;
Paper pending published: 19 January 2011;
Accepted: 26 March 2011;
Published online: 03 May 2011.
Edited by:
Paula Teixeira, Catholic University, PortugalReviewed by:
Kiiyukia Matthews Ciira, Jomo Kenyatta University of Agriculture and Technology, KenyaPaul Anthony Gibbs, Catholic University, Portugal
Copyright: © 2011 Vinderola, Binetti, Burns and Reinheimer. This is an open-access article subject to a non-exclusive license between the authors and Frontiers Media SA, which permits use, distribution and reproduction in other forums, provided the original authors and source are credited and other Frontiers conditions are complied with.
*Correspondence: Gabriel Vinderola, Instituto de Lactología Industrial, Facultad de Ingeniería Química, Universidad Nacional del Litoral, 1° de Mayo 3250, Santa Fe 3000, Argentina. e-mail:Z3ZpbmRlQGZpcS51bmwuZWR1LmFy