- 1Institute of Soil Ecology, Technical University Munich, Neuherberg, Germany
- 2Research Unit Environmental Genomics, Helmholtz Zentrum München, Neuherberg, Germany
- 3Institute of Ecology, Technical University Berlin, Berlin, Germany
- 4Agrosphere Institute, Forschungszentrum Jülich, Jülich, Germany
In a field experiment, the impact of repeated application of the antibiotic sulfadiazine (SDZ)-contaminated pig manure was assessed on functional microbial communities involved in ammonia and nitrite oxidation in the root-rhizosphere complexes (RRCs) of diverse plants composing a pasture. We surveyed the abundance of ammonia-oxidizing archaea (AOA) and bacteria (AOB) as well as Nitrobacter- and Nitrospira-like nitrite-oxidizing bacteria (NOB) by quantitative PCR (qPCR), and the diversity of amoA AOA and Nitrobacter-like nxrA amplicons using a cloning-sequencing approach. Whereas the first SDZ-contaminated manure application caused only slight effects on the investigated microbial communities and did not change the diversity and abundance pattern significantly, the second application of SDZ-contaminated manure induced an up to 15-fold increased ratio of AOA:AOB and a reduction of nrxA genes. The diversity of AOA amoA increased after the second application of SDZ-contaminated manure compared to the control treatment whereas a clear reduction of nrxA OTUs was visible in the same samples. The results indicate that the application of SDZ may principally affect nitrite oxidation by NOB and alternative pathways like nitrite reduction might be favored under these conditions.
Introduction
Nitrification rates in soils can be considered as an important indicator for sustainable use. Ammonium derived from ammonia is of high importance as the base product of this process, for plant nutrition and biomass formation. Catabolism and leaching of the end product, nitrate, often causes significant losses of nitrogen (N) as well as significant environmental problems such as contamination of groundwater by leaching, or the formation of the greenhouse gas N2O by denitrifying microbes (Ollivier et al., 2011). Nitrification is a two-step processes including (i) the oxidation of NH+4 to NO−2 via hydroxylamine by ammonia-oxidizing microbes (Kowalchuk and Stephen, 2001; Leininger et al., 2006) and (ii) the oxidation of NO−2 to NO−3 by nitrite-oxidizing bacteria (NOB) (Prosser, 1989). Ammonia oxidation and nitrite oxidation are both performed by phylogenetically well separated microorganisms. Ammonia oxidation is performed by autotrophic bacteria belonging to two specific groups of β- and γ-proteobacteria (AOB; Bock and Wagner, 2006) and probably mixotrophic archaea (AOA) recently assigned to the phylum Thaumarchaeota (Spang et al., 2010); NOB are broadly distributed among the α-, β-, γ-, and δ-proteobacteria as well as the Nitrospira phylum (Spieck and Bock, 2005). So far, no organism capable of performing both the oxidation of ammonia and the oxidation of nitrite has been identified (Schramm et al., 1996; Gieseke et al., 2003).
Despite the fact that an efficient nitrification requires the presence of both ammonia oxidizers and nitrite oxidizers, most studies in have focused on factors driving abundance, diversity and activity of ammonia oxidizers. ISI Web of Knowledge (http://www.webofknowledge.com/) reveals almost 2500 articles using the keywords “ammonia oxidation” and “soil,” whereas only 600 hits were found using “nitrite oxidation” and “soil.” Less than 30 peer-reviewed studies investigated both processes. The reason for this strong focus on ammonia oxidation is mainly related to several studies from the last century where the oxidation of ammonia has been considered as rate limiting for the whole process of nitrification (Prosser, 1989). Main findings from that time include varying copy numbers of AOB (Phillips et al., 2000) and nitrite concentrations below the detection limit in many soil samples (Burns et al., 1995), indicating that once nitrite is formed it can be quickly oxidized to nitrate. During that time, the existence of ammonia-oxidizing archaea (AOA) was not proven and ammonia oxidation in soil was thought related to some proteobacteria.
With the detection of AOA, the paradigm of nitrification changed and new questions have been raised concerning the role of AOA for nitrification, including (i) the transformation of hydroxylamine (NH2OH) to nitrite by AOA, since no homolog of bacterial HAO gene (hao) encoding the enzyme catalyzing the oxidation of NH2OH to NO−2 has been found in the genome of AOA (ii) the functional role of the described nirK sequences (encoding a putative nitrite reductase) from AOA (Bartossek et al., 2010) (iii) possible pathways indicating mixotrophy of AOA, and (iv) the functional interplay between AOA and NOB populations. Differences between AOA and AOB may result in dissimilarities in the response of these communities to inhibitive agents (Schauss et al., 2009). Therefore, the aim of this study was to investigate the response of ammonia (AOA and AOB) and nitrite oxidizers (NOB) to a repeated application of antibiotics (sulfadiazine; SDZ) in the root rhizosphere complex (RRC) of a mixture of typical grassland plant species at different plant development stages during the growing season in a field study, and thus to link the data to ammonium and nitrate fluxes in soil. In order to assess changes in the genetic potential, we analyzed the abundance pattern of genes encoding key enzymes of ammonia oxidation (amoA encoding the ammonia monooxygenase) in both AOB and AOA, and nitrite oxidation in Nitrobacter-like NOB (nxrA encoding the nitrite oxidoreductase). Nitrospira-like NOB were quantified based on analysis of 16S rRNA genes. Moreover, the functional diversity of archaeal amoA and Nitrobacter-like nxrA was determined using a cloning-sequencing approach.
As the administration of antibiotics to treat infectious diseases is a common practice in animal husbandry, substances like the sulfonamide, which is mainly used in pig production (Burkhardt et al., 2005), are poorly adsorbed by the animal and excreted mostly unaltered in urine and feces together with various metabolites (Elmund et al., 1971; Alcock et al., 1999; Halling-Sørensen, 2001; Lamshöft et al., 2007), and thus reach the soil ecosystem via manuring. By inhibiting folic acid metabolism, SDZ impairs growth of most Gram-positive and many Gram-negative bacteria (Brown, 1962). The occurrence of SDZ in soil might therefore alter the microbial community structure as well as its activity, and modify kinetics of important turnover processes such as nitrogen (N) cycling. We hypothesized that SDZ mainly affects the ratio of archaeal vs. bacterial ammonia oxidizers, whereas Nitrospira- and Nitrobacter-like nitrite oxidizers are both inhibited by the application of the contaminated manure, despite their different phylogenetic classification. Therefore, under antibiotic pressure, we postulate that nitrite oxidation might be the rate limiting step of nitrification.
Materials and Methods
Experimental Design
An agricultural field located near Merzenhausen, Germany (50° 56′ 3″ N, 6° 17′ 31″ E) which only received inorganic fertilizer within the last 30 years and therefore has not been in contact with manure derived antibiotics during this period, was chosen as experimental site. The management included a cropping sequence consisting of potatoes, wheat, barley, sugar beets, and pasture The soil has been characterized as a silt loam (pH 7.2, Orthic Luvisol; Table 1). The experiment was setup as a randomized split plot design (plots size: 3 × 6 m) with a mixture of pasture plants (47% Lolium perenne, 17% Phleum pratense, 20% Festuca pratensis, 10% Poa pratensis, and 6% Trifolium repens) using manure from untreated pigs (PM) and SDZ-treated pigs (PMSDZ), respectively, with four replicates for each variant providing height plots in total.
Manure was applied twice during the vegetation season (applied total N ranged from 16 to 19 g N m−2 and from 3 to 6 g N m−2, respectively; Table 2). The first manure application (30 m3 ha−1) was completed in May 2009. The second application (10 m3 ha−1) occurred 48 d later. Pasture plots were cut one day prior to the second manure application. The amount of SDZ applied in treatments with PMSDZ was equivalent for each of the two applications to 100 mg SDZ m−2. The amount of the CaCl2-extractable SDZ recovered from soil during the experimental period declined quickly after each manure application (Rosendahl et al., 2011).
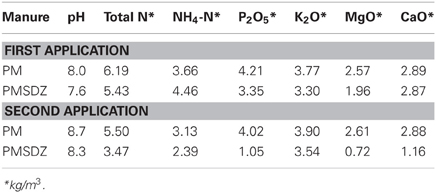
Table 2. Chemical characterization of the pig manure (PM) and SDZ-contaminated pig manure (PMSDZ) applied in the experiment.
Rhizosphere samples were collected from all plots at day 1 (after the first application of the manure) 7, 14, and 42 d; 49, 56, 63, and 106 d (1, 8, 15, and 58 d after the second manure application, respectively). For each plot, 10 subsamples were randomly collected, mixed, and homogenized to obtain one composite sample per plot. Composite samples taken from plots with the same treatments were considered replicates. After shaking the roots vigorously, the RRC samples (roots and adhering soil) were divided equally into two sub-samples. One part was immediately shock-frozen in liquid nitrogen and stored at −80°C prior to DNA extraction; the other part was directly extracted with 0.01 M CaCl2 for determination of ammonium-N (NH+4-N) and nitrate-N (NO−3-N) concentrations.
Inorganic Nitrogen Fraction in the RRC
RRC (300 mg) was agitated using an overhead shaker for 30 min with 5 ml of 0.01 M CaCl2. After filtration, ammonium-N and nitrate-N measurements were performed on Nanocolor 300D photometer from Macherey Nagel (Germany) by using the Nanocolor Ammonium 3 kit and the Nanocolor nitrate 50 kit, respectively (Macherey Nagel, Germany), according to the manufacturer's suggested protocols.
Nucleic Acid Extraction
RRC DNA was directly extracted after a bead beater lysis step (Bertin Technologie, France), using the FastDNA SPIN kit for soil according to the manufacturer's suggested protocol (MP biomedicals, Germany). Quality and quantity of the extracted DNA were checked with a spectrophotometer (Nanodrop, PeqLab, Germany).
Abundance of Functional Genes
Quantitative PCR (qPCR) of genes encoding key enzymes of ammonia oxidation (amoA encoding the ammonia monooxygenase) in both ammonia-oxidizing bacteria (AOB) and AOA, and nitrite oxidation in Nitrobacter-like NOB (nxrA encoding the nitrite oxidoreductase) was used to determine the density of the functional communities involved in nitrification. In addition, the abundance of Nitrospira-like NOB was quantified using the 16S rRNA Nitrospira gene as target since no primers targeting Nitrospira-like nxrA were available (Wertz et al., 2012). An absolute quantification of all investigated target genes using a SYBR® Green I-based detection (Applied Biosystems, Germany) was carried out in 25 μL in triplicates on the ABI Prism 7300 Cycler (Applied Biosystems). The reaction mixture consisted of 15 μg bovine serum albumin (Sigma-Aldrich, Germany), 0.2 μM of each primer for amoA AOA, nxrA, and 16S rRNA Nitrospira gene amplification and 0.3 μM of each primer for amoA AOB amplification, respectively (Metabion, Germany), 1× Power SYBR Green PCR master mix (Applied Biosystems), and 40 ng DNA template. All PCR reactions started with an initial enzyme activation step performed at 95°C for 10 min. The subsequent thermal profile was different for each gene amplified (Table 3). The specificity of the amplification products was confirmed by melting-curve analysis and migration on 2% agarose gel. No template controls gave null or negligible values. To avoid inhibitory effects on qPCR, samples were diluted 10-fold based on a pre-experiment (data not shown). Dilution series of a plasmid with cloned Nitrosomonas multiformis ATCC25196 amoA gene (amoA AOB), the fosmid clone 54d9 (Leininger et al., 2006) for archaeal amoA, Nitrobacter hamburgensis X14 (DSMZ 10229) nxrA gene (Nitrobacter-like nxrA), and the Nitrospira 16S rRNA gene (Accession No. FJ529918; Nitrospira-like 16S rRNA gene), were used to generate respective standard curves ranging from 101 to 106 gene copies μl−1 with efficiencies ranging from 94 to 98%, 98% to 100%, 93 to 98%, and 93% to 99%, respectively.
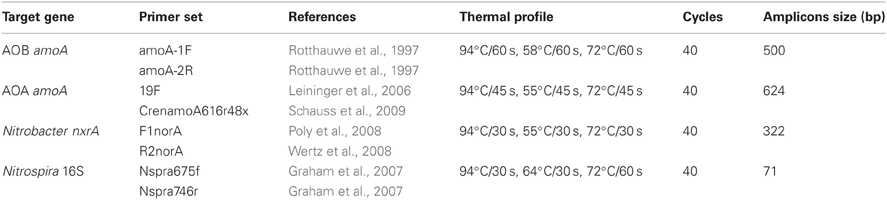
Table 3. Primers and thermal profiles used for real-time PCR quantification of bacterial and archaeal amoA, Nitrobacter-like nxrA, and Nitrospira-like 16S rRNA gene.
Cloning and Sequencing of Archaeal amoA and Nitrobacter-Like nxrA Fragments Sequences and Phylogenetic Analysis
Prior to PCR amplification, replicates corresponding to the treatments PM and PMSDZ 1 d, 49 d and 106 d after the first manure application were pooled together to constitute one sample corresponding to one treatment at one time point.
Archaeal amoA and nxrA gene amplicons were generated by PCR using the primers described for qPCR assay (Table 3). The reaction mixture (50 μL) contained 1× PCR buffer, 1× CoralLoad concentrate, 1× Q-solution, 1 U TopTaq (Qiagen, Germany), 200 μM of each dNTP, 0.2 μM of each primer, and 30 ng template DNA. The PCR thermocycling program for nxrA amplification was 94°C for 3 min, followed by 35 cycles of 94°C for 30 s, 55°C for 30 s and 72°C for 1 m, and a final elongation step at 72°C for 10 min. A similar program was used for amoA AOA amplification with an annealing temperature of 50°C. The cloning was carried out using the TA cloning kit (Invitrogen, Germany) in accordance with the manufacturer's instructions. Thirty clones were picked randomly for each treatment and time point. Plasmids were extracted using the NucleoSpin plasmid kit (Machery-Nagel, Germany). Inserts from clones amplified with specific primers (M13 forward and M13 reverse) using the BigDye Terminator cycle sequencing kit (Applied Biosystems) were purified by ethanol precipitation. amoA AOA and nxrA fragments were sequenced using an ABI 3730 DNA analyzer (Applied Biosystems). Sequences were run through a mega BLAST search (http://blast.ncbi.nlm.nih.gov/Blast.cgi) using the nr database and were deposited in the Genbank with the accession numbers KC137376-KC137546 and KC152658-KC152839 for amoA and nxrA, respectively. For further analysis nucleotide sequences were transcribed to aminoacid sequences. These were aligned using clustal W protein alignment (Thompson et al., 1994) implemented in ARB (Ludwig et al., 2004). The nucleotide sequences were realigned according to aligned protein sequences. DNA-based maximum likelihood trees were reconstructed applying PhyML (Guindon and Gascuel, 2003) implemented in ARB. Rarefaction curves were created using Mothur for a distance of 0.02 (98% similarity level) (Schloss et al., 2009). The clustering of amoA AOA was done according to the method of Pester et al. (2012).
Statistical Analysis
Inorganic nitrogen and gene abundance data were analyzed by two-way analysis of variance (ANOVA) with treatment (PM, PMSDZ) and time (after the first [1 d, 7 d, 14 d, and 42 d] and the second [49 d, 56 d, 63 d, and 106 d] manure application) as independent factors. In addition, the Dunnett-T3 was used as a post-hoc test. Homogeneity of the variances was checked by the Levene test. The significance was set to α = 0.05. Prior to analysis, gene abundance data were ln-transformed to achieve normal distribution. Independent T-tests were used to test for a significant difference between the two treatments at a given time point with significance level corrected by the Šidák's equation to α = 1 − (1 − 0.05)1/4 = 0.013 (Šidák, 1967), as each manure application was followed by four sampling time points. Statistical tests were calculated in SPSS 11.5 (SPSS, Inc., IL, USA).
Results
Inorganic Nitrogen
The highest NH+4-N concentrations were measured at day 1, independent from the treatment (up to 22.8 μg NH+4-N per gram of dry weight RRC). Significant time-dependent differences were detected after the two applications of manure (Table A1). Only one week later the values dropped to below 10 μg NH+4-N g−1 RRC in the PM treatments and below 5 μg NH+4-N g−1 RRC in the PMSDZ treatments. However, differences between the two treatments were not significant. This level remained constant during the experimental period; the second manure application at day 49 did not influence NH+4-N concentrations in the RRC (Figure 1).
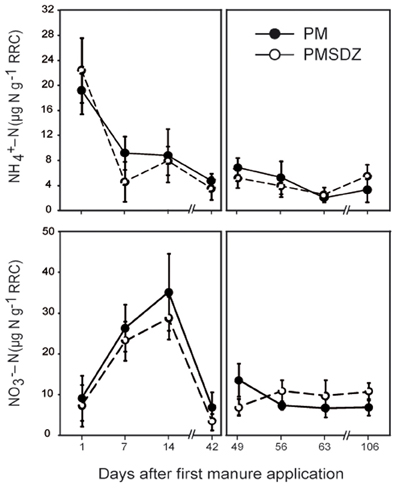
Figure 1. Ammonium and nitrate concentrations in the root-rhizosphere complex (RRC) of pasture plants after addition of pig manure (PM; full circles, •) or SDZ-contaminated pig manure (PMSDZ; empty circles, ◦) 1 d, 7 d, 14 d, and 42 d after primary manure application. Also included are measurements at 49 d, 56 d, 63 d, and 106 d post primary application, made after second manure application occurring 48 d post primary application. Error bars represent standard deviation of mean of replicate samples (n = 4). Abbreviations: RRC, root-rhizosphere complex.
Time had a significant effect on NO−3-N concentrations after the first manure application (Table A1). NO−3-N concentrations peaked independently from the antibiotic treatment 14 d after the first application of manure (up to 35.4 μg NO−3-N g−1 RRC). Toward day 42, the values dropped sharply and were close to the detection limit in some of the replicates. Similarly to the observation regarding NH+4-N concentrations, the second manure application had no effect on the NO−3-N concentrations in the RRC independent of the treatment (Figure 1).
Gene Abundance
The first application of manure did not influence amoA gene copy numbers for AOA and AOB 1, 7, and 14 d after application (Figure 2, Table A2). Higher copy numbers (in the range of 6.5 × 107 copy numbers per gram of dry weight RRC) were measured for AOB; AOA copy numbers were at the same time points were slightly lower (in the range of 2.5 × 107 copies g−1 RRC) resulting in an AOA:AOB ratio of 0.4. No significant influence of SDZ was visible at these time points either on AOA or AOB. However, 42 d after application, AOB amoA gene copy numbers in the treatments with control manure (PM) increased up to 1.8 × 108 copies g−1 RRC, whereas in the plots where contaminated manure (PMSDZ) was applied no changes were visible compared to the earlier time points. AOA amoA gene abundance did not differ 42 d after application of the manure compared to the earlier time points. Therefore, at this time point AOA:AOB ratio in the PM treatment was the lowest measured (0.1). After the second manure application amoA AOA and AOB copy numbers in the RRC of plants from the PM treated plots were comparable, as amoA AOA copy numbers increased compared to earlier sampling time points (ratio AOA:AOB = 1). A clear influence of the antibiotic was visible on both AOA and AOB at all time points investigated. Copy numbers for amoA AOB decreased in PMSDZ plots compared to the control samples. Thus, values were in the range of 2 × 107 gene copies g−1 RRC in PMSDZ plots compared to 7 × 107 g−1 RRC in the plots treated with PM. In contrast AOA amoA gene copy numbers increased significantly in the RRC of plants in PMSDZ-treated plots compared to control plots, with gene copy numbers in the range of 3 × 108 copies g−1 RRC, whereas copy numbers in the control treatment were approximately 1 × 108 copies g−1 RRC. Interestingly, the described effect was stable at day 106. Consequently, AOA:AOB ratio increased up to 15 after the second application of SDZ-contaminated manure.
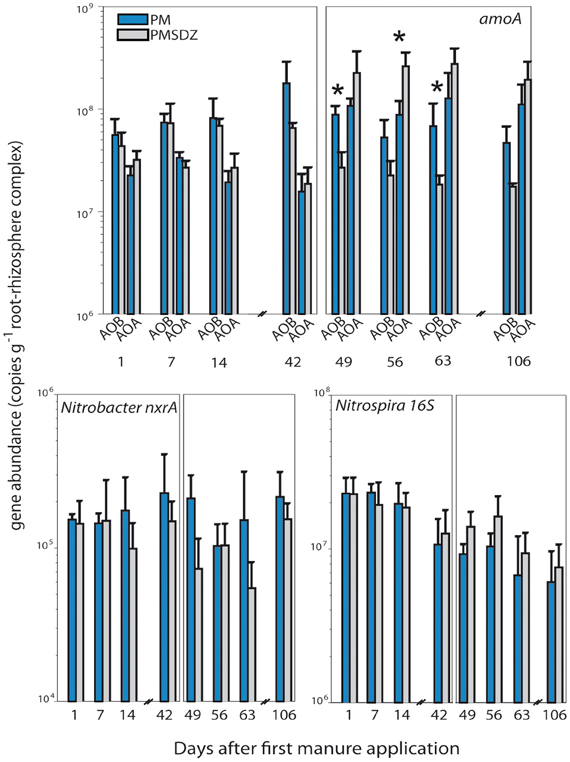
Figure 2. Quantification of amoA (AOB and AOA), Nitrobacter-like nxrA and Nitrospira-like 16S rRNA genes in the root-rhizosphere complex (RRC) of pasture plants after addition of pig manure (PM, blue bars) or SDZ-contaminated pig manure (PMSDZ, gray bars) 1 d, 7 d, 14 d, and 42 d after primary manure application. Also included are measurements at 49 d, 56 d, 63 d, and 106 d post primary application, made after second manure application occurring 48 d post primary application. Significant differences between the two treatments at a particular time point are indicated by asterisks (*) for each investigated gene. Error bars represent standard deviation of mean (n = 4). Abbreviations: RRC, root-rhizosphere complex.
Copy numbers for 16S rRNA genes from Nitrospira ranged from 6.1 × 106 to 2.3 × 107 gene copies g−1 RRC during the experimental period (Figure 2). The highest gene copy numbers were measured after the first manure application at time point 1 d. The lowest gene copy numbers were detected at the end of the experimental period at day 106. The second manure application did not increase 16S rRNA gene copies of Nitrospira. Surprisingly SDZ had no significant influence on the abundance of Nitrospira over the experimental period. The abundance of nxrA genes from Nitrobacter were 2 orders of magnitude lower compared to 16S rRNA gene copy numbers of Nitrospira. Over all time points, none of the two manure applications changed the nxrA gene abundance significantly. Copy numbers ranged between 1.0 × 105 and 2.3 × 105 copies g−1 RRC in PM-treated plots. We observed a tendency for reduced gene copies 49 d after the first application of the PMSDZ compared to the control PM treatment group (P = 0.030).
Diversity of AOA amoA and Nitrobacter-Like nxrA Genes
The clones per library sequenced for each treatment did not account for the total diversity of AOA amoA OTUs present in the samples as indicated by the rarefaction curves (Figure 3). The total number of OTUs for amoA AOA varied from 12 to 14 in the PM-treated plots, independent from the time point of sampling. In contrast in the PMSDZ treatment, the number of OTUs decreased between day 1 and day 49, and thereafter increased between day 49 and day 106 after the first application. Overall, 85% of the sequences sampled were contained in Nitrosphaera subclusters 1, 4, and 9; and in the Nitrosphaera cluster (soil metagenome fragment 54d9). Nitrosphaera subclusters 1 and 4 were represented by sequences from all treatments and time points. However, in Nitrosphaera subcluster 9, the abundance of sequences from the treatment PMSDZ one day after the second manure application (day 49) was higher compared to PM. In contrast, in the Nitrosphaera cluster (soil metagenome fragment 54d9), at the 106-d point, the abundance of sequences from the control treatment was higher compared to PMSDZ (Figures 4, A1).
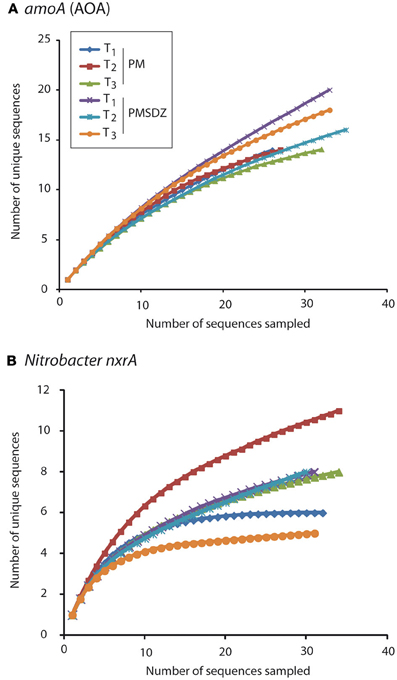
Figure 3. Rarefaction curves for amoA AOA (A) and Nitrobacter-like nxrA (B) sequences obtained at three different time points (1 d, T1; 49 d, T2; and 106 d, T3) after application of pig manure (PM) or SDZ-contaminated pig manure (PMSDZ). The curves express the number of OTUs as a function of the number of the sequences sampled in each library.
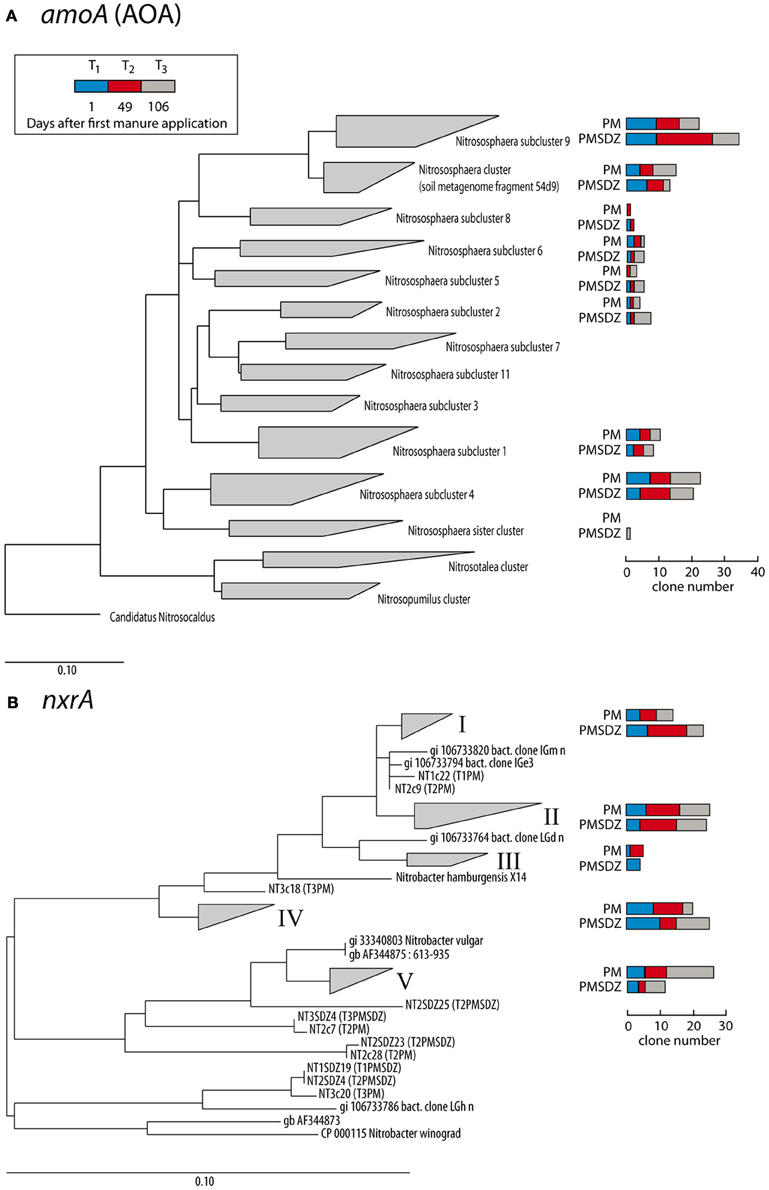
Figure 4. Maximum likelihood trees of partial amoA AOA (A) and Nitrobacter-like nxrA (B) nucleic acid sequences (624 and 322 bp, respectively) obtained at three different time points (1 d, blue bars; 49 d, red bars; and 106 d, gray bars) after application of pig manure (PM) or SDZ-contaminated pig manure (PMSDZ) and reference sequences. AOA amoA-clustering was performed according to Pester et al. (2012). Ungrouped trees are available in Figures A1 and A2.
The number of different OTUs for nrxA was lower compared to AOA amoA. Therefore, the investigated 30 clones per library largely reflected the diversity present in the samples and the collector's curves indicated saturation. The total number of OTUs for nrxA ranged between 4 and 11. However, the observed diversity pattern followed the opposite trend compared to AOA amoA. The highest number of OTUs was observed in the treatments with control manure one day after the second application (day 49). The lowest number of OTUs was observed at the last sampling time point (day 106) in the PMSDZ-treated plots. However, the number of OTUs was already reduced at this time point also in the control samples compared to day 49 (Figure 3). Four major clusters were detected, containing 89% of the total nxrA sequences sampled (I, II, IV, and V; Figures 4, A2). Each individual cluster was represented by sequences from both treatments and all three time points. In the treatment where contaminated manure was added, the relative abundance of sequences 1 d after the second application of the manure was higher in cluster I vs. the control treatment. A similar trend was observed for the last sampling time point (day 106) for cluster IV. An opposite trend was observed in cluster V, where a higher number of sequences was found from the control treatment at the last sampling time point (day 106).
Discussion
Effects on Ammonia Oxidizers
The antibiotic tended to abolish the increase of the abundance of AOB after 42 d in response to the manure application in RRC of the pasture plants (P = 0.091) (Figure 2). Similar results have been reported in analyses of bulk soil (Schauss et al., 2009) and in the rhizosphere of agricultural crops (Ollivier et al., 2010). These results demonstrate that SDZ inhibits the growth of AOB populations (considering gene abundance as proxy). The response of bacterial and archaeal ammonia oxidizers, respectively, differed one day after the second SDZ-contaminated manure application. From this time point onward, the amoA AOB and AOA gene abundance patterns were decreased and increased, respectively (Figure 2). Although it has been already shown that AOA were impacted to a lower extent by SDZ than AOB in greenhouse experiments (Schauss et al., 2009; Ollivier et al., 2010), in this study we observed for the first time a significant increase in amoA AOA gene copies (P = 0.005 at day 56) while amoA AOB gene copies were significantly decreased (P = 0.005 and P = 0.013 at day 49 and 63, respectively) with PMSDZ treatment, confirming the potential occurrence of functional redundancy between the two communities under antibiotic stress (Schauss et al., 2009). However, caution must be taken when relating significant impacts of the antibiotic on the gene level to associated functions. Indeed, whereas SDZ has been shown to affect corresponding gene expression to a lower extent, Ollivier et al. (2010) suggested that microbial subpopulations intrinsically able to cope with the antibiotic stressor could take advantage of the altered competitive environment and maintain the function.
Possibly, the reduced susceptibility of AOA to PMSDZ can be explained by a shift in the AOA diversity toward more SDZ tolerant phylotypes over time. In this respect Nitrosphaera subcluster 9 might be of interest: whereas the number of clones which could be assigned to this cluster did not differ between both treatments 1 d after the first application of manure, 1 d after the second application of manure, an increased number of clones derived from PCR products obtained from the PMSDZ-treated soil samples showed high similarities to this subcluster (Figure 4). However, it should be noted that the development of antibiotic resistance in AOA has not been described so far, as the first cultures of soil AOA have been isolated only recently (Jung et al., 2011; Tourna et al., 2011; Kim et al., 2012).
Effects on Nitrite Oxidizers
Because of the broad-spectrum nature of the SDZ antibiotic, we hypothesized that Nitrospira- and Nitrobacter-like nitrite oxidizers are both inhibited by the application of the contaminated manure. However, parallel to the inhibitory effects directly affecting the functional communities investigated, e.g., related to their respective activity status and related susceptibility (Lewis, 2007; Ollivier et al., 2010) or to their abilities to regulate their internal pH, which affect the accumulation and speciation of the SDZ in the cells (Tappe et al., 2008; Zarfl et al., 2008), effects that can be considered as indirect and potentially related to other nutrient cycles than nitrogen turnover, food web development, susceptibility to phages or changes in soil physicochemical properties (e.g., changed redox conditions due to an overall reduced activity of the soil microflora in response to the antibiotic) may explain their response to the antibiotic stress. While niche differentiation and competition is known to influence the composition of functional microbial communities, the components of the nitrite-oxidizing communities investigated, respectively, in this study have been reported to possess different substrate affinities and therefore are adapted to distinct N availabilities (Schramm et al., 1999). Thus, it has been suggested that Nitrobacter-like NOB bacteria are r-strategists with higher growth rate/specific activity and lower affinity for nitrite and oxygen, whereas Nitrospira-like NOB are K-strategists with a higher substrate affinity (Schramm et al., 1999; Attard et al., 2010). However, Maixner et al. (2006) have shown that the nitrite concentration influences the structure of Nitrospira-like bacterial communities, and assumed that sublineages may occupy different positions on a scale reaching from K- to r-strategists within the genus Nitrospira. In the RRC of the pasture plants from 42 days after the first manure application, the reduction of AOB abundance and activity may have resulted in lower nitrite availability and consequently favorable conditions for Nitrospira-like NOB compared to Nitrobacter-like NOB, explaining the reduction of Nitrobacter-like nxrA abundance (P = 0.030) and the increase of Nitrospira 16S rRNA gene abundance (P = 0.036) at day 49 with PMSDZ treatment. Moreover, whereas nitrite reductase homologues have been reported in AOA (Bartossek et al., 2010), the increase in abundance of AOA could have further decreased nitrite availability, and consequently collapsed the niche of Nitrobacter-like NOB. However, this would require changes in the ecophysiology of AOA (from autotrophic to heterotrophic lifestyle) and shifts in the redox potential at the present microsites toward oxygen limitation. Furthermore, a putative disturbance of the carbon cycle under the antibiotic treatment could have also indirectly influenced the abundance of different Nitrospira sublineages as some Nitrospira-like bacteria are mixotrophic (Daims et al., 2001).
A community shift due to SDZ treatment was observed for Nitrobacter-like NOB at the 49-d point in cluster I. Moreover, the antibiotic treatment had a long-lasting effect on these communities as differences between the two communities, specifically in the relative abundance for cluster IV and V, were observed 106 d after the first application of manure, as well as a decrease in diversity (Figure 3). Therefore, whereas SDZ-resistant phylotypes might have developed in response to the antibiotic stress, there was no recovery of microbial diversity after the PMSDZ treatment during the experimental period. Possibly, critical nitrifying consortia might have been disturbed owing to the antibiotic stress, which may imply negative effects on the ability of the soil to respond to future disturbances (Ives et al., 2000; McCann, 2000). Furthermore, the observed changes in Nitrobacter-like NOB diversity could be indirectly explained by the increased AOA abundance, as mentioned above.
Consequences for Nitrification and N Turnover
This field experiment revealed that the application of manure contaminated with the antibiotic SDZ has a lasting effect on the abundance and diversity of nitrifying microbial communities. However, although SDZ impaired the growth of certain microbial populations, there was no significant effect of the PMSDZ treatment on the concentrations of nitrate and ammonium in the rhizosphere of the plants composing the pasture. Differences in tolerance between the different microbial functional communities, or the development of tolerant populations (Heuer et al., 2011) could contribute to functional redundancy of ammonia-oxidizing microbes (Schauss et al., 2009) and NOB, and therefore to the stability of nitrification pattern in the rhizosphere. However, the studied system was not shown to recover in terms of nxrA diversity after the PMSDZ treatment during the experimental period, which could indicate that mostly nitrite oxidation by NOB might be affected by the application of SDZ. Possibly, alternative pathways like nitrite reduction might be favored under these conditions; as many soil microbes are able to use nitrite as terminal electron acceptors under anaerobic conditions, the extent of the SDZ-contamination effects on denitrification may be reduced.
Potential Pitfalls of this Study
On the first glance, one might speculate that ammonia oxidation is more resilient and influenced to a limited extent by the antibiotic due to functional redundancy of AOA and AOB. However, this study only gives data on the abundance of genes (genetic potential) and does not take into account enzymes, which drive the particular process. Therefore, additional proteomic based approaches might be needed to clarify if indeed functional redundancy exists and AOA actually takes over the capability to oxidize ammonia from AOB. Furthermore, while AOB gene abundance might be at least a proxy for ammonia oxidation activity (as an increase in abundance of AOB requires the oxidation of ammonia due to their purely autotrophic lifestyle), this is different for AOA, where also heterotrophic growth, independent from ammonia oxidation, could be proven by using urea as alternative nitrogen source (Tourna et al., 2011). Therefore, in the case of AOA, changes in the abundance are not necessarily linked to ammonia oxidation.
Another issue of concern for data interpretation is the difference in quality between the manure produced by animals that were treated with antibiotics and control animals. It is well known that antibiotics change the physiology of mammals and therefore differences in the composition of the manure would not be surprising (Antunes et al., 2011). Thus, it cannot be completely excluded that some of the observed differences in response to the second application of the antibiotic are related to the slight differences in the nutrient composition of the manure. However, spiking the manure with SDZ would also result in a biased picture, as important metabolites of SDZ are only formed during the passage through the body of the pigs (Lamshöft et al., 2007).
Outlook
In this study, the rhizosphere as an important soil compartment has been investigated. However, the type of response of the targeted functional communities might be differing in other soil compartments, e.g., the drilosphere where microbes form different types of consortia and produce extracellular polymeric substances (EPS) in different amounts (Brown et al., 2000), which might reduce the effects of the antibiotic compared to the rhizosphere. Of course, the soil type and the environmental conditions present at the site may also highly influence the response pattern of the microflora toward antibiotics, as well as the land use history of the soil (essentially the repeated application of manure contaminated with antibiotics) in the years before the experiment started (Berg and Smalla, 2009; Heuer et al., 2011; Rosendahl et al., 2011). These aspects may be considered in a study where attempts are made to generalize the obtained data. Moreover, whereas plant diversity and species composition are known to influence the magnitude and the stability of ecosystem processes over time, as well as the size and composition of associated microbial communities (Hooper and Vitousek, 1997; Kowalchuk et al., 2002; Steenwerth et al., 2002; Johnson et al., 2003; Balvanera et al., 2006; Millard and Singh, 2010), the question of how interactive effects between the antibiotic and plant diversity might influence microbial communities needs to be investigated into further experiments. Most important, however, will be the use of proteomic-based approaches to link the genetic potential of a soil to turnover processes in order to unveil the secrets of “functional redundancy.”
Conflict of Interest Statement
The authors declare that the research was conducted in the absence of any commercial or financial relationships that could be construed as a potential conflict of interest.
Acknowledgments
This work was part of the German Research Foundation (DFG) research group (FOR 566) “Veterinary Medicines in Soils: Basic Research for Risk Analysis.”
References
Alcock, R. E., Sweetman, A., and Jones, K. C. (1999). Assessment of organic contaminant fate in waste water treatment plants I: selected compounds and physicochemical properties. Chemosphere 38, 2247–2262.
Antunes, L. C. M., Han, J., Ferreira, R. B. R., Lolic, P., Borchers, C. H., and Finlay, B. B. (2011). Effect of antibiotic treatment on the intestinal metabolome. Antimicrob. Agents Chemother. 55, 1494–1503.
Attard, E., Poly, F., Commeaux, C., Laurent, F., Terada, A., Smets, B. F., et al. (2010). Shifts between Nitrospira- and Nitrobacter-like nitrite oxidizers underlie the response of soil potential nitrite oxidation to changes in tillage practices. Environ. Microbiol. 12, 315–326.
Balvanera, P., Pfisterer, A. B., Buchmann, N., He, J.-S., Nakashizuka, T., Raffaelli, D., et al. (2006). Quantifying the evidence for biodiversity effects on ecosystem functioning and services. Ecol. Lett. 9, 1146–1156.
Bartossek, R., Nicol, G. W., Lanzen, A., Klenk, H. P., and Schleper, C. (2010). Homologues of nitrite reductases in ammonia-oxidizing archaea: diversity and genomic context. Environ. Microbiol. 12, 1075–1088.
Berg, G., and Smalla, K. (2009). Plant species and soil type cooperatively shape the structure and function of microbial communities in the rhizosphere. FEMS Microbiol. Ecol. 68, 1–13.
Bock, E., and Wagner, M. (2006). “Oxidation of inorganic nitrogen compounds as an energy source,” in The Prokaryotes, ed M. Dworkin (New York, NY: Springer Verlag), 457–495.
Brown, G. G., Barois, I., and Lavelle, P. (2000). Regulation of soil organic matter dynamics and microbial activityin the drilosphere and the role of interactionswith other edaphic functional domains. Eur. J. Soil Biol. 36, 177–198.
Brown, G. M. (1962). The biosynthesis of folic acid. II. Inhibition by sulfonamides. J. Biol. Chem. 237, 536–540.
Burkhardt, M., Stamm, C., Waul, C., Singer, H., and Muller, S. (2005). Surface runoff and transport of sulfonamide antibiotics and tracers on manured grassland. J. Environ. Qual. 34, 1363–1371.
Burns, L. C., Stevens, R. J., Smith, R. V., and Cooper, J. E. (1995). The occurrence and possible sources of nitrite in a grazed, fertilized, grassland soil. Soil Biol. Biochem. 27, 47–59.
Daims, H., Nielsen, J. L., Nielsen, P. H., Schleifer, K. H., and Wagner, M. (2001). In situ characterization of Nitrospira-like nitrite oxidizing bacteria active in wastewater treatment plants. Appl. Environ. Microbiol. 67, 5273–5284.
Elmund, G. K., Morrison, S. M., Grant, D. W., and Nevins, M. P. (1971). Role of excreted chlortetracycline in modifying the decomposition process in feedlot waste. Bull. Environ. Contam. Toxicol. 6, 129–132.
Gieseke, A., Bjerrum, L., Wagner, M., and Amann, R. (2003). Structure and activity of multiple nitrifying bacterial populations co-existing in a biofilm. Environ. Microbiol. 5, 355–369.
Graham, D. W., Knapp, C. W., Van Vleck, E. S., Bloor, K., Lane, T. B., and Graham, C. E. (2007). Experimental demonstration of chaotic instability in biological nitrification. ISME J. 1, 385–393.
Guindon, S., and Gascuel, O. (2003). A simple, fast, and accurate algorithm to estimate large phylogenies by maximum likelihood. Syst. Biol. 52, 696–704.
Halling-Sørensen, B. (2001). Inhibition of aerobic growth and nitrification of bacteria in sewage sludge by antibacterial agents. Arch. Environ. Contam. Toxicol. 40, 451–460.
Heuer, H., Solehati, Q., Zimmerling, U., Kleineidam, K., Schloter, M., Muller, T., et al. (2011). Accumulation of sulfonamide resistance genes in arable soils due to repeated application of manure containing sulfadiazine. Appl. Environ. Microbiol. 77, 2527–2530.
Hooper, D. U., and Vitousek, P. M. (1997). The effects of plant composition and diversity on ecosystem processes. Science 277, 1302–1305.
Ives, A. R., Klug, J. L., and Gross, K. (2000). Stability and species richness in complex communities. Ecol. Lett. 3, 399–411.
Johnson, D., Booth, R. E., Whiteley, A. S., Bailey, M. J., Read, D. J., Grime, J. P., et al. (2003). Plant community composition affects the biomass, activity and diversity of microorganisms in limestone grassland soil. Eur. J. Soil Sci. 54, 671–677.
Jung, M. Y., Park, S. J., Min, D., Kim, J. S., Rijpstra, W. I. C., Damste, J. S. S., et al. (2011). Enrichment and characterization of an autotrophic ammonia-oxidizing archaeon of mesophilic crenarchaeal group I.1a from an agricultural soil. Appl. Environ. Microbiol. 77, 8635–8647.
Kim, J. G., Jung, M. Y., Park, S. J., Rijpstra, W. I. C., Damste, J. S. S., Madsen, E. L., et al. (2012). Cultivation of a highly enriched ammonia-oxidizing archaeon of thaumarchaeotal group I.1b from an agricultural soil. Environ. Microbiol. 14, 1528–1543.
Kowalchuk, G. A., Buma, D. S., de Boer, W., Klinkhamer, P. G. L., and van Veen, J. A. (2002). Effects of above-ground plant species composition and diversity on the diversity of soil-borne microorganisms. Antonie Van Leeuwenhoek 81, 509–520.
Kowalchuk, G. A., and Stephen, J. R. (2001). Ammonia-oxidzing bacteria: a model for molecular microbial ecology. Annu. Rev. Microbiol. 55, 485–529.
Lamshöft, M., Sukul, P., Zühlke, S., and Spiteller, M. (2007). Metabolism of 14C-labelled and non-labelled sulfadiazine after administration to pigs. Anal. Bioanal. Chem. 388, 1733–1745.
Leininger, S., Urich, T., Schloter, M., Schwark, L., Qi, J., Nicol, G. W., et al. (2006). Archaea predominate among ammonia-oxidizing prokaryotes in soils. Nature 442, 806–809.
Ludwig, W., Strunk, O., Westram, R., Richter, L., Meier, H., Yadhukumar., et al. (2004). ARB: a software environment for sequence data. Nucleic Acids Res. 32, 1363–1371.
Maixner, F., Noguera, D. R., Anneser, B., Stoecker, K., Wegl, G., Wagner, M., et al. (2006). Nitrite concentration influences the population structure of Nitrospira-like bacteria. Environ. Microbiol. 8, 1487–1495.
Millard, P., and Singh, B. (2010). Does grassland vegetation drive soil microbial diversity? Nutr. Cycl. Agroecosys. 88, 147–158.
Ollivier, J., Kleineidam, K., Reichel, R., Thiele-Bruhn, S., Kotzerke, A., Kindler, R., et al. (2010). Effect of sulfadiazine-contaminated pig manure on the abundances of genes and transcripts involved in nitrogen transformation in the root-rhizosphere complexes of maize and clover. Appl. Environ. Microbiol. 76, 7903–7909.
Ollivier, J., Töwe, S., Bannert, A., Hai, B., Kastl, E.-M., Meyer, A., et al. (2011). Nitrogen turnover in soil and global change. FEMS Microbiol. Ecol. 78, 3–16.
Pester, M., Rattei, T., Flechl, S., Gröngröft, A., Richter, A., Overmann, J., et al. (2012). amoA-based consensus phylogeny of ammonia-oxidizing archaea and deep sequencing of amoA genes from soils of four different geographic regions. Environ. Microbiol. 14, 525–539.
Phillips, C. J., Harris, D., Dollhopf, S. L., Gross, K. L., Prosser, J. I., and Paul, E. A. (2000). Effects of agronomic treatments on structure and function of ammonia-oxidizing communities. Appl. Environ. Microbiol. 66, 5410–5418.
Poly, F., Wertz, S., Brothier, E., and Degrange, V. (2008). First exploration of Nitrobacter diversity in soils by a PCR cloning-sequencing approach targeting functional gene nxrA. FEMS Microbiol. Ecol. 63, 132–140.
Prosser, J. I. (1989). “Autotrophic nitrification in bacteria,” in Advances in Microbial Physiology, eds A. H. Rose and D. W. Tempest (New York, NY: Academic Press), 125–181.
Rosendahl, I., Siemens, J., Groeneweg, J., Linzbach, E., Laabs, V., Herrmann, C., et al. (2011). Dissipation and sequestration of the veterinary antibiotic sulfadiazine and its metabolites under field conditions. Environ. Sci. Technol. 45, 5216–5222.
Rotthauwe, J. H., Witzel, K. P., and Liesack, W. (1997). The ammonia monooxygenase structural gene amoA as a functional marker: molecular fine-scale analysis of natural ammonia-oxidizing populations. Appl. Environ. Microbiol. 63, 4704–4712.
Schauss, K., Focks, A., Leininger, S., Kotzerke, A., Heuer, H., Thiele-Bruhn, S., et al. (2009). Dynamics and functional relevance of ammonia-oxidizing archaea in two agricultural soils. Environ. Microbiol. 11, 446–456.
Schloss, P. D., Westcott, S. L., Ryabin, T., Hall, J. R., Hartmann, M., Hollister, E. B., et al. (2009). Introducing mothur: open-source, platform-independent, community-supported software for describing and comparing microbial communities. Appl. Environ. Microbiol. 75, 7537–7541.
Schramm, A., de Beer, D., van den Heuvel, J. C., Ottengraf, S., and Amann, R. (1999). Microscale distribution of populations and activities of nitrosospira and nitrospira spp. along a macroscale gradient in a nitrifying bioreactor: quantification by in situ hybridization and the use of microsensors. Appl. Environ. Microbiol. 65, 3690–3696.
Schramm, A., Larsen, L. H., Revsbech, N. P., Ramsing, N. B., Amann, R., and Schleifer, K. H. (1996). Structure and function of a nitrifying biofilm as determined by in situ hybridization and the use of microelectrodes. Appl. Environ. Microbiol. 62, 4641–4647.
Šidák, Z. (1967). Rectangular confidence regions for the means of multivariate normal distributions. J. Am. Stat. Assoc. 62, 626–633.
Spang, A., Hatzenpichler, R., Brochier-Armanet, C., Rattei, T., Tischler, P., Spieck, E., et al. (2010). Distinct gene set in two different lineages of ammonia-oxidizing archaea supports the phylum Thaumarchaeota. Trends Microbiol. 18, 331–340.
Spieck, E., and Bock, E. (2005). “The lithoautotrophic nitriteoxidizing bacteria,” in Bergey's Manual of Systematic Bacteriology, 2nd Edn., eds G. Garrity, D. J. Brenner, N. R. Krieg, and J. T. Staley (New York, NY: Springer-Verlag), 149–153.
Steenwerth, K. L., Jackson, L. E., Calderόn, F. J., Stromberg, M. R., and Scow, K. M. (2002). Soil microbial community composition and land use history in cultivated and grassland ecosystems of coastal California. Soil Biol. Biochem. 34, 1599–1611.
Tappe, W., Zarfl, C., Kummer, S., Burauel, P., Vereecken, H., and Groeneweg, J. (2008). Growth-inhibitory effects of sulfonamides at different pH: dissimilar susceptibility patterns of a soil bacterium and a test bacterium used for antibiotic assays. Chemosphere 72, 836–843.
Thompson, J. D., Higgins, D. G., and Gibson, T. J. (1994). CLUSTAL W: improving the sensitivity of progressive multiple sequence alignment through sequence weighting, position-specific gap penalties and weight matrix choice. Nucleic Acids Res. 22, 4673–4680.
Tourna, M., Stieglmeier, M., Spang, A., Konneke, M., Schintlmeister, A., Urich, T., et al. (2011). Nitrososphaera viennensis, an ammonia oxidizing archaeon from soil. Proc. Natl. Acad. Sci. U.S.A. 108, 8420–8425.
Wertz, S., Leigh, A. K. K., and Grayston, S. J. (2012). Effects of long-term fertilization of forest soils on potential nitrification and on the abundance and community structure of ammonia oxidizers and nitrite oxidizers. FEMS Microbiol. Ecol. 79, 142–154.
Wertz, S., Poly, F., Le Roux, X., and Degrange, V. (2008). Development and application of a PCR-denaturing gradient gel electrophoresis tool to study the diversity of Nitrobacter-like nxrA sequences in soil. FEMS Microbiol. Ecol. 63, 261–271.
Zarfl, C., Matthies, M., and Klasmeier, J. (2008). A mechanistical model for the uptake of sulfonamides by bacteria. Chemosphere 70, 753–760.
Appendix
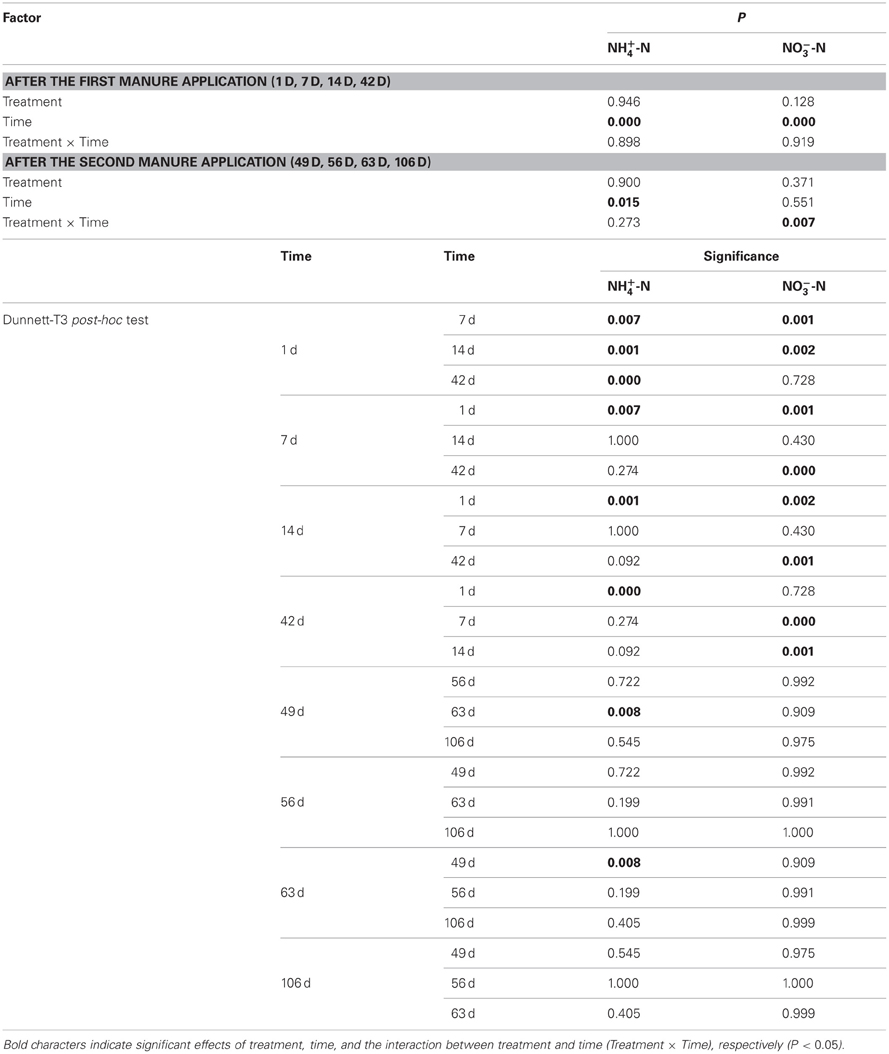
Table A1. Statistical evaluation of ammonium-N and nitrate-N concentrations in the root-rhizosphere complex by two-way ANOVA.
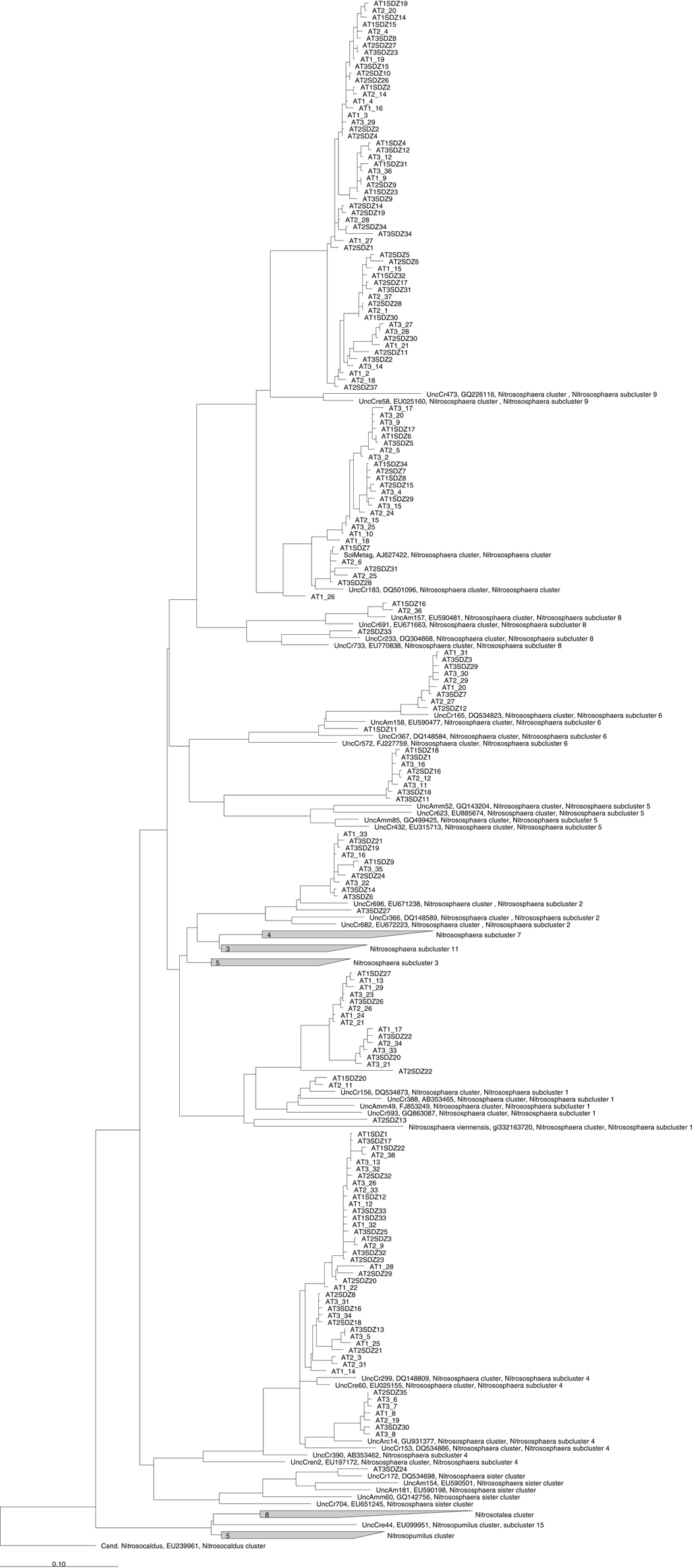
Figure A1. Phylogenetic analysis of partial amoA nucleic acid sequences (624 bp). This figure represents the ungrouped phylogenetic tree found in Figure 4A. Clone names include indications on the sampling time point (T1, T2, T3) as well as the treatment (SDZ for PMSDZ).
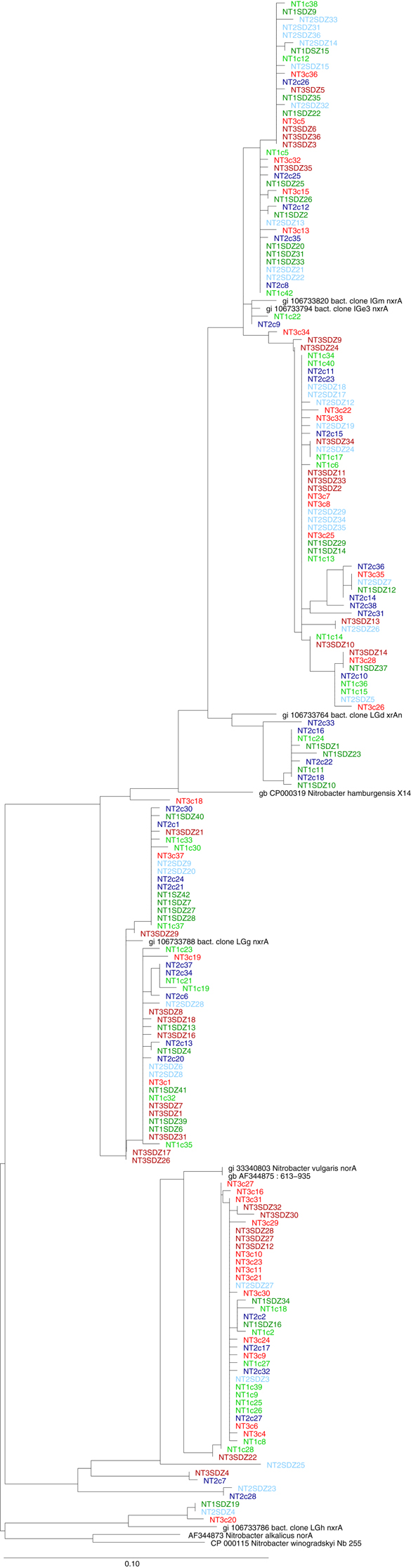
Figure A2. Phylogenetic analysis of partial nxrA nucleic acid sequences (322 bp). This figure represents the ungrouped phylogenetic tree found in Figure 4B. Clone names include indications on the sampling time point (T1, T2, T3) as well as the treatment (SDZ for PMSDZ).
Keywords: sulfadiazine, rhizosphere, ammonia-oxidizing microbes, nitrite-oxidizing bacteria, nitrification
Citation: Ollivier J, Schacht D, Kindler R, Groeneweg J, Engel M, Wilke B-M, Kleineidam K and Schloter M (2013) Effects of repeated application of sulfadiazine-contaminated pig manure on the abundance and diversity of ammonia and nitrite oxidizers in the root-rhizosphere complex of pasture plants under field conditions. Front. Microbio. 4:22. doi: 10.3389/fmicb.2013.00022
Received: 23 November 2012; Accepted: 28 January 2013;
Published online: 14 February 2013.
Edited by:
Rich Boden, University of Plymouth, UKReviewed by:
Eric Boyd, Montana State University, USARichard S. Winder, Natural Resources Canada, Canada
Copyright © 2013 Ollivier, Schacht, Kindler, Groeneweg, Engel, Wilke, Kleineidam and Schloter. This is an open-access article distributed under the terms of the Creative Commons Attribution License, which permits use, distribution and reproduction in other forums, provided the original authors and source are credited and subject to any copyright notices concerning any third-party graphics etc.
*Correspondence: Julien Ollivier, Institute of Soil Ecology, Technical University Munich, Ingolstädter Landstraße 1, 85764 Neuherberg, Germany. e-mail:anVsaWVuLm9sbGl2aWVyQGhlbG1ob2x0ei1tdWVuY2hlbi5kZQ==