- 1School of Agriculture, Meiji University, Kawasaki, Japan
- 2RIKEN, Center for Sustainable Resource Science, Yokohama, Japan
- 3Department of Chemical Science and Engineering, Graduate School of Engineering, Kobe University, Kobe, Japan
The study of the primary metabolism of cyanobacteria in response to light conditions is important for environmental biology because cyanobacteria are widely distributed among various ecological niches. Cyanobacteria uniquely possess circadian rhythms, with central oscillators consisting from three proteins, KaiA, KaiB, and KaiC. The two-component histidine kinase SasA/Hik8 and response regulator RpaA transduce the circadian signal from KaiABC to control gene expression. Here, we generated a strain overexpressing rpaA in a unicellular cyanobacterium Synechocystis sp. PCC 6803. The rpaA-overexpressing strain showed pleiotropic phenotypes, including slower growth, aberrant degradation of an RNA polymerase sigma factor SigE after the light-to-dark transition, and higher accumulation of sugar catabolic enzyme transcripts under dark conditions. Metabolome analysis revealed delayed glycogen degradation, decreased sugar phosphates and organic acids in the tricarboxylic acid cycle, and increased amino acids under dark conditions. The current results demonstrate that in this cyanobacterium, RpaA is a regulator of primary metabolism and involved in adaptation to changes in light conditions.
Introduction
Cyanobacteria are organisms performing oxygenic photosynthesis that exist in various environmental niches such as fresh water, seawater, soil, and hot springs. Studying the regulatory mechanism of cyanobacterial metabolism is important in environmental biology and biotechnology. One of the most widely studied cyanobacteria is the non-nitrogen fixing species Synechocystis sp. PCC 6803 (hereafter Synechocystis 6803). Synechocystis 6803 cells grow fast and are naturally transformable with homologous recombination (Berla et al., 2013).
Cyanobacteria have a circadian rhythm and their central oscillator consists of three proteins KaiABC, first found in the unicellular cyanobacterium Synechococcus sp. PCC 7942 (hereafter Synechococcus 7942; Ishiura et al., 1998). An important issue for study in the circadian system is metabolic compensation, which is the persistence and entrainment of circadian rhythms in response to various nutrient conditions (Johnson and Egli, 2014). KaiC is an enzyme that phosphorylates and dephosphorylates its own residues in a strict order (Nishiwaki et al., 2007; Rust et al., 2007). ATP is a substrate of KaiC phosphorylation, and the ATP/ADP ratio is important for entrainment of the circadian clocks (Rust et al., 2011). KaiC phosphorylation is inhibited by ADP and integrates metabolic information into KaiC through the kinase-stimulation activity of KaiA (Rust et al., 2011). ATP is decreased under dark conditions and treatment with a dark pulse leads to a phase shift in the circadian clock (Rust et al., 2011). Oxidized quinones also input signals into the circadian clock in Synechococcus 7942 via the inhibition of KaiC phosphorylation (Kim et al., 2012). Thus, information on the availability of biochemical energy and light/dark conditions is transduced into circadian clocks. Glycogen metabolism entrains the circadian oscillator by providing ATP, and the mutants deficient in glycogen synthesis show the phenotype of a circadian clock that is hypersensitive to dark pulses (Pattanayak et al., 2014).
SasA is a histidine kinase interacting with KaiC in Synechococcus 7942 (Iwasaki et al., 2000). SasA is autophosphorylated by its histidine residue and the phosphate moiety is transferred to the cognate response regulator RpaA (Takai et al., 2006). The gene rpaA is the “regulator of phycobilisome association,” whose protein is involved in energy transfer from phycobilisome to Photosystem I (Ashby and Mullineaux, 1999). The phosphorelay from SasA to RpaA is enhanced in the presence of KaiC (Takai et al., 2006). The knockout mutants of sasA or rpaA in Synechococcus 7942 grow normally under continuous light conditions, but the growth is severely retarded under light/dark cycle conditions (Takai et al., 2006). The knockout of rpaA alters the gene expression in Synechococcus 7942 widely; the genes, whose peaks of the expression are subjective dusk or dawn, are down-regulated or up-regulated, respectively, in the rpaA mutant (Markson et al., 2013). Chromatin-immunoprecipitation with high throughput sequencing (ChIP-Seq) analysis reveals 110 binding sites in the Synechococcus 7942 genome, which has A/T-rich motif included in the promoters of kaiBC and rpoD6 (encoding an RNA polymerase sigma factor). In vitro assay demonstrates that phosphorylated RpaA binds with the promoter regions, while non-phosphorylated RpaA does not (Hanaoka et al., 2012; Markson et al., 2013).
The mechanistic implications of a circadian clock in Synechocystis 6803 have remained obscure due to the redundancy of kaiABC genes, with one kaiA, three kaiB (kaiB1–B3), and three kaiC (kaiC1–3; Kanesaki et al., 2012). The kaiAB1C1 and kaiC2B2 genes constitute an operon in the Synechocystis 6803 genome. KaiC1 phosphorylation is dependent on KaiA and KaiC directly interacting with KaiA (Wiegard et al., 2013). The phosphorylation of KaiC2 and KaiC3 is not dependent on KaiA, and therefore KaiAB1C1 proteins seem to be the central oscillator in Synechocystis 6803 (Wiegard et al., 2013). Synechocystis 6803 contains SasA (Hik8, sll0750) and RpaA (Rre31, slr0115) orthologs. Hik8 interacts with KaiC1, but not KaiC2 in vivo (Osanai et al., 2015). The knockout of hik8 results in pleiotropic phenotypes, with the gene expression of enzymes in the glycogen catabolism, glycolysis, and the oxidative pentose phosphate (OPP) pathway altered (Singh and Sherman, 2005). The hik8 overexpression also leads to changes in primary metabolism (Osanai et al., 2015). Glycogen and sugar phosphate levels are decreased under light conditions and amino acid levels such as glycine and lysine are increased by hik8 overexpression (Osanai et al., 2015). The involvement of RpaA in salt and hyperosmotic stress has been shown by microarray, with the knockout of rpaA/rre31 down-regulating the salt-induced gene expression (Shoumskaya et al., 2005). Nevertheless, in these studies, the involvement of RpaA in the regulation of primary metabolism and the effect of rpaA modification on metabolic alteration has remained unclear due to lack of metabolome data.
Here, we generated a Synechocystis 6803 strain overexpressing rpaA. The rpaA-overexpressing strain showed pleiotropic and similar phenotypes with the hik8-overexpressing strain. Genetic and metabolomic analyses indicate that RpaA plays pivotal roles in metabolic regulation under both light and dark conditions.
Materials and Methods
Bacterial Strains and Culture Conditions
The glucose-tolerant (GT) strain of Synechocystis sp. PCC 6803, isolated by Williams (1988), and the rpaA-overexpressing strain, designated as ROX310, were grown in modified BG-11 medium, which consisted of BG-110 liquid medium (Rippka, 1988) containing 5 mM NH4Cl (buffered with 20 mM HEPES-KOH, pH 7.8). Among GT substrains, the GT-I strain was used in this study (Kanesaki et al., 2012). Liquid cultures were bubbled with 1% (v/v) CO2 in air and incubated at 30°C under continuous white light (ca. 50–70 μmol photons m-2 s-1). Growth and cell densities were measured at OD730 with a Hitachi U-3310 spectrophotometer (Hitachi High-Tech., Tokyo, Japan). Kanamycin (10 μg/mL) was added to ROX310 during pre-culture.
Construction of Plasmids for rpaA Overexpression
A region of the Synechocystis 6803 genome encoding the rpaA (slr0115, rre31) ORF was amplified by PCR using KOD Plus Neo polymerase (Toyobo, Osaka, Japan) and the specific primers 5′-GAATTATAACCATATGCCTCGAATACTGATC-3′ (forward) and 5′-ATCCAATGTGAGGTTAACCTACGTTGGACTACCGCC-3′ (reverse). The amplified PCR fragment was inserted into the NdeI-HpaI sites of the pTKP2031V vector, using an In-Fusion HD cloning kit (Takara Bio, Shiga, Japan). The resultant plasmid was confirmed by sequencing and transformed into GT-I as described previously (Osanai et al., 2011).
Construction of Plasmids for Protease-Knockout Mutants
To construct protease-knockout mutants, the coding regions of proteases were amplified by PCR with KOD Plus Neo and the following primer sets: clpB1(slr1641) 5′-GGGAATTCTGCGGGATCGCAAACTA-3′ (forward) and 5′-GCGCATGCGAGCGTTGAATGGCTTCG-3′ (reverse); clpB2 (slr0156) 5′-GGGAATTCTCCGCGCGTTTAACCTTG-3′ (forward) and 5′-GCGCATGCCCCGCTCAGCTTTTTCT-3′ (reverse); clpC(sll0020) 5′-GGGAATTCGCTTCCTGCCCGATAAG-3′ (forward) and 5′-GCGCATGCCACGTCTTCCAACAGGC-3′ (reverse); clpX(sll0535) 5′-GGGAATTCGAAGGAACGGTGGCCAA-3′ (forward) and 5′-GCGCATGCCCGTCGTTGTCCAACCA-3′ (reverse); degP(slr1204) 5′-GGGAATTCGTGCTGGGGGGACATTT-3′ (forward) and 5′-GCGCATGCCAGTTTGCCCACTAGGG-3′ (reverse); degQ(sll1679) 5′-GGGAATTCCTTGGTTACGCCGCATC-3′ (forward) and 5′-GCGCATGCACTATGCGCTGTAGGCG-3′ (reverse); and degS(sll1427) 5′-GGGAATTCGTGGCCGTGCTTTTACT-3′ (forward) and 5′-GCGCATGCCTTCCACCCGTTCTTGA-3′ (reverse). PCR fragments were isolated with the Wizard SV Gel and PCR Clean-up System (Promega, Madison, WI, USA) and the fragments were digested with EcoRI and SphI. Each of the resulting fragments was cloned into pUC119 (Clontech) digested with EcoRI and SphI. The chloramphenicol-resistant cassette obtained by digesting pKRP10 (Reece and Phillips, 1995) with SmaI or PstI was inserted into the HincII sites of clpB1 and clpC, the SmaI sites of clpB2 and clpX, and the PstI sites of degP, degQ, and degS. The resultant vectors were transformed into GT. The chloramphenicol-resistant (20 μg/mL) cells were isolated and streaked on plates several times.
Immunoblotting
Cells grown under light or dark conditions were collected by centrifugation (5,800 × g for 2 min), and the supernatant was removed by pipetting. The cells were frozen by liquid nitrogen. Cells were dissolved in PBS-T (137 mM NaCl, 2.7 mM KCl, 8.1 mM Na2HPO4, 1.5 mM KH2PO4, 0.1% Tween-20) and disruption by sonication and immunoblotting was performed as described previously (Osanai et al., 2014a). Antisera against SigE and GlgP(sll1356) were generated previously (Osanai et al., 2009, 2011).
RNA Isolation and Quantitative Real-Time PCR
RNA isolation and cDNA synthesis were performed as described previously (Osanai et al., 2014b). The cDNAs were synthesized with the SuperScript III First-Strand Synthesis System (Life Technologies Japan, Tokyo, Japan) with 2 μg of total RNA. Quantitative real-time PCR was performed with the StepOnePlus Real-Time PCR System (Life Technologies) according to the manufacturer’s instructions, using the primers listed in Supplementary Table S2. The transcript level of rnpB, which encodes RNaseP subunit B, was used as an internal standard as previously described (Osanai et al., 2015).
Glycogen Measurement
Glycogen levels were measured at the Biotechnology Center of Akita Prefectural University (Akita, Japan), as described previously (Osanai et al., 2014b).
LC–MS/MS Analysis
Equal amounts of cells (10 mL of cell culture with OD730 = 1.0) were harvested by rapid filtration. LC–MS/MS analysis was performed using a 100-mL aliquot of the upper phase as previously described (Osanai et al., 2014b). All metabolite analyses were performed with the cells grown without external carbon sources except CO2.
Amino Acid Analysis by GC–MS
Equal amounts of cells (50 mL of cell culture with OD730 = 1.0) were harvested by rapid filtration. Amino acids were quantified by GC-MS as previously described (Osanai et al., 2014a).
Organic Acid Analysis by GC–MS
Equal amounts of cells (10 mL of cell culture with OD730 = 1.0) were harvested by rapid filtration using a previously described method (Osanai et al., 2014b). GC-MS was carried out using a GCMS-QP2010 Ultra equipped with a CP-Sil 8 CB-MS capillary column (30 m × 0.25 mm × 0.25 μm; Agilent, Palo Alto, CA, USA) as previously described (Osanai et al., 2014b).
Results
Slower Growth of rpaA-Overexpressing Strain
We generated a strain overexpressing rpaA by fusing the promoter of psbAII (encoding Photosystem II D1 protein; Figure 1A) and the strain was named ROX310. Quantitative real-time PCR confirmed that the expression levels of rpaA in ROX310 were higher than in the wild-type, glucose-tolerant (GT) strain under both light and dark conditions (Figure 1B). Under both photoautotrophic and photomixotrophic conditions, the rpaA-overexpressing strain grew more slowly than did GT (Figure 1C). ROX310 grew similarly to GT under light-activated heterotrophic growth (LAHG) conditions until 1 day, although it lost viability under prolonged LAHG conditions (Figure 1C).
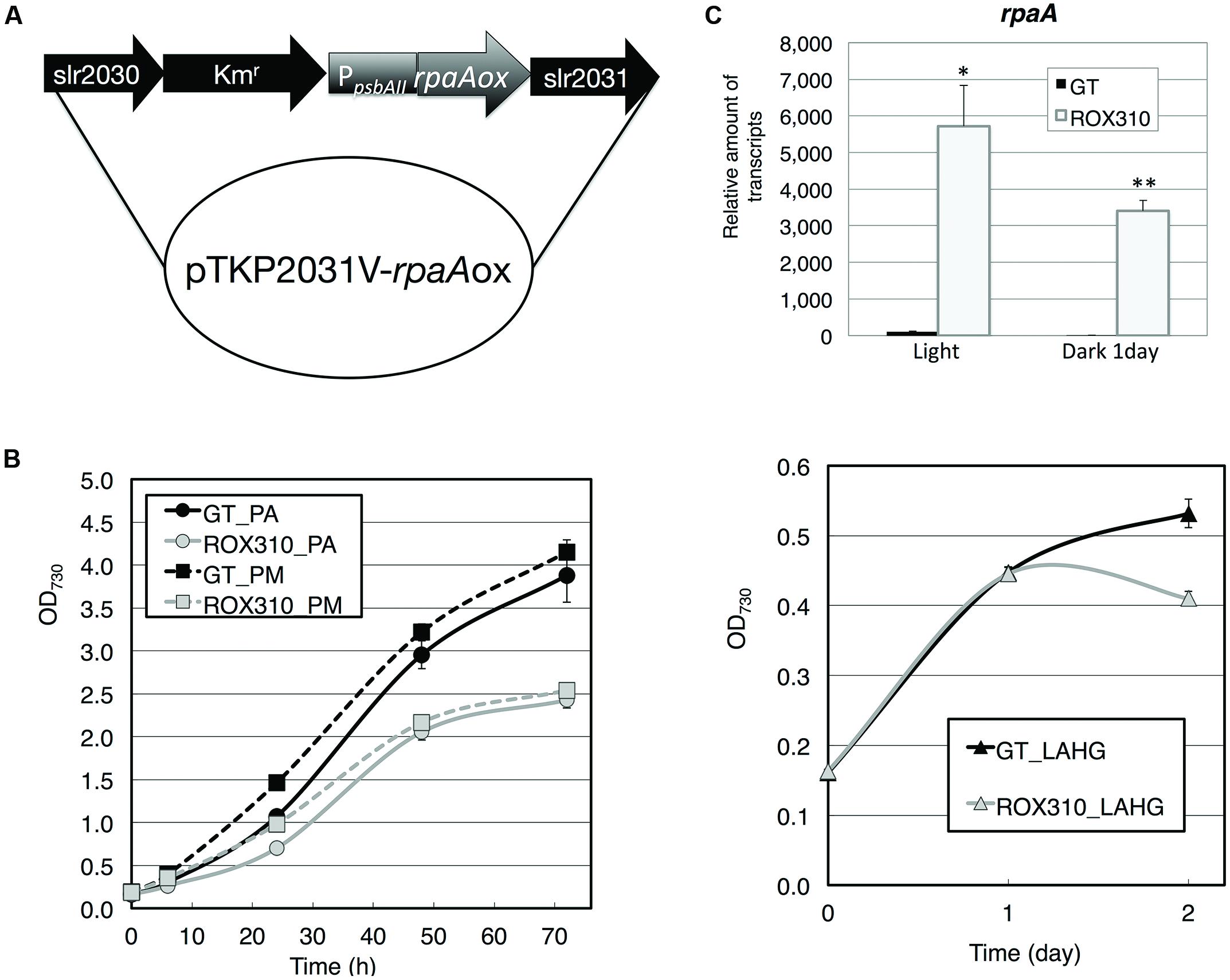
FIGURE 1. (A) Plasmid for rpaA overexpression in Synechocystis 6803. The kanamycin resistance cassette was located upstream of the psbAII promoter. (B) Levels of rpaA transcripts in GT and ROX310 cells. Quantitative real-time PCR was performed with total RNA from cells grown under light and dark conditions. (C) Growth of GT and ROX310 cells. Left: growth of cells under photoautotrophic (PA) and photomixotrophic (PM, grown with 1 mM glucose) conditions. Right: growth of cells under light-activated heterotrophic growth conditions (LAHG, grown with 1 mM glucose under continuous dark conditions except for 15 min light per day).
Altered Protein and Transcript Levels of SigE in ROX310
Previously, the hik8-overexpressing strain showed aberrant protein degradation of SigE, RNA polymerase sigma factors activating sugar catabolism that is essential for dark/heterotrophic conditions, after the light-to-dark transition (Osanai et al., 2015). Immunoblotting demonstrated that rpaA overexpression reduced the degradation of SigE proteins under dark conditions (Figure 2A). To identify the proteases of SigE, we tested seven mutants lacking orthologous proteinases related to dark conditions in Synechococcus 7942 (Hosokawa et al., 2011), but these knockouts did not affect SigE protein levels (Figure 2A). The level of sigE transcripts was higher in ROX310 than in GT under both light and dark conditions, although the levels were similarly decreased in both strains by the light-to-dark transition (Figure 2B). We then quantified the levels of sugar catabolic enzymes by immunoblotting. The protein levels of GlgP(sll1356), one of two glycogen phosphorylases involved in glycogen degradation in Synechocystis 6803, were not induced by the rpaA-overexpressing strain under dark conditions (Figure 2C).
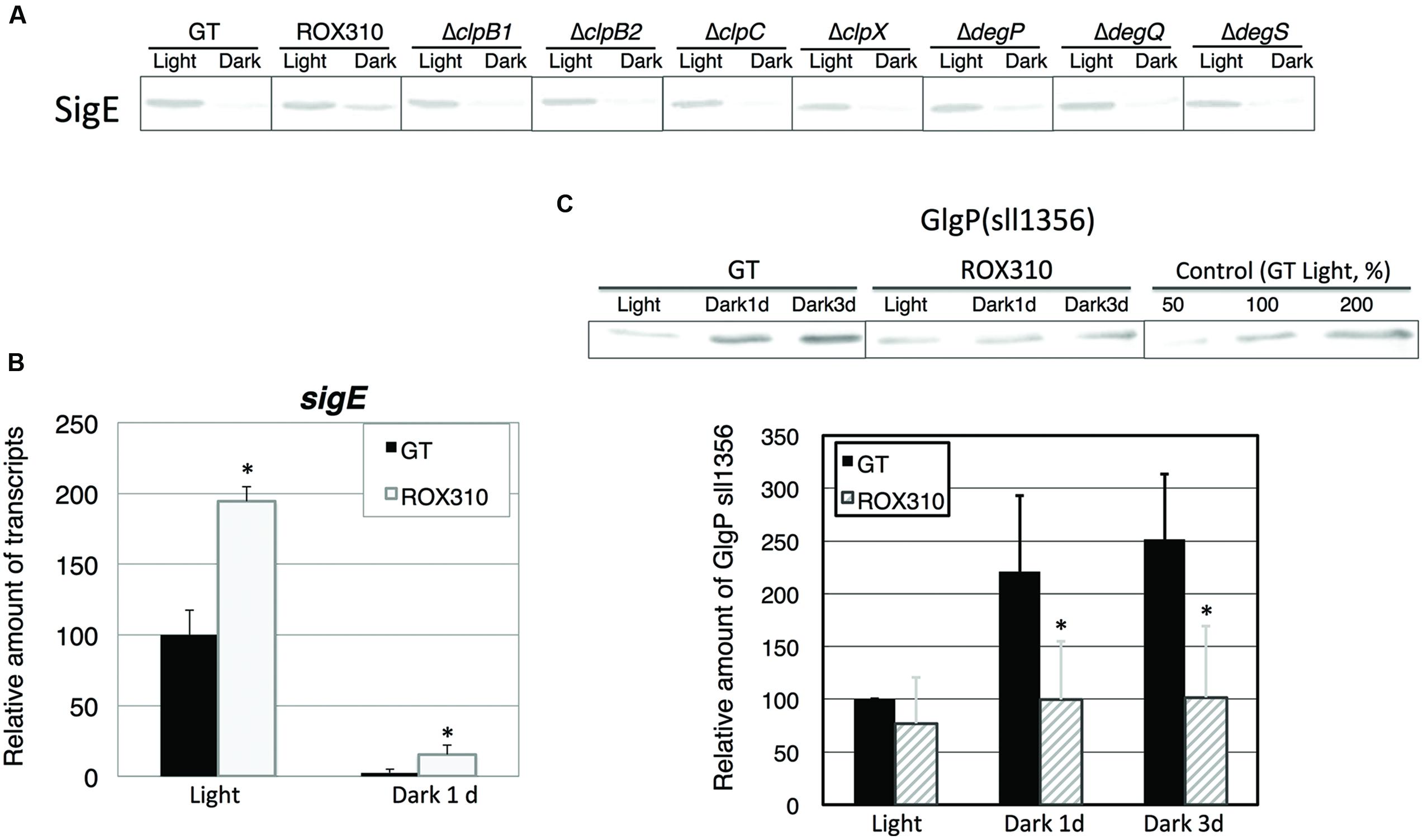
FIGURE 2. (A) Protein levels of SigE in GT, ROX310, and protease-knockout mutants. Immunoblotting was performed with 12 mg of total protein from cells grown under light and dark conditions (1 day). (B) Transcript levels of sigE in GT and ROX310. Quantitative real-time PCR was performed with total RNA from cells grown under light and dark conditions (1 day). Data represent mean ± SD from three independent experiments. (Student’s t-test; *P < 0.05). (C) Protein levels of GlgP(sll1356), one of two glycogen phosphorylases, under light and dark conditions. Immunoblotting was performed with 14 mg of total protein from cells grown under light and dark conditions (1 or 3 days). Protein levels were calibrated relative to that of the corresponding protein in GT (set at 100%). Data represent means ± SD from three independent experiments. Asterisks indicate statistically significant differences between GT and ROX310 (Student’s t-test; *P < 0.05).
We measured the transcript levels of genes related to sugar catabolism in ROX310 (Figure 3). The transcript levels of pfkA(sll0765) and fbaII were enhanced by rpaA overexpression under light conditions, while that of pfkA(sll1196) was repressed (Figure 3). The transcript levels of all 12 genes were decreased at 1 day after the light-to-dark transition in both GT and ROX310; however, there were higher transcript levels of these genes in ROX310 than in GT (Figure 3).
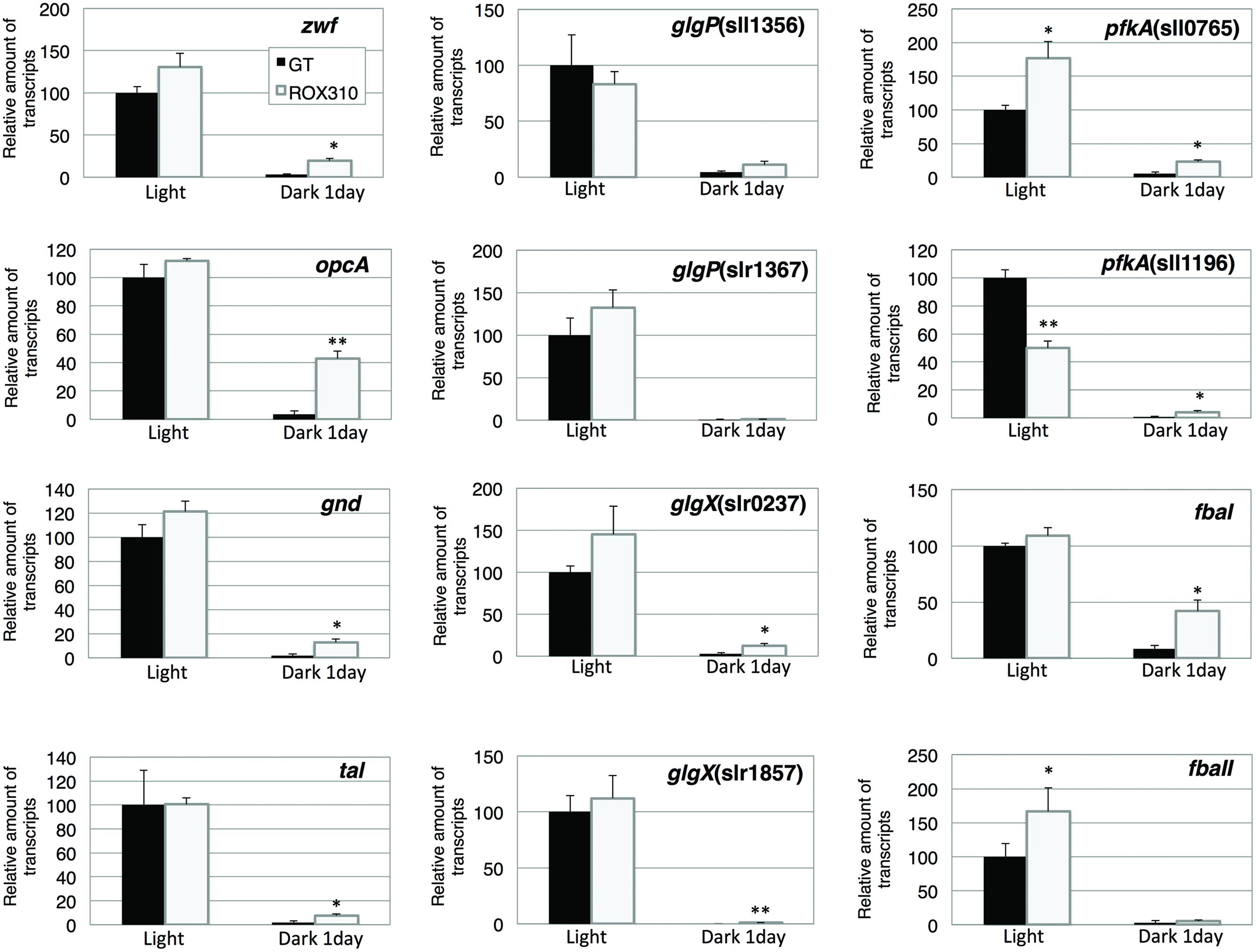
FIGURE 3. Quantitative real-time PCR analysis of transcription in GT and ROX310. Figure shows relative transcript levels of 12 genes involved in the OPP pathway (zwf, opcA, gnd, tal), glycogen catabolism [glgP(sll1356), glgP(slr1367), glgX(slr0237), glgX(slr1857)], and glycolysis [pfkA(sll0745), pfkA(sll1196), fbaI, fbaII]. Data represent mean ± SD from three independent experiments. Transcript levels were calibrated relative to that of the corresponding transcript in GT (set at 100%). Asterisks indicate statistically significant differences between GT and ROX310 (Student’s t-test; *P < 0.05, **P < 0.005).
Metabolome Analysis using the Cells Grown under Light and Dark Conditions
The glycogen levels were quantified under light and dark conditions. Glycogen rapidly disappeared in GT after the light-to-dark transition (Table 1). In ROX310, glycogen decreased after the light-to-dark transition, but the glycogen degradation was slower than GT (Table 1).
LC–MS/MS analysis revealed that the levels of sugar phosphates (glucose-6-phosphate, ribose-5-phosphate, sedoheptulose-7-phosphate, fructose-6-phosphate, ribulose-5-phosphate, fructose-1,6-bisphosphate) and dihydroxyacetone phosphate were lower in ROX310 than in GT under both light and dark conditions (Table 2). Phosphoenolpyruvate levels were higher in ROX310 than in GT under light and dark conditions (Table 2). Fumarate and isocitrate levels were lower in ROX310 than in GT under light conditions (Table 2). Malate could not be detected in ROX310 under light conditions (Table 2). Organic acids in the TCA cycle were lesser in ROX310 than in GT under dark conditions (Table 2). NADP levels increased by rpaA overexpression, but other nucleotides did not (Table 2).
Amino acid analyses showed that the levels of glycine and proline were higher in ROX310 than in GT under light conditions (Figure 4). The levels of alanine, glycine, threonine, and lysine were higher in ROX310 than in GT under dark conditions (Figure 4). The level of ornithine was lower in ROX310 than in GT under dark conditions (Figure 4).
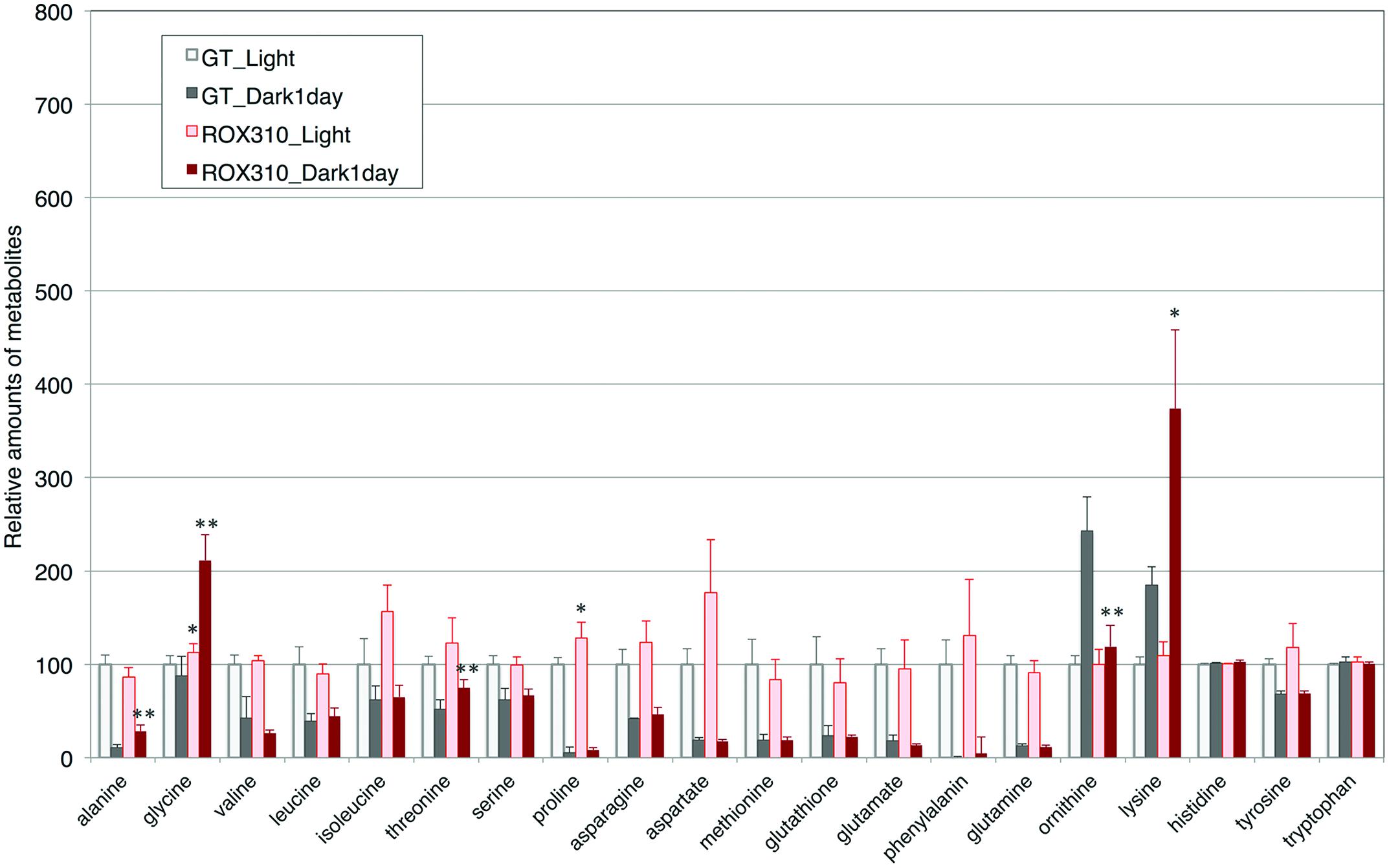
FIGURE 4. Levels of 18 amino acids, ornithine, and glutathione in ROX310. Data represent means ± SD from four independent experiments. Levels were calibrated relative to that of GT grown under light conditions (set at 100%). Asterisks indicate statistically significant differences between GT and ROX310 (Student’s t-test; *P < 0.05, **P < 0.005).
Alteration in Transcript Levels of Genes Encoding Circadian Clocks
Finally, expression of kaiABC genes was quantified. The transcript levels of kaiA, B1, C1, B2, and C2 were doubled by rpaA overexpression under light conditions (Figure 5). The transcript levels of kaiB3 and kaiC3 were marginally increased in ROX310 under light conditions (Figure 5). All the transcripts of kaiABC were decreased after 1 day of dark cultivation, and the levels remained higher in ROX310 than those in GT (Figure 5).
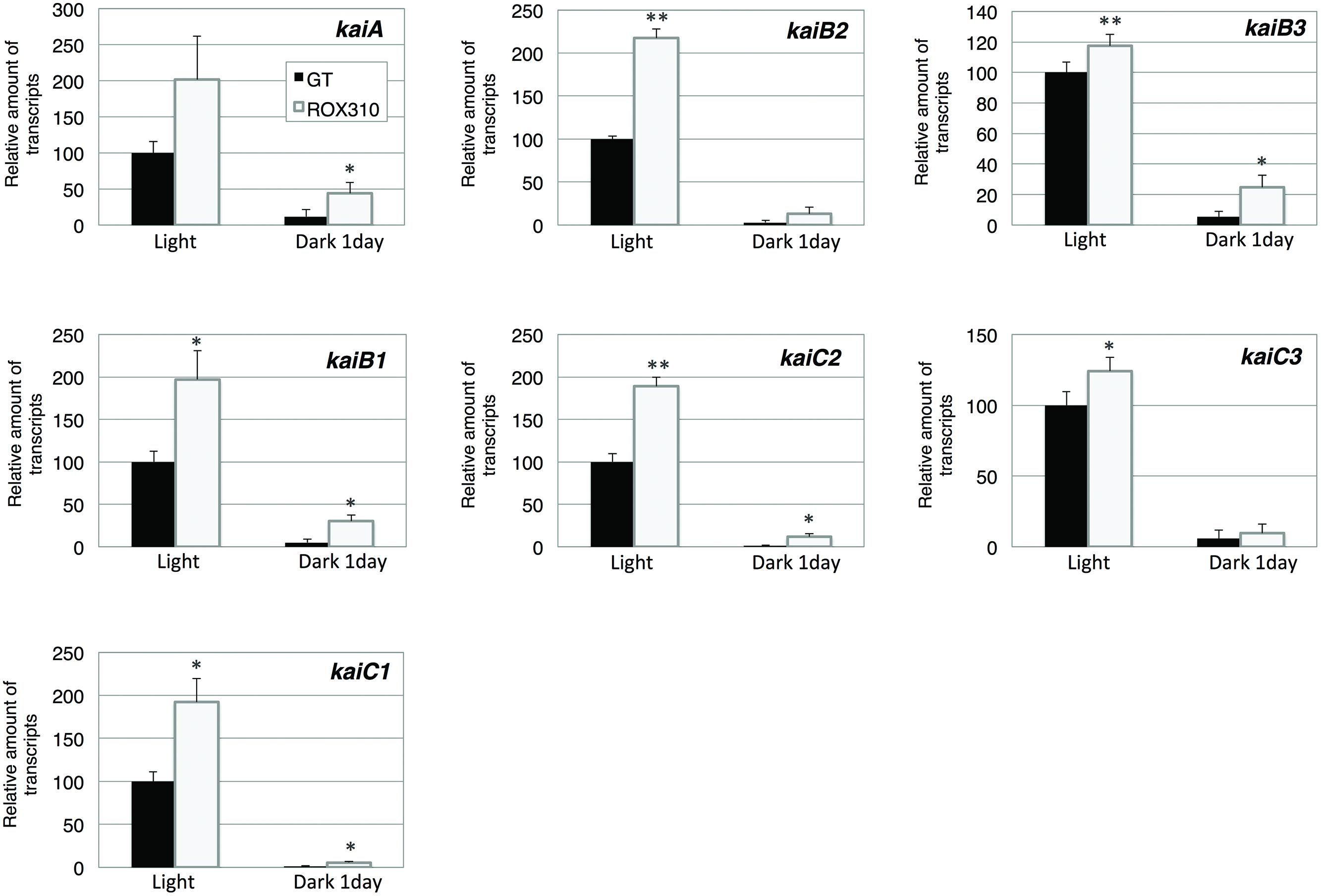
FIGURE 5. Quantitative real-time PCR analysis of transcription in GT and ROX310. Figure shows relative transcript levels of seven genes encoding KaiABC proteins. Data represent mean ± SD from three independent experiments. Transcript levels were calibrated relative to that of the corresponding transcript in GT (set at 100%). Asterisks indicate statistically significant differences between GT and ROX310 (Student’s t-test; *P < 0.05, **P < 0.005).
Discussion
Here, we have performed genetic and metabolomic analyses using an rpaA-overexpressing strain and revealed that RpaA is involved in the regulation of primary metabolism in this unicellular cyanobacterium. The mechanism of circadian clocks in Synechocystis 6803, which could be different from Synechococcus 7942, has been less studied except Drs. Axmann’s and Hellingwerf’s groups (Wiegard et al., 2013; Beck et al., 2014; van Alphen and Hellingwerf, 2015), and we proceeded metabolome analyses with the circadian-related mutants of Synechocystis 6803. ChIP-Seq analysis in Synechococcus 7942 shows that RpaA binds the promoters of kaiBC, sasA, rpaA, and genes encoding sigma factors (rpoD2, D5, D6) and sugar catabolic enzymes (glgP, malQ, zwf, opcA, gap1, fbaII), activating their gene expression at night (Markson et al., 2013). These results are consistent with our analysis: the rpaA overexpression altered the gene expression of sigE, a sugar catabolic enzyme, and kaiABC (Figures 2, 3, and 5). SigE is a sigma factor activating glycogen catabolism, glycolysis, and the OPP pathway (Osanai et al., 2005, 2011), and its expression peaks before night (Kucho et al., 2005). Combining the results of previous and current genetic analyses, the signal transduction from the circadian clock to sugar metabolism consists of the cascade of the proteins KaiABC-Hik8-RpaA-SigE in Synechocystis sp. PCC 6803, although further promoter analysis is required. Since RpaA altered the expression of kaiABC genes (Figure 5), feedback or feedforward regulation from RpaA to the central circadian oscillator may exist to entrain the clock by metabolic information.
The rpaA-overexpressing strain of Synechocystis 6803 exhibited several phenotypes. Results from the rpaA-overexpressing strain of Synechocystis 6803 were similar to the rpaA-null mutant of Synechococcus 7942, which showed decreased glycogen catabolism (Diamond et al., 2015). The rpaA knockout mutant in Synechocystis 6803 exhibited high light sensitive phenotype (Majeed et al., 2012), which is consistent with our results that RpaA is important in light acclimation. A previous study demonstrated that introduction of RpaA(D53E; which mimics phosphorylated RpaA) restored the RpaA function, but that the introduction of RpaA(D53A; which mimics non-phosphorylated RpaA) could not (Markson et al., 2013). Therefore, the rpaA overexpression in our study may have increased non-phosphorylated RpaA in the Synechocystis 6803 cells, leading to phenotypes that were the mixture of gain-of-function and loss-of-function of RpaA. Introduction of a phospho-mimic RpaA into Synechocystis 6803 may be intriguing to distinguish these phenotypes.
Overexpression of rpaA led to phenotypes similar to those of the hik8 overexpressor (Osanai et al., 2015); that is, growth defects under light-activated heterotrophic conditions (Figure 1), aberrant degradation of SigE after the light-to-dark transition (Figure 2A), accumulated transcripts during darkness (Figure 3), decreased levels of sugar phosphates (Table 2), and increased levels of several amino acids under dark conditions (Figure 4). These similarities may be due to the overexpression of hik8 accelerated the phosphorylation of RpaA proteins. The glycogen metabolism mutant could not grow under dark/heterotrophic conditions (Osanai et al., 2005; Singh and Sherman, 2005; Tabei et al., 2007), or treatment with high salt and oxidative stress (Suzuki et al., 2010). Thus, the growth phenotypes of ROX310 under LAHG conditions may be caused by the changes in primary metabolism (Figure 1C). Genetic analyses have suggested that the mutants of Clp proteases alter circadian oscillation in Synechococcus 7942 (Holtman et al., 2005; Imai et al., 2013). Nevertheless the aberrant degradation of SigE proteins under dark conditions in the rpaA-overexpressing strain (Figure 2A), glycogen catabolism was slowed under dark conditions (Table 1). These results suggest that proteins other than SigE concertedly determine the degree of glycogen degradation in Synechocystis 6803. Several sugar catabolic regulators, including Hik31, Rre37, and AbrB are known in Synechocystis 6803 (Kahlon et al., 2006; Tabei et al., 2007; Yamauchi et al., 2011). For example, Rre37 preferentially activates the gene expression of glycogen catabolic and glycolytic enzymes such as pfkA(sll1196; Azuma et al., 2009), and thus, further study of the relationships among several sugar catabolic regulators is necessary to elucidate the regulatory mechanism of sugar catabolism in Synechocystis 6803.
Control of primary carbon metabolism by a circadian clock is an important theme in cyanobacteria (Diamond et al., 2015). Decrease in glycogen and sugar phosphates was observed during dark conditions (Tables 1 and 2), which indicates glycogen and sugar phosphates are positively correlated in this condition. On the other hand, organic acids in the TCA cycle kept higher levels under dark conditions (Table 2). Thus, organic acids in the TCA cycle were not correlated with the metabolites in glycolysis and the OPP pathway. The rpaA-overexpressing strain showed decreased levels of sugar phosphates under both light and dark conditions (Table 2), which is consistent with the fact that RpaA is important for the expression of genes related to glycogen catabolism in unicellular cyanobacteria (Diamond et al., 2015). Our immunoblotting showed that GlgP(sll1356) proteins were decreased with rpaA overexpression (Figure 2C), which may be one reason for the down-regulation of glycogen catabolism in this mutant. The metabolomic analysis also revealed that organic acids in the TCA cycle (fumarate, malate, and oxaloacetate) were lowered by rpaA overexpression (Table 2). The organic acids in the TCA cycle are an important pool of carbon sources in this cyanobacterium (Osanai et al., 2014a). Thus, the data also indicates that RpaA widely regulates primary metabolism related to the carbon sinks in this cyanobacterium. KaiC regulates the production of lysine, which has been shown to be lowered during light/dark cycles in Synechococcus 7942 (Diamond et al., 2015). The analysis showed lysine and glycine levels were up-regulated by rpaA-overexpression during the light-to-dark transition (Figure 4), demonstrating the involvement of RpaA in amino acid metabolism in response to light conditions. In summary, our metabolome analyses have revealed the RpaA-regulation in primary sugar and amino acid metabolism of Synechocystis 6803.
Conflict of Interest Statement
The authors declare that the research was conducted in the absence of any commercial or financial relationships that could be construed as a potential conflict of interest.
Acknowledgments
This work was supported by the Ministry of Education, Culture, Sports, Science, and Technology, Japan, by a grant to TO from ALCA (Project name “Production of cyanobacterial succinate by the genetic engineering of transcriptional regulators and circadian clocks”) from the Japan Science and Technology Agency.
Supplementary Material
The Supplementary Material for this article can be found online at: http://journal.frontiersin.org/article/10.3389/fmicb.2015.00888
References
Ashby, M. K., and Mullineaux, C. W. (1999). Cyanobacterial ycf27 gene products regulate energy transfer from phycobilisomes to photosystems I and II. FEMS Microbiol. Lett. 181, 253–260. doi: 10.1111/j.1574-6968.1999.tb08852.x
Azuma, M., Osanai, T., Hirai, M. Y., and Tanaka, K. (2009). A response regulator Rre37 and an RNA polymerase sigma factor SigE represent two parallel pathways to activate sugar catabolism in a cyanobacterium Synechocystis sp. PCC 6803. Plant Cell Physiol. 52, 404–412. doi: 10.1093/pcp/pcq204
Beck, C., Hertel, S., Rediger, A., Lehmann, R., Wiegard, A., Kölsch, A., et al. (2014). Daily expression pattern of protein-encoding genes and small noncoding RNAs in Synechocystis sp. strain PCC 6803. Appl. Environ. Microbiol. 80, 5195–5206. doi: 10.1128/AEM.01086-14
Berla, B. M., Saha, R., Immethun, C. M., Maranas, C. D., Moon, T. S., and Pakrasi, H. B. (2013). Synthetic biology of cyanobacteria: unique challenges and opportunities. Front. Microbiol. 4:246. doi: 10.3389/fmicb.2013.00246
Diamond, S., Jun, D., Rubin, B. E., and Golden, S. S. (2015). The circadian oscillator in Synechococcus elongatus controls metabolite partitioning during diurnal growth. Proc. Natl. Acad. Sci. U.S.A. 112, E19160–E19245. doi: 10.1073/pnas.1504576112
Hanaoka, M., Takai, N., Hosokawa, N., Fujiwara, M., Akimoto, Y., Kobori, N., et al. (2012). RpaB, another response regulator operating circadian clock-dependent transcriptional regulation in Synechococcus elongatus PCC 7942. J. Biol. Chem. 287, 26321–26327. doi: 10.1074/jbc.M111.338251
Holtman, C. K., Chen, Y., Sandoval, P., Gonzales, A., Nalty, M. S., Thomas, T. L., et al. (2005). High-throughput functional analysis of the Synechococcus elongatus PCC 7942 genome. DNA Res. 12, 103–115. doi: 10.1093/dnares/12.2.103
Hosokawa, N., Hatakeyama, T. S., Kojima, T., Kikuchi, Y., Ito, H., and Iwasaki, H. (2011). Circadian transcriptional regulation by the posttranslational oscillator without de novo clock gene expression in Synechococcus. Proc. Natl. Acad. Sci. U.S.A. 108, 15396–15401. doi: 10.1073/pnas.1019612108
Imai, K., Kitayama, Y., and Kondo, T. (2013). Elucidation of the role of clp protease components in circadian rhythm by genetic deletion and overexpression in cyanobacteria. J. Bacteriol. 195, 4517–4526. doi: 10.1128/JB.00300-13
Ishiura, M., Kutsuna, S., Aoki, S., Iwasaki, H., Andersson, C. R., Tanabe, A., et al. (1998). Expression of a gene cluster kaiABC as a circadian feedback process in cyanobacteria. Science 281, 1519–1523. doi: 10.1126/science.281.5382.1519
Iwasaki, H., Williams, S. B., Kitayama, Y., Ishiura, M., Golden, S. S., and Kondo, T. (2000). A kaiC-interacting sensory histidine kinase, SasA, necessary to sustain robust circadian oscillation in cyanobacteria. Cell 101, 223–233. doi: 10.1016/S0092-8674(00)80832-6
Johnson, C. H., and Egli, M. (2014). Metabolic compensation and circadian resilience in prokaryotic cyanobacteria. Annu. Rev. Biochem. 83, 221–247. doi: 10.1146/annurev-biochem-060713-035632
Kahlon, S., Beeri, K., Ohkawa, H., Hihara, Y., Murik, O., Suzuki, I., et al. (2006). A putative sensor kinase, Hik31, is involved in the response to Synechocystis sp. PCC 6803 to the presence of glucose. Microbiology 152, 647–655. doi: 10.1099/mic.0.28510-0
Kanesaki, Y., Shiwa, Y., Tajima, N., Suzuki, M., Watanabe, S., Sato, N., et al. (2012). Identification of substrain-specific mutations by massively parallel whole-genome resequencing of Synechocystis sp. PCC 6803. DNA Res. 19, 67–79. doi: 10.1093/dnares/dsr042
Kim, Y. I., Vinyard, D. J., Ananyev, G. M., Dismukes, G. C., and Golden, S. S. (2012). Oxidized quinones signal onset of darkness directly to the cyanobacterial circadian oscillator. Proc. Natl. Acad. Sci. U.S.A. 109, 177651–177769. doi: 10.1073/pnas.1216401109
Kucho, K., Okamoto, K., Tsuchiya, Y., Nomura, S., Nango, M., Kanehisa, M., et al. (2005). Global analysis of circadian expression in the cyanobacterium Synechocystis sp. strain PCC 6803. J. Bacteriol. 187, 2190–2199. doi: 10.1128/JB.187.6.2190-2199.2005
Majeed, W., Zhang, Y., Xue, Y., Ranade, S., Blue, R. N., and He, Q. (2012). RpaA regulates the accumulation of monomeric photosystem I and PsbA under high light conditions in Synechocystis sp. PCC 6803. PLoS ONE 7:e45139. doi: 10.1371/journal.pone.0045139
Markson, J. S., Piechura, J. R., Puszynska, A. M., and O’Shea, E. K. (2013). Circadian control of global gene expression by the cyanobacterial master regulator RpaA. Cell 155, 1396–1408. doi: 10.1016/j.cell.2013.11.005
Nishiwaki, T., Satomi, Y., Kitayama, Y., Terauchi, K., Kiyohara, R., Takao, T., et al. (2007). A sequential program of dual phosphorylation of KaiC as a basis for circadian rhythm in cyanobacteria. EMBO J. 26, 4029–4037. doi: 10.1038/sj.emboj.7601832
Osanai, T., Imashimizu, M., Seki, A., Sato, S., Tabata, S., Imamura, S., et al. (2009). ChlH, the H subunit of the Mg-chelatase, is an anti-sigma factor for SigE in Synechocystis sp. PCC 6803. Proc. Natl. Acad. Sci. U.S.A. 106, 6860–6865. doi: 10.1073/pnas.0810040106
Osanai, T., Kanesaki, Y., Nakano, T., Takahashi, H., Asayama, M., Shirai, M., et al. (2005). Positive regulation of sugar catabolic pathways in the cyanobacterium Synechocystis sp. PCC 6803 by the group 2 sigma factor SigE. J. Biol. Chem. 280, 30653–30659. doi: 10.1074/jbc.M505043200
Osanai, T., Oikawa, A., Azuma, M., Tanaka, K., Saito, K., Hirai, M. Y., et al. (2011). Genetic engineering of group 2 sigma factor SigE widely activates expressions of sugar catabolic genes in Synechocystis species PCC 6803. J. Biol. Chem. 286, 30962–30971. doi: 10.1074/jbc.M111.231183
Osanai, T., Oikawa, A., Numata, K., Kuwahara, A., Iijima, H., Doi, Y., et al. (2014a). Pathway-level acceleration of glycogen catabolism by a response regulator in the cyanobacterium Synechocystis species PCC 6803. Plant Physiol. 164, 1831–1841. doi: 10.1104/pp.113.232025
Osanai, T., Oikawa, A., Shirai, T., Kuwahara, A., Iijima, H., Tanaka, K., et al. (2014b). Capillary electrophoresis-mass spectrometry reveals the distribution of carbon metabolites during nitrogen starvation in Synechocystis sp. PCC 6803. Environ. Microbiol. 16, 512–524. doi: 10.1111/1462-2920.12170
Osanai, T., Shirai, T., Iijima, H., Kuwahara, A., Suzuki, I., Kondo, A., et al. (2015). Alteration of cyanobacterial sugar and amino acid metabolism by overexpression hik8, encoding a KaiC-associated histidine kinase. Environ. Microbiol. 17, 2430–2440. doi: 10.1111/1462-2920.12715
Pattanayak, G. K., Phong, C., and Rust, M. (2014). Rhythms in energy storage control the ability of the cyanobacterial circadian clock to reset. Curr. Biol. 24, 1934–1938. doi: 10.1016/j.cub.2014.07.022
Reece, K. S., and Phillips, G. J. (1995). New plasmids carrying antibiotic-resistance cassettes. Gene 165, 141–142. doi: 10.1016/0378-1119(95)00529-F
Rippka, R. (1988). Isolation and purification of cyanobacteria. Methods Enzymol. 167, 3–27. doi: 10.1016/0076-6879(88)67004-2
Rust, M. J., Golden, S. S., and O’Shea, E. K. (2011). Light-driven changes in energy metabolism directly entrain the cyanobacterial circadian oscillator. Science 331, 220–223. doi: 10.1126/science.1197243
Rust, M. J., Markson, J. S., Lane, W. S., Fisher, D. S., and O’Shea, E. K. (2007). Ordered phosphorylation governs oscillation of a three-protein circadian clock. Science 318, 809–812. doi: 10.1126/science.1148596
Shoumskaya, M. A., Paithoonragsarid, K., Kanesaki, Y., Los, D. A., Zinchenko, V. V., Tanticharoen, M., et al. (2005). Identical Hik-Rre systems are involved in perception and transduction of salt signals and hyperosmotic signals but regulate the expression of individual genes to different extents in Synechocystis. J. Biol. Chem. 280, 21531–21538. doi: 10.1074/jbc.M412174200
Singh, A. K., and Sherman, L. A. (2005). Pleiotropic effect of a histidine kinase on carbohydrate metabolism in Synechocystis sp. strain PCC 6803 and its requirement for heterotrophic growth. J. Bacteriol. 187, 2368–2376. doi: 10.1128/JB.187.7.2368-2376.2005
Suzuki, E., Ohkawa, H., Moriya, K., Matsubara, T., Nagaike, Y., Iwasaki, I., et al. (2010). Carbohydrate metabolism in mutants of the cyanobacterium Synechococcus elongatus PCC 7942 defective in glycogen synthesis. Appl. Environ. Microbiol. 76, 3153–3159. doi: 10.1128/AEM.00397-08
Tabei, Y., Okada, K., and Tsuzuki, M. (2007). Sll1330 controls the expression of glycolytic genes in Synechocystis sp. PCC 6803. Biochem. Biophys. Res. Commun. 355, 1045–1050. doi: 10.1016/j.bbrc.2007.02.065
Takai, N., Nakajima, M., Oyama, T., Kito, R., Sugita, C., Sugita, M., et al. (2006). A KaiC-associating SasA-RpaA two-component regulatory system as a major circadian timing mediator in cyanobacteria. Proc. Natl. Acad. Sci. U.S.A. 103, 12109–12114. doi: 10.1073/pnas.0602955103
van Alphen, P., and Hellingwerf, K. J. (2015). Sustained circadian rhythms in continuous light in Synechocystis sp. PCC6803 growing in a well-controlled photobioreactor. PLoS ONE 10:e0127715. doi: 10.1371/journal.pone.0127715
Wiegard, A., Dörrich, A. K., Deinzer, H. T., Beck, C., Wilde, A., Holtzendorff, J., et al. (2013). Biochemical analysis of three putative KaiC clock proteins from Synechocystis sp. PCC 6803 suggests their functional divergence. Microbiology 159, 948–958. doi: 10.1099/mic.0.065425-0
Williams, J. G. K. (1988). Construction of specific mutations in photosystem II photosynthetic reaction center by genetic engineering methods in Synechocystis 6803. Methods Enzymol. 167, 766–778. doi: 10.1016/0076-6879(88)67088-1
Keywords: amino acids, cyanobacteria, Synechocystis, response regulator, sugar metabolism
Citation: Iijima H, Shirai T, Okamoto M, Kondo A, Hirai MY and Osanai T (2015) Changes in primary metabolism under light and dark conditions in response to overproduction of a response regulator RpaA in the unicellular cyanobacterium Synechocystis sp. PCC 6803. Front. Microbiol. 6:888. doi: 10.3389/fmicb.2015.00888
Received: 10 July 2015; Accepted: 14 August 2015;
Published: 26 August 2015.
Edited by:
Weiwen Zhang, Tianjin University, ChinaReviewed by:
Lei Chen, Tianjin University, ChinaGopal K. Pattanayak, The University of Chicago, USA
Copyright © 2015 Iijima, Shirai, Okamoto, Kondo, Hirai and Osanai. This is an open-access article distributed under the terms of the Creative Commons Attribution License (CC BY). The use, distribution or reproduction in other forums is permitted, provided the original author(s) or licensor are credited and that the original publication in this journal is cited, in accordance with accepted academic practice. No use, distribution or reproduction is permitted which does not comply with these terms.
*Correspondence: Takashi Osanai, School of Agriculture, Meiji University, 1-1-1, Higashimita, Tama-Ku, Kawasaki, Kanagawa 214-8571, Japan, tosanai@meiji.ac.jp
†These authors have contributed equally to this work.