- Geomicrobiology Group, Institute for Earth and Environmental Sciences, University of Potsdam, Potsdam, Germany
High-pressure is a key feature of deep subsurface environments. High partial pressure of dissolved gasses plays an important role in microbial metabolism, because thermodynamic feasibility of many reactions depends on the concentration of reactants. For gases, this is controlled by their partial pressure, which can exceed 1 MPa at in situ conditions. Therefore, high hydrostatic pressure alone is not sufficient to recreate true deep subsurface in situ conditions, but the partial pressure of dissolved gasses has to be controlled as well. We developed an incubation system that allows for incubations at hydrostatic pressure up to 60 MPa, temperatures up to 120°C, and at high gas partial pressure. The composition and partial pressure of gasses can be manipulated during the experiment. To keep costs low, the system is mainly made from off-the-shelf components with only very few custom-made parts. A flexible and inert PVDF (polyvinylidene fluoride) incubator sleeve, which is almost impermeable for gases, holds the sample and separates it from the pressure fluid. The flexibility of the incubator sleeve allows for sub-sampling of the medium without loss of pressure. Experiments can be run in both static and flow-through mode. The incubation system described here is usable for versatile purposes, not only the incubation of microorganisms and determination of growth rates, but also for chemical degradation or extraction experiments under high gas saturation, e.g., fluid–gas–rock-interactions in relation to carbon dioxide sequestration. As an application of the system we extracted organic compounds from sub-bituminous coal using H2O as well as a H2O–CO2 mixture at elevated temperature (90°C) and pressure (5 MPa). Subsamples were taken at different time points during the incubation and analyzed by ion chromatography. Furthermore we demonstrated the applicability of the system for studies of microbial activity, using samples from the Isis mud volcano. We could detect an increase in sulfate reduction rate upon the addition of methane to the sample.
Introduction
The incubation of deep subsurface microorganisms under high-pressure conditions is necessary because under non-in situ conditions (especially low pressure) metabolic processes and survival of microorganisms adapted to high hydrostatic pressure are negatively impacted (Yayanos and Dietz, 1983; Fang et al., 2010). Since the first isolation of a pressure-adapted bacterium by Yayanos et al. (1979) numerous studies on the effect of elevated pressure on genetic, metabolic, and physiological aspects of microorganisms were carried out. Multiple biological effects of pressure on organisms were observed: shifts in metabolic activity (Abe et al., 1999; Bothun et al., 2004), transcription profiles (e.g., Boonyaratanakornkit et al., 2007), and the dissociation of ribosomes (e.g., Schulz et al., 1976), changes in growth rates (Yayanos, 1986; Boonyaratanakornkit et al., 2006; Takai et al., 2009), gene regulation (Bartlett et al., 1989), stabilization of proteins (Hei and Clark, 1994; Sun and Clark, 2001), and the composition of membrane lipids (Delong and Yayanos, 1985; Kaneshiro and Clark, 1995). For reviews of pressure effects on biological processes see Jaenicke (1983) and Bartlett (2002). Biochemical processes are also influenced by physical implications of high hydrostatic pressure, because the thermal expansion coefficient (Frank, 1970) as well as viscosity and fluidity of water (Horne and Courant, 1965) affect chemical reactions and cellular processes.
The idea of constructing and using a high-pressure vessel for studying deep-sea life is quite old. Zobell and Oppenheimer (1950) described a simple pressure vessel for the application of high hydrostatic pressure on microorganisms. Pressure was applied to a culture tube with a neoprene stopper working as piston for transmitting pressure to the sample. This type of pressure application is still being used today (Orcutt et al., 2008). Yayanos (1969) and later Taylor and Jannasch (1976) presented techniques for sub-sampling of media and bacteria and the determination of reaction rates without decompression, thereby eliminating the repetitive and time-consuming decompression. The use of glass syringes or a flexible Teflon container instead of a sealed culture tube (Schmid et al., 1978) had the benefit of an inert reaction chamber. However, the leakage of gases from the media into the pressure liquid or vice versa required a gas-tight incubation chamber. Bernhardt et al. (1987) used flexible nickel tubes for incubations of methanogenic microorganisms with hydrogen. Also flexible cells made of gold (Seyfried, 1979) or titanium (Seyfried and Janecky, 1985) were used as high-pressure reaction chamber. However, such devices were designed for studies of hydrothermal alteration of basalt and therefore made for much higher temperatures than what is necessary for biological incubations. All described techniques are still in use. Recently Parkes et al. (2009) presented a high-pressure system that can accept drill cores, taken with a high-pressure corer without decompression. The system also allows for sub-sampling without decompression.
Temperature also has an effect on growth rates and other physiological characteristics of all microorganisms. Thermophilic and thermotolerant microorganisms can be found at hydrothermal vents, terrestrial hot springs, and intraterrestrial habitats (Pedersen, 2000) like salt mines (Vreeland et al., 1998), groundwater deep within Earth (Lin et al., 2006; Chivian et al., 2008), or oil reservoirs (e.g., L’Haridon et al., 1995).
Several techniques for the incubation of these thermophilic microorganisms are used: thermistors (e.g., Bernhardt et al., 1987), drying ovens (Miller et al., 1988; Takai et al., 2008), and water baths (e.g., Jannasch et al., 1996). Incubators and water baths became the most commonly used techniques for keeping pressure vessels at the desired temperature.
The application of elevated gas concentration in high-pressure incubations started about 25 years ago. Gases were applied to high-pressure vessels to maintain anaerobic conditions in incubations of hyperthermophilic archaea (e.g., Raven et al., 1992), to obtain higher cell densities during incubation (e.g., Mukhopadhyay et al., 1999) or as substrate for methanogenic microorganisms (Bernhardt et al., 1987; Takai et al., 2008). Nauhaus et al. (2002) incubated sediment samples from a methane hydrate field at different partial pressures of methane and showed a strong correlation between microbial activity and methane partial pressure. None of these incubation systems allowed manipulation of the gas partial pressure during the incubation or sub-sampling without decompression.
Here, we present an inexpensive high-pressure high-temperature incubation system that allows the incubation of a sample at high hydrostatic pressure as well as the manipulation of the composition and concentration of the dissolved gasses in the medium during incubation. It is designed for both static and flow-through experiments and allows for sub-sampling the liquid phase including the dissolved gases without decompression. The key objective was to build a moderately priced incubation system that can easily be constructed and operated. To keep costs low we used standardized off-the-shelf items and only a few custom-made parts.
With this system not only microbiological experiments under high hydrostatic and gas partial pressure can be performed. Geochemical experiments, for example the extraction of organic and inorganic compounds from rock samples under specific pressure and temperature conditions or mineral alteration studies are also possible.
Initial tests of the system included applications for geochemical and microbiological experiments. The effect of high concentrations of CO2 dissolved in water on the release of low molecular weight organic acids from sub-bituminous coal from the Waikato Basin (NZ) was studied, as well as the effect of high methane partial pressure on microbial activity in samples from the Isis mud volcano (IMV), off the Mediterranean coast of Egypt.
Materials and Methods
The high-pressure incubation system (Figure 1) is composed of a reservoir vessel and a reaction vessel for the application of hydrostatic pressure on an incubator sleeve, which hangs inside the pressure vessel and holds the sample. A sub-sampling system allows the retrieval of liquid subsamples during the experiment without decompression. Temperature is maintained by a heating/cooling bath (Julabo Labortechnik GmbH, Seelbach, Germany) that pumps the liquid through heating jackets around the reservoir and the reaction vessel. Medium is circulated in a closed loop and HPLC pumps maintain hydrostatic pressure. A photograph of the entire unit is shown in Figure 2.
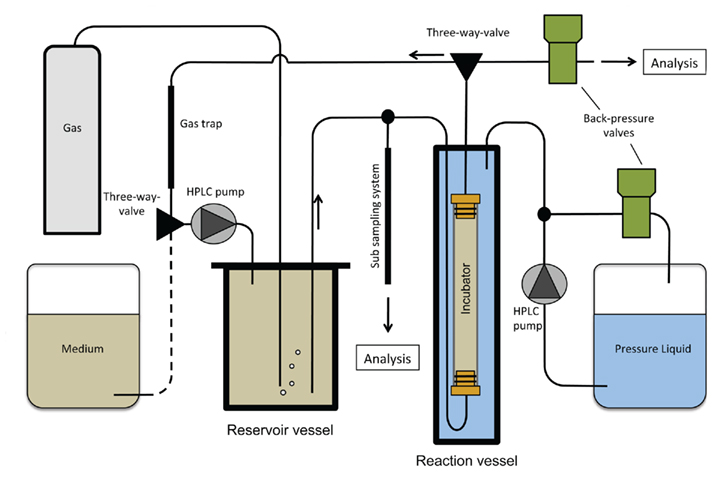
Figure 1. Schematic drawing of the high-pressure incubation system. The medium is pressurized and enriched with gas in the reservoir vessel prior to flowing through the incubator sleeve that hangs inside the reaction vessel. Pressure in the reaction vessel is set and kept constant by a HPLC pump and a backpressure valve.
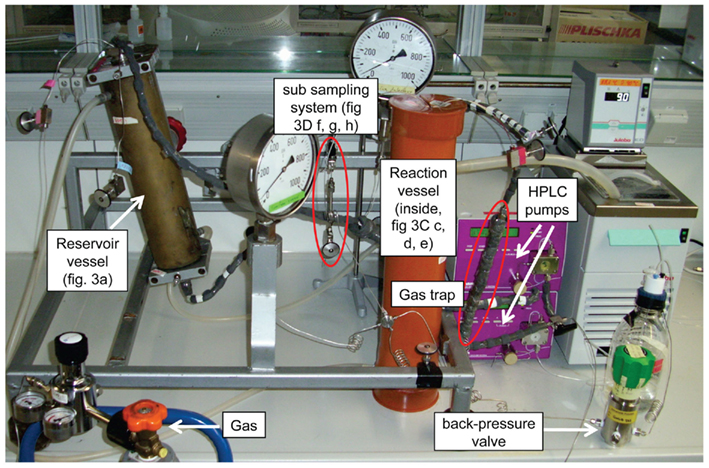
Figure 2. Photograph of the high-pressure incubation system as seen in Figure 1. Reservoir vessel, reaction vessel, incubator, and sub-sampling device are shown in detail in Figure 3.
For microbiological experiments all parts of the high-pressure incubation system can be sterilized by autoclaving.
Reservoir Vessel
The task of the temperature-controlled reservoir vessel is to saturate the medium with gas to the desired level and to hold a reservoir of medium that is pumped through the system.
The reservoir vessel is stainless steel cylinder (Dunze GmbH, Hamburg, Germany), with a volume of 255 cm3 (inner dimensions: 3.4 cm diameter, 28.15 cm high; Figure 3A). Top and bottom are closed with plugs with bores for 1/16′′ HPLC lines to allow for transfer of gas and medium in and out of the vessel.
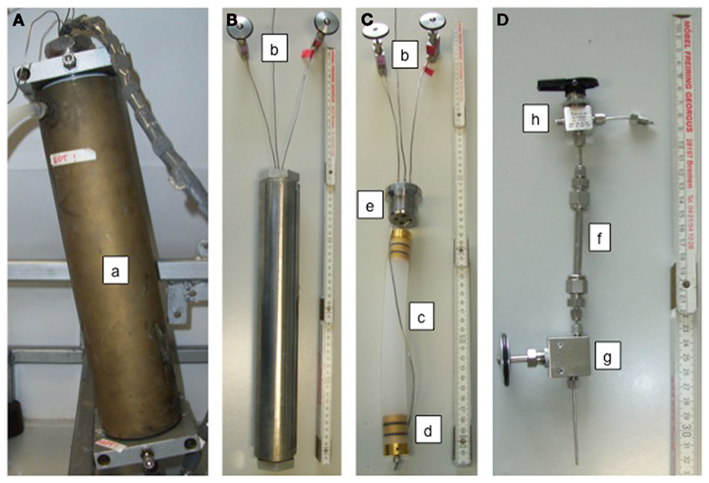
Figure 3. (A) Reservoir vessel with a total volume of 255 cm3; (a) pressure cylinder (invisible) with heating/cooling jacket; (B) high-pressure reaction vessel including connections with (b) valves for in- and out-flow of medium via the incubator; the third line (without a valve) is for the application of pressure to the reaction vessel; (C) PVDF-incubator with (b) valves, (c) PVDF incubation sleeve; maximum volume 60 cm3, connected to in- and out-flow lines via the plug of the pressure vessel (e), (d) gold-coated stainless steel plugs with FFKM O-rings; (D) sub-sampling device; (f) 1/4′′ high-pressure line, three-way-valve (h), shut-off valve (g).
Reaction Vessel
The reaction vessel (Figure 3B) is a stainless steel cylinder (Dunze GmbH, Hamburg, Germany). The cylinder has an inner diameter of 3.5 and 27.0 cm in length (volume of 259.7 cm3). Top and bottom are closed with plugs, each with bores for four 1/16′′ HPLC lines each. The vessel is sealed with banjo screws that push the plugs into their seals.
Incubator Sleeve
The incubator (Figure 3C) is a sleeve of polyvinylidene fluoride (PVDF, Novoplast, Halberstadt, Germany), a polymer that is inert to almost all chemicals. Although this material is not as flexible as polytetrafluoroethylene (PTFE) or fluorinated ethylene propylene (FEP), it was chosen due to its very low permeability for gases (Table 1). The sleeve is closed with two gold-coated stainless steel plugs (3 μm gold thickness; Schempp and Decker, Berlin, Germany) with two gutters, each holding a perfluoro-elastomer O-ring (FFKM, Parker Hannifin, Pleidelsheim, Germany). FFKM was used for its chemical resistance. Both stoppers have a central threaded bore for the connection with a 1/16′′ HPLC line: one for inflow of medium at the bottom of the incubator and one for outflow at the top of the incubator. The incubator was designed to avoid corrosion, therefore only inert materials (PVDF, FFKM, and gold) were used. The sleeve has a diameter of 25 mm and can have a maximum length of 22 cm, which leads to a maximum volume of 68 cm3. Inside the incubator the medium first has to pass through a 1.5 cm thick layer of 2 mm diameter glass beads, followed by a 1.5 cm thick layer of quartz wool (organic free by annealing in a muffle furnace) so that the stream of medium passes evenly through the sample material over the entire cross section of the incubator sleeve. On top of the sample, the described layers follow in opposite order; first quartz wool for holding back most of the fine particles that could clog the lines and valves, followed by glass beads until the incubator is full and the sample well packed.
Additional to the chemical resistance and the almost complete impermeability for gases, further advantages of using a PVDF sleeve is its relatively low price, allowing for the possibility of using it as a disposable article. Thereby, cross contamination between samples can be excluded. Furthermore, mechanical stress leading to a weakening of the material and, therefore, a possible leakage of medium or inflow of pressure fluid will be prevented. The incubator sleeve hangs inside the reaction vessel and is connected to the top plug of the incubation vessel via the 1/16′′ HPLC lines.
Sub-Sampling System
A sub-sampling device allows taking fluid samples during the experiment without decompression (Figure 3D). It is attached to the high-pressure line between the reservoir vessel and the reaction vessel. The sub-sampler is made from 1/4′′ stainless steel tubing (7.9 cm in length, inner diameter 0.225 cm, total volume 0.513 cm3) and has a three-way-valve (Swagelok Limited, Tromode, UK) at the top and a shut-off valve (Supelco) at the bottom. The three-way-valve connects the sub-sampler to the incubation system. The third connection of the valve is used to either apply vacuum to the sub-sampler prior to sampling to avoid oxidation of the sample or to apply overpressure (nitrogen gas) to push out the remainder of the sample. After the sub-sampler is evacuated, the three-way-valve is turned and the sample enters the sub-sampler. Then the three-way-valve is closed and the shut-off valve at the bottom of the system is opened and the sample transferred into a sampling vial. Nitrogen Gas is added through the three-way-valve to push out the remaining sample.
Gages and Pumps, Other Hardware
Pressure is generated by a modified HPLC pressure pump (SYKAM S 1122, Sykam GmbH, Fuerstenfeldbruck, Germany, modifications according to Kallmeyer et al., 2003). A second identical pump is used to circulate the medium through the reservoir vessel and the incubator sleeve. Pressure is kept constant through a backpressure valve (pressure regulator series KHB, Swagelok Limited, Tromode, UK).
All pressure vessels (reservoir and reaction vessel) are connected to 100 MPa pressure gages (WIKA Alexander Wiegand SE and Co. KG, Klingenberg, Germany). All pumps and vessels are connected with 1/16′′ HPLC lines (CS Chromatographie, Langerwehe, Germany). If not mentioned otherwise all valves were obtained from Supelco, Bellefonte, PA, USA.
Gas Trap
To avoid the possible entry of gas bubbles into the pump, an empty HPLC column (25.1 cm in length, inner diameter 0.45 cm, total volume 4 cm3; Sykam GmbH, Fuerstenfeldbruck, Germany) is used as a gas trap, mounted vertically between the reaction vessel and the pump to collect any gas bubbles that may form. The medium flows from top to bottom, so the gas bubbles are trapped at the top.
Application of the System
We used the system for the extraction of low molecular weight organic acids, which are a potential microbial energy source, from a coal sample using water and water–carbon dioxide mixture at 90°C and 5 MPa. In a second application we incubated sediment from a mud volcano that is known to exhibit high rates of anaerobic methane oxidation at 23°C and 10 MPa total pressure and 4 MPa methane partial pressure (96 mmol/l) and measured an increase in sulfide concentration.
Coal Sample
We selected a sub-bituminous coal sample [vitrinite reflectance (R0): 0.29%] from the Whangamarino formation (latest Miocene to late Pliocene), which is part of the Tauranga group. The sample was from a depth of 64.69 m below surface, taken from the DEBITS-1 well, which was drilled in 2004 within the scope of the deep biosphere in terrestrial systems (DEBITS) project at Ohinewai in the Waikare Coal Field of the Waikato Basin on the North Island of New Zealand.
The well had a total depth of 148 m and penetrated interbedded layers of organic-rich carbon (lignites and sub-bituminous coals) as well as mudstones, siltstones, and sandstones. The total organic carbon (TOC) content in the sample is approximately 30%. For further information about the sample material and the geology of the Waikato Basin see Glombitza et al. (2009).
Low molecular weight organic acids (LMWOAs) such as formate, acetate, and oxalate are known to be the main organic compounds obtained from aqueous extractions of lignites and coals (Vieth et al., 2008). The LMWOAs are also components of the macromolecular organic material of the coal (Glombitza et al., 2009) and are released from the coal matrix during ongoing maturation into the surrounding pore water.
Mud Volcano Sediment
Isis mud volcano lies on the Egyptian continental margin in the Nile deep-sea fan (NDSF) in a water depth of ∼991 m and covers an area of approximately 10 km2. The NDSF is a sedimentary wedge that is deposited since the late Miocene by the Nile river (Loncke et al., 2004) with an assumed thickness of up to 10 km. Deeper sediments become strongly overpressured by the thick sedimentary overburden, resulting in an upward migration of fluids and gases (Loncke et al., 2004). Among other mud volcanoes in the area, IMV is emitting large volumes of gas (Dupré et al., 2008), including methane, ethane, and propane (Mastalerz et al., 2009). These gases are probably a mixture from different sources, because their isotopic composition is rather inconclusive with regards to a thermogenic or microbial origin (Mastalerz et al., 2007).
The emitted gases – mostly methane – are substrates for microorganisms. In sediment samples of the IMV Omoregie et al. (2009) found several genera of sulfate-reducing bacteria (Desulfosarcina, Desulfococcus, Desulfocapsa, Desulfobulbus) as well as Methanococcoides, a methanogenic Archea, and the anaerobic methane oxidizers ANME-1, ANME-2, and ANME-3.
The sample was taken during the NAUTINIL expedition in 2003 at 32°22′N; 31°23′E in 1020 m water depth and stored in a glass bottle at 4°C with a nitrogen headspace. About a week prior to the experiments, the headspace was flushed with methane. Immediately prior to the incubation experiments, concentration of hydrogen sulfide and methane in the pore water was around 6 and 4.8 mmol/l, respectively.
Experimental Procedure
We extracted the coal sample at elevated temperature (90°C) and pressure (5 MPa) using deionized water in the first experiment and a water–carbon dioxide mixture in the second. Five grams (approx. 9.4 cm3) of the freeze-dried and powdered coal sample were placed in the incubator sleeve. For the water extraction, the experiment was started after reservoir and reaction vessel had reached 5 MPa and 90°C. In the second experiment with the H2O–CO2 mixture, gas was added to the reservoir vessel after heating to 90°C and left overnight for equilibration. The experiment and the circulation of the gas-saturated medium started after equilibration. Pressure was generated by adding CO2 until 5 MPa were reached and pressure had stabilized as maximum gas saturation had been reached (approximately 106 g/l or 2.4 mol/l of dissolved CO2).
Extractions were carried out for a total of 48 h. Subsamples (0.513 cm3) of the medium were taken after 6, 22, 30, and 48 h and flushed into 513 μl of a 3.6/3.4 mmol/l solution of Na2CO3/NaHCO3 containing 2% isopropanol to reduce the volatility of the LMWOAs thereby avoiding a loss of these compounds. The subsamples were immediately frozen until analysis (within 1 week). Sample analysis was performed by ion chromatography (IC) without further dilution.
The incubation of sediment samples from IMV was performed at a pressure of 10 MPa and a temperature of 23°C, using an artificial seawater medium (Widdel and Bak, 1992) with a sulfate concentration of 27 mmol/l.
In the first experiment the medium contains just 0.1 mmol/l of methane (from the dilution of the sediment sample pore water methane), whereas in the second experiment the medium contained 96 mmol/l methane. In order to be able to detect even small amounts of hydrogen sulfide, the volume of the reservoir vessel was reduced to 145 ml by adding glass beads (5 mm diameter). Inside an anaerobic glove box 10 cm3 of IMV sediment were loaded into the incubator sleeve. The experiment with only artificial anoxic seawater medium was started after reservoir and reaction vessel were equilibrated to 10 MPa and 23°C. In the second experiment the medium was first pressurized with methane to 4 MPa, leading to a methane concentration of approximately 1.6 g/l or 96 mmol/l of dissolved methane and left overnight for equilibration. After methane saturation was complete, pressure was increased hydrostatically to 10 MPa with anoxic artificial seawater medium. The sample was loaded into the incubation vessel and pressurized prior to the experiment; circulation of the gas-saturated medium started after equilibration.
Incubations were carried out for a total of 432 h (9 days). Subsamples (0.513 cm3) of the medium were taken every 2 days. The subsamples were mixed with equal volumes of a 5% (w/v) zinc acetate solution to fix the volatile sulfide as zinc sulfide. The fixed subsamples were immediately frozen until photometric analysis.
Sample Analysis by Ion Chromatography
The samples were analyzed in replicates using IC. The IC system (Sykam GmbH, Fuerstenfeldbruck, Germany) was equipped with an LCA A 20 column, a suppressor (SAMS, SeQuant, Sweden) and a SYKAM S3115 conductivity detector. The mobile phase was a 1.8/1.7 mmol/l Na2CO3/NaHCO3 mixture. Elution was performed at isocratic conditions. The eluent flow was set to 0.8 ml/min. A blank sample (deionized water) and a multi-compound standard containing each 50 mg/l formate, acetate, and oxalate (for the extraction of coal) were measured prior to each sample. Standard deviation of sample and standard quantification was below 10% (determined from replicate analysis).
Hydrogen Sulfide Quantification
Hydrogen sulfide concentration was quantified according to Cline (1969). In brief, 5 ml of deionized water and 400 μl of Cline-reagent (1.6 g of N,N-dimethyl-p-phenylenediamine sulfate plus 2.4 g of FeCl3·6H2O in 100 ml 50% HCl) are added to the sample. Adsorption is measured in a photometer at 680 nm after 20 min. The minimum detection limit with a 1 cm cell is around 50 μmol/l.
Results
The main organic compounds extracted with water at 5 MPa and 90°C are formate, acetate, and oxalate. Vieth et al. (2008) reported comparable results from aqueous Soxhlet extraction of similar sample material from the DEBITS-1 well. The amounts of extracted LMWOAs increase over the course of the experiment (Figure 4, blue circles). The strongest increase in concentration of LMWOAs was observed during the first 22 h of extraction. In the following 26 h a slow but steady increase of extracted LMWOA was observed, suggesting that the experiment did not run to completion after 48 h. Nevertheless, the yields of the extracted LMWOAs are approaching steady state. Total amounts of extracted LMWOAs after 48 h were 2.3 mg/gTOC formate, 3.8 mg/gTOC acetate, and 5.2 mg/gTOC oxalate.
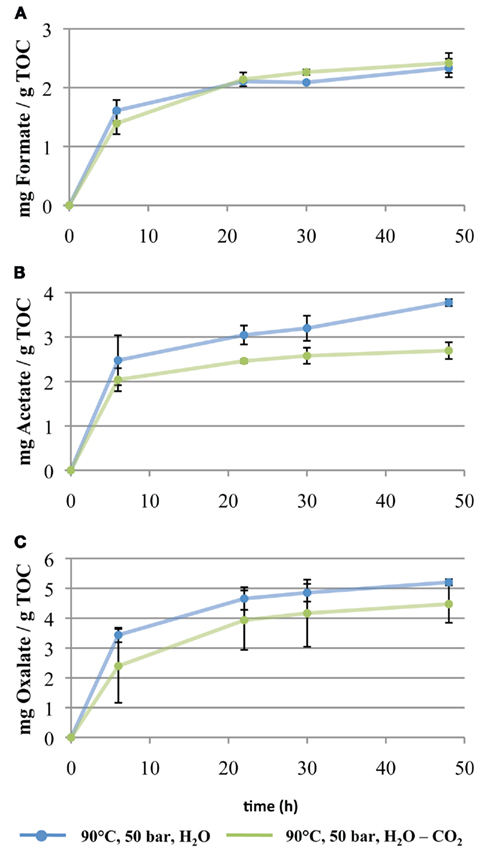
Figure 4. Extraction of (A) formate, (B) acetate, and (C) oxalate from sub-bituminous coal sample taken from the DEBITS-1 well at Waikato Coal Area, North Island, New Zealand. Five grams of coal (approximately 30% TOC) were extracted for 48 h at 5 MPa and 90°C. Extractions were performed with water (blue circles) and water–carbon dioxide mixture (green triangles). Sample analysis was performed by ion chromatography.
In the second coal extraction experiment we used an H2O–CO2 mixture under the same pressure and temperature conditions (90°C, 5 MPa) but with 2.4 mol/l CO2. Under these conditions, the same organic compounds (formate, acetate, and oxalate) were extracted (Figure 4, green circles). Like in the experiment with deionized water, the amounts of extracted LMWOAs increase with increasing extraction time, with the main increase during the first 20 h. The total amounts of extracted LMWOAs after 48 h were 2.4 mg/gTOC for formate, 2.7 mg/gTOC for acetate, and 4.5 mg/gTOC for oxalate. These numbers are somewhat lower than in the pure water extraction experiment (Figure 4).
During the first 48 h of both IMV sediment incubation experiment, hydrogen sulfide concentration increased to about 0.3 mmol/l due to the mixing of the sediment’s pore water hydrogen sulfide with the medium. Over the course of the first experiment the concentration remained around 0.3 mmol/l for the remainder of the experiment (Figure 5, red circles).
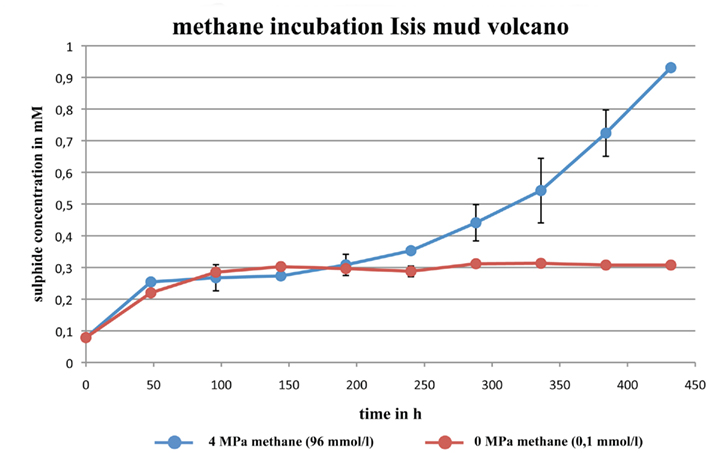
Figure 5. Incubation of sediment samples from Isis mud volcano (IMV). Ten cubic centimeter of sediment were incubated for 432 h at 10 MPa and 23°C. Incubations were performed with 4 MPa methane (blue circles) and without addition of methane (red circles).
In the second experiment with the added methane (4 MPa, 96 mmol/l), sulfide concentration remained around 0.3 mmol/l until ca. 144 h, before increasing almost exponentially (Figure 5, blue circles). The experiment was stopped after 432 h at a hydrogen sulfide concentration of 0.93 mmol/l.
Discussion
The extracted LMWOAs (formate, acetate, and oxalate) have also been found to be the main organic acids obtained from water extraction of other low mature coals (Bou-Raad et al., 2000; Vieth et al., 2008; Glombitza, 2011).
High-pressure (5 MPa) extraction with pure water in our system resulted in significantly higher yields (2.4–4.5 mg organic acids/gTOC) than what was reported from Soxhlet extraction of coal samples from a similar depth interval of the DEBITS-1 well, which yielded between 0.7 and 1.4 mg organic acid/gTOC for individual LMWOAs (Vieth et al., 2008). The extraction of LMWOAs resulted in a decrease of pH in the extraction medium. The pH in the reservoir of a Soxhlet apparatus decreases to approximately pH 4 after 48 h of extraction with deionized water (data not shown). However, the sample only gets into contact with freshly distilled water (pH 7) that drips over the sample. In the high-pressure system the extraction medium containing the extracted organic acids circulates through the system, thereby extracting the coal with a low-pH medium. The lower pH is supposed to enhance the release of LMWOAs from macromolecular organic matter in the coal by hydrolysis (Glombitza, 2011). This might explain the higher extraction yields of LMWOAs in our reactor system as compared to Soxhlet extraction.
Under in situ conditions, the extracted LMWOAs will remain in the pore water and thereby cause a drop in pH before they are eventually removed by diffusion or fluid flow. As Fry et al. (2009) reported from samples from the DEBITS-1 well, the majority of microbial activity and abundance is not found in the coals but rather in the surrounding and more porous sandstones. So the consumption of the produced LMWOA does not take place inside the coal seams but above or below them and removal of these substances from the coals is controlled by diffusion or fluid flow, not by microbial activity. It is therefore reasonable to assume that our high-pressure system provides reaction conditions that are much more realistic than Soxhlet extraction because the extracted compounds are not removed from the reaction.
When comparing the results of the first extraction with deionized water and the second extraction with an H2O–CO2 mixture, it becomes obvious that CO2 reduces the amount of extracted acetate by a factor of 1.39 and oxalate by a factor of 1.16. For formate no clear influence of CO2 on the extraction efficiency could be observed. At first sight, this result is surprising because carbon dioxide dissolved in water lowers the pH due to formation of carbonic acid (Meyassami et al., 1992). The lower pH was expected to enhance hydrolysis and, therefore, increase the yield of extractable LMWOAs. However, we observed a suppressing effect of CO2.
The LMWOAs found in the extraction fluid may not just result from the actual extraction of the coal but also from different secondary reactions. It was suggested that oxalate in aqueous extracts of coals is a result of the decomposition of 1,2-dihydroxy-carboxylic acids (Bou-Raad et al., 2000). Therefore it has to be assumed that at least for oxalate (and maybe for other LMWOAs as well) the extraction yield is not only affected by hydrolysis but also by secondary reactions, which may be inhibited or suppressed in the presence of CO2 in the extraction medium.
The incubation experiment with sediment samples from the Isis mud volcano clearly showed the positive effect of elevated methane concentration on the rate of sulfide production, which is a direct result of sulfate reduction. Omoregie et al. (2009) conducted whole core radiotracer incubations of samples from the same mud volcano at atmospheric pressure (0.1 MPa) and with a maximum methane concentration of >10 μmol l−1. They measured sulfate reduction rates of 7–240 nmol cm−3 day−1. We conducted our experiment at much higher methane concentration (96 mmol l−1) and in situ pressure (10 MPa), and measured a significantly higher sulfate reduction rate of ca. 2000 nmol cm−3 day−1, which we attribute to the elevated methane concentration, as already shown by Nauhaus et al. (2002).
The aim of this paper is to present a new high-pressure incubation system and experiments to demonstrate the application of elevated temperature and pressure as well the use of elevated gas saturation and their effects on geochemical and microbiological processes. Therefore, we can only speculate about the reasons for the observed suppressing effect of the CO2. This effect still remains puzzling and will be the topic of future investigations.
Conclusion
The high-pressure high-temperature incubation system is a moderately priced alternative to existing systems. Furthermore, it is easy to construct and to handle. Initial experiments demonstrate that the system is suitable for a wide range of applications in geo- and bio-sciences. The system allows the incubations at elevated pressure and temperature conditions (up to 120°C and 60 MPa) as well as manipulating the dissolved gases throughout the experiment. The system also allows sub-sampling of the fluid phase during the course of the experiment without decompression. Extraction of a sub-bituminous coal samples under high-pressure and temperature conditions showed a higher yield in LMWOAs from macromolecular organic matter as compared to an extraction with a Soxhlet apparatus. The high-pressure extraction of the coal sample with CO2-saturated water revealed a suppressing effect of the CO2 on the extraction yield or secondary formations of LMWOAs. Possible reasons for this effect are not identified yet and will be in the focus of future investigations. The incubation of sediment samples from a mud volcano harboring sulfate-reducing methanotrophs showed a clear positive response of methane addition on the sulfate reduction rate. Our high-pressure high-temperature incubation system has proven its suitability for a broad range of scientific applications.
Conflict of Interest Statement
The authors declare that the research was conducted in the absence of any commercial or financial relationships that could be construed as a potential conflict of interest.
Acknowledgments
We would like to thank Harald Huber from the University of Regensburg for providing high-pressure equipment. The Helmholtz Centre Potsdam is gratefully acknowledged for providing the sample material for the test experiment. We thank Ann-Kathrin Scherf from the German Research Centre for Geosciences (GFZ) for the Soxhlet extraction of coal samples and pH measurement as well as Dr. Gunter Wegener from the Max Planck Institute fro Marine Microbiology for the sediment samples from Isis mud volcano. Furthermore we thank the Federal Ministry of Education and Research (BMBF) for funding this work within the “Forschungsverbund Geoenergien” (GeoEn, research network geoenergy).
References
Abe, F., Kato, C., and Horikoshi, K. (1999). Pressure-regulated metabolism in microorganisms. Trends Microbiol. 7, 447–453.
Bartlett, D. H. (2002). Pressure effects on in vivo microbial processes. Biochim. Biophys. Acta 1595, 367–381.
Bartlett, D. H., Wright, M., Yayanos, A. A., and Silverman, M. (1989). Isolation of a gene regulated by hydrostatic pressure in a deep-sea bacterium. Nature 342, 572–574.
Bernhardt, G., Jaenicke, R., and Lüdemann, H. D. (1987). High-pressure equipment for growing methanogenic microorganisms on gaseous substrates at high temperature. Appl. Environ. Microbiol. 53, 1876–1879.
Boonyaratanakornkit, B. B., Cordova, J., Park, C. B., and Clark, D. S. (2006). Pressure affects transcription profiled of Methanocaldococcus jannaschii despite the absence of barophilic growth under gas-transfer limitation. Environ. Microbiol. 8, 2031–2035.
Boonyaratanakornkit, B. B., Miao, L. Y., and Clark, D. S. (2007). Transcriptional response of the deep-sea hyperthermophile Methanocaldococcus jannaschii under shifting extremes of temperature and pressure. Extremophiles 11, 495–503.
Bothun, G. D., Knutson, B. L., Berberich, J. A., Strobel, H. J., and Nokes, S. E. (2004). Metabolic selectivity and growth of Clostridium thermocellum in continuous culture under elevated hydrostatic pressure. Appl. Microbiol. Biotechnol. 65, 149–157.
Bou-Raad, M., Hobday, M. D., and Rix, C. J. (2000). Aqueous extraction of oxalate and other anions from coal. Fuel 79, 1185–1193.
Chivian, D., Brodie, E. L., Alm, E. J., Culley, D. E., Dehal, P. S., Desantis, T. Z., Gihring, T. M., Lapidus, A., Lin, L.-H., Lowry, S. R., Moser, D. P., Richardson, P. M., Southam, G., Wanger, G., Pratt, L. M., Anderson, G. L., Hazen, T. C., Brockman, F. J., Arkin, A. P., and Onstott, T. C. (2008). Environmental genomics reveals a single-species ecosystem deep within earth. Science 322, 275–278.
Cline, J. D. (1969). Spectrophotometric determination of hydrogen sulfide in natural waters. Limnol. Oceanogr. 14, 454–458.
Delong, E. F., and Yayanos, A. A. (1985). Adaptation of the membrane lipids of a deep-sea bacterium to changes in hydrostatic pressure. Science 228, 1101–1103.
Dupré, S., Buffet, G., Mascle, J., Foucher, J. P., Gauger, S., Boetius, A., and Marfia, C. The AsterX AUV Team, The Quest ROV Team, and The BIONIL scientific party. (2008). High-resolution mapping of large gas emitting mud volcanoes on the Egyptian continental margin (Nile deep sea fan) by AUV surveys. Mar. Geophys. Res. 29, 275–290.
Fang, J., Zhang, L., and Bazylinski, D. A. (2010). Deep-sea piezosphere and piezophiles: geomicrobiology and biogeochemistry. Trends Microbiol. 18, 413–422.
Fry, J. C., Horsfield, B., Sykes, R., Cragg, B. A., Heywood, C., Kim, G. T., Mangelsdorf, K., Mildenhall, D. C., Rinna, J., Vieth, A., Zink, K.-G., Sass, H., Weightman, A. J., and Parkes, R. J. (2009). Prokaryotic populations and activities in an interbedded coal deposit, including a previously deeply buried section (1.6-2.3 km) above (∼150 Ma basement rock. Geomicrobiol. J. 26, 163–178.
Glombitza, C. (2011). New Zealand Coals – A Potential Feedstock for Deep Microbial Life. Thesis, Technical University Berlin, Berlin.
Glombitza, C., Mangelsdorf, K., and Horsfield, B. (2009). A novel procedure to detect low molecular weight compounds released by alkaline ester cleavage from low maturity coals to assess its feedstock potential for deep microbial life. Org. Geochem. 40, 175–183.
Hei, D. J., and Clark, D. S. (1994). Pressure stabilization of proteins from extreme thermophiles. Appl. Environ. Microbiol. 60, 932–939.
Horne, R. A., and Courant, R. A. (1965). Protonic conduction in the water I region. J. Phys. Chem. 69, 2224–2230.
Jaenicke, R. (1983). Biochemical processes under high hydrostatic pressure. Naturwissenschaften 70, 332–341.
Jannasch, H. W., Wirsen, C. O., and Doherty, K. W. (1996). A pressurized chemostat for the study of marine barophilic and oligotrophic bacteria. Appl. Environ. Microbiol. 62, 1593–1596.
Kallmeyer, J., Ferdelman, T. G., Jansen, K.-H., and Jorgensen, B. B. (2003). A high-pressure thermal gradient block for investigating microbial activity in multiple deep-sea samples. J. Microbiol. Methods 55, 165–172.
Kaneshiro, S. M., and Clark, D. S. (1995). Pressure effects on the composition and thermal behavior of lipids from the deep-sea thermophile. J. Bacteriol. 177, 3668–3672.
L’Haridon, S., Reysenbach, A.-L., Glénat, P., Prieur, D., and Jeanthon, C. (1995). Hot subterranean biosphere in a continental oil reservoir. Nature 377, 223–224.
Lin, L.-H., Wang, P.-W., Rumble, D., Lippmann-Pipke, J., Boice, E., Pratt, L. M., Sherwood-Lollar, B., Brodie, E. L., Hazen, T. C., Anderson, G. L., Desantis, T. Z., Moser, D. P., Kershaw, D., and Onstott, T. C. (2006). Long-term sustainability of a high-energy, low-diversity crustal biome. Science 314, 479–482.
Loncke, L., Mascle, J., and Fanil Scientific Parties. (2004). Mud volcanoes, gas chimneys, pockmarks and mounds in the Nile deep-sea fan (Eastern Mediterranean): geophysical evidences. Mar. Petrol. Geol. 21, 669–689.
Mastalerz, V., deLange, G. J., and Dählmann, A. (2009). Differential aerobic and anaerobic oxidation of hydrocarbon gases discharged at mud volcanoes in the Nile deep-sea fan. Geochim. Cosmochim. Acta 73, 3849–3863.
Mastalerz, V., de Lange, G. J., Dählmann, A., and Feseker, T. (2007). Active venting at the Isis mud volcano, offshore Egypt: origin and migration of hydrocarbons. Chem. Geol. 246, 87–106.
Meyassami, B., Balaban, M. O., and Teixera, A. A. (1992). Prediction of pH in model systems pressurized with carbon dioxide. Biotechnol. Prog. 8, 149–154.
Miller, J. F., Almond, E. L., Shah, N. N., Ludlow, J. M., Zollweg, J. A., Streett, W. B., Zinder, S. H., and Clark, D. S. (1988). High-pressure-temperature bioreactor for studying pressure-temperature relationships in bacterial growth and productivity. Biotechnol. Bioeng. 31, 407–413.
Mukhopadhyay, B., Johnson, E. F., and Wolfe, R. S. (1999). Reactor-scale cultivation of the hyperthermophilic methanarchaeon Methanococcus jannaschii to high cell densities. Appl. Environ. Microbiol. 65, 5059–5065.
Nauhaus, K., Boetius, A., Kruger, M., and Widdel, F. (2002). In vitro demonstration of anaerobic oxidation of methane coupled to sulphate reduction in sediment from a marine gas hydrate area. Environ. Microbiol. 4, 296–305.
Omoregie, E. O., Niemann, H., Mastalerz, V., de Lange, G. J., Stadnitskaia, A., Mascle, J., Foucher, J.-P., and Boetius, A. (2009). Microbial methane oxidation and sulfate reduction at cold seeps of the deep Eastern Mediterranean Sea. Mar. Geol. 261, 114–127.
Orcutt, B., Samarkin, V., Boetius, A., and Joye, S. (2008). On the relationship between methane production and oxidation by anaerobic methanotrophic communities from cold seeps of the Gulf of Mexico. Environ. Microbiol. 10, 1108–1117.
Parkes, R. J., Sellek, G., Webster, G., Martin, D., Anders, E., Weightman, A. J., and Sass, H. (2009). Culturable prokaryotic diversity of deep, gas hydrate sediments: first use of a continuous high-pressure, anaerobic, enrichment and isolation system for subseafloor sediments (DeepIsoBUG). Environ. Microbiol. 11, 3140–3153.
Pedersen, K. (2000). Exploration of deep intraterrestrial microbial life: current perspectives. FEMS Microbiol. Lett. 185, 9–16.
Raven, N., Ladwa, N., Cossar, D., and Sharp, R. (1992). Continuous culture of the hyperthermophilic archaeon Pyrococcus furiosus. Appl. Microbiol. Biotechnol. 38, 263–267.
Schmid, G., Lüdemann, H.-D., and Jaenicke, R. (1978). Oxidation of sulfhydryl groups in lactate dehydrogenase under high hydrostatic pressure. Eur. J. Biochem. 86, 219–224.
Schulz, E., Lüdemann, H.-D., and Jaenicke, R. (1976). High pressure equilibrium studies on the dissociation – association of E. coli ribosomes. FEBS Lett. 64, 40–43.
Seyfried, W. E. Jr. (1979). A new reaction cell for hydrothermal solution equipment. Am. Mineral. 64, 646–649.
Seyfried, W. E. Jr., and Janecky, D. R. (1985). Heavy metal and sulfur transport during subcritical and supercritical hydrothermal alteration of basalt: influence of fluid pressure and basalt composition and crystallinity. Geochim. Cosmochim. Acta 49, 2545–2560.
Sun, M. M. C., and Clark, D. S. (2001). Pressure effects on activity and stability of hyperthermophilic enzymes. Meth. Enzymol. 334, 316–327.
Takai, K., Miyazaki, M., Hirayama, H., Nakagawa, S., Querellou, J., and Godfroy, A. (2009). Isolation and physiological characterization of two novel, piezophilic, thermophilic chemolithoautotrophs from a deep-sea hydrothermal vent chimney. Environ. Microbiol. 11, 1983–1997.
Takai, K., Nakamura, K., Toki, T., Tsunogai, U., Miyazaki, M., Miyazaki, J., Hirayama, H., Nakagawa, S., Nunoura, T., and Horikoshi, K. (2008). Cell proliferation at 122°C and isotopically heavy CH4 production by a hyperthermophilic methanogen under high-pressure cultivation. Proc. Natl. Acad. Sci. U.S.A. 105, 10949–10954.
Taylor, C. D., and Jannasch, H. W. (1976). Subsampling techniques for measuring growth of bacterial cultures under high hydrostatic pressure. Appl. Environ. Microbiol. 32, 355–359.
Vieth, A., Mangelsdorf, K., Sykes, R., and Horsfield, B. (2008). Water extraction of coals – potential for estimating low molecular weight organic acids as carbon feedstock for the deep terrestrial biosphere. Org. Geochem. 39, 985–991.
Vreeland, R. H., Piselli, A. F. Jr., Mcdonnough, S., and Meyers, S. S. (1998). Distribution and diversity of halophilic bacteria in a subsurface salt formation. Extremophiles 2, 321–331.
Widdel, F., and Bak, F. (1992). “Gram negative mesophilic sulphate-reducing bacteria,” in The Prokaryotes, eds A. Balows, H. G. Trüper, M. Dworkin, W. Harder, and K.-H. Schleifer (New York: Springer), 3352–3378.
Yayanos, A. A. (1969). A technique for studying biological reaction rates at high pressure. Rev. Sci. Instrum. 40, 961–963.
Yayanos, A. A. (1986). Evolutional and ecological implications of the properties of deep-sea barophilic bacteria. Proc. Natl. Acad. Sci. U.S.A. 83, 9542–9546.
Yayanos, A. A., and Dietz, A. S. (1983). Death of a Hadal deep-sea bacterium after decompression. Science 220, 497–498.
Yayanos, A. A., Dietz, A. S., and Van Boxtel, R. (1979). Isolation of a deep-sea barophilic bacterium and some of its growth characteristics. Science 205, 808–810.
Keywords: high-pressure incubation system, gas partial pressure, sub-sampling, carbon dioxide, low molecular weight organic acids
Citation: Sauer P, Glombitza C and Kallmeyer J (2012) A system for incubations at high gas partial pressure. Front. Microbio. 3:25. doi: 10.3389/fmicb.2012.00025
Received: 09 September 2011;
Accepted: 15 January 2012;
Published online: 03 February 2012.
Edited by:
Andreas Teske, University of North Carolina at Chapel Hill, USAReviewed by:
Doug Bartlett, Scripps Institution of Oceanography, USAGordon Webster, Cardiff University, UK
Copyright: © 2012 Sauer, Glombitza and Kallmeyer. This is an open-access article distributed under the terms of the Creative Commons Attribution Non Commercial License, which permits non-commercial use, distribution, and reproduction in other forums, provided the original authors and source are credited.
*Correspondence: Patrick Sauer, Geomicrobiology Group, Institute for Earth- and Environmental Sciences, University of Potsdam, Karl-Liebknecht-Straße 24-25, 14476 Potsdam, Germany. e-mail:c2F1ZXJAZ2VvLnVuaS1wb3RzZGFtLmRl