- 1School of Life Sciences, Arizona State University, Tempe, AZ, USA
- 2The Biodesign Institute, Arizona State University, Tempe, AZ, USA
Cyanobacteria are considered good models for biohydrogen production because they are relatively simple organisms with a demonstrable ability to generate H2 under certain physiological conditions. However, most produce only little H2, revert readily to H2 consumption, and suffer from hydrogenase sensitivity to O2. Strains of the cyanobacteria Lyngbya aestuarii and Microcoleus chthonoplastes obtained from marine intertidal cyanobacterial mats were recently found to display much better H2 production potential. Because of their ecological origin in environments that become quickly anoxic in the dark, we hypothesized that this differential ability may have evolved to serve a role in the fermentation of the photosynthate. Here we show that, when forced to ferment internal substrate, these cyanobacteria display desirable characteristics of physiological H2 production. Among them, the strain L. aestuarii BL J had the fastest specific rates and attained the highest H2 concentrations during fermentation of photosynthate, which proceeded via a mixed acid fermentation pathway to yield acetate, ethanol, lactate, H2, CO2, and pyruvate. Contrary to expectations, the H2 yield per mole of glucose was only average compared to that of other cyanobacteria. Thermodynamic analyses point to the use of electron donors more electronegative than NAD(P)H in Lyngbya hydrogenases as the basis for its strong H2 production ability. In any event, the high specific rates and H2 concentrations coupled with the lack of reversibility of the enzyme, at the expense of internal, photosynthetically generated reductants, makes L. aestuarii BL J and/or its enzymes, a potentially feasible platform for large-scale H2 production.
Introduction
Cyanobacteria have great potential to act as cell factories, because they have the ability to use light to split water, potentially generating H2 (Weaver et al., 1980; Akkerman et al., 2002; Prince and Kheshgi, 2005). They do in fact evolve H2 naturally, but as a by-product of N2 fixation, or as an end-product of fermentation. Very transitorily, a burst in H2 production is sometimes seen when the light is switched on suddenly during dark fermentative metabolism. The latter is the only known form of direct “photohydrogen” production in cyanobacteria. The enzyme responsible for N2 fixation, nitrogenase, does also reduce protons and releases H2 as an unavoidable side reaction (Peterson and Burris, 1978; Eisbrenner and Evans, 1983). This process requires significant cellular energy inputs and most often does not result in any net H2 production, because it is reoxidized via an uptake hydrogenase (Peterson and Burris, 1978). It has been proposed that the enzyme bidirectional hydrogenase is involved in fermentative H2 production (Stal and Moezelaar, 1997; Troshina et al., 2002) and photohydrogen generation (Appel et al., 2000). As the name implies this enzyme has the ability to both produce and oxidize H2 (Fujita and Myers, 1965). Direct photohydrogen production in cyanobacteria is extremely short-lived (a few seconds) with rather negligible H2 yields (Appel et al., 2000). Fermentative H2 production represents an indirect hydrophotolytic route that proceeds through an organic intermediary (glycogen). It is relatively long-lived (hours) with somewhat better H2 yields than photohydrogen production (Cournac et al., 2002; Troshina et al., 2002). Fermentative H2 production is in fact the natural mode by which cyanobacteria release H2 for extended periods of time in nature, making it of potential biotechnological interest.
Cyanobacteria have the intrinsic ability to ferment in order to survive dark anaerobic conditions (Gottschalk, 1979). Depending on strain, they have been shown to carry out a variety of fermentative metabolisms including the homolactate, homoacetate, heterolactate, and mixed acid pathways (Stal and Moezelaar, 1997). The homolactate pathway primarily produces lactate (Oren and Shilo, 1979), whereas the heterolactate pathway evolves lactate along with ethanol and acetate (Heyer et al., 1989). The homoacetate pathway produces mostly acetate along with minor quantities of lactate, CO2, and H2 (Heyer et al., 1989; De Philippis et al., 1996). The mixed acid fermentation pathway is known to produce acetate, lactate, ethanol, formate and/or CO2, and H2 (Van der Oost et al., 1989; Moezelaar et al., 1996; Aoyama et al., 1997; Troshina et al., 2002). Thus, the mixed acid and, to a certain extent, the homoacetate pathways result in H2 production.
Cyanobacteria are not known to respire external electron acceptors other than O2, and thus, when subjected to nighttime anoxia must resort to fermentation in order to maintain ATP production and regenerate excess reduction equivalents. A classic example of an environment conducive to this is cyanobacterial benthic mats (Walter, 1976; Bauld, 1981; Javor and Castenholz, 1981). In these mats, oxygenic photosynthetic activity causes the top mat layers to become supersaturated with O2 during the daytime, but strong respiration rates overwhelm diffusive O2 import in the dark, establishing strong anoxia (Revsbech et al., 1983) and forcing the constituent cyanobacteria to ferment its daytime photosynthate. Fermentation products have been directly detected in hot spring microbial mats (Anderson et al., 1987; Nold and Ward, 1996). Amongst the mat inhabiting cyanobacteria, fermentation has been reported in Oscillatoria terebriformis (Richardson and Castenholz, 1987) and Synechococcus sp. strains OS-A and OS-B (Steunou et al., 2006) from hot springs. Fermentation has also been studied in marine microbial mat-building Lyngbya aestuarii CCY 9616 (= PCC 8106, also known as Oscillatoria limosa in the early literature) and Oscillatoria sp. SAG 3192 (Garcia-Pichel et al., 1996) [also referred to as Microcoleus chthonoplastes 11 or M. chthonoplastes SAG 3192 before (Stal and Krumbein, 1985)]. L. aestuarii CCY 9616 follows a homoacetate–heterolactate pathway (Heyer et al., 1989) whereas Oscillatoria sp. SAG 3192 ferments via a mixed acid fermentation pathway (Moezelaar et al., 1996).
Owing to the presence, multiplicity, and avidity of potential H2 consumers in the complex microbial communities where H2 is being produced, steady-state concentrations of H2 tend to remain very low, usually undetectable in natural systems (Ebert and Brune, 1997; Schink, 1997). This general rule finds a clear exception in some intertidal microbial mats, where net H2 accumulation and export has been reported (Skyring et al., 1989; Hoehler et al., 2001). Similarly, it was observed that intertidal microbial mats from Baja California, maintained in a greenhouse setting for more than 3 years under an artificial intertidal regime, continue to produce H2 at night, exporting significant amounts to the overlying waters (Hoffmann and Maldonado, personal communication). The organisms fermenting under such conditions must thus be able to produce H2 even against high partial pressures in the mat.
In an earlier report surveying a set of cyanobacterial strains for H2 production in presence of excess reductants, two different patterns were observed. Pattern 1, known from fresh water strains such as Synechocystis sp. PCC 6803, exhibited low rates and steady-state H2 concentrations followed by uptake of most of the produced H2 whereas the novel Pattern 2, found only in L. aestuarii and M. chthonoplastes strains from the marine intertidal mats, exhibited much higher rates, steady-state H2 concentrations, and a lack of H2 uptake throughout the assay (Kothari et al., 2012). Indeed, the cyanobacterial strains isolated from these mats displayed an extraordinary potential to produce/sustain H2 under the unusually high concentrations of H2 prevailing in their micro-environment. However, all of this was done using standard assays (Kothari et al., 2012) that externally provide excess reductant and anaerobic conditions. Now these studies are extended to include the innate H2 evolving capacity under fermentative conditions, using microbiological and genomic evidence.
Materials and Methods
Strains, Media, and Growth Conditions
Five strains of cyanobacteria were used for this work. L. aestuarii BL J, L. aestuarii BL AA, and M. chthonoplastes BM 003 were isolated from marine intertidal microbial mats in Baja California (Kothari et al., 2012). M. chthonoplastes PCC 7420 was originally isolated from a microbial mat in a salt marsh, Woods Hole, Massachusetts.
Synechocystis sp. PCC 6803, originally a freshwater isolate, has been used in this study since it is a popular model cyanobacterium for biohydrogen research. The latter two strains were obtained from the Pasteur Culture Collection (http://www.pasteur.fr/ip/easysite/pasteur/en/institut-pasteur). L. aestuarii strains were grown in IMR medium with 3% salinity (Eppley et al., 1968), modified to incorporate commercially available Instant Ocean salt mixture instead of natural seawater. M. chthonoplastes strains were grown in a 1:1 mixture of IMR and ASN III media (Rippka et al., 1979) with 3% salinity. Synechocystis sp. PCC 6803 was grown in BG11 medium (Rippka et al., 1979). All media were supplemented with 0.5 mM (final concentration) NiSO4 to ensure adequate supply of nickel for the working of Ni–Fe hydrogenases.
The strains L. aestuarii BL J, L. aestuarii BL AA, and M. chthonoplastes BM 003 were clonal and monocyanobacterial, but not always axenic. Therefore, phase contrast microscopy was used to confirm that the level of contaminating bacteria was less than 0.01% of the cyanobacterial biomass (assessed as bio-volume) for the physiological experiments. M. chthonoplastes PCC 7420 and Synechocystis sp. PCC 6803 were always used in axenic form.
For the purpose of whole genome sequencing, an axenic culture of L. aestuarii BL J was established by picking up the motile hormogonia developing on IMR medium—1% nobel agar plates (Rippka, 1988). These hormogonia were allowed to grow on IMR–PGY medium 1% nobel agar plates (0.25% peptone, 0.25% yeast extract, 0.25% glucose, 1.5% agar), and axenicity was determined by lack of heterotrophic bacterial growth, and through direct microscopic observation. All strains were maintained in 250 ml Erlenmeyer flasks, with 100 ml medium, starting with similar amounts of inoculum in presence of light at an intensity of 100 μmol photon m−2 s−1.
Fermentative H2 Production Assay
All strains were subjected to two different sets of growth conditions for the fermentation assays. In the first set, filaments were grown in continuous light without any bubbling (CL). In the second set, the strains were grown in 12-h light and 12-h dark cycle. The cultures were bubbled with air in the light period and with N2 in the dark period to establish anoxia, forcing cells to ferment. These conditions are referred to as “Light Oxic Dark Anoxic” (LODA) conditions. All cultures were incubated for a minimum of two weeks before making any measurements. The assay itself was carried out in the dark using whole cells (in vivo) without the addition of any external reductants. Particularly, for the cultures growing in LODA conditions, the assay was commenced at the beginning of the dark period. Small pea size pellets of biomass from log phase cultures were placed in a custom-made, 2.5 ml volume chamber with continuous stirring. Fresh medium was added to completely fill the chamber, which was sealed with no headspace. The chamber was endowed with two miniature Clark-type electrodes to monitor H2 and O2 partial pressure. The electrodes were connected to a pico-ammeter set at a voltage of 0.8 V for H2 and -0.8 V for O2. An A/D converter allowed the current signal data to be read on a computer using Sensor Trace Basic software. All electrodes and peripherals were from Unisense, Aarhus, Denmark. Before each measurement, the H2 electrode was subject to a two-point calibration in culture medium bubbled with either air (0% H2) or with a custom gas mixture (10% H2 in N2). The O2 electrode was also subjected to a two-point calibration system wherein culture medium was bubbled with either air (21% O2) or with 100% N2 (0% O2). During calibration the sealed chamber showed negligible leakage over a period of 2–3 h.
Each strain was measured in independent triplicate experiments. From the electrode traces, the following parameters were derived: the initial specific rate of fermentative H2 production, RH; the maximum steady-state H2 concentration reached, [H2]M; and the time after which H2 production stopped and reverted to consumption, TR. The measurements lasted for 24 h. At the end of the assay, chlorophyll was extracted from the biomass with 100% methanol and measured spectrophotometrically (MacKinney, 1941). This was done to ensure that all assays had roughly comparable biomass and to obtain specific rates of initial H2 production (i.e., per unit biomass).
Analysis of Fermentation Metabolism in L. aestuarii BL J
L. aestuarii BL J that had been grown in LODA conditions from three different flasks (replicates) were used in this assay. A couple of hours before the onset of a dark anaerobic period, biomass was harvested by centrifugation, acclimatized to fresh medium, and washed twice with fresh medium to get rid of any potentially existing fermentation products. The filaments form tight clumps, and hence, attempts were made to break the clumps using forceps and mild sonication at the lowest speed setting for 4 s to get a homogeneous cell suspension. This was required to split the biomass into two aliquots with approximately equal amounts of biomass to conduct the initial and final analyses quantifying the fermentation substrates and products. Since a non-destructive procedure that does not impart any kind of stress to the cells was necessary for quantifying the biomass, wet weights were used. Optical density cannot be employed for biomass estimation given the filamentous and clumpy nature of this strain. As described below, since only the wet weights from the two halves of the same filter were compared to each other, the errors in biomass estimation were minimized.
For obtaining two aliquots with approximately equal amounts of biomass using wet weights, the following procedure was adopted. The biomass was vacuum filtered onto a 0.4 μm polycarbonate filter to establish a homogenous layer on it. The filter was cut into half, biomass scrapped off, and the wet weight of the cells on each half was measured. The biomass from each half of the filter was then introduced into a 10 ml serum bottle (one for initial and one for final analyses). To each bottle 5 ml of fresh medium was added and the bottles were sealed. To confirm the initial absence of fermentation products, 1 ml of medium was drawn out from the “initial” serum bottle for later High Pressure Liquid Chromatography (HPLC) analysis. The rest was immediately frozen in liquid N2 and stored at −80°C to be used eventually in determining the initial fermentable glycogen content in the cells. The “final” serum bottle was bubbled with nitrogen for 30 min to establish anoxia. Gas Chromatography (GC) confirmed the absence of O2, and the serum bottle was incubated in the dark on a rocking bench for 24 h at 25°C.
After incubation, 1 ml of medium was withdrawn for HPLC analyses of organic acids and ethanol. Hydrochloric acid was then added to the serum bottle to lower the pH of the solution and ensure that all the inorganic carbon was present as CO2. The gases in the headspace (CO2, H2, and/or O2) were sampled by syringe and quantified by GC equipped with a thermal conductivity detector. GC was performed with Helium as the carrier gas and the concentrations of H2 and CO2 in the headspace were estimated as described before (Parameswaran et al., 2009). Total masses of gases were back-calculated according to volumetric partitioning. The contents of the serum bottle were then frozen in liquid N2 and kept at −80°C for eventual glycogen content quantification.
Glycogen was extracted as per Ernst et al. (1984) and quantified using a BioAssay Systems glycogen assay kit. To determine organic acids and ethanol, HPLC was employed. All the liquid samples for HPLC were filtered through a 0.2 mm PVDF filter and the filtrate used. The HPLC was performed with Aminex HPX–87H column at 50°C with 2.5 mM sulfuric acid as eluent at a flow rate of 0.6 ml/min using a photodiode array and refractive index indicator (Parameswaran et al., 2009). Most of the common products of bacterial fermentation can be detected under these settings.
Genomic and Bioinformatics Analyses
The genomic DNA preparation of L. aestuarii BL J was subjected to MiSeq 250 Illumina sequencing, assembly, and annotation (Kothari et al., 2013). The genomic sequence of L. aestuarii BL J was checked for the presence of orthologs of genes potentially coding for key enzymes involved in fermentation. Protein sequences coding for cyanobacterial fermentation enzymes from NCBI database were used as query and Psi BLAST was performed against the entire L. aestuarii BL J genome. Given that the genome is not closed, the absence of any one gene does not necessarily imply its absence from the genome, as there is a small probability that it is found in unsequenced regions. This Whole Genome Shotgun project has been deposited at DDBJ/EMBL/GenBank under the accession AUZM00000000. The version described in this paper is version AUZM01000000.
Results
Fermentative H2 Production
The strains L. aestuarii BL J, L. aestuarii BL AA, M. chthonoplastes BM 003, M. chthonoplastes PCC 7420, and Synechocystis sp. PCC 6803 were all capable of fermentative H2 production. All strains reached anoxic conditions solely by dark respiration without the addition of any external reductants, anoxia-inducing compounds, or fermentable substrates. As soon as anoxia was established, H2 production commenced without any measurable lag time in all strains.
Some variation in the parameters of fermentative H2 production could be detected. These main parameters are initial specific rate, RH, the maximum H2 steady-state concentration, [H2]M, and the time after which the enzyme reverts in direction, TR (Figure 1). Table 1 gathers information on these parameters for all the five tested strains. In general, Lyngbya and Microcoleus strains from microbial mats produced H2 faster and could reach higher equilibrium concentrations of H2 than the standard strain Synechocystis sp. PCC 6803. Lyngbya and Microcoleus strains did not consume the H2 produced during the assay (for up to 24 h) unlike Synechocystis sp. PCC 6803. The highest specific rate of H2 production was seen in L. aestuarii BL AA and the highest steady-state H2 concentration was seen in M. chthonoplastes BM 003.
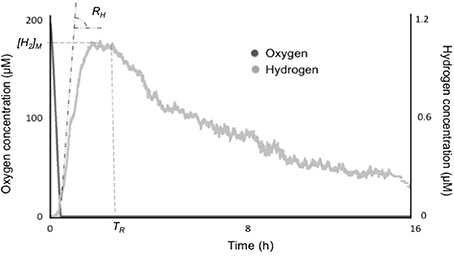
Figure 1. Oxygen and hydrogen concentrations during a fermentative H2 production assay in Synechocystis sp. PCC 6803. Anoxia is established in a few minutes by respiration in dark followed by onset of fermentative H2 production. The parameters of H2 production studied are the maximal initial rate of H2 production, RH, the maximum steady-state H2 concentration [H2]M, and the time, after which hydrogenase reverses in direction, TR.
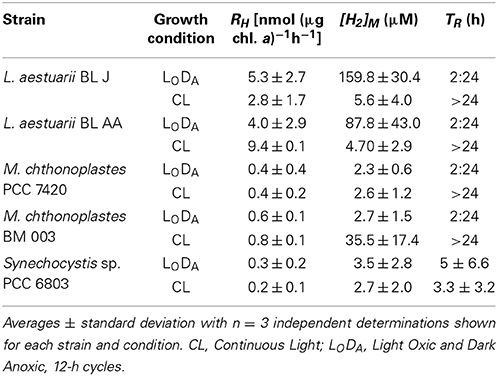
Table 1. Parameters characterizing the dynamics of fermentative H2 production in various cyanobacterial strains along with the effect of prior exposure to nighttime anoxia.
Optimization of Fermentative H2 Production
Attempts were made to optimize the H2 produced by acclimatizing the cells to 12-h light/dark cycles wherein the cells were exposed to anoxia in the dark (LODA). Synechocystis sp. PCC 6803 showed no significant improvements by this preconditioning in any of the parameters. The specific rates and steady-state concentrations of fermentative H2 production attained in Lyngbya strains, but not those of Microcoleus strains, could be enhanced when cultures were pre-acclimated to recurrent nighttime anaerobiosis during growth. On subjecting the strains to LODA preconditioning all strains retained their characteristic feature of reversibility of reaction direction (or the lack of it). L. aestuarii BL J reached the highest specific rates and steady-state concentrations of H2. The RH of L. aestuarii BL J grown in LODA condition doubled compared to that of cells grown in continuous light conditions; its [H2]M increased 28-fold (Figure 2). In LODA conditions, the strain BL J performed exceptionally better than the standard Synechocystis sp. PCC 6803, [its RH was 20-fold faster and [H2]M 45-fold higher (Figure 2)]. While calculating the average RH of L. aestuarii BL J grown in LODA one abnormally high specific rate of 44.2 nmol (μg chl. a)−1h−1 was removed from the tally. Had this been incorporated, the RH value would have been 13.1 ± 17.5 nmol (μg chl. a)−1h−1.
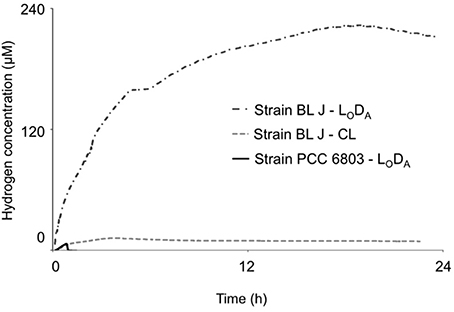
Figure 2. Comparison of the dynamics of fermentative hydrogen production in continuous light (CL) grown L. aestuarii BL J, along with Light Oxic Dark Anoxic (LODA) cycle grown L. aestuarii BL J and Synechocystis sp. PCC 6803.
Attempts made to further optimize the fermentative H2 production from L. aestuarii BL J in LODA conditions by varying the salinity, nickel and nitrate content in the medium did not lead to any significant increase in the specific rates or steady-state H2 concentrations (data not shown). On starving cells of nickel, however, a 15-fold decrease in the specific rates of fermentative H2 production was observed, indicating the nickel dependency of the enzyme system involved in the process.
Fermentation in L. aestuarii BL J
Since L. aestuarii BL J displayed the highest RH and [H2]M, it was chosen for further analysis. Along with H2, fermentative production of lactate, ethanol, acetate, and CO2 was observed. The ratio of the products of fermentation remained similar for the three independent replicate experiments. Small amounts of pyruvate were also excreted. Other common bacterial fermentation products such as formate, succinate, propionate, and butyrate were not detected. Table 2 depicts a quantitative balance analysis of the fermentation process in L. aestuarii BL J. Ethanol and acetate were produced in equimolar amounts. Lactate, ethanol, and acetate were seen in 1:2:2 molar ratios. One mol of H2 was produced for every 2 moles of CO2. In our experiments, the stoichiometry of carbon recovery and the recovery of H available was 100.07 and 100.58%, respectively.
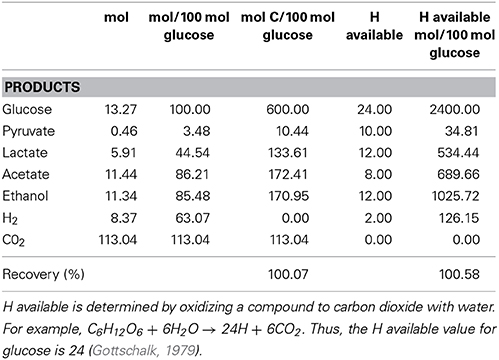
Table 2. Stoichiometry of fermentation of endogenous polyglucose and the fermentation mass balance of L. aestuarii strain BL J, after 24 h of dark incubation.
Genomic Evidence
Based on the whole genome sequence of the strain BL J (Kothari et al., 2013), orthologs of the following genes involved in glycogen metabolism were detected: Glucose-1-phosphate adenylyltransferase, glycogen synthase, ADP-glucose transglucosylase, glycogen branching enzyme (GH-57-type, archaeal), 1,4-alpha-glucan (glycogen) branching enzyme (GH-13-type), glycogen debranching enzyme, glycogen phosphorylase, and 4-alpha-glucanotransferase (amylomaltase). Orthologs of genes coding for all the enzymes involved in the pentose phosphate pathway and glycolysis, potential routes for the breakdown of glucose into pyruvate, were detected.
Genomic evidence for the presence of mixed acid fermentation pathway was clear. L. aestuarii BL J has orthologs coding for the enzymes pyruvate:ferredoxin oxidoreductase, ferredoxin:NADP oxidoreductase, bidirectional hydrogenase, lactate dehydrogenase, phosphotransacetylase, acetaldehyde dehydrogenase, alcohol dehydrogenase, and acetate kinase (see supplementary information for gene accession numbers). Based on the fermentation products obtained experimentally and the presence of these orthologs, the pathway proposed for fermentative degradation of glycogen is depicted in Figure 3. Notably, the gene for pyruvate formate lyase, involved in the reversible conversion of pyruvate and coenzyme-A into formate and acetyl-CoA, was not detected. This was consistent with a lack of formate amongst the fermentation products. Also worth noting is that no genomic evidence could be found for formate hydrogen lyase, involved in splitting of formate into H2 and CO2.
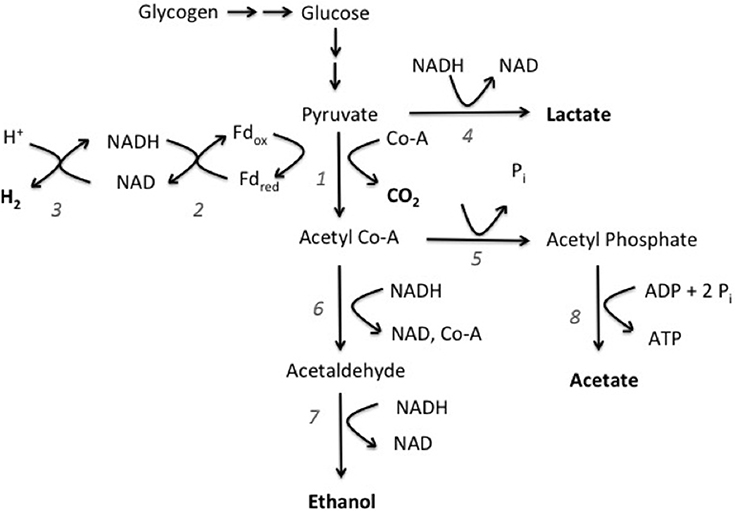
Figure 3. Proposed fermentation pathway of glycogen (polyglucose) fermentation in L. aestuarii BL J. Compounds in bold are fermentation products. The numbers refer to the enzymes involved: 1, pyruvate ferredoxin oxidoreductase; 2, ferredoxin NADP oxidoreductase; 3, bidirectional hydrogenase; 4, lactate dehydrogenase; 5, phosphotransacetylase; 6, acetaldehyde dehydrogenase; 7, alcohol dehydrogenase; 8, acetate kinase.
It was of interest to positively identify the enzyme responsible for the intense H2 evolution observed in this study, as this may be the target of future studies. H2 production in fermentative pathways can be either of Enteric type, with H2 evolving from formate breakdown by formate hydrogen lyase, or of Clostridial type, wherein H2 is evolved by pyruvate:ferredoxin oxidoreductase along with a hydrogenase (Hallenbeck, 2009). Based on the genome, L. aestuarii BL J lacks both enzymes for the Enteric pathway, but contains a pyruvate:ferredoxin oxidoreductase and two [Ni–Fe] hydrogenases, an uptake-type hydrogenase and a bidirectional-type hydrogenase. One of these two must be involved, given that fermentative H2 production in L. aestuarii BL J was found to be Ni-dependent. Because uptake hydrogenases are not known to produce H2 under physiological conditions (Houchins and Burris, 1981; Houchins, 1984) we propose that the Ni–Fe bidirectional hydrogenase is the source of the fermentative H2 produced in L. aestuarii BL J.
The strain BL J contains homologs of genes coding for nitrogenase, an enzyme that could in theory contribute to H2 production in this strain. However, since the fermentation assays were performed in presence of nitrate, a condition in which the nitrogenase is known to be inactive (Ferreira, 2009), the role of the enzyme in production of H2 is ruled out. The homologs of genes coding for uptake hydrogenase are also present in the strain BL J. However, the uptake hydrogenase is not capable of H2 production in physiological conditions; in fact, it has to be knocked out to attain considerable H2production via the nitrogenase (Lindberg et al., 2002; Lindblad et al., 2002; Masukawa et al., 2002; Yoshino et al., 2007) and also via the fermentation pathway (Kim et al., 2006; Zhao et al., 2009).
Discussion
The Physiological Basis for Strong Fermentative Hydrogen Production
We had previously demonstrated that in standard H2 production assays, strains of Lyngbya and Microcoleus displayed optimal H2 evolution characteristics compared to a large number of other strains from diverse environments (Kothari et al., 2012). Since excess reductants were externally provided during that assay, the results likely are maximal potential specific rates and do not actually reflect physiologically realistic conditions. This prompted us to study the actual H2 production capacity of these strains. Here we demonstrate that all the four strains studied had the capacity to produce fermentative H2 naturally, at the expense of photosynthetically fixed carbon, as did the standard strain Synechocystis sp. PCC 6803, which is included for reference. As reported earlier (Cournac et al., 2002), the fermentative H2 evolution in Synechocystis sp. PCC 6803 commenced without any lag time, as was the case in Microcoleus and Lyngbya strains, and unlike what was observed in Microcystis aeruginosa M-176 (Asada and Kawamura, 1984). In general, the specific H2 production rates and the steady-state concentrations under fermentative conditions were about an order of magnitude lower than the potential seen in standard assays in presence of excess reductant (Kothari et al., 2012). The Microcoleus and Lyngbya strains from the marine intertidal mats are capable of sustained fermentative H2 production for at least 24 h. This was in accordance to the Pattern 2 H2 production earlier reported in these strains via the hydrogenase activity assay wherein sustained H2 production was also measured for up to 24 h. In comparison, the H2 production phase did not last more than about 3 h in the standard strain Synechocystis sp. PCC 6803 (in agreement with Pattern 1 H2 production earlier reported in this strain). This is consistent with the notion that cyanobacteria isolated from environments experiencing recurring nighttime anoxia (marine microbial mats) may be innately better H2 producers, thus validating a general approach of bio-prospecting in nature for biotechnologically useful properties of extant but little known microbes.
That H2 production metabolism in intertidal mat harboring Lyngbya strains was enhanced by prior exposure to recurrent dark anaerobic growth conditions was expected under the premise that this type of fermentative metabolism would be regulated and thus subject to induction. This was clearly not the case in Microcoleus strains, where the capacity for fermentative H2 generation, while high, seemed to be constitutive. Lyngbya typically colonizes microbial mats that desiccate frequently and are not exposed to nighttime anoxia as frequently as Microcoleus, which tends to dominate mats lower in the tidal gradient, with more recurrent flooding or always flooded (Javor and Castenholz, 1981; Rothrock and Garcia-Pichel, 2005). Perhaps the different responsiveness of the fermentative H2 physiology has to do with this differential ecology. Synechocystis sp. PCC 6803, which has been in culture since 1968 does presumably not see many periods of dark anoxia during cultivation, and displayed a low-yield, non-inducible H2 physiology.
When forced to ferment on a diel cycle, the highest specific rate and steady-state H2 concentration was exhibited by L. aestuarii BL J. In comparison, to Synechocystis sp. PCC 6803, the initial rates of H2 production were 17-fold higher in L. aestuarii BL J in the optimized fermentation assays. Most likely, this is due to increased content of bidirectional hydrogenase in the strain BL J or because Synechocystis sp. PCC 6803 employed alternative strategies in dark anaerobic conditions to regenerate NAD(P)+ or because the Michaelis constant (KM) of the bidirectional hydrogenase in the strain BL J is more favorable for H2 production.
The major difference between L. aestuarii BL J and Synechocystis sp. PCC 6803 was that the latter exhibited a decline in the concentrations of H2 leading to the consumption of almost all the H2 produced. This decline was seen in presence of excess external reductants (Kothari et al., 2012) and internal reductants (Figure 1) implying that the concentration of reductants was not the limiting factor. The observed decline also has little to do with the loss of enzyme activity, since the bidirectional hydrogenase works in the direction of H2 consumption and is thus still active. We must thus postulate a regulatory cause for these differences.
The optimized steady-state H2 concentrations in fermentative assays in the strain BL J were only three-fold lower in magnitude than those seen in standard assays in the presence of excess reductant (Kothari et al., 2012). This is suggestive of the presence of a strong H2 producing system, which, might be of particular fitness value in the uniquely H2 accumulating intertidal mats. In optimized fermentation assays, the steady-state H2 concentration in the strain BL J was 45-fold higher than that reached by Synechocystis sp. PCC 6803. Free sugar concentrations during fermentation are unlikely to be so different between the two cyanobacteria to account for such differences, and, even in the presence of excess externally provided reductants, the steady-state H2 concentration in Synechocystis sp. PCC 6803 was 15-fold lower than the strain BL J (Kothari et al., 2012). Again here, the only possible explanation for this behavior is some sort of regulation of the hydrogenase enzyme in the strain PCC 6803.
On the basis of thermodynamics it may be theoretically possible (albeit difficult) for NAD(P)H to act as the sole electron donor to the bidirectional hydrogenase in Synechocystis sp. PCC 6803 during the fermentation assay, The intracellular ratio of [NAD(P)H] to [NAD(P)+] required for Synechocystis sp. PCC 6803 to produce 3 μM H2 at equilibrium is 7.63. In Synechocystis sp. PCC 6803, the measured [NADPH]/[NADP+] is 3.03 under light oxic conditions (Cooley and Vermaas, 2001), but in dark anaerobic conditions the cell is even more reduced, so the ratios are expected to be higher. This is not unlike the ratios found in heterotrophic bacteria, which can go up to 6.66–0.7 (Decker and Pfitzer, 1972; Lee et al., 2009; Siedler et al., 2011). However, the [NAD(P)H]/[NAD(P)+] theoretically required to produce 150 μM H2 at equilibrium, like L. aestuarii BL J does, is 385. It is highly unlikely such ration can be achieved by the cell and hence it is implausible for NAD(P)H to act as the sole electron donor to the bidirectional hydrogenase in L. aestuarii BL J during the fermentation assay.
We propose that the Lyngbya can make so much H2 because they efficiently use more electronegative electron donors for the bidirectional hydrogenase than NAD(P)H. Flavin adenine dinucleotide (FAD) (−0.219 to −0.400 V) (Nelson et al., 2008; Faro et al., 2002), thioredoxin (−0.200 to −0.350 V) (Krause et al., 1991) and ferredoxin (−0.432 V) (Nelson et al., 2008), could all act as potential electron donors for the bidirectional hydrogenase enzyme. In fact, a recent study (Gutekunst et al., 2014) proposed that the bidirectional hydrogenase mediated H2 production in Synechocystis sp. PCC 6803 is coupled to ferredoxin and flavodoxin. If this is indeed true, it is of interest to speculate why the diaphorase subunit [involved in interactions with NAD(P)H and NAD(P)+] is associated with bidirectional hydrogenase in most cyanobacterial strains. Perhaps the main role of the diaphorase is in the oxidation of H2, to regenerate NAD(P)H.
As an aside and a caveat, if the intracellular pH of the strain BL J was as low as five, that would bring the required [NAD(P)H]/[NAD(P)+] to 3.8, making it feasible to attain high H2 concentrations using NAD(P)H as an electron donor. However, such intracellular pH would be unprecedented, particularly given the extreme sensitivity of cyanobacteria to even moderately acidic conditions.
Fermentation Pathways Among the Filamentous Non-Heterocystous Cyanobacteria
L. aestuarii BL J has all the products and the orthologs of genes coding for all enzymes of the mixed acid fermentation pathway. In view of the stoichiometric ratios of the products of fermentation (Table 2), our strain does not follow any one ideal fermentation pathway or even a combination of pathways. This is also the case for Cyanothece sp. PCC 7822 (Van der Oost et al., 1989), Microcystis sp. PCC 7806 (Moezelaar and Stal, 1994), and Oscillatoria sp. SAG 3192 (Moezelaar et al., 1996). The fermentation pathway is likely to be similar to that observed in Microcystis sp. PCC 7806 (Moezelaar and Stal, 1994) and Gloeocapsa alpicola CALU 743 (Troshina et al., 2002).
Since L. aestuarii BL J accumulates very high concentrations of H2, it was of significance to characterize the molar conversion ratio of glucose to H2. Theoretically 1 mole of glucose can give rise to a maximum of 4 moles of H2 via fermentation (Thauer, 1977). Amongst cyanobacteria, this theoretical maximum has been observed only in G. alpicola CALU 743 (Troshina et al., 2002). Microcystis sp. PCC 7806 yields 0.51 (Moezelaar and Stal, 1994) and Cyanothece sp. PCC 7822 yields 0.76 moles/mol (Van der Oost et al., 1989). L. aestuarii BL J, at 0.6 moles/mol, was certainly not the best. The strong H2 production characteristics of L. aestuarii BL J cannot be attributed to a high glucose to H2 molar conversion ratio, but likely reside in the bidirectional hydrogenase enzyme system.
It may be of interest to discuss the fermentative metabolism of strain BL J in comparison to the few other closely related cyanobacterial species whose fermentation has been studied in any detail. L. aestuarii CCY 9616, a strain phylogenetically close to L. aestuarii BL J (99% identity based on 16S rRNA), behaves quite differently: it can ferment trehalose, its osmoprotectant, via the homoacetate pathway into mostly acetate with small amounts of H2 and CO2 (Heyer et al., 1989). It can also ferment glycogen by a heterolactic fermentation pathway producing equimolar amounts of ethanol, lactate, and CO2 (Heyer et al., 1989). It is unknown if L. aestuarii BL J has the same osmoprotectant, or if it can be metabolized via a similar fermentation. This is however unlikely, in that neither the enzyme trehalase, involved in trehalose breakdown, nor the carbon monoxide dehydrogenase, a key enzyme for homoacetic fermentation, could be detected in its genome. Notably, the genome had orthologs of the enzymes trehalose synthase and trehalose-6-phosphate synthetase involved in trehalose synthesis. This may be worth a direct assessment. Another closely related strain, the thermophilic O. terebriformis, was also fermentatively distinct; it produced lactate by anaerobic degradation of photosynthetically accumulated glycogen, without producing acetate, butyrate, isobutyrate or n-butyrate (Richardson and Castenholz, 1987). In comparison, L. aestuarii BL J produces equimolar amounts of ethanol and acetate, a characteristic feature also seen in more distantly related Oscillatoria sp. SAG 3192, which also uses the mixed acid fermentation pathway (Moezelaar et al., 1996). Phylogeny seems thus to be a poor predictor of fermentative pathways.
In conclusion, the H2 production in the strain BL J is unique in accumulating high steady-state concentrations of H2 thus simplifying harvest of the end product and making it desirable for long-term applications.
Author Contributions
Concept by Ankita Kothari and Ferran Garcia-Pichel, all the experimental work and analysis done by Ankita Kothari and with assistance from Prathap Parameswaran on HPLC analysis. Writing by Ankita Kothari and editorial help by Ferran Garcia-Pichel.
Conflict of Interest Statement
The authors declare that the research was conducted in the absence of any commercial or financial relationships that could be construed as a potential conflict of interest.
Acknowledgments
This research and Ankita Kothari were supported by an endowment from B. Swette through the ASU President's Fusion Fund. The funding source had no involvement in experimental design, data collection, analysis, report writing or decision to submit article. We thank Dr. Willem F. J. Vermaas, Dr. Cesar Torres, and Dr. Jens Appel for assistance with thermodynamic calculations.
Supplementary Material
The Supplementary Material for this article can be found online at: http://www.frontiersin.org/journal/10.3389/fmicb.2014.00680/abstract
References
Akkerman, I., Janssen, M., Rocha, J., and Wijffels, R. H. (2002). Photobiological hydrogen production: photochemical efficiency and bioreactor design. Int. J. Hydrogen Energy 27, 1195–1208. doi: 10.1016/S0360-3199(02)00071-X
Anderson, K. L., Tayne, T. A., and Ward, D. M. (1987). Formation and fate of fermentation products in hot spring cyanobacterial mats. Appl. Environ. Microbiol. 53, 2343–2352.
Aoyama, K., Uemura, I., Miyake, J., and Asada, Y. (1997). Fermentative metabolism to produce hydrogen gas and organic compounds in a cyanobacterium, Spirulina platensis J. J. Ferment. Bioeng. 83, 17–20. doi: 10.1016/S0922-338X(97)87320-5
Appel, J., Phunpruch, S., Steinmuller, K., and Schulz, R. (2000). The bidirectional hydrogenase of Synechocystis sp. PCC 6803 works as an electron valve during photosynthesis. Arch. Microbiol. 173, 333–338. doi: 10.1007/s002030000139
Pubmed Abstract | Pubmed Full Text | CrossRef Full Text | Google Scholar
Asada, Y., and Kawamura, S. (1984). Hydrogen evolution by Microcystis aeruginosa in darkness. Agric. Biol. Chem. 48, 2595–2596. doi: 10.1271/bbb1961.48.2595
Bauld, J. (1981). “Occurrence of benthic microbial mats in saline lakes,” in Salt Lakes, ed W. D. Williams (Dordrecht: Springer), 87–111.
Cooley, J. W., and Vermaas, W. F. J. (2001). Succinate dehydrogenase and other respiratory pathways in thylakoid membranes of Synechocystis sp. strain PCC 6803: capacity comparisons and physiological function. J. Bacteriol. 183, 4251–4258. doi: 10.1128/JB.183.14.4251-4258.2001
Pubmed Abstract | Pubmed Full Text | CrossRef Full Text | Google Scholar
Cournac, L., Mus, F., Bernard, L., Guedeney, G., Vignais, P., and Peltier, G. (2002). Limiting steps of hydrogen production in Chlamydomonas reinhardtii and Synechocystis PCC 6803 as analysed by light-induced gas exchange transients. Int. J. Hydrogen Energy 27, 1229–1237. doi: 10.1016/S0360-3199(02)00105-2
Decker, K., and Pfitzer, S. (1972). Determination of steady-state concentrations of adenine nucleotides in growing C. kluyveri cells by biosynthetic labeling. Anal. Biochem. 50, 529–539.
De Philippis, R., Margheri, M. C., and Vincenzini, M. (1996). Fermentation in symbiotic and free-living cyanobacteria. Arch. Hydrobiol. 83, 459–468.
Ebert, A., and Brune, A. (1997). Hydrogen concentration profiles at the oxic-anoxic interface: a microsensor study of the hindgut of the wood-feeding lower termite Reticulitermes flavipes (Kollar). Appl. Environ. Microbiol. 63, 4039–4046.
Eisbrenner, G., and Evans, H. J. (1983). Aspects of hydrogen metabolism in nitrogen-fixing legumes and other plant-microbe associations. Annu. Rev. Plant Physiol. Plant Mol. Biol. 34, 105–136. doi: 10.1146/annurev.pp.34.060183.000541
Eppley, R. W., Holmes, R. W., and Strickland, J. D. H. (1968). Sinking rates of marine phytoplankton measured with a fluorometer. J. Exp. Mar. Biol. Ecol. 1, 191–208.
Ernst, A., Kirschenlohr, H., Diez, J., and Boger, P. (1984). Glycogen content and nitrogenase activity in Anabaena variabilis. Arch. Microbiol. 140, 120–125. doi: 10.1007/BF00454913
Faro, M., Gómez-Moreno, C., Stankovich, M., and Medina, M. (2002). Role of critical charged residues in reduction potential modulation of ferredoxin-NADP+ reductase. Eur. J. Biochem. 269, 2656–2661. doi: 10.1046/j.1432-1033.2002.02925.x
Pubmed Abstract | Pubmed Full Text | CrossRef Full Text | Google Scholar
Ferreira, D. (2009). Nitrogen Fixation and Transcription/Regulation of Genes Related to Hydrogenases in Lyngbya spp. Porto: Universidade do Porto.
Fujita, Y., and Myers, J. (1965). Hydrogenase and NADP-reduction reactions by a cell-free preparation of Anabaena cylindrica. Arch. Biochem. Biophys. 111, 619–625.
Garcia-Pichel, F., Prufert-Bebout, L., and Muyzer, G. (1996). Phenotypic and phylogenetic analyses show Microcoleus chthonoplastes to be a cosmopolitan cyanobacterium. Appl. Environ. Microbiol. 62, 3284–3291.
Gottschalk, G. (1979). Bacterial Metabolism. Gottingen, Germany: Springer. doi: 10.1007/978-1-4684-0465-4
Gutekunst, K., Chen, X.i., Schreiber, K., Kaspar, U., Makam, S., and Appel, J. (2014). The bidirectional NiFe-hydrogenase in Synechocystis sp. PCC 6803 is reduced by flavodoxin and ferredoxin and is essential under mixotrophic, nitrate-limiting conditions. J. Biol. Chem. 289, 1930–1937. doi: 10.1074/jbc.M113.526376
Pubmed Abstract | Pubmed Full Text | CrossRef Full Text | Google Scholar
Hallenbeck, P. C. (2009). Fermentative hydrogen production: principles, progress, and prognosis. Int. J. Hydrogen Energy 34, 7379–7389. doi: 10.1016/j.ijhydene.2008.12.080
Heyer, H., Stal, L., and Krumbein, W. E. (1989). Simultaneous heterolactic and acetate fermentation in the marine cyanobacterium Oscillatoria limosa incubated anaerobically in the dark. Arch. Microbiol. 151, 558–564. doi: 10.1007/BF00454875
Hoehler, T. M., Bebout, B. M., and Des Marais, D. J. (2001). The role of microbial mats in the production of reduced gases on the early Earth. Nature 412, 324–327. doi: 10.1038/35085554
Pubmed Abstract | Pubmed Full Text | CrossRef Full Text | Google Scholar
Houchins, J. P. (1984). The physiology and biochemistry of hydrogen metabolism in cyanobacteria. Biochim. Biophys. Acta 768, 227–255. doi: 10.1016/0304-4173(84)90018-1
Houchins, J. P., and Burris, R. H. (1981). Comparative characterization of two distinct hydrogenases from Anabaena sp. strain 7120. J. Bacteriol. 146, 215–221.
Javor, B. J., and Castenholz, R. W. (1981). Laminated microbial mats, Laguna Guerrero Negro, Mexico. Geomicrobiol. J. 2, 237–273. doi: 10.1080/01490458109377766
Juntarajumnong, W., Eaton-Rye, J. J., and Incharoensakdi, A. (2007). Two-component signal transduction in Synechocystis sp. PCC 6803 under phosphate limitation: role of acetyl phosphate. J. Biochem. Mol. Biol. 40, 708–714.
Kim, M. S., Baek, J. S., and Lee, J. K. (2006). Comparison of H2 accumulation by Rhodobacter sphaeroides KD131 and its uptake hydrogenase and PHB synthase deficient mutant. Int. J. Hydrogen Energy 31, 121–127. doi: 10.1016/j.ijhydene.2004.10.023
Kothari, A., Potrafka, R., and Garcia-Pichel, F. (2012). Diversity in hydrogen evolution from bidirectional hydrogenases in cyanobacteria from terrestrial, freshwater and marine intertidal environments. J. Biotechnol. 162, 105–114. doi: 10.1016/j.jbiotec.2012.04.017
Pubmed Abstract | Pubmed Full Text | CrossRef Full Text | Google Scholar
Kothari, A., Vaughn, M., and Garcia-Pichel, F. (2013). Comparative genomic analyses of the cyanobacterium, Lyngbya aestuarii BL J, a powerful hydrogen producer. Front. Microbiol. 4:363. doi: 10.3389/fmicb.2013.00363
Pubmed Abstract | Pubmed Full Text | CrossRef Full Text | Google Scholar
Krause, G., Lundström, J., Barea, J. L., De La Cuesta, C. P., and Holmgren, A. (1991). Mimicking the active site of protein disulfide-isomerase by substitution of proline 34 in Escherichia coli thioredoxin. J. Biol. Chem. 266, 9494–9500.
Lee, H. C., Kim, J. S., Jang, W., and Kim, S. Y. (2009). Thymidine production by overexpressing NAD+ kinase in an Escherichia coli recombinant strain. Biotechnol. Lett. 31, 1929–1936. doi: 10.1007/s10529-009-0097-z
Pubmed Abstract | Pubmed Full Text | CrossRef Full Text | Google Scholar
Lindberg, P., Schutz, K., Happe, T., and Lindblad, P. (2002). A hydrogen-producing, hydrogenase-free mutant strain of Nostoc punctiforme ATCC 29133. Int. J. Hydrogen Energy 27, 1291–1296. doi: 10.1016/S0360-3199(02)00121-0
Lindblad, P., Christensson, K., Lindberg, P., Fedorov, A., Pinto, F., and Tsygankov, A. (2002). Photoproduction of H2 by wild-type Anabaena PCC 7120 and a hydrogen uptake deficient mutant: from laboratory experiments to outdoor culture. Int. J. Hydrogen Energy 27, 1271–1281. doi: 10.1016/S0360-3199(02)00111-8
Masukawa, H., Mochimaru, M., and Sakurai, H. (2002). Disruption of the uptake hydrogenase gene, but not of the bidirectional hydrogenase gene, leads to enhanced photobiological hydrogen production by the nitrogen-fixing cyanobacterium Anabaena sp. PCC 7120. Appl. Microbiol. Biotechnol. 58, 618–624. doi: 10.1007/s00253-002-0934-7
Pubmed Abstract | Pubmed Full Text | CrossRef Full Text | Google Scholar
Moezelaar, R., Bijvank, S. M., and Stal, L. J. (1996). Fermentation and sulfur reduction in the mat-building cyanobacterium Microcoleus chthonoplastes. Appl. Environ. Microbiol. 62, 1752–1758.
Moezelaar, R., and Stal, L. J. (1994). Fermentation in the unicellular cyanobacterium Microcystis PCC 7806. Arch. Microbiol. 162, 63–69. doi: 10.1007/BF00264374
Nelson, D. L., Lehninger, A. L., and Cox, M. M. (2008). Lehninger Principles of Biochemistry. Worth, New York: Macmillan publishers.
Nold, S. C., and Ward, D. M. (1996). Photosynthate partitioning and fermentation in hot spring microbial mat communities. Appl. Environ. Microbiol. 62, 4598–4607.
Oren, A., and Shilo, M. (1979). Anaerobic heterotrophic dark metabolism in the cyanobacterium Oscillatoria limnetica: sulfur respiration and lactate fermentation. Arch. Microbiol. 122, 77–84. doi: 10.1007/BF00408049
Parameswaran, P., Torres, C., Lee, H. S., Krajmalnik-Brown, R., and Rittmann, B. E. (2009). Syntrophic interactions among anode respiring bacteria (ARB) and Non-ARB in a biofilm anode: electron balances. Biotechnol. Bioenergy 103, 513–523. doi: 10.1002/bit.22267
Pubmed Abstract | Pubmed Full Text | CrossRef Full Text | Google Scholar
Peterson, R. B., and Burris, R. H. (1978). Hydrogen metabolism in isolated heterocysts of Anabaena sp. PCC 7120. Arch. Microbiol. 116, 125–132.
Prince, R. C., and Kheshgi, H. S. (2005). The photobiological production of hydrogen: Potential efficiency and effectiveness as a renewable fuel. Crit. Rev. Microbiol. 31, 19–31. doi: 10.1080/10408410590912961
Pubmed Abstract | Pubmed Full Text | CrossRef Full Text | Google Scholar
Revsbech, N. P., Jorgensen, B. B., Blackburn, T. H., and Cohen, Y. (1983). Microelectrode studies of the photosynthesis and O2, H2S, and pH profiles of a microbial mat. Limnol. Oceanogr. 28, 1062–1074. doi: 10.4319/lo.1983.28.6.1062
Richardson, L. L., and Castenholz, R. W. (1987). Enhanced survival of the cyanobacterium Oscillatoria terebriformis in darkness under anaerobic conditions. Appl. Environ. Microbiol. 53, 2151–2158.
Rippka, R. (1988). Isolation and purification of cyanobacteria. Meth. Enzymol. 167, 3–27. doi: 10.1016/0076-6879(88)67004-2
Pubmed Abstract | Pubmed Full Text | CrossRef Full Text | Google Scholar
Rippka, R., Deruelles, J., Waterbury, J. B., Herdman, M., and Stanier, R. Y. (1979). Generic assignments, strain histories and properties of pure cultures of cyanobacteria. J. Gen. Microbiol. 111, 1–61. doi: 10.1099/00221287-111-1-1
Rothrock, M. J., and Garcia-Pichel, F. (2005). Microbial diversity of benthic mats along a tidal desiccation gradient. Environ. Microbiol. 7, 593–601. doi: 10.1111/j.1462-2920.2005.00728.x
Pubmed Abstract | Pubmed Full Text | CrossRef Full Text | Google Scholar
Schink, B. (1997). Energetics of syntrophic cooperation in methanogenic degradation. Microbiol. Mol. Biol. Rev. 61, 262–280.
Siedler, S., Bringer, S., and Bott, M. (2011). Increased NADPH availability in Escherichia coli: improvement of the product per glucose ratio in reductive whole-cell biotransformation. Appl. Microbiol. Biotechnol. 92, 929–937. doi: 10.1007/s00253-011-3374-4
Pubmed Abstract | Pubmed Full Text | CrossRef Full Text | Google Scholar
Skyring, G. W., Lynch, R. M., and Smith, G. D. (1989). “Quantitative relationships between carbon, hydrogen, and sulfur metabolism in cyanobacterial mats,” in Microbial Mats: Physiological Ecology of Benthic Microbial Communities, ed Y. Cohen, and E. Rosenberg (Washington, DC: American Society for Microbiology), 170–179.
Stal, L. J., and Moezelaar, R. (1997). Fermentation in cyanobacteria. FEMS Microbiol. Rev. 21, 179–211. doi: 10.1016/S0168-6445(97)00056-9
Stal, L., and Krumbein, W. (1985). Isolation and characterization of cyanobacteria from a marine microbial mat. Botanica Marina 28, 351–365. doi: 10.1515/botm.1985.28.8.351
Steunou, A. S., Bhaya, D., Bateson, M. M., Melendrez, M. C., Ward, D. M., Brecht, E., et al. (2006). In situ analysis of nitrogen fixation and metabolic switching in unicellular thermophilic cyanobacteria inhabiting hot spring microbial mats. Proc. Natl. Acad. Sci. U.S.A. 103, 2398–2403. doi: 10.1073/pnas.0507513103
Pubmed Abstract | Pubmed Full Text | CrossRef Full Text | Google Scholar
Thauer, R. (1977). “Limitation of microbial hydrogen formation via fermentation,” in Microbial Energy Conversion, ed E. K. J. Goltze (Elmsford, NY: Pergamon Press, Inc.), 201–204.
Troshina, O., Serebryakova, L., Sheremetieva, M., and Lindblad, P. (2002). Production of H2 by the unicellular cyanobacterium Gloeocapsa alpicola CALU 743 during fermentation. Int. J. Hydrogen Energy 27, 1283–1289. doi: 10.1016/S0360-3199(02)00103-9
Pubmed Abstract | Pubmed Full Text | CrossRef Full Text | Google Scholar
Van der Oost, J., Bulthuis, B. A., Feitz, S., Krab, K., and Kraayenhof, R. (1989). Fermentation metabolism of the unicellular cyanobacterium Cyanothece PCC 7822. Arch. Microbiol. 152, 415–419. doi: 10.1007/BF00446921
Walker, M. C., Pueyo, J., Gómez-Moreno, C., and Tollin, G. (1990). Comparison of the kinetics of reduction and intramolecular electron transfer in electrostatic and covalent complexes of ferredoxin-NADP+ reductase and flavodoxin from Anabaena PCC 7119. Arch. Biochem. Biophys. 281, 76–83. doi: 10.1016/0003-9861(90)90415-U
Pubmed Abstract | Pubmed Full Text | CrossRef Full Text | Google Scholar
Weaver, P. F., Lien, S., and Seibert, M. (1980). Photobiological production of hydrogen. Solar Energy 24, 3–45. doi: 10.1016/0038-092X(80)90018-3
Yoshino, F., Ikeda, H., Masukawa, H., and Sakurai, H. (2007). High photobiological hydrogen production activity of a Nostoc sp. PCC 7422 uptake hydrogenase-deficient mutant with high nitrogenase activity. Mar. Biotechnol. 9, 101–112. doi: 10.1007/s10126-006-6035-3
Pubmed Abstract | Pubmed Full Text | CrossRef Full Text | Google Scholar
Keywords: bidirectional hydrogenase, cyanobacteria, Lyngbya aestuarii, Microcoleus chthonoplastes, Synechocystis, hydrogen, fermentation
Citation: Kothari A, Parameswaran P and Garcia-Pichel F (2014) Powerful fermentative hydrogen evolution of photosynthate in the cyanobacterium Lyngbya aestuarii BL J mediated by a bidirectional hydrogenase. Front. Microbiol. 5:680. doi: 10.3389/fmicb.2014.00680
Received: 30 September 2014; Accepted: 20 November 2014;
Published online: 10 December 2014.
Edited by:
Biswarup Mukhopadhyay, Virginia Tech, USAReviewed by:
Peter Lindblad, Uppsala University, SwedenTeresa Thiel, University of Missouri-St. Louis, USA
Copyright © 2014 Kothari, Parameswaran and Garcia-Pichel. This is an open-access article distributed under the terms of the Creative Commons Attribution License (CC BY). The use, distribution or reproduction in other forums is permitted, provided the original author(s) or licensor are credited and that the original publication in this journal is cited, in accordance with accepted academic practice. No use, distribution or reproduction is permitted which does not comply with these terms.
*Correspondence: Ferran Garcia-Pichel, School of Life Sciences, Arizona State University, Life Sciences E Wing, Room 423, Tempe, AZ 85287-4501, USA e-mail:ZmVycmFuQGFzdS5lZHU=