- Rosenstiel School of Marine and Atmospheric Sciences, University of Miami, Miami, FL, USA
The dominant process adding nitrogen (N) to the ocean, di-nitrogen (N2) fixation, is mediated by prokaryotes (diazotrophs) sensitive to a variety of environmental factors. In particular, it is often assumed that consequential rates of marine N2 fixation do not occur where concentrations of nitrate (NO−3) and/or ammonium (NH+4) exceed 1μM because of the additional energetic cost associated with assimilating N2 gas relative to NO−3 or NH+4. However, an examination of culturing studies and in situ N2 fixation rate measurements from marine euphotic, mesopelagic, and benthic environments indicates that while elevated concentrations of NO−3 and/or NH+4 can depress N2 fixation rates, the process can continue at substantial rates in the presence of as much as 30μM NO−3 and/or 200μM NH+4. These findings challenge expectations of the degree to which inorganic N inhibits this process. The high rates of N2 fixation measured in some benthic environments suggest that certain benthic diazotrophs may be less sensitive to prolonged exposure to NO−3 and/or NH+4 than cyanobacterial diazotrophs. Additionally, recent work indicates that cyanobacterial diazotrophs may have mechanisms for mitigating NO−3 inhibition of N2 fixation. In particular, it has been recently shown that increasing phosphorus (P) availability increases diazotroph abundance, thus compensating for lower per-cell rates of N2 fixation that result from NO−3 inhibition. Consequently, low ambient surface ocean N:P ratios such as those generated by the increasing rates of N loss thought to occur during the last glacial to interglacial transition may create conditions favorable for N2 fixation and thus help to stabilize the marine N inventory on relevant time scales. These findings suggest that restricting measurements of marine N2 fixation to oligotrophic surface waters may underestimate global rates of this process and contribute to uncertainties in the marine N budget.
Introduction
Phytoplankton growing in the sunlit surface ocean (euphotic zone) produce organic matter via photosynthesis at a rate of ~50PgC year−1 (Westberry et al., 2008), and thus play an important role in the global carbon (C) cycle. However, phytoplankton growth in the euphotic zone is commonly limited by the availability of nutrients such as nitrogen (N); consequently, the processes that add and remove N to and from the ocean, respectively, influence the C cycle and thus climate. Unlike the physical processes that supply other biologically necessary elements like phosphorus (P) and iron (Fe) to the ocean (such as atmospheric deposition and fluvial inputs), the dominant process adding N to the ocean, di-nitrogen (N2) fixation, is unique in that it is biologically mediated. While N2 gas is unavailable to most organisms, certain groups of prokaryotes known as diazotrophs have the enzymatic ability to reduce dissolved N2 to ammonium (NH+4) and assimilate it into their biomass. The ultimate fate of N in diazotrophic biomass is to be cycled into more bio-available forms, including nitrate (NO−3), that serve as the primary source of assimilative N for non-diazotrophic phytoplankton and bacteria in the ocean. In spite of the fundamental importance of N2 fixation in the global C cycle and in supporting the base of the food web, the locations and rates of N2 fixation in the ocean are poorly known.
Uncertainty in the rates of marine N2 fixation contributes to ambiguity as to whether the modern marine N budget is balanced. Some estimates suggest that rates of N fluxes to the ocean only compensate for one third to one half of the fluxes of N out of the ocean (Codispoti et al., 2001; Codispoti, 2007), while constraints from paleoceanographic and modeling studies indicate that the marine N budget has been balanced to within ~10% over at least the Holocene (Brandes and Devol, 2002; Deutsch et al., 2004). Assuming that the marine N budget is essentially balanced, the discrepancy in N flux estimates requires that rates of marine N2 fixation are underestimated and/or that rates of N loss are overestimated. The constraint of an approximately balanced marine N budget also implies that there are feedback mechanisms allowing N2 fixation and denitrification, the dominant pathway by which N is lost from the ocean, to respond to each other on relatively short (i.e., ≤1000 years) timescales. Currently, both the size of the fluxes of N to and from the ocean, as well as the nature of potential feedback mechanisms that maintain a balanced marine N budget, remain ill-defined.
While improved knowledge of the marine N cycle requires a multifaceted approach, characterizing the physical and chemical sensitivities of marine diazotrophs to various environmental conditions provides constraints on regions of the ocean that may support diazotrophy. A better understanding of the sensitivities of marine diazotrophs may also reveal mechanisms by which marine N2 fixation can respond to changes in rates of marine denitrification. However, our ability to describe the sensitivities of N2 fixation depends on the degree to which we understand and have characterized the diversity of marine diazotrophs, an understanding presently limited by the small number of marine diazotrophs isolated for manipulative culture-based experiments.
The majority of marine N2 fixation has historically been attributed to the filamentous, non-heterocystous cyanobacteria Trichodesmium spp. resident in the warm, stratified, and nutrient-depleted regions of the surface ocean (Carpenter, 1983; Capone et al., 1997, 2005). However, the past decade has seen a number of challenges to the paradigm that N2 fixation by Trichodesmium spp., especially in the tropical North Atlantic, is the primary source of N to the global ocean. For example, molecular tools have identified novel diazotrophs present in environments with physical and/or chemical characteristics different from their more well-studied counterparts in tropical and subtropical seas (Zehr et al., 2001, 2008; Montoya et al., 2004; Langlois et al., 2008; Moisander et al., 2010; Fernandez et al., 2011). Additionally, indirect evidence such as remote sensing (Westberry et al., 2005; Westberry and Siegel, 2006) and geochemical modeling (Deutsch et al., 2007) describes geographic distributions of N2 fixers, including Trichodesmium spp., that differs from our expectation of oligotrophic dominance. Finally, a number of both in situ and culture-based studies challenge some long-held notions of diazotrophic sensitivities to nutrients, including the degree to which inorganic N inhibits N2 fixation. All of these findings raise the possibility that the geographic distribution and sensitivities of marine diazotrophs may be different than previously thought. As recognition of both the breadth of oceanic conditions supportive of diazotrophy and the diversity of marine diazotrophs increases, so too does the possibility that considerable rates of N2 fixation occur in environments beyond the surface waters of the oligotrophic gyres. If so, global marine N2 fixation rates may be greater than previously estimated.
In spite of an incomplete knowledge of marine diazotroph diversity, environmental and culture-based observations can establish criteria consistent with diazotrophic success. Environmental factors that are known to regulate marine diazotrophy include light (Carpenter et al., 1993; Milligan et al., 2007; Breitbarth et al., 2008), temperature (Chen et al., 1998; Breitbarth et al., 2007; Stal, 2009), oxygen (Robson and Postgate, 1980; Capone and Budin, 1982; Stal and Heyer, 1987), and metal availability (Rueter et al., 1990; Berman-Frank et al., 2001; Kustka et al., 2003; Chappell and Webb, 2010; Saito et al., 2011). Here, the sensitivity of marine diazotrophs to dissolved inorganic N (DIN), in particular NO−3 and NH+4, is evaluated, and evidence for the inhibition of N2 fixation by DIN in (1) the euphotic zone, (2) the sub-euphotic zone, and (3) benthic marine environments, is reviewed. In particular, the question of whether significant rates of N2 fixation can occur when ambient DIN concentrations are significant, i.e., ≥1μM, is examined. The findings of this review are that: (1) reports of substantial rates of N2 fixation in euphotic and benthic environments with ≥1μM DIN indicate that elevated DIN does not necessarily preclude large N2 fixation fluxes; (2) certain benthic marine diazotrophs may be less sensitive to chronic exposure to elevated concentrations of DIN than diazotrophs in the euphotic zone; (3) while benthic N2 fixation is widespread and can occur at significant rates, global estimates are poorly known, likely contributing significant uncertainty to global estimates of marine N2 fixation fluxes, and, (4) euphotic zone diazotrophs may respond to changes in ambient N:P ratios, providing a potential mechanism for diazotrophs to respond to changes in denitrification rates and thus to stabilize the marine N inventory. These findings are investigated below.
Nutrient Inhibition of Euphotic Zone N2 Fixation
There are three primary lines of evidence for the inhibition of marine N2 fixation by inorganic N. The first results from circumstances associated with the origins of marine diazotrophic research. Before molecular tools became widely available, our understanding of marine diazotrophs was largely limited to the study of macroscopic cyanobacteria that could be readily identified and manipulated in field and culture-based studies. The most conspicuous and well-studied marine diazotroph, Trichodesmium spp., has predominantly been observed in warm, nutrient depleted regions of the surface ocean (Carpenter, 1983; Capone et al., 1997, 2005). The association of Trichodesmium spp. with these environmental characteristics, and the strong bias of studies of marine diazotrophs towards Trichodesmium spp., has perhaps unintentionally lead to the expectation that other marine diazotrophs will share the same environmental preferences. The second line of evidence for DIN inhibition of N2 fixation comes from calculations showing that it requires ~25% more energy to reduce N2 (87 kcal) than NO−3 (69 kcal) to NH+4 (Falkowski, 1983). Together with the majority of field observations of diazotrophs from nutrient-depleted tropical surface waters, this additional energetic cost has lead to the assumption that significant rates of N2 fixation do not occur in marine environments with ≥1μM DIN.
The third line of evidence for the inhibition of N2 fixation by DIN comes from culture studies of marine diazotrophs that test the effects of short-term and/or chronic exposure to NO−3 and NH+4 (e.g., Ohki et al., 1991; Mulholland and Capone, 1999; Mulholland et al., 2001; Fu and Bell, 2003; Holl and Montoya, 2005) (Table 1) (the numerous studies of DIN inhibition of fresh water diazotrophs are not reviewed here). These studies have demonstrated that NH+4 is more effective at inhibiting N2 fixation than NO−3 (Ohki and Fujita, 1982; Ohki et al., 1991; Mulholland et al., 2001), presumably because of the larger energetic cost associated with assimilating N2 vs. NH+4 than with assimilating N2 vs. NO−3. Additionally, these studies have shown that chronic exposure to both NO−3 and NH+4 more strongly inhibits N2 fixation than does short-term (i.e., less than 24 h) exposure (Ohki et al., 1991; Mulholland et al., 2001; Fu and Bell, 2003; Milligan et al., 2007; Dekaezemacker and Bonnet, 2011; Sandh et al., 2011; Knapp et al., 2012) (Table 1). Supporting these observations of depressed N2 fixation rates, physiological changes in Trichodesmium have also been documented when cultures are grown with NO−3 as a source of assimilative N instead of dissolved N2 gas. After chronic exposure of Trichodesmium cultures to 100 μM NO−3 (Milligan et al., 2007) demonstrated a down-regulation of Mehler activity relative to cultures grown on N2 gas, while (Sandh et al., 2011) found an inhibition of nitrogenase expression and diazocyte development. These effects of NO−3 on diazotroph physiology suggest that chronic exposure to DIN has a greater impact on N2 fixation rates than does short-term exposure.
The relatively small impact on N2 fixation rates by short-term exposure to NO−3 (Table 1) has implications for proposed mechanisms for diazotrophs to acquire limiting nutrients such as P. For example, short-term exposure to DIN could take place during the vertical migration of Trichodesmium spp. (Capone et al., 1990) showed that nitrogenase in Trichodesmium spp. is synthesized each morning prior to the initiation of nitrogenase activity. Consequently, the downward migration of Trichodesmium spp. at night (Villareal and Carpenter, 1990) to acquire P (Villareal and Carpenter, 2003) at the top of the nutricline (where NO−3 is also present) might not strongly depress peak daytime N2 fixation rates in Trichodesmium spp. if exposure to NO−3 is brief and occurs at night before new nitrogenase is synthesized. While studies of the effects of DIN inhibition on marine diazotrophs have largely been restricted to Trichodesmium spp., recent culturing work suggests that Crocosphaera has similar sensitivities to short-term vs. chronic NO−3 exposure (Dekaezemacker and Bonnet, 2011; Knapp et al., 2012). Given the similarity in response of Trichodesmium and Crocosphaera spp. and the limited genetic divergence in nitrogenase amino acid sequences in marine diazotrophic cyanobacteria (Zehr, 2011), the smaller effect of short-term vs. long-term DIN exposure on N2 fixation rates may be common among other diazotrophic cyanobacteria as well.
Culturing studies clearly show that DIN can inhibit N2 fixation; however most inhibition studies have been performed with concentrations of N and/or P in the culture media that exceed those typically found in the euphotic zone (Table 1). This discrepancy between nutrient concentrations in the environment and in cultures leaves open the possibility that culturing studies overestimate the degree to which DIN inhibits N2 fixation in the environment. Recent culturing work using concentrations of NO−3 and PO3−4 typically found in the euphotic zone show that chronic exposure of Trichodesmium and Crocosphaera to 5 to 16 μM NO−3 depresses N2 fixation rates relative to cultures grown with no NO−3, but that N2 fixation did not stop even in cultures amended with as much as 16 μM NO−3 (Knapp et al., 2012). Moreover, the same work showed that higher concentrations of PO3−4 can offset NO−3 inhibition of per-cell N2 fixation rates by increasing diazotroph abundance. Consequently, the volume-integrated rate of N2 fixation in treatments grown with 5.0 μM NO−3 and 1.0 μM PO3−4 was comparable to the volume-integrated rate of N2 fixation in treatments not amended with NO−3 and grown with 0.5 μM PO3−4 (Knapp et al., 2012).
The finding of increased diazotroph abundance as a function of increasing P availability is consistent with the well-recognized role that P availability plays in regulating the biomass of microbes [e.g., (Elser et al., 2007; Loladze and Elser, 2011; Scott et al., 2012)]. Investigations into variability in phytoplankton biomass N:P ratios indicate that P is preferentially used to create new biomass (e.g., in DNA) whereas N is required both for the production of new biomass as well as for the production of proteins, especially associated with resource acquisition (Klausmeier et al., 2004; Loladze and Elser, 2011). Consequently, the results of (Knapp et al., 2012) documenting a two- to three-fold greater abundance of both the diazotrophs Crocosphaera watsonii and Trichodesmium erythraeum in batch cultures grown with 1.0 vs. 0.5 μM PO3−4 are perhaps unsurprising. What is surprising is that the increase in diazotrophic biomass was sufficient to offset the lower per-trichome rates of N2 fixation resulting from inhibition by 5.0 μM NO−3. This work shows that NO−3 present at typical surface ocean concentrations does not necessarily preclude N2 fixation fluxes comparable to those observed in NO−3-depleted environments, and suggests that field and numerical modeling investigations of marine N2 fixation that exclude surface ocean environments with ≥1μM NO−3 may overlook potentially significant regions of N2 fixation.
Additionally, the work of (Knapp et al., 2012) identifies a potential mechanism for euphotic zone diazotrophs to respond to changes in surface ocean concentrations of NO−3 and PO3−4. Specifically, while it has been assumed that low ambient N:P ratios (a condition created by denitrification occurring below the euphotic zone) would stimulate higher rates of N2 fixation (Haug et al., 1998; Deutsch et al., 2004), no mechanism has been proposed for how a diazotroph would sense and respond favorably to lower N:P ratios. The results of Knapp et al. (2012) describe how the separate physiological effects of relatively high concentrations of P (i.e., increased diazotroph abundance) and relatively low concentrations of N (i.e., lessened NO−3 inhibition of N2 fixation) together can create conditions that can support significant N2 fixation fluxes. While relatively low N and high P concentrations have distinct effects on diazotrophs, combining these effects results in a perceived advantage for diazotrophs growing in environments with low ambient N:P ratios and may provide a feedback mechanism for diazotrophs to respond to increases in denitrification and thus help stabilize the marine N inventory. This finding also has implications for diazotroph biogeography, and suggests that significant abundances and/or N2 fixation fluxes may not be restricted to oligotrophic surface waters such as the North Atlantic, but may occur in more nutrient-replete regions of the surface ocean such as the surface waters overlying ODZs where rates of N loss are high.
Indeed, these culture-based results are consistent with recent field observations by (Fernandez et al., 2011; Sohm et al., 2011) who document N2 fixation rates of 0.1–7.5 nmol N L−1 d−1 in surface ocean waters with 5–20 μM NO−3 (Table 2), although molecular analyses indicate that this fixation was carried out by diazotrophs other than Trichodesmium or Crocospahera spp. These rates of N2 fixation in NO−3-replete coastal waters are comparable to the range in N2 fixation rates measured at Station ALOHA in the North Pacific gyre of 0.5–11 nmol N L−1 d−1 (Church et al., 2009) and where surface NO−3 concentrations are consistently ≤100 nM (Fujieki et al., 2011). Similarly, (Halm et al., 2012) found higher euphotic zone rates of N2 fixation in regions of the South Pacific gyre with higher concentrations of NO−3 (as well as PO3−4) compared to more oligotrophic regions of the gyre, i.e., average N2 fixation rates of 1.5 ± 0.3 nmol N L−1 d−1 vs. 0.4 ± 0.3 nmol N L−1 d−1, respectively. These N2 fixation rate measurements are supported by numerous other field observations documenting significant abundances of and/or N2 fixation by diazotrophs including Trichodesmium spp. in other near-shore locations (Lenes et al., 2001; White et al., 2007; Rodier and Le Borgne, 2008; Grosse et al., 2010; Rodier and Le Borgne, 2010; Bombar et al., 2011) (Table 2).
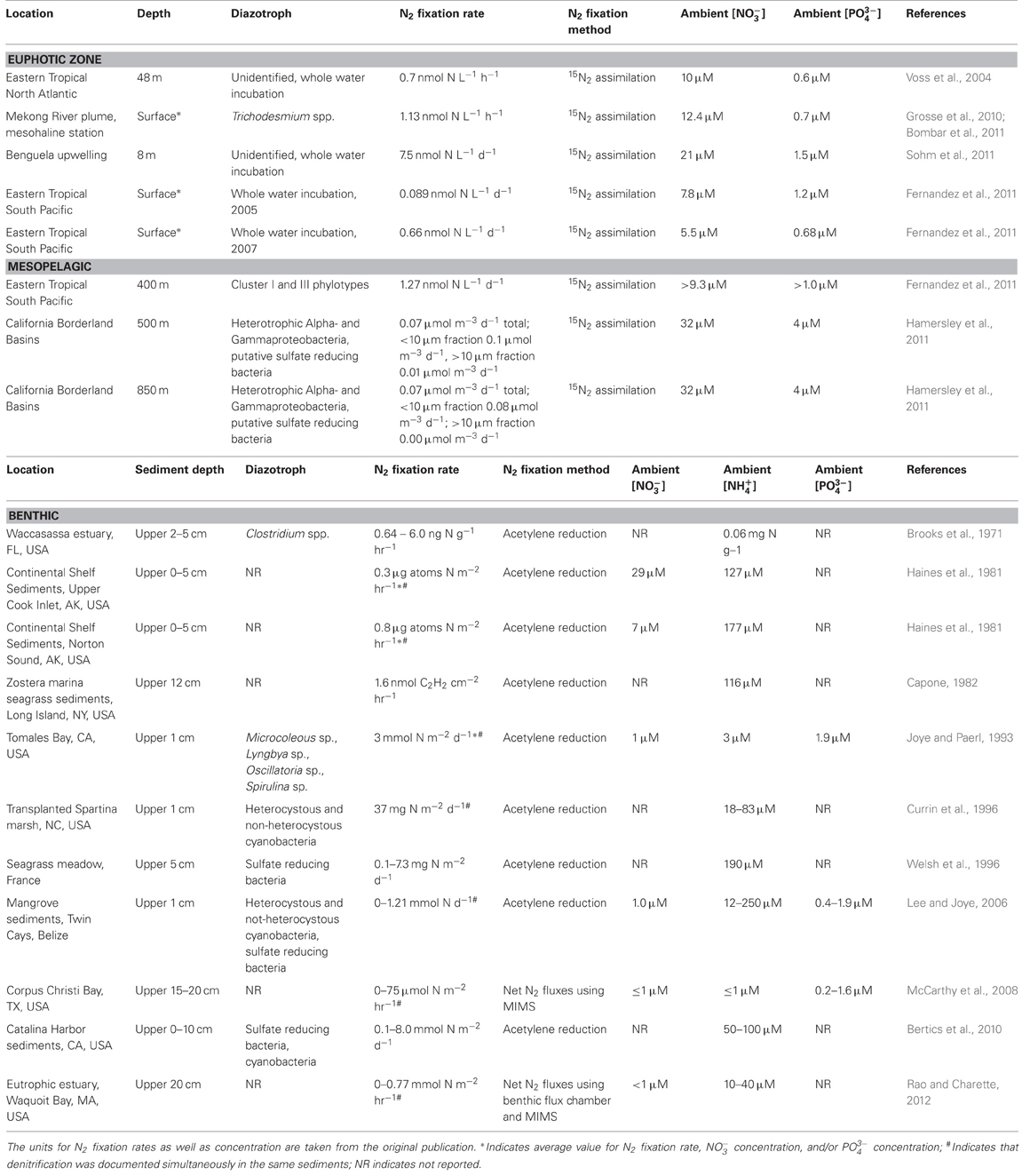
Table 2. Reported rates of N2 fixation in euphotic, mesopelagic, and benthic marine environments with significant (i.e., ≥ 1 μM) ambient concentrations of NO−3 and/or NH+4.
These reports of substantial rates of N2 fixation in NO−3-bearing surface waters, especially in upwelling and coastal regions, underscore the potential bias of prior field campaigns documenting N2 fixation predominantly in the nutrient-depleted oligotrophic gyres, and suggest that N2 fixation may have a broader geographic distribution in marine euphotic waters that episodically and/or chronically have significant DIN concentrations. Indeed, the strains of Trichodesmium erythraeum commonly used in culture studies, i.e., NIBB1067 and IMS101, were collected from the coastal waters of Japan and North Carolina, respectively (Ohki and Fujita, 1982; Prufert-Bebout et al., 1993), where surface water DIN concentrations are at least episodically elevated. That Trichodesmium spp. are frequently found in coastal waters that can have relatively high DIN concentrations is relevant considering that recent remote sensing (Westberry and Siegel, 2006) and geochemical modeling (Deutsch et al., 2007) studies have predicted high abundances of diazotrophs and/or rates of N2 fixation in regions of the surface ocean with NO−3 concentrations consistently ≥5 μM (Garcia et al., 2010). The results reviewed here suggest that NO−3 is not as inhibitive of N2 fixation by euphotic-zone diazotrophs as previously thought, especially if P and the necessary trace metals are abundant, and have implications for field studies documenting marine N2 fixation fluxes as well as for the parameterization of N2 fixation in models.
Nutrient Inhibition of Mesopelagic N2 Fixation
While there are only a handful of reports of N2 fixation occurring in the mesopelagic (i.e., sub-euphotic) water column, advances in molecular techniques capable of identifying diazotrophs and the improved sensitivity of mass spectrometers for detecting the incorporation of labeled 15N2 into suspended particulate organic N (PNsusp) have improved our ability to evaluate N2 fixation in this environment. It is expected that N2 fixation in this portion of the water column would be carried out by diazotrophs that have substantially different physiologies than those living in the euphotic zone: mesopelagic diazotrophs require a different energy source than their photosynthetic counterparts, they need to tolerate lower temperatures, and due to the higher concentrations of NO−3 below the base of the euphotic zone, they would also presumably be less inhibited by NO−3. Perhaps unsurprisingly then, diazotrophs collected from meso- and bathypelagic waters contain nifH sequences distinct from euphotic zone diazotrophs. In samples collected from the deep North Pacific (Mehta et al., 2003, 2005) identified a number of nifH sequences associated with methanogens and anaerobic sulfate reducers from hydrothermal vent systems, and was able to document growth and N2 fixation in a culture of thermophilic archeal methanogens (Mehta and Baross, 2006). (Hewson et al., 2007) identified nifH genes in samples collected throughout the water column of the Sargasso Sea and detected nifH in meso- and abyssopelagic samples more consistently than in euphotic zone samples, suggesting the potential for diazotrophy below the euphotic zone. However, (Hewson et al., 2007) recovered nifH sequences of the cyanobacterial diazotrophs Trichodesmium thiebautii and Crocosphaera watsonii at 250 and 1000 m, respectively, demonstrating that the nifH associated with diazotrophs active in other environments persists upon transport to the deep ocean in a reasonably robust form, as has been recently reported for RuBisCO (Orellana and Hansell, 2012). However, (Hewson et al., 2007) also detected nifH expression in some mesopelagic samples, indicating some diazotrophs may be active in this NO−3-rich environment. Similarly, (Jayakumar et al., 2012) found both nifH DNA and cDNA sequences associated with strictly anaerobic proteobacteria in samples collected from the oxygen minimum zone of the Arabian Sea, also indicating potential activity of diazotrophs in sub-euphotic zone waters.
In addition to the molecular studies described above, two recent reports document relatively low rates of N2 fixation in mesopelagic samples collected from coastal environments. In the NO−3-rich coastal waters of the Eastern Tropical South Pacific (ETSP), (Fernandez et al., 2011) measured N2 fixation both in the euphotic zone and in mesopelagic waters, including in the core of the local oxygen deficient zone (ODZ) where they reported rates of 1.3 nmol N L−1 d−1 (Table 2). While (Fernandez et al., 2011) recovered numerous nifH sequences, they amplified no cyanobacterial phylotypes in surface or subsurface waters; instead most of the nifH sequences aligned with Cluster I, and to a lesser extent, Cluster III nifH genes, including representatives of anaerobic sulfate reducers. In mesopelagic samples collected in the California Borderland Basins (i.e., San Pedro and Santa Monica Basins) (Hamersley et al., 2011) report similar N2 fixation rates of 0.07 μmol N m−3 d−1 (Table 2). The most common nifH phylotype recovered by (Hamersley et al., 2011) was from the UCYN-A group found both in surface and mesopelagic samples. Additionally, (Hamersley et al., 2011) recovered heterotrophic nifH sequences in mesopelagic samples from Cluster I as well as a number of Cluster III sequences that correspond to strict anaerobes, including alpha- and gamma-proteobacteria, as well as sulfate reducing bacteria (SRB). While both (Fernandez et al., 2011; Hamersley et al., 2011) suggest that diazotrophy in these mesopelagic environments may be associated with oxygen deficiency, the similarity of some mesopelagic nifH sequences to those of diazotrophs found both in surface waters and in benthic environments (see below) raises the possibility that some of the diazotrophs recovered in these near-shore mesopelagic samples are introduced via sinking particles (from the euphotic zone) or via nepheloid layer from sediments to the water column further offshore. Given that a number of the phylotypes collected by (Hamersley et al., 2011) are similar to sequences from microbial mats and/or to cultivated strains of strict anaerobes, a condition not met in the water column of the San Pedro Basin where ambient oxygen concentrations are ~11μM, it raises the possibility that sedimentary microbes are resuspended and then detected in mesopelagic waters.
The determination of N2 fixation rates in mesopelagic waters presents unique analytical challenges as it depends on the incorporation of 15N2 by living, active diazotrophs into particulate organic matter that can then be analyzed by combustion on an isotope ratio mass spectrometer (Montoya et al., 1996). Even with increasingly sensitive instrumentation, the concentration of PNsusp in mesopelagic waters is extremely low. Thus, even with “large volume,” i.e., 4 L, incubations and given a typical detection limit of ~1.4 μmol N for GC-MS systems commonly used to analyze these samples (e.g., http://stableisotopefacility.ucdavis.edu/), a PNsusp concentration of ~0.35 μM is required to generate a signal above typical analytical detection limits. Since most open-ocean PNsusp concentrations are only this high within the euphotic zone, and then decrease sharply in the mesopelagic (i.e., PNsusp concentrations at 300 m at BATS and HOT are 0.05 μM) (Michaels and Knap, 1996; Fujieki et al., 2011), even larger volume incubations and/or more sensitive analytical approaches are required to reliably to detect N2 fixation rates in these waters. While PNsusp concentrations in mesopelagic waters of near-shore environments are higher than those in the oligotrophic ocean, e.g., (Hamersley et al., 2011) report PNsusp of 0.23 and 0.25 μM for their samples collected at 500 and 850 m, respectively, ensuring that mesopelagic samples have sufficient PNsusp to generate a signal above detection limits remains a significant challenge for documenting mesopelagic N2 fixation rates. Moreover, it is not clear that improving incubation techniques to increase 15N2 gas solubility (Mohr et al., 2010) will improve the ability to measure mesopelagic N2 fixation rates, as this modification does not increase the initial quantity of PNsusp in a mesopelagic sample. Given the very low PNsusp concentration in mesopelagic waters, great care must be taken to quantify blanks for these incubations and to demonstrate that N2 fixation rates generated by these methods contain a sufficient quantity of N to exceed analytical detection limits. Consequently, it may be warranted to view the water-column integrated mesopelagic N2 fixation rates of 55 μmol N m−2 d−1 in the California Borderland Basins (Hamersley et al., 2011) and 5.4 ± 2.4 μmol N m−2 d−1 in the ETSP (Fernandez et al., 2011), and their potential to help resolve global marine N budget imbalances, as provisional estimates until supporting measurements confirm the activity of N2 fixation in mesopelagic environments. If these early reports of relatively low N2 fixation rates in sub-euphotic zone waters (Table 2) are broadly characteristic of mesopelagic environments, they may be the consequence of NO−3 inhibition. A better understanding of the capacity of mesopelagic environments to support diazotrophy will benefit from methodological and analytical improvements of in situ N2 fixation rate measurements, as well as successful culturing of microbes recovered from these environments.
Nutrient Inhibition of Benthic Marine N2 Fixation
From intertidal cyanobacterial mats to dark muds, and from low to high latitudes, numerous reports from diverse marine ecosystems demonstrate that benthic diazotrophy is widespread (Capone, 1983 and references therein). N2 fixation in marine sediments has received renewed attention based on evidence that the net flux of N2 gas in certain coastal sediments may have changed from efflux, via denitrification, to influx, via N2 fixation, potentially forced by climate change (Fulweiler et al., 2007). Due to the high concentrations of NO−3 and/or NH+4 that can accumulate as a result of organic matter degradation, N2 fixation in benthic environments presents perhaps the greatest challenge to the expectation for DIN to inhibit diazotrophy. Table 2 includes the small subset of all studies documenting benthic marine N2 fixation that reported both N2 fixation rates as well as concentrations of ambient NO−3 and/or NH+4 that exceeded 1μM. While the culture-based studies described above indicate that NH+4 significantly depresses N2 fixation rates in Trichodesmium and Crocosphaera spp., rates of 7–521μMol N m−2 d−1 have been documented in seagrass-bearing, NH+4-rich (190 μM) sediments on the French coast (Welsh et al., 1996). Similar rates have been reported in other NH+4-rich benthic environments, including mangrove sediments (Lee and Joye, 2006) and in coastal sediments from Alaska (Haines et al., 1981) to California (Bertics et al., 2010) to Florida (Brooks et al., 1971), indicating that benthic N2 fixation can occur at considerable rates in spite of high ambient NH+4 concentrations.
Given that the highest rates of N loss in the ocean occur in marine sediments (Brandes and Devol, 2002), it is perplexing that both N2 fixation and denitrification have frequently been observed in the same sediments (Haines et al., 1981; Slater and Capone, 1984; Joye and Paerl, 1993; Currin et al., 1996; An and Joye, 2001; Gardner et al., 2006; Lee and Joye, 2006; Fulweiler et al., 2007; McCarthy et al., 2008; Bertics et al., 2012; Rao and Charette, 2012). Indeed, Azospirillum, a bacteria associated with seagrasses (Patriquin, 1978) is thought to carry out both denitrification and N2 fixation (Bothe et al., 1981). These observations raise the question: if N2 fixation is an energetically costly process whose role is to provide a source of assimilatory N to the ecosystem, and if diazotrophs are inhibited by DIN, why does N2 fixation happen at significant rates in benthic environments rich in DIN and that also support denitrification?
Benthic diazotrophy has been investigated with a variety of biological and geochemical tools that together indicate that benthic N2 fixation is carried out by a diverse suite of microbes at environmentally significant rates (Table 2). Many benthic N2 fixation rates have been measured using acetylene reduction, and concerns have been raised regarding its use in these environments because of the capacity for acetylene to inhibit other microbial processes including denitrification, methanogenesis, methane oxidation, sulfate reduction, nitrification, and even N2 fixation [(Capone, 1983) and references therein]. In spite of these and other more general concerns regarding the limitation of methods to measure absolute rates of benthic microbial processes, including the high degree of spatial heterogeneity due to microsites and steep geochemical gradients on millimeter spatial scales, benthic diazotrophy has been validated using 15N2 assimilation (Patriquin and Knowles, 1972; Burris, 1976; Carpenter et al., 1978; Capone and Budin, 1982; Dekas et al., 2009) and net N2 gas flux measurements made using membrane inlet mass spectrometry (MIMS) (An and Joye, 2001; Gardner et al., 2006; Fulweiler et al., 2007; McCarthy et al., 2008; Rao and Charette, 2012). Based on visual identification of diazotrophs and differences in N2 fixation rates between light and dark incubations, cyanobacteria are thought to contribute to N2 fixation fluxes in intertidal microbial mat consortia (Joye and Paerl, 1993; Currin et al., 1996; An et al., 2001; Lee and Joye, 2006). Additionally, a number of benthic studies have used molybdate amendment experiments to inhibit sulfate reduction and have simultaneously inhibited N2 fixation in the same sediments; such experiments have been used to attribute N2 fixation in certain benthic marine environments to SRB (Gandy and Yoch, 1988; Welsh et al., 1996; Nielsen et al., 2001; Burns et al., 2002; Steppe and Paerl, 2002; Bertics et al., 2010). Molecular tools have also verified the presence of nif genes, and thus the metabolic potential for N2 fixation, in various benthic marine microbes including in SRB (Burns et al., 2002; Steppe and Paerl, 2002, 2005; Dekas et al., 2009; Bertics et al., 2010, 2012), anaerobic methane-oxidizing archaea (Dekas et al., 2009), and benthic cyanobacteria (Steppe and Paerl, 2005; Bertics et al., 2010).
Previous studies provide some insight into the role of DIN in regulating N2 fixation and denitrification in some benthic environments. Specifically, (Joye and Paerl, 1993, 1994) established seasonality in patterns of N2 fixation and denitrification in Tomales Bay, CA sediments that are consistent with studies documenting DIN inhibition of N2 fixation. (Joye and Paerl, 1993, 1994) observed that when ambient benthic DIN concentrations were relatively low, N2 fixation rates were high and denitrification rates were low, but when runoff or other sources introduced NO−3 to sediments, denitrification rates increased and N2 fixation rates decreased. These observations from Tomales Bay indicate both that denitrification is NO−3 limited and that N2 fixation is inhibited by NO−3. The sensitivity of benthic N2 fixation and denitrification rates to changes in ambient DIN concentration in Tomales Bay has been replicated in manipulated core studies and observed in other benthic N cycling studies. For example, in the estuarine sands of Waquoit Bay, MA (Rao and Charette, 2012) documented net N2 fixation, and suggested that denitrification occurring elsewhere in the estuary removes DIN, permitting N2 fixation to proceed downstream. Similarly, in a study of N2 fixation rates associated with seagrass roots in a French estuary (Welsh et al., 1996) observed peak N2 fixation rates when ambient NH+4 concentrations reached their annual minima of 190 μM, relative to the peak concentration of 290 μM.
Many of these studies also document complex interactions between oxygen, DIN, and/or organic carbon, and their relationship with N2 fixation and/or denitrification rates in benthic environments. For example, (Fulweiler et al., 2007) attributed a change from net denitrification to net N2 fixation in Narragansett Bay, RI sediments to a decrease in the organic matter flux to the sediments due to diminished winter-spring blooms in the Bay. (Fulweiler et al., 2007) tested this hypothesis, observing a change from net N2 fixation to net denitrification after adding organic matter to incubated sediment cores that had previously shown net N2 fixation. In the past, benthic remineralization of winter-spring bloom material in Narragansett Bay provided a source of DIN to the sediment and overlying water column, which is nutrient-poor in summers; presumably the reduction in the magnitude of the organic matter flux to Narragansett Bay sediments corresponds to a reduced DIN flux to the sediments, and is proposed by Fulweiler et al. (2007) to be the cause of the switch to net N2 fixation from net denitrification.
Observations of decreased rates of benthic N2 fixation when ambient DIN concentrations increase, either because of runoff or remineralization, are generally consistent with the observations described above that show that DIN inhibits, but does not stop, pelagic diazotrophy. However, the observations of decreased benthic N2 fixation rates when DIN concentrations increase are not consistent with other observations of high rates of benthic N2 fixation in dark, NH+4-rich environments [e.g., (Haines et al., 1981; Capone, 1982; Welsh et al., 1996; Bertics et al., 2010)]. Some previous studies of benthic N2 fixation have suggested that oxygen and organic carbon availability also play a role in mitigating DIN inhibition (Yoch and Whiting, 1986; McGlathery et al., 1998). Another explanation for why N2 fixation may occur at considerable rates in DIN-rich benthic environments invokes a role for N2 fixation that is entirely different from providing a source of assimilatory N to the ecosystem. Specifically, there is evidence that in the presence of high concentrations of NH+4 benthic N2 fixation can serve as a sink for excess electrons to help bacteria achieve redox balance, especially in the absence of a viable Calvin–Benson–Bassham pathway (Joshi and Tabita, 1996; Tichi and Tabita, 2000). Ultimately, sensitivity studies of benthic diazotrophs to these parameters are limited by the lack of isolated diazotrophs for manipulative culture studies.
A better understanding of the sensitivities of the diverse suite of benthic diazotrophs to oxygen, organic carbon and DIN is critical for refining models of benthic N cycling, and in particular determining whether marine sediments are a net source or sink of fixed N to the marine environment. While marine sediments are normally considered a net sink for fixed N (Seitzinger, 1988), a variety of reports show that some benthic environments can be a net source of bioavailable N at least on seasonal timescales, if not annually, as well (Currin et al., 1996; Lee and Joye, 2006; Fulweiler et al., 2007; McCarthy et al., 2008). Moreover, if environmental conditions change to favor diazotrophy (e.g., Fulweiler et al., 2007), it is plausible that even if marine sediments do not overwhelmingly become a source of fixed N, they might at least not be as large of a sink as previously thought. Benthic N2 fixation deserves more attention as it is a poorly constrained term in the global marine N budget; the process is not always included in marine N budget estimates, although (Capone, 1983) estimated it may contribute 15 Tg N year−1, which would increase some estimates of N fluxes to the marine environment by 10–15% (Brandes and Devol, 2002).
Conclusions
Rates of the dominant fluxes of N to and from the ocean are highly uncertain, leaving open the question of whether the modern marine N budget is balanced. Some estimates suggest that rates of N fluxes to the ocean only compensate for one-third to one-half of the fluxes of N out of the ocean (Codispoti et al., 2001; Codispoti, 2007), while paleoceanographic and modeling studies require a balanced N budget, implying that either rates of N2 fixation are underestimated, and/or that rates of N loss are overestimated (Brandes and Devol, 2002; Deutsch et al., 2004). One potential liability in previous estimates of N fluxes to the ocean is the assumption that the highest rates of marine N2 fixation occur in the warm, nutrient-depleted regions of the surface ocean. However, culture and field evidence reviewed here indicates that low concentrations of NO−3 and/or NH+4 (≤1μM) are not a strict requirement for high rates of marine N2 fixation, and that numerical models using this as a criteria for significant diazotroph abundance and/or N2 fixation fluxes may not accurately represent diazotroph sensitivities to DIN. Generally, the best-studied cyanobacterial diazotrophs show little inhibition by short-term exposure to inorganic N. Instead, depressed rates of N2 fixation occur after long-term exposure of diazotrophs to elevated concentrations of DIN, although long-term exposure does not necessarily stop N2 fixation. Recent field and culturing work has shown that NO−3 concentrations commonly found in marine surface waters, i.e., up to 20 μM, do not preclude rates of N2 fixation comparable to those measured in the NO−3-depleted surface waters of the North Pacific gyre (Fernandez et al., 2011; Sohm et al., 2011). Moreover, field and culture evidence suggests that well-studied cyanobacterial diazotrophs such as Trichodesmium spp. are more tolerant of NO−3 than previously assumed, especially when P is relatively abundant. Together with molecular evidence documenting novel diazotrophs in cooler euphotic zone waters [e.g., (Needoba et al., 2007; Moisander et al., 2010)], these observations imply that surface waters other than those in the warm, nutrient-poor oligotrophic gyres may support substantial rates of N2 fixation, and that overlooking these potential diazotrophic contributions may compound uncertainties in the marine N budget, as well as modeled estimates of global marine diazotroph distributions and rates of N2 fixation.
While the nascent case for significant N2 fixation fluxes by mesopelagic diazotrophs is ambiguous, it is clear that N2 fixation occurs in diverse benthic environments at significant rates in the presence of DIN concentrations in excess of 100 μM. Benthic N2 fixation is peculiar in that it presents the strongest challenge to DIN inhibition of N2 fixation, and because it often occurs in environments that also support high rates of N loss via denitrification and/or anammox. While traditionally it has been thought that benthic environments represent a net loss of bioavailable N from the marine ecosystem, previous work has shown that the net flux of N2 gas to or from the sediments varies seasonally, and may be sensitive to environmental perturbations that may accelerate due to anthropogenic activities. These observations underscore the importance of developing and testing models of what controls benthic N2 fixation (and denitrification) to generate more robust estimates of benthic N fluxes.
Our current understanding of the sensitivity of even the most well studied marine diazotrophs is incomplete, and we have considerably more to learn about diazotrophs that have only recently been identified using molecular tools. These are critical uncertainties to resolve if we are to understand how the marine N inventory can remain balanced on 100–1000 year time scales. Better constraints of diazotroph sensitivities will help us understand N cycle changes in the past, and to predict future changes as atmospheric carbon dioxide concentrations and temperatures increase and potentially stimulate N2 fixation by Trichodesmium (Breitbarth et al., 2007; Hutchins et al., 2007; Levitan et al., 2007; Ramos et al., 2007; Levitan et al., 2010), if not other diazotrophs as well.
Conflict of Interest Statement
The author declares that the research was conducted in the absence of any commercial or financial relationships that could be construed as a potential conflict of interest.
Acknowledgments
D. A. Hansell and two reviewers provided comments that improved this manuscript. This work was funded by NSF grants OCE-0850905 and OCE-0933076.
References
An, S., and Joye, S. B. (2001). Enhancement of coupled nitrification-denitrification by benthic photosynthesis in shallow estuarine sediments. Limnol. Oceanogr. 46, 62–74.
An, S. M., Gardner, W. S., and Kana, T. (2001). Simultaneous measurement of denitrification and nitrogen fixation using isotope pairing with membrane inlet mass spectrometry analysis. Appl. Environ. Microbiol. 67, 1171–1178.
Berman-Frank, I., Cullen, J. T., Shaked, Y., Sherrell, R. M., and Falkowski, P. G. (2001). Iron availability, cellular iron quotas, and nitrogen fixation in Trichodesmium. Limnol. Oceanogr. 46, 1249–1260.
Bertics, V. J., Sohm, J. A., Magnabosco, C., and Ziebis, W. (2012). Denitrification and nitrogen fixation dynamics in the area surrounding an individual ghost shrimp (Neotrypaea californiensis) burrow system. Appl. Environ. Microbiol. 78, 3864–3872.
Bertics, V. J., Sohm, J. A., Treude, T., Chow, C. E. T., Capone, D. G., Fuhrman, J. A., et al. (2010). Burrowing deeper into benthic nitrogen cycling: the impact of bioturbation on nitrogen fixation coupled to sulfate reduction. Mar. Ecol. Prog. Ser. 409, 1–15.
Bombar, D., Moisander, P. H., Dippner, J. W., Foster, R. A., Voss, M., Karfeld, B., et al. (2011). Distribution of diazotrophic microorganisms and nifH gene expression in the Mekong River plume during intermonsoon. Mar. Ecol. Prog. Ser. 424, U39–U55.
Bothe, H., Klein, B., Stephan, M. P., and Dobereiner, J. (1981). Transformations of inorganic nitrogen by Azospirillum spp. Arch. Microbiol. 130, 96–100.
Brandes, J. A., and Devol, A. H. (2002). A global marine-fixed nitrogen isotopic budget: implications for holocene nitrogen cycling. Global Biogeochem. Cycles 16. doi: 10.1029/2001GB001856
Breitbarth, E., Oschlies, A., and Laroche, J. (2007). Physiological constraints on the global distribution of Trichodesmium–effect of temperature on diazotrophy. Biogeosciences 4, 53–61.
Breitbarth, E., Wohlers, J., Klaes, J., Laroche, J., and Peeken, I. (2008). Nitrogen fixation and growth rates of Trichodesmium IMS-101 as a function of light intensity. Mar. Ecol. Prog. Ser. 359, 25–36.
Brooks, R. H., Brezonik, P. L., Putnam, H. D., and Keirn, M. A. (1971). Nitrogen fixation in an estuarine environment–Waccasassa on Florida Gulf Coast. Limnol. Oceanogr. 16, 701–710.
Burns, J. A., Zehr, J. P., and Capone, D. G. (2002). Nitrogen-fixing phylotypes of Chesapeake Bay and Neuse River estuary sediments. Microb. Ecol. 44, 336–343.
Burris, R. H. (1976). Nitrogen fixation by blue-green algae of the lizard island area of the great barrier reef. Aust. J. Plant Physiol. 3, 41–51.
Capone, D. G. (1982). Nitrogen-fixation (acetylene-reduction) by rhizosphere sediments of the Eelgrass Zostera-Marina. Mar. Ecol. Prog. Ser. 10, 67–75.
Capone, D. G. (1983). “Benthic nitrogen fixation,” in Nitrogen in the Marine Environment, eds E. J. Carpenter and D. G. Capone (New York, NY: Academic Press), 105–137.
Capone, D. G., and Budin, J. M. (1982). Nitrogen-fixation associated with rinsed roots and rhizomes of the Eelgrass Zostera-Marina. Plant Physiol. 70, 1601–1604.
Capone, D. G., Burns, J. A., Montoya, J. P., Subramaniam, A., Mahaffey, C., Gunderson, T., et al. (2005). Nitrogen fixation by Trichodesmium spp.: an important source of new nitrogen to the tropical and subtropical North Atlantic Ocean. Global Biogeochem. Cycles 19. doi: 10.1029/2004GB002331
Capone, D. G., Oneil, J. M., Zehr, J., and Carpenter, E. J. (1990). Basis for diel variation in nitrogenase activity in the marine planktonic cyanobacterium Trichodesmium-Thiebautii. Appl. Environ. Microbiol. 56, 3532–3536.
Capone, D. G., Zehr, J. P., Paerl, H. W., Bergman, B., and Carpenter, E. J. (1997). Trichodesmium, a globally significant marine cyanobacterium. Science 276, 1221–1229.
Carpenter, E. J. (1983). “Nitrogen fixation by marine Oscillatoria (Trichodesmium) in the world's oceans,” in Nitrogen in the Marine Environment, eds D. G. Capone and E. J. Carpenter (New York, NY: Academic Press), 65–103.
Carpenter, E. J., Oneil, J. M., Dawson, R., Capone, D. G., Siddiqui, P. J. A., Roenneberg, T., et al. (1993). The tropical diazotrophic phytoplankter Trichodesmium - biological characteristics of 2 common species. Mar. Ecol. Prog. Ser. 95, 295–304.
Carpenter, E. J., Vanraalte, C. D., and Valiela, I. (1978). Nitrogen-fixation by algae in a massachusetts salt-marsh. Limnol. Oceanogr. 23, 318–327.
Chappell, P. D., and Webb, E. A. (2010). A molecular assessment of the iron stress response in the two phylogenetic clades of Trichodesmium. Environ. Microbiol. 12, 13–27.
Chen, Y. B., Dominic, B., Mellon, M. T., and Zehr, J. P. (1998). Circadian rhythm of nitrogenase gene expression in the diazotrophic filamentous nonheterocystous Cyanobacterium Trichodesmium sp strain IMS101. J. Bacteriol. 180, 3598–3605.
Church, M. J., Mahaffey, C., Letelier, R. M., Lukas, R., Zehr, J. P., and Karl, D. M. (2009). Physical forcing of nitrogen fixation and diazotroph community structure in the North Pacific subtropical gyre. Global Biogeochem. Cycles 23. doi: 10.1029/2008GB003418
Codispoti, L. A. (2007). An oceanic fixed nitrogen sink exceeding 400 Tg Na-1 vs the concept of homeostasis in the fixed-nitrogen inventory. Biogeosciences 4, 233–253.
Codispoti, L. A., Brandes, J. A., Christensen, J. P., Devol, A. H., Naqvi, S. W. A., Paerl, H., et al. (2001). The oceanic fixed nitrogen and nitrous oxide budgets: moving targets as we enter the anthropocene? Sci. Mar. 65, 85–105.
Currin, C. A., Joye, S. B., and Paerl, H. W. (1996). Diel rates of N-2-fixation and denitrification in a transplanted Spartina alterniflora marsh: implications for N-flux dynamics. Estuar. Coast. Shelf Sci. 42, 597–616.
Dekaezemacker, J., and Bonnet, S. (2011). Sensitivity of N-2 fixation to combined nitrogen forms (NO3- and NH4+) in two strains of the marine diazotroph Crocosphaera watsonii (Cyanobacteria). Mar. Ecol. Prog. Ser. 438, 33–46.
Dekas, A. E., Poretsky, R. S., and Orphan, V. J. (2009). Deep-sea archaea fix and share nitrogen in methane-consuming microbial consortia. Science 326, 422–426.
Deutsch, C., Sarmiento, J. L., Sigman, D. M., Gruber, N., and Dunne, J. P. (2007). Spatial coupling of nitrogen inputs and losses in the ocean. Nature 445, 163–167.
Deutsch, C., Sigman, D. M., Thunell, R. C., Meckler, A. N., and Haug, G. H. (2004). Isotopic constraints on glacial/interglacial changes in the oceanic nitrogen budget. Global Biogeochem. Cycles 18. doi: 10.1029/2003GB002189
Elser, J. J., Bracken, M. E. S., Cleland, E. E., Gruner, D. S., Harpole, W. S., Hillebrand, H., et al. (2007). Global analysis of nitrogen and phosphorus limitation of primary producers in freshwater, marine and terrestrial ecosystems. Ecol. Lett. 10, 1135–1142.
Falkowski, P. G. (1983). “Enzymology of Nitrogen Assimilation,” in Nitrogen in the Marine Environment, eds E. J. Carpenter and D. G. Capone (New York, NY: Academic Press), 839–868.
Fernandez, C., Farias, L., and Ulloa, O. (2011). Nitrogen fixation in denitrified marine waters. PLoS ONE 6:e20539. doi: 10.1371/journal.pone.0020539
Fu, F. X., and Bell, P. R. F. (2003). Factors affecting N-2 fixation by the cyanobacterium Trichodesmium sp GBR-TRLI101. FEMS Microbiol. Ecol. 45, 203–209.
Fujieki, L. A., Santiago-Mandujano, F., Lethaby, P., Lukas, R., and Karl, D. M. (2011). Hawaii Ocean Time-series Data Report 20: 2008, University of Hawaii, Honolulu, HI, USA.
Fulweiler, R. W., Nixon, S. W., Buckley, B. A., and Granger, S. L. (2007). Reversal of the net dinitrogen gas flux in coastal marine sediments. Nature 448, 180–182.
Gandy, E. L., and Yoch, D. C. (1988). Relationship between nitrogen-fixing sulfate reducers and fermenters in salt-marsh sediments and roots of Spartina-Alterniflora. Appl. Environ. Microbiol. 54, 2031–2036.
Garcia, H. E., Locarnini, R. A., Boyer, T. B., Antonov, J. I., Zweng, M. M., Baranova, O. K., et al. (2010). “Nutrients (phosphate, nitrate, silicate),” in World Ocean Atlas 2009, ed S. Levitus (Washington, DC: US Government Printing Office), 398.
Gardner, W. S., Mccarthy, M. J., An, S. M., Sobolev, D., Sell, K. S., and Brock, D. (2006). Nitrogen fixation and dissimilatory nitrate reduction to ammonium (DNRA) support nitrogen dynamics in Texas estuaries. Limnol. Oceanogr. 51, 558–568.
Grosse, J., Bombar, D., Hai Nhu, D., Lam Ngoc, N., and Voss, M. (2010). The Mekong River plume fuels nitrogen fixation and determines phytoplankton species distribution in the South China Sea during low- and high-discharge season. Limnol. Oceanogr. 55, 1668–1680.
Haines, J. R., Atlas, R. M., Griffiths, R. P., and Morita, R. Y. (1981). Denitrification and nitrogen-fixation in alaskan continental-shelf sediments. Appl. Environ. Microbiol. 41, 412–421.
Halm, H., Lam, P., Ferdelman, T. G., Lavik, G., Dittmar, T., Laroche, J., et al. (2012). Heterotrophic organisms dominate nitrogen fixation in the South Pacific Gyre. ISME J. 6, 1238–1249.
Hamersley, M. R., Turk, K. A., Leinweber, A., Gruber, N., Zehr, J. P., Gunderson, T., et al. (2011). Nitrogen fixation within the water column associated with two hypoxic basins in the Southern California Bight. Aquat. Microb. Ecol. 63, 193–205.
Haug, G. H., Pedersen, T. F., Sigman, D. M., Calvert, S. E., Nielsen, B., and Peterson, L. C. (1998). Glacial/interglacial variations in production and nitrogen fixation in the Cariaco Basin during the last 580 kyr. Paleoceanography 13, 427–432.
Hewson, I., Moisander, P. H., Achilles, K. M., Carlson, C. A., Jenkins, B. D., Mondragon, E., et al. (2007). Characteristics of diazotrophs in surface to abyssopelagic waters of the Sargasso Sea. Aquat. Microb. Ecol. 46, 15–30.
Holl, C. M., and Montoya, J. P. (2005). Interactions between nitrate uptake and nitrogen fixation in continuous cultures of the marine diazotroph Trichodesmium (Cyanobacteria). J. Phycol. 41, 1178–1183.
Hutchins, D. A., Fu, F. X., Zhang, Y., Warner, M. E., Feng, Y., Portune, K., et al. (2007). CO2 control of Trichodesmium N-2 fixation, photosynthesis, growth rates, and elemental ratios: Implications for past, present, and future ocean biogeochemistry. Limnol. Oceanogr. 52, 1293–1304.
Jayakumar, A., Al-Rshaidat, M. M. D., Ward, B. B., and Mulholland, M. R. (2012). Diversity, distribution and expression of diazotroph nifH genes in oxygen deficient waters of the Arabian Sea. FEMS Microbiol. Ecol. doi: 10.1111/j.1574-6941.2012.01430.x. [Epub ahead of print].
Joshi, H. M., and Tabita, F. R. (1996). A global two component signal transduction system that integrates the control of photosynthesis, carbon dioxide assimilation, and nitrogen fixation. Proc. Natl. Acad. Sci. U.S.A. 93, 14515–14520.
Joye, S. B., and Paerl, H. W. (1993). Contemporaneous nitrogen-fixation and denitrification in intertidal microbial mats - rapid response to runoff events. Mar. Ecol. Prog. Ser. 94, 267–274.
Joye, S. B., and Paerl, H. W. (1994). Nitrogen cycling in microbial mats–rates and patterns of denitrification and nitrogen-fixation. Mar. Biol. 119, 285–295.
Klausmeier, C. A., Litchman, E., Daufresne, T., and Levin, S. A. (2004). Optimal nitrogen-to-phosphorus stoichiometry of phytoplankton. Nature 429, 171–174.
Knapp, A. N., Dekaezemacker, J., Bonnet, S., Sohm, J. A., and Capone, D. G. (2012). Sensitivity of Trichodesmium and Crocosphaera abundance and N2 fixation rates to varying NO3- and PO43- concentrations in batch cultures. Aquat. Microb. Ecol. 66, 223–236.
Kustka, A. B., Sanudo-Wilhelmy, S. A., Carpenter, E. J., Capone, D., Burns, J., and Sunda, W. G. (2003). Iron requirements for dinitrogen- and ammonium-supported growth in cultures of Trichodesmium (IMS 101): comparison with nitrogen fixation rates and iron: carbon ratios of field populations. Limnol. Oceanogr. 48, 1869–1884.
Langlois, R. J., Huemmer, D., and Laroche, J. (2008). Abundances and distributions of the dominant nifH phylotypes in the Northern Atlantic Ocean. Appl. Environ. Microbiol. 74, 1922–1931.
Lee, R. Y., and Joye, S. B. (2006). Seasonal patterns of nitrogen fixation and denitrification in oceanic mangrove habitats. Mar. Ecol. Prog. Ser. 307, 127–141.
Lenes, J. M., Darrow, B. P., Cattrall, C., Heil, C. A., Callahan, M., Vargo, G. A., et al. (2001). Iron fertilization and the Trichodesmium response on the West Florida shelf. Limnol. Oceanogr. 46, 1261–1277.
Levitan, O., Brown, C. M., Sudhaus, S., Campbell, D., Laroche, J., and Berman-Frank, I. (2010). Regulation of nitrogen metabolism in the marine diazotroph Trichodesmium IMS101 under varying temperatures and atmospheric CO(2) concentrations. Environ. Microbiol. 12, 1899–1912.
Levitan, O., Rosenberg, G., Setlik, I., Setlikova, E., Grigel, J., Klepetar, J., et al. (2007). Elevated CO(2) enhances nitrogen fixation and growth in the marine cyanobacterium Trichodesmium. Glob. Change Biol. 13, 531–538.
Loladze, I., and Elser, J. J. (2011). The origins of the Redfield nitrogen-to-phosphorus ratio are in a homoeostatic protein-to-rRNA ratio. Ecol. Lett. 14, 244–250.
McCarthy, M. J., McNeal, K. S., Morse, J. W., and Gardner, W. S. (2008). Bottom-water hypoxia effects on sediment-water interface nitrogen transformations in a seasonally hypoxic, shallow bay (Corpus christi bay, TX, USA). Estuar. Coast. 31, 521–531.
McGlathery, K. J., Risgaard-Petersen, N., and Christensen, P. B. (1998). Temporal and spatial variation in nitrogen fixation activity in the eelgrass Zostera marina rhizosphere. Mar. Ecol. Prog. Ser. 168, 245–258.
Mehta, M. P., and Baross, J. A. (2006). Nitrogen fixation at 92 degrees C by a hydrothermal vent archaeon. Science 314, 1783–1786.
Mehta, M. P., Butterfield, D. A., and Baross, J. A. (2003). Phylogenetic diversity of nitrogenase (nifH) genes in deep-sea and hydrothermal vent environments of the Juan de Fuca ridge. Appl. Environ. Microbiol. 69, 960–970.
Mehta, M. P., Huber, J. A., and Baross, J. A. (2005). Incidence of novel and potentially archaeal nitrogenase genes in the deep Northeast Pacific Ocean. Environ. Microbiol. 7, 1525–1534.
Michaels, A. F., and Knap, A. H. (1996). Overview of the US JGOFS Bermuda Atlantic time-series study and the hydrostation S program. Deep Sea Res. Part II Top. Stud. Oceanogr. 43, 157–198.
Milligan, A. J., Berman-Frank, I., Gerchman, Y., Dismukes, G. C., and Falkowski, P. G. (2007). Light-dependent oxygen consumption in nitrogen-fixing cyanobacteria plays a key role in nitrogenase protection. J. Phycol. 43, 845–852.
Mohr, W., Grosskopf, T., Wallace, D. W. R., and Laroche, J. (2010). Methodological underestimation of oceanic nitrogen fixation rates. PLoS ONE 5:e12583. doi: 10.1371/journal.pone.0012583
Moisander, P. H., Beinart, R. A., Hewson, I., White, A. E., Johnson, K. S., Carlson, C. A., et al. (2010). Unicellular cyanobacterial distributions broaden the oceanic N(2) fixation domain. Science 327, 1512–1514.
Montoya, J. P., Holl, C. M., Zehr, J. P., Hansen, A., Villareal, T. A., and Capone, D. G. (2004). High rates of N-2 fixation by unicellular diazotrophs in the oligotrophic Pacific Ocean. Nature 430, 1027–1031.
Montoya, J. P., Voss, M., Kahler, P., and Capone, D. G. (1996). A simple, high-precision, high-sensitivity tracer assay for N-2 fixation. Appl. Environ. Microbiol. 62, 986–993.
Mulholland, M. R., and Capone, D. G. (1999). Nitrogen fixation, uptake and metabolism in natural and cultured populations of Trichodesmium spp. Mar. Ecol. Prog. Ser. 188, 33–49.
Mulholland, M. R., Ohki, K., and Capone, D. G. (1999). Nitrogen utilization and metabolism relative to patterns of N(2) fixation in cultures of Trichodesmium NIBB1067. J. Phycol. 35, 977–988.
Mulholland, M. R., Ohki, K., and Capone, D. G. (2001). Nutrient controls on nitrogen uptake and metabolism by natural populations and cultures of Trichodesmium (Cyanobacteria). J. Phycol. 37, 1001–1009.
Needoba, J. A., Foster, R. A., Sakamoto, C., Zehr, J. P., and Johnson, K. S. (2007). Nitrogen fixation by unicellular diazotrophic cyanobacteria in the temperate oligotrophic North Pacific Ocean. Limnol. Oceanogr. 52, 1317–1327.
Nielsen, L. B., Finster, K., Welsh, D. T., Donelly, A., Herbert, R. A., De Wit, R., et al. (2001). Sulphate reduction and nitrogen fixation rates associated with roots, rhizomes and sediments from Zostera noltii and Spartina maritima meadows. Environ. Microbiol. 3, 63–71.
Ohki, K., and Fujita, Y. (1982). Laboratory culture of the pelagic blue-green-alga Trichodesmium-Thiebautii–conditions for unialgal culture. Mar. Ecol. Prog. Ser. 7, 185–190.
Ohki, K., Zehr, J. P., Falkowski, P. G., and Fujita, Y. (1991). Regulation of nitrogen-fixation by different nitrogen-sources in the marine nonheterocystous Cyanobacterium Trichodesmium sp Nibb1067. Arch. Microbiol. 156, 335–337.
Orellana, M. V., and Hansell, D. A. (2012). Ribulose-1, 5-bisphosphate carboxylase/oxygenase (RuBisCO): a long-lived protein in the deep ocean. Limnol. Oceanogr. 57, 826–834.
Patriquin, D. (1978). “Nitrogen fixation (acetylene reduction) associated with cord grass, Spartina alterniflora Loisel,” in Environmental Role of Nitrogen-Fixing Blue-Green Algae and Asymbiotic Bacteria, ed U. Granhall (Stockholm: Ecological Bulletin), 20–27.
Patriquin, D., and Knowles, R. (1972). Nitrogen fixation in rhizosphere of marine angiosperms. Mar. Biol. 16, 49–58.
Prufert-Bebout, L., Paerl, H. W., and Lassen, C. (1993). Growth, nitrogen-fixation, and spectral attenuation in cultivated Trichodesmium species. Appl. Environ. Microbiol. 59, 1367–1375.
Ramos, J. B. E., Biswas, H., Schulz, K. G., Laroche, J., and Riebesell, U. (2007). Effect of rising atmospheric carbon dioxide on the marine nitrogen fixer Trichodesmium. Global Biogeochem. Cycles 21. doi: 10.1029/2006GB002898
Rao, A. M. F., and Charette, M. A. (2012). Benthic nitrogen fixation in an eutrophic estuary affected by groundwater discharge. J. Coast. Res. 28, 477–485.
Robson, R. L., and Postgate, J. R. (1980). Oxygen and hydrogen in biological nitrogen-fixation. Annu. Rev. Microbiol. 34, 183–207.
Rodier, M., and Le Borgne, R. (2008). Population dynamics and environmental conditions affecting Trichodesmium spp. (filamentous cyanobacteria) blooms in the south-west lagoon of New Caledonia. J. Exp. Mar. Biol. Ecol. 358, 20–32.
Rodier, M., and Le Borgne, R. (2010). Population and trophic dynamics of Trichodesmium thiebautii in the SE lagoon of New Caledonia. Comparison with T. erythraeum in the SW lagoon. Mar. Pollut. Bull. 61, 349–359.
Rueter, J. G., Ohki, K., and Fujita, Y. (1990). The effect of iron nutrition on photosynthesis and nitrogen-fixation in cultures of Trichodesmium (Cyanophyceae). J. Phycol. 26, 30–35.
Saito, M. A., Bertrand, E. M., Dutkiewicz, S., Bulygin, V. V., Moran, D. M., Monteiro, F. M., et al. (2011). Iron conservation by reduction of metalloenzyme inventories in the marine diazotroph Crocosphaera watsonii. Proc. Natl. Acad. Sci. U.S.A. 108, 2184–2189.
Sandh, G., Ran, L., Xu, L., Sundqvist, G., Bulone, V., and Bergman, B. (2011). Comparative proteomic profiles of the marine cyanobacterium Trichodesmium erythraeum IMS101 under different nitrogen regimes. Proteomics 11, 406–419.
Scott, T., Cotner, J., and Lapara, T. (2012). Variable stoichiometry and homeostatic regulation of bacterial biomass elemental composition. Front. Microbiol. 3:42. doi: 10.3389/fmicb.2012.00042
Seitzinger, S. P. (1988). Denitrification in fresh-water and coastal marine ecosystems–ecological and geochemical significance. Limnol. Oceanogr. 33, 702–724.
Slater, J., and Capone, D. G. (1984). Effects of metals on nitrogen-fixation and denitrification in slurries of anoxic saltmarsh sediment. Mar. Ecol. Prog. Ser. 18, 89–95.
Sohm, J. A., Hilton, J. A., Noble, A. E., Zehr, J. P., Saito, M. A., and Webb, E. A. (2011). Nitrogen fixation in the South Atlantic Gyre and the Benguela Upwelling System. Geophys. Res. Lett. 38. doi: 10.1029/2011GL048315
Stal, L. J., and Heyer, H. (1987). Dark anaerobic nitrogen-fixation (acetylene-reduction) in the cyanobacterium Oscillatoria sp. FEMS Microbiol. Ecol. 45, 227–232.
Stal, L. J. (2009). Is the distribution of nitrogen-fixing cyanobacteria in the oceans related to temperature? Environ. Microbiol. 11, 1632–1645.
Steppe, T. F., and Paerl, H. W. (2002). Potential N-2 fixation by sulfate-reducing bacteria in a marine intertidal microbial mat. Aquat. Microb. Ecol. 28, 1–12.
Steppe, T. F., and Paerl, H. W. (2005). Nitrogenase activity and nifH expression in a marine intertidal microbial mat. Microb. Ecol. 49, 315–324.
Tichi, M. A., and Tabita, F. R. (2000). Maintenance and control of redox poise in Rhodobacter capsulatus strains deficient in the Calvin-Benson-Bassham pathway. Arch. Microbiol. 174, 322–333.
Villareal, T. A., and Carpenter, E. J. (1990). Diel buoyancy regulation in the marine diazotrophic cyanobacterium Trichodesmium-Thiebautii. Limnol. Oceanogr. 35, 1832–1837.
Villareal, T. A., and Carpenter, E. J. (2003). Buoyancy regulation and the potential for vertical migration in the oceanic cyanobacterium Trichodesmium. Microb. Ecol. 45, 1–10.
Voss, M., Croot, P., Lochte, K., Mills, M., and Peeken, I. (2004). Patterns of nitrogen fixation along 10N in the tropical Atlantic. Geophys. Res. Lett. 31. doi: 10.1029/2004GL020127
Welsh, D. T., Bourgues, S., Dewit, R., and Herbert, R. A. (1996). Seasonal variations in nitrogen-fixation (acetylene reduction) and sulphate-reduction rates in the rhizosphere of Zostera noltii: nitrogen fixation by sulphate reducing bacteria. Mar. Biol. 125, 619–628.
Westberry, T., Behrenfeld, M. J., Siegel, D. A., and Boss, E. (2008). Carbon-based primary productivity modeling with vertically resolved photoacclimation. Global Biogeochem. Cycles 22. doi: 10.1029/2007GB003078
Westberry, T. K., and Siegel, D. A. (2006). Spatial and temporal distribution of Trichodesmium blooms in the world's oceans. Global Biogeochem. Cycles 20. doi: 10.1029/2005GB002673
Westberry, T. K., Siegel, D. A., and Subramaniam, A. (2005). An improved bio-optical model for the remote sensing of Trichodesmium spp. blooms. J. Geophys. Res. Oceans 110. doi: 10.1029/2004JC002517
White, A. E., Prahl, F. G., Letelier, R. M., and Popp, B. N. (2007). Summer surface waters in the Gulf of California: prime habitat for biological N-2 fixation. Global Biogeochem. Cycles 21. doi: 10.1029/2006GB002779
Yoch, D. C., and Whiting, G. J. (1986). Evidence for Nh4+ switch-off regulation of nitrogenase activity by bacteria in salt-marsh sediments and roots of the grass Spartina-Alterniflora. Appl. Environ. Microbiol. 51, 143–149.
Zehr, J. P., Bench, S. R., Carter, B. J., Hewson, I., Niazi, F., Shi, T., et al. (2008). Globally distributed uncultivated oceanic N(2)-fixing cyanobacteria lack oxygenic photosystem II. Science 322, 1110–1112.
Keywords: N2 fixation, diazotroph, inhibition, sensitivity, nitrate, ammonium
Citation: Knapp AN (2012) The sensitivity of marine N2 fixation to dissolved inorganic nitrogen. Front. Microbio. 3:374. doi: 10.3389/fmicb.2012.00374
Received: 08 July 2012; Accepted: 02 October 2012;
Published online: 19 October 2012.
Edited by:
Bess B. Ward, Princeton University, USAReviewed by:
Scott D. Wankel, Harvard University, USAShawn R. Campagna, University of Tennessee, Knoxville, USA
Copyright © 2012 Knapp. This is an open-access article distributed under the terms of the Creative Commons Attribution License, which permits use, distribution and reproduction in other forums, provided the original authors and source are credited and subject to any copyright notices concerning any third-party graphics etc.
*Correspondence: Angela N. Knapp, Rosenstiel School of Marine and Atmospheric Science, University of Miami, 4600 Rickenbacker Cswy, Miami, FL 33149, USA. e-mail: aknapp@rsmas.miami.edu