- 1Department of Renewable Resources, University of Alberta, Edmonton, AB, Canada
- 2Department of Biological Sciences, University of Alberta, Edmonton, AB, Canada
- 3Department of Civil and Environmental Engineering, University of Alberta, Edmonton, AB, Canada
Dispersed clay particles in mine tailings and soft sediments remain suspended for decades, hindering consolidation and challenging effective management of these aqueous slurries. Current geotechnical engineering models of self-weight consolidation of tailings do not consider microbial contribution to sediment behavior, however, here we show that microorganisms indigenous to oil sands tailings change the porewater chemistry and accelerate consolidation of oil sands tailings. A companion paper describes the role of microbes in alteration of clay chemistry in tailings. Microbial metabolism in mature fine tailings (MFT) amended with an organic substrate (hydrolyzed canola meal) produced methane (CH4) and carbon dioxide (CO2). Dissolution of biogenic CO2 lowered the pH of amended MFT to pH 6.4 vs. unamended MFT (pH 7.7). About 12% more porewater was recovered from amended than unamended MFT during 2 months of active microbial metabolism, concomitant with consolidation of tailings. The lower pH in amended MFT dissolved carbonate minerals, thereby releasing divalent cations including calcium (Ca2+) and magnesium (Mg2+) and increasing bicarbonate (HCO−3) in porewater. The higher concentrations increased the ionic strength of the porewater, in turn reducing the thickness of the diffuse double layer (DDL) of clay particles by reducing the surface charge potential (repulsive forces) of the clay particles. The combination of these processes accelerated consolidation of oil sands tailings. In addition, ebullition of biogenic gases created transient physical channels for release of porewater. In contrast, saturating the MFT with non-biogenic CO2 had little effect on consolidation. These results have significant implications for management and reclamation of oil sands tailings ponds and broad importance in anaerobic environments such as contaminated harbors and estuaries containing soft sediments rich in clays and organics.
Introduction
The oil sands in Alberta, Canada are the world's third largest proven reserve comprising ~170 billion barrels of recoverable bitumen, with production expected to surpass 3.5 million barrels day−1 by 2020. Bitumen is extracted from surface-mined ores using hot water and hydrocarbon solvent (Schramm et al., 2000), generating ~1 million m3 fluid fine tailings day−1 that are deposited and retained in tailings ponds. The current total volume (>920 million m3) and surface area (~182 km2) of the ponds will continue to increase with resource exploitation (Alberta Environment and Sustainable Resource Development, 2013) (http://www.aer.ca/rules-and-regulations/directives/tailings-plans-2012). Two major challenges plague the oil sands surface mining industry: (1) recovering water from tailings for re-use (7.5–10 barrels of water for every barrel of bitumen produced), so as to reduce the need for fresh water, and (2) consolidating the tailings solids to decrease the volume of stored tailings for subsequent reclamation.
The tailings deposited into ponds (initially at ~10 wt% solids) settle by gravity in 2–4 years to form mature fine tailings (MFT, ~30 wt% solids), a colloidal suspension comprising slightly alkaline water, silt, clay, unrecovered bitumen (~5 wt%) and residual solvent (<1 wt%) such as naphtha, a mixture of C3–C14 aliphatic and monoaromatic hydrocarbons. MFT then requires decades to consolidate to >60 wt% solids (Fedorak et al., 2003; Jeeravipoolvarn et al., 2009) through close packing of clay particles accompanied by interstitial water (porewater) expression to form a “cap water” layer. Tailings ponds harbor indigenous microbial communities (Penner and Foght, 2010; Siddique et al., 2011, 2012) that anaerobically biodegrade organic compound, such as solvent hydrocarbons, to methane (CH4) plus carbon dioxide (CO2) (Siddique et al., 2006, 2007, 2011). Biogenic gas production has been associated with accelerated consolidation of MFT and recovery of porewater both in situ and in the laboratory (Fedorak et al., 2003; Bressler et al., 2010). To date, only simple physical mechanisms such as creation of transient channels for water transport by biogenic gas bubbles (Brown et al., 2013; Voordouw, 2013) have been proposed to explain this phenomenon, but this mechanism inadequately describes consolidation when gas is formed but ebullition is minimal.
Because biodensification is slow in situ when supported solely by endogenous substrates, we amended MFT with low concentrations of labile organic substrates to enhance anaerobic microbial activity and accelerate the processes ex situ. Here we describe a laboratory study conducted using 50-L columns (Figure 1). The results reveal biogeochemical reactions that impact the chemistry of three tailings components—porewater, expressed porewater (cap water) and solids—and accelerate consolidation of MFT. We describe how microbial metabolism alters the chemistry of porewater that in turn influences consolidation of clay particle suspensions. A companion paper (Siddique et al., 2014) describes microbe:mineral interactions in the solids fraction. The combined results and biogeochemical models are relevant for proposed reclamation strategies for oil sands tailings in uplands and end pit lakes.
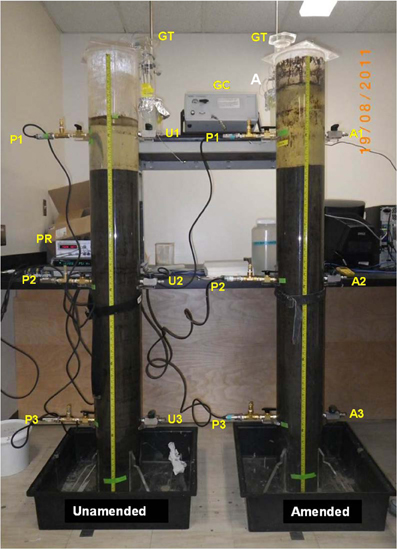
Figure 1. Experimental 50-L columns used for measuring consolidation, porewater recovery and gas release from oil sands mature fine tailings (MFT) either amended (A) with an organic carbon source or unamended (U). The photograph was taken after 105 d incubation at ~20°C. Three sampling ports for collecting MFT or cap water (A1–A3; U1–U3) and three instrumentation ports (P1–P3) fitted with pressure transducers and a pressure reading unit (PR) for recording in situ pore pressure were installed in each column; see Methods for details. The columns were sealed under a N2 gas atmosphere. Stainless steel tubing connected the headspace to a micro-gas chromatograph (GC) to determine the composition of released biogenic gas, and to gas traps (GT) for measuring cumulative emitted gas volumes.
In addition, the fundamental mechanisms reported here have broad implications for developing geotechnical models to predict behavior of organic-rich saturated soft sediments (i.e., contaminated harbors, and marine, lacustrine and estuarine sediments), predicting contaminant transport from sediments to overlying water, and assessing remedial strategies for containing contaminants through in situ sediment capping (Himmelheber et al., 2011).
Materials and Methods
Preparation of 50-L Columns
Mature fine tailings (MFT; Table 1) and cap water were collected from Mildred Lake Settling Basin (MLSB; 57°4′27″N, 111°38′19″W UTM) at Syncrude Canada Ltd. in Fort McMurray, Alberta, Canada in April 2011, and stored at room temperature (~20°C, ca. tailings pond in situ temperature) for a few days before using for the experiment. The solids and bitumen contents of the MFT were determined using the Dean and Stark method (Dean and Stark, 1920) to be ~35 and ~2.5%, respectively. The solids content was adjusted to 25 wt% solids using tailings pond “cap water” immediately before beginning the experiment, to allow this study to be compared to previous studies (Young et al., in preparation). For each of two parallel 50-L columns (described below), 30 L MFT was combined with 15.45 L tailings pond cap water by gentle but thorough manual mixing under a curtain of N2 gas to achieve a solids content of 25 wt% while maintaining anaerobic conditions. For the test column, the added cap water contained soluble canola meal hydrolysate (preparation and composition described in Table 2) sufficient to provide a final concentration of 400 mg C L−1 MFT. The cap water used for adjusting MFT in the other column was unaltered, serving as a baseline control in which only endogenous carbon was available to support microbial activity.
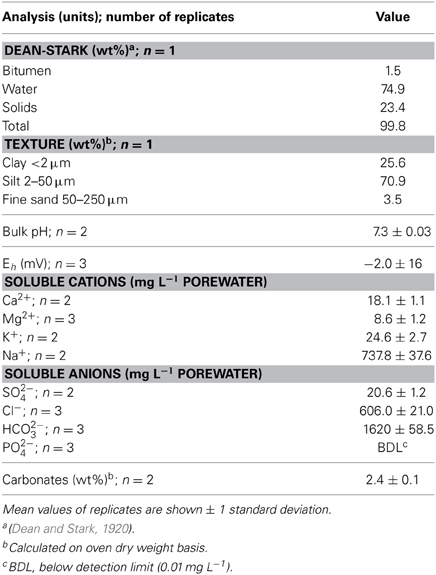
Table 1. Chemical characteristics of mature fine tailings used in 50-L column experiment prior to amendment and incubation.
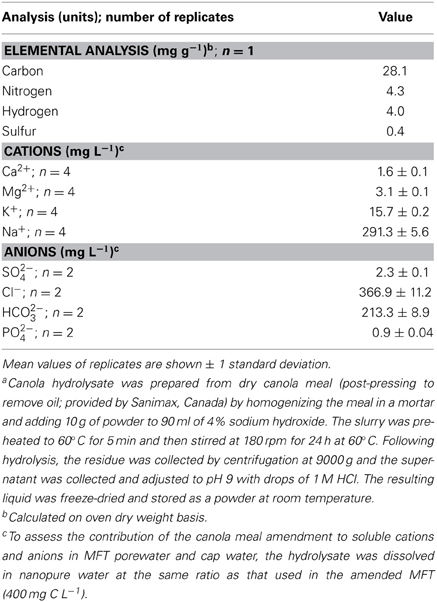
Table 2. Elemental composition of hydrolyzed canola meala used as the labile organic amendment in the experiment.
Two cylindrical acrylic columns (30.48 cm outer diameter, 0.64 cm wall thickness, and 195.6 cm height; Johnston Industrial Plastics Ltd., Edmonton, AB, Canada) were sealed at the base with acrylic plates and lateral supports (Figure 1). The cylinders were equipped with brass sampling ports (U1–U3 for unamended and A1–A3 for amended columns) for removal of MFT or water samples from the inner portion of the column, and with PX409-005G5V (OMEGADYNE Inc.) pressure transducers (P1–P3) and an Agilent 34972A Data Acquisition/Data Logger pressure reading unit (PR) for measuring pore pressure in situ.
The experiment was established on May 6, 2011 by filling each column with 45 L prepared MFT under a plastic curtain flushed with a continuous stream of nitrogen (N2) to maintain anaerobic conditions and minimize exposure of MFT to air. In both columns, the adjusted MFT formed a single layer of suspended clays with no free water at the surface. After filling, the columns were sealed under an N2 headspace using acrylic plates fitted with gas-tight stainless steel gas ports and tubing (0.15 cm diam.) connecting the headspace to a gas trap and a micro-gas chromatograph to determine the volume and composition of emitted gas, respectively. The columns were incubated in dim natural light at ~20°C. Real time measurements of biogenic gas production and composition, in situ pH, porewater recovery and consolidation of tailings were recorded during 213 d incubation. Consolidation and porewater recovery were measured as the height of the cap water-solid interface (the “mud line”) and corrected for trapped gas volume as described below.
At the end of the experiment, cap water and MFT samples were collected from the center of the column via sampling ports, using 4 mm stainless steel tubing and syringes. Samples were analyzed for carbonate minerals, soluble and exchangeable cations, and soluble anions, as described below. These samples were also subjected to solid phase chemical and molecular microbiological analyses described and discussed in the companion manuscript (Siddique et al., 2014).
We were unable to include sterilized MFT as a control condition for several reasons. First, we have observed previously (unpublished results) that it is very difficult to ensure sterilization of the thick MFT suspension, even with lengthy, repeated autoclaving over three or four successive days. Second, we did not wish to alter the structure or chemistry of the tailings by subjecting them to heat, as this could affect consolidation. Finally, we did not have access to a gamma source of sufficient capacity for irradiative sterilization of ~90 L of MFT.
Small-Volume Complementary Experiments
To observe the effect of non-biogenic (exogenous) gas amendment on consolidation of MFT, four parallel 2-L columns were prepared. Each column was filled with 1.7 L MFT (adjusted to 25% solids) under anaerobic conditions. One column received MFT that had been sparged with N2 gas (5.0 Ultra high purity, Praxair) at a flow rate of 62.5 mL min−1 for 1 h prior to dispensing into the column. MFT for two replicate columns was sparged with CO2 (4.0 Anaerobic, Praxair) at a flow rate of 72 mL min−1 for 1 h until pH stabilized between 6.54 and 6.68, then was placed in the columns. MFT for the fourth column did not receive any gas sparging (untreated control). All columns were sealed under an anaerobic (N2) headspace and incubated undisturbed at ~20°C in the dark for 120 d. The columns were monitored regularly for expressed porewater recovery (cap water) and solids consolidation by measuring the height of the mud line and water surface and converting to volume.
To test the effect of different cations, ionic strength and pH on the settling behavior of clays particles separately from microbial effects, a small-volume, short-term experiment was conducted in triplicate in 15-mL conical Falcon tubes (Fisher Scientific, Canada). Seven milliliters of MFT was placed in each Falcon tube and amended with 7 mL of one of the following solutions: 1N NaCl; 1N CaCl2; 1N FeCl2; 0.2M ammonium oxalate buffer (pH 3); 6M HCl (pH 1); or Nanopure water (Barnstead; Thermo Scientific). The tubes were shaken on a reciprocating shaker for 2 h and then placed upright on the bench to consolidate without disturbance. The mud line height in each tube was recorded after 30 min and again after 24 h.
Analytical Methods and Calculations
We define here two water compartments analyzed in our experiments: (1) “porewater” is interstitial water associated with the colloidal MFT suspension, and is a component of the MFT; (2) “cap water” is porewater that has been expressed from the MFT and has collected above the mud line during consolidation. The term “mud line” used here is defined as the interface between cap water and MFT.
Porewater recovery as cap water, and MFT consolidation
The height of the mud line was used to measure solids consolidation and calculate porewater recovery. The volumes of cap water (Vcw) and MFT (solids plus porewater plus gas below the mud line; VMFT) were determined, respectively, by measuring the height of the cap water level above the mud line and height of the mud line in each column. The measured heights were converted to volumes using the following equation:
where, V = volume of cap water or MFT,
r = inner radius of the column,
hmud line = height of the cap water level or mud line.
Then, water recovery (WR) defined as the cap water volume (above the mud line) was calculated as a percentage of the initial volume of porewater in MFT:
where, WR = water recovery (%),
Vcw = measured volume of cap water,
Vin = initial volume of porewater MFT.
Consolidation of MFT is defined as the MFT volume (below the mud line) calculated as a percentage of the initial volume of MFT
where, VMFT = measured volume of MFT and Vin = initial volume of MFT.
Estimation of MFT consolidation in 50-L columns by deducting volume of entrapped gas bubbles
Estimated consolidation of MFT in unamended and amended columns was calculated using the following equation:
where, Vin is the initial volume of MFT (45 L), and VMFT−d is volume of MFT without gas at different densities that are calculated as:
where, mMFT−In is the initial mass of MFT (54.9 kg); mwr is the mass of released water. D is the density of the MFT on different days, determined from the mean weight of five replicate 10-mL subsamples of MFT.
Volume and composition of gas emitted by ebullition
The volume of gas emitted from 50-L columns was measured using a NaCl-citric acid brine gas trap (Boone, 1982). The composition of produced gas for CH4 and CO2 was determined using a Varian CP-2003 portable Micro-GC (gas chromatograph) equipped with a GMT-2HP moisture trap and thermal conductivity detector (TCD). The minimum detection limit of this GC was 50 ppm.
pH and redox potential (Eh)
The pH was determined in situ at intervals during incubation by inserting a pH meter (Hach H170multi) fitted with an ISFET pH stainless steel micro probe (PHW17-SS) through side ports (Figure 1). For Eh measurements, MFT samples were collected from ports 2 and 3 under a continuous flow of N2 and immediately subjected to Eh measurement using a TX100 pH/mV-meter fitted with redox-sensitive electrode (Sensorex 651CD-ORP).
Soluble cations and anions
MFT samples removed from the columns were centrifuged (3075 g for 1 h) in a Sorvall RC 5B Superspeed centrifuge to collect the porewater. The solids fraction was analyzed as described below. For the soluble cations calcium (Ca2+), magnesium (Mg2+), sodium (Na+) and potassium (K+), porewater was filtered and diluted with 1% HNO3 (trace metal grade) and analyzed using Inductively Coupled Plasma Mass Spectrometry (ICP-MS; Perkin Elmer SCIEX ELAN 9000) with appropriate internal and external standards. Chloride (Cl−), nitrate (NO−3) and sulfate (SO2−4) soluble anion concentrations were determined using an ion chromatograph (Dionex DX 600) equipped with a 4 mm analytical column (AS9-HC). Dissolved bicarbonates (HCO−3) were determined using the methyl orange indicator method (US EPA, 1974) and Smartchem Discrete Wet Chemistry Analyzer 200 (Westco Scientific) at 550 nm. The PO3−4 concentration was determined using the colorimetric Molybdenum Blue method (US EPA, 1993) using the SmartChem at λ 880 nm.
Moisture correction factor
Data from chemical analyses of the solid phase (carbonates and exchangeable cations) were corrected for moisture and expressed on a solid dry weight basis. Moisture content in the samples was determined by drying in an oven at 105°C to constant weight (ISO 11465, 1993). The moisture content was obtained in weight percent and the corresponding correction factor was developed using the following equation:
Exchangeable cations
Five grams of MFT were collected in 50-mL polypropylene centrifuge tubes (Corning Inc.) and 20 mL of 0.1M BaCl2/0.1M NH4Cl solution was added to displace the exchangeable cations (Sparks, 1996; Carter and Gregorich, 2008; Rayment and Lyons, 2012). The samples were shaken for 2 h and centrifuged (3075g for 1 h) in a Sorvall RC 5B Superspeed centrifuge. The supernatant was diluted with 1% HNO3 (trace metal grade) and analyzed using ICP-MS. In a few cases where the samples were not analyzed immediately after centrifugation, the supernatant was preserved by acidification to pH 1–2 using HNO3. The concentrations of exchangeable cations were calculated by subtracting concentrations of soluble cations (So et al., 2006). The results were expressed on dry weight basis.
Ionic strength (i) and diffuse double layer (DDL)
Ionic strength of the MFT porewater was calculated using the following formula (Essington, 2004):
where, Mi is the molar concentration of charged species (i) with charge Zi in the porewater and the sum includes all charged species measured in the porewater.
The thickness of the DDL was calculated using the following formula (Essington, 2004):
where, k−1 is the thickness (cm) of the DDL, Z is the average mean charge of the counterions (exchangeable cations), and I is the ionic strength of the porewater. Because MFT is a polyionic system having ions of different charges, the average mean charge of the exchangeable cations was calculated using the following equation:
where, Ciis the concentration of ions on the exchangeable surface and Zi is the charge of the species (i).
Carbonate minerals
The total carbonates in the MFT solid phase were determined by acid digestion (Pansu and Gautheyrou, 2006). The MFT (~10 mL) was centrifuged to separate the solid phase which was washed twice with 30 mL methanol to remove any soluble HCO−3. The MFT solid phase (~2 g) was placed in a serum bottle capped with a butyl rubber stopper and the headspace in the bottle was flushed with N2 at atmospheric pressure. The carbonates were dissolved by adding 20 mL of 1 M HCl to the bottles using a syringe. The bottle was gently shaken for 2 h and the amount of CO2 released was measured using GC-TCD (HP 5890). After CO2 measurement, the contents of the bottle were analyzed for Ca and Mg using an Atomic Absorption Spectrometer (AAS; SpectrAA 880). These concentrations were used to calculate CaCO3 and CaMg(CO3)2 in the MFT.
Results
We investigated contributions of microbial activity (methanogenesis) to consolidation in a series of experiments conducted at different scales from 2-L to 50-L column studies. The results reveal that methanogenesis is important for rapid consolidation of MFT, an effect that is superimposed on tailings self-weight consolidation. Acetate, a well-known substrate for methanogenesis, resulted in greater porewater recovery and solids consolidation when added to MFT as compared to unamended MFT (Figure 2; constructed using data retrieved from Bressler et al., 2010). The same phenomenon has also been observed in our previous experiments (Arkell et al., in review; Young et al., in preparation). The increase in total volume in the acetate-amended MFT shown in Figure 2A, left panel is due to biogas entrained in the MFT solids. However, specifically inhibiting methanogenesis by adding sodium 2-bromoethanesulfonate (BES) resulted in minimal consolidation, presumably equivalent to self-weight settling, minimal porewater recovery and no visible pockets of entrained gas (Figure 2). Thus, it appears that active methanogenesis is a prerequisite for biodensification of MFT incubated under these conditions.
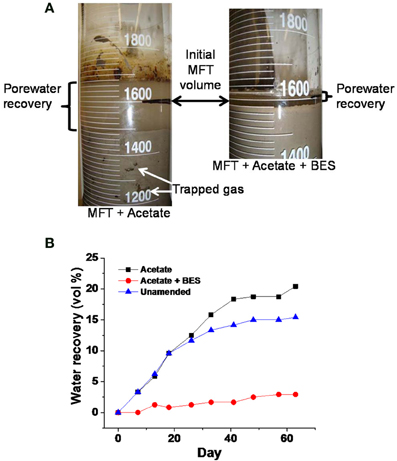
Figure 2. Effect of adding 10 mM sodium 2-bromoethanesulfonate (BES), a specific inhibitor of methanogens, on dewatering of 1.6 L MFT in 2-L columns incubated undisturbed for >60 d at ambient temperature (~22°C). (A) Photographs of porewater recovery and total volume of MFT solids and porewater, including trapped biogenic gases (white arrows) in parallel columns containing MFT amended with acetate (140 mg L−1 MFT) or with acetate plus BES (few, tiny bubbles; not visible), or unamended (no bubbles; not shown). (B) Quantitation of porewater recovery from MFT amended with acetate, or with acetate plus BES, or unamended, illustrating the magnitude of the microbial effect (Figure is constructed using the data retrieved from Bressler et al. 2010).
To further demonstrate the contribution of biogenic vs. abiotic gases to porewater recovery and consolidation, small-scale (2-L column) experiments were performed in which unamended MFT was sparged with CO2 to simulate biogenic CO2 production, or with N2 gas to simulate the effect of insoluble gas ebullition (by creating transient physical channels for expression of porewater). Only marginal differences in porewater recovery and tailings consolidation were observed compared to non-sparged MFT (Figure 3). The small proportion and slow rates of consolidation and porewater recovery (compared to acetate-amended MFT; Figure 2) presumably are due to methanogenic activity supported by endogenous organic substrates in MFT.
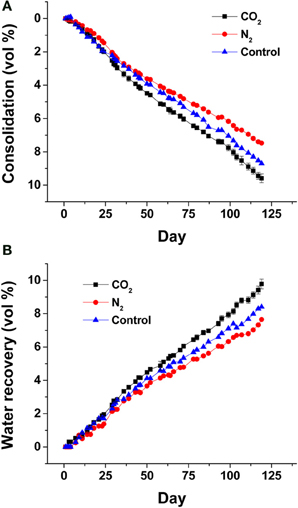
Figure 3. Effect of abiotic gas purging on MFT consolidation (A) and porewater recovery (B). Four replicate 2-L columns containing 1.7 L unamended MFT were purged with CO2 (n = 2; error bars, where visible, show the standard error) or N2 (n = 1) or no gas purging (n = 1) prior to dispensing MFT into columns. Consolidation was calculated from the volume of solids below the mud line as a fraction of the total volume of MFT plus water plus any trapped gas. Porewater recovery was calculated using the recovered porewater volume above the mud line (i.e., cap water volume) as a fraction of the initial total volume of porewater in MFT.
Biogenic Gas Production and Decreased pH Affect Porewater Recovery from MFT in 50-L Columns
In previous small-volume experiments we used complex or simple fermentable organic amendments to accelerate dewatering and consolidation of MFT (Bressler et al., 2010; Arkell et al., in review; Young et al., in preparation), and determined that a low concentration of hydrolyzed canola meal (400 mg C L−1 MFT) was sufficient to promote tailings biodensification under methanogenic conditions. In the current experiment, it is apparent that methanogenic conditions were established in the 50-L columns, as anaerobic metabolism of the soluble canola hydrolysate resulted in emission of ~2.8 L CH4 and ~0.5 L CO2 (CH4:CO2 ratio of 5.6:1) to the headspace by 75 d, with minor gas production from unamended MFT (Figure 4A). Additional biogenic gas was trapped within amended MFT (Figure S1 Panel B), contributing to the total apparent volume of MFT. Stoichiometric calculation of theoretical gas production from canola, based on the Symons and Buswell equation (Roberts, 2002), predicted a CH4:CO2 ratio of ~3:1, but substantially less CO2 than predicted was measured in the headspace (Figure 4A). The deficit in emitted CO2 is explained by dissolution of biogenic CO2 in porewater, which decreased the pH of amended MFT from 7.7 to 6.4 by 147 d, whereas the pH of unamended MFT was essentially unchanged in >200 d (Figure 4B). We initially observed an increase in pH in the amended MFT during the first 35 d, after which it significantly decreased. During active gas biogenesis in the first 2 months, 12% more porewater was released from amended MFT than unamended (Figure 4C), most of which coincided with pH reduction, a major ebullition event at 50 d, and rapid consolidation of amended MFT. Ebullition of biogenic CH4 created transient channels for escape of porewater from MFT, observed particularly near the mud line (MFT–cap water interface; Figure S1). Other experiments (unpublished) confirmed that transient water- and gas-filled channels can form in MFT to allow passage of porewater to the surface during this phase. Gas bubbles remaining in the amended MFT did not affect subsequent consolidation behavior (Figure S2), but did contribute to the apparent total solids volume; complete degassing theoretically would have yielded ~15 vol% more consolidation in the amended column (Figure 4D).
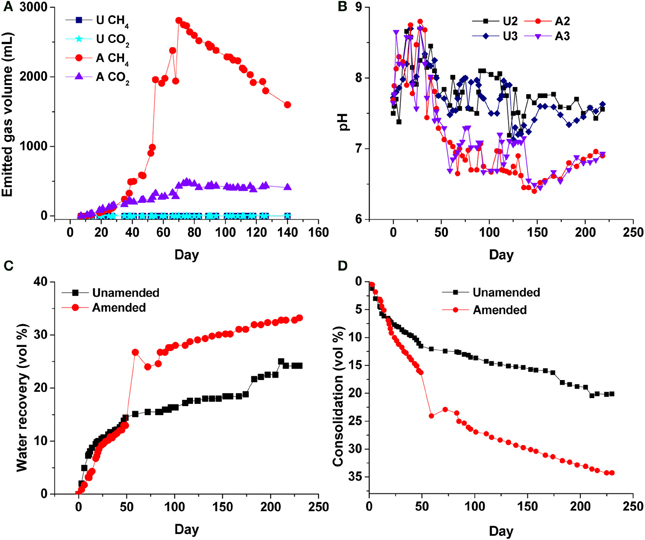
Figure 4. Physico-chemical changes to unamended (U) and amended (A) MFT in 50-L columns. (A) Cumulative emitted methane (CH4) and carbon dioxide (CO2) in headspace (not accounting for gases trapped or dissolved in MFT); (B) bulk pH of MFT, measured at ports 2 and 3; (C) Proportion of initial porewater recovered above the mud line as cap water (i.e., expressed porewater). The sudden increase in cap water in the amended column corresponded to a large ebullition event at ~50 days recorded in A; (D) Consolidation of solids, corrected for trapped gas volumes by subtracting the estimated volume of trapped gas from the measured total MFT volume. Initial and final densities of unamended (D = 1.22 and 1.30 g mL−1, respectively) and amended MFT (D = 1.22 and 1.49 g mL−1, respectively) were used to calculate consolidation; see Methods for details.
pH Affects Dissolution of Carbonate Minerals and Porewater Chemistry
The decreased pH in amended MFT due to dissolution of biogenic CO2 increased dissolution of carbonate minerals in MFT (Figure 5A): at 213 d, the carbonate mineral content in amended MFT (1.7 wt%) was significantly lower than unamended MFT (2.7 wt%). Carbonate dissolution increased soluble cation concentrations in porewater and cap water: Ca2+ and Mg2+ concentrations were greater in amended MFT (~32 and 19 mg L−1, respectively) than unamended MFT (~17 and 10 mg L−1, respectively; Figure 5B). In contrast, soluble K+ decreased in amended MFT (Figure 5B) whereas the soluble Na+ concentration remained unaffected in amended MFT porewater (Figure 5C). A very small concentration of soluble phosphate (PO3−4) was detected only in amended MFT porewater (0.36 mg L−1) with low concentrations of sulfate (SO2−4, <5 mg L−1) (Figure 5D). Amended MFT had slightly higher porewater concentrations of soluble HCO−3 (~1500 mg L−1) than unamended MFT (~1380 mg L−1) but Cl− concentrations were comparable in both amended and unamended MFTs (Figure 5E).
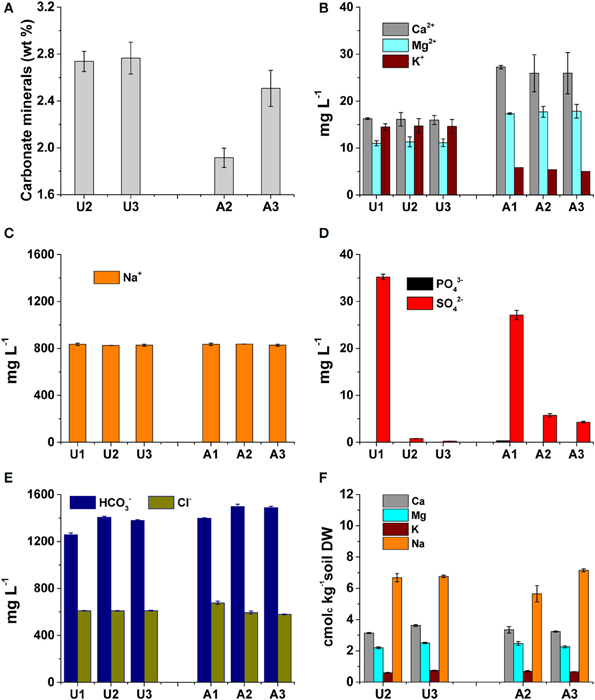
Figure 5. Concentrations of carbonate minerals and major cations and anions in unamended (U) and amended (A) 50-L columns after 213 d incubation. Labels 1, 2, and 3 refer to samples collected from ports on the columns (see Figure 1); Port 1 accessed cap water only by 213 d; ports 2 and 3 were below the mud line and accessed MFT. Bars represent the mean from analyses of duplicate or triplicate samples taken from each port and error bars, where visible, represent 1 standard deviation. (A) Carbonate mineral content of MFT samples (n = 3). (B,C) Concentrations of major soluble cations in cap water and interstitial porewater (n = 2). (D,E) Concentrations of major soluble anions in cap water and interstitial porewater (n = 2). (F) Concentrations of exchangeable cations in MFT (n = 2).
Exchangeable Cations, Ionic Strength and Diffuse Double Layer (DDL)
Although dissolution of carbonates increased Ca2+ and Mg2+ concentrations in porewater of amended MFT, no significant change in the composition of exchangeable cations on clay surfaces was observed in MFT (Figure 5F). However, increased concentrations of soluble cations (Ca2+ and Mg2+) and anions (HCO−3) increased the ionic strength (I) of the porewater (Figure 6A). The greater I (~0.055 mol L−1) was calculated for the porewater from amended MFT vs. unamended MFT (~0.04 mol L−1). The I of a solution has a profound effect on the DDL of clay particles. Increased I in the porewater of amended MFT decreased the DDL thickness (~10 × 10 −8 cm) of clay particles in amended MFT as compared to the DDL thickness (~18 × 10−8 cm) calculated for clay particles in unamended MFT (Figure 6B).
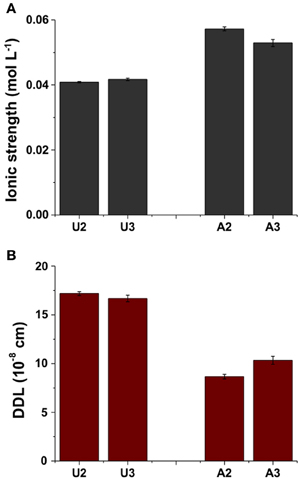
Figure 6. (A) Calculated ionic strength (I) of the porewater and (B) thickness of diffuse double layer (DDL) of clay particles in unamended (U) and amended (A) MFT in the 50-L columns at ports 2 and 3 (Figure 1). Ionic strength was calculated using the data presented in Figure 5, the DDL thickness was calculated using the ionic strength, and charges of exchangeable cations were calculated from data shown in Figure 5F. Bars represent the mean values from analyses of duplicate samples taken from each port and error bars, where visible, represent 1 standard deviation.
Discussion
Dewatering of tailings is a major challenge faced by the surface-mining oil sands industry to comply with government directives for managing the ever-growing inventory of oil sands tailings (http://www.aer.ca/rules-and-regulations/directives/directive-074). Any process that increases the settling of clay particles in oil sands tailings ponds will enhance consolidation of tailings to reduce the inventory volume and enable reclamation, and will increase recovery of porewater from MFT for re-use and reduction of fresh water demand; even marginal increases in consolidation translate into enormous volumes, given the scale of existing tailings ponds (>920 million m3). Thus, microbially-mediated consolidation and dewatering (biodensification) can mitigate this problem, whether supported by endogenous substrates in situ (Fedorak et al., 2003) or by amendment with organic substrates in an engineered process ex situ. In this paper, we have focused on how microbial activities alter porewater chemistry to enhance tailings consolidation and dewatering; in the companion paper (Siddique et al., 2014), we consider the effect on tailings solids.
The mechanism of microbially-mediated consolidation (biodensification) of oil sands tailings is multi-faceted, embracing different biogeochemical processes occurring simultaneously in the MFT (Figure 7). Stimulating the activity of indigenous anaerobes by amending MFT with labile organic carbon (hydrolyzed canola meal in this study, acetate in previous studies; Fedorak et al., 2003; Arkell et al., in review) enhanced biogenic CH4 and CO2 production. Gas production resulted in ebullition of bubbles dominated by CH4 (due to poor solubility of CH4 in water), creating transient channels for escape of pressurized porewater, particularly in MFT near the mud line. Methanogenesis in MFT might be responsible for the observed initial pH increase as acetate and CO2 were consumed (Fotidis et al., 2013) but the dissolution of entrapped CO2 reduced porewater pH, thereby dissolving carbonate minerals and releasing divalent cations (Pathway I in Figure 7). Our results are very similar to the findings of Wersin et al. (2011) who studied biogeochemical processes in a clay formation in situ. They observed carbonate dissolution, high pCO2 and alkalinity (HCO−3 and CO2−3) and a decrease in pH from 7.7 to 6.8 during anaerobic degradation of an organic substrate under sulfate-reducing and methanogenic conditions. Similar results were also reported by Schlegel et al. (2011) studying fluid geochemistry and microbiology of multiple organic-rich reservoirs in the Illinois Basin, USA. Our results are also supported by other studies where the influence of benthic bacterial activity on carbonate mineral dissolution in marine sediments has been described (Moulin et al., 1985) and dissolution of marine carbonate minerals by acidification due to increased atmospheric CO2 has been comprehensively reviewed (Morse et al., 2007). Solubility of CaCO3 increases 20-fold between pH 8 and pH 6 (Chou et al., 1989) and siderite can be dissolved in anaerobic aqueous environments at pH~7 (Jensen et al., 2002).
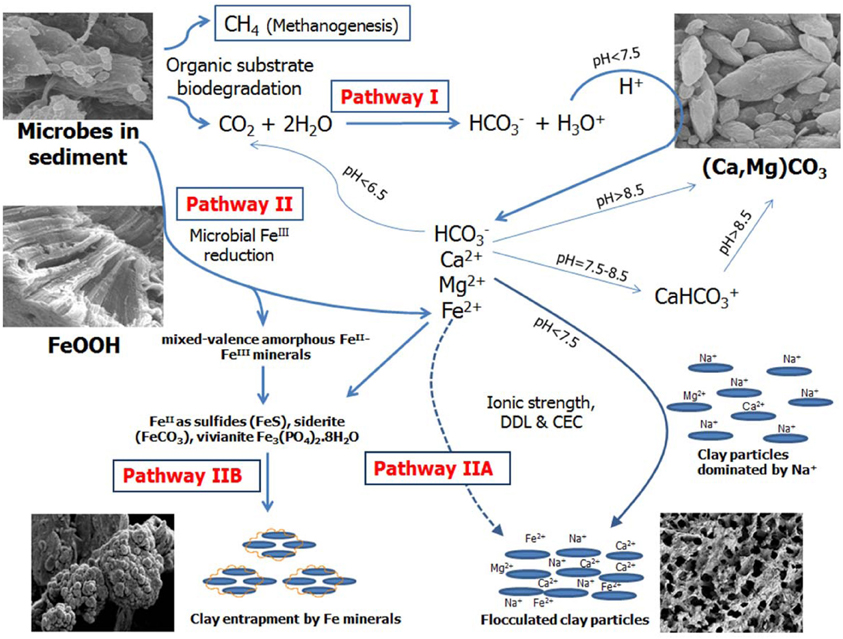
Figure 7. Proposed model for microbially-mediated geochemical pathways of clay consolidation. This study focuses on Pathway I; the companion paper (Siddique et al., 2014) focuses on Pathway II. Dissolution of biogenic CO2 in tailings decreases porewater pH by producing hydronium ion (H3O+). Increased H3O+ dissolves carbonate minerals such as CaMg(CO3)2 and releases calcium (Ca2+) and magnesium (Mg2+). Dissolution of CO2 and/or carbonate minerals increases bicarbonate (HCO−3) ions in porewater. Liberated Ca2+ and Mg2+ plus HCO−3 increase ionic strength (I) of porewater, thus reducing the diffuse double layer (DDL) of clay particles and facilitating their consolidation. Ca2+ and Mg2+ can replace some dominant exchangeable Na from clay exchanger surfaces via the cation exchange process, reducing DDL of clay particles. Competing reactions are shown by solid arrows while dashed arrows indicate pathways not considered significant in our study.
Dissolution of MFT carbonate minerals (presumably calcite/dolomite) increased Ca2+ and Mg2+ concentrations in amended porewater, whereas K+ decreased in amended MFT, which might be attributed to reducing conditions prevailing in amended MFT (Siddique et al., 2014) facilitating K+ fixation in reduced 2:1 phyllosilicates (Eslinger et al., 1979; Shen and Stucki, 1994). Phyllosilicate analysis of MFT showed that fine clay (0.08–0.2 μm) comprised ~45% kaolinite-smectite (94% kaolinite) and 55% illite-smectite (93% illite) group minerals whereas clay (0.2–2 μm) consisted of 62% kaolinite-smectite (100% kaolinite) and 36% illite-smectite (96% illite) groups (Siddique et al., 2014). Among anions, a relatively higher concentration of HCO−3 in the porewater of amended MFT was observed, presumably due to dissolution of biogenic CO2 in porewater and/or dissolution of carbonate minerals (Morse et al., 2007). The change in porewater chemistry of amended MFT due to microbial metabolism did not alter the cationic composition on clay exchanger surfaces despite significant increases in divalent cation concentrations (Ca2+ and Mg2+) in the porewater. However, the divalent cation concentrations were dwarfed by the Na+ concentration, which dominated the porewater composition. Another possible reason for the apparent lack of cation exchange could be technical, since no method has been optimized yet to determine the exchangeable cations in oil sands tailings. Although we cannot exclude cation exchange processes based on our results, we do not consider cation exchange to be a significant or essential process in MFT consolidation (Pathway I, Figure 7, broken lines).
The phenomenon of clay dispersion in aqueous systems is understood using the concept of diffuse double layer (DDL), the thickness of which is governed by the charge potential on the clay surface (one electric layer, generally with net negative charge on clay surface) and valence of the counterions in solution (second electric layer, positive charge contributed by cations) neutralizing the surface charge potential of clay. Decreasing thickness of DDL increases clay flocculation. In our study, the greater ionic strength (I) of porewater from amended MFT, attributed to increased concentrations of soluble ions (Ca2+, Mg2+, and HCO−3), would decrease the thickness of the DDL of clay particles (Figure 6) and enhance consolidation of tailings. Electrostatic repulsion between the clay particles is reduced at higher I due to ion shielding of the clay surface charges, decreasing thickness of the DDL. Greater consolidation of amended MFT might also be attributed to dominance of kaolinite phyllosilicates in the MFT, in addition to greater I. The charges on kaolinite are largely pH-dependent, and the observed lower pH of MFT would decrease the net negative charges on the clay surfaces. Nasser and James (2006) examined the settling behavior of kaolinite in aqueous media in response to I and found that, at higher I, particles settled in flocculated form. Similar results were reported by Mietta et al. (2009) for flocculation of a kaolinite suspension as function of pH and salt concentration, and they found that clay floc size was 3-fold greater at pH 4 than at pH 7. Our small-scale, short-term (24-h) abiotic experiment conducted with unamended MFT confirmed that adding different salts or decreasing bulk pH by adding acid markedly increased consolidation and porewater recovery from tailings (Figure S3). Greater consolidation occurred in MFT amended with FeCl2 compared to untreated MFT, followed by the effects of CaCl2 and then NaCl; maximum consolidation was achieved using 6N HCl (pH 1). These results support our proposed biogeochemical model (Pathway I, Figure 7), in that: (1) increased I of the MFT decreased DDL of clay particles; (2) divalent cations can exchange monovalent cations from exchanger surfaces (although not observed in current study, this was a significant effect in an acetate-amended 2-L MFT column study; Arkell et al., in review), thereby decreasing DDL; and (3) lower MFT pH dissolved carbonate minerals and changed pH-dependent charges on clays, leading to consolidation of MFT.
The results of this study are important for the management of oil sands tailings. Microbially-enhanced tailings consolidation apparently is occurring in situ (Fedorak et al., 2003), likely supported by endogenous substrates such as light hydrocarbons used in bitumen extraction, where concomitant recovery of porewater can decrease on-site demand for freshwater for oil sand ore processing. Biodensification may also facilitate dry landscape reclamation of MFT by complementing or possibly foregoing current chemical and physical management practices like chemical flocculant addition and energy-intensive centrifugation processes. In addition to oil sands tailings, it would be prudent to consider the roles of indigenous microbes in the consolidation of other organic-rich soft sediments, such as those in contaminated harbors and river deltas, and to incorporate biological factors into consolidation models.
Conflict of Interest Statement
The authors declare that the research was conducted in the absence of any commercial or financial relationships that could be construed as a potential conflict of interest.
Acknowledgments
We gratefully acknowledge funding from Alberta Innovates–Energy and Environment Solutions (Tariq Siddique, Selma Guigard, Julia M. Foght), Genome Canada (Julia M. Foght), Helmholtz-Alberta Initiative (Tariq Siddique, Julia M. Foght), NSERC Discovery Grants (Tariq Siddique, Julia M. Foght) and the School of Energy and the Environment (Julia M. Foght, Tariq Siddique). We thank Syncrude Canada Ltd. for providing tailings and Sanimax for providing dried canola meal. At the University of Alberta, we thank Kathleen Semple for technical assistance and Phillip Fedorak and David Bressler for discussions.
Supplementary Material
The Supplementary Material for this article can be found online at: http://journal.frontiersin.org/journal/10.3389/fmicb.2014.00106/abstract
References
Alberta Environment and Sustainable Resource Development. (2013). Oil sands landcover status 2011. Edmonton, AB: AERSD 1995.
Boone, D. R. (1982). Terminal reactions in the anaerobic digestion of animal waste. Appl. Environ. Microbiol. 43, 57–64.
Bressler, D., Cardenas, M., Fedorak, P. M., Guigard, S., Gupta, R., Siddique, T., et al. (2010). “Microorganisms in oil sand tailings ponds influence the properties and behaviour of mature fine tailings,” in Proceedings of 2nd International Oil Sands Tailings Conference (Edmonton, AB), 44.
Brown, D., Ramos-Padrón, E., Gieg, L., and Voordouw, G. (2013). Effect of calcium ions and anaerobic microbial activity on sedimentation of oil sands tailings. Int. Biodeterior. Biodegradation 81, 9–16. doi: 10.1016/j.ibiod.2012.07.006
Carter, M. R., and Gregorich, E. G. (2008). Soil Sampling and Methods of Analysis. Can. Soc. Soil Sci. Oxford: CRC Press.
Chou, L., Garrels, R. M., and Wollast, R. (1989). Comparative study of the kinetics and mechanisms of dissolution of carbonate minerals. Chem. Geol. 78, 269–282. doi: 10.1016/0009-2541(89)90063-6
Dean, E. W., and Stark, D. D. (1920). A convenient method for the determination of water in petroleum and other organic emulsions. Ind. Eng. Chem. 12, 486–490. doi: 10.1021/ie50125a025
Eslinger, E., Highsmith, P., Albers, D., and De Mayo, B. (1979). Role of iron reduction in the conversion of smectite to illite in bentonites in the disturbed belt, Montana. Clays Clay Miner. 27, 327–338. doi: 10.1346/CCMN.1979.0270503
Essington, M. E. (2004). Soil and Water Chemistry: An Integrative Approach. Boca Raton, FL: CRC Press LLC.
Fedorak, P. M., Coy, D. L., Dudas, M. J., Simpson, M. J., Renneberg, A. J., and MacKinnon, M. D. (2003). Microbially-mediated fugitive gas production from oil sands tailings and increased tailings densification rates. J. Environ. Eng. Sci. 2, 199–211. doi: 10.1139/s03-022
Fotidis, I. A., Karakashev, D., Kotsopoulos, T. A., Martzopoulos, G. G., and Angelidaki, I. (2013). Effect of ammonium and acetate on methanogenic pathway and methanogenic community composition. FEMS Microbiol. Ecol. 83, 38–48. doi: 10.1111/j.1574-6941.2012.01456.x
Himmelheber, D. W., Pennell, K. D., and Hughes, J. B. (2011). Evaluation of a laboratory-scale bioreactive in situ sediment cap for the treatment of organic contaminants. Water Res. 45, 5365–5374. doi: 10.1016/j.watres.2011.06.022
ISO 11465. (1993). Soil Quality – Determination of Dry Matter and Water Content on a Mass Basis – Gravimetric Method. Geneva: Int. Org. Standard.
Jeeravipoolvarn, S., Scott, J. D., and Chalaturnyk, R. J. (2009). 10 m standpipe tests on oil sands tailings: long term experimental results and prediction. Can. Geotech. J. 46, 875–888. doi: 10.1139/T09-033
Jensen, D. L., Boddum, J. K., Tjell, J. C., and Christensen, T. H. (2002). The solubility of rhodochrosite (MnCO3) and siderite (FeCO3) in anaerobic aquatic environments. Appl. Geochem. 17, 503–511. doi: 10.1016/S0883-2927(01)00118-4
Mietta, F., Chassagne, C., and Winterwerp, J. C. (2009). Shear-induced flocculation of a suspension of kaolinite as function of pH and salt concentration. J. Colloid Interface Sci. 336, 134–141. doi: 10.1016/j.jcis.2009.03.044
Morse, J. W., Arvidson, R. S., and Lüttge, A. (2007). Calcium carbonate formation and dissolution. Chem. Rev. 107, 342–381. doi: 10.1021/cr050358j
Moulin, E., Jordens, A., and Wollast, R. (1985). “Influence of the aerobic bacterial respiration on the early dissolution of carbonates in coastal sediments,” in Progress in Belgian Oceanographic Research, eds R. Van Grieken and R. Wollast (Brusells: University of Antwerpen), 196–208.
Nasser, M. S., and James, A. E. (2006). The effect of polyacrylamide charge density and molecular weight on the flocculation and sedimentation behaviour of kaolinite suspensions. Sep. Purif. Technol. 52, 241–252. doi: 10.1016/j.seppur.2006.04.005
Pansu, M., and Gautheyrou, J. (2006). Handbook of Soil Analysis. Mineralogical, Organic and Inorganic Methods. Berlin; Heidelberg: Springer-Verlag. doi: 10.1007/978-3-540-31211-6
Penner, T. J., and Foght, J. M. (2010). Mature fine tailings from oil sands processing harbour diverse methanogenic communities. Can. J. Microbiol. 56, 459–470. doi: 10.1139/W10-029
Rayment, G. E., and Lyons, D. J. (2012). New, comprehensive soil chemical methods book for Australasia. Commun. Soil Sci. Plant Anal. 43, 412–418. doi: 10.1080/00103624.2012.641802
Roberts, D. J. (2002). “Methods for assessing anaerobic biodegradation potential,” in Manual of Environmental Microbiology, eds C. J. Hurst, R. L. Crawford, G. R. Knudson, M. J. McInerney, and L. D. Stetzenbach (Washington, DC: ASM Press), 1008–1017.
Schlegel, M. E., McIntosh, J. C., Bates, B. L., Kirk, M. F., and Martini, A. M. (2011). Comparison of fluid geochemistry and microbiology of multiple organic-rich reservoirs in the Illinois Basin, USA: evidence for controls on methanogenesis and microbial transport. Geochim. Cosmochim. Acta 75, 1903–1919. doi: 10.1016/j.gca.2011.01.016
Schramm, L. L., Stasiuk, E. N., and MacKinnon, M. D. (2000). “Surfactants in Athabasca oil sands slurry conditioning, flotation recovery, and tailings processes,” in Surfactants, Fundamentals, and Applications in the Petroleum Industry, ed L. L. Schramm (Cambridge: Cambridge University Press), 365–430. doi: 10.1017/CBO9780511524844.011
Shen, S., and Stucki, J. (1994). “Effects of iron oxidation state on the fate and behavior of potassium in soils,” in Soil Testing: Prospects for Improving Nutrient Recommendations, eds J. L. Havlin and J. S. Jacobsen (Madison, WI: SSSA Special Publication 40), 173–185.
Siddique, T., Fedorak, P. M., and Foght, J. M. (2006). Biodegradation of short-chain n-alkanes in oil sands tailings under methanogenic conditions. Environ. Sci. Technol. 40, 5459–5464. doi: 10.1021/es060993m
Siddique, T., Fedorak, P. M., Mackinnon, M. D., and Foght, J. M. (2007). Metabolism of BTEX and naphtha compounds to methane in oil sands tailings. Environ. Sci. Technol. 41, 2350–2356. doi: 10.1021/es062852q
Siddique, T., Kuznetsov, P., Kuznetsova, A., Li, C., Young, R., Arocena, J. M., et al. (2014). Microbially-accelerated consolidation of oil sands tailings. Pathway II: solid phase biogeochemistry. Front. Microbiol. 5:107. doi: 10.3389/fmicb.2014.00107
Siddique, T., Penner, T., Klassen, J., Nesbø, C., and Foght, J. M. (2012). Microbial communities involved in methane production from hydrocarbons in oil sands tailings. Environ. Sci. Technol. 46, 9802–9810. doi: 10.1021/es302202c
Siddique, T., Penner, T., Semple, K., and Foght, J. M. (2011). Anaerobic biodegradation of longer-chain n-alkanes coupled to methane production in oil sands tailings. Environ. Sci. Technol. 45, 5892–5899. doi: 10.1021/es200649t
So, H., Menzies, N., Bigwood, R., and Kopittke, P. (2006). Examination into the accuracy of exchangeable cation measurement in saline soils. Commun. Soil Sci. Plant Anal. 37, 1819–1832. doi: 10.1080/00103620600762927
US EPA. (1974). Method 310.2. Alkalinity (Colorimetric, Automated, Methyl Orange). Available online at: http://water.epa.gov/scitech/methods/cwa/methods_index.cfm
US EPA. (1993). Method 365.1 Revision 2.0. Determination of Phosphorous by Semi-Automated Colorimetry. Available online at: http://water.epa.gov/scitech/methods/cwa/methods_index.cfm
Voordouw, G. (2013). Interaction of oil sands tailings particles with polymers and microbial cells: first steps toward reclamation to soil. Biopolymers 99, 257–262. doi: 10.1002/bip.22156
Wersin, P., Leupin, O. X., Mettler, S., Gaucher, E. C., Mäder, U., De Cannière, P., et al. (2011). Biogeochemical processes in a clay formation in situ experiment: part A - overview, experimental design and water data of an experiment in the Opalinus Clay at the Mont Terri Underground Research Laboratory, Switzerland. Appl. Geochem. 26, 931–953. doi: 10.1016/j.apgeochem.2011.03.004
Keywords: methanogenesis, porewater chemistry, biogeochemical pathways, consolidation, oil sands tailings
Citation: Siddique T, Kuznetsov P, Kuznetsova A, Arkell N, Young R, Li C, Guigard S, Underwood E and Foght JM (2014) Microbially-accelerated consolidation of oil sands tailings. Pathway I: changes in porewater chemistry. Front. Microbiol. 5:106. doi: 10.3389/fmicb.2014.00106
Received: 03 December 2013; Accepted: 02 March 2014;
Published online: 21 March 2014.
Edited by:
Jason Raymond, Arizona State University, USAReviewed by:
Amy Michele Grunden, North Carolina State University, USAMark Alexander Lever, Aarhus University, Denmark
Copyright © 2014 Siddique, Kuznetsov, Kuznetsova, Arkell, Young, Li, Guigard, Underwood and Foght. This is an open-access article distributed under the terms of the Creative Commons Attribution License (CC BY). The use, distribution or reproduction in other forums is permitted, provided the original author(s) or licensor are credited and that the original publication in this journal is cited, in accordance with accepted academic practice. No use, distribution or reproduction is permitted which does not comply with these terms.
*Correspondence: Tariq Siddique, Department of Renewable Resources, University of Alberta, 442-Earth Sciences Building, Edmonton, AB T6G 2E3, Canada e-mail:dGFyaXEuc2lkZGlxdWVAdWFsYmVydGEuY2E=