- Department of Agricultural, Food and Nutritional Science, University of Alberta, Edmonton, AB, Canada
High hydrostatic pressure is commercially applied to extend the shelf life of foods, and to improve food safety. Current applications operate at ambient temperature and 600 MPa or less. However, bacteria that may resist this pressure level include the pathogens Staphylococcus aureus and strains of Escherichia coli, including shiga-toxin producing E. coli. The resistance of E. coli to pressure is variable between strains and highly dependent on the food matrix. The targeted design of processes for the safe elimination of E. coli thus necessitates deeper insights into mechanisms of interaction and matrix-strain interactions. Cellular targets of high pressure treatment in E. coli include the barrier properties of the outer membrane, the integrity of the cytoplasmic membrane as well as the activity of membrane-bound enzymes, and the integrity of ribosomes. The pressure-induced denaturation of membrane bound enzymes results in generation of reactive oxygen species and subsequent cell death caused by oxidative stress. Remarkably, pressure resistance at the single cell level relates to the disposition of misfolded proteins in inclusion bodies. While the pressure resistance E. coli can be manipulated by over-expression or deletion of (stress) proteins, the mechanisms of pressure resistance in wild type strains is multi-factorial and not fully understood. This review aims to provide an overview on mechanisms of pressure-mediated cell death in E. coli, and the use of this information for optimization of high pressure processing of foods.
Introduction
Processing with high hydrostatic pressure in the range of 400–600 MPa has become a commercially viable unit operation in food production. The commercial application of pressure processing since the early 1990 ties was favored by an increasing body of research documenting beneficial effects on food quality and safety (Buckow et al., 2013; Balasubramaniam et al., 2015), the need to introduce alternative processing methods to maintain the safety of ready-to-eat foods (Gottlieb et al., 2006; Farber et al., 2011), and the increasing availability and suitability of commercial-scale high pressure equipment (Tonello, 2011). The commercial use of high pressure applications particularly includes its use as an alternative to thermal preservation (San Martín et al., 2002; Zhu et al., 2005; Tonello, 2011; Balasubramaniam et al., 2015). Pressure applications aiming to achieve food preservation are designed to obtain a bactericidal effect comparable to pasteurization but an improved retention of nutritional or sensory attributes when compared to thermally processed products (Tonello, 2011; Balasubramaniam et al., 2015; Georget et al., 2015).
Hydrostatic pressure in the range of 400–600 MPa inactivates food-borne viruses (Kingsley et al., 2002) and vegetative bacterial cells including many of the food-associated spoilage organisms and pathogens (Patterson et al., 1995; Garriga et al., 2004; Bièche et al., 2009; Jofré et al., 2010). Despite the current commercial use of pressure to eliminate bacteria in food, several concerns hamper the more widespread use of pressure in food preservation:
- Most bacterial endospores and few fungal ascospores resist pressure application at ambient temperature without loss of viability (Butz et al., 1996; Black et al., 2007). Pressure resistant ascospores and endospores are particularly relevant as spoilage organisms in fruit juices (Lee et al., 2006).
- The thermal elimination of microorganisms is typically predicted on the basis of D-and z-values that are derived from log-linear models. While this approach is error-prone, it is simple, robust, and of sufficient accuracy to allow the design of safe commercial processes. Despite numerous successful approaches to achieve a mathematical description of pressure-death-time data (Kilimann et al., 2006; Chen, 2007; Koseki and Yamamoto, 2007), these models do not exhibit sufficient simplicity, accuracy, or widespread acceptance to predict the bactericidal effect of pressure in commercial applications.
- The post pressure survival of target organisms is as relevant as the direct lethal effect of pressure treatment. Depending on the choice of food matrix, pH, and target organism, a post-treatment quarantine period allows for the elimination of surviving bacterial cells (Garcia-Graells et al., 1998; Jordan et al., 2001; Kilimann et al., 2005). However, post-pressure storage or incubation also supports resuscitation of injured cells that were undetectable after pressure treatment (Garriga et al., 2004; Koseki and Yamamoto, 2006).
- Bacterial resistance to pressure exhibits a large intra-species variability (Alpas et al., 1999; Benito et al., 1999; Liu et al., 2015) and particularly the species Escherichia coli and Staphylococcus aureus comprise strains that resist application of 600 MPa at ambient temperature with only a minimal reduction of cell counts (Alpas et al., 2000; Tassou et al., 2008). Pressure resistant mutant strains of Listeria monocytogenes and E. coli are readily isolated in the laboratory and wild type strains with a comparable and exceptional resistance to pressure occur in food (Hauben et al., 1997; Karatzas and Bennik, 2002; Liu et al., 2015). Validated strain cocktails for use in high pressure challenge studies have been described only for few bacterial species (Garcia-Hernandez et al., 2015).
- The bactericidal effect of pressure is highly dependent on the food matrix. The synergistic and antagonistic interactions of pressure and low pH, high temperature, and low water activity on bacterial inactivation are well understood (Garcia-Graells et al., 1998; Smelt, 1998; Molina-Gutierrez et al., 2002; Molina-Höppner et al., 2004). Effects of low-temperature treatments (Luscher et al., 2004), or additional interactions with the food matrix, however, are less well described and often require a case-by-case evaluation of the bactericidal effect of pressure in a given food matrix.
The further exploitation of pressure as preservation technology thus requires an improved understanding of pressure-mediated cell death and sublethal injury and the interaction of pressure with intrinsic or extrinsic factors prevailing in food. Recent reviews provide an excellent overview on the role of pH and water activity on the inactivation of vegetative bacterial cells and bacterial endospores (Georget et al., 2015). This communication aims to complement past reviews by providing an overview on the current knowledge of mechanisms of pressure-mediated cell death and injury and their relevance for food preservation, focusing on pathogenic E. coli. The physiology and genetics of this organisms are well understood, moreover, this species comprises strains that are of major public health concern (Croxen et al., 2013), as well as strains that exhibit exceptional resistance to pressure (Hauben et al., 1997; Vanlint et al., 2012; Liu et al., 2015).
Pressure-mediated Elimination of E. coli in Food: An Overview
Numerous studies provide data on the inactivation of E. coli in food; Table 1 categorizes literature data by product type with reference to serotype and pathotype. Table 1 highlights the variability of the effects of pressure on E. coli in food, demonstrating that pressure effects are strain and matrix dependent. In each product category, some studies report a reduction of cell counts of less than 99% after treatment with 400–600 MPa at ambient temperature, while other studies report a reduction of cell counts exceeding 8 log(cfu/g) (Table 1). Likewise, treatment of the same strain in different food products at comparable conditions resulted in highly variable lethal effects (Table 1). Despite this substantial variability, three major trends can be derived from the data compiled in Table 1. First, studies employing strain cocktails or single strains selected for pressure resistance typically report lower process lethality when compared to studies employing single (outbreak) strains (Table 1). Second, the resistance of E. coli in meat and (fluid) milk is higher when compared to the resistance in low-pH fruit products. In meat and milk, treatments at 400–600 MPa at ambient temperature result in a reduction of cell counts by 5 to less than 1 log(cfu/g) while comparable treatments in some fruit juices reduced cell counts by more than 6 log(cfu/g). Third, treatment at elevated temperature (>40°C) greatly enhances process lethality and eliminates even pressure-resistant strains (Table 1). The combination of pressure treatment with elevated temperature and/or low pH, however, is not suitable for all food products and preservation of low-acid and temperature sensitive food thus required the identification of additional antimicrobial hurdles that act synergistically with pressure. Pressure sensitive targets in cells of E. coli and the possible exploitation of these targets for development of hurdle technologies are discussed in the subsequent sections.
Pressure Mediated Disruption of the Outer Membrane
The barrier properties of the Gram-negative outer membrane mediate resistance against antimicrobial peptides including lysozyme, lactoferrin, and bacteriocins from lactic acid bacteria, and hydrophobic inhibitors including bile acids, which are ingredients of most selective media for E. coli or coliform bacteria (Vaara, 1992; Gänzle et al., 1999; Nikaido, 2003). The observation that pressure permeabilizes the outer membrane of Gram-negative bacteria was initially based on the synergistic activity of pressure and pediocin or nisin (Kalchayanand et al., 1992). Pressure application also sensitizes E. coli to lactoferrin and lysozyme, lactoferrin, and the lactoperoxidase system (Hauben et al., 1996; Garcia-Graells et al., 2000; Masschalck et al., 2001a,b). In situ determination of the permeabilization of the outer membrane suggested that the outer membrane is reversibly permeabilized concomitant with compression, followed by the irreversible loss of lipid A and outer membrane proteins (Figure 1; Ritz et al., 2000; Gänzle and Vogel, 2001). The outer membrane is stabilized by electrostatic interactions of Ca2+ and lipid A (Vaara, 1992); electrostatic interactions are pressure sensitive. Outer membrane porins are over-expressed during growth at elevated pressure (Nakashima et al., 1995) and the pressure-resistant strain E. coli AW1.7 is distinguished by most other strains of E. coli by expression of the porin NmpC (Ruan et al., 2011; Liu et al., 2012). The outer membrane lipoproteins NlpI, YbaY, and OsmE increase pressure resistance of E. coli, presumably through stabilization of the outer membrane (Charoenwong et al., 2011).
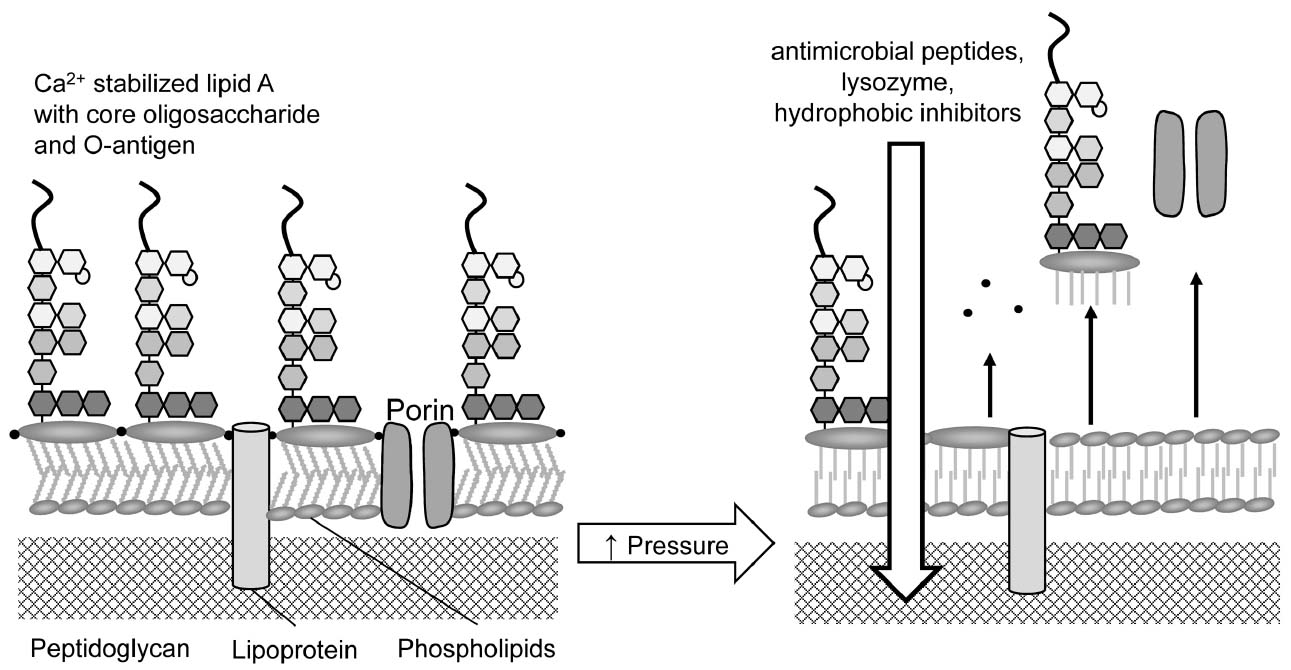
Figure 1. Pressure effects on the outer membrane of E. coli. The outer leaflet of the outer membrane is composed of a lipopolysaccharide layer which prevents penetration of large or hydrophobic molecules to the periplasm. Lipid A molecules are cross-linked by divalent cations. Porins provide channels for small hydrophilic compounds; lipoproteins that are anchored in the peptidoglycan stabilize the outer membrane. Pressure application disrupts the electrostatic interactions between divalent cations and negatively charged LPS molecules, resulting in dissociation of lipid A from the outer membrane and the integration of phospholipids in the outer leaflet. Outer membrane proteins also dissociate from the membrane (Ritz et al., 2000). This process permits entry of hydrophobic inhibitors (Kalchayanand et al., 1992; Hauben et al., 1996; Gänzle and Vogel, 2001). The uncommon porin NmpC may contribute to pressure resistance in E. coli AW1.7 (Ruan et al., 2011), and the porin OmpX is over-expressed in E. coli during grown at elevated pressure (Nakashima et al., 1995). Lipoproteins including OsmB and NlpI contribute to structural integrity of E. coli, and mediate pressure resistance (Charoenwong et al., 2011).
Pressure-mediated disruption of the outer membrane does not compromise the viability of E. coli, however, it may allow synergistic elimination of E. coli by combination of pressure with outer membrane-impermeant inhibitors. The synergistic activity of pressure with bacteriocins, lactoferrin, or the lactoperoxidase system was demonstrated in selected applications. Bacteriocin-producing cheese starter cultures acted synergistically with pressure to eliminate E. coli O157:H7 in raw milk cheese (Rodriguez et al., 2005), the differential inactivation when compared to non-bacteriocin producing cultures was modest but significant. Synergistic activity of bacteriocins and pressure against E. coli was also demonstrated in a meat model system (Garriga et al., 2002). Combination of the lactoperoxidase system and 600 MPa resulted in a reduction of cell counts of E. coli MG1655 by 4 log (cfu/mL) while use of lactoperoxidase or pressure alone was not bactericidal (Garcia-Graells et al., 2000).
Pressure Mediated Damage to the Cytoplasmic Membrane, pH Homeostasis, and Osmoregulation
Bacterial membranes are among the most pressure sensitive targets in bacterial cells. An overview on pressure-mediated damage to the cytoplasmic membrane is provided in Figure 2. Pressure application induces a phase transition from the physiological, liquid-crystalline phase to the gel phase (Winter, 2002). The pressure-induced phase transition of the cytoplasmic membrane also inhibits membrane bound enzymes (Wouters et al., 1998) and dissipates the proton motive force (Molina-Gutierrez et al., 2002). The in vivo observation of pressure-induced membrane phase transitions was achieved in Lactobacillus plantarum and Lactococcus lactis (Molina-Gutierrez et al., 2002; Ulmer et al., 2002) but not in E. coli, where observations of phase transitions of the cytoplasmic membrane are confounded by the outer membrane. The rapid dissipation of the proton motive force by pressure, however, was confirmed in E coli by in situ observation of the pH-dependent GFP fluorescence (Kilimann et al., 2005). Pressure as low as 10 MPa inhibits motility and substrate transport in E. coli (Bartlett, 2002). Remarkably, transport enzymes that are related to pH homeostasis of E. coli exhibit a differential resistance to pressure. Treatment of E. coli with 300 MPa inactivated arginine- and glucose dependent pH homeostasis but not the glutamate decarboxylase system (Figure 2; Kilimann et al., 2005). Pressure resistance is influenced by membrane fluidity and fatty acid composition (Casadei et al., 2002). Exponential phase cell are more sensitive to pressure when compared to stationary phase cells (Pagan and Mackey, 2000; Casadei et al., 2002). Stationary phase cells of E. coli convert unsaturated membrane lipids to cyclopropane fatty acids (Brown et al., 1997; Grogan and Cronan, 1997). Stationary phase cells also have a higher degree of crosslinking among membrane proteins and are less prone to lateral phase transition (Mirelman and Siegel, 1979; Souzu, 1986). Disruption of the cyclopropane fatty acid synthase has a decisive influence on the pressure resistance of E. coli (Charoenwong et al., 2011), confirming the prominent role of membrane properties in pressure-mediated cell death.
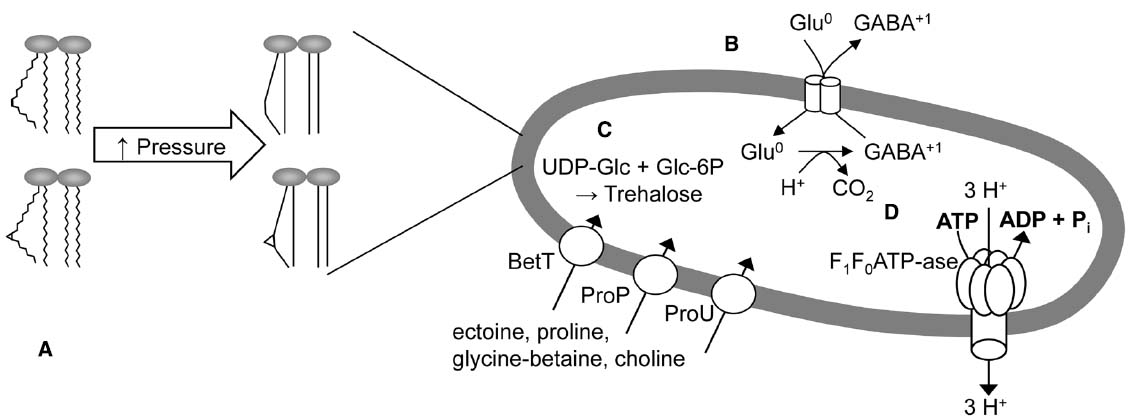
Figure 2. Pressure effects on the cytoplasmic membrane and membrane bound proteins in E. coli. (A) High pressure decreases lateral motion and induces phase transition in the phospholipid bilayers of E. coli, and promotes gelation of the membrane lipids (Pagan and Mackey, 2000; Winter, 2002; Mañas and Mackey, 2004). Pressure resistance is influenced by membrane fluidity and fatty acid composition (Casadei et al., 2002). Exponential phase cell are more sensitive to pressure when compared to stationary phase cells (Pagan and Mackey, 2000; Casadei et al., 2002). Stationary phase cell express cfa encoding for cyclopropane fatty acyl phospholipid synthase (CFA). CFA converts unsaturated fatty acids to cyclopropane fatty acids, which contribute to acid resistance (Brown et al., 1997; Grogan and Cronan, 1997) and pressure resistance in E. coli (Charoenwong et al., 2011). (B) Sublethal pressure inactivates acid resistance in E. coli. The glutamate decarboxylase system for acid resistance is more resistant to pressure than other acid resistance mechanisms, and glutamic acid decarboxylation improved the survival of E. coli during post-pressure acid challenge (Kilimann et al., 2005). (C) The accumulation of compatible solutes including glycine-betaine, choline and sucrose, and the synthesis of trehalose protects against pressure-induced cell death (Van Opstal et al., 2003; Molina-Höppner et al., 2004; Charoenwong et al., 2011); BetT, ProP, and ProU are the major transporters for compatible solutes in E. coli. Mutants that are defective in trehalose synthesis exhibit a reduced resistance to pressure (Charoenwong et al., 2011). (D) Pressure inactivates F0F1-ATPase, which causes disruption of the acid efflux system (Wouters et al., 1998).
Pressure resistance of bacterial cells is intimately linked to osmoregulation and the expression of outer membrane porins. Uptake of compatible solutes in response to osmotic up-shock generally increases bacterial resistance to pressure (Molina-Höppner et al., 2004; Smiddy et al., 2004); sucrose concentrations exceeding 30% also protect E. coli against pressure-mediated cell death (Van Opstal et al., 2003). E. coli responds to osmotic up-shock by import of ectoine, proline, glycine-betaine, and choline, and by synthesis of trehalose (Figure 2; Sleator and Hill, 2002; Charoenwong et al., 2011). Disruption of trehalose biosynthesis substantially reduced the resistance of E. coli to pressure (Malone et al., 2006; Charoenwong et al., 2011). It is noteworthy that piezophilic adaptation to pressure also includes the accumulation of compatible solutes (Bartlett, 2002).
The strong link between bacterial adaptation to high osmotic pressure and their resistance to pressure makes the pressure-mediated elimination of E. coli in foods with low water activity challenging or even impossible. However, pressure mediated membrane damage and disruption of pH homeostasis allows the elimination of E. coli in acidic food products, particularly fruit juices (Table 1, Garcia-Graells et al., 1998; Jordan et al., 2001). The loss of acid resistance results in a pH- and pressure dependent reduction of cell counts within a few days after pressure treatment (Garcia-Graells et al., 1998; Jordan et al., 2001). It remains unclear, however, whether the substantial difference of pressure resistance of E. coli in fruit juices relates only to the low pH, or also involves presence (or absence) of other food constituents that affect pressure resistance in E. coli. For example, the inactivation of E. coli in fruit juices is strongly enhanced by essential oils (Espina et al., 2012).
Pressure-mediated Damage to Cytoplasmic Components: Ribosomes, Oxidative Stress, and Protein Folding
An overview on pressure-induced changes to cytoplasmic components is provided in Figure 3. E. coli incubated at an inhibitory but sublethal pressure of 55 MPa respond by over-expression of heat shock proteins and ribosomal proteins, suggesting that protein synthesis and protein folding are major targets for pressure-mediated cell death and injury (Welch et al., 1993).
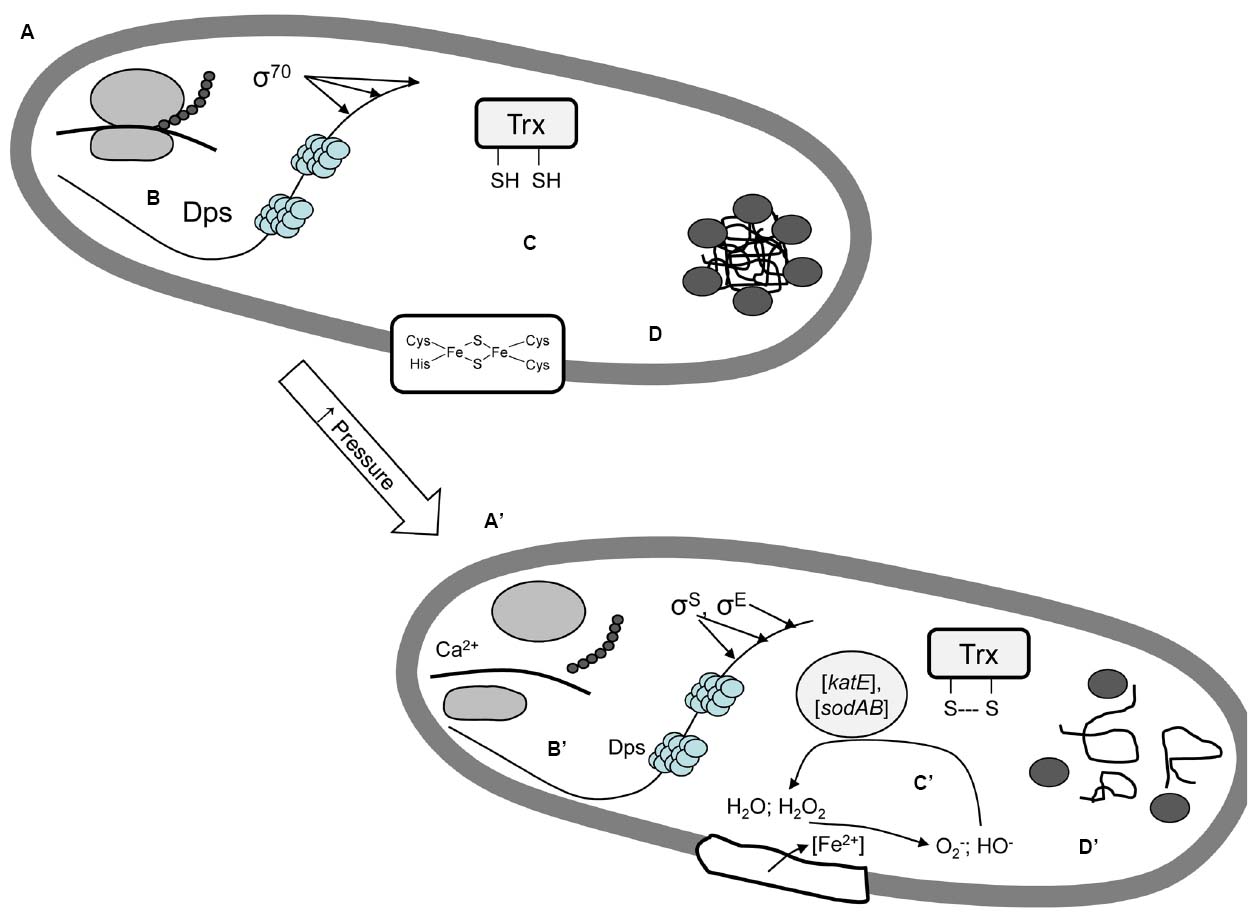
Figure 3. Pressure effects on cytoplasmic proteins and nucleic acids in E. coli. (A,A’) Pressure dissociates ribosomes and inhibits protein synthesis; ribosomes are stabilized by addition of divalent cations (Hauben et al., 1998; Niven et al., 1999; Gayan et al., 2013). (B,B’) Dps (DNA binding protein from starved cells) binds DNA as homo-dodecamer and protects E. coli against oxidative stress-, pressure-, and acid-induced DNA damage (Choi et al., 2000; Zhao et al., 2002; Malone et al., 2006). Deletion of the genes coding for the alternative sigma factors σE or σS increases the sensitivity of E. coli to pressure; indicating that the general stress response (σS) and the heat shock response (σE) increase pressure resistance (Robey et al., 2001; Aertsen et al., 2004, 2005; Malone et al., 2006). (C,C’) High pressure-induces oxidative stress in E. coli. Proteins that protect against peroxide and superoxide stress (thioredoxin, catalase, superoxide dismutase, and proteins that regulate their expression) also increase pressure resistance in E. coli (Aertsen et al., 2005; Malone et al., 2006; Charoenwong et al., 2011). The presence of iron and iron sulfur cluster proteins increases the lethality of pressure on E. coli (Malone et al., 2006; Yan et al., 2013), likely because free intracellular iron accumulates and catalyses the formation of reactive oxygen species. (D,D’) Pressure disassembles protein aggregates bodies in vivo, re-growth of sublethally injured cells after pressure treatment is dependent on the time required for re-assembly of protein aggregates. The presence of the locus of heat resistance which predominantly encodes genes involved in protein folding and protein turnover is generally associated with pressure resistance in E. coli and loss of the locus of heat resistance reduces the pressure resistance in E. coli AW1.7 (Garcia-Hernandez et al., 2015; Liu et al., 2015; Mercer et al., personal communication). Deletion of the inclusion body binding proteins IbpA and IbpB decreases pressure resistance (Charoenwong et al., 2011). The heat shock proteins DnaK and DnaJ contribute to assembly and segregation of protein aggregates (Aertsen et al., 2004; Govers et al., 2014), and mediate pressure resistance.
Pressure dissociates ribosomes and inhibits protein synthesis (Niven et al., 1999, Figure 3A). Ribosomes are stabilized by addition of divalent cations (Niven et al., 1999). Pressure-induced changes to the ribosome and DNA damage are less pronounced in stationary-phase cells of E. coli, possibly reflecting the protective effect of the σS mediated overexpression of stress proteins preventing DNA damage (Mañas and Mackey, 2004). A direct relationship of ribosome dissociation, accumulation of compatible solutes and cellular survival was shown for heat resistance (Pleitner et al., 2012) but not for pressure resistance in E. coli AW1.7. The significant baro-protective effect of divalent cations on E. coli may partially relate to the stabilization of ribosomes (Hauben et al., 1998; Niven et al., 1999; Gayan et al., 2013).
The relationship between protein (mis)-folding, protein turnover and pressure resistance was also initially suggested by Welch et al. (1993). Resistance of E. coli to lethal pressure also relates to the expression of heat shock proteins (Aertsen et al., 2004; Figure 3D). Although results obtained in different studies are not always consistent, disruption of genes coding for the cold shock protein CspA, the heat shock proteins DnaK and DnaJ, and the chaperones IbpAB decrease resistance of E. coli to pressure (Malone et al., 2006; Charoenwong et al., 2011; Govers et al., 2014). Direct evidence for the relationship between protein (mis)-folding and pressure resistance was provided by Govers et al. (2014). Exposure of E. coli to 300 MPa dissociated GFP-labeled aggregates of misfolded proteins. Remarkably, the lag time of individual cells after pressure treatment was correlated to the time required for the re-assembly of protein aggregates (Govers et al., 2014).
Pressure treatment of E. coli in buffer systems inflicts oxidative stress (Figure 3C). Aertsen et al. (2005) directly quantified oxidative stress using cytoplasmic alkaline phosphatase as a probe. Pressure application strongly increased the oxidation of cytoplasmic disulfide bonds. The disruption of genes related to protection against oxidative stress (catalase and superoxide dismutase) decreased resistance to pressure. Malone et al. (2006) confirmed that genes providing protection against oxidative stress (DbpS, thioredoxin) also confer resistance to pressure. Remarkably, the deletion of genes coding for assembly of iron-sulfur clusters increased the resistance of E. coli to pressure (Malone et al., 2006), and intracellular free iron accelerates pressure-mediated cell death (Yan et al., 2013). Taken together, these studies indicate that pressure denatures proteins containing iron-sulfur clusters, resulting in the accumulation of iron in the cytoplasm. Iron catalyzes the formation of reactive oxygen species, causing oxidative stress. Consequently, proteins that detoxify reactive oxygen species, and proteins that are involved in the cytoplasmic redox-homeostasis also increase resistance to pressure (Aertsen et al., 2005; Malone et al., 2006; Charoenwong et al., 2011).
Many of the proteins involved in pressure resistance of E. coli are stress proteins and their expression is governed by stress-responsive alternative sigma factors, including σE or σS. Deletion of rpoE coding for σE decreased the stress resistance of E. coli (Malone et al., 2006) but proteins of the σE regulon that are responsible for this effect remain to be identified. The σS regulon plays a central role in the general stress response of E. coli, many of the proteins that contribute to baroresistance are up-regulated by σS (Landini et al., 2014). Examples include osmoresponsive outer membrane proteins, cyclopropane fatty acid synthase, Dps, catalase, and superoxide dismutase (Landini et al., 2014, Figures 2 and 3). Consequently, deletion of rpoS strongly increases the sensitivity of E. coli to pressure (Aertsen et al., 2005; Malone et al., 2006; Charoenwong et al., 2011). Loss of the anti-σS regulator RssB increased resistance of E. coli to 300 MPa (Vanlint et al., 2013b). The variation of pressure resistance between different strains of E. coli relates to σS sequence diversity (Robey et al., 2001) and exposure of E. coli to sublethal pressure selects for σS activity (Vanlint et al., 2013a).
Despite the involvement of the stress-responsive σE and σS in pressure resistance of E. coli, a general correlation of pressure resistance to the resistance to other stressors has not been observed. Detailed information for more than 100 strains is available on the correlation of heat- and pressure resistance in E. coli (Liu et al., 2015). Pressure-resistant mutant strains of E. coli also exhibit elevated heat resistance (Hauben et al., 1997) and extremely heat resistant strains of E. coli are also resistant to pressure (Garcia-Hernandez et al., 2015; Liu et al., 2015). Extreme heat resistance in E. coli is conferred by the locus of heat resistance, a 14 kb genomic island containing 16 predicted open reading frames encoding putative heat shock proteins and proteases (Mercer et al., personal communication). Loss of the locus of heat resistance is accompanied by a substantial increase of the sensitivity to thermal inactivation (Pleitner et al., 2012) but causes only a modest increase of the sensitivity to pressure (Liu et al., 2015). Moreover, pressure resistance is also observed in strains that do not harbor the locus of heat resistance (Liu et al., 2015; Mercer et al., personal communication), demonstrating that multiple routes of acquiring pressure resistance exist in E. coli.
The loss of genetic material that appears unrelated to the stress response in E. coli increases its resistance to pressure (Malone et al., 2006; Vanlint et al., 2013b). The loss of genetic material is readily achieved by laboratory selection for resistance to pressure or membrane perturbators (Vanlint et al., 2012; Pleitner et al., 2012). The loss of genetic material rather than specific mutations may account for the ease of selection of pressure-resistance in E. coli (Hauben et al., 1997; Vanlint et al., 2012) as well as the large variation of pressure resistance in the species (Table 1, Liu et al., 2015).
Pressure effects on cytoplasmic proteins account for some of the synergistic or antagonistic interaction of pressure with food constituents. The baroprotecive effect of Ca2+ and Mg2+ may relate to the resistance of E. coli in milk and meat (Table 1). The concentration of free cytoplasmic iron correlates to pressure-induced cell death (Yan et al., 2013), however, the iron-rich meat matrix supports a high pressure resistance of E. coli (Table 1). The survival of pressure treated E. coli is significantly improved when incubated anaerobically compared to aerobic incubation (Aertsen et al., 2005) and pressure-treated foods are generally packaged without inclusion of air. A systematic screening of natural antimicrobial inhibitors revealed that only thiol-reactive inhibitory compounds exhibit synergistic antimicrobial activity with pressure (Feyaerts et al., 2015). This finding directly relates the mode of action of antimicrobial compounds to the “oxidative suicide” mechanism of pressure-mediated cell death (Figure 3; Feyaerts et al., 2015); however, this synergistic activity remains to be documented in food.
Concluding Remarks
Research in the past two decades has identified multiple pressure-sensitive targets in E. coli that contribute to sublethal injury and cell death, including the composition and barrier properties of the outer and cytoplasmic membranes, ribosome assembly and functionality, protein folding, and oxidative stress caused by metabolic imbalance and/or the release of iron from denatured proteins (Figures 1,2, and 3). It remains unclear whether these targets are simultaneously or sequentially affected during high pressure treatment; however, survival during pressure treatment and post-pressure survival under adverse conditions are based on different mechanisms. Corresponding to the multiple pressure-sensitive targets in E. coli, pressure resistance is apparently a multi-factorial phenotype. The high frequency of E. coli strains with extreme pressure resistance (Liu et al., 2015) as well as the reproducible occurrence of pressure resistant mutant strains (Vanlint et al., 2012) indicates that several alternative routes to pressure resistance exist in E. coli. Some of these apparently include the mere loss of a few genes with no direct relation to membrane stress or the stress response (Vanlint et al., 2013b).
Owing to the multi-faceted pressure resistance of E. coli and the multiple factors influencing post-pressure survival in foods, the elimination of E. coli with high pressure as sole preservation step remains challenging or impossible. Current knowledge allows the safe elimination of E. coli by pressure treatment at a moderately elevated temperature, or by combination of pressure treatment at a pH of less than 4.5 in combination with a post-treatment incubation period to eliminate sublethally injured cells (Table 1). The improved knowledge on mechanisms of pressure-induced cell death and sublethal injury in E. coli as well as an improved understanding of the physiological and genetic determinants of pressure resistance in E. coli may allow the development of additional hurdle technologies to achieve food preservation with high pressure technology.
Conflict of Interest Statement
The authors declare that the research was conducted in the absence of any commercial or financial relationships that could be construed as a potential conflict of interest.
Acknowledgments
The Alberta Livestock and Meat Agency is acknowledged for funding; MG acknowledges the Canada Research Chairs Program for funding.
References
Aertsen, A., Vanoirbeek, K., De Spiegeleer, P., Sermon, J., Hauben, K., Farewell, A., et al. (2004). Heat shock protein-mediated resistance to high hydrostatic pressure in Escherichia coli. Appl. Environ. Microbiol. 70, 2660–2666. doi: 10.1128/AEM.70.5.2660-2666.2004
Aertsen, A., De Spiegeleer, P., Vanoirbeek, K., Lavilla, M., and Michiels, C. W. (2005). Induction of oxidative stress by high hydrostatic pressure in Escherichia coli. Appl. Environ. Microbiol. 71, 2226–2231. doi: 10.1128/AEM.71.5.2226-2231.2005
Alpas, H., and Bozoglu, F. (2000). The combined effect of high hydrostatic pressure, heat and bacteriocins on inactivation of foodborne pathogens in milk and orange juice. World J. Microbiol. Biotechnol. 16, 387–392. doi: 10.1023/A:1008936607413
Alpas, H., Kalchayanand, N., Bozoglu, F., and Ray, B. (2000). Interactions of high hydrostatic pressure, pressurization temperature and pH on death and injury of pressure-resistant and pressure-sensitive strains of foodborne pathogens. Intl. J. Food Microbiol. 60, 33–42. doi: 10.1016/S0168-1605(00)00324-X
Alpas, H., Kalchayanand, N., Bozoglu, F., Sikes, A., Dunne, C. P., and Ray, B. (1999). Variation in resistance to hydrostatic pressure among strains of food-borne pathogens. Appl. Environ. Microbiol. 65, 4248–4251.
Baccus-Taylor, G. S., Falloon, O. C., and Henry, N. (2015). Pressure resistance of cold-shocked Escherichia coli O157:H7 in ground beef, beef gravy and peptone water. J. Appl. Microbiol. 118, 1521–1529. doi: 10.1111/jam.12794
Balasubramaniam, V. M., Martinez-Monteagudo, S. I., and Gupta, R. (2015). Principles and application of high pressure—based technologies in the food industry. Ann. Rev. Food Sci. Technol. 6, 435–462. doi: 10.1146/annurev-food-022814-015539
Bartlett, D. H. (2002). Pressure effects on in vivo microbial processes. Biochim. Biophys. Acta 1595, 367–381. doi: 10.1016/S0167-4838(01)00357-0
Benito, A., Venoura, G., Casadei, M., Robinson, T., and Mackey, B. (1999). Variation in resistance of natural isolates of Escherichia coli O157 to high hydrostatic pressure, mild heat, and other stresses. Appl. Environ. Microbiol. 65, 1564–1569.
Bièche, D., Ritz, M., Tresse, O., Federighi, M., and de Lamballerie, M. (2009). Impacts of treatment parameters on the inactivation of Campylobacter jejuni by high pressure: a statistical study of main effects and interactions. Lett. Appl. Microbiol. 48, 198–202. doi: 10.1111/j.1472-765X.2008.02511.x
Black, E. P., Hirneisen, K. A., Hoover, D. G., and Kniel, K. E. (2010). Fate of Escherichia coli O157:H7 in ground beef following high-pressure processing and freezing. J. Appl. Microbiol. 108, 1352–1360. doi: 10.1111/j.1365-2672.2009.04532.x
Black, E. P., Setlow, P., Hocking, A. D., Stewart, C. M., Kelly, A. L., and Hoover, D. G. (2007). Response of spores to high-pressure processing. Comp. Rev. Food Sci. Food Safety. 6, 103–119. doi: 10.1111/j.1541-4337.2007.00021.x
Brown, J. L., Ross, T., McMeekin, T., and Nichols, P. D. (1997). Acid habituation of Escherichia coli and the potential role of cyclopropane fatty acids in low pH tolerance. Int. J. Food Microbiol. 37, 163–173. doi: 10.1016/S0168-1605(97)00068-8
Buckow, R., Sikes, A., and Tume, R. (2013). Effect of high pressure on physicochemical properties of meat. Crit. Rev. Food Sci. Nutr. 53, 770–786. doi: 10.1080/10408398.2011.560296
Butz, P., Funtenberger, S., Haberditzl, T., and Tauscher, B. (1996). High pressure inactivation of Byssochlamys nivea ascospores and other heat resistant molds. Lebensm. Wiss. Technol. 29, 404–410. doi: 10.1006/fstl.1996.0062
Buzrul, S., Alpas, H., Largeteau, A., and Demazeau, G. (2008). Inactivation of Escherichia coli and Listeria innocua in kiwifruit and pineapple juices by high hydrostatic pressure. Int. J. Food Microbiol. 124, 275–278. doi: 10.1016/j.ijfoodmicro.2008.03.015
Casadei, M. A., Mañas, P., Niven, G. W., Needs, E., and Mackey, B. M. (2002). Role of membrane fluidity in pressure resistance of Escherichia coli NCTC 8164. Appl. Environ. Microbiol. 68, 5965–5972. doi: 10.1128/AEM.68.12.5965-5972.2002
Charoenwong, D., Andrews, S., and Mackey, B. (2011). Role of rpoS in the development of cell envelope resilience and pressure resistance in stationary-phase Escherichia coli. Appl. Environ. Microbiol. 77, 5220–5229. doi: 10.1128/AEM.00648-11
Chen, H. (2007). Use of linear, Weibull, and log-logistic functions to model pressure inactivation of seven foodborne pathogens in milk. Food Microbiol. 24, 197–204. doi: 10.1016/j.fm.2006.06.004
Choi, S. H., Baumler, D. J., and Kaspar, C. W. (2000). Contribution of dps to acid stress tolerance and oxidative stress tolerance in Escherichia coli O157:H7. Appl. Environ. Microbiol. 66, 3911–3916. doi: 10.1128/AEM.66.9.3911-3916.2000
Croxen, M. A., Law, R. J., Scholz, R., Keeney, K. M., Wlodarska, M., and Finlay, B. B. (2013). Recent advances in understanding enteric pathogenic Escherichia coli. Clin. Microbiol. Rev. 26, 822–880. doi: 10.1128/CMR.00022-13
De Lamo-Castellví, S., Capellas, M., Roig-Sagués, A. X., López-Pedemonte, T., Hernández-Herrero, M. M., and Guamis, B. (2006). Fate of Escherichia coli strains inoculated in model cheese elaborated with or without starter and treated by high hydrostatic pressure. J. Food Prot. 69, 2856–2864.
Drake, M. A., Harrison, S. L., Asplund, M., Barbosa-Canovas, G., and Swanson, B. G. (1997). High pressure treatment of milk and effects on microbiological and sensory quality of Cheddar cheese. J. Food Sci. 62, 843–845. doi: 10.1111/j.1365-2621.1997.tb15468.x
Espina, L., García-Gonzalo, D., Laglaoui, A., Mackey, B. M., and Pagán, R. (2012). Synergistic combinations of high hydrostatic pressure and essential oils or their constituents and their use in preservation of fruit juices. Int. J. Food Microbiol. 161, 23–30. doi: 10.1016/j.ijfoodmicro.2012.11.015
Farber, J. M., Kozaka, G. K., and Duquette, S. (2011). Changing regulation: canada’s new thinking on Listeria. Food Control. 22, 1506–1509. doi: 10.1016/j.foodcont.2010.07.019
Feyaerts, J., Rogiers, G., Corthouts, J., and Michiels, C. W. (2014). Thiol-reactive natural antimicrobials and high pressure treatment synergistically enhance bacterial inactivation. Innov. Food Sci. Emerg. Technol. 27, 26–34. doi: 10.1016/j.ifset.2014.12.005
Gänzle, M. G., Hertel, C., and Hammes, W. P. (1999). Resistance of Escherichia coli and Salmonella against nisin and curvacin A. Int. J. Food Microbiol. 48, 37–50. doi: 10.1016/S0168-1605(99)00026-4
Gänzle, M. G., and Vogel, R. F. (2001). On-line fluorescence determination of pressure-mediated outer membrane damage in Escherichia coli. Syst. Appl. Microbiol. 24, 477–485. doi: 10.1078/0723-2020-00069
Garcia-Graells, C., Hauben, K. J., and Michiels, C. W. (1998). High-pressure inactivation and sublethal injury of pressure-resistant Escherichia coli mutants in fruit juices. Appl. Environ. Microbiol. 64, 1566–1568.
Garcia-Graells, C., Valckx, C., and Michiels, C. W. (2000). Inactivation of Escherichia coli and Listeria innocua in milk by combined treatment with high hydrostatic pressure and the lactoperoxidase system. Appl. Environ. Microbiol. 66, 4173–4179. doi: 10.1128/AEM.66.10.4173-4179.2000
Garcia-Hernandez, R., McMullen, L., and Gänzle, M. G. (2015). Development and validation of a surrogate strain cocktail to evaluate bactericidal effects of pressure on verotoxigenic Escherichia coli. Int. J. Food Microbiol. 205, 16–22. doi: 10.1016/j.ijfoodmicro.2015.03.028
Garriga, G., Aymerich, M. T., Costa, S., Montfort, J. M., and Hugas, M. (2002). Bactericidal synergism through bacteriocins and high pressure in a meat mode3l system during storage. Food Microbiol. 19, 509–518. doi: 10.1006/fmic.2002.0498
Garriga, M., Grèbol, N., Aymerich, M. T., Monfort, J. M., and Hugas, M. (2004). Microbial inactivation after high-pressure processing at 600 MPa in commercial meat products over its shelf life. Innov. Food Sci. Emerg. Technol. 5, 451–457. doi: 10.1016/j.ifset.2004.07.001
Gayan, E., Condón, S., Alvarez, I., Nabakabaya, M., and Mackey, B. (2013). Effect of pressure-induced changes in the ionization equilibria of buffers on inactivation of Escherichia coli and Staphylococcus aureus. Appl. Environ. Microbiol. 79, 4041–4047. doi: 10.1128/AEM.00469-13
Georget, E., Sevenich, R., Reineke, K., Mathys, A., Heinz, V., Callanan, M., et al. (2015). Inactivation of microorganisms by high isostatic pressure processing in complex matrices: a review. Inoov. Food Sci. Emerg. Technol. 27, 1–14. doi: 10.1016/j.ifset.2014.10.015
Gill, A. O., and Ramaswamy, H. O. S. (2008). Application of high pressure processing to kill Escherichia coli O157 in ready-to-eat meats. J. Food Prot. 71, 2182–2189.
Gottlieb, S. L., Newbern, E. C., Griffin, P. M., Graves, L. M., Hoekstra, R. M., Baker, N. L., et al. (2006). Multistate outbreak of Listeriosis linked to turkey deli meat and subsequent changes in US regulatory policy. Clin. Infect. Dis. 42, 29–36. doi: 10.1086/498113
Govers, S. K., Dutré, P., and Aertsen, A. (2014). In vivo disassembly and reassembly of protein aggregates in Escherichia coli. J. Bacteriol. 196, 2325–2332. doi: 10.1128/JB.01549-14
Grogan, D. W., and Cronan, J. E. (1997). Cyclopropane ring formation in membrane lipids of bacteria. Microbiol. Mol. Biol. Rev. 61, 429–441.
Hauben, K. J. A., Bernaerts, K., and Michiels, C. W. (1998). Protective effect of calcium on inactivation of Escherichia coli by high hydrostatic pressure. J. Appl. Microbiol. 85, 678–684. doi: 10.1111/j.1365-2672.1998.00577.x
Hauben, K. J. A., Wuytack, E. Y., Soontjens, C. C. F., and Michiels, C. W. (1996). High-pressure transient sensitization of Escherichia coli to lysozyme and nisin by disruption of outer-membrane permeability. J. Food Prot. 59, 350–355.
Hauben, K. J., Bartlett, D. H., Soontjens, C. C., Wuytack, E. Y., and Michiels, C. W. (1997). Escherichia coli mutants resistant to inactivation by high hydrostatic pressure. Appl. Environ. Microbiol. 63, 945–950.
Hsu, H., Sheen, S., Sites, J., Cassidy, J., Scullen, B., and Sommers, C. (2015). Effect of high pressure processing on the survival of Shiga Toxin-producing Escherichia coli (Big Six vs. O157:H7) in ground beef. Food Microbiol. 48, 1–7. doi: 10.1016/j.fm.2014.12.002
Hsu, H., Sheen, S., Sites, J., Huang, L., and Wu, J. S. (2014). Effect of high pressure treatment on the survival of Shiga toxin-producing Escherichia coli in strawberry puree. Food Microbiol. 40, 25–30. doi: 10.1016/j.fm.2013.11.019
Huang, Y., Ye, M., and Chen, H. (2013). Inactivation of Escherichia coli O157:H7 and Salmonella spp. in strawberry puree by high hydrostatic pressure with/without subsequent frozen storage. Int. J. Food Microbiol. 160, 337–343. doi: 10.1016/j.ijfoodmicro.2012.11.008
Jofré, A., Aymerich, T., Bover-Cid, S., and Garriga, M. (2010). Inactivation and recovery of Listeria monocytogenes, Salmonella enterica, and Staphylococcus aureus after high hydrostatic pressure treatments up to 900 MPa. Int. Microbiol. 13, 105–112.
Jordan, S. L., Pascual, C., Bracey, E., and Mackey, B. M. (2001). Inactivation and injury of pressure-resistant strains of Escherichia coli O157 and Listeria monocytogenes in fruit juices. J. Appl. Microbiol. 91, 463–469. doi: 10.1046/j.1365-2672.2001.01402.x
Kalchayanand, M., Hanlin, M. B., and Ray, B. (1992). Sublethal injury makes Gram-negative and resistant Gram-positive bacteria sensitive to the bacteriocins pediocin AcH and nisin. Lett. Appl. Microbiol. 15, 239–243. doi: 10.1111/j.1472-765X.1992.tb00773.x
Karatzas, K. A., and Bennik, M. H. (2002). Characterization of a Listeria monocytogenes Scott A isolate with high tolerance towards high hydrostatic pressure. Appl. Environ. Microbiol. 68, 3183–3189. doi: 10.1128/AEM.68.7.3183-3189.2002
Kilimann, K. V., Hartmann, C., Vogel, R. F., and Gänzle, M. G. (2005). Differential inactivation of glucose- and glutamate-dependent acid resistance of Escherichia coli TMW 2.497 by high pressure treatments. Syst. Appl. Microbiol. 28, 663–671. doi: 10.1016/j.syapm.2005.05.004
Kilimann, K. V., Kitsubun, P., Delgado, A., Gänzle, M. G., Chapleau, N., Le Bail, A., et al. (2006). Experimental and numerical study of heterogeneous pressure-temperature induced lethal and sublethal injury of Lactococcus lactis in a medium scale high pressure autoclave. Biotechnol. Bioeng. 94, 655–666. doi: 10.1002/bit.20852
Kingsley, D. H., Hoover, D. G., Papafragkou, E., and Richards, G. P. (2002). Inactivation of hepatitis A virus and a calicivirus by high hydrostatic pressure. J. Food. Prot. 65, 1605–1609.
Koseki, S., and Yamamoto, K. (2006). Recovery of Escherichia coli ATCC 25922 in phosphate buffered saline after treatment with high hydrostatic pressure. Int. J. Food Microbiol. 110, 108–111. doi: 10.1016/j.ijfoodmicro.2006.01.039
Koseki, S., and Yamamoto, K. (2007). A novel approach to predicting microbial inactivation kinetics during high pressure processing. Int. J. Food Microbiol. 116, 275–282. doi: 10.1016/j.ijfoodmicro.2007.01.008
Landini, P., Egli, T., Wolf, J., and Lacour, S. (2014). SigmaS, a major player in the response to environmental stresses in Escherichia coli: role, regulation and mechanisms of promoter recognition. Environ. Microbiol. Rep. 6, 1–13. doi: 10.1111/1758-2229.12112
Lavinas, F. C., Miguel, M. A. L., Lopes, M. L. M., and Valente-Mesquita, V. L. (2008). Effect of high hydrostatic pressure on cashew apple (Anacardium occidentale L.) juice preservation. J. Food Sci. 73, 273–277. doi: 10.1111/j.1750-3841.2008.00791.x
Lee, S. Y., Chung, H. J., and Kang, D. H. (2006). Combined treatment of high pressure and heat on killing spores of Alicyclobacillus acidoterrestris in apple juice concentrate. J. Food Prot. 69, 1056–1060.
Liu, Y., Betti, M., and Gänzle, M. G. (2012). High pressure inactivation of Escherichia coli, Campylobacter jejuni, and spoilage microbiota on poultry meat. J. Food Prot. 75, 497–503. doi: 10.4315/0362-028X.JFP-11-316
Liu, Y., Gill, A., McMullen, L., and Gänzle, M. G. (2015). Variation in heat and pressure resistance of verotoxigenic and non-toxigenic Escherichia coli. J. Food Prot. 78, 111–120. doi: 10.4315/0362-028X.JFP-14-267
Luscher, C., Balasa, A., Fröhling, A., Ananta, E., and Knorr, D. (2004). Effect of high-pressure-induced ice I-to-ice III phase transitions on inactivation of Listeria innocua in frozen suspension. Appl. Environ. Microbiol. 70, 4021–4029. doi: 10.1128/AEM.70.7.4021-4029.2004
Malone, A. S., Chuang, Y.-K., and Yousef, A. E. (2006). Genes of Escherichia coli O157:H7 that are involved in high-pressure resistance. Appl. Environ. Microbiol. 72, 2661–2671. doi: 10.1128/AEM.72.4.2661-2671.2006
Mañas, P., and Mackey, B. M. (2004). Morphological and physiological changes induced by high hydrostatic pressure in exponential- and stationary-phase cells of Escherichia coli: relationship with cell death. Appl. Environ. Microbiol. 70, 1545–1554. doi: 10.1128/AEM.70.3.1545-1554.2004
Masschalck, B., Van Houdt, R., Van Haver, E. G., and Michiels, C. W. (2001a). Inactivation of gram-negative bacteria by lysozyme, denatured lysozyme, and lysozyme-derived peptides under high hydrostatic pressure. Appl. Environ. Microbiol. 67, 339–344. doi: 10.1128/AEM.67.1.339-344.2001
Masschalck, B., Van Houdt, R., and Michiels, C. W. (2001b). High pressure increases bactericidal activity and spectrum of lactoferrin, lactoferricin and nisin. Int. J. Food Microbiol. 64, 325–332. doi: 10.1016/S0168-1605(00)00485-2
Mirelman, D., and Siegel, R. C. (1979). Oxidative deamination of aminolysine residues and formation of Schiff base cross linkages in cell envelopes of Escherichia coli. J. Biol. Chem. 254, 571–574
Molina-Gutierrez, A., Stippl, V., Delgado, A., Gänzle, M. G., and Vogel, R. F. (2002). Effect of pH on pressure inactivation and intracellular pH of Lactococcus lactis and Lactobacillus plantarum. Appl. Environ. Microbiol., 68, 4399–4406. doi: 10.1128/AEM.68.9.4399-4406.2002
Molina-Höppner, A., Doster, W., Vogel, R. F., and Gänzle, M. G. (2004). Protective effect of sucrose and sodium chloride for Lactococcus lactis during sublethal and lethal high-pressure treatments. Appl. Environ. Microbiol. 70, 2013–2020. doi: 10.1128/AEM.70.4.2013-2020.2004
Morales, P., Calzada, J., Avila, M., and Nuñez, M. (2008). Inactivation of Escherichia coli O157:H7 in ground beef by single-cycle and multiple-cycle high-pressure treatments. J. Food Prot. 71, 811–815.
Nakashima, K., Horikosh, K., and Mizuno, T. (1995). Effect of hydrostatic pressure on the synthesis of outer membrane proteins in Escherichia coli. Biosci. Biotechnol. Biochem. 59, 130–132. doi: 10.1271/bbb.59.130
Nakimbugwe, D., Masschalck, B., Anim, G., and Michiels, C. W. (2006). Inactivation of Gram-negative bacteria in milk and banana juice by hen egg white and lambda-lysozyme under high hydrostatic pressure. Int. J. Food Microbiol. 112, 19–25. doi: 10.1016/j.ijfoodmicro.2006.06.010
Nikaido, H. (2003). Molecular basis of bacterial outer membrane permeability revisited. Microbiol. Mol. Biol. Rev. 67, 593–656. doi: 10.1128/MMBR.67.4.593-656.2003
Niven, G. W., Miles, C. A., and Mackey, B. M. (1999). The effects of hydrostatic pressure on ribosome conformation in Escherichia coli: an in vivo study using differential scanning calorimetry. Microbiology 145, 419–425. doi: 10.1099/13500872-145-2-419
Omer, M. K., Alvseike, O., Holck, A., Axelsson, L., Prieto, M., Skjerve, E., et al. (2010). Application of high pressure processing to reduce verotoxigenic E. coli in two types of dry-fermented sausage. Meat Sci. 86, 1005–1009. doi: 10.1016/j.meatsci.2010.08.008
Pagan, R., and Mackey, B. (2000). Relationship between membrane damage and cell death in pressure-treated Escherichia coli cells: differences between exponential-and stationary-phase cells and variation among strains. Appl. Environ. Microbiol. 66, 2829–2834. doi: 10.1128/AEM.66.7.2829-2834.2000
Patterson, M. F., Quinn, M., Simpson, R., and Gilmour, A. (1995). Sensitivity of vegetative pathogens to high hydrostatic pressure treatment in phosphate-buffered saline and foods. J. Food Prot. 58, 524–529.
Pleitner, A., Zhai, Y., Winter, R., Ruan, L., McMullen, L. M., and Gänzle, M. G. (2012). Compatible solutes contribute to heat resistance and ribosome stability in Escherichia coli AW1.7. Biochim. Biophys. Acta. 1824, 1351–1357. doi: 10.1016/j.bbapap.2012.07.007
Porto-Fett, A. C. S., Call, J. E., Shoyer, B. E., Hill, D. E., Pshebniski, C., Cocoma, G. J., et al. (2010). Evaluation of fermentation, drying, and/or high pressure processing on viability of Listeria monocytogenes, Escherichia coli O157:H7, Salmonella spp., and Trichinella spiralis in raw pork and Genoa salami. J. Food Microbiol. 140, 61–75. doi: 10.1016/j.ijfoodmicro.2010.02.008
Reineke, K., Sevenich, R., Hertwig, C., Janßen, T., Fröhling, A., Knorr, D., et al. (2015). Comparative study on the high pressure inactivation behavior of the Shiga toxin-producing Escherichia coli O104:H4 and O157:H7 outbreak strains and a non-pathogenic surrogate. Food Microbiol. 46, 184–194. doi: 10.1016/j.fm.2014.07.017
Ritz, M., Feulet, M., Orange, N., and Federighi, M. (2000). Effects of high hydrostatic pressure on membrane proteins of Salmonella typhimurium. Int. J. Food Microbiol. 55, 115–119. doi: 10.1016/S0168-1605(00)00165-3
Robey, M., Benito, A., Hutson, R. H., Pascual, C., Park, S. F., and Mackey, B. M. (2001). Variation in resistance to high pressure and rpoS heterogeneity in natural isolates of Escherichia coli 0157:H7. Appl. Environ. Microbiol. 67, 4901–4907. doi: 10.1128/AEM.67.10.4901-4907.2001
Rodriguez, E., Arques, J. L., Nuñez, M., Gaya, P., and Medina, M. (2005). Combined effect of high-pressure treatments and bacteriocin-producing lactic acid bacteria on inactivation of Escherichia coli O157:H7 in raw-milk cheese. Appl. Environ. Microbiol. 71, 3399–3404. doi: 10.1128/AEM.71.7.3399-3404.2005
Ruan, L., Pleitner, A., Gänzle, M. G., and McMullen, L. M. (2011). Solute transport proteins and the outer membrane protein NmpC contribute to heat-resistance of Escherichia coli AW1.7. Appl. Environ. Microbiol. 77, 2961–2967. doi: 10.1128/AEM.01930-10
San Martín, M. F., Barbosa-Cánovas, G. V., and Swanson, B. G. (2002). Food processing by high hydrostatic pressure. Crit. Rev. Food Sci. Nutr. 42, 627–645. doi: 10.1080/20024091054274
Sleator, R. D., and Hill, C. (2002). Bacterial osmoadaptation: the role of osmolytes in bacterial stress and virulence. FEMS Microbiol. Rev. 26, 49–71. doi: 10.1111/j.1574-6976.2002.tb00598.x
Smelt, J. P. P. M. (1998). Recent advances in the microbiology of high pressure processing. Trends Food Sci. Technol. 9, 152–158. doi: 10.1016/S0924-2244(98)00030-2
Smiddy, M., Sleator, R. D., Patterson, M. F., Hill, C., and Kelly, A. L. (2004). Role for compatible solutes glycine betaine and L-carnitine in listerial barotolerance. Appl. Environ. Mcirobiol. 70, 7555–7557. doi: 10.1128/AEM.70.12.7555-7557.2004
Souzu, H. (1986). Fluorescence polarization studies on Escherichia coli membrane stability and its relation to the resistance of the cell to freeze-thawing. I. Membrane stability in cells of different growth phase. Biochim. Biophys. Acta 861, 353–360. doi: 10.1016/0005-2736(86)90438-4
Tassou, C. C., Panagou, E. Z., Samaras, F. J., Galiatsatou, P., and Mallidis, C. G. (2008). Temperature-assisted high hydrostatic pressure inactivation of Staphylococcus aureus in a ham model system: evaluation in selective and nonselective medium. J. Appl. Microbiol. 104, 1764–1773. doi: 10.1111/j.1365-2672.2007.03698.x
Teo, A. Y., Ravishankar, S., and Sizer, C. E. (2001). Effect of low temperature, high-pressure treatment on the survival of Escherichia coli O157:H7 and Salmonella in unpasteurized fruit juices. J. Food Prot. 64, 1122–1127.
Tonello, C. (2011). “Case studies for high pressure processing of foods,” in Non-Thermal Processing Technologies for Food, eds H. Q. Zhang, G. V. Barbosa-Canovas, and V. M. Balasubramaniam (WestSussex: Whiley-Blackwell), 36–50.
Ukuku, D. O., Yamamoto, K., Bari, L., Mukhopadhaya, S., Juneja, V., and Kawamoto, S. (2013). Membrane damage and viability loss of E. coli O157:H7 and Salmonella spp in apple juice treated with high hydrostatic pressure and thermal death time disks. J. Food Process. Technol. 4, 236. doi: 10.4172/2157-7110.1000236
Ulmer, H. M., Herberhold, H., Fahsel, S., Gänzle, M. G., Winter, R., and Vogel, R. F. (2002). Effects of pressure induced membrane phase transitions on HorA inactivation in Lactobacillus plantarum. Appl. Environ. Microbiol. 68, 1088–1095. doi: 10.1128/AEM.68.3.1088-1095.2002
Vaara, M. (1992). Agents that increase the permeability of the outer membrane. Microbiol. Rev. 56, 395–411.
Vanlint, D., Rutten, N., Michiels, C. S., and Aertsen, A. (2012). Emergence and stability of high pressure resistance in different foodborne pathogens. Appl. Environ. Microbiol. 78, 3234–3241. doi: 10.1128/AEM.00030-12
Vanlint, D., Rutten, N., Govers, S. K., Michiels, C. W., and Aertsen, A. (2013a). Exposure to high hydrostatic pressure rapidly selects for increased RpoS activity and general stress-resistance in Escherichia coli O157:H7. Int. J. Food Microbiol. 163, 28–33. doi: 10.1016/j.ijfoodmicro.2013.02.001
Vanlint, D., Pype, B. J., Rutten, N., Vanoirbeek, K. G., Michiels, C. W., and Aertsen, A. (2013b). Loss of cAMP/CRP regulation confers extreme high hydrostatic pressure resistance in Escherichia coli O157:H7. Int. J. Food Microbiol. 166, 65–71. doi: 10.1016/j.ijfoodmicro.2013.06.020
Van Opstal, I., Vanmuysen, S. C., and Michiels, C. W. (2003). High sucrose concentration protects E. coli against high pressure inactivation but not against high pressure sensitization to the lactoperoxidase system. Int. J. Food Microbiol. 88, 1–9. doi: 10.1016/S0168-1605(03)00070-9
Vercammen, A., Vanoirbeek, K. G. A., Lemmens, L., Lurquin, I., Hendrickx, M. E. G., and Michiels, C. W. (2012). High pressure pasteurization of apple pieces in syrup: microbiological shelf-life and quality evolution during refrigerated storage. Innov. Food Sci. Emerg. Technol. 16, 259–266. doi: 10.1016/j.ifset.2012.06.009
Welch, T. J., Farewell, A., Neidhardt, F. C., and Bartlett, D. H. (1993). Stress response of Escherichia coli to elevated hydrostatic pressure. J. Bacteriol. 175, 7170–7177.
Whitney, B. M., Williams, R. C., Eifert, J., and Marcy, J. (2007). High-pressure resistance variation of Escherichia coli O157:H7 strains and Salmonella serovars in tryptic soy broth, distilled water, and fruit juice. J. Food Prot. 70, 2078–2083.
Whitney, B. M., Williams, R. C., Eifert, J., and Marcy, J. (2008). High pressures in combination with antimicrobials to reduce Escherichia coli O157:H7 and Salmonella Agona in apple juice and orange juice. J. Food Prot. 71, 820–824.
Winter, R. (2002). Synchrotron X-ray and neutron small-angle scattering of lyotropic lipid mesophases, model biomembranes and proteins in solution at high pressure. Biochim. Biophys. Acta. 1595, 160–184. doi: 10.1016/S0167-4838(01)00342-9
Wouters, P. C., Glaasker, E., and Smelt, J. P. P. M. (1998). Effects of high pressure on inactivation kinetics and events related to proton efflux in Lactobacillus plantarum. Appl. Environ. Microbiol. 64, 509–514.
Yan, Y., Waite-Cusic, J. G., Kuppusamy, P., and Yousef, A. E. (2013). Intracellular free iron and its potential role in ultrahigh-pressure-induced inactivation of Escherichia coli. Appl. Environ. Microbiol. 79, 722–724. doi: 10.1128/AEM.02202-12
Zhao, G., Ceci, P., Ilari, A., Giangiacomo, L., Laue, T. M., Chiancone, E., et al. (2002). Iron and hydrogen peroxide detoxification properties of DNA-binding protein from starved cells. A ferritin-like DNA-binding protein of Escherichia coli. J. Biol. Chem. 277, 27689–22796. doi: 10.1074/jbc.M202094200
Keywords: Escherichia coli, EHEC, STEC, high hydrostatic pressure, food preservation
Citation: Gänzle M and Liu Y (2015) Mechanisms of pressure-mediated cell death and injury in Escherichia coli: from fundamentals to food applications. Front. Microbiol. 6:599. doi: 10.3389/fmicb.2015.00599
Received: 23 April 2015; Accepted: 01 June 2015;
Published: 24 June 2015.
Edited by:
Alexander Mathys, German Institute of Food Technologies (DIL), GermanyReviewed by:
Erika S. Georget, Leibniz Universität Hannover, GermanyRobert Sevenich, Technische Universität Berlin, Germany
Copyright © 2015 Gänzle and Liu. This is an open-access article distributed under the terms of the Creative Commons Attribution License (CC BY). The use, distribution or reproduction in other forums is permitted, provided the original author(s) or licensor are credited and that the original publication in this journal is cited, in accordance with accepted academic practice. No use, distribution or reproduction is permitted which does not comply with these terms.
*Correspondence: Michael Gänzle, Department of Agricultural, Food and Nutritional Science, University of Alberta, 4-10 Ag/For Centre, Edmonton, AB T6G 2P5, Canada,bWdhZW56bGVAdWFsYmVydGEuY2E=