- 1 Research Institute for Environmental Management Technology, National Institute of Advanced Industrial Science and Technology, Tsukuba, Japan
- 2 Department of Ocean Sciences, Tokyo University of Marine Science and Technology, Minato-ku, Tokyo, Japan
We measured pools of dissolved phosphorus (P), including dissolved inorganic P (DIP), dissolved organic P (DOP) and alkaline phosphatase (AP)-hydrolyzable labile DOP (L-DOP), and kinetic parameters of AP activity (APA) in the euphotic zone in the western North Pacific Ocean. Samples were collected from one coastal station in Sagami Bay, Japan, and three offshore stations between the North Pacific subtropical gyre (NPSG) and the Kuroshio region. Although DIP concentrations in the euphotic zone at all stations were equally low, around the nominal method detection limit of 20 nmol L-1, chlorophyll a (Chl a) concentrations were one order of magnitude greater at the coastal station. DOP was the dominant P pool, comprising 62–92% of total dissolved P at and above the Chl a maximum layer (CML). L-DOP represented 22–39% of the total DOP at the offshore stations, whereas it accounted for a much higher proportion (about 85%) in the coastal surface layers. Significant correlations between maximum potential AP hydrolysis rates and DIP concentrations or bacterial cell abundance in the offshore euphotic zone suggest that major APA in the oligotrophic surface ocean is from bacterial activity and regulated largely by DIP availability. Although the range of maximum potential APA was comparable among the environmental conditions, the in situ hydrolysis rate of L-DOP in the coastal station was 10 times those in the offshore stations. L-DOP turnover time at the CML ranged from 4.5 days at the coastal station to 84.4 days in the NPSG. The ratio of the APA half-saturation constant to the ambient L-DOP concentration decreased markedly from the NPSG to the coastal station. There were substantial differences in the rate and efficiency of DOP remineralization and its contribution as the potential P source between the low-phosphate/high-biomass coastal ecosystem and the low-phosphate/low biomass oligotrophic ocean.
Introduction
Phosphorus (P) is an essential nutrient for sustaining marine life (Karl, 2000). Among the P reservoirs in the ocean, dissolved inorganic P (DIP) is directly and readily available to microorganisms. In surface waters of extensive oligotrophic ocean regions, DIP concentrations are often below the detection limit of standard analytical methods. Highly sensitive methods have revealed that DIP concentrations are often around 10 nmol L−1 and sometimes <1 nmol L−1 in the North Atlantic Subtropical Gyre, the North and South Pacific Subtropical Gyres, and the eastern Mediterranean Sea (Karl and Tien, 1997; Wu et al., 2000; Cavender-Bares et al., 2001; Thingstad et al., 2005; Mather et al., 2008; Duhamel et al., 2011).
Dissolved organic P (DOP) typically comprises the major fraction (approximately 80%) of the dissolved P pool in oligotrophic surface waters (Cavender-Bares et al., 2001; Karl and Björkman, 2002). Because the DOP pool includes many compounds that can be utilized by marine microorganisms (Orrett and Karl, 1987; Björkman and Karl, 1994; Karl, 2007), it represents a potential P source for sustaining community productivity in DIP-depleted surface waters. The majority of DOP is not readily available to microbes, as it cannot be directly accessed through the cell membrane; hence these compounds must be remineralized to bioavailable phosphate prior to uptake (Cembella et al., 1984; Björkman and Karl, 2003; Paytan and McLaughlin, 2007). It is therefore essential to characterize the components of the DOP pool in order to evaluate their reactivity and lability for biological breakdown. However, surprisingly little is known about the nature and composition of marine DOP (see reviews by Karl and Björkman, 2002; Dyhrman et al., 2007; Karl, 2007). Some specific compounds or operationally defined groups of organic P, including dissolved nucleotide triphosphates and hydrophobic P, have been quantified but were typically found to comprise a few percent of total DOP in the euphotic zone of open ocean environments (Suzumura and Ingall, 2004; Björkman and Karl, 2005).
Enzymatic hydrolysis by alkaline phosphatase (AP) is arguably the most important pathway for marine DOP remineralization, as AP is found in a wide variety of eukaryotic phytoplankton and both autotrophic and heterotrophic prokaryotes (Cembella et al., 1984; Hoppe, 2003). AP is a subclass of phosphomonoesterase or phosphoric monoester hydrolase enzymes that catalyze ester (C-O-P) bond cleavage, sharing characteristic alkaline pH optima. Direct molecular analyses using 31P nuclear magnetic resonance have shown that the majority of P in both the high-molecular-weight fraction and the bulk fraction of the marine DOP pool is in phosphate esters, although these analyses could not distinguish phosphate mono- and di-esters (Kolowith et al., 2001; Young and Ingall, 2010). AP thus has the potential to hydrolyze a broad spectrum of marine DOP components.
AP activity (APA) is usually regulated by the DIP supply, as the activity increases with a decrease in DIP concentration (Cembella et al., 1984). Therefore, APA is considered a useful indicator of phosphate deficiency in ecological studies examining the marine P cycle (Van Wambeke et al., 2002; Dyhrman and Ruttenberg, 2006; Lomas et al., 2010). APA in marine environments can be measured by using phosphate monoester analogs. Fluorogenic artificial substrates are useful and sensitive analogs for examining APA even in oligotrophic marine environments with relatively low biological activity (Hoppe, 1983). Methods using single-substrate addition in excess of saturation have been generally used for estimating the maximum potential hydrolysis rate (Vmax) of APA (Koike and Nagata, 1997; Hoppe and Ullrich, 1999; Mather et al., 2008). Kinetic parameters of APA have also been assayed using multiple concentrations of the substrate (Thingstad et al., 1993; Sebastián and Niell, 2004; Sebastián et al., 2004; Labry et al., 2005). Though the procedures are complicated and time-consuming, multiple-concentration methods can provide detailed APA kinetic parameters, including the half-saturation constant and DOP turnover time as well as Vmax. To date, however, there is only limited detailed information for kinetic parameters of APA in oligotrophic open ocean environments. Recently Duhamel et al. (2011) performed an in-depth characterization of APA in the North and South Pacific Subtropical Gyres, investigating the kinetic parameters and dissolved P pools. However, the role and characteristics of APA in P cycling in the rest of the Pacific Ocean are still largely unknown.
Here we measured dissolved P pools in the euphotic zone of the western North Pacific Ocean, from the biomass-rich coastal environment adjacent to Japan to the oligotrophic oceanic environment in the North Pacific subtropical gyre (NPSG), as well as in the Kuroshio region and transition zone. In samples from all of these environments, we compared Vmax as measured by single- and multiple-concentration methods. We obtained kinetic parameters for APA, including the DOP turnover time and half-saturation constant as well as Vmax. Our major objectives were to provide information on the characteristics of dissolved P pools and APA in the western North Pacific, where such information about P cycling is largely unknown. We also examined the importance of APA to P availability under phosphate-depleted conditions from coastal to offshore regions to better understand marine P cycling.
Materials and Methods
Sampling and Pretreatments
Field data were obtained from on board the R/V Tansei-maru on cruise KT10-13 from 8 to 19 July 2010. Samples were collected at three offshore stations along longitude 137°E in the western North Pacific (station A, 30°00.1′N; station B, 32°00.3′N; station C, 33°00.1′N) and from a coastal station (station H; 35°00.0′N, 139°20.5′E) in Sagami Bay, Japan (Figure 1). A light–depth profile was measured at station B with an underwater quantum sensor (MDS-MkV/L, JFE Advantech Corp., Hyogo, Japan). Surface seawater samples were collected with a plastic bucket and transferred to a 20-L Carboy equipped with a spigot (2322-0050, Thermo Scientific Nalgene, Rochester, NY, USA). Seawater samples were also collected at depths from 10 to 200 m using 12-L Niskin sampling bottles on a multiple-bottle rosette sampler with attached conductivity/temperature/depth (CTD) sensor. A 250-mL aliquot of water samples were passed through a 100-μm nylon mesh filter (47-mm diameter, NY1H04700, Millipore, Billerica, MA, USA) in an in-line filter holder (PFA-47, Advantec, Tokyo, Japan) connected with tubing to the outlets of the Carboy or Niskin bottle to remove large organisms and macro-detritus. A portion of the 100-μm-filtered sample was further filtered through a 0.45-μm membrane filter on a filtration apparatus (Steritop and Stericup vacuum filter cups, 150-mL funnel and bottle, SCHV U01 RE, Millipore) under gentle vacuum (<26 kPa). The filtrates were stored frozen at −30°C until P analyses at the laboratory within 1 month of sampling.
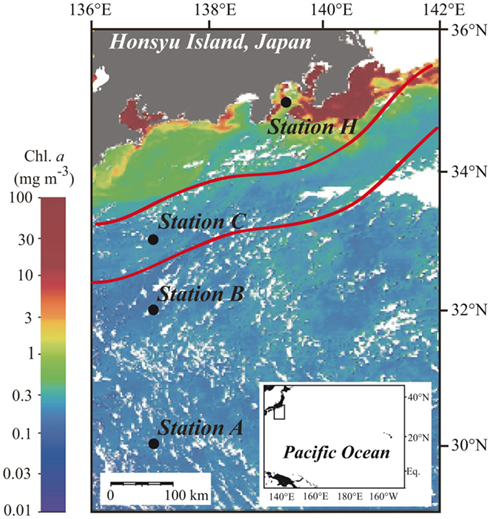
Figure 1. Locations of sampling stations in the western North Pacific Ocean superimposed on the moderate resolution imaging spectroradiometer (MODIS) chlorophyll image from 19 July 2010. White areas have no data because of cloud cover. The MODIS chlorophyll image is courtesy of the Japan Aerospace Exploration Agency (JAXA) and Tokai University. The red lines indicate the northern and southern boundaries of the Kuroshio Current on 14 July 2010 (Marine Information Service Office, Oceanographic Data Information Division, Hydrographic and Oceanographic Department, Japan Coast Guard, 2010).
Chlorophyll a and Bacterial Cell Abundance
Chlorophyll a (Chl a) was measured on 200-mL samples filtered onto 0.7-μm pore-size glass fiber filters (GF/F, 25-mm diameter; Whatman, Kent, UK), using N,N-dimethylformamide extraction and a fluorometer (model 10-AU, Turner Designs, Sunnyvale, CA, USA; Suzuki and Ishimaru, 1990). Bacterial cell abundance was determined using the filtrates from 0.45-μm-filtered samples. The sample (10 mL) was fixed in neutral buffered formaldehyde at a final concentration of 0.74% (weight:vol) and stored at 4°C. Bacterial cells were collected on a 0.2-μm pore-size, black Nuclepore filter (Whatman), and enumerated using epifluorescence microscopy after staining with 4′,6-diamidino-2-phenylindole (DAPI; Porter and Feig, 1980). All reagents used in these and other analyses were of analytical grade unless otherwise specified.
Dissolved P Analysis
Dissolved P was measured in 0.45-μm filtrates. DIP was measured spectrophotometrically on 20-mL samples by the standard molybdenum blue method (Strickland and Parsons, 1972) using a double-beam spectrophotometer (UV-1600, Shimadzu, Kyoto, Japan) with a 10-cm pathlength. We also used a highly sensitive colorimetric assay for DIP determination (Hashihama et al., 2009) in samples collected from offshore stations A, B, and C. For this more sensitive assay, seawater samples were collected directly from the Niskin bottles without any prefiltration in order to minimize contamination. The apparatus for the sensitive method consisted of a gas-segmented continuous flow analyzer (AutoAnalyzer II, Technicon, now SEAL Analytical, Hampshire, UK) equipped with a 1-m long liquid waveguide capillary cell (LWCC-2100, World Precision Instruments, Sarasota, FL, USA) We used the manifold configurations, flow diagrams, and analytic reagent preparation procedures of Hansen and Koroleff (1999). The limits of detection for DIP were 20 and 3 nmol L−1 by the standard molybdenum blue method and the sensitive method, respectively, as estimated from three times the SD of the blank.
Samples for total dissolved P (TDP) were processed according to the persulfate oxidation method of Ridal and Moore (1990). Triplicate 10-mL samples were autoclaved at 125°C for 4 h with the addition of potassium persulfate (N and P analytical grade, Wako Pure Chemical Industries, Osaka, Japan) at 4 mg mL−1. After samples had cooled to room temperature, ascorbic acid was added to reduce any excess free chlorine that had formed (Hansen and Koroleff, 1999). The DIP concentration in the oxidized samples was then measured spectrophotometrically by the manual method. DOP concentration was calculated as the difference between TDP and DIP.
We defined labile DOP (L-DOP) as the AP-hydrolyzable fraction of the DOP pool. We used the procedure of Feuillade and Dorioz (1992) to determine L-DOP. This method was originally developed to determine enzymatically hydrolyzable P in sediment samples and has been applied to the hydrolysis of high-molecular-weight L-DOP in seawater (Suzumura et al., 1998). For this assay, triplicate 8-mL aliquots of 0.45-μm-filtered seawater samples were collected immediately after filtration and mixed with 80-μL Tris–Mg buffer solution [1 mol L−1 Tris-(hydroxymethyl)aminomethane, 0.01 mol L−1 magnesium chloride, 500 mmol L−1 sodium azide, pH 7.5] containing bovine intestinal mucosa AP (Sigma-Aldrich, St. Louis, MO, USA) at 500 units mL−1 and incubated on board at 30°C in the dark for 4 h. After incubation, the samples were stored frozen at −30°C until DIP analysis. The L-DOP concentration was calculated as the increase in DIP concentration following the AP treatment.
Measurement of APA
AP activity was measured in the whole-water samples that had passed through the 100-μm nylon mesh filter. APA was measured using 4-methylumbelliferyl phosphate (MUF-P, Sigma-Aldrich) as the fluorogenic substrate (Hoppe, 1983). Stock solutions of MUF-P at 20 mmol L−1 in 2-methoxyethanol (Wako Pure Chemical Industries) were stored at −20°C and diluted with autoclaved, filtered seawater just prior to use. Three replicate measurements were taken on all samples to determine Vmax with an excess concentration of the substrate (200 μmol L−1). To determine the other kinetic parameters at the sample depths, the assay was performed using a series of five MUF-P concentrations (50, 100, 200, 500, and 1000 nmol L−1) with duplicate samples at each concentration. Samples were incubated for 5–10 h in the dark at 25 (stations A, B, and C) or 20°C (station H). The concentration of 4-methylumbelliferone (MUF) liberated from the hydrolysis of MUF-P was measured using a spectrofluorometer (RF-5300 PC, Shimadzu) with excitation at 365 nm and emission at 450 nm. The concentrations of MUF were calculated from a standard curve prepared at each sampling station using MUF (Sigma-Aldrich) at concentrations from 50 to 1000 nmol L−1. The linearity of the assay within 14 h was verified using the samples from 0- to 100-m depths at station A (Yosuke Yamada, The University of Tokyo, personal communication). Killed controls prepared using autoclaved, filtered seawater were run together with the samples and indicated no significant autohydrolysis of the substrate.
The autoclaved, filtered seawater that served as the killed controls and diluent of the MUF-P stock solution was prepared from water collected at 2000 m in the western North Pacific Ocean (15°N, 128°E) in June 2006. The deep seawater was autoclaved at 120°C for 30 min and passed through an ultrafiltration membrane with a nominal molecular-weight cut-off of 5000 Da (Vivaflow 200, Vivascience, Stonehouse, UK).
Estimation of Kinetic Parameters
We used two independent protocols to determine APA kinetic parameters. The single-concentration method using an excess concentration of the substrate (200 μmol L−1) allowed for determination of Vmax. The multiple-concentration method using different substrate concentrations (50–1000 nmol L−1) allowed for estimation of various parameters including L-DOP turnover time, the half-saturation constant, and the in situ hydrolysis rate, as well as Vmax. The ranges of substrate concentrations used in each method are consistent with those used in previous studies as compiled by Sebastián and Niell (2004) and Duhamel et al. (2010).
In the multiple-concentration method, the hydrolysis rate (v) of the added substrate (MUF-P) can be described in terms of the Michaelis–Menten equation as follows:

where Sa is the added substrate concentration, Sn is the concentration of natural substrate (likely equivalent to L-DOP), and Km is the half-saturation constant. We assumed that the natural and added substrates had comparable enzyme reactivity. Additional information is obtained from a form of the Lineweaver–Burk equation, derived by inverting Eq.1 and multiplying both sides by Sa:

The increase in the fraction of added substrate hydrolyzed by APA with time follows an exponential model:

where r is the fraction of added substrate hydrolyzed (dimensionless), t is the incubation time (h), and Ta is the turnover time of the added MUF-P. Ta can therefore be determined from a single time-point measurement (Thingstad et al., 1993), when Eq.3 is solved for Ta:

As Ta is the ratio between the substrate concentration (Sa, nmol L−1) and the hydrolysis rate (v, nmol L−1 h−1), Eq.2 can be rearranged to:

Equation 5 is in the form of y = mx + c, which is the equation of a straight line; a plot of y (i.e., Ta) against x (Sa) has a slope m (1/Vmax). The least squares linear regression of Eq.5 using Ta which is calculated from the experimental data using Eq.4, gives the turnover time of the natural L-DOP (TDOP) as the y-intercept when Sa = 0, and the sum of the half-saturation constant and the natural substrate concentration (Km + Sn) as the absolute value of the x-intercept. We estimated Km by subtracting the L-DOP concentration from Km + Sn.
Calculation and Statistical Analysis
Results are reported as mean ± 1 SD, if available. For statistical analysis, we used the program Sigmastat, included in the Sigmaplot 11 software package (Systat Software, Chicago, IL, USA). We used a significance level of P < 0.05.
Results and Discussion
Study Area Characteristics
Station A was in the NPSG, and station C was located within the Kuroshio Current (Marine Information Service Office, Oceanographic Data Information Division, Hydrographic and Oceanographic Department, Japan Coast Guard, 2010; Figure 1). Station B was likely in the transition zone between the NPSG and the Kuroshio. Salinity at 5 m was between 33.8 and 34.3 at all stations (Figure 2A). The influence of freshwater was negligible even at the coastal station. Water temperature at station H was 23.5°C at the surface and 11.9°C at 200 m. The offshore stations exhibited relatively high temperatures of around 26.9°C at 0 m and 19.5°C at 200 m (Figure 2B). The moderate resolution imaging spectroradiometer (MODIS) chlorophyll image for 19 July 2010 shows a phytoplankton bloom in the coastal area that includes station H, and low phytoplankton biomass in the offshore area (Figure 1). Measured surface Chl a concentrations are consistent with the satellite observations. The surface Chl a concentration of 1.54 μg L−1 at station H was much higher than those at the offshore stations (0.08–0.12 μg L−1; Figure 2C).
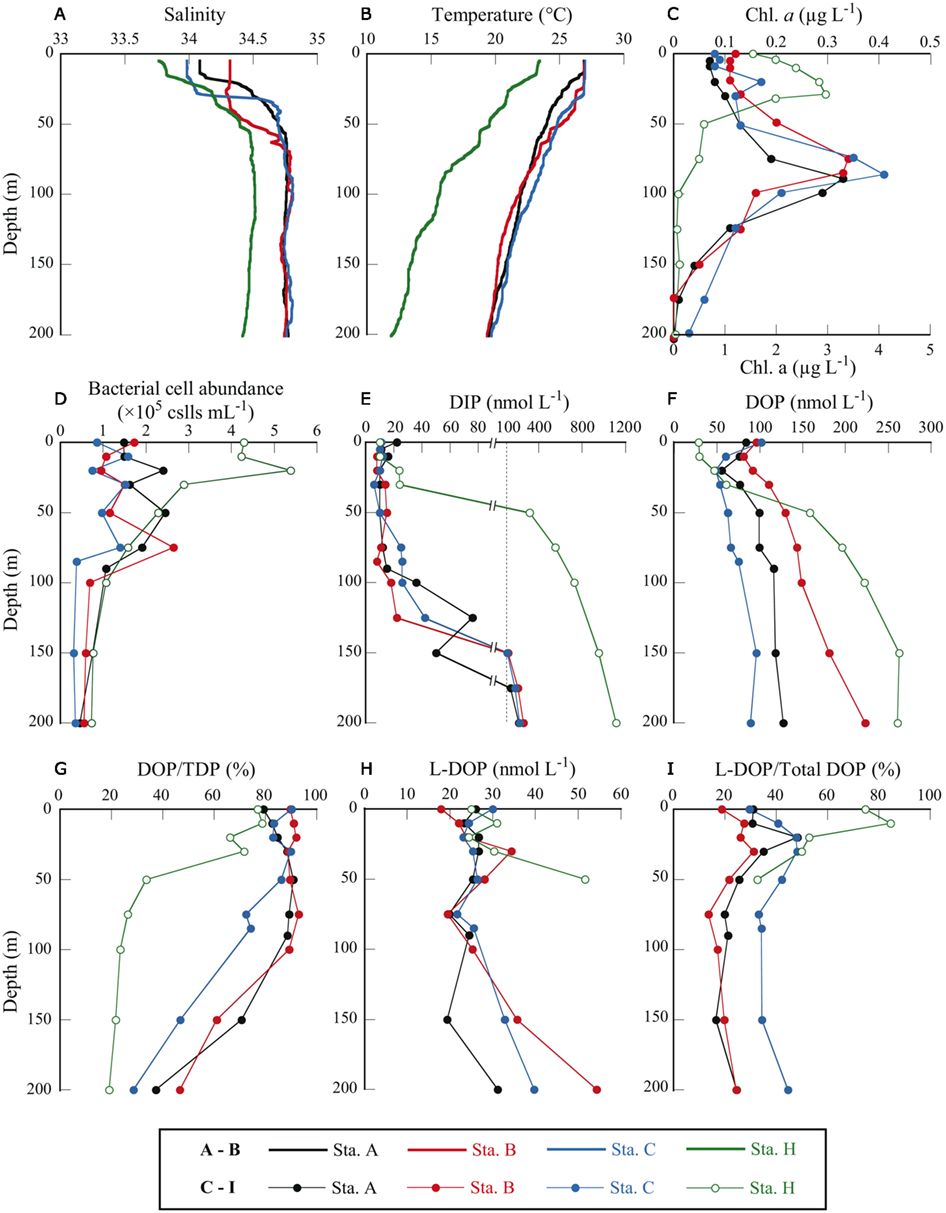
Figure 2. Depth profiles of physical and biological parameters at four sampling stations in the western North Pacific Ocean. (A) salinity, (B) water temperature, (C) Chl a concentration, (D) bacterial cell abundance, (E) DIP concentration, (F) DOP concentration, (G) DOP as a percentage of TDP, (H) L-DOP concentration, and (I) L-DOP as a percentage of total DOP. Note that in (C), Chl a concentrations for station H only are plotted against the bottom axis with maximum value of 5 μg L−1.
The euphotic zone was defined as the upper water column from the surface to the depth at which the photosynthetically active radiation was 1% of the value just below the surface. We could only carry out light-depth observations at station B, where the euphotic zone was estimated as the upper 130 m. From the similarities in the depth profiles and levels of Chl a concentrations among the offshore stations (Figure 2C) we believe that our sampling depth (0–200 m) covered the euphotic zone at these stations. The euphotic zone at station H was likely the upper 30–40 m according to the results of Hashihama et al. (2008), who monitored the depth of light penetration at a station close to station H. The depths of the Chl a maximum layer (CML) at each station are included in Table 1. The CMLs at the offshore stations were at depths between 75 and 90 m, with maximum Chl a concentrations of 0.33–0.41 μg L−1. We observed a much shallower CML depth (30 m) and a Chl a concentration one order of magnitude higher (2.96 μg L−1) at coastal station H.
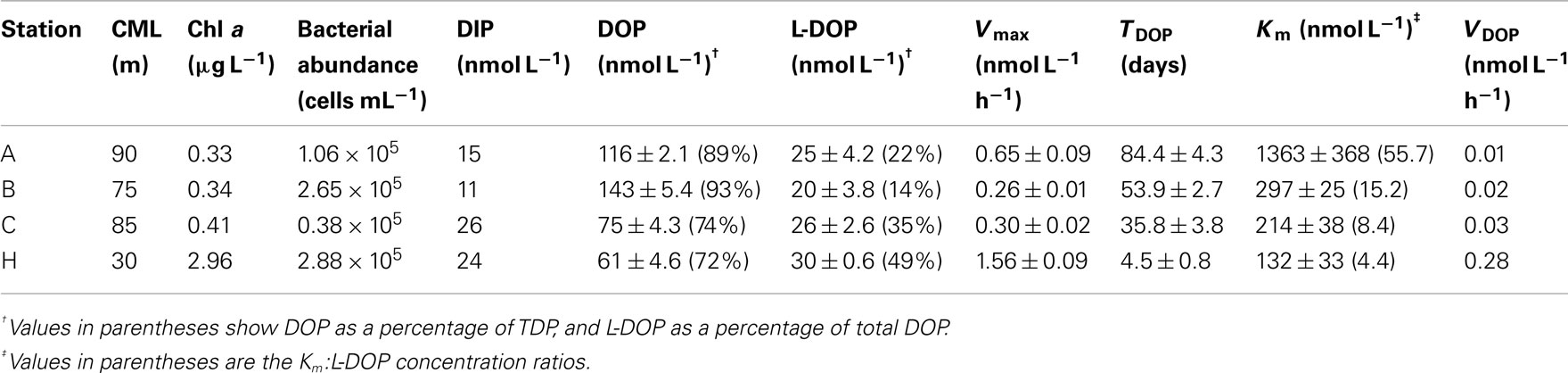
Table 1. Depth of CML, Chl a concentration, bacterial cell abundance, concentrations of P pools, and APA kinetic parameters at the CML at three offshore stations and one coastal station in the western North Pacific Ocean.
Bacterial cell abundance in the surface waters at station H was considerably higher than at the offshore stations (Figure 2D). Cell abundance at station H, however, rapidly decreased with depth and was in the same range as the offshore stations below 30 m. Because we only counted DAPI-stained cells in the “dissolved” fraction (<0.45 μm) and not in the whole-water sample, we did not count bacteria >0.45 μm that were removed from the samples by filtration and presumably represented a considerable proportion of the total bacterial community. Unfortunately, data for total bacterial abundance in the unfiltered seawater samples are unavailable. Only one matched set of data for bacterial cell abundance in both the whole and dissolved fractions is available for the sample collected from 200 m at station H. The bacterial cell abundance in the filtered sample was 0.73 × 105 cell mL−1 and in the whole sample, 1.58 × 105 cell mL−1. In this sample, approximately half (54%) of all bacterial cells were >0.45 μm and were removed by filtration.
DIP and DOP Distribution
At all stations, DIP concentrations were very low in the upper layers (Figure 2E). DIP concentrations at and above the CML were at or below the detection limit for the standard molybdenum blue method (i.e., 20 nmol L−1). The more sensitive method revealed that DIP concentrations in these samples were between 11 and 26 nmol L−1. Although DIP concentrations in the surface samples (0 and 10 m) at station H were below the detection limit of the standard method, we did not apply the sensitive method to station H samples. For the purposes of discussion herein, DIP concentrations in these samples were regarded as 10 nmol L−1, or half the detection limit. This assumed DIP concentration was also used in calculations of DOP and L-DOP concentrations. As a result, estimated DOP and L-DOP concentrations, which were determined from a change in DIP concentrations, must include an error of ±10 nmol L−1 because of the uncertainty in the estimated DIP concentrations.
The DIP concentration in the CML at station H (30 m) was 24 nmol L−1, just above the detection limit. Below the CML, DIP concentrations increased rapidly with depth at all stations. The maximum DIP concentration at each offshore station was 214–255 nmol L−1, observed at 200 m. A more drastic increase in DIP concentration with depth was observed at station H, where the DIP concentration reached 310 nmol L−1 at 50 m and increased to 1100 nmol L−1 at 200 m.
The low DIP concentrations observed in the offshore euphotic zone could partly explain the low Chl a biomass in these areas, although the availability of other nutrients including nitrogen and iron should be considered as well. Even though the Chl a biomass at the coastal station was one order magnitude higher than at the offshore stations, DIP concentrations in the coastal euphotic zone were as low as those at the offshore stations (Figure 2). This implies that the apparent DIP concentration is not the definitive criterion for evaluating P limitation. The kinetic parameters of DIP, including turnover time and uptake rate, can potentially be used to evaluate DIP availability for microbial assemblages (Moutin et al., 2005, 2008; Tanaka et al., 2011). We have no available dataset on the kinetic parameters of DIP around the research areas. However, the substantial difference in biomass observed between the coastal and oceanic environments both under similarly DIP-depleted conditions may indicate that there were potential differences in the rate and extent of microbial DIP cycling.
Dissolved organic P is considered a potential source of bioavailable P under phosphate-depleted conditions. Among the offshore stations, the surface DOP concentration of 84 ± 3 nmol L−1 at station A was significantly lower than those of 96 ± 4 and 102 ± 4 nmol L−1 at stations B and C, respectively (P < 0.05, Student’s t-test, triplicate measurements). The depth profiles of DOP at the offshore stations showed minimum values below the surface (20–30 m) that gradually increased with depth, reaching 89–223 nmol L−1 at 200 m (Figure 2F). At the coastal station, the surface DOP concentration of 34 ± 13 nmol L−1 was considerably lower than those at the offshore stations. However, the concentration increased rapidly with depth, reaching 263 ± 15 nmol L−1 at 150 m.
At all stations, DOP concentrations exceeded DIP concentrations at depths at or above the CML. We estimated the proportional abundance of DOP in the TDP pools (Figure 2G). DOP comprised a major proportion, representing 72.4–91.9% (mean 86.3 ± 5.7%, n = 20) of TDP at or above the CML at the offshore stations. At station H the proportion of DOP was also high above the CML, between 62.0 and 71.7%. The percentage decreased below the CML to 28.6–46.6% at 200 m at the offshore stations and to 33.5% at 50 m at station H. The decrease in the DOP percentage with depth was due to rapid increases in DIP concentrations with depth that greatly exceeded those in DOP concentrations (Figures 2E,F). The high proportion of DOP in the dissolved P pools emphasizes its potential role in sustaining productivity in the euphotic zone under phosphate-depleted conditions. We examined this potential role by determining the bioavailability and cycling of DOP through remineralization by APA.
L-DOP Concentration and Proportion of DOP
For partial characterization and evaluation of the DOP fraction that can be easily utilized by microorganisms, we estimated L-DOP, defined as that hydrolyzable to phosphate by APA. The concentration of L-DOP was determined as the increase in DIP concentration after incubation with the enzyme. Most samples showed a significant increase in DIP concentration (Student’s t-test, P < 0.05 from three replicate analyses). However, in the samples collected from deep layers at station H (≥75 m), the L-DOP concentration could not be determined because there was a decrease in DIP concentration after incubation instead of an increase. The estimated L-DOP concentrations were 52 ± 7 nmol L−1 at 50 m and 0 ± 2 nmol L−1 at 75 m at Station H, but at 200 m, the calculations yielded an estimate of −87 ± 4 nmol L−1. These depths correspond to those with exceptionally high DIP concentrations (>550 nmol L−1) compared with other stations or with the shallower depths at Station H (Figure 2E). As phosphate competes with L-DOP for the active sites of AP, this could explain why there was low or no hydrolysis of L-DOP if the DIP concentration was very high. However, competition by DIP is not enough to explain the considerable negative estimates for L-DOP. Although the mechanism is unknown, it is possible that high DIP concentrations produce inconsistencies in L-DOP measurements. Therefore, it was necessary to ignore the L-DOP data from the deep samples (≥75 m) at station H.
Concentrations of L-DOP at and above the CLM were relatively invariant among depths and locations (Figure 2H) and slightly exceeded the ambient DIP concentrations. L-DOP concentrations in the euphotic zone at the oligotrophic offshore stations were 18–34 nmol L−1, comparable to 24–31 nmol L−1 at coastal station H. Moutin et al. (2008) and Duhamel et al. (2010) found that L-DOP was below the detection limits of 20 and 5 nmol L−1 for their respective DIP analytical methods in the Pacific Subtropical Gyres. In their studies, they used the procedures of AP treatment of the conventional method for determination of L-DOP proposed by Strickland and Parsons (1972). The higher L-DOP concentrations found in our study could be caused by the difference in procedures for L-DOP estimation. For the method used in the present study, sodium azide was added to minimize biological interference during incubation. In a radio-labeled phosphate incorporation experiment, it was shown that the addition of azide effectively inhibited biotic DIP uptake (Lebo, 1990). Feuillade and Dorioz (1992), in developing an assay for L-DOP in sediments, found greater DIP liberation by enzymatic hydrolysis with the addition of azide than without the inhibitor. Furthermore, the enzymatically liberated DIP concentration reached a plateau in the presence of the inhibitor, whereas the concentration decreased after 5 h incubation without the inhibitor. Enhancement of DIP liberation by AP treatment with the addition of azide has also been demonstrated for L-DOP determination in oceanic seawater samples (Hashihama et al., submitted). These results strongly suggest that L-DOP assays in samples with potentially high DIP uptake activity should include some controls on biological activity.
The other major differences between the methods in this and previous studies are the sources and concentrations of the enzymes, which could result in different efficiencies of hydrolysis. We used bovine intestinal mucosa AP at a final concentration of 5 units mL−1 instead of Escherichia coli AP at 0.004–0.02 units mL−1 in the previous studies. Thus, the concentration of enzyme in our assay was 250–1250 times that in the conventional method. In tests for hydrolysis efficiency of DOP analogs, the hydrolysis of adenosine 5′-triphosphate (ATP) increased from 70% in the conventional method to 100% in the present method (Suzumura et al., 1998; Hashihama et al., submitted). ATP alone might be a negligible component of oceanic DOP pools, as it was found to comprise less than 1% of total DOP in open ocean environments (Björkman and Karl, 2005). However, it is possible that a significant fraction of marine DOP pools consists of other organic P compounds that would be hydrolyzed less efficiently under low enzyme concentration.
Although the addition of the azide inhibitor and increases in the enzyme concentration seemed to enhance the recovery of L-DOP in seawater, there is room for criticism of a method for L-DOP determination based on the addition of a single enzyme (Duhamel et al., 2011). In natural marine environments, DOP is not hydrolyzed by one enzyme from a single source – here E. coli or bovine intestinal mucosa – but by an assortment of enzymes from various eukaryotic and prokaryotic microorganisms. Furthermore, different species, and even a single bacterial species, can produce a number of enzymes having different biochemical properties and roles (Chichester et al., 2008). The use of multiple enzymes in combination lead to higher recovery of DIP, even from broad classes of organic P compounds, compared to single enzymes (Feuillade and Dorioz, 1992; Suzumura et al., 1998; Turner et al., 2002). More accurate estimates of true L-DOP concentration require further research to characterize the roles of enzymes and the properties of DOP compounds.
Although L-DOP concentrations in the euphotic zone were relatively constant, there was a marked difference between stations in the proportional abundance of L-DOP in the total DOP pool. The shapes of the depth profiles of L-DOP as a percentage of total DOP at the three offshore stations were similar, with subsurface maxima of 31–48% around 20–30 m and relatively constant values below 50 m (Figure 2I). The percent L-DOP values at station C were about 1.5 times those at stations A and B throughout the depths sampled. The mean values over the depths sampled were 28 ± 10, 22 ± 6, and 39 ± 7% at stations A, B, and C, respectively.
Although the proportional abundances of L-DOP below 10 m at station H were comparable to those at the offshore stations, the values at 0 and 10 m were considerably higher, accounting for 75 and 85% of the total DOP, respectively. Here, the possible errors involved in L-DOP determination must be carefully considered in those samples where DIP concentrations were below the detection limit and assumed to be 10 nmol L−1. Including the potential error of ±10 nmol L−1 in the DIP determination, the L-DOP percentage at station H was recalculated as 64–80% at 0 m and 79–88% at 10 m. These percentages are still quite high compared with those at the offshore stations.
Comparison of Single- and Multiple-Concentration Methods for Vmax Determination
The abundance of L-DOP is a function of both its production and utilization by the ambient microorganisms in seawater. It is difficult to measure the DOP production rate in natural environments. We therefore evaluated APA kinetics to derive the important parameters of DOP utilization by marine microorganisms.
In this study, Vmax of APA was estimated by two different methods: the single-concentration method, wherein the hydrolysis rate was measured with a single, excessively high concentration of the substrate (200 μmol L−1), and the multiple-concentration method, using kinetic calculations from measurements of the hydrolysis rate over a range of lower substrate concentrations from 50 to 1000 nmol L−1. Figure 3A shows a plot of the data from the multiple-concentration method for the samples from the CML at each station, and the relationship between the measured hydrolysis rates and substrate concentrations. Non-linear least squares regression of these data based on the Michaelis–Menten equation (Eq. 1) showed good correlation in all samples [r2 > 0.845, P < 0.01, n = 10 (duplicate measurements for each of five concentrations)]. Using Eq.4, we calculated Ta from the experimental data for the hydrolyzed proportion of added MUF-P in the multiple-concentration approach. Then by linear regression analysis using Eq.5, we estimated Vmax as the inverse of the slope of the regression line for Ta vs. substrate concentration (Figure 3B).
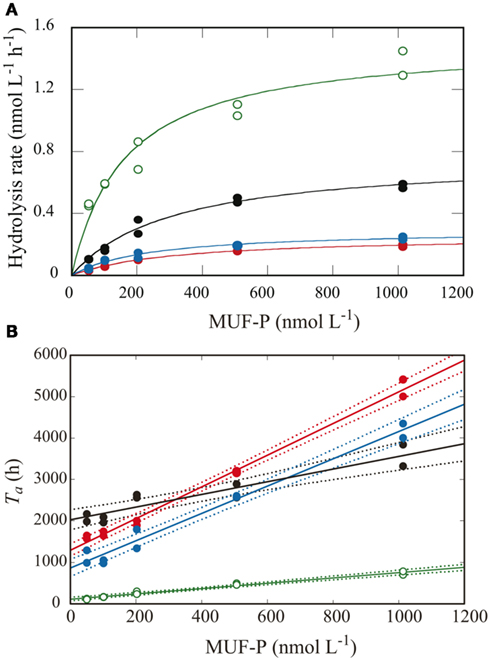
Figure 3. Kinetic analysis of APA using the multiple-concentration method in samples from the CML at each station. Lines and symbols are the same as in Figure 2. (A) APA as a function of added MUF-P concentration using Michaelis–Menten kinetics. (B) Turnover time of the added MUF-P as a function of added MUF-P concentration, with the fitted regression lines (solid) and 95% confidential intervals (dotted lines).
The two methods yielded consistent results for the offshore samples (Figure 4). This result implies that the Michaelis–Menten model adequately described APA over a wide range of substrate concentrations, from the in situ level of 50 nmol L−1 to the extremely high concentration of 200 μmol L−1. In contrast, we observed considerable differences between the results from the two methods at the coastal station. At station H, Vmax obtained by the single-concentration method was one order of magnitude higher than that by the multiple-concentration method. For example, Vmax in the surface water at this station was estimated as 29.7 ± 0.9 nmol L−1 h−1 by the single-concentration method and 3.1 ± 0.3 nmol L−1 h−1 by the multiple-concentration method. As suggested by Sebastián et al. (2004), who also observed considerable differences in APA at different substrate concentrations, the discrepancy between the two methods can be explained by multiphasic APA kinetics. At the offshore stations, APA seemed to be driven by a predominance of microorganisms producing AP with identical or very similar kinetic properties, whereas at station H, mixed and abundant microbial populations might produce a variety of enzymes with different kinetic properties, depending on the range of substrate concentrations.
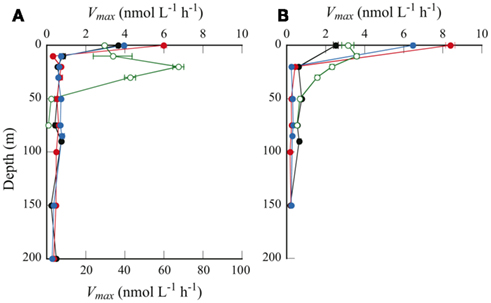
Figure 4. Depth profiles of Vmax as determined by (A) the single-concentration method, and (B) the multiple-concentration method. Symbols are same as in Figure 2. Note that in (A), Vmax only for station H is plotted against the bottom axis with maximum value of 100 nmol L−1 h−1.
Previous studies also found multiphasic kinetics of AP or other hydrolytic enzymes in marine phytoplankton and bacterial assemblages (e.g., Rivkin and Swift, 1980; Tholosan et al., 1999; Dyhrman and Palenik, 2003). Sebastián and Niell (2004) concluded that complete kinetic experiments should be carried out using multiple-concentration methods, because the use of single additions of excess substrate could give erroneous results. Our results support their observations, and therefore we also recommend the use of multiple-concentration methods for determining Vmax. Considering the time-consuming procedures involved, it could be difficult to thoroughly apply multiple-concentration methods on board. It might be more practical to use both methods jointly – the single-concentration method for most samples and the multiple-concentration method for selected samples.
Considering the greater biomass at station H, that is, Chl a concentrations higher by one order of magnitude and bacterial abundance several times higher than at the offshore stations (Figures 2C,D), the higher Vmax obtained by the single-concentration method is plausible. Nevertheless, the addition of substrate at concentrations four orders of magnitude higher than the ambient DOP concentration seems to cause changes in APA kinetics in some environments that are not likely under in situ conditions. The use of substrate concentrations comparable to those of the ambient L-DOP in the multiple-concentration method is preferable, as they reflect the in situ condition. Even though the single-concentration method can show the maximum potential rate of APA, in this study we used the Vmax values obtained by the multiple-concentration method for all subsequent calculations.
Distribution of APA Kinetic Parameters
A distinguishing feature of the depth profiles of Vmax is the remarkably high values at the sea surface at the offshore stations (Figure 4). We found no such uniquely high values for any other parameters in the surface samples, including DIP, DOP, L-DOP, and Chl a concentrations, or bacterial cell abundance (Figure 2), nor was the dissolved organic carbon concentration unusually high (Hiroshi Ogawa, per. comm.). However, enzymatic peptide hydrolysis is reportedly enhanced in the sea surface microlayer (Kuznetsova and Lee, 2001). A similar enhancement in APA in the surface microlayer could explain the markedly higher Vmax at the sea surface observed in this study.
Vmax in the euphotic zone of the offshore stations (20–150 m) was 0.18–0.77 nmol L−1 h−1. A higher Vmax (1.6–3.6 nmol L−1 h−1) was observed in the euphotic zone at coastal station H (10–30 m). Previous studies have shown that APA varies over a wide range, even in similarly DIP-depleted waters (summarized by Duhamel et al., 2010). Values consistent with the present study were reported from widely separated geographic locations; for example, in the subtropical central North Pacific Ocean (Koike and Nagata, 1997), the Sargasso Sea (Cotner et al., 1997), and the Mediterranean Sea (Van Wambeke et al., 2002). Some studies have reported remarkably high values for Vmax: 28 ± 5 nmol L−1 h−1 in the Bay of Villefranche (Thingstad et al., 1998), and up to 500 nmol L−1 h−1 in the southern Baltic Sea (Nausch, 1998). In contrast, relatively low values have also been observed; around 0.10 nmol L−1 h−1 in surface samples from the NPSG and the central South Pacific subtropical gyre (Duhamel et al., 2011).
From linear regression analysis of the data from the multiple-concentration assay (Figure 3B), we estimated TDOP and Km + L-DOP as the y-intercept and the absolute value of the x-intercepts, respectively. TDOP in the surface samples from stations B and C was low enough that the intercept of the fitted line was not significantly different from zero (P > 0.05). Consequently, we could not estimate a significant Km from these samples. Depth profiles of TDOP (Figure 5A) show an increasing trend with depth. At the offshore stations, TDOP at 20 m was between 16.4 and 35.5 days, and increased to 49.1–101 days at 150 m. At station H, we found a remarkably low TDOP, increasing gradually from 1.6 days at the surface to 4.5 days at the CML. TDOP then increased sharply to 43.5 days at 50 m, a value comparable to those at the offshore stations.
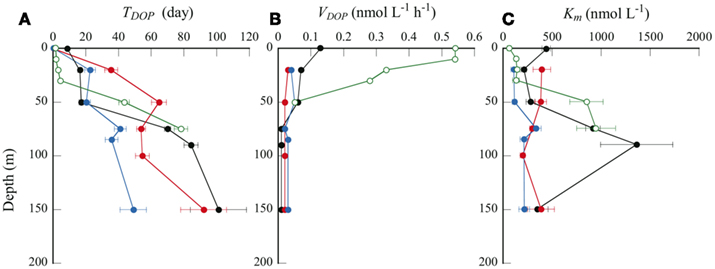
Figure 5. Depth profiles of (A) TDOP, (B) VDOP, and (C) Km. Symbols are same as in Figure 2.
We estimated in situ DOP hydrolysis rates (VDOP) by dividing the L-DOP concentration by TDOP in the corresponding sample (Figure 5B). We could not calculate VDOP below 50 m at station H because of the lack of L-DOP concentration data for the reasons stated above. VDOP was consistently low over the depths sampled at the offshore stations; from 0.01 to 0.07 nmol L−1 h−1. In contrast, VDOP was high and variable at station H. A considerably high VDOP of 0.55 nmol L−1 h−1 was observed at 10 m, decreasing rapidly to 0.28 nmol L−1 h−1 at 30 m, and reaching 0.05 nmol L−1 h−1 at 50 m. Duhamel et al. (2011) estimated VDOP in surface seawaters from the North and South subtropical Pacific Ocean, comparable with those in this study. Although their estimates were maximum potential rates, assuming that the ambient DOP was fully AP-hydrolyzable, VDOP was between 0.005 and 0.20 nmol L−1 h−1 in low biomass, oligotrophic stations and 0.72 nmol L−1 h−1 at one station within a phytoplankton bloom.
Factors Controlling APA and the DOP Cycle
We performed Spearman’s rank-order correlation analysis between seawater chemical and biological variables including concentrations of DIP, DOP and Chl a, and bacteria cell abundance, and APA kinetic parameters including Vmax, TDOP, Km, and VDOP, for data from 20 to 150 m in the euphotic zone at offshore stations A, B, and C (n = 15; Table 2). There were no significant correlations between Chl a and the kinetic parameters, whereas a significant positive correlation was found between bacterial cell abundance and Vmax (Figure 6A). Also, there was a significant negative correlation between DIP concentration and Vmax (Figure 6B).

Table 2. Correlation coefficients (ρ) from Spearman’s rank-order correlation analysis between chemical and biological variables of seawater and APA kinetic parameters in the phosphate-depleted euphotic zone (20–150 m) of offshore stations A, B, and C in the western North Pacific Ocean (n = 15).
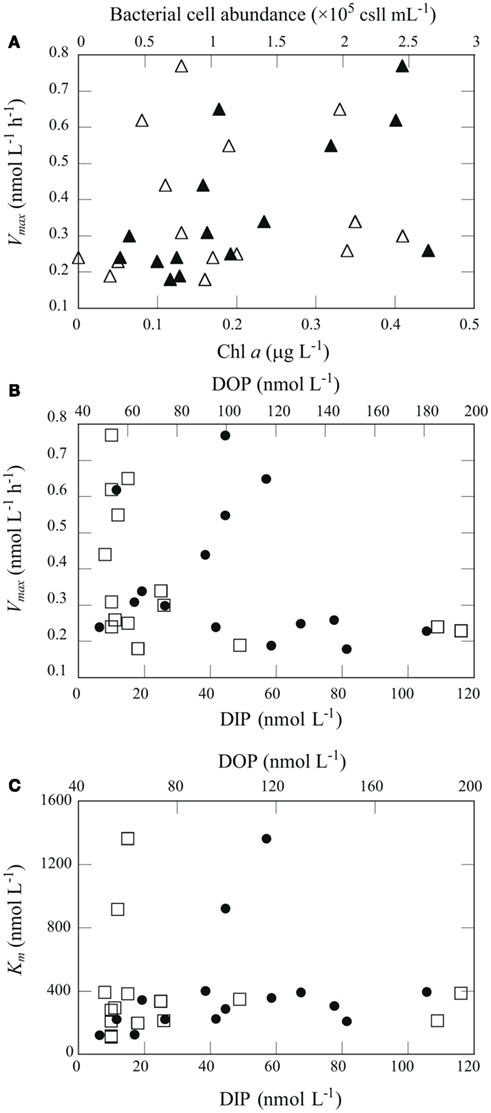
Figure 6. Relationships between chemical and biological variables in seawater and APA kinetic parameters. (A) Relationship between Vmax and Chl a concentration (open triangles) and bacterial abundance (filled triangles). (B) Relationship between Vmax and DIP (open squares) or DOP (filled circles). (C) Relationship between Km and DIP (open squares) or DOP (filled circles).
AP activity is considered to be regulated by the degree of P stress or P limitation, which is largely dependent on phosphate availability. Nausch (1998) also reported a significant negative correlation between Vmax and DIP concentration in the Baltic Sea. Similar but non-significant trends between Vmax and DIP concentration have been found in many studies. For example, Dyhrman and Ruttenberg (2006) reported that APA normalized to Chl a concentration was elevated in conjunction with the lowest DIP concentration off the northern Oregon (USA) coast, and mesocosm and laboratory experiments suggest that excess DIP can suppress APA (Tanaka et al., 2006; Duhamel et al., 2010). APA regulation by DIP has been shown to follow an inverse hyperbolic relationship rather than a linear relationship, with a transition from high to low activity occurring at DIP concentrations of 10–100 nmol L−1 (Ammerman, 1991; Labry et al., 2005; Lomas et al., 2010). In this study, the threshold DIP concentration for the transition from high to low APA was found around 20 nmol L−1 (Figure 6B). Taken together, these results indicate that APA in the oligotrophic oceanic environments studied here was derived primarily from bacterial activity and regulated largely by DIP availability.
Km is a measure of a substrate’s affinity for an enzyme. Km was relatively constant with depth at stations B and C, ranging from 111 to 395 nmol L−1 (Figure 5C). We found substantially higher Km values at 75–90 m at station A (916–1360 nmol L−1) and at 50 m at station H (850 nmol L−1). We found a significant correlation between Km and DOP concentration in the euphotic zone at the offshore stations (Table 2; Figure 6C). Duhamel et al. (2011) investigated the relationships between APA parameters and dissolved P pools in the North and South Pacific Subtropical Gyres and found that Km in the south Pacific Subtropical Gyre increased with DIP and DOP concentrations.
A high value of Km indicates a low affinity for the enzyme, meaning that higher substrate concentrations are required for the hydrolysis rate to approach Vmax. In all samples studied here, Km was higher than the ambient total and L-DOP concentrations. We obtained the mean Km:L-DOP ratio of 10.2 ± 4.7 (n = 17), with particularly high values of 46–56 in 75–90 m layers at station A. This result indicates that microbial assemblages had a relatively low affinity for the ambient L-DOP. However, this result also implies that the microbial assemblages had the potential to maximize their APA at higher L-DOP concentrations. A high Km relative to the ambient DOP concentrations has often been found in oligotrophic environments such as in the Atlantic subtropical gyres (Mather et al., 2008). Duhamel et al. (2011) also found relatively high Km in the Pacific subtropical gyres and suggested that microorganisms were prepared to benefit from intermittent pulses of bioavailable DOP. In the present study, the Km:L-DOP ratios at the CML decreased substantially from 55.7 at station A in the NPSG to 4.4 at coastal station H (Table 1). We have no direct information on DOP production rates in the study areas; nevertheless, it is reasonable to expect a greater and more constant supply of fresh dissolved organic matter including DOP to ambient waters from the considerably greater biomass in the coastal area. Considerable changes in Km likely reflect adaptation of the microbes to changes in substrate supply in each environment. Our results suggest that the microorganisms in each environment studied had adapted by adjusting their APA metabolisms to potential pulsed or constitutive inputs of available DOP. It is suggested also that the different types of microorganisms responsible for APA dominate microbial communities in each region, which produce the different types of enzymes of adapting to the ambient L-DOP availability.
As shown in Table 1, the geographic variations in the stocks of dissolved P pools at the CML were relatively small compared to those in the APA kinetic parameters. Concentrations of DIP and DOP varied within the relatively narrow ranges of 11–26 and 61–143 nmol L−1, respectively. In contrast, there were relatively large variations in TDOP and VDOP of 4.4–84.4 days and 0.01–0.28 nmol L−1 h−1, respectively, as well as those in the Km:L-DOP ratios discussed above. This suggests that despite the apparent invariability in the size of dissolved P pools, the P cycle in the coastal ecosystem was much more dynamic than those in the oligotrophic oceanic environments. In particular, the observed high VDOP and low TDOP show that a large proportion of L-DOP at the coastal station was hydrolyzed and recycled rapidly compared to the offshore areas. Because we have no data for DIP kinetic parameters such as the uptake rate or turnover time in the study areas, we cannot directly evaluate the relative contribution of bioavailable P supplied through L-DOP hydrolysis by APA to the microbial P requirement. In order to evaluate the significance of APA in the P cycle, Duhamel et al. (2011) calculated the daily fraction of DIP potentially released by APA as the ratio of VDOP to the DIP concentration, expressed as a percentage of the ambient DIP pool (VDOP:DIP, % days−1). They found that this daily proportion represented 2.3 ± 2.6% days−1 of the DIP measured in the oligotrophic surface waters in the NPSG, excluding a station within a bloom that had an extremely high value of 63.2% days−1. Our results are consistent with those of Duhamel et al. (2011). We found relatively low values of 1.9, 3.3, and 2.8% days−1 at the CML at offshore stations A, B, and C, respectively, and a typically high value of 27.9% days−1 at coastal station H. The low VDOP:DIP values suggested that AP hydrolysis of DOP might be a minor process to provide for P requirements. However, the DIP concentration does not necessarily reflect the concentration of bioavailable phosphate (Tanaka et al., 2006; Moutin et al., 2008), which also may explain the low VDOP:DIP values observed.
Conclusion
This study highlights substantial differences in euphotic zone P cycles between the low-P/low biomass open ocean and low-P/high-biomass coastal ecosystems. Concentrations of DIP and L-DOP were low at both offshore and coastal stations. This suggests that the apparent pools of bioavailable P are not the crucial factors behind the considerable differences in the chlorophyll and bacterial biomass between the ecosystems. Measuring APA kinetic parameters, we showed that DOP remineralization rates were higher in the high-biomass coastal area than in the open ocean environment. Despite the apparent utilization of DOP, the VDOP:DIP values (27.9% days−1) suggest other potential P sources could be important, such as luxury uptake and accumulation of P in cells of phytoplankton and bacteria during P-replete conditions (e.g., Thingstad, 2005).
Because we measured APA in the bulk fraction of seawater samples, our results reveal the bulk community response under the given conditions. However, the contribution and response of AP derived from various sources, including eukaryotic phytoplankton, heterotrophic and autotrophic prokaryotes, and dissolved enzymes as well, must differ greatly among geographic locations and environmental conditions (Labry et al., 2005; Duhamel et al., 2010, 2011). Enzyme-labeled fluorescence assays have identified and quantified cell-specific APA of prokaryotic and eukaryotic cells in marine microbial assemblages (González-Gil et al., 1998; Dyhrman et al., 2002; Dyhrman and Ruttenberg, 2006; Duhamel et al., 2010). Further studies using cellular-level analyses as well as bulk community responses are needed to understand the functions of APA in marine microbial ecosystems. Furthermore, there have been new insights into the significance of bacterial intracellular APA, which occurs in the cytoplasm and cannot be quantified using common substrates such as MUF-P (Luo et al., 2009; White, 2009). Further research into the environmental factors and physiological metabolisms controlling production and utilization of DOP is crucial for increasing our understanding of the P cycle and its contribution to marine productivity.
Conflict of Interest Statement
The authors declare that the research was conducted in the absence of any commercial or financial relationships that could be construed as a potential conflict of interest.
Acknowledgments
We thank Hiroshi Ogawa (Atmosphere and Ocean Research Institute, The University of Tokyo), chief scientist of cruise KT10-13 on R/V Tansei-maru (Japan Agency for Marine-Earth Science and Technology). We also appreciate the efforts of the captain and crew during the cruise. This manuscript benefited from thoughtful review comments from M. W. Lomas and M. Sebastián. This work was supported by a Grant-in-Aid for Scientific Research on Priority Areas: “Western Pacific Air-Sea Interaction Study (W-PASS)” (no. 18067007). This is a contribution to the Surface Ocean Lower Atmosphere Study (SOLAS) Core Project of the International Geosphere-Biosphere Programme (IGBP).
References
Ammerman, J. B. (1991). “Role of ecto-phosphohydrolases in phosphorus regeneration in estuarine and coastal ecosystems,” in Microbial Enzymes in Aquatic Environments, ed. R. J. Chrost (New York: Springer-Verlag), 165–186.
Björkman, K. M., and Karl, D. M. (1994). Bioavailability of inorganic and organic phosphorus compounds to natural assemblages of microorganisms in Hawaiian coastal waters. Mar. Ecol. Prog. Ser. 111, 265–273.
Björkman, K. M., and Karl, D. M. (2003). Bioavailability of dissolved organic phosphorus in the euphotic zone at station ALOHA, North Pacific subtropical gyre. Limnol. Oceanogr. 48, 1049–1057.
Björkman, K. M., and Karl, D. M. (2005). Presence of dissolved nucleotides in the North Pacific Subtropical Gyre and their role in cycling of dissolved organic phosphorus. Aquat. Microb. Ecol. 39, 193–203.
Cavender-Bares, K. K., Karl, D. M., and Chisholm, S. W. (2001). Nutrient gradients in the western North Atlantic Ocean: relationship to microbial community structure and comparison to patterns in the Pacific Ocean. Deep Sea Res. Part I Oceanogr. Res. Pap. 48, 2373–2395.
Cembella, A. D., Antia, N. J., and Harrison, P. J. (1984). The utilization of inorganic and organic phosphorus-compounds as nutrients by eukaryotic microalgae – a multidisciplinary perspective. Part 1. Crit. Rev. Microbiol. 10, 317–391.
Chichester, K. D., Sebastián, M., Ammerman, J. W., and Colyer, C. L. (2008). Enzymatic assay of marine bacterial phosphatases by capillary electrophoresis with laser-induced fluorescence detection. Electrophoresis 29, 3810–3816.
Cotner, J. B., Ammerman, J. W., Peele, E. R., and Bentzen, E. (1997). Phosphorus-limited bacterio-plankton growth in the Sargasso Sea. Aquat. Microb. Ecol. 13, 141–149.
Duhamel, S., Björkman, K. M., Van Wambeke, F., Moutin, T., and Karl, D. M. (2011). Characterization of alkaline phosphatase activity in the North and South Pacific subtropical gyres: implications for phosphorus cycling. Limnol. Oceanogr. 56, 1244–1254.
Duhamel, S., Dyhrman, S. T., and Karl, D. M. (2010). Alkaline phosphatase activity and regulation in the North Pacific subtropical gyre. Limnol. Oceanogr. 55, 1414–1425.
Dyhrman, S. T., Ammerman, J. W., and Van Mooy, B. A. S. (2007). Microbes and the marine phosphorus cycle. Oceanography 20, 110–116.
Dyhrman, S. T., and Palenik, B. (2003). Characterization of ectoenzyme activity and phosphate-regulated proteins in the coccolithophorid Emiliania huxleyi. J. Plankton Res. 25, 1215–1225.
Dyhrman, S. T., and Ruttenberg, K. C. (2006). Presence and regulation of alkaline phosphatase activity in eukaryotic phytoplankton from the coastal ocean: implications for dissolved organic phosphorus remineralization. Limnol. Oceanogr. 51, 1381–1390.
Dyhrman, S. T., Webb, E. A., Anderson, D. M., Moffett, J. W., and Waterbury, J. B. (2002). Cell-specific detection of phosphorus stress in Trichodesmium from the Western North Atlantic. Limnol. Oceanogr. 47, 1832–1836.
Feuillade, M., and Dorioz, J. M. (1992). Enzymatic release of phosphate in sediments of various origins. Water Res. 26, 1195–1201.
González-Gil, S., Keafer, B. A., Jovine, R. V. M., Aguilera, A., Lu, S., and Anderson, D. M. (1998). Detection and quantification of alkaline phosphatase in single cells of phosphorus-starved marine phytoplankton. Mar. Ecol. Prog. Ser. 164, 21–35.
Hansen, H. P., and Koroleff, F. (1999). “Determination of nutrients,” in Methods of Seawater Analysis, eds K. Grasshoff, K. Lremling, and M. Ehrhardt (Weinheim: Wiley-VCH), 159–228.
Hashihama, F., Furuya, K., Kitajima, S., Takeda, S., Takemura, T., and Kanda, J. (2009). Macro-scale exhaustion of surface phosphate by dinitrogen fixation in the western North Pacific. Geophys. Res. Lett. 36, L03610.
Hashihama, F., Horimoto, N., Kanda, J., Furuya, K., Ishimaru, T., and Saino, T. (2008). Temporal variation in phytoplankton composition related to water mass properties in the central part of Sagami Bay. J. Oceanogr. 64, 23–37.
Hoppe, H.-G. (1983). Significance of exoenzymatic activities in the ecology of brackish water: measurements by means of methylumbelliferyl-substrates. Mar. Ecol. Prog. Ser. 11, 299–308.
Hoppe, H.-G., and Ullrich, S. (1999). Profiles of ectoenzymes in the Indian Ocean: phenomena of phosphatase activity in the mesopelagic zone. Aquat. Microb. Ecol. 19, 139–148.
Karl, D. M. (2007). “The marine phosphorus cycle,” in Manual of Environmental Microbiology, ed. C. J. Hurst (Washington, DC: ASM Press), 523–539.
Karl, D. M., and Björkman, K. M. (2002). “Dynamics of DOP,” in Biogeochemistry of Marine Dissolved Organic Matter, eds D. A. Hansell and C. A. Carlson (Boston, MA: Elsevier Science), 249–366.
Karl, D. M., and Tien, G. (1997). Temporal variability in dissolved phosphorus concentrations in the subtropical North Pacific Ocean. Mar. Chem. 56, 77–96.
Koike, I., and Nagata, T. (1997). High potential activity of extracellular alkaline phosphatase in deep waters of the central Pacific. Deep Sea Res. Part II Top. Stud. Oceanogr. 44, 2283–2294.
Kolowith, L. C., Ingall, E. D., and Benner, R. (2001). Composition and cycling of marine organic phosphorus. Limnol. Oceanogr. 46, 309–320.
Kuznetsova, M., and Lee, C. (2001). Enhanced extracellular enzymatic peptide hydrolysis in the sea-surface microlayer. Mar. Chem. 73, 319–332.
Labry, C., Delmas, D., and Herbland, A. (2005). Phytoplankton and bacterial alkaline phosphatase activities in relation to phosphate and DOP availability within the Gironde plume waters (Bay of Biscay). J. Exp. Mar. Biol. Ecol. 318, 213–225.
Lomas, M. W., Burke, A. L., Lomas, D. A., Bell, D. W., Shen, C., Dyhrman, S. T., and Ammerman, J. W. (2010). Sargasso Sea phosphorus biogeochemistry: an important role fordissolved organic phosphorus (DOP). Biogeosciences 7, 695–710.
Luo, H., Benner, R., Long, R. A., and Hu, J. (2009). Subcellular localization of marine bacterial alkaline phosphatases. Proc. Natl. Acad. Sci. U.S.A. 160, 21219–21233.
(2010). Quick Bulletin of Ocean Conditions No. 132. Available at: http://www1.kaiho.mlit.go.jp/KANKYO/KAIYO/qboc/2010cal/Jul/20100715.html
Mather, R., Reynolds, S., Wolff, G., Williams, R. G., Torres-Valdes, S., Woodward, E. M. S., Landolfi, A., Pan, X., Sanders, R. W., and Achterberg, E. (2008). Phosphorus cycling in the North and South Atlantic Ocean subtropical gyres. Nat. Geosci. 1, 439–443.
Moutin, T., Karl, D. M., Duhamel, S., Rimmelin, P., Raimbault, P., Van Mooy, B. A. S., and Claustre, H. (2008). Phosphate availability and the ultimate control of new nitrogen input by nitrogen fixation in the tropical Pacific Ocean. Biogeosciences 5, 95–109.
Moutin, T., Van Den Broeck, N., Beker, B., Dupouy, C., Rimmelin, P., and Le Bouteiller, A. (2005). Phosphate availability controls Trichodesmium spp. biomass in the SW Pacific Ocean. Mar. Ecol. Prog. Ser. 297, 15–21.
Nausch, M. (1998). Alkaline phosphatase activities and the relationship to inorganic phosphate in the Pomeranian Bight (southern Baltic Sea). Aquat. Microb. Ecol. 16, 87–94.
Orrett, K., and Karl, D. M. (1987). Dissolved organic phosphorus production in surface seawaters. Limnol. Oceanogr. 32, 383–395.
Porter, K. G., and Feig, Y. S. (1980). The use of DAPI for identifying and counting aquatic microflora. Limnol. Oceanogr. 25, 943–948.
Ridal, J. J., and Moore, R. M. (1990). A re-examination of the measurement of dissolved organic phosphorus in seawater. Mar. Chem. 29, 19–31.
Rivkin, R., and Swift, E. (1980). Characterization of alkaline phosphatase and organic phosphorous utilization in the oceanic dinoflagellate, Pyrocystis noctiluca. Mar. Biol. 61, 1–8.
Sebastián, M., Arístegui, J., Montero, M. F., and Niell, F. X. (2004). Kinetics of alkaline phosphatase activity, and effect of phosphate enrichment: a case study in the NW African upwelling region. Mar. Ecol. Prog. Ser. 270, 1–13.
Sebastián, M., and Niell, F. X. (2004). Alkaline phosphatase activity in marine oligotrophic environments: implications of single-substrate addition assays for potential activity estimations. Mar. Ecol. Prog. Ser. 277, 285–290.
Strickland, J. D. H., and Parsons, T. R. (1972). A Practical Handbook of Seawater Analysis, 2nd Edn. Ottawa: Fisheries Research Board of Canada.
Suzuki, R., and Ishimaru, T. (1990). An improved method for the determination of phytoplankton chlorophyll using N, N-dimethylformamide. J. Oceanogr. 46, 190–194.
Suzumura, M., and Ingall, E. D. (2004). Distribution and dynamics of various forms of phosphorus in seawater: insights from field observations in the Pacific Ocean and a laboratory experiment. Deep Sea Res. Part I Oceanogr. Res. Pap. 51, 113–1,130.
Suzumura, M., Ishikawa, K., and Ogawa, H. (1998). Characterization of dissolved organic phosphorus in coastal seawater using ultrafiltration and phosphohydrolytic enzymes. Limnol. Oceanogr. 43, 1553–1564.
Tanaka, T., Henriksen, P., Lignell, R., Olli, K., Seppälä, J., Tamminen, T., and Thingstad, T. F. (2006). Specific affinity for phosphate uptake and specific alkaline phosphatase activity as diagnostic tools for detecting phosphorus-limited phytoplankton and bacteria. Estuaries Coast 29, 1226–1241.
Tanaka, T., Thingstad, T. F., Christaki, U., Colombet, J., Cornet-Barthaux, V., Courties, C., Grattepanche, J.-D., Lagaria, A., Nedoma, J., Oriol, L., Psarra, S., Pujo-Pay, M., and Van Wambeke, F. (2011). Lack of P-limitation of phytoplankton and heterotrophic prokaryotes in surface waters of three anticyclonic eddies in the stratified Mediterranean Sea. Biogeosciences 8, 525–538.
Thingstad, T. F. (2005). Simulating the response to phosphate additions in the oligotrophic eastern Mediterranean using an idealized four-member microbial food web model. Deep Sea Res. Part II Top. Stud. Oceanogr. 52, 3074–3089.
Thingstad, T. F., Krom, M. D., Mantoura, R. F. C., Flaten, G. A. F., Groom, S., Herut, B., Kress, N., Law, C. S., Pasternak, A., Pitta, P., Psarra, S., Rassoulzadegan, F., Tanaka, T., Tselepides, A., Wassmann, P., Woodward, E. M. S., Wexels Riser, C., Zodiatis, G., and Zohary, T. (2005). Nature of phosphorus limitation in the ultraoligotrophic eastern Mediterranean. Science 309, 1068–1071.
Thingstad, T. F., Skojoldal, E. F., and Bohne, R. A. (1993). Phosphorus cycling and algal–bacterial competition in Sandsfjord, western Norway. Mar. Ecol. Prog. Ser. 99, 239–259.
Thingstad, T. F., Zweifel, U. L., and Rassoulzadegan, F. (1998). P limitation of heterotrophic bacteria and phytoplankton in the northwest Mediterranean. Limnol. Oceanogr. 43, 88–94.
Tholosan, O., Lamy, F., Garcin, J., Polychronaki, Y., and Bianchi, A. (1999). Biphasic extracellular proteolytic enzyme activity in benthic water and sediment in the northwestern Mediterranean Sea. Appl. Environ. Microbiol. 65, 1619–1626.
Turner, B. L., McKelvie, I. D., and Haygarth, P. M. (2002). Characterisation of water-extractable soil organic phosphorus by phosphatase hydrolysis. Soil Biol. Biochem. 34, 27–35.
Van Wambeke, F., Christaki, U., Giannakourou, A., Moutin, T., and Souvemerzoglou, K. (2002). Longitudinal and vertical trends of bacterial limitation by phosphorus and carbon in the Mediterranean Sea. Microb. Ecol. 43, 119–133.
White, A. E. (2009). New insights into bacterial acquisition of phosphorus in the surface ocean. Proc. Natl. Acad. Sci. U.S.A. 106, 21013–21014.
Wu, J., Sunda, W., Boyle, E., and Karl, D. (2000). Phosphate depletion in the western North Atlantic Ocean. Science 289, 759–762.
Keywords: phosphorus, alkaline phosphatase activity, dissolved organic phosphorus, dissolved inorganic phosphorus, north Pacific Ocean, euphotic zone
Citation: Suzumura M, Hashihama F, Yamada N and Kinouchi S (2012) Dissolved phosphorus pools and alkaline phosphatase activity in the euphotic zone of the western North Pacific Ocean. Front. Microbio. 3:99. doi: 10.3389/fmicb.2012.00099
Received: 01 December 2011; Accepted: 28 February 2012;
Published online: 19 March 2012.
Edited by:
Sonya Dyhrman, Woods Hole Oceanographic Institution, USAReviewed by:
Michael William Lomas, Bermuda Institute of Ocean Sciences, BermudaMarta Sebastian, CSIC, Spain
Copyright: © 2012 Suzumura, Hashihama, Yamada and Kinouchi. This is an open-access article distributed under the terms of the Creative Commons Attribution Non Commercial License, which permits non-commercial use, distribution, and reproduction in other forums, provided the original authors and source are credited.
*Correspondence: Masahiro Suzumura, National Institute of Advanced Industrial Science and Technology, AIST Tsukuba West, 16-1 Onogawa, Tsukuba 305-8569, Japan. e-mail: suzumura@ni.aist.go.jp