- Marine Biology Research Division, Center for Marine Biotechnology and Biomedicine, Scripps Institution of Oceanography, University of California, San Diego, La Jolla, CA, USA
Much of microbial life on Earth grows and reproduces under the elevated hydrostatic pressure conditions that exist in deep-ocean and deep-subsurface environments. In this study adaptive laboratory evolution (ALE) experiments were conducted to investigate the possible modification of the piezosensitive Escherichia coli for improved growth at high pressure. After approximately 500 generations of selection, a strain was isolated that acquired the ability to grow at pressure non-permissive for the parental strain. Remarkably, this strain displayed growth properties and changes in the proportion and regulation of unsaturated fatty acids that indicated the acquisition of multiple piezotolerant properties. These changes developed concomitantly with a change in the gene encoding the acyl carrier protein, which is required for fatty acid synthesis.
Introduction
Elevated hydrostatic pressure promotes reduced system volumes and volume changes of activation in chemical equilibria and rates of reactions, respectively (Meersman and McMillan, 2014). Microorganisms growing preferentially at elevated hydrostatic pressures exist in large portions of Earth's biosphere including deep-sea and deep-subsurface locations (Meersman et al., 2013). Despite the fact that the growth attributes of these piezophiles appear to require relatively little evolutionary change (Prieur et al., 2009), increased hydrostatic pressure exerts pervasive effects on many aspects of cellular function and the adaptations required remain incompletely defined (Lauro et al., 2008). Arguably the best understood adaptation is the need for bacterial piezophiles to maintain a sufficient proportion of unsaturated fatty acids in their membranes as a means of tuning phase, viscosity, and/or ion permeability (Bartlett, 2002; Kawamoto et al., 2011).
Escherichia coli is the most studied piezosensitive microorganism with the ability to grow to pressures of up to 50 MPa (Zobell and Cobet, 1963). It has been successfully used as a model organism to investigate the effects of increasing pressure on cell processes and structures. E. coli belongs to the class of Gammaproteobacteria which includes a large proportion of the characterized piezophiles, while as a foodborne pathogen has been subjected to high pressure pasteurization processes potentially promoting the emergence of piezoresistant strains (Vanlint et al., 2012). High pressure affects many cellular processes in E. coli, including replication, transcription, and translation. Previous studies have demonstrated DNA synthesis inhibition at 50 MPa while RNA synthesis was abolished completely at 77 MPa (Yayanos and Pollard, 1969; Welch et al., 1993). Interestingly, a moderate pressure of 30 MPa was sufficient to suppress gene transcription (Sato et al., 1996). Protein synthesis was completely inhibited at 68 MPa; aminoacyl-tRNA binding, ribosome translocation, and ribosome stability have all been implicated as the basis of high pressure impacts on translation (Schwarz and Landau, 1972; Groß and Jaenicke, 1990; Alpas et al., 2003). Cell division was affected at pressures ranging from 20 to 50 MPa resulting in elongation of the cells and a filamentous morphology since cell division is affected prior to cell growth and accumulation of biomass (Zobell and Cobet, 1963). Pressure of 10 MPa and higher affect flagellation by disrupting filament polymerization and flagellum rotation (Meganathan and Marquis, 1973). Other structural changes under high pressure include a compact nucleoid structure (Welch et al., 1993).
High pressure growth of E. coli at 30 or 50 MPa induces a cascade of stress responses with the concomitant regulation of a series of genes; such cascade networks include heat and cold stress responses (Welch et al., 1993; Welch and Bartlett, 1998; Aertsen et al., 2004a; Ishii et al., 2005). Interestingly, heat shock pre-treatment improved the pressure resistance of E. coli in subsequent high pressure treatment, suggesting that heat shock proteins might play a protective role (Aertsen et al., 2004a). Finally, sub-lethal pressure treatments (100–500 MPa) increased expression of stress sigma factors and genes involved in spontaneous mutations and triggered the SOS and oxidative stress responses (Aertsen et al., 2004b, 2005; Malone et al., 2006). Recently, a genome wide screening approach confirmed the pleiotropic effects of pressure on E. coli identifying a series of genes required for growth at high pressure, including genes involved in DNA replication, cell division, cytoskeleton, and cell envelope physiology (Black et al., 2013).
In this report we investigate the feasibility of employing adaptive laboratory evolution (ALE) techniques to drive the phenotype of a model mesophile in the direction of piezophily. ALE is a powerful tool that relies on the Darwinian principles of mutation and selection (Pourmir and Johannes, 2012). In some cases the selections involve a single selective step (Vanlint et al., 2011), in others a few hundred to a few thousand generations (Lee et al., 2011), and in the extreme case of the long-term studies of Lenski and colleagues they may proceed for more than 50,000 generations (Barrick et al., 2009). Examples of ALE experiments selecting for more extremophilic characteristics in mesophiles include increased salt tolerance (Zhou et al., 2013), increased radiation resistance (Harris et al., 2009), the conversion of a mesophile into a facultative thermophile (Blaby et al., 2012) and the selection for survival to exposures of more than 2 gigapascals of pressure (Hauben et al., 1997). It should be noted that resistance to ultra-high pressure does not confer improved growth at elevated pressures (Hauben et al., 1997; Lauro et al., 2008). In this study, following 505 generations of selection we have isolated a strain with the ability to grow at 62 MPa and alter membrane fatty acid composition in response to pressure. A mutation has been identified in a gene involved in lipid metabolism that could contribute in the improved piezotolerance of the newly isolated strain.
Materials and Methods
Growth Conditions
E. coli K-12 MG1655 was grown in triplicate lineages (A, B, and C) under fermentative conditions at 37°C using Luria Bertani (LB) medium supplemented with glucose (11 mM) and HEPES buffer (100 mM, pH 7.5) in 5 ml polyethylene transfer pipette bulbs kept within stainless steel pressure vessels (Figure 1A). Initial incubations were performed at 41 megapascal (MPa) and the growth was measured after 48 h of incubation. The cultures were allowed to reach a minimum optical density (OD) at 600 nm of 0.45 (corresponding to a cell density of 4.5 × 108 cells ml−1), before the cultures were diluted 1000-fold in fresh medium and reincubated at high pressure. Each triplicate subculture was assigned a new lineage number (i.e., L2A, 2B, 2C). The pressure was gradually increased in increments of 7 MPa when cell yield exceeded 4.5 × 108 cells ml−1. Single clonal isolates from each culture were obtained by streaking LB agar plates and incubating for 48 h at 37°C.
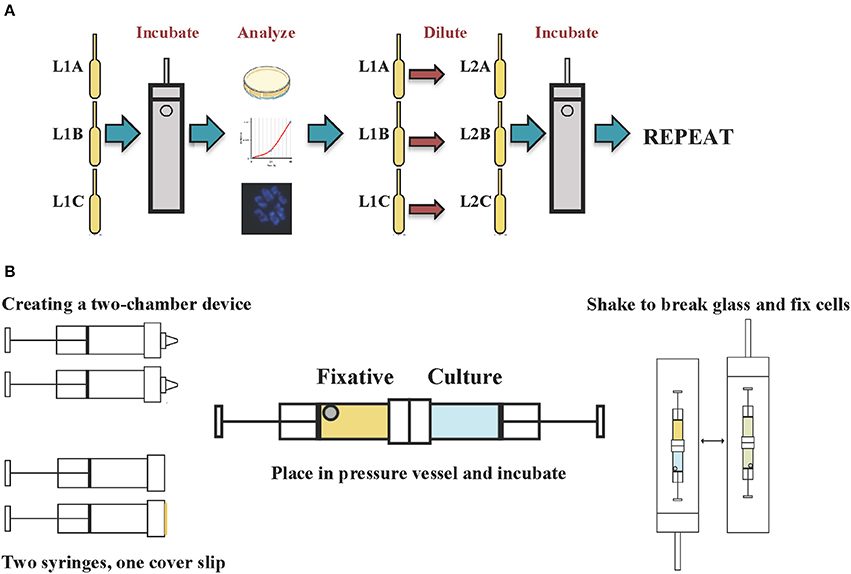
Figure 1. Schematic diagram of the (A) adaptive laboratory evolution experimental procedure and (B) cell fixation under in situ pressure conditions.
Microscopy and Image Analysis
Cells were fixed with 2% glutaraldehyde in 0.1 M sodium cacodylate buffer (pH 7.4) under in situ pressure conditions (Figure 1B) (Chastain and Yayanos, 1991). Two Sarstedt syringes were joined together using silicone adhesive creating a device with two chambers separated by a circular glass cover slip. The first chamber contained the culture while the second chamber contained the fixative solution and a stainless steel ball. The conjoined syringe was placed in a pressure vessel and incubated at 37°C, at the desired pressure. To fix the cells the pressure vessel was shaken vigorously to cause the steel ball to break the glass cover slip and mix the fixative with the culture at in situ pressure conditions.
For epifluorescence microscopy the fixed cells were immobilized onto a 0.2 μm polycarbonate membrane (EMD Millipore) and stained using 4′, 6′ -diamidino-2-phenylindole (DAPI) nucleic acid stain (Vector Laboratories, Inc). The stained samples were viewed at 1000 fold magnification on an Olympus BX51 fluorescence microscope (Olympus). TEM samples were post fixed with 1% osmium and 2% of uranyl acetate and embedded in Ducurpan at 60°C for 36 h. Ultra-thin sections (60 nm) were cut with a diamond knife on Leica Ultracut UCT Microtome and post stained with uranyl acetate and lead. Images were captured on FEI Tecnai spirit at 80 KV.
Fatty Acid Analysis
Fatty acid analysis was performed by MIDI Labs (Newark, DE) on frozen cell pellets harvested during the early logarithmic phase of growth. Saponification, methylation, extraction, and base wash were performed before the fatty acid methyl esters were analyzed on an Agilent/HP 6890 gas chromatograph. Using a pattern recognition software, Sherlock MIS, the fatty acid composition of each sample was compared to a stored database. The software was able to identify each component of the analyzed sample, producing a composition report including the relative amount (%) of the named fatty acids.
DNA Purification and Genetic Analysis
Cells growing at exponential phase were centrifuged at 14,000 × g for 2 min and the cell pellets were used for DNA purification. The Wizard Genomic DNA purification kit (Promega) was used according to the manufacturer's instructions. The purified DNA was used as template for the amplification of the acpP, fabA, fabB, and fabF genes (see Table S1 for primer sequences and cycling parameters). Each PCR reaction contained 45 μl of Platinum PCR Supermix (Life Technologies), 2 μl of each primer (10 μM) and 1 μl of template DNA. The PCR products were purified using the QIAquick PCR Purification kit (Qiagen) and submitted for direct sequencing by Retrogen Inc. (San Diego, CA) using the respective primers in both directions.
Results
E. coli Growth at High Pressure
The ALE of E. coli for growth at high pressure was initiated by incubating triplicate cultures at 41 MPa. E. coli was grown at 41 MPa for 103 generations prior to increasing the pressure to 48 MPa for 247 generations, followed by incubation at 55 MPa for 130 generations, and finally further increased to 62 MPa for 25 generations (Figure 2). The total time required was 126 days and the total number of generations was 505. Improved high pressure growth of isolated strains was not evident until after selection for growth at the last pressure tested, 62 MPa, and this was only evident in cells derived from the lineage A population. Lineage B and C did not respond as well as lineage A to the increasing pressure, failing to produce any cells capable of growth above 55 MPa. The last subculture collected was L62A, and from this population a single clonal isolate, designated strain AN62, was obtained from an LB agar plate.
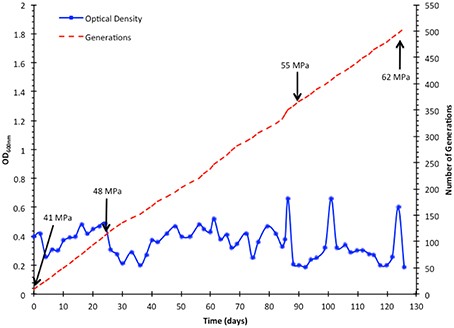
Figure 2. Directed evolution of E. coli K-12 MG1655 to growth at high pressure. Cultures were grown under fermentative conditions to a minimum OD of 0.45 before they were diluted and incubated at increasing pressure at increments of 7 MPa. The OD values presented were recorded after 48 h of incubation for each subculture for lineage A.
At atmospheric pressure the growth of clonal isolate AN62 was slower than the parental strain in liquid medium (Figure 3). The parental strain had a doubling time of 17 min while AN62 strain had a doubling time of 44 min when grown at 0.1 MPa. In contrast at 60 MPa the parental strain was unable to grow, while AN62 grew after an extended lag phase of ~20 h with a doubling time of 70 min (Figure 3). The same was true for colonial growth on agar plates; AN62 strain formed smaller colonies following a 48-h incubation compared to the parental strain (Figure 4).
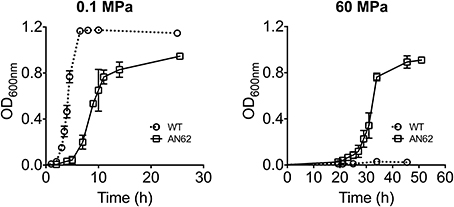
Figure 3. Growth curves of E. coli K-12 MG1655 parental and pressure adapted AN62 strain at atmospheric (0.1 MPa) and high (60 MPa) pressure. Cultures were grown micro-aerobically in LB supplemented with glucose and HEPES buffer at 37°C. WT, parental strain.
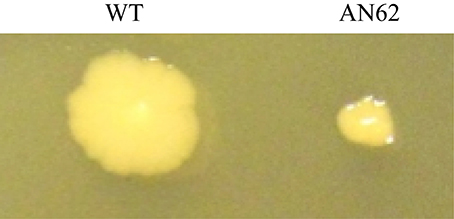
Figure 4. Colony morphology of E. coli parental and AN62 strain. Cells were plated on LB plates and incubated for 48 h at 37°C at 0.1 MPa. WT, parental strain.
Cell Morphology at High Pressure
Epifluorescence microscopy and transmission electron microscopy (TEM) was used to determine whether any morphological changes accompanied the improved high pressure growth phenotype. The DAPI-stained parental strain (2 ± 0.5 μm) and evolved strain AN62 (2 ± 0.4 μm) exhibited the typical rod shaped morphology of E. coli at atmospheric pressure (Figure 5). At 60 MPa the few parental strain cells that were present maintained a rod shaped morphology, while AN62 cells primarily existed as long filaments with an average size of 6.6 ± 2 μm (Figure 5). The parental strain cells appeared longer at 60 MPa (2.5 ± 1 μm, p = 0.037) compared to atmospheric pressure.
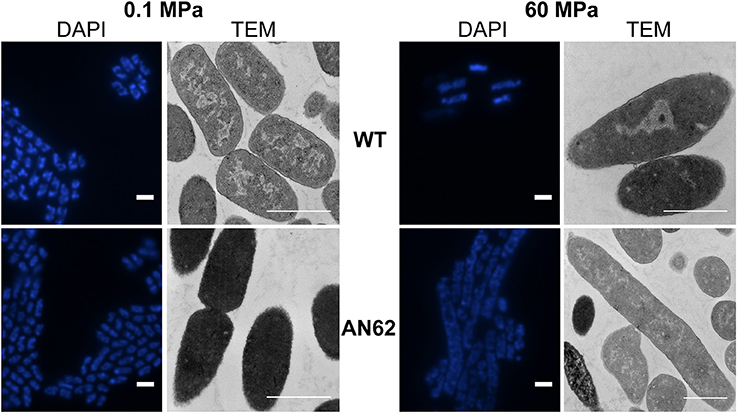
Figure 5. Epifluorescence and TEM microphotographs of in situ fixed parental and AN62 strain cells at atmospheric (0.1 MPa) and high (60 MPa) pressure. Cultures were grown micro-aerobically in LB supplemented with glucose and HEPES buffer at 37°C. WT, parental strain. Scale bar indicates 1 μm.
TEM confirmed the overall morphology indicated by the epifluorescence observations. Interestingly AN62 cells appeared darker at atmospheric pressure compared to the parental strain, suggesting the presence of a dense intracellular matrix (Figure 5). At 60 MPa the majority of the AN62 cells appeared as long filaments (7.2 ± 2 μm). Growth at high pressure caused a change in the distribution of the nucleoid in the parental strain as it appeared condensed in the mid-cell region, while the internal structure of AN62 at high pressure resembled that of the parental strain at atmospheric pressure.
Membrane Fatty Acid Composition
Because a hallmark feature of all piezophilic bacteria is a high ratio of unsaturated to saturated membrane fatty acids, the fatty acid profiles of the parental and evolved strain were also compared. Under atmospheric pressure growth conditions the ratio of unsaturated to saturated fatty acids was similar for both the parental strain and AN62, although the former produced more palmitoleic acid (16:1 ω 7c) and the latter more cis-vaccenic acid (18:1 ω 7c). Remarkably, at 60 MPa evolved strain AN62 increased its production of cis-vaccenic acid by about 50%, up to more than 30% of the total fatty acid abundance, largely at the expense of the saturated fatty acid palmitic acid (16:0) (Table 1). There was insufficient biomass production at 60 MPa for the parental strain membrane fatty acid analysis.
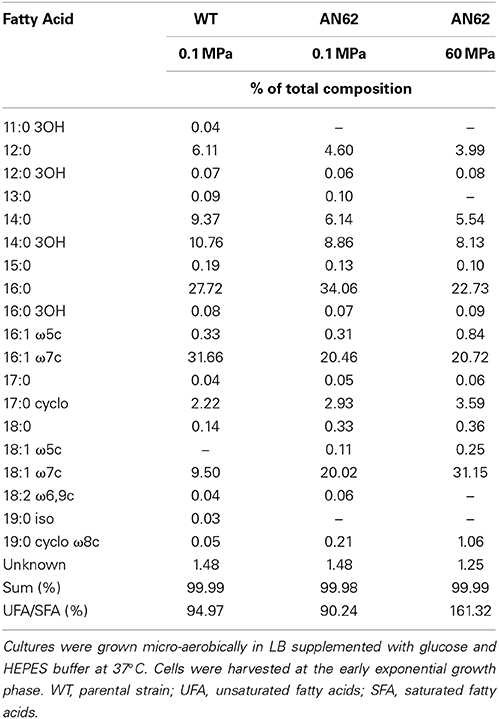
Table 1. Fatty acid composition of E. coli K-12 MG1655 parental and AN62 strain at atmospheric (0.1 MPa) and high (60 MPa) pressure.
Genetic Analysis
Based on the altered unsaturated fatty acid species abundances produced in AN62 selected genes involved in fatty acid biosynthesis were sequenced. These genes included acpP, encoding acyl carrier protein ACP, fabA, encoding beta-hydroxydecanoyl thioester dehydrase, fabB, encoding beta-ketoacyl-ACP synthase I (KAS I), and fabF, encoding beta-ketoacyl-ACP synthase II (KAS II). Only one of these genes was discovered to possess a sequence difference with its homolog in the parental strain. The AN62 acpP gene contains a transversion mutation (T to G) at nucleotide position 131 resulting in a valine to glycine (V43G) amino acid change (Figure 6). The evolutionary history of this mutation was followed by assessing its presence or absence in strains derived from past lineages. Based on these analyses it was found that this mutation first appeared in lineage 60A, the same lineage found to possess the first cells adapted for improved high pressure growth (data not shown).
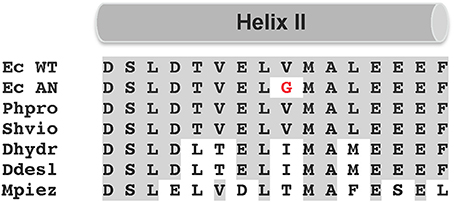
Figure 6. Multiple sequence alignment of helix II region of various ACP. Identical residues are shaded gray, while red is used to highlight the glycine in AN62 strain. Ec WT, E. coli parental strain; Ec AN, E. coli AN62 strain; Phpro, Photobacterium profundum SS9; Shvio, Shewanella violacea DSS12; Dhydr, Desulfovibrio hydrothermalis AM13; Ddesl, Desulfovibrio desulfuricans 27774; Mpiez, Marinitoga piezophila KA3.
Discussion
In this study, we used ALE to isolate a novel E. coli strain with the ability to grow at pressures that impede parental strain from growing. No E. coli strain has ever been documented to grow at pressures exceeding 50 MPa (Ishii et al., 2004). Although AN62 did not exhibit piezophilic growth, which would necessitate improved growth at high pressure compared to atmospheric pressure, its growth characteristics were altered at both ends of the pressure spectrum in a direction toward piezophily. This is the first time any microorganism has had its growth phenotype changed at both low and high pressure, although improved growth at high pressure has been noted in two other studies (Marquis and Bender, 1980; Abe and Horikoshi, 2000).
AN62 strain grew at 60 MPa following an extended lag phase with a doubling time of 70 min (Figure 3). The extended lag phase observed for AN62 at 60 MPa could be attributed to the physiological and molecular changes that the cells have to adjust before exponential growth can proceed at high pressure. Thus, even though AN62 strain has evolved to grow at 60 MPa the changes required to switch from growth at atmospheric pressure to growth at high pressure suggest that the cells required a substantial amount of “fine-tuning,” perhaps reflecting changes in transcription, translation, and/or the activity of key enzymes. Fatty acid biosynthesis is among the processes that are up-regulated during lag phase (Rolfe et al., 2012) and the response of the fatty acid machinery to changes in pressure could have contributed to the observed extended lag phase. Finally, the relatively slow growth rate of AN62 under all pressure conditions indicates that this mutant did not evolve improved growth efficiency but rather improved high pressure growth capacity. The basis of its growth rate reduction, is unknown, but ATP supply and demand is strongly influenced by pressure in piezosensitive bacteria (Marquis, 1982). The significantly slower growth rate of AN62 at 60 MPa could also reflect the constraints imposed on a larger cell volume due to inhibition of cell division at high pressure (Klumpp et al., 2009).
At 60 MPa, AN62 exhibited filamentous morphology in contrast to the parental strain which exhibited only a slightly elongated cell shape. It has previously been reported that E. coli assumes a typical filamentous morphology at the non-permissive pressure of 40 MPa (Ishii et al., 2004). Filamentation is a well-documented response of mesophilic bacteria to elevated pressures sufficiently high to inhibit cell division, but not so great as to prevent biomass accumulation (Jannasch, 1987; Yayanos and DeLong, 1987; Kawarai et al., 2004). The basis of this effect may stem from direct effects on the tubulin-like cell division protein FtsZ (Ishii et al., 2004) or through the induction of a DNA damage stress response (Aertsen and Michiels, 2005). When pressures high enough to prevent growth were applied to AN62 (75 MPa), no change in morphology occurred. Thus, along with its growth properties the filamentation response of AN62 was shifted to higher pressures.
Growth at suboptimal pressures also causes substantial changes to the morphology of known piezophiles. Marinitoga piezophila KA3 cells are short rods (1–1.5 μm) when grown at the optimal pressure of 40 MPa (Alain et al., 2002). However, when the cells are grown at 10 MPa or atmospheric pressure they appear elongated (Alain et al., 2002). Similarly, Profundimonas piezophila cells are rods (4–5 μm) when grown at 60 MPa (pressure optimum 50 MPa) and their morphology changes to 0.8–1.0 μm cocci when grown at pressures lower than 20 MPa (Cao et al., 2014). Interestingly, following nine transfers at atmospheric pressure M. piezophila KA3 exhibited the short rod morphotype. These observations highlight the effect of pressure on cell morphology and indicate that adaptation to higher or lower pressures is accompanied by cell size changes.
Strain AN62 produced on overall more cis-vaccenic acid, while its relative amount increased further at 60 MPa. These results indicate that not only had the mutant strain acquired the ability to produce more unsaturated fatty acids, but also it had gained the ability to regulate their abundance in response to the pressure applied. Both of these phenotypes are shared with piezophilic bacteria (Allen and Bartlett, 2000). The molecular mechanisms underlying such a response to increasing pressure remains largely unexplored.
Previous studies highlighted the importance of fatty acid synthesis genes in temperature and pressure response modulation (Garwin and Cronan, 1980; Garwin et al., 1980; Allen and Bartlett, 2000). ACP is a conserved protein that interacts with several other proteins including some with known role in lipid metabolism (Worsham et al., 2003). ACP carries fatty acid synthesis intermediates as thiosters (Rock and Jackowski, 2002). The V43G mutation is located in the helix II region; a site of interaction with several proteins involved in fatty acid synthesis (Jackowski and Rock, 1987). There is a high degree of identity among type II ACP homologs, including position V43 (Jackowski and Rock, 1987). This is also true for most but not all piezophiles. For example, the well-studied piezophiles Photobacterium profundum and Shewanella violacea possess V43, whereas the piezophiles Desulfovibrio hydrothermalis and M. piezophila have isoleucine and threonine at position V43, respectively. The presence of V43 in well studied piezophiles is indicating that modification of this residue is not a universal feature of high pressure adaptation.
Previous studies in E. coli have established that a V43I substitution affects the ACP equilibrium to a more compact, tightly-folded conformation by stabilizing the hydrophobic core of the protein and decreasing the molecular radius (Keating and Cronan, 1996; Roujeinikova et al., 2002). The V43I substitution is hypothesized to also increase the efficiency of unsaturated fatty acid synthesis (Roujeinikova et al., 2002).
We hypothesize that the mutation uncovered in acpP is linked to KAS II activity, both because of the associated increased production of cis-vaccenic acid synthesis observed in AN62 (Garwin et al., 1980; Jackowski and Rock, 1987; Allen and Bartlett, 2000) and because of prior evidence linking ACP structure with KAS II activity (Jackowski and Rock, 1987). KAS II is responsible for increased cis-vaccenic acid production in response to lower growth temperature (Garwin et al., 1980). It catalyzes the elongation of palmitoleic (16:1) to cis-vaccenic acid (18:1). Interestingly, thermal regulation of cis-vaccenic acid synthesis is not controlled at the transcriptional or translation level but is depended on KAS II activity (Garwin and Cronan, 1980). KAS II mutants with impaired activity possessed ACP proteins with the V43I mutation suggesting that V43 residue has an important role in KAS II/ACP interaction with effects on fatty acid synthesis (Jackowski and Rock, 1987).
It is likely that the observed genetic change is necessary but insufficient for piezoadaptation. Additional studies will be needed to identify and confirm all mutations necessary and sufficient for the high pressure phenotypes of AN62. It will also be of interest to determine how much further ALE experiments can drive E. coli and other non-piezophiles along the gradient of piezophily. In the case of obligate piezophiles ALE experiments could be used to determine the adaptations required for the evolution of growth and survival at decreased pressures.
ALE could improve our understanding of the evolutionary steps required for the adaptation of life at extremes of pressure. The environmentally relevant exposure of piezosensitive surface microbes to increases in pressure could proceed by a variety of different mechanisms including in association with metazoans undergoing extensive vertical migrations, or in association with large carrion or smaller aggregates of particulate organic carbon. The vertical travel speeds of particle-associated microbes have been estimated to range from 10 to 150 m d−1 (McDonnell et al., 2010). At increasing depths travel speed increases by a factor of 10–60%, while pressure increases by 1 MPa every 100 m (Berelson, 2001). Sufficient growth could occur during these transits to enable the acquisition of the requisite number and types of mutations needed for selection of high pressure growth mutants of increased fitness.
Our results demonstrate that it is possible to drive the evolutionary trajectory of a piezosensitive bacterium along the pressure continuum toward piezophily, and to archive the evolutionary history of this process for the subsequent examination of the associated mutational events. ALE experiments performed in this way provide a new approach to characterize the genetic underpinnings enabling microbial life to flourish under the extreme physical constraint of high pressure (low volume change).
Conflict of Interest Statement
The authors declare that the research was conducted in the absence of any commercial or financial relationships that could be construed as a potential conflict of interest.
Acknowledgment
This work was supported by grants from the National Science Foundation (EF-0801793 and EF-0827051) and the Sloan Foundation Deep Carbon Observatory to Douglas Bartlett.
Supplementary Material
The Supplementary Material for this article can be found online at: http://www.frontiersin.org/journal/10.3389/fmicb.2014.00749/abstract
References
Abe, F., and Horikoshi, K. (2000). Tryptophan permease gene TAT2 confers high-pressure growth in Saccharomyces cerevisiae. Mol. Cell. Biol. 20, 8093–8102. doi: 10.1128/MCB.20.21.8093-8102.2000
Pubmed Abstract | Pubmed Full Text | CrossRef Full Text | Google Scholar
Aertsen, A., De Spiegeleer, P., Vanoirbeek, K., Lavilla, M., and Michiels, C. W. (2005). Induction of oxidative stress by high hydrostatic pressure in Escherichia coli. Appl. Environ. Microbiol. 71, 2226–2231. doi: 10.1128/AEM.71.5.2226-2231.2005
Pubmed Abstract | Pubmed Full Text | CrossRef Full Text | Google Scholar
Aertsen, A., Houdt, R. V., Vanoirbeek, K., and Michiels, C. W. (2004b). An SOS response induced by high pressure in Escherichia coli. J. Bacteriol. 186, 6133–6141. doi: 10.1128/JB.186.18.6133-6141.2004
Pubmed Abstract | Pubmed Full Text | CrossRef Full Text | Google Scholar
Aertsen, A., and Michiels, C. W. (2005). Mrr instigates the SOS response after high pressure stress in Escherichia coli. Mol. Microbiol. 58, 1381–1391. doi: 10.1111/j.1365-2958.2005.04903.x
Pubmed Abstract | Pubmed Full Text | CrossRef Full Text | Google Scholar
Aertsen, A., Vanoirbeek, K., De Spiegeleer, P., Sermon, J., Hauben, K., Farewell, A., et al. (2004a). Heat shock protein-mediated resistance to high hydrostatic pressure in Escherichia coli. Appl. Environ. Microbiol. 70, 2660–2666. doi: 10.1128/AEM.70.5.2660-2666.2004
Pubmed Abstract | Pubmed Full Text | CrossRef Full Text | Google Scholar
Alain, K., Marteinsson, V. T., Miroshnichenko, M. L., Bonch-Osmolovskaya, E. A., Prieur, D., and Birrien, J. L. (2002). Marinitoga piezophila sp. nov., a rod-shaped, thermo-piezophilic bacterium isolated under high hydrostatic pressure from a deep-sea hydrothermal vent. Int. J. Syst. Evol. Microbiol. 52, 1331–1339. doi: 10.1099/ijs.0.02068-0
Pubmed Abstract | Pubmed Full Text | CrossRef Full Text | Google Scholar
Allen, E. E., and Bartlett, D. H. (2000). FabF is required for piezoregulation of cis-vaccenic acid levels and piezophilic growth of the deep-sea bacterium Photobacterium profundum strain SS9. J. Bacteriol. 182, 1264–1271. doi: 10.1128/JB.182.5.1264-1271.2000
Pubmed Abstract | Pubmed Full Text | CrossRef Full Text | Google Scholar
Alpas, H., Lee, J., Bozoglu, F., and Kaletunc, G. (2003). Evaluation of high hydrostatic pressure sensitivity of Staphylococcus aureus and Escherichia coli 157:H7 by differential scanning calorimetry. Int. J. Food Microbiol. 87, 229–237. doi: 10.1016/S0168-1605(03)00066-7
Pubmed Abstract | Pubmed Full Text | CrossRef Full Text | Google Scholar
Barrick, J. E., Yu, D. S., Yoon, S. H., Jeong, H., Oh, T. K., Schneider, D., et al. (2009). Genome evolution and adaptation in a long-term experiment with Escherichia coli. Nature 461, 1243–1247. doi: 10.1038/nature08480
Pubmed Abstract | Pubmed Full Text | CrossRef Full Text | Google Scholar
Bartlett, D. H. (2002). Pressure effects on in vivo processes. Biochim. Biophys. Acta. 1595, 367–381. doi: 10.1016/S0167-4838(01)00357-0
Pubmed Abstract | Pubmed Full Text | CrossRef Full Text | Google Scholar
Berelson, W. M. (2001). Particle settling rates increase with depth in the ocean. Deep Sea Res. Part II Top. Stud. Oceanogr. 49, 237–251. doi: 10.1016/S0967-0645(01)00102-3
Blaby, I. K., Lyons, B. J., Wroclawska-Hughes, E., Phillips, G. C., Pyle, T. P., Chamberlin, S. G., et al. (2012). Experimental evolution of a facultative thermophile from a mesophilic ancestor. Appl. Environ. Microbiol. 78, 144–155. doi: 10.1128/AEM.05773-11
Pubmed Abstract | Pubmed Full Text | CrossRef Full Text | Google Scholar
Black, S. L., Dawson, A., Ward, F. B., and Allen, R. J. (2013). Genes required for growth at high hydrostatic pressure in Escherichia coli K-12 identified by genome-wide screening. PLoS ONE 8:e73995. doi: 10.1371/journal.pone.0073995
Pubmed Abstract | Pubmed Full Text | CrossRef Full Text | Google Scholar
Cao, Y., Chastain, R. A., Eloe, E. A., Nogi, Y., Kato, C., and Bartlett, D. H. (2014). Novel psychropiezophilic Oceanospirillales species Profundimonas piezophila gen. nov., sp. nov., isolated from the deep-sea environment of the Puerto Rico Trench. Appl. Environ. Microbiol. 80, 54–60. doi: 10.1128/AEM.02288-13
Pubmed Abstract | Pubmed Full Text | CrossRef Full Text | Google Scholar
Chastain, R. A., and Yayanos, A. A. (1991). Ultrastructural changes in an obligately barophilic marine bacterium after decompression. Appl. Environ. Microbiol. 57, 1489–1497.
Garwin, J. L., and Cronan, J. E. (1980). Thermal modulation of fatty acid synthesis in Escherichia coli does not involve de novo enzyme synthesis. J. Bacteriol. 141, 1457–1459.
Garwin, J. L., Klages, A. L., and Cronan, J. E. (1980). Beta-ketoacyl-acyl carrier protein synthase II of Escherichia coli. Evidence for function in the thermal regulation of fatty acid synthesis. J. Biol. Chem. 255, 3263–3265.
Groß, M., and Jaenicke, R. (1990). Pressure-induced dissociation of tight couple ribosomes. FEBS Lett. 267, 239–241. doi: 10.1016/0014-5793(90)80934-B
Pubmed Abstract | Pubmed Full Text | CrossRef Full Text | Google Scholar
Harris, D. R., Pollock, S. V., Wood, E. A., Goiffon, R. J., Klingele, A. J., Cabot, E. L., et al. (2009). Directed evolution of ionizing radiation resistance in Escherichia coli. J. Bacteriol. 191, 5240–5252. doi: 10.1128/JB.00502-09
Pubmed Abstract | Pubmed Full Text | CrossRef Full Text | Google Scholar
Hauben, K. J., Bartlett, D. H., Soontjens, C. C., Cornelis, K., Wuytack, E. Y., and Michiels, C. W. (1997). Escherichia coli mutants resistant to inactivation by high hydrostatic pressure. Appl. Environ. Microbiol. 63, 945–950.
Ishii, A., Oshima, T., Sato, T., Nakasone, K., Mori, H., and Kato, C. (2005). Analysis of hydrostatic pressure effects on transcription in Escherichia coli by DNA microarray procedure. Extremophiles 9, 65–73. doi: 10.1007/s00792-004-0414-3
Pubmed Abstract | Pubmed Full Text | CrossRef Full Text | Google Scholar
Ishii, A., Sato, T., Wachi, M., Nagai, K., and Kato, C. (2004). Effects of high hydrostatic pressure on bacterial cytoskeleton FtsZ polymers in vivo and in vitro. Microbiology 150, 1965–1972. doi: 10.1099/mic.0.26962-0
Pubmed Abstract | Pubmed Full Text | CrossRef Full Text | Google Scholar
Jackowski, S., and Rock, C. O. (1987). Altered molecular form of acyl carrier protein associated with beta-ketoacyl-acyl carrier protein synthase II (fabF) mutants. J. Bacteriol. 169, 1469–1473.
Jannasch, H. W. (1987). “Effects of hydrostatic pressure on growth of marine bacteria,” in Current Perspectives in High Pressure Biology, eds H. W. Jannasch, R. E. Marquis, and A. M. Zimmerman (Toronto, ON: Academic Press), 1–15.
Kawamoto, J., Sato, T., Nakasone, K., Kato, C., Mihara, H., Esaki, N., et al. (2011). Favourable effects of eicosapentaenoic acid on the late step of the cell division in a piezophilic bacterium, Shewanella violacea DSS12, at high- hydrostatic pressures. Environ. Microbiol. 13, 2293–2298. doi: 10.1111/j.1462-2920.2011.02487.x
Pubmed Abstract | Pubmed Full Text | CrossRef Full Text | Google Scholar
Kawarai, T., Wachi, M., Ogino, H., Furukawa, S., Suzuki, K., Ogihara, H., et al. (2004). SulA-independent filamentation of Escherichia coli during growth after release from high hydrostatic pressure treatment. Appl. Microbiol. Biotechnol. 64, 255–262. doi: 10.1007/s00253-003-1465-6
Pubmed Abstract | Pubmed Full Text | CrossRef Full Text | Google Scholar
Keating, D. H., and Cronan, J. E. (1996). An isoleucine to valine substitution in Escherichia coli acyl carrier protein results in a functional protein of decreased molecular radius at elevated pH. J. Biol. Chem. 271, 15905–15910. doi: 10.1074/jbc.271.27.15905
Pubmed Abstract | Pubmed Full Text | CrossRef Full Text | Google Scholar
Klumpp, S., Zhang, Z., and Hwa, T. (2009). Growth rate-dependent global effects on gene expression in bacteria. Cell 139, 1366–1375. doi: 10.1016/j.cell.2009.12.001
Pubmed Abstract | Pubmed Full Text | CrossRef Full Text | Google Scholar
Lauro, F. M., Tran, K., Vezzi, A., Vitulo, N., Valle, G., and Bartlett, D. H. (2008). Large-scale transposon mutagenesis of Photobacterium profundum SS9 reveals new loci important for growth at low temperature and high pressure. J. Bacteriol. 190, 1699–1709. doi: 10.1128/JB.01176-07
Pubmed Abstract | Pubmed Full Text | CrossRef Full Text | Google Scholar
Lee, D. H., Feist, A. M., Barrett, C. L., and Palsson, B. Ø. (2011). Cumulative number of cell divisions as a meaningful timescale for adaptive laboratory evolution of Escherichia coli. PLoS ONE 6:e26172. doi: 10.1371/journal.pone.0026172
Pubmed Abstract | Pubmed Full Text | CrossRef Full Text | Google Scholar
Meganathan, R., and Marquis, R. E. (1973). Loss of bacterial motility under pressure. Nature 246, 525–527. doi: 10.1038/246525a0
Pubmed Abstract | Pubmed Full Text | CrossRef Full Text | Google Scholar
Malone, A. S., Chung, Y. K., and Yousef, A. E. (2006). Genes of Escherichia coli O157:H7 that are involved in high-pressure resistance. Appl. Environ. Microbiol. 72, 2661–2671. doi: 10.1128/AEM.72.4.2661-2671.2006
Pubmed Abstract | Pubmed Full Text | CrossRef Full Text | Google Scholar
Marquis, R. E., and Bender, G. R. (1980). Isolation of a variant of Streptococcus faecalis with enhanced barotolerance. Can. J. Microbiol. 26, 371–376. doi: 10.1139/m80-060
Pubmed Abstract | Pubmed Full Text | CrossRef Full Text | Google Scholar
McDonnell, Z., Andrew, M. P., and Buesseler, K. O. (2010). Variability in the average sinking velocity of marine particles. Limnol. Oceanogr. 55, 2085–2096. doi: 10.4319/lo.2010.55.5.2085
Meersman, F., Daniel, I., Bartlett, D. H., Winter, R., Hazael, R., and McMillain, P. F. (2013). High-pressure biochemistry and biophysics. Rev. Mineral. Geochem. 75, 607–648. doi: 10.2138/rmg.2013.75.19
Meersman, F., and McMillan, P. F. (2014). High hydrostatic pressure: a probing tool and a necessary parameter in biophysical chemistry. Chem. Commun. 50, 766–775. doi: 10.1039/c3cc45844j
Pubmed Abstract | Pubmed Full Text | CrossRef Full Text | Google Scholar
Pourmir, A., and Johannes, T. W. (2012). Directed evolution: selection of the host organism. Comput. Struct. Biotechnol. J. 2:e201209012. doi: 10.5936/csbj.201209012
Pubmed Abstract | Pubmed Full Text | CrossRef Full Text | Google Scholar
Prieur, D., Jebbar, M., Bartlett, D., Kato, C., and Oger, P. (2009). “Piezophilic prokaryotes,” in Comparative High Pressure Biology, ed E. Sebert (Enfield, NH: Science Publishers), 285–322.
Rock, C. O., and Jackowski, S. (2002). Forty years of bacterial fatty acid synthesis. Biochem. Biophys. Res. Commun. 292, 1155–1166. doi: 10.1006/bbrc.2001.2022
Pubmed Abstract | Pubmed Full Text | CrossRef Full Text | Google Scholar
Rolfe, M. D., Rice, C. J., Lucchini, S., Pin, C., Thompson, A., Cameron, A. D. S., et al. (2012). Lag phase is a distinct growth phase that prepares bacteria for exponential growth and involves transient metal accumulation. J. Bacteriol. 194, 686–701. doi: 10.1128/JB.06112-11
Pubmed Abstract | Pubmed Full Text | CrossRef Full Text | Google Scholar
Roujeinikova, A., Baldock, C., Simon, W. J., Gilroy, J., Baker, P. J., Stuitje, A. R., et al. (2002). X-ray crystallographic studies on butyryl-ACP reveal flexibility of the structure around a putative acyl chain binding site. Structure 10, 825–835. doi: 10.1016/S0969-2126(02)00775-X
Pubmed Abstract | Pubmed Full Text | CrossRef Full Text | Google Scholar
Sato, T., Nakamura, Y., Nakashima, K. K., Kato, C., and Horikoshi, K. (1996). High pressure represses expression of the malB operon in Escherichia coli. FEMS Microbiol. Lett. 135, 111–116. doi: 10.1111/j.1574-6968.1996.tb07974.x
Pubmed Abstract | Pubmed Full Text | CrossRef Full Text | Google Scholar
Schwarz, J. R., and Landau, J. V. (1972). Hydrostatic pressure effects on Escherichia coli: site of inhibition of protein synthesis. J. Bacteriol. 109, 945–948.
Vanlint, D., Mitchell, R., Bailey, E., Meersman, F., McMillan, P. F., Michiels, C. W., et al. (2011). Rapid acquisition of gigapascal-high-pressure resistance by Escherichia coli. MBio 2, e00130–e00110. doi: 10.1128/mBio.00130-10
Pubmed Abstract | Pubmed Full Text | CrossRef Full Text | Google Scholar
Vanlint, D., Rutten, N., Michiels, C. W., and Aertsen, A. (2012). Emergence and stability of high-pressure resistance in different food-borne pathogens. Appl. Environ. Microbiol. 78, 3234–3241. doi: 10.1128/AEM.00030-12
Pubmed Abstract | Pubmed Full Text | CrossRef Full Text | Google Scholar
Welch, T. J., and Bartlett, D. H. (1998). Identification of a regulatory protein required for pressure−responsive gene expression in the deep−sea bacterium Photobacterium species strain SS9. Mol. Microbiol. 27, 977–985. doi: 10.1046/j.1365-2958.1998.00742.x
Pubmed Abstract | Pubmed Full Text | CrossRef Full Text | Google Scholar
Welch, T. J., Farewell, A., Neidhardt, F. C., and Bartlett, D. H. (1993). Stress response of Escherichia coli to elevated hydrostatic pressure. J. Bacteriol. 175, 7170–7177.
Worsham, L. M. S., Earls, L., Jolly, C., Langston, K. G., Trent, M. S., and Ernst-Fonberg, M. L. (2003). Amino acid residues of Escherichia coli acyl carrier protein involved in heterologous protein interactions. Biochemistry 42, 167–176. doi: 10.1021/bi0261950
Pubmed Abstract | Pubmed Full Text | CrossRef Full Text | Google Scholar
Yayanos, A. A., and DeLong, E. F. (1987). “Deep-sea bacterial fitness to environmental temperatures and pressures,” in Current Perspectives in High Pressure Biology, eds H. W. Jannasch, R. E. Marquis, and A. M. Zimmerman (Toronto, ON: Academic Press), 17–32.
Yayanos, A. A., and Pollard, E. C. (1969). A study of the effects of hydrostatic pressure on macromolecular synthesis in Escherichia coli. Biophys. J. 9, 1464–1482. doi: 10.1016/S0006-3495(69)86466-0
Pubmed Abstract | Pubmed Full Text | CrossRef Full Text | Google Scholar
Zhou, A., Baidoo, E., He, Z., Mukhopadhyay, A., Baumohl, J. K., Benke, P., et al. (2013). Characterization of NaCl tolerance in Desulfovibrio vulgaris Hildenborough through experimental evolution. ISME J. 7, 1790–1802. doi: 10.1038/ismej.2013.60
Pubmed Abstract | Pubmed Full Text | CrossRef Full Text | Google Scholar
Keywords: adaptive laboratory evolution, Escherichia coli, high pressure, piezophile, acpP
Citation: Marietou A, Nguyen ATT, Allen EE and Bartlett DH (2015) Adaptive laboratory evolution of Escherichia coli K-12 MG1655 for growth at high hydrostatic pressure. Front. Microbiol. 5:749. doi: 10.3389/fmicb.2014.00749
Received: 11 October 2014; Accepted: 10 December 2014;
Published online: 07 January 2015.
Edited by:
Mark Alexander Lever, Swiss Federal Institute of Technology in Zürich, SwitzerlandReviewed by:
Aude Picard, Harvard University, USAChristian Tamburini, Mediterranean Institute of Oceanography, France
Copyright © 2015 Marietou, Nguyen, Allen and Bartlett. This is an open-access article distributed under the terms of the Creative Commons Attribution License (CC BY). The use, distribution or reproduction in other forums is permitted, provided the original author(s) or licensor are credited and that the original publication in this journal is cited, in accordance with accepted academic practice. No use, distribution or reproduction is permitted which does not comply with these terms.
*Correspondence: Douglas H. Bartlett, Marine Biology Research Division, Center for Marine Biotechnology and Biomedicine, Scripps Institution of Oceanography, University of California, San Diego, 9500 Gilman Drive, La Jolla, CA 92093-0202, USA e-mail:ZGJhcnRsZXR0QHVjc2QuZWR1
†These authors have contributed equally to this work.