- 1 Environmental and Life Sciences Graduate Program, Trent University, Peterborough, ON, Canada
- 2 Chemistry Department, Trent University, Peterborough, ON, Canada
- 3 Water Quality Center, Trent University, Peterborough, ON, Canada
Dissolved organic matter (DOM) is a universal part of all aquatic systems that largely originates with the decay of plant and animal tissue. Its polyelectrolytic and heterogeneous characters make it an effective metal-complexing agent with highly diverse characteristics. Microbes utilize DOM as a source of nutrients and energy and their enzymatic activity may change its composition, thereby altering the bioavailability and toxicity of metals. This study investigated the impacts of microbial inoculation upon the optical and copper-binding properties of freshly produced leaf-litter leachate over 168 h. Copper speciation was measured using voltammetry, and using fluorescence quenching analysis of independent fluorophores determined using parallel factor analysis (PARAFAC). Two protein/polyphenol-like and two fulvic/humic-like components were detected. Thirty-five percent of total protein/polyphenol-like fluorescence was removed after 168-h of exposure to riverine microbes. The microbial humic-like and tryptophan-like PARAFAC components retained significantly different log K values after 168 h of incubation (p < 0.05), while their complexing capacities were similar. Using voltammetry, a sixfold increase in copper-complexing capacity (CC, from 130 to 770 μmol Cu g C−1) was observed over the exposure period, while the conditional binding constant (log K) decreased from 7.2 to 5.8. Overall binding parameters determined using voltammetry and fluorescence quenching were in agreement. However, the electrochemically based binding strength was significantly greater than that exhibited by any of the PARAFAC components, which may be due to the impact of non-fluorescent DOM, or differences in the concentration ranges of metals analyzed (i.e., different analytical windows). It was concluded that the microbial metabolization of maple leaf leachate has a significant impact upon DOM composition and its copper-binding characteristics.
Introduction
Dissolved organic matter (DOM) is a ubiquitous, complex, and polymorphous mixture of molecules that originates chiefly from the degradation of plant and animal matter. At the point of production, this molecular soup includes proteins, carbohydrates, polyphenols, and other vital compounds, many of which are quickly metabolized by microbes (Sutton and Sposito, 2005). Selective microbial metabolization of labile DOM components changes its overall chemical character (Fellman et al., 2008), which may affect its reactivity and environmental functioning. In particular, since DOM controls the mobility, speciation, and therefore toxicity of metals (Guéguen and Dominik, 2003; Nogueira et al., 2009), microbial processing may alter metal-binding properties, and thereby change the level of toxicity.
Leaf litter is a readily available source of DOM, has an important impact throughout freshwater systems, and serves as a key source of nutrients and energy for microorganisms (Tank et al., 2010). Given its abundance, importance, and the ease and reproducibility of leaching DOM from leaf litter, it is an excellent candidate for studying microbially mediated changes in the metal-binding characteristics of DOM. Copper, an essential metal that can also be toxic at higher concentrations, has been widely employed as a representative for metal behavior with respect to DOM-binding properties (Ružic, 1982; Ryan and Weber, 1982; Perdue and Lytle, 1983; Tipping, 1998; Manceau and Matynia, 2010).
Anodic stripping voltammetry (ASV) is a very sensitive method for determining the key metal-binding parameters involved in copper-DOM speciation, such as the conditional equilibrium constant or binding strength (log K), and metal-complexing capacity (CC), which may be derived from the equilibrium relationship between bound and unbound sites:
where [M] and [L] are the concentrations of free copper and ligand, respectively, and [ML] is the concentration of bound ligand. CC and K may be obtained from the linearized titration curve (Ružic, 1982; Durán and Nieto, 2011):
Fluorescence spectroscopy permits the three-dimensional mapping of the DOM complex into excitation–emission matrices (EEMs), revealing multiple fluorophore groups that are related to specific classes of molecules, and therefore potentially correspond to different binding sites (Ohno and Bro, 2006). Leaf leachate is highly fluorescent in regions related to proteins and polyphenols, and this fluorescence is quenched during the electrostatic interaction involved in metal binding (Ryan and Weber, 1982). Thus, the metal-binding characteristics determined using ASV and the single-site model (1:1 metal:DOM stoichiometry; Ružic, 1982) may also be determined for distinct fluorescent sites. Further, the proportion of fluorescence that is quenched by binding (% f) at each site may be determined, which may differ according to site properties. Parallel factor analysis (PARAFAC; Stedmon et al., 2003) of EEMs resolves fluorophore groups into statistically independent components, so that binding-site identification is more precise (Yamashita and Jaffé, 2008). Site-specific binding information also facilitates the incorporation of competition for metals among binding sites (Smith and Kramer, 1998), which is a likely occurrence in the complex mixture of molecules in leaf-litter leachate. Hence, fluorescence quenching using PARAFAC extracts site-specific metal-binding characteristics, and increases the information yield obtained by ASV.
In this study, fresh DOM was leached from leaf litter, inoculated with microbes, and analyzed for its optical and copper-binding properties over the course of 7 days of incubation using ASV and fluorescence quenching with PARAFAC. The unique combination of quenching with EEM–PARAFAC and ASV conducted under similar matrix conditions (i.e., pH and ionic strength) exploits the strength of both methods, permitting deeper insight into the metal-binding characteristics of DOM. This approach provides unprecedented information about the metal-binding properties of fluorescing DOM, as well as the bulk DOM leached directly from leaves.
Materials and Methods
Initial Sampling/Storage
Leaf litter
Sugar maple (Acer saccharum) leaves were sampled from trees on the Trent University campus (Latitude 44.36°N, Longitude 78.29°W) in Peterborough, Ontario. Tree species identity was confirmed by a federally certified expert (Andrew McDonough, Trent University). Samples were taken on August 26th, 2011 by shaking the lower branches of two mature (∼70″ height) trees and gathering freshly fallen litter, and by collecting litter dislodged by wind. Litter was air-dried, frozen, and prepared following the procedure used previously.
Microbial inocula
Naturally occurring microbes were collected from the Otonabee River (Peterborough, ON, Canada) using a pre-combusted, acid-washed, 500-mL amber-glass bottle. The sample was immediately transported to the laboratory, filtered through a 5-μm nitrocellulose filter (Millipore), and separated into 10.0-mL portions. Microbes used in experiment LL1 were stored overnight in the refrigerator (≤5°C), while the inoculum for LL2 was frozen to minimize adaptive disruptions to community structure over the longer storage period (≤−5°C; Koponen et al., 2006) in 15-mL acid-washed polypropylene centrifuge tubes. The latter was moved to the refrigerator 7 days prior to use to allow a return to normal levels of respiration (Feng et al., 2007).
Leaching, Inoculation, Sampling, and Fluorescence
Leaching and organic carbon
Litter was leached by placing whole leaves in a 250-mL Pyrex beaker and filling with 200 mL milli-Q water (MQW, ≤18 MΩ cm−1; Millipore). Two leaching experiments were conducted (LL1 and LL2), using 10 and 7 leaves (5.2 and 3.7 g wet weight, respectively). Leaf cleaning and leachate consistency were achieved by decanting the leachate and refreshing it with fresh MQW after 1 and 3 h of leaching, and using the complete volume from the 5-h leachates for the experiment. Five-hour leachates were consecutively filtered through Millipore pre-combusted glass-fiber (0.7 μm) and nitrocellulose (0.22 μm) filters that were pre-rinsed with MQW and leachate (respectively) to remove loose fibers and minimize the introduction of nitrogen. Prior to adding microbes directly to the leachate in a 250-mL Pyrex beaker, samples were adjusted to pH 7 using NaOH (Sigma).
Since leaching and initial biodegradation in Southern Ontario take place mainly in the late fall and early spring when temperatures oscillate between −5 and 10°C, the leaching, storage, and inoculation conditions were completed at 5°C to reflect natural spring and autumn conditions in southern Ontario. Similarly, freezing constitutes a normal stress upon the structure of the microbial community (Koponen et al., 2006).
Inoculation and sampling
Immediately after inoculation, a sample (B0) was taken to evaluate the immediate effects of microbial addition. The inoculated leachate was then stored in the dark at 5°C. Samples (Bi) were taken from the active leachate at i = 24, 48, 96, and 168 h after inoculation. In experiment LL1, additional dissolved organic carbon (DOC) analyses were conducted after 2.5 and 4 h, and a control sample (i.e., non-inoculated leachate) was stored at 5°C in a pre-combusted, amber-glass vial for the duration of the experiment. Biological controls (i.e., NaN3) were not added to the control, as they have been found to affect optical properties and cause undesirable reactions (Scully et al., 2004). The DOC concentration of all samples was measured using a TOC analyzer (Shimadzu TOC-VCPH). Samples were filtered (0.22-μm nitrocellulose; Millipore) and stored at 5°C in pre-combusted amber-glass vials prior to EEM, quenching, and voltammetric analyses, all of which took place within 7 days.
Fluorescence and PARAFAC
Fluorescence was analyzed following manual injection into the stopped-flow cell of an on-line fluorescence detector (Agilent 1200-series model G1321A). MQW was used to clean the cell between injections, ensured by monitoring continuous scanning channels at excitation/emission wavelengths (Ex/Em) of 270, 300, 355, 370/460 nm. Injections of 0.01 M NaCl (pH 6) were periodically analyzed between samples to convert fluorescence to Raman units (r.u.) using the area under the Raman water peak at Ex = 350 nm (r.u.; Lawaetz and Stedmon, 2009). Wavelengths of the on-line fluorescence detector were calibrated daily following the manufacturer-recommended procedure.
Excitation–emission matrices were captured in ratio (S/R) mode by scanning over an excitation (Ex) and emission range (Em) of 200–450 and 280–600 nm in 1 and 5 nm increments, respectively. Fluorescence EEMs were measured for three distinct subsamples of each leachate for each sample Bi from both LL1 and LL2, and once for each quenched sample (LL2 only). Fourfold dilution of all leachate samples ensured that absorbance was low enough to avoid inner filtering effects (A254 < 0.01A.U. in a 1-cm cell, Lakowicz, 2006). Blank EEMs (0.01 M NaCl; pH = 6) were subtracted from each sample EEM to remove background fluorescence. PARAFAC analysis was conducted after Raman normalization, blank subtraction, and removal of Raman and Rayleigh scatter lines in MATLAB R2010a using an in-house modified EEMCut algorithm and the DOMFluor toolbox (Stedmon and Bro, 2008).
Speciation Analyses
Quenching
A 2.5-mM Cu2+ copper stock solution was made in 0.001 M NaCl solution (pH 6) using hydrated cupric nitrate (Baker), and was diluted to 0.5 mM Cu2+ on each day immediately prior to use. Samples were diluted fourfold with 0.001 M NaCl/0.1 M MES (pH = 6) prior to analysis to ensure that A254 < 0.01 AU. Copper was added to 14 individual samples for each Bi from experiment LL2 with final copper concentrations ranging from 2.4 to 320 μM (Figure A1 in Appendix). Quenched samples were stored overnight at 5°C in the dark to ensure equilibration of the complexation reaction.
Fluorescence quenching was described using the multi-site Ryan-Weber model following Smith and Kramer(1998, 2000). The Ryan-Weber model derives a non-linear regression equation from the equilibrium equation for the formation constant of the bound metal (Ryan and Weber, 1982), assuming a single binding site. The Smith and Kramer model accounts for multiple sites, incorporates competition among binding sites, and assumes that fluorescence is a linear function of metal concentration. A 95% CI was calculated for K and CC at each binding site and sampling time by fitting quenching curves with the maxima and minima of the 95% CI for each point, as determined from triplicate EEMs of each Bi (Statistica 8; StatSoft).
Voltammetry
A 0.5-mM Cu2+ stock solution was prepared by diluting copper reference standard solution (1000 ppm, >99.0%; Fisher) in 0.01 M NaNO3/0.1 M MES (SigmaUltra) adjusted to pH 6.0. Samples were diluted 10-fold to <5 ppm C with 0.01 M NaNO3/0.1 M MES (pH = 6) prior to analysis to minimize interferences caused by the adsorption of DOM on the electrode surface. Copper was added to 14 individual samples for each Bi from experiment LL2 with final concentrations ranging from 0.32 to 5.2 μM (Figure A2 in Appendix).
Square wave ASV analysis of copper speciation was conducted on samples from LL2 using a 663 VA polarographic stand (Metrohm) coupled to an Eco-Chemie AutoLab PGSTAT10 running in static mercury drop mode. The analytical procedure followed Durán and Nieto (2011), with the following instrument settings: accumulation potential, −1.1 V; accumulation time, 2 min.; equilibration time, 20 s; scan range, −1.1 to 0.2 V at 25 Hz, an amplitude of 25 mV and a 2-mV scan increment. The automatic stirrer was set to maximum during the purging and accumulation steps. The peak current at a potential of 0.056 V was measured for 14 metal additions along each voltammetric curve.
The conditional stability constant K and complexation capacity CC were determined for each sample Bi by fitting the linear portion of plots of free copper concentration against the ratio of free to bound copper, and assuming single-site Langmuirian adsorption with a 1:1 stoichiometry (Ružic, 1982; Durán and Nieto, 2011). The SD of the voltammetric procedure was determined using three distinct subsamples of B168. The initial copper concentration of the leachate solution was found to be negligible (1.13 ± 0.04 ppb; ±SD) following analysis by inductively coupled mass spectrophotometer (XSeries II, Thermo Fisher).
Results
Dissolved Organic Carbon
A twofold difference in DOC concentration was found in the 5-h leachates between the two experiments (2.3 mg C g−1 litter−1 vs. 1.2 mg C g−1 litter−1 for LL1 and LL2, respectively; Table 1). No significant change in DOC concentration was observed due to the introduction of microbes in LL2 (p > 0.05). Unfortunately, no DOC data were available for LL1 B0. Since the DOC concentration of the leachate was not significantly different from that of the pre-inoculated sample, it is referred to as initial.
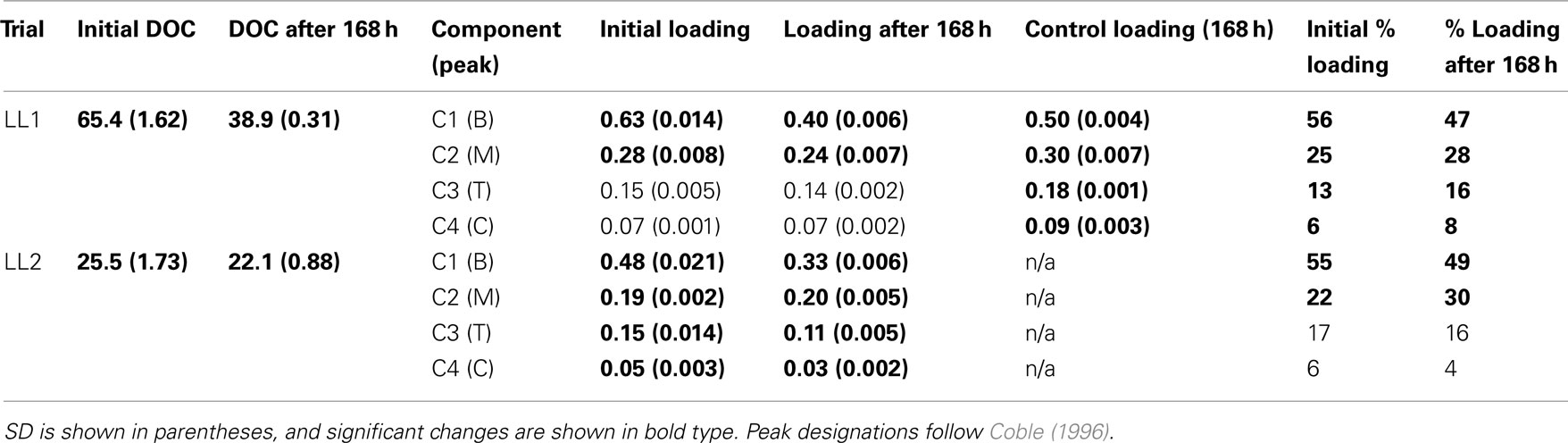
Table 1. DOC concentrations (ppm) and PARAFAC loadings of leachate samples over 168 h of degradation by river microbes (n = 3).
Dissolved organic carbon losses over incubation were well-described using the recalcitrant-labile exponential decay model (Scully et al., 2004):
where R and L denote the recalcitrant and labile compartments of DOC, respectively, and b is the rate of change in h−1 (Figure 1). DOC decreased very rapidly in both experiments, showing more than 95% of overall losses within the first 6 h. The modeled proportions of labile carbon and loss rates were 37%, 0.59 h−1, and 13%, 2.6 h−1 for LL1 and LL2, respectively. Differences in the rates of DOC loss may have been caused by freezing the microbes prior to experiment LL2, or by differences in the concentrations of leachates. A 20% decrease in DOC concentration (from 65.4 to 52.4 mg L−1) was observed in the control sample over 7 days of storage in experiment LL1.
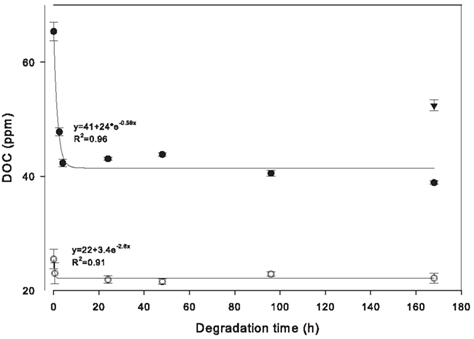
Figure 1. Leaching curves for LL1 (•) and LL2 (◦) and the final concentration of the leachate control sample for LL1 (▼). Error bars show 95% CIs.
Fluorescence Properties
PARAFAC
The initial PARAFAC dataset included 189 EEMs, and one outlier was excluded during preliminary analysis (i.e., B48 + 190 μM Cu). Since the outlier constituted only 1 of 14 titration points at the tail of the quenching curve, it is unlikely that the results were seriously affected.
A six-component PARAFAC model was cross-validated, which identified four components (Figure 2) previously associated with DOM from fresh and marine waters (Coble, 1996; Stedmon and Markager, 2005), and with leaf leachates (Wickland et al., 2007; Wong and Williams, 2010). Component 1 (C1, Ex/Em = 220, 280/320 nm) resembled tyrosine/polyphenol-like peak B; component 2 (C2, 210, 315/430 nm) was similar to humic/carbohydrate-like peak M; C3 (225, 275/350 nm) was similar to tryptophan/polyphenol-like peak T; and C4 (235, 380/465 nm) resembled fulvic-like peak C. In all EEMs, the loading of C1 was dominant.
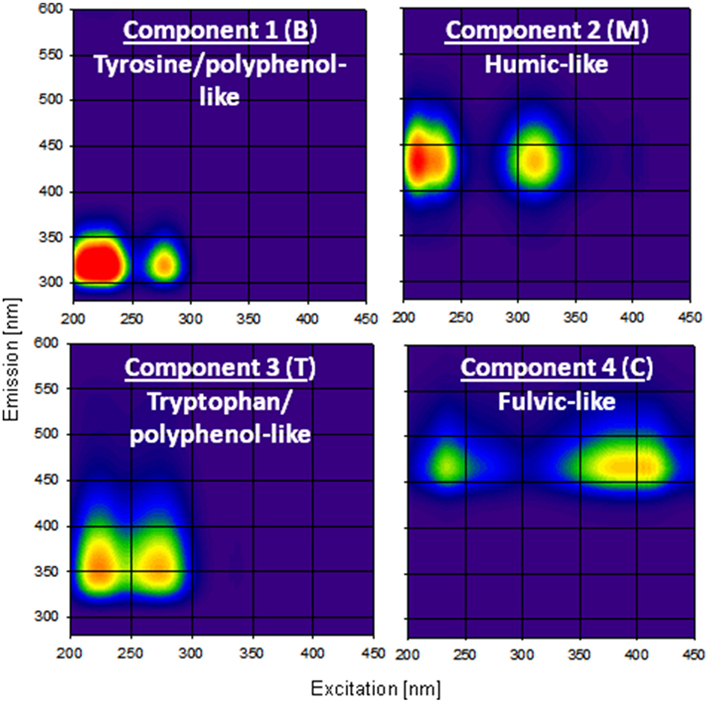
Figure 2. PARAFAC components found in this study and their associated identities following Coble (1996).
The two additional components had sharply defined excitation wavelengths and emission spectra that spanned all wavelengths (Ex/Em = 205/370 and 220/320 nm), and were associated with the nitrate added to the quenching samples (Stewart and Wetzel, 1980). Samples containing nitrate-related components (42%) were removed from the data set and a second PARAFAC analysis was completed. The second analysis was cross-validated for four components, which were identical to the non-anomalous components from the first analysis in shape, loadings, and order of importance. Consequently, all EEMs were included in the PARAFAC model, and the components associated with nitrate were ignored.
Changes after inoculation
As with DOC losses over incubation (Figure 1), changes in PARAFAC loadings were best described using the recalcitrant-labile exponential decay model (Figure 3). In general, the loadings of all PARAFAC components either decreased or remained the same after 168 h in both experiments, with the exception of a slight increase in C2 in experiment LL2 (Table 1). However, as a proportion of overall loading only the changes to C1 and C2 were significant in LL2 (p < 0.05). The proportions of all components were quite similar in both experiments, both before and after inoculation. Despite high variability in DOC losses over the two experiments, the changes in total component loading were similar to those of DOC, with an average (±SD) loading reduction of 23.9 ± 1.37%, and an average DOC loss of 26.9 ± 19.2%.
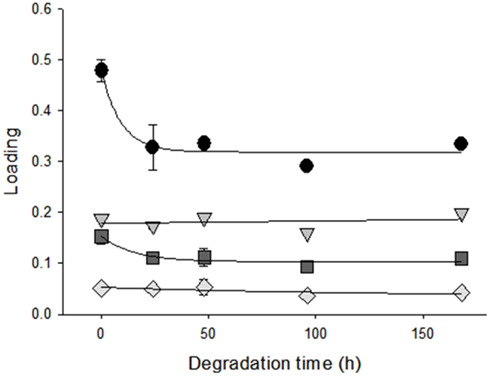
Figure 3. Changes in average PARAFAC component loadings [C1 (•), C2 (▼), C3 (■), and C4 (♦)] over the microbial incubation period during experiment LL2. Error bars (± SD) that are not apparent are smaller than their respective symbols.
To assess the reproducibility of the experiment in terms of biodegradable DOM, component loadings were partitioned into two groups (protein/polyphenol-like and humic/fulvic-like), which have been respectively associated with relatively labile and recalcitrant DOM (Stedmon and Markager, 2005; Fellman et al., 2008, 2009). The average protein/polyphenol-like loading (C1 + C3) was calculated for each sample, and fit with the recalcitrant-labile exponential model (Eq. 3; Figure 4A). The relatively recalcitrant fraction (i.e., that remaining after incubation; Eq. 3) of the protein/polyphenol-like fluorescence constituted 64 and 66% of total protein/polyphenol-like fluorescence for LL1 and LL2, respectively, so that the portion lost over the incubation period was considered to be more labile (p < 0.0005 for both experiments). To assess the corresponding impact on overall DOM quality, the combined protein/polyphenol-like (C1 + C3) and humic/fulvic-like (C2 + C4) loadings were converted to proportional loadings [e.g., (C1 + C3)/ΣC1–4], and transformed to ensure normality of the distribution (arcsin–square root; Figure 4B). Statistically identical linear decreases in the proportion of protein-like components were found for LL1 and LL2 (p > 0.05). The corresponding loss rates (i.e., slopes) and original protein/polyphenol concentrations (i.e., intercepts) were 0.04% h−1 and 69.9%, and 0.03% h−1 and 69.0% of DOM for LL1 and LL2, respectively (Figure 4B).
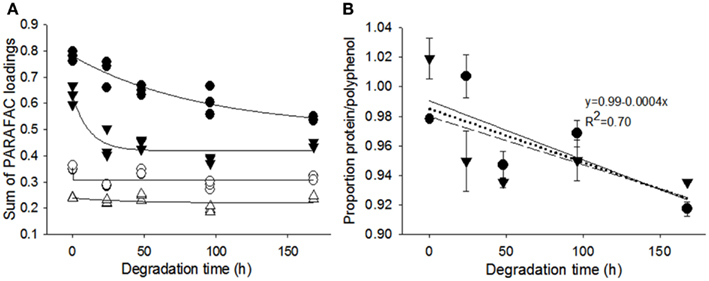
Figure 4. Changes in (A) PARAFAC component loadings and (B) mean proportions (arcsin-square root transformed) of grouped protein/polyphenol- (filled) and humic/fulvic-like constituents (open) for experiments LL1 (•) and LL2 (▼) over the course of degradation. The regression equation is for the overall mean proportion of protein/polyphenol-like loadings for both experiments (dotted line). Some error bars (±SD) are smaller than their respective symbols.
Compared to Bo, changes in the control sample mirrored those observed over the microbial exposure period, but were decidedly less severe. The DOC concentration and PARAFAC loadings for components C1–4 in the control sample were 52.4 mg L−1, and 0.50 ± 0.004, 0.30 ± 0.007, 0.18 ± 0.001, and 0.09 ± 0.003, respectively. These changes correspond to percentage losses of 19.8% DOC and 12.6% total loading, respectively (Table 1).
Quenching
Quenching behaviors and binding characteristics differed by PARAFAC component and over the period of microbial exposure for all components, generally changing from significantly different (p < 0.05) to more similar (Figures 5A–C; Table 2). The log K and CC values of all components were significantly different from each other (by component) in B0, and both parameters were significantly different for each component in B0 compared to B168 (p < 0.05; Table 2).
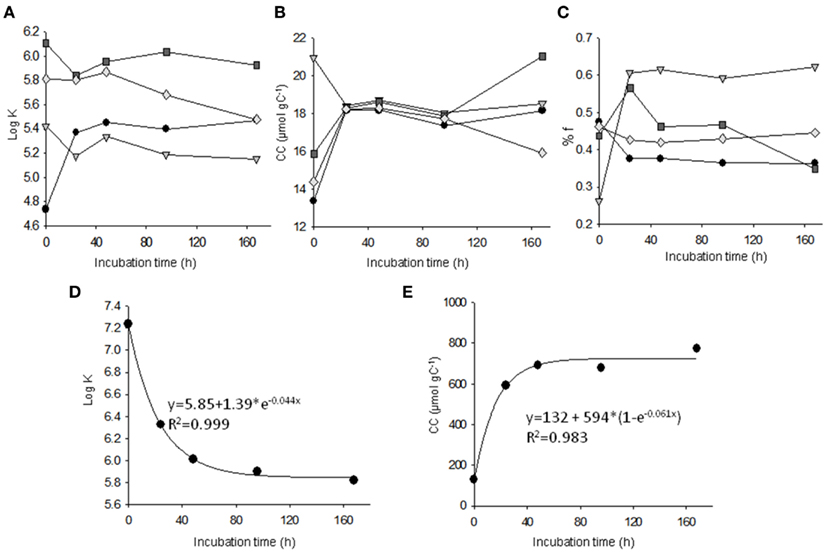
Figure 5. Changes in binding parameters after incubation determined by fluorescence quenching for (A–C) PARAFAC components C1 (•), C2 (▼), C3 (■), and C4 (♦) and (D,E) voltammetry.
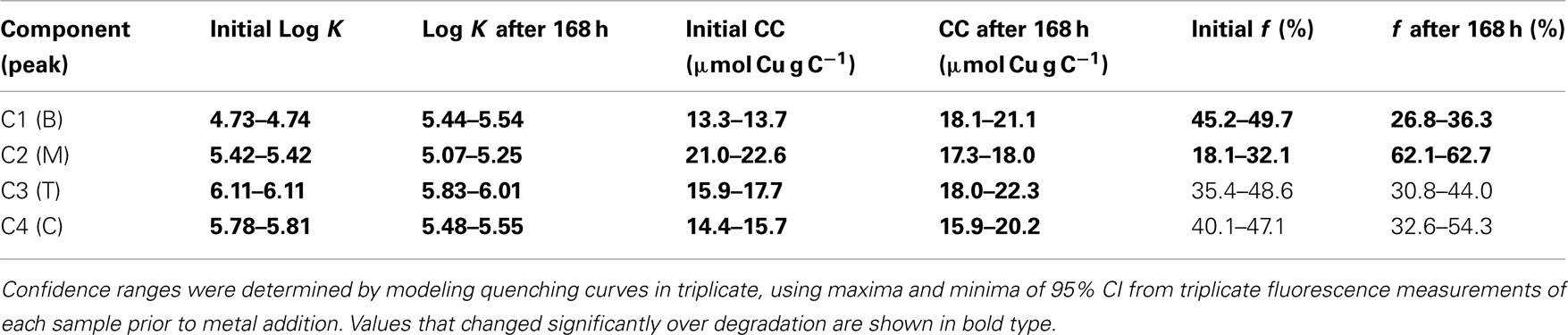
Table 2. Confidence ranges (95%) for conditional binding constant (log K), complexing capacity (CC), and proportion of fluorescence quenched (% f) determined by the fluorescence quenching of leaf-litter leachate over 168 h of degradation by microbes.
In the leachate, protein/polyphenol-like C1 visibly quenched more slowly than other components and had a significantly lower log K value (4.73 vs. 5.42–6.11). However, the differences in log K were significantly reduced after inoculation, corresponding with an increase in CC from 13.3 to 18.2 μmol Cu g C−1 (Figures 5A,B) and a decrease in the proportion of fluorescence quenched by binding (% f) from 47.5 to 31.6% (Figure 5C). For C2 the opposite trends were apparent, with decreases observed in log K and CC (from 5.42 to 5.15 and from 21.0 to 18.5 μmol Cu g C−1, respectively) and a 2.4-fold increase in % f (from 26.1 to 62.3%). The patterns of change for CC and % f in C1 and C2 closely resembled the exponential curves observed for DOC and fluorescence, while only the log K of C1 underwent exponential-type change (Figures 2, 5A). The log K values of C3 and C4 were also significantly lower after 168 h of leaching, whereas their CC values were significantly higher (p < 0.05). Exponential patterns of change were observed for CC and % f. However, no significant differences in % f were observed between B0 and B168 for C3 or C4 (Table 2).
Voltammetry
Binding parameters (log K and CC) determined by voltammetry exhibited significant, exponential change after inoculation (from 7.2 to 5.82 and from 130 to 770 μmol Cu g C−1, respectively; p < 0.005), and the rates of change were very similar for both parameters (0.044 and 0.061, respectively; p > 0.05; Figures 5D,E).
Discussion
Biodegradation
Changes in the leaf-litter leachate occurred at an extremely rapid rate (exponential loss rates of 0.59 and 2.6 h−1 for DOC in LL1 and LL2, respectively) and a significant difference was observed for all PARAFAC components between both experiments, before and after inoculation (p < 0.05). The multiple consistent sampling points observed along the tail of exponential curves imply that the early stages of decomposition have reached a relatively steady state. However, only protein/polyphenol-like component C1 decreased in its overall average proportion (from 56 to 47% of total loading). The proportional increase in C2 (from 22 to 30%; Table 1) was relatively high in LL2, suggesting that the net effect of microbial action may have been the production of C2 and consumption of C1. Although the loadings of components C3 and C4 decreased over incubation, their proportional contribution to fluorescent DOC did not change significantly in LL2 (p > 0.05; Figure 3; Table 1). These changes were different from those observed in experiment LL1, where the proportions of C3 and C4 increased significantly (Table 1; p < 0.05). Differences in DOC processing and component loadings observed between experiments LL1 and LL2 may have been caused by the freezing of the microbial community in LL1, and subsequent nutrient depletion over the 7-day recuperation period (Jansson et al., 2006). Despite this difference, the overall effect of rapid protein/polyphenol-like consumption (C1, C3) and carbohydrate/humic-like production (C2) was consistent across experiments, and is generally considered to reflect microbial processing (Parlanti et al., 2000; Scully et al., 2004; Wickland et al., 2007; Bowen et al., 2009; Fellman et al., 2009; Hur et al., 2009).
Similar changes were observed in the DOC concentration and component loadings of the control sample, suggesting some microbial processing may be due to ambient microbes, or the possibility that ambient and riverine populations effect similar changes in leaf leachate. However, the possibility of preferential decomposition of different DOM compartments by one microbial community, and concomitant transformation of waste products by other communities makes the intricacies of processing unclear (Covert and Moran, 2001; Docherty et al., 2006). Since absolutely abiotic conditions are practically impossible in leaf leachates, ambient microbial populations may have contributed to some of the observed changes. The changes in the control sample were significantly lower than in the inoculated samples, suggesting that the observed changes in copper-binding properties were mainly due to the inoculum.
The observed rapidity of initial DOC decline has also been attributed to the adsorption of relatively large components to the surfaces of colloids and microbes introduced during inoculation (Maurice et al., 2004; Young et al., 2004). To explore this possibility, colloid formation was investigated by observing second-order fluorescence scattering at Ex/Em = 300/600 nm, following the procedure used by Guéguen et al. (2002). No substantial increases from the level of scattering in the leachate were evident immediately after inoculation, during the observed changes in optical properties, or in the control sample. The apparent absence of particles, similarities between the patterns of change in DOC and protein/polyphenol-like components, and recent reports of extremely rapid protein and carbohydrate consumption by microbes under natural and artificial conditions, together imply that much of these losses are due to the processing of labile constituents (Fellman et al., 2009; Huang et al., 2011).
Changes in DOM Composition and Metal-Binding Properties
Exponential increases in the binding strength and ligand concentration of C1 were contrasted by an exponential decrease in ligand concentration and a linear decrease in binding strength in C2 (Figures 5A,B; Table 2). Further, % f decreased exponentially for C1, and increased exponentially for C2. Overall, exponential changes in metal-binding properties over incubation coincided with those of component loadings for C1 and C2, and for DOC (Figures 2 and 5). Components C3 and C4 also exhibited significantly lower log K and increased CC after inoculation (p < 0.05), but % f was not significantly different after 168 h of microbial exposure for these components (p > 0.05).
The binding strength and complexing capacities of PARAFAC components changed from being significantly different in leachates, to being more similar in biodegraded samples (Figure 5; Table 2). These changes may reflect the partial consumption or transformation of protein/polyphenol-like molecules (C1 and C3). The results of binding parameters based on fluorescence showed a marked increase in variability after incubation (i.e., width of 95% CI increased substantially; Table 2). Changes in copper-binding characteristics measured by voltammetric analysis were also exponential in nature, paralleling rates of change for component loadings and DOC concentration (Figures 2 and 5D,E). Decreases in the binding strength of the overall leachate measured by voltammetry reflected those observed for components C2–4 in fluorescence quenching rather than the increase observed for C1. However, the complexing capacity determined using voltammetry increased in harmony with changes to C1, C3, and C4 (Figures 5B,E). Thus, voltammetric and fluorescence quenching measurements were found to be in general agreement about the effects of inoculation on the copper-binding parameters of leaf leachate. However, the cumulative copper-binding strength of the leachate determined by voltammetry was significantly greater than the binding strength exhibited by any of the PARAFAC components in the quenching experiments (7.2 vs. 4.73–6.11; Figures 5A,D; Table 2), which may be due to the impact of non-fluorescent DOM, or differences in the concentration ranges of metals analyzed (i.e., different analytical windows; Buffle, 1988). Nonetheless, fluorescence quenching analysis yields key information about how the effects of changes in the character of DOM upon binding properties, as it provides site-specific binding parameters for several PARAFAC components that have been related to known constituents of DOM.
Despite the similarities in fluorescence characteristics and chemical properties attributed to protein/polyphenol-like components C1 and C3, and to humic/fulvic-like C2 and C4, both the individual responses of components to incubation and their binding properties were distinct. These unique responses and properties underscore the complex nature of fluorescent DOM both in fresh leaf-litter leachate, and in leachates exposed to microbial action.
Meaningful comparison of specific results with the findings of other researchers is challenging, as studies of copper-binding characteristics that use fresh leaf-litter leachates are scarce and research groups tend to employ distinct methods of analysis, binding models, numbers of sites, litter conditioning, matrix characteristics, etc. Increases in copper-complexing capacity of the same magnitude as those observed using voltammetric analysis have been observed elsewhere for extracts of wheat straw and crimson clover following inoculation with soil microbes, which were also related to similar changes in fluorescence (0.11–6.28 to 0.29–32.1 mmol Cu g C−1; Merritt and Erich, 2003). The log K values found in bulk and low-molecular-weight fractions of wheat straw and crimson clover leachates also tended to become similar over 7 days of exposure to soil microbes, which was attributed to increased polymerization measured as increasing molecular weight. In general, the copper-binding strengths of fresh litter leachates (Merritt and Erich, 2003; Hur and Lee, 2011) are greater than those of microbially degraded litter leachates (Merritt and Erich, 2003) which agrees with the results of this study (Table 2). Thus, the overall changes and general ranges in binding characteristics measured using voltammetry are on the lower end of the general range observed by other researchers.
This study has shown that the copper-binding capability of leachates extracted from maple leaves increased rapidly after inoculation with riverine microbes. Fresh leachates were found to have a relatively low copper-binding capacity and a higher overall binding strength, while degraded material was capable of complexing more material, but with a lower binding strength. Generally, metal toxicity decreases when it is bound to organic matter (Nogueira et al., 2009; Sánchez-Marín et al., 2010), but this is not true for all metals, organisms, or aquatic chemistries (Meyer et al., 1999; Sánchez-Marín et al., 2007). Based on the results of this study, differences in toxicities may also be caused by differences in the degree of organic matter processing, with corresponding differences in the exposure of organisms to metals that are bound to DOM constituents with variable binding strengths. This result underscores the potential importance of the dynamic relationship between microbes, metals, and organic matter, where DOM serves both as a source of nutrition and as a regulator of metal speciation and its effectiveness in the latter role is partially controlled by microbial activities.
Conflict of Interest Statement
The authors declare that the research was conducted in the absence of any commercial or financial relationships that could be construed as a potential conflict of interest.
Acknowledgments
The authors wish to thank the many people involved in making this project a success: B. Marcere for DOC analysis; W. Chen for assistance with the modeling of metal speciation; and A. McDonough for tree species identification. We would like to thank the Editor Veronique Schoemann and the three anonymous reviewers for improving this manuscript with their constructive comments. This work was funded in part by the Canada Research Chairs program, Natural Sciences and Engineering Research Council grants. Chad. W. Cuss gratefully acknowledges the financial support accorded by the Ontario Student Assistance Program Ontario Graduate Scholarship.
References
Bowen, S. R., Gregorich, E. G., and Hopkins, D. W. (2009). Biochemical properties and biodegradation of dissolved organic matter from soils. Biol. Fertil. Soils 45, 733–742.
Buffle, J. (1988). Complexation Reactions in Aquatic Systems: An Analytical Approach. Chichester: Ellis Horwood Limited.
Coble, P. G. (1996). Characterization of marine and terrestrial DOM in seawater using excitation-emission matrix spectroscopy. Mar. Chem. 51, 325–246.
Covert, J. S., and Moran, M. A. (2001). Molecular characterization of estuarine bacterial communities that use high- and low-molecular weight fractions of dissolved organic carbon. Aquat. Microb. Ecol. 25, 127–139.
Docherty, K. M., Young, K. C., Maurice, P. A., and Bridgham, S. D. (2006). Dissolved organic matter concentration and quality influences upon structure and function of freshwater microbial communities. Microb. Ecol. 52, 378–388.
Durán, I., and Nieto, O. (2011). Electrochemical speciation of dissolved Cu, Pb and Zn in an estuarine ecosystem (Ria de Vigo, NW Spain): comparison between data treatment methods. Talanta 85, 1888–1896.
Fellman, J. B., D’Amore, D. V., Hood, E., and Boone, R. D. (2008). Fluorescence characteristics and biodegradability of dissolved organic matter in forest and wetland soils from coastal temperate watersheds in southeast Alaska. Biogeochemistry 88, 169–184.
Fellman, J. B., Hood, E., Edwards, R. T., and Jones, J. B. (2009). Uptake of allochthonous dissolved organic matter from soil and salmon in coastal temperate rainforest streams. Ecosystems 12, 747–759.
Feng, X., Nielsen, L. L., and Simpson, M. J. (2007). Responses of soil organic matter and microorganisms to freeze-thaw cycles. Soil Biol. Biochem. 39, 2027–2037.
Guéguen, C., Belin, C., and Dominik, J. (2002). Organic colloid separation in contrasting aquatic environments with tangential flow filtration. Water Res. 36, 1677–1684.
Guéguen, C., and Dominik, J. (2003). Partitioning of trace metals between particulate, colloidal and truly dissolved fractions in a polluted river: the Upper Vistula River (Poland). Appl. Geochem. 18, 457–470.
Huang, G., Meng, F., Zheng, X., Wang, Y., Wang, Z., Liu, H., and Jekel, M. (2011). Biodegradation behavior of natural organic matter (NOM) in a biological aerated filter (BAF) as a pretreatment for ultrafiltration (UF) of river water. Appl. Microbiol. Biotechnol. 90, 1795–1803.
Hur, J., and Lee, B.-M. (2011). Characterization of binding site heterogeneity for copper within dissolved organic matter fractions using two-dimensional correlation fluorescence spectroscopy. Chemosphere 83, 1603–1611.
Hur, J., Park, M.-H., and Schlautman, M. A. (2009). Microbial transformation of dissolved leaf litter organic matter and its effects on selected organic matter operational descriptors. Environ. Sci. Technol. 43, 2315–2321.
Jansson, M., Bergström, A.-K., Lymer, D., Vrede, K., and Karlsson, J. (2006). Bacterioplankton growth and nutrient use efficiencies under variable organic carbon and inorganic phosphorous ratios. Microb. Ecol. 52, 358–364.
Koponen, H. T., Jaakkoa, T., Keinänen-Toivola, M. M., Kaipainen, S., Tuomainen, J., Servomaa, K., and Martikainen, P.-J. (2006). Microbial communities, biomass, and activities in soils as affected by freeze thaw cycles. Soil Biol. Biochem. 38, 1861–1871.
Lakowicz, J. R. (2006). Principles of Fluorescence Spectroscopy, 3rd Edn. New York: Springer, 54–57.
Lawaetz, A. J., and Stedmon, C. A. (2009). Fluorescence intensity calibration using the Raman scatter peak of water. Appl. Spectrosc. 63, 936–940.
Manceau, A., and Matynia, A. (2010). The nature of Cu bonding to natural organic matter. Geochim. Cosmochim. Acta 74, 2256–2580.
Maurice, P. A., Manecki, M., Fein, J. B., and Schaefer, J. (2004). Fractionation of an aquatic fulvic acid upon adsorption to the bacterium, Bacillus subtilis. Geomicrobiol. J. 21, 69–78.
Merritt, K. A., and Erich, M. S. (2003). Influence of organic matter decomposition on soluble carbon and its copper-binding capacity. J. Environ. Qual. 32, 2122–2131.
Meyer, J. S., Santore, R. C., Bobbitt, J. P., Debrey, L. D., Boese, C. J., Paquin, P. R., Allen, H. E., Bergman, H. L., and Ditoro, D. M. (1999). Binding of nickel and copper to fish gills predicts toxicity when water hardness varies, but free-ion activity does not. Environ. Sci. Technol. 33, 913–916.
Nogueira, P. F. M., Melão, M. G. G., Lombardi, A. T., and Nogueira, M. M. (2009). Natural DOM affects copper speciation and bioavailability to bacteria and ciliate. Arch. Environ. Contam. Toxicol. 57, 274–281.
Ohno, T., and Bro, R. (2006). Dissolved organic matter characterization using multiway spectral decomposition of fluorescence landscapes. Soil Sci. Soc. Am. J. 70, 2028–2037.
Parlanti, E., Wörz, K., Geoffroy, L., and Lamotte, M. (2000). Dissolved organic matter fluorescence spectroscopy as a tool to estimate biological activity in a coastal zone submitted to anthropogenic inputs. Org. Geochem. 31, 1765–1781.
Perdue, E. M., and Lytle, C. R. (1983). Distribution model for binding of protons and metal ions by humic substances. Environ. Sci. Technol. 17, 654–660.
Ružic, I. (1982). Theoretical aspects of the direct titration of natural waters and its information yield for trace metal speciation. Anal. Chim. Acta 140, 99–113.
Ryan, D. K., and Weber, J. H. (1982). Fluorescence quenching titration for determination of complexing capacities and stability constants of fulvic acid. Anal. Chem. 54, 986–990.
Sánchez-Marín, P., Lorenzo, J. I., Blust, R., and Beiras, R. (2007). Humic acids increase dissolved lead bioavailability for marine invertebrates. Environ. Sci. Technol. 41, 5679–5684.
Sánchez-Marín, P., Santos-Echeandía, J., Nieto-Cid, M., Álvarez-Salgado, X. A., and Beiras, R. (2010). Effect of dissolved organic matter (DOM) of contrasting origins on Cu and Pb speciation and toxicity to Paracentrotus lividus larvae. Aquat. Toxicol. 96, 90–102.
Scully, N. M., Maie, N., Dailey, S. K., Boyer, J. N., Jones, R. D., and Jaffé, R. (2004). Early diagenesis of plant-derived dissolved organic matter along a wetland, mangrove, estuary ecotone. Limnol. Oceanogr. 49, 1667–1678.
Smith, D. S., and Kramer, J. R. (1998). Multi-site aluminum speciation with natural organic matter using multiresponse fluorescence data. Anal. Chim. Acta 363, 21–29.
Smith, D. S., and Kramer, J. R. (2000). Multi-site metal binding to fulvic acid determined using multiresponse fluorescence. Anal. Chim. Acta 416, 211–220.
Stedmon, C. A., and Bro, R. (2008). Characterizing dissolved organic matter fluorescence using parallel factor analysis: a tutorial. Limnol. Oceanogr. Methods 6, 572–579.
Stedmon, C. A., and Markager, S. (2005). Resolving the variability in dissolved organic matter fluorescence in a temperate estuary and its catchment using PARAFAC analysis. Limnol. Oceanogr. 50, 686–697.
Stedmon, C. A., Markager, S., and Bro, R. (2003). Tracing dissolved organic matter in aquatic environments using a new approach to fluorescence spectroscopy. Mar. Chem. 82, 239–254.
Stewart, A. J., and Wetzel, R. G. (1980). Fluorescence:absorbance ratios-a molecular-weight tracer of dissolved organic matter. Limnol. Oceanogr. 25, 559–564.
Sutton, R., and Sposito, G. (2005). Molecular structure in soil humic substances: the new view. Environ. Sci. Technol. 39, 9009–9015.
Tank, J. L., Rosi-Marshall, E. J., Griffiths, N. A., Entrekin, S. A., and Stephen, M. L. (2010). A review of allochthonous organic matter dynamics and metabolism in streams. J. North Am. Benthol. Soc. 29, 118–146.
Tipping, E. (1998). Humic ion-binding model VI: and improved description of the interactions of protons and metal ions with humic substances. Aquat. Geochem. 4, 3–48.
Wickland, K. P., Neff, J. C., and Aiken, G. R. (2007). Dissolved organic carbon in Alaskan boreal forest: sources, chemical characteristics, and biodegradability. Ecosystems 10, 1323–1340.
Wong, J. C. Y., and Williams, D. D. (2010). Sources and seasonal patterns of dissolved organic matter (DOM) in the hyporheic zone. Hydrobiologia 647, 99–111.
Yamashita, Y., and Jaffé, R. (2008). Characterizing the interactions between trace metals and dissolved organic matter using excitation-emission matrix and parallel factor analysis. Environ. Sci. Technol. 42, 7374–7379.
Young, K. C., Maurice, P. A., Docherty, K. M., and Bridgham, S. D. (2004). Bacterial degradation of dissolved organic matter from two northern Michigan streams. Geomicrobiol. J. 21, 521–528.
Appendix
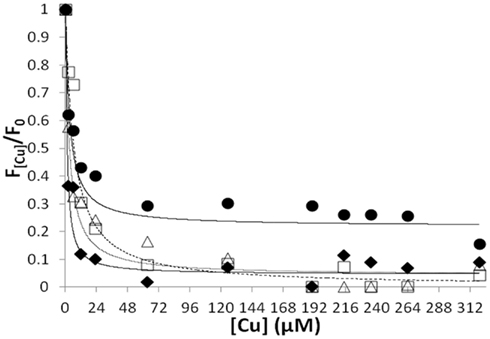
Figure A1. Quenching data and fitted models (following Smith and Kramer, 1998) for PARAFAC components in leaf leachate after 168 h of microbial degradation. PARAFAC component C1-circles, C2-squares, C3-diamonds, C4-triangles.
Keywords: biodegradation, metal binding, dissolved organic matter, dissolved organic carbon, voltammetry, parallel factor analysis, fluorescence quenching, copper
Citation: Cuss CW and Guéguen C (2012) Impacts of microbial activity on the optical and copper-binding properties of leaf-litter leachate. Front. Microbio. 3:166. doi: 10.3389/fmicb.2012.00166
Received: 05 December 2011; Accepted: 13 April 2012;
Published online: 07 May 2012.
Edited by:
Veronique Schoemann, Royal Netherlands Institute for Sea Research, NetherlandsReviewed by:
Rachel Narehood Austin, Bates College, USASylvia McDevitt, Skidmore College, USA
Jeroen De Jong, Royal Netherlands Institute for Sea Research, Netherlands
Copyright: © 2012 Cuss and Guéguen. This is an open-access article distributed under the terms of the Creative Commons Attribution Non Commercial License, which permits non-commercial use, distribution, and reproduction in other forums, provided the original authors and source are credited.
*Correspondence: Celine Guéguen, Department of Chemistry, Trent University, 1600 West Bank Drive, Peterborough, ON, Canada, K9J 7B8. e-mail:Y2VsaW5lZ3VlZ3VlbkB0cmVudHUuY2E=