- Research Center for Environmental Changes – Academia Sinica, Taipei, Taiwan
We investigated the influence of varying cobalt (Co) and B12 concentrations to growth and nitrogen fixation of Trichodesmium, a major diazotroph in the tropical and subtropical oligotrophic ocean. Here we show that sufficient inorganic Co, 20 pmol L-1, sustains the growth of Trichodesmium either with or without an additional B12 supply. We also found that in these culture conditions, nitrogen levels fixed by Trichodesmium were higher in treatments with insufficient B12 than in treatments with higher B12 availability. Under limited inorganic Co availability, ranging from 0.2 to 2 pmol L-1, Trichodesmium growth was significantly compromised in cultures without B12. In these low Co concentrations, addition of 400 pmol L-1 of B12 supported phytoplankton growth indicating that B12 supply augmented for the low Co concentrations. Our study demonstrates that Trichodesmium has an absolute Co requirement, which is not replaceable with Zn, and that B12 supply alleviates stress in cases where Co is limiting. These results show that the interlocking availabilities of Co and B12 may influence the growth and nitrogen fixation of Trichodesmium in the ocean.
Introduction
Minor nutrients including essential trace metals and vitamins play important roles in regulating phytoplankton growth and community structure in the ocean (Morel and Price, 2003; Sunda, 2012; Sañudo-Wilhelmy et al., 2014). The interaction of biologically active trace metals with various phytoplankton groups has been broadly investigated through laboratory culture studies, which have contributed to our understanding of varied roles of trace metals in shaping the phytoplankton community structure. Among the different trace metals, there is limited information regarding the cobalt (Co) requirement by phytoplankton aside from the reported Co need by the picocyanobacteria Synechococcus and Prochlorococcus (Sunda and Huntsman, 1995; Saito et al., 2002). These pioneering works paved the way for further researches that widened the understanding of Co concentrations and its role in phytoplankton growth, its distribution and speciation in the ocean, and its interaction with other trace metals (Saito and Moffett, 2002; Saito et al., 2005, 2010; Bertrand et al., 2007; Panzeca et al., 2008, 2009; Noble et al., 2012; Swanner et al., 2014). Dissolved Co concentrations can resemble a nutrient-like vertical profile and may become limiting for phytoplankton growth because both total and bioavailable concentrations in the euphotic zone are generally low (Saito and Moffett, 2001; Saito et al., 2004; Panzeca et al., 2008). For example, dissolved Co concentrations commonly occur in the range between 15 and 81 pmol L-1 in the North Atlantic Ocean and between 26 and 59 pmol L-1 in the Southern Ocean (Panzeca et al., 2008, 2009).
The major biological demand for Co is associated with cobalamin (B12 hereafter), an essential organic growth factor required in several biochemical processes. It is essential because B12 is a cofactor of several enzymes such as methylmalonyl-CoA mutase, methionine synthase, and type II ribonucleotide reductase (Martens et al., 2002; Panzeca et al., 2008; Sañudo-Wilhelmy et al., 2014). Vitamin B12 is a Co-containing tetrapyrrole complex synthesized by some prokaryotes, i.e., archaea and eubacteria, while majority of eukaryotes require an exogenous source (Raux et al., 2000; Warren et al., 2002; Croft et al., 2005). The dependence of most eukaryotes on external B12 supply in the ocean has significant effects on phytoplankton dynamics and composition. This has been demonstrated by stimulated growth of phytoplankton upon B12 enrichment and observed species succession from blooms involving B12-producing phytoplankton to auxotrophic (B12-requiring) species (Panzeca et al., 2008; Koch et al., 2011; Sañudo-Wilhelmy et al., 2012). Recent field studies show further proof that B12 can be a limiting factor for phytoplankton growth in some oceanic regions forcing phytoplankton thriving in these areas to live under a vitamin B12-limiting regime (Panzeca et al., 2008, 2009; Bertrand and Allen, 2012; Bertrand et al., 2012; Sañudo-Wilhelmy et al., 2012, 2014).
In this work, we set out to determine the role of B12 on Trichodesmium growth using batch cultures with replete Fe and P supply. The non-axenic strain Trichodesmium erythraeum was chosen for this study on Co and B12 interaction because it has been successfully cultured in the laboratory using YBC-II culture medium with Co and B12 as components (Chen et al., 1996). The presence of Co in B12 indicates that its synthesis and biological functions may be influenced by ambient bioavailable Co concentrations in seawater leading to a complex interaction between Co and B12 availabilities. Here, we studied this interaction and how it affects the growth of Trichodesmium and we also studied how nitrogen fixation is influenced by B12 availability. Trichodesmium, a non-heterocystous diazotroph, is a ubiquitous phytoplankton considered as a critical source of bioavailable nitrogen in nitrogen-limited regions of the tropical and sub-tropical oceans (Capone et al., 1997; Carpenter et al., 2004; Zehr and Kudela, 2011). The genome of Trichodesmium shows that it contains the genes required for B12 synthesis (Sañudo-Wilhelmy et al., 2014); however, no study pertaining to Co and B12 requirement of Trichodesmium has been reported. The results from this work provides additional information to better understand the Co and B12 requirements and their possible interaction in Trichodesmium, which may have implications in the dynamics of phytoplankton succession and nutrient cycling in the ocean.
Materials and Methods
Culture Conditions
Non-axenic Trichodesmium erythraeum IMS101, obtained from the National Center for Marine Algae and Microbiota, was grown in polycarbonate bottles with trace-metal defined medium (Ho, 2013) modified from the original YBC-II recipe (Chen et al., 1996). Before addition of desired trace metals, we determined background trace metal concentrations in seawater using an automated flow injection-ion chromatograph pretreatment system prior to element detection by high resolution inductively coupled plasma mass spectrometer (HR-ICPMS, Element XR, Thermo Scientific; Ho et al., 2010). The background total dissolved concentrations of Fe, Mn, Zn, Ni, Cu, and Co were 0.14, 0.02, 0.21, 0.04, 0.03, and 0.002 nmol L-1, respectively. The trace metals were then added to the culture medium to achieve these total dissolved concentrations: Fe, Ni, Mo, Mn, Cu, Zn, and Se at 400, 100, 100, 10, 10, 10, and 10 nmol L-1, respectively. Metal bioavailability was controlled by adding ethylenediaminetetraacetic acid (EDTA) at 20 μmol L-1. EDTA can maintain the bioavailable (inorganic) concentrations of trace metals in batch culture medium to be relatively constant because EDTA would bind most of the dissolved metals and regulate the dissolved bioavailable concentrations through the reversible reaction between the metal-EDTA complex and the inorganic form. For example, when bioavailable Co is consumed by the phytoplankton, the Co-EDTA complex would dissociate to keep the complexation reaction at equilibrium and thus maintain a stable inorganic Co concentration in the medium. To maintain bioavailable Co concentrations stable, the total dissolved Co concentrations need to be relatively constant in the culture medium through the experimental period too. As shown in Table 1, the total dissolved Co concentration added was 10 nM and the intracellular Co concentrations only ranged from 7.6 to 11.2 pM, only accounting for about 0.1% of the total concentrations added. The total initial concentration of phosphorus was 50 μmol L-1. These culture conditions were intended to have sufficient P and trace metal supplies in the culture medium. The other B-vitamins, thiamine, and biotin, were added at the suggested levels of 300 and 2.0 nmol L-1, respectively (Chen et al., 1996).
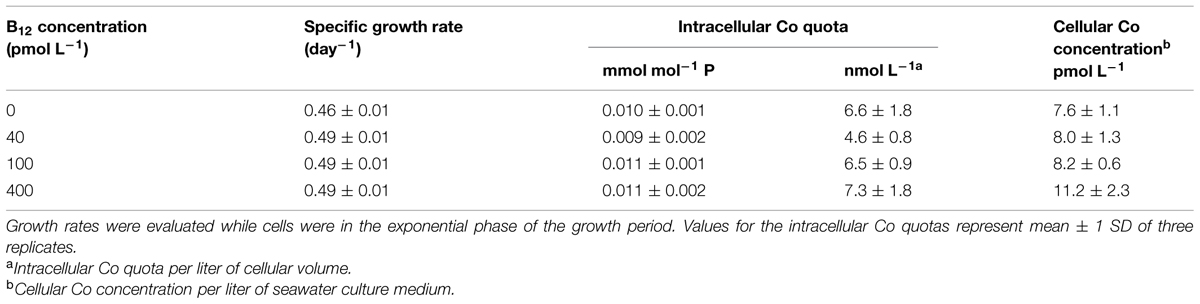
TABLE 1. Growth rates and intracellular cobalt (Co) quotas of Trichodesmium grown in culture media with 20 pmol L-1 Co′ and varying B12 concentrations.
The cultures were kept in 1 L bottles initially leaving a headspace volume equivalent to 150 mL. This headspace volume increased as the incubation period progressed because a 20-mL aliquot was taken from each replicate bottle for cellular volume determination, which was done every 2 days. The bottles were manually closed until finger tight and gently shaken top over bottom to allow homogenization prior to pouring out aliquots for cell counting. Cultures were kept in a temperature-controlled growth chamber fixed at 26°C under a square-wave 12:12 h light:dark regime with photon irradiance set at 600 μmol quanta m-2 s-1, the typical irradiance in the water column where Trichodesmium blooms have been observed (Letelier and Karl, 1998). All cultures during the acclimatization and final experiments were subjected to this temperature and light intensity. The light intensity was achieved by placing culture bottles at appropriate distances from the light source and intensity was validated by measuring light penetration PAR using a submersible radiometer (Biospherical Instruments Inc. QSL 2100). Materials used for culturing were carefully washed with 2% Micro-90® solution, rinsed, soaked with 10% hydrochloric acid solution, and rinsed thoroughly with ultrapure water prepared using a Milli-Q system. All necessary procedures were carried out in a class 100 trace-metal clean laboratory.
Effect of Co and B12 on the Growth of Trichodesmium
In this study, we varied both Co and B12 concentrations to study their effects on the growth of Trichodesmium. The Co concentrations were varied with total dissolved concentrations of 0, 0.1, 1, and 10 nmol L-1 in specific treatments, which would result in inorganic Co (Co′) concentrations of 0, 0.2, 2, and 20 pmol L-1, respectively (Westall et al., 1976). B12 was varied such that treatments either have 0, 40, 100, or 400 pmol L-1. All treatments were carried out in triplicate 1 L polycarbonate bottles. We first carried out a set of experiments where all treatments were supplied with 20 pmol L-1 Co′ while varying the B12 concentrations. For this set, we acclimatized the cells in respective B12 concentrations for two transfers prior to the final experiment. The cultures were transferred to fresh media with desired Co and B12 concentrations when cellular volume reached about 2 × 106 μm3 mL-1 and while cells were in the exponential growth phase. For each transfer, 2 mL of culture was aseptically transferred to new culture medium, which resulted in initial cellular volumes within the range 5 to 8 × 103 μm3 mL-1. This volume used for inoculation also resulted in 500 times dilution of carried over trace metals and B12 from the previous medium. We then carried out another set of experiments with low Co′ concentrations (0, 0.2, and 2 pmol L-1) and two B12 conditions (with or without 400 pmol L-1 B12). For this set of experiments, all treatments in the first transfer were inoculated using cells grown in a medium with 20 pmol L-1 Co′ and 400 pmol L-1 B12. For the second transfer, cells from treatments with B12 in the first transfer were used to inoculate corresponding Co treatments because of low cellular volumes in the treatments without B12.
Phytoplankton Growth and Intracellular Metal Quota Determination
Growth of the phytoplankton was monitored by measuring total cellular volume using a Beckman Coulter Counter Multisizer 3 with a 100 μm aperture tube (Goebel et al., 2008; Ho, 2013). Coulter Counter, based on the Coulter principle widely applied for determination of size and cellular counts, was used for quantification of cellular volume to reflect growth of Trichodesmium. The use of the Coulter Counter has been explained in detail in a previous report (Ho, 2013). Although other parameters such as total organic carbon or fluorescence would be more robust for monitoring phytoplankton growth, we found that use of Coulter Counter is a reliable method for this purpose. The Coulter Counter offers a precision in the 1–5% range and can be used for cultures with cellular volume as low as 5 × 103 μm3 mL-1, which is equivalent to presence of 1–2 trichomes (consisting of about 10–20 cells per trichome) per ml of culture medium. During the exponential phase of growth until early stationary stage, Trichodesmium in different treatments were present as single trichome, and we only observed formation of colonies when it reached the latter stages of the stationary phase. Thus, we made sure to transfer cells to new media while still in the exponential phase of growth to ensure comparable biomass in the inoculum. Growth rates and intracellular metal quotas for the experiments with 20 pmol L-1 Co′ were evaluated while cells were in the exponential growth phase. The growth rates were evaluated between days 3 and 14, where the correlation coefficient between the natural logarithm of cellular volumes and number of days of incubation were equivalent to 0.99 or better. Intracellular metal quotas were determined by harvesting cells by filtration onto acid-washed polycarbonate filters (25 mm with 5 μm pore size) during the light period of the light:dark regime. The filtered cells were washed with ultrapure water and digested using concentrated HNO3 prior to elemental analysis using HR-ICPMS.
Effect of B12 Supply on Nitrogen Fixation
We studied the effect of B12 availability, when Co is sufficient (20 pmol L-1 Co′), on the nitrogen fixation of Trichodesmium. Results from this experiment yielded different nitrogen fixation rates with varying B12 availabilities. Thus a similar experiment, focusing only on the lowest and highest B12 treatments, was carried out to ascertain that the trends observed in the preceding experiment were repeatable. Nitrogen fixation rates were determined using the acetylene reduction method (Capone and Montoya, 2001) following procedures outlined elsewhere (Ho, 2013). In brief, 10 mL aliquots of the cultures (duplicate samples were prepared from each of the triplicate bottles per treatment for each time point) were transferred to 20 mL vials (Agilent). The vials were sealed using Teflon-coated caps and 2 mL air was drawn using a syringe, which was replaced by 2 mL of freshly prepared acetylene to initiate the experiment. The vials were then incubated at the same growth conditions as the original cultures. The time-point experiment was designed so that nitrogen fixation was stopped after every 2 h during the 12 h light phase. The purity of acetylene used for the estimation of nitrogen fixation rates was different during the first and validation experiments. Acetylene prepared in the laboratory by reacting calcium carbide with water was used in the first experiment while commercially available acetylene was used in the validation experiment (99.9999% purity, Kouatsu Gas Kogyo, Japan). Laboratory-prepared acetylene resulted in blank measurements that were three times higher than in commercially available acetylene. Respective blank values were subtracted from sample measurements based on the acetylene used. All acetylene reduction assays were carried out while cells were in the exponential phase of growth and when biomass was higher than 1 × 106 μm3 mL-1. Estimation of dinitrogen reduction was taken from the acetylene reduction using a conversion ratio of 4:1 (Capone and Montoya, 2001), and the assumption that the Bunsen coefficient for ethylene is 0.084 (Breitbarth et al., 2004).
Results
The set of treatments with 20 pmol L-1 Co′ yielded comparable values for growth and intracellular Co quotas. Growth rate for the treatment without B12 was 0.46 ± 0.01 d-1, while the rates determined for the treatments with 40, 100, and 400 pmol L-1 B12 were all at 0.49 ± 0.01 d-1. The growth rates were similar to the maximum growth rates we have obtained previously (Ho, 2013; Ho et al., 2013; Rodriguez and Ho, 2014). The comparable biomass and growth rates indicate that 20 pmol L-1 Co′ was sufficient for Trichodesmium to reach maximum growth rates and that B12 does not significantly affect the growth at this Co′ concentration (Figure 1; Table 1). The determined intracellular Co quotas, normalized against P as biomass indicator, were also comparable suggesting that Co uptake rates were independent of B12 concentrations when Co supply was sufficient (Table 1).
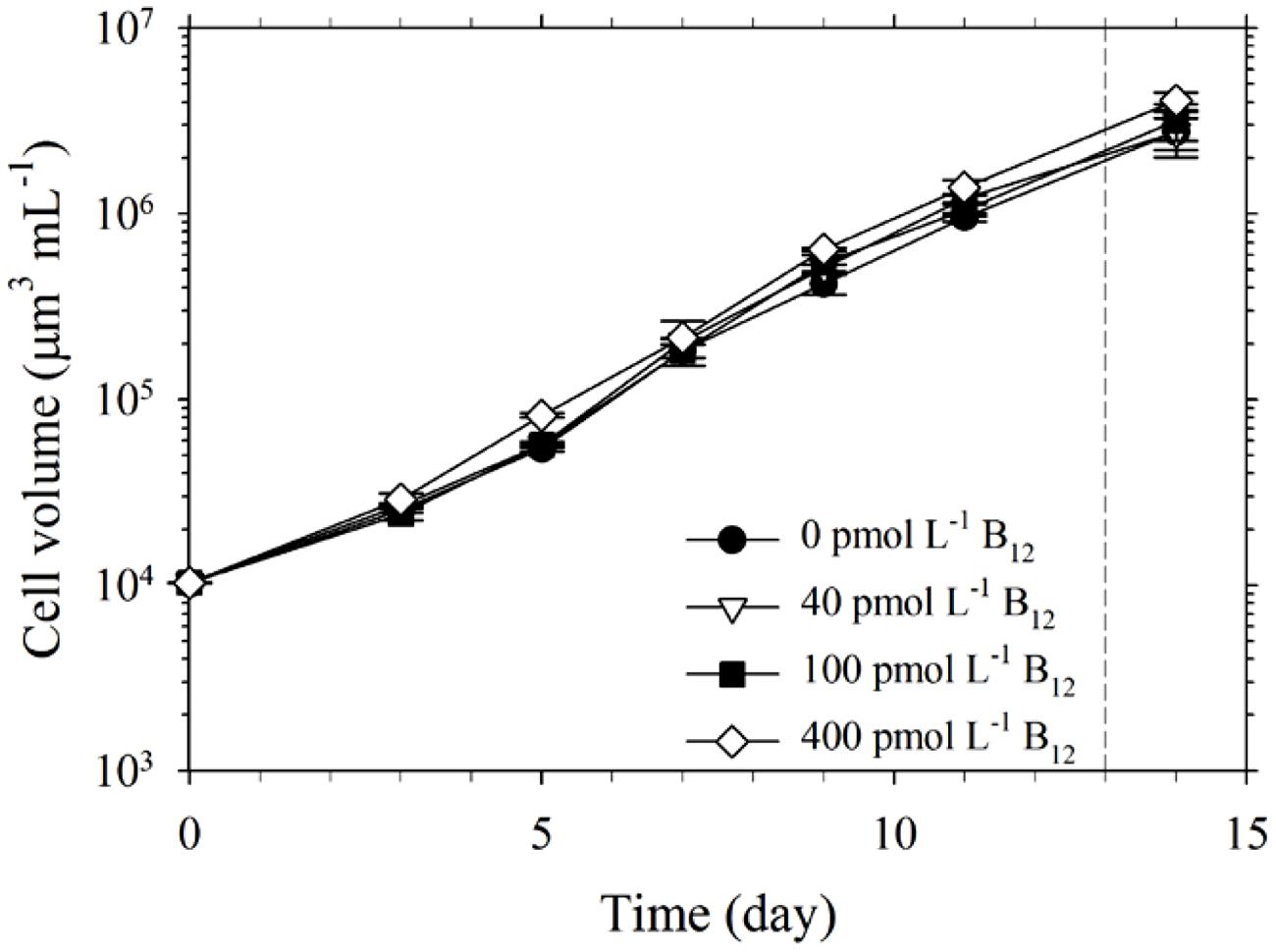
FIGURE 1. Growth of Trichodesmium in culture media with 20 pmol L-1 Co′ and varying B12 concentrations. Cells were harvested for intracellular cobalt (Co) quota determination while in exponential phase of growth (indicated by the dashed line). Values represent mean and error bars represent SD of triplicate culture bottles.
We then varied both Co and B12 availabilities to study whether these would interact and affect Trichodesmium growth. To achieve this, we conducted parallel experiments with different Co′ and B12 concentrations, where Co′ concentrations were 0, 0.2, and 2 pmol L-1 and B12 concentration was either 0 or 400 pmol L-1 for a total of six different treatments (Figure 2). The cells used as inoculum for first transfer were previously grown in a medium with 20 pmol L-1 Co′ and 400 pmol L-1 B12 for about 10 generations. We opted to monitor growth upon first transfer because prior acclimatization experiments we conducted resulted in low density or cell death in cultures with low Co′ concentrations. Thus, we decided to monitor growth upon transfer to low Co′ concentrations and observe the phytoplankton response. All of the different treatments increased in biomass upon transfer but either a noticeable decline or only a slight change in biomass was observed after about 8 days (Figures 2A–C). The treatments with B12 then eventually increased in biomass reaching cell densities needed for the second transfer. For the treatments without B12, only cultures with 2 pmol L-1 Co′ were able to attain a stationary biomass that reached a maximum of 105 μm3 mL-1. The cells in the first transfer from different Co treatments and with B12 added were used to inoculate the second transfer for corresponding Co treatments (Figures 2D–F). In the 0 pmol L-1 Co′ treatments, cultures in both B12 conditions declined in biomass until these reached cellular volumes that were less than 103 μm3 mL-1 (Figure 2D). For cultures with 0.2 pmol L-1 Co′ added, the biomass in both B12 treatments initially declined and then eventually increased after about 25 days of incubation (Figure 2E). The growth curves of cultures with 2 pmol L-1 Co′ (Figure 2F) were similar to corresponding treatments in the first transfer. In this Co′ concentration, the treatments with B12 attained higher cell density than in the treatments without B12.
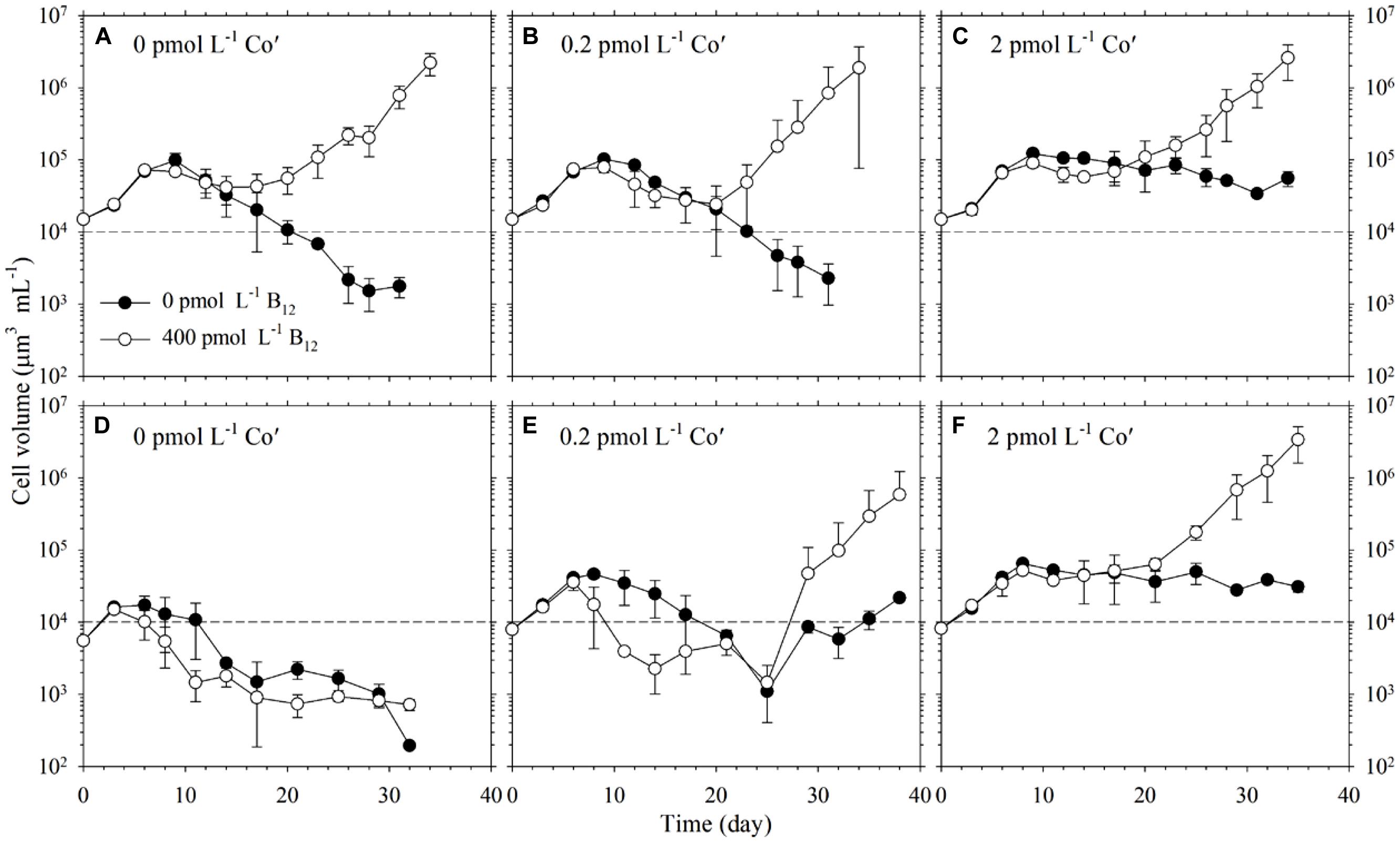
FIGURE 2. Growth of Trichodesmium in varying Co′ and B12 concentrations. (A–C) Top panels show growth curves of the first transfer of cells in different Co and B12 conditions. All treatments were inoculated using cells grown in a medium with 20 pmol L-1 Co′ and 400 pmol L-1 B12 for more than 10 generations. (D–F) Bottom panels show growth curves of the second transfer, which were inoculated from cells in the first transfer grown in respective Co treatments with 400 pmol L-1 B12. Values represent mean and error bars represent SD of triplicate culture bottles. Negative bars for several points in (B,E) were long and were not plotted as a result of the log-scale used in the Y-axis.
The effect of B12 concentration on nitrogen fixation by Trichodesmium was also studied under conditions of sufficient Co availability. Our results show that B12 concentration influenced the nitrogen fixation by Trichodesmium (Figure 3A). Cultures without B12 and lower B12, i.e., 0 and 40 pmol L-1, fixed higher amount of N2 compared to cultures with higher B12 concentrations, i.e., 100 and 400 pmol L-1. This trend was also observed in the validation experiment carried out focusing only on treatments with 0 and 400 pmol L-1 B12 (Figure 3B). Values for nitrogen fixed by Trichodesmium during the validation experiment were higher than values determined in the first experiment because of different purity of acetylene used in the assays. High purity acetylene that was obtained commercially was used in the validation experiment while laboratory-prepared acetylene was used in the first experiment. The trends observed in both experiments indicate that B12 availability influences the nitrogen fixing capacity of this phytoplankton.
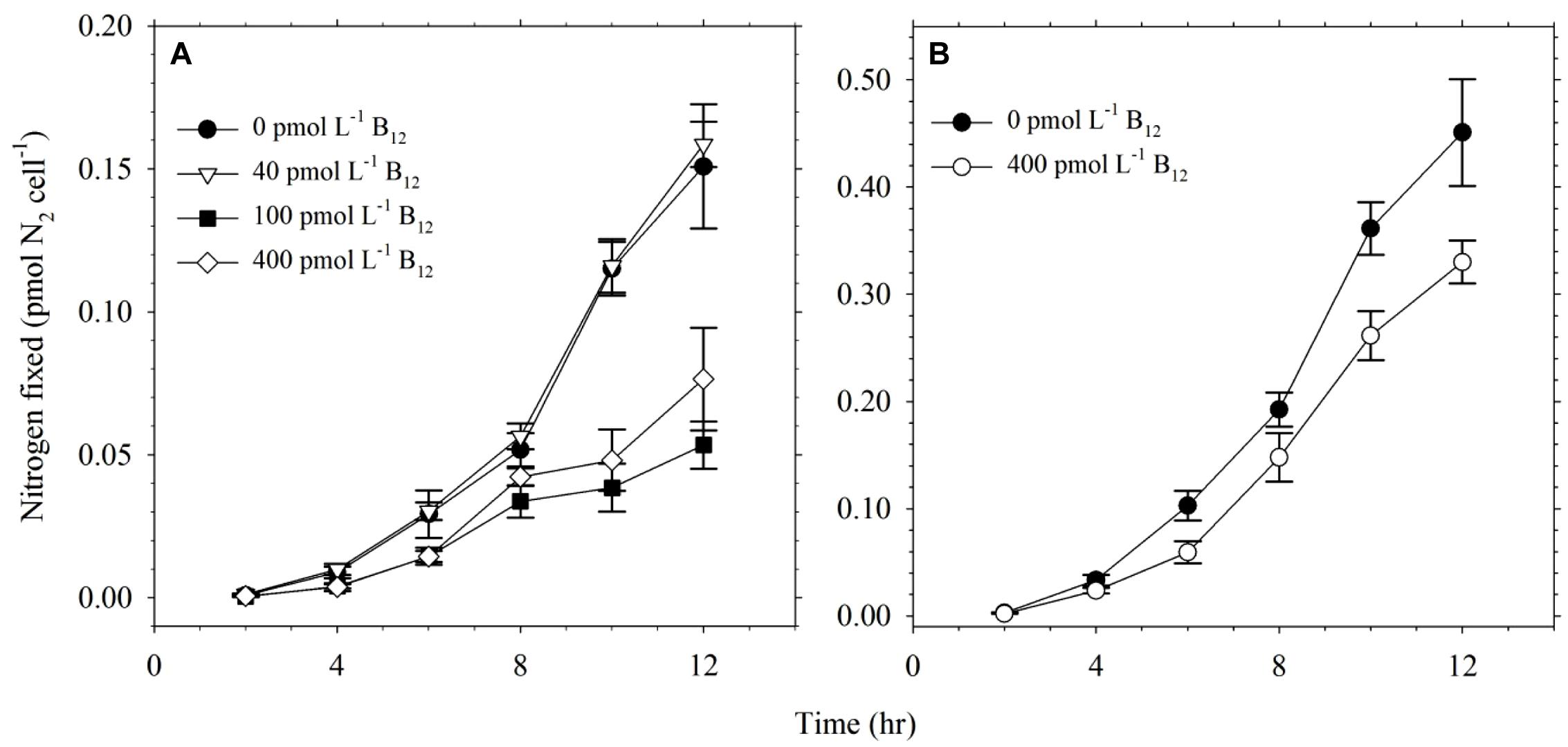
FIGURE 3. Nitrogen fixed by Trichodesmium grown in culture media with 20 pmol L-1 Co′ and different B12 concentrations. (A) Nitrogen levels fixed by Trichodesmium in media with 0, 40, 100, and 400 pmol L-1 B12. (B) Nitrogen levels fixed by Trichodesmium in media with 0 and 400 pmol L-1 B12 during the validation experiment. The higher values observed in the validation experiment was due to use of commercially available high purity acetylene as opposed to laboratory-prepared acetylene used in the original experiment. Nitrogen fixation was evaluated using acetylene reduction assay carried out during the 12 h light period while cells were in exponential phase of growth. Values represent mean and error bars represent SD of triplicate culture bottles.
Discussion
Trichodesmium is an important diazotroph but its Co and B12 requirements and their possible interaction have not been reported; thus we deemed it imperative to understand this link. In this study, we observed comparable growth rates and biomass of Trichodesmium in treatments with 20 pmol L-1 Co′ and varying B12 concentrations (Figure 1). This inorganic Co concentration is similar to the labile dissolved Co concentration (17.1 pmol L-1) that Panzeca et al. (2008) observed in one of their study areas in the North Atlantic Ocean. Dissolved Co levels in marine waters occur in low pmol L-1 levels reaching up to 1900 pmol L-1 in areas where there are anthropogenic sources, but Co is bound by strong ligands rendering it unavailable (Saito and Moffett, 2001; Panzeca et al., 2009). The intracellular Co quotas provide useful information to evaluate Co requirement and uptake by Trichodesmium (Table 1). The average Co uptake rates were estimated by multiplying specific growth rate with cellular concentration. In growth conditions with 20 pmol L-1 Co′, we observed that P-normalized Co quotas were comparable among different B12 treatments. These Co quotas were three orders of magnitude lower than Fe quotas measured in this study, which generally ranged from 15 to 20 mmol mol-1 P. The intracellular Co concentrations in culture medium volume were within 9 ± 2 pmol L-1 among all treatments and account for about half of Co′ concentrations initially added to the culture medium. The quotas normalized to cellular volume, ranged from 4.6 to 7.3 nmol L-1, indicating a concentration factor in the range 500–700-fold. These quotas support the notion that 20 pmol L-1 Co′ was sufficient for Trichodesmium to reach maximum growth under the culture conditions.
It has long been posited that Co may indirectly control phytoplankton growth and influence community structure because it is inherently needed in B12 synthesis (Panzeca et al., 2009; Bertrand et al., 2012; Sañudo-Wilhelmy et al., 2012). Results from our experiments denoted that B12 supply was not a major factor for Trichodesmium growth when ample Co was supplied although the growth rate observed in the 0 pmol L-1 B12 treatment were slightly different from the rates observed in the higher B12 treatments (P = 0.0213, Table 1). Our subsequent results, however, show that under Co′ values equal to or lower than 2 pmol L-1, B12 availability plays an important role in relieving stress due to Co limitation and it may augment the low Co supply (Figure 2). Growth of Trichodesmium in culture media with limiting Co′ values reveals that the Co conditions are not suitable to sustain growth rates that were observed in cultures with 20 pmol L-1 Co′. With sufficient Co, Trichodesmium achieved the highest biomass after only 15 days of incubation (Figure 1). At low Co conditions, however, some treatments only reached the equivalent biomass after more than 30 days of incubation (Figure 2). The first transfer with varying Co and B12 concentrations, inoculated using cells grown in media with 20 pmol L-1 Co′ and 400 pmol L-1 B12, show that Co′ values equal to or lower than 2 pmol L-1 were insufficient to support higher phytoplankton biomass if B12 was not added (Figures 2A–C). We monitored the cell growth starting from first transfer because prior experiments intended for acclimatization to varying Co and B12 conditions resulted in cell death, which is also evident in some treatments shown in Figure 2. The second transfer of Trichodesmium, inoculated from respective Co treatments and with 400 pmol L-1 B12 in the first transfer, revealed similar trends as in the first transfer (Figures 2D–F). This similarity indicates that the observed response may be attributed to the Co and B12 concentrations used. The stationary values or declining biomass observed in the treatments without B12 show that B12 supply was critical for Trichodesmium to grow at these Co′ concentrations. This was also directly validated by growth of the phytoplankton in respective Co treatments with B12. We speculate that B12 transformation, either intracellularly or extracellularly, provides extra bioavailable Co for Trichodesmium to recover its growth. Previous studies on Crocosphaera watsonii and Cyanothece show that these diazotrophs are able to recycle intracellular trace metals in molecules under stressed condition (e.g., Saito et al., 2011; Jacq et al., 2014; Rabouille et al., 2014). There is a possibility that Trichodesmium may be able to reuse Co by breaking down B12 to compensate for the low Co availability in the culture medium.
Our results clearly show that Trichodesmium has an absolute Co requirement and in Co-limiting conditions, this requirement can be partly met with sufficient B12 supply. So far, Co has been mainly studied in its role associated to the corrin ring of B12 but there are other Co-containing proteins like methionine aminopeptidase, prolidase, nitrile hydratase, glucose isomerase, methylmalonyl-CoA carboxytransferase, aldehyde decarbonylase, lysine-2,3-aminomutase, and bromoperoxidase (Kobayashi and Shimizu, 1999). These non-corrin Co-containing enzymes have been isolated and characterized but further research is needed to fully understand their biological functions. Aside from its role in the enzymes mentioned, Co may also substitute for Zn in carbonic anhydrase under Zn-limited conditions for some eukaryotic phytoplankton (Sunda and Huntsman, 1995). Moreover, a Co-containing alkaline phosphatase has been identified in bacteria isolated from a hot spring although alkaline phosphatase normally contains Zn as a metal cofactor (Gong et al., 2005). The role of Co in phosphorus acquisition has been demonstrated in a culture study by Jakuba et al. (2008) using the coccolithopore E. huxleyi and they found that low Co treatments resulted in low alkaline phosphatase activity. All treatments in our culture study were supplemented with 12.5 pmol L-1 Zn′ but the observed trends suggest that Co was not replaced with Zn. The existence of various enzymes that require Co warrants further studies to comprehensively understand biochemical processes involving Co and identify which of these may be important in Trichodesmium.
B12 and other B-vitamins may come from biological production and other sources including allochthonous sources, such as influxes from rivers and streams, and regenerated vitamins from phytoplankton cell death or lysis (Gobler et al., 2007). These sources lead to B12 concentrations that are higher in coastal waters than in the open ocean and which generally reflects abundance of total Co concentration (Panzeca et al., 2008). Low B12 concentrations are typical in the tropical and subtropical oceans characterized by intense light and high temperatures explaining the relatively short half-life of B12 in the field (Carlucci et al., 1969). The highest B12 concentration we used in this work (400 pmol L-1 B12) is approximately equivalent to the suggested B12 level in YBC-II medium widely used to cultivate Trichodesmium in the laboratory. Trichodesmium has been shown to harbor various bacteria especially when present in colonies (Sheridan et al., 2002; Roe et al., 2012). The strain used in this work is non-axenic, thus it may contain bacteria taken along with it since it was isolated and subsequently cultivated in the laboratory. Bacteria and other organisms associated with Trichodesmium are both particle attached or free-living and were found to be specific, indicating a certain degree of relationship between host and associated bacteria (Sheridan et al., 2002). B12 synthesis is a feature unique to some prokaryotic organisms and some of the associated bacteria in Trichodesmium may be capable of B12 synthesis, thus this association is possibly related to B12 cycling. The genome of Trichodesmium shows that it contains genes necessary for B12 synthesis (Sañudo-Wilhelmy et al., 2014). Therefore, it is imperative to take into account both diazotroph and associated organisms in Trichodesmium cultures when considering the results we observed. Our results show that growth of Trichodesmium in media with sufficient Co was independent of B12 concentration (Figure 1). B12 supply, however, was crucial for the growth of the diazotroph when Co was insufficient (Figure 2). These results demonstrate that Trichodesmium in cultures may either synthesize the B12 it requires or rely on associated bacteria to produce B12 for optimal growth. Whether it is Trichodesmium or its associated bacteria producing B12, the process certainly merits further study because this represents an additional source of the important organic growth factor in otherwise B12-limited environments.
B12 is a nitrogen-rich molecule with multiple uses in important biochemical processes. Thus we studied whether its availability will affect the nitrogen fixing capacity of Trichodesmium or not. We carefully prepared culture media for cultivating this diazotroph to ensure that nitrate concentration was kept at a minimum. The nitrate concentrations in the culture media were close to the detection limit, which is around 0.05 μmol L-1. Results from the acetylene reduction assay reveal that this diazotroph fixed more N in conditions without B12 or with lower B12 concentration (Figure 3A). The nitrogen levels fixed by cultures with higher B12 concentrations were comparable to values we obtained in our previous work (Rodriguez and Ho, 2014). Trends observed in the validation experiments ascertain our observation that B12 availability influences the nitrogen fixing capacity of Trichodesmium (Figure 3B). Levels of nitrogen fixed were lower in the first experiment due to use of laboratory-prepared acetylene as opposed to commercially available acetylene used in the validation experiment. It has been reported that use of high purity acetylene results in a more sensitive detection (Kitajima et al., 2009), which we have also observed in this work. Although the difference in purity of acetylene produced significant discrepancies in determined values, both experiments produced similar trends showing that lower B12 availability result in higher nitrogen fixation by the diazotroph. These results show that B12 availability affects nitrogen fixation up to a certain extent and this difference may be of significance in certain oceanic regions where B12 is depleted. The higher amounts of nitrogen fixed by cultures grown in low-B12 and without-B12 treatments indicate that B12 concentrations in the open ocean, which typically occurs in the range 0.3–60 pmol L-1 (Panzeca et al., 2009; Sañudo-Wilhelmy et al., 2012), may influence nitrogen fixation by this phytoplankton. Although it is tempting to propose that higher nitrogen fixation in treatments with low B12 was due to nitrogen requirement for B12 synthesis, the presence of 14 N atoms in a B12 molecule cannot quantitatively account for the observed difference. The role of B12 in nitrogen fixation by Trichodesmium requires further in-depth probing to understand its function in this diazotroph.
The observation that low B12 concentration enhances nitrogen fixation by Trichodesmium entails that it may play a crucial role in the oceanic regions where it thrives. A recent work surveying the B12 distributions in the open ocean found that B12 is severely depleted in oligotrophic areas forcing most phytoplankton to live under B12 deficiency (Sañudo-Wilhelmy et al., 2012). The presence of B12 producers in this environment that is also nitrogen-limited may therefore radically change predominant conditions. Trichodesmium and its associated bacteria can thrive in oceanic waters that have limited bioavailable nitrogen because the diazotroph can take advantage of the unlimited atmospheric N2 resource. Whether it is Trichodesmium or its associated bacteria producing B12, both can benefit from bioavailable nitrogen produced by the diazotroph. This is an inherent advantage over other B12 producers that are limited by bioavailable nitrogen supply as has been shown in the non-diazotrophic species Synecochoccus (Bonnet et al., 2010). The colimitation between B12 and nitrogen has been noted to impact phytoplankton group composition (Bertrand and Allen, 2012). These advantages further highlight the role of Trichodesmium in the dynamics of phytoplankton community structure and in global cycling of nutrients. The availability of Co, however, will dictate the growth and nitrogen fixation of Trichodesmium. While we cannot attribute the absolute Co requirement to a specific biochemical demand, it is clear from our results that Trichodesmium requires Co for its growth. Because of the importance of Trichodesmium in oligotrophic ocean, Co availability can therefore impact primary production and nutrient cycling in these regions.
Conflict of Interest Statement
The authors declare that the research was conducted in the absence of any commercial or financial relationships that could be construed as a potential conflict of interest.
Acknowledgments
The authors are grateful to William Sunda for helpful discussions and Mei-Chen Lu and Chih-Ping Lee for technical support. This work was supported financially by Taiwan National Science Council through grants 101-2611-M-001-002 and 102-2611-M-001-004-MY3, and by Academia Sinica through the grant titled “Ocean Acidification: Comparative biogeochemistry in shallow water tropical coral reef ecosystems in a naturally acidic marine environment.”
References
Bertrand, E. M., and Allen, A. E. (2012). Influence of vitamin B auxotrophy on nitrogen metabolism in eukaryotic phytoplankton. Front. Microbiol. 3:375. doi: 10.3389/fmicb.2012.00375
Bertrand, E. M., Allen, A. E., Dupont, C. L., Norden-Krichmar, T. M., Bai, J., Valas, R. E., et al. (2012). Influence of cobalamin scarcity on diatom molecular physiology and identification of a cobalamin acquisition protein. Proc. Natl. Acad. Sci. U.S.A. 109, E1762–E1771. doi: 10.1073/pnas.1201731109
Bertrand, E. M., Saito, M. A., Rose, J. M., Riesselman, C. R., Lohan, M. C., Noble, A., et al. (2007). Vitamin B12 and iron colimitation of phytoplankton growth in the Ross Sea. Limnol. Oceanogr. 52, 1079–1093. doi: 10.4319/lo.2007.52.3.1079
Bonnet, S., Webb, E. A., Panzeca, C., Karl, D. M., Capone, D. G., and Sañudo-Wilhelmy, S. A. (2010). Vitamin B12 excretion by cultures of the marine cyanobacteria Crocosphaera and Synechococcus. Limnol. Oceanogr. 55, 1959–1964. doi: 10.4319/lo.2010.55.5.1959
Breitbarth, E., Mills, M. M., Friedrichs, G., and Laroche, J. (2004). The Bunsen gas solubility coefficient of ethylene as a function of temperature and salinity and its importance for nitrogen fixation assays. Limnol. Oceanogr. Methods 2, 282–288. doi: 10.4319/lom.2004.2.282
Capone, D. G., and Montoya, J. P. (2001). “Nitrogen fixation and denitrification,” in Method in Microbiology, Marine Microbiology, ed. J. Paul (Dordrecht: Kluwer Academic), 501–515.
Capone, D. G., Zehr, J. P., Paerl, H. W., Bergman, B., and Carpenter, E. J. (1997). Trichodesmium, a globally significant marine cyanobacterium. Science 276, 1221–1229. doi: 10.1126/science.276.5316.1221
Carlucci, A. F., Silbernagel, S. B., and Mcnally, P. M. (1969). Influence of temperature and solar radiation on persistence of vitamin B12, thiamine, and biotin in seawater. J. Phycol. 5, 302–305. doi: 10.1111/j.1529-8817.1969.tb02618.x
Carpenter, E. J., Subramaniam, A., and Capone, D. G. (2004). Biomass and primary productivity of the cyanobacterium, Trichodesmium sp. in the southwestern tropical N Atlantic Ocean. Deep Sea Res. 51, 173–203. doi: 10.1016/j.dsr.2003.10.006
Chen, Y. B., Zehr, J. P., and Mellon, M. (1996). Growth and nitrogen fixation of the diazotrophic filamentous nonheterocystous cyanobacterium Trichodesmium sp. IMS 101 in defined media: evidence for a circadian rhythm. J. Phycol. 32, 916–923. doi: 10.1111/j.0022-3646.1996.00916.x
Croft, M. T., Lawrence, A. D., Raux-Deery, E., Warren, M. J., and Smith, A. G. (2005). Algae acquire vitamin B12 through a symbiotic relationship with bacteria. Nature 438, 90–93. doi: 10.1038/nature04056
Gobler, C. J., Norman, C., Panzeca, C., Taylor, G. T., and Sañudo-Wilhelmy, S. A. (2007). Effect of B-vitamins (B1, B12) and inorganic nutrients on algal bloom dynamics in a coastal ecosystem. Aquat. Microb. Ecol. 49, 181–194. doi: 10.3354/ame01132
Goebel, N. L., Edwards, C. A., Carter, B. J., Achilles, K. M., and Zehr, J. P. (2008). Growth and carbon content of three different-sized diazotrophic cyanobacteria observed in the subtropical North Pacific. J. Phycol. 44, 1212–1220. doi: 10.1111/j.1529-8817.2008.00581.x
Gong, N., Chen, C., Xie, L., Chen, H., Lin, X., and Zhang, R. (2005), Characterization of a thermostable alkaline phosphatase from a novel species Thermus yunnanensis sp. nov. and investigation of its cobalt activation at high temperature. Biochim. Biophys. Acta 1750, 103–111. doi: 10.1016/j.bbapap.2005.05.007
Ho, T.-Y. (2013). Nickel limitation of nitrogen fixation in Trichodesmium. Limnol. Oceanogr. 58, 112–120. doi: 10.4319/lo.2013.58.1.0112
Ho, T.-Y., Chien, C. T., Wang, B. N., and Siriraks, A. (2010). Determination of trace metals in seawater by an automated flow injection ion chromatograph pretreatment system with ICPMS. Talanta 82, 1478–1484. doi: 10.1016/j.talanta.2010.07.022
Ho, T.-Y., Chu, T.-H., and Hu, C.-L. (2013). Interrelated influence of light and Ni on Trichodesmium growth. Front. Microbiol. 4:139. doi: 10.3389/fmicb.2013.00139
Jacq, V., Ridame, C., L’Helguen, S., Kaczmar, F., and Saliot, A. (2014). Response of the unicellular diazotrophic cyanobacterium Crocosphaera watsonii to iron limitation. PLoS ONE 9:e86749. doi: 10.1371/journal.pone.0086749
Jakuba, R. W., Moffett, J. W., and Dyhrman, S. T. (2008). Evidence for the linked biogeochemical cycling of zinc, cobalt, and phosphorus in the western North Atlantic Ocean. Global Biogeochem. Cycles 22, GB4012. doi: 10.1029/2007GB003119
Kitajima, S., Furuya, K., Hashihama, F., and Takeda, S. (2009). Latitudinal distribution of diazotrophs and their nitrogen fixation in the tropical and subtropical western North Pacific. Limnol. Oceanogr. 54, 537–547. doi: 10.4319/lo.2009.54.2.0537
Kobayashi, M., and Shimizu, S. (1999). Cobalt proteins. Eur. J. Biochem. 261, 1–9. doi: 10.1046/j.1432-1327.1999.00186.x
Koch, F., Marcoval, M. A., Panzeca, C., Bruland, K. W., Sañudo-Wilhelmy, S. A., and Gobler, C. J. (2011). The effect of vitamin B12 on phytoplankton growth and community structure in the Gulf of Alaska. Limnol. Oceanogr. 56, 1023–1034. doi: 10.4319/lo.2011.56.3.1023
Letelier, R. M., and Karl, D. M. (1998). Trichodesmium sp. physiology and nutrient fluxes in the North Pacific subtropical gyre. Aquat. Microb. Ecol. 15, 265–276. doi: 10.3354/ame015265
Martens, J. H., Barg, H., Warren, M. J., and Jahn, D. (2002). Microbial production of vitamin B12. Appl. Microbiol. Biotechnol. 58, 275–285. doi: 10.1007/s00253-001-0902-7
Morel, F. M. M., and Price, N. M. (2003). The biogeochemical cycles of trace metals in the oceans. Science 300, 944–947. doi: 10.1126/science.1083545
Noble, A. E., Lamborg, C. H., Ohnemus, D. C., Lam, P. J., Goepfert, T. J., Measures, C. I., et al. (2012). Basin-scale inputs of cobalt, iron, and manganese from the Benguela-Angola front to the South Atlantic Ocean. Limnol. Oceanogr. 57, 989–1010. doi: 10.4319/lo.2012.57.4.0989
Panzeca, C., Beck, A. J., Leblanc, K., Taylor, G. T., Hutchins, D. A., and Sañudo-Wilhelmy, S. A. (2008). Potential cobalt limitation of vitamin B12 synthesis in the North Atlantic Ocean. Global Biogeochem. Cycles 22, GB2029. doi: 10.1029/2007GB003124
Panzeca, C., Beck, A. J., Tovar-Sanchez, A., Segovia-Zavala, J., Taylor, G. T., Gobler, C. J., et al. (2009). Distributions of dissolved vitamin B12 and Co in coastal and open-ocean environments. Estuar. Coast. Mar. Sci. 85, 223–230. doi: 10.1016/j.ecss.2009.08.016
Rabouille, S., Van de Waal, D., Matthijs, H. C. P., and Huisman, J. (2014). Nitrogen fixation and respiratory electron transport in the cyanobacterium Cyanothece under different light/dark cycles. FEMS Microbiol. Ecol. 87, 630–638. doi: 10.1111/1574-6941.12251
Raux, E., Schubert, H. L., and Warren, M. J. (2000). Biosynthesis of cobalamin (vitamin B12): a bacterial conundrum. Cell. Mol. Life Sci. 57, 1880–1893. doi: 10.1007/PL00000670
Rodriguez, I. B., and Ho, T.-Y. (2014). Diel nitrogen fixation pattern of Trichodesmium: the interactive control of light and Ni. Sci. Rep. 4, 4445. doi: 10.1038/srep04445
Roe, K. L., Barbeau, K., Mann, E. L., and Haygood, M. G. (2012). Acquisition of iron by Trichodesmium and associated bacteria in culture. Environ. Microbiol. 14, 1681–1695. doi: 10.1111/j.1462-2920.2011.02653.x
Saito, M. A., Bertrand, E. M., Dutkiewicz, S., Bulygin, V. V., Moran, D. M., Monteiro, F. M., et al. (2011). Iron conservation strategies by reduction of metal enzymes inventories in the marine diazotroph Crocosphaera watsonii. Proc. Natl. Acad. Sci. U.S.A. 108, 2184–2189. doi: 10.1073/pnas.1006943108
Saito, M. A., Goepfert, T. J., Noble, A. E., Bertrand, E. M., Sedwick, P. N., and DiTullio, G. R. (2010). A seasonal study of dissolved cobalt in the Ross Sea, Antarctica: micronutrient behavior, absence of scavenging, and relationships with Zn, Cd and P. Biogeosciences 7, 4059–4082. doi: 10.5194/bg-7-4059-2010
Saito, M. A., and Moffett, J. W. (2001). Complexation of cobalt by natural organic ligands in the Sargasso Sea as determined by a new high-sensitivity electrochemical cobalt speciation method suitable for open ocean work. Mar. Chem. 75, 49–68. doi: 10.1016/S0304-4203(01)00025-1
Saito, M. A., and Moffett, J. W. (2002). Temporal and spatial variability of cobalt in the Atlantic Ocean. Geochim. Cosmochim. Ac. 66, 1943–1953. doi: 10.1016/S0016-7037(02)00829-3
Saito, M. A., Moffett, J. W., Chisholm, S. W., and Waterbury, J. B. (2002). Cobalt limitation and uptake in Prochlorococcus. Limnol. Oceanogr. 47, 1629–1636. doi: 10.4319/lo.2002.47.6.1629
Saito, M. A., Moffett, J. W., and Ditullio, G. R. (2004). Cobalt and nickel in the Peru upwelling region: a major flux of labile cobalt utilized as a micronutrient. Global Biogeochem. Cycles 18, GB4030. doi: 10.1029/2003GB002216
Saito, M. A., Rocap, G., and Moffett, J. W. (2005). Production of cobalt binding ligands in a Synechococcus feature at the Costa Rica upwelling dome. Limnol. Oceanogr. 50, 279–290. doi: 10.4319/lo.2005.50.1.0279
Sañudo-Wilhelmy, S. A., Cutter, L. S., Durazo, R., Smail, E. A., Gomez-Consarnau, L., Webb, E. A., et al. (2012). Multiple B-vitamin depletion in large areas of the coastal ocean. Proc. Natl. Acad. Sci. U.S.A. 109, 14041–14045. doi: 10.1073/pnas.1208755109
Sañudo-Wilhelmy, S. A., Gomez-Consarnau, L., Suffridge, C., and Webb, E. A. (2014). The role of B vitamins in marine biogeochemistry. Annu. Rev. Mar. Sci. 6, 339–367. doi: 10.1146/annurev-marine-120710-100912
Sheridan, C. C., Steinberg, D. K., and Kling, G. W. (2002). The microbial and metazoan community associated with colonies of Trichodesmium sp.: a quantitative survey. J. Plankton Res. 24, 913–922. doi: 10.1093/plankt/24.9.913
Sunda, W. G. (2012). Feedback interactions between trace metal nutrients and phytoplankton in the ocean. Front. Microbiol. 3:204. doi: 10.3389/fmicb.2012.00204
Sunda, W. G., and Huntsman, S. A. (1995). Cobalt and zinc interreplacement in marine phytoplankton: biological and geochemical implications. Limnol. Oceanogr. 40, 1404–1417. doi: 10.4319/lo.1995.40.8.1404
Swanner, E. D., Planavsky, N. J., Lalonde, S. V., Robbins, L. J., Bekker, A., Rouxel, O. J., et al. (2014). Cobalt and marine redox evolution. Earth Planet. Sci. Lett. 390, 253–263. doi: 10.1016/j.epsl.2014.01.001
Warren, M. J., Raux, E., Schubert, H. L., and Escalante-Semerena, J. C. (2002). The biosynthesis of adenosylcobalamin (vitamin B12). Nat. Prod. Rep. 19, 390–412. doi: 10.1039/b108967f
Westall, J. C., Zachary, J. L., and Morel, F. M. M. (1976). A Computer Program for the Calculation of Chemical Equilibrium Composition of Aqueous Systems, Civil Eng. Tech. Note 18. Cambridge: Massachusetts Institute of Technology.
Keywords: B12, cyanobacteria, cobalt, nitrogen fixation, trace metal limitation, Trichodesmium
Citation: Rodriguez IB and Ho T-Y (2015) Influence of Co and B12 on the growth and nitrogen fixation of Trichodesmium. Front. Microbiol. 6:623. doi: 10.3389/fmicb.2015.00623
Received: 17 February 2015; Accepted: 08 June 2015;
Published: 18 June 2015
Edited by:
George S. Bullerjahn, Bowling Green State University, USAReviewed by:
Nico Salmaso, Research and Innovation Centre Fondazione Mach – Istituto Agrario di S. Michele all’Adige, ItalySophie Rabouille, Centre National de la Recherche Scientifique, France
Copyright © 2015 Rodriguez and Ho. This is an open-access article distributed under the terms of the Creative Commons Attribution License (CC BY). The use, distribution or reproduction in other forums is permitted, provided the original author(s) or licensor are credited and that the original publication in this journal is cited, in accordance with accepted academic practice. No use, distribution or reproduction is permitted which does not comply with these terms.
*Correspondence: Tung-Yuan Ho, Research Center for Environmental Changes – Academia Sinica, 128, Section 2, Academia Road, Nankang, Taipei 11529, Taiwan,dHlob0BnYXRlLnNpbmljYS5lZHUudHc=