- 1Biochemical Conversion Division, Sardar Swaran Singh National Institute of Bio-Energy, Kapurthala, India
- 2I.K Gujral Punjab Technical University, Kapurthala, India
The progressive rise in energy crisis followed by green house gas (GHG) emissions is serving as the driving force for bioethanol production from renewable resources. Current bioethanol research focuses on lignocellulosic feedstocks as these are abundantly available, renewable, sustainable and exhibit no competition between the crops for food and fuel. However, the technologies in use have some drawbacks including incapability of pentose fermentation, reduced tolerance to products formed, costly processes, etc. Therefore, the present study was carried out with the objective of isolating hexose and pentose fermenting thermophilic/thermotolerant ethanologens with acceptable product yield. Two thermotolerant isolates, NIRE-K1 and NIRE-K3 were screened for fermenting both glucose and xylose and identified as Kluyveromyces marxianus NIRE-K1 and K. marxianus NIRE-K3. After optimization using Face-centered Central Composite Design (FCCD), the growth parameters like temperature and pH were found to be 45.17°C and 5.49, respectively for K. marxianus NIRE-K1 and 45.41°C and 5.24, respectively for K. marxianus NIRE-K3. Further, batch fermentations were carried out under optimized conditions, where K. marxianus NIRE-K3 was found to be superior over K. marxianus NIRE-K1. Ethanol yield (Yx∕s), sugar to ethanol conversion rate (%), microbial biomass concentration (X) and volumetric product productivity (Qp) obtained by K. marxianus NIRE-K3 were found to be 9.3, 9.55, 14.63, and 31.94% higher than that of K. marxianus NIRE-K1, respectively. This study revealed the promising potential of both the screened thermotolerant isolates for bioethanol production.
Introduction
Production of ethanol is an important task worldwide for improving global energy demand. Bioethanol has been produced from different renewable feedstocks like fermentable sugars, starchy and lignocellulosic materials such as agricultural residues (paddy straw, wheat straw, sugarcane bagasse), woody plants and waste papers (Viikari et al., 2012; Behera et al., 2014a,b; Kumar et al., 2015). Currently, agricultural biomass is of the prime focus in the renewable energy production. The major components like cellulose and hemicellulose in lignocellulosic biomass can be converted to fermentable sugars such as glucose and xylose through saccharification, which can further be fermented to ethanol by various microorganisms (Kumar et al., 2009a; Pakarinen et al., 2011; Narayanaswamy et al., 2013).
Glucose is the most abundant sugar in lignocelluloses, which can be easily metabolized for the production of bioethanol. The yeast Saccharomyces cerevisiae is a traditional glucose fermenting yeast with the highest ethanol yield and tolerance (Behera and Ray, 2012). The second most abundant sugar is xylose which cannot be easily fermented by the industrial strains and can add up to 25% of the composition of lignocellulosic feedstocks (Olofsson et al., 2008). Several xylose fermenting yeasts such as Pichia segobiensis, Scheffersomyces stipitis, Pachysolen tannophilus, Scheffersomyces shehatae, C. lyxosophila, C. prachuapensis, C. intermedia, C. tenuis C. jeffriesii, Brettanomyces naardenensis, Spathaspora passalidarum, Spathaspora arborariae, etc. has been reported by different researchers (Cadete et al., 2009; Nitiyon et al., 2011; Lorliam et al., 2013). But the rate of xylose consumption is reported to be very low by these strains. However, utilization of both sugars present in lignocellulosic biomass is an important parameter to increase the production of ethanol. Co-fermentation of glucose and xylose sugars through co-cultivation of microbial strains shows unsatisfactory results due to their difference in ethanol tolerance and fermentation conditions (Jeffries, 2006).
Currently, majority of bioethanol is produced using mesophilic microorganisms. However, thermo-ethanologenic yeasts receive considerable interest due to current challenges of increasing temperature, which could potentially overcome many obstacles. The use of thermophillic/thermotolerant yeast for bioethanol production have several process advantages including broad substrate utilization range, higher saccharification and fermentation rates, minimized contamination risk, lower costs of pumping and stirring and no cooling problems, less energy requirement for mixing and product recovery (Dung et al., 2012; Kumar et al., 2013; Arora et al., 2015a; Scully and Orlygsson, 2015). The thermophilic/thermotolerant microorganisms produce distinctive enzymes that function under extreme conditions comparable to those existing in several industrial processes (Arora et al., 2015b). Hence, these microorganisms are of great interest for industrial applications.
Many researchers have reported isolation of thermoethanologenic species including Kluyveromyces marxianus, Issatachenkia orientalis, Geobacillus thermoglucosidasius, Clostridium sp., Thermoanaerobacterium AK54, Thermoanaerobacter pentosaceus (Riyanti and Rogers, 2009; Kwon et al., 2011; Orlygsson, 2012; Sigurbjornsdottir and Orlygsson, 2012; Tomás et al., 2013; Arora et al., 2014). Although, these species exhibit ethanol production at high temperature but still there are certain challenges including lower yield from pentoses, lower tolerance to ethanol, sensitivity to fermentation inhibitors and production of various by-products. Hence, optimization of growth and fermentation parameters is essential for higher fermentation efficiency.
Response Surface Methodology (RSM) is a statistical tool, which is used to design the experiments, build models, thereby, evaluate the effect of various variables on one or more responses and sets an optimal solution for the responses with reduction in the number of experimental runs (Bas and Boyaci, 2007; Uncu and Cekmecelioglu, 2011). Most commonly used design in RSM is Central Composite Design (CCD) which is characterized by flexible rotation in the design space, more precise predictions about the response of the variables along with the information about the experimental errors (Montgomery, 2009). Optimization using CCDs have been applied by many researchers for the optimization of various parameters for bioethanol production (Manikandan and Viruthagiri, 2010; Zhao et al., 2010; El-Gendy et al., 2013; Manivannan and Narendhirakannan, 2014).
The present study was carried out for the new search of thermotolerant/thermophilic co-fermenting yeasts for bioethanol production. The parameters like temperature and pH were optimized for growth and fermentation of isolated thermotolerant ethanologens with RSM using statistical software for enhancing their growth rate which results in augmented production of ethanol.
Materials and Methods
Sample Collection
Soil samples were collected from various sites in the different states of India (Table 1) by exploring each site for 60–90 min by walking-through in varying climatic conditions. Soil samples were placed in the plastic bags and their details were recorded. All the samples were stored in the refrigerator at 4 ± 0.5°C for further isolation process.
Isolation, Screening and Identification of Yeasts for Ethanol Production
A pinch of soil sample was suspended in 10 ml of sterile water and vortexed for 2 min at maximum speed before 10 × serial dilution. 100 μl from each dilution in series was spread onto the surface of yeast extract-peptone (YEP) phytagel (dextrose, 2%; yeast extract, 1%; peptone, 2%; phytagel, 1.5%; ampicillin, 50 mg/ml; pH, 5.5) plates having 1% glucose or xylose sugar and incubated at different temperature (40–50°C) for 24–48 h. Xylose utilizing yeasts were isolated by using xylose sugar (2%) in place of dextrose to the above medium. Based on the morphology, size and color, various colonies were selected and subcultured on to separate phytagel plates to ensure their purity. The isolated strains were carefully studied on the basis of cultural characteristics the colony and their growth pattern. Strain selection was based on the anaerobic growth and production of ethanol on media containing both glucose as well as xylose. Further, the selective yeast cultures were identified on the basis of sequencing of Internal transcribed spacer (ITS) and D1D2 domain of rRNA gene from Microbial Type Culture Collection and Gene Bank (MTCC), Chandigarh, India. The homologies of the sequences were determined using the Basic Local Alignment Search Tool (BLAST) system of the DNA Data Bank of National Center for Biotechnology Information (NCBI).
Microorganism and Culture Condition
The isolated yeast cultures were maintained on YEP medium having dextrose (YEPD) or xylose (YEPX) sugar according to the use by the isolates. The culture was stored at 4 ± 0.5°C for further use. The stock culture was maintained in glycerol 30% at −80°C.
Growth Conditions
The inoculums for both K. marxianus NIRE-K1 and NIRE-K3 were prepared in 100 ml salt medium with composition (in g l−1) ammonium sulfate, 2.0; di-sodium hydrogen ortho phosphate, 0.15; potassium di-hydrogen ortho phosphate, 0.15; yeast extract, 1.0; glucose, 10.0 at pH 5.5, 45°C, taken in sterilized (at 121°C for 20 min) 500 mL erlenmeyer flask. The flask was inoculated with 100 μl of mother inoculums from YP medium and incubated for 24 h at 150 rpm in an orbital shaker incubator. The cells grown after 24 h were used as the inoculum used for subsequent runs for optimization.
Optimization of Temperature and pH Using FCCD
Physical and chemical variables essential for growth of both the screened isolates K. marxianus NIRE-K1 and NIRE-K3 were optimized according to RSM using Design Expert software version 8.0 (STAT-EASE Inc., Minneapolis, USA). FCCD was employed to study the combined effect of temperature and pH on the maximum specific growth rate (μmax) as the response. Both the growth parameters were studied at three levels viz. low (−1), middle (0) and high (+1), with alpha value of 1. The real and coded values of these variables applicable for both the isolates have been presented in Table 2. The software was designed with 13 experimental runs with 5 runs at the center points to ensure the reproducibility of the model. The responses, μmax for both the cultures, were calculated using Monod model from the Equation (1), as described below:
Where, μ is the specific growth rate (h−1), S is rate limiting substrate concentration (g l−1), Ks is saturation constant or half velocity constant or substrate utilization constant (g l−1). The values of the responses were the means of two replications to enhance the accuracy of the model.
After regression analysis, the statistical significance of the model was evaluated using analysis of variance (ANOVA) and lack of fit tests. The variables that significantly affected the responses were determined using a confidence level above 95% or a p-value less than 0.05. The response of the dependent variable was evaluated using the second order polynomial Equation (2) with variance for each variable divided into linear, quadratic and interactive components as described below:
Where, Y is predicted response (μmax, h−1), x1 and x2 are the coded levels of independent variables, b0 is the offset term, b1 and b2 are the linear effects, b11 and b22 are the quadratic effects and b12 is the interaction effect.
The quality of the model developed was estimated by coefficient of determination (R2), adjusted R2 (R2adj) and predicted R2 (R2predict). Contour and three dimensional plots were drawn to illustrate the relationship between the responses and the variables to be optimized. The interaction of one parameter with other can be studies from the pattern of the contour plots. For obtaining the optimal solutions, numerical optimization method of Design Expert software was employed.
Model Validity
To validate the authenticity of software generated model, a confirmatory experiment with duplicate sets was performed under the optimized conditions to verify the predicted values for maximum specific growth rate.
Fermentation Conditions
Inoculums for fermentation by isolates K. marxianus NIRE-K1 and K. marxianus NIRE-K3 were prepared in the salt medium with similar composition as that of growth medium containing 10 g l−1 glucose concentration. The cells from the exponential phase were pumped into a bioreactor of 5 L working volume (NBS BioFlo-CelliGen 115) with initial glucose concentration of 100 g l−1. The pH and temperature for both the isolates were maintained at optimized conditions suggested by the software.
Analytical Procedures
Samples were withdrawn at 4 h intervals and centrifuged at 10,000 rpm for 15 min. The collected samples were stored at 4°C prior to analysis. Various metabolites in fermentation broth (glucose, ethanol, glycerol, and acetic acid) were analyzed using High Performance Liquid Chromatography (Agilent Technologies) using HiPlex H column at 57°C with 1 mM H2SO4 as the mobile phase at a flow rate of 0.7 ml min−1 and detected by Refractive Index Detector at 50°C.
Dry cell weight (DCW) was obtained by centrifuging 1 ml of sample in pre-dry weighted Eppendorf tube using Eppendorf centrifuge 5430 R at 10,000 rpm for 15 min, followed by washing the pellet twice with distilled water and further drying in a vacuum oven at 80°C to a constant weight. All the experiments were carried out in triplicate, and the given values are the mean values. Fermentation kinetics for both the screened isolates was calculated using the formulae by Bailey and Ollis (1986).
Results and Discussion
Isolation, Screening and Identification of Yeasts for Ethanol Production
A wide variety of microorganisms are present in the environment, which can be harnessed for the purpose of mankind. The isolation and screening of the efficient therrmophilic/thermotolerant ethanol producing yeasts can be helpful to overcome the current challenges in biofuel production. Therefore, the thermophilic/thermotolerant character was taken into the consideration in this study. A total of 103 thermophilic strains of yeast were isolated from different samples collected from various sites (Table 1). The isolated strains were carefully identified by cultural characteristics and growth pattern studies using microscope. About 14 yeast isolates were found to be positive for ethanol production from glucose. However, in fermentation studies on defined media for screening of isolates, two yeasts (NIRE-K1 and NIRE-K3) showed to possess the ability to ferment both glucose and xylose to ethanol under anaerobic conditions (Table 1).
Aerobic batch fermentations were carried out using both the yeast strains of K. marxianus NIRE-K1 and NIRE-K3 on YEP medium containing 2% glucose and xylose solely and further mixture of both in equal ratio at 45°C. Both the strains of K. marxianus NIRE-K1 and NIRE-K3 could produce maximum ethanol concentration with ethanol yield of 0.31 ± 0.023 and 0.36 ± 0.022 g g−1, respectively in YEPD medium with complete utilization of glucose (20 g l−1) in 16 h (Figure 1). However, both the strains could utilize xylose for the production of ethanol with concomitant xylitol production. In case of xylose containing YEPX media using cells of K. marxianus NIRE-K1, maximum ethanol and xylitol concentration of 0.3 ± 0.01 and 4.34 ± 0.03 g l−1 were obtained in 24 h of duration through the utilization of 13.18 ± 0.029 g l−1 xylose sugar (Figure 2). Similarly with the same duration of fermentation, K. marxianus NIRE-K3 could be able to utilize 11.18 ± 0.04 g l−1 of xylose for the production of 0.88 ± 0.01 and 0.80 ± 0.01 g l−1 of ethanol and xylitol, respectively (Figure 2). In other hand, both the strains of K. marxianus NIRE-K1 and NIRE-K3 was capable of simultaneously using mixture of glucose and xylose in YEPDX medium, achieving maximum ethanol concentration of 3.4 ± 0.051 and 3.5 ± 0.057 g l−1, respectively in 24 h of fermentation. Both the strains could be able to completely utilize glucose, while 6.63 ± 0.1 and 4.39 ± 0.01 g l−1 of residual xylose was left in case of K. marxianus NIRE-K1 and NIRE-K3, respectively (Figure 3).
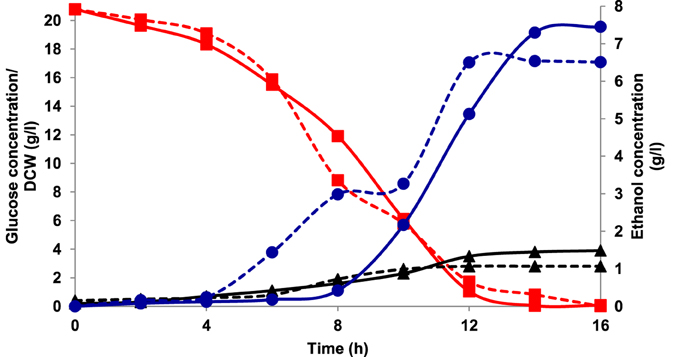
Figure 1. Growth of K. marxianus NIRE-K1 and NIRE-K3 in YEPD medium, (---) K. marxianus NIRE-K1; (—) K. marxianus NIRE-K3; (■) Glucose; (▴) Dry cell weight (DCW); (•) Ethanol.
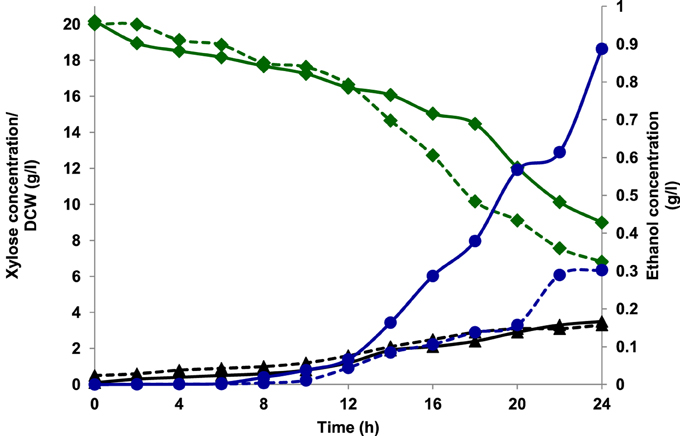
Figure 2. Growth of K. marxianus NIRE-K1 and NIRE-K3 in YEPX medium, (---) K. marxianus NIRE-K1; (—) K. marxianus NIRE-K3; (♦) Xylose; (▴) Dry cell weight (DCW); (•) Ethanol.
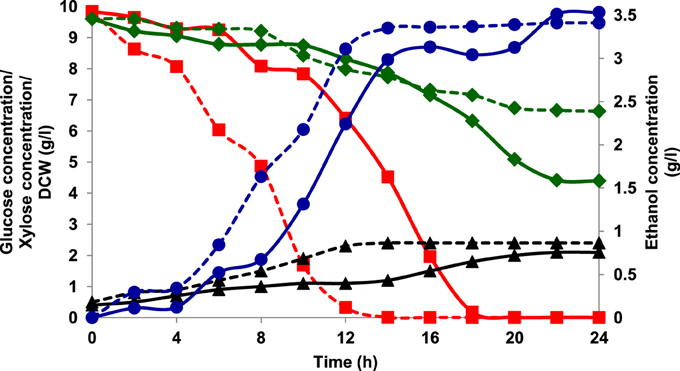
Figure 3. Growth of K. marxianus NIRE-K1 and NIRE-K3 in YEPDX medium, (---) K. marxianus NIRE-K1; (—) K. marxianus NIRE-K3; (■) Glucose; (♦) Xylose; (▴) Dry cell weight (DCW); (•) Ethanol.
On the basis of above study, these two isolates were selected, identified and optimized for further study. Both the screened isolates, NIRE-K1 and NIRE-K3 were sequenced and identified as Kluyveromyces marxianus NIRE-K1 and Kluyveromyces marxianus NIRE-K3, respectively. Both the cultures, K. marxianus NIRE-K1 and K. marxianus NIRE-K3 have been deposited at MTCC, Chandigarh with the deposition no. MTCC 5933 and MTCC 5934, respectively. Subsequently, partial genome sequence of Kluyveromyces marxianus NIRE-K1 and NIRE-K3 (ascomycetes yeasts of the fungal family Saccharomycetaceae and order Saccharomycetales) have been submitted in NCBI gene bank with GenBank accession number KP405925.1 and KP405926.1, respectively.
The phylogenetic tree is the study of evolutionary relatedness between the species. In this study, phylogenetic tree was drawn on the basis of distance matrix of homology sequence of similar microorganisms by BLAST where isolate NIRE-K1 was found to be related to the ascomycetes group and have maximum homology similarity with K. marxianus strain CHY1612 which has been depicted in Figure 4. Similarly, other isolate NIRE-K3 showed maximum similarity with both the strains of K. marxianus 1.2 18S and K. marxianus B.WHX.12 (Figure 5). Further, sequence of NIRE-K1 is the substem of K. marxianus DMKU3-1042 with 99% homology. Therefore, on the basis of the above similarity, the isolate NIRE-K1 and NIRE-K3 were confirmed as the K. marxianus which were named as K. marxianus NIRE-K1 and K. marxianus NIRE-K3.
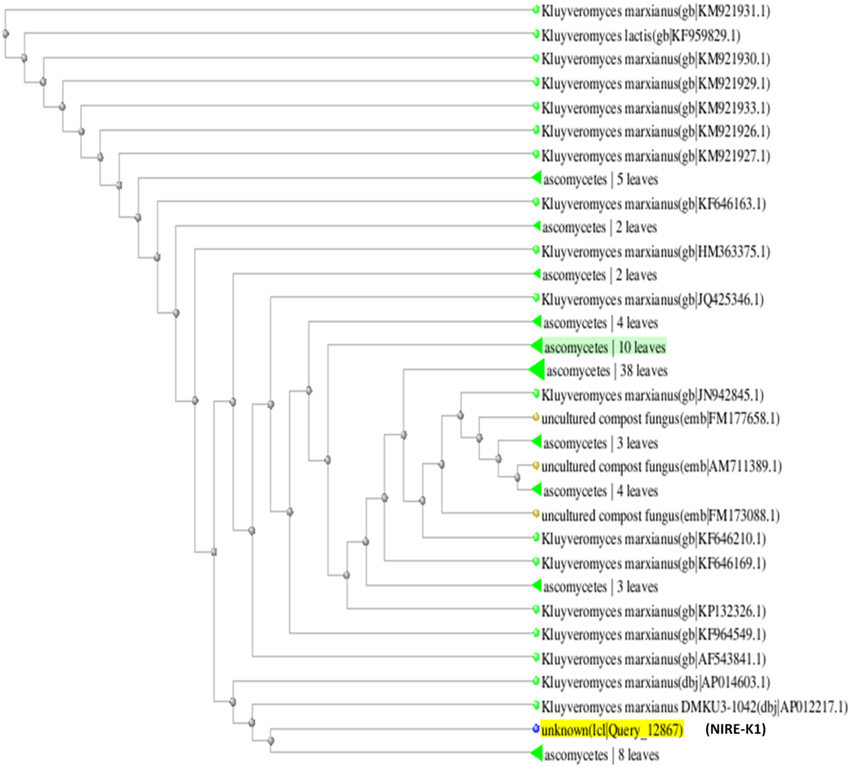
Figure 4. Phylogenetic tree drawn through BLAST showing genetic relationship between K. marxianus NIRE-K1 and similar organisms.
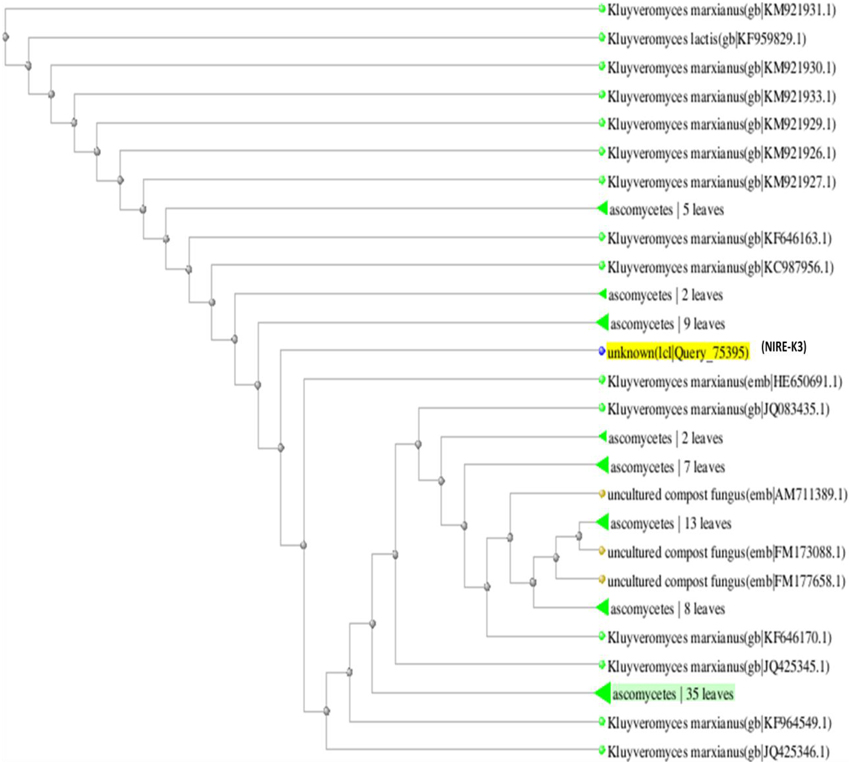
Figure 5. Phylogenetic tree drawn through BLAST showing genetic relationship between K. marxianus NIRE-K3 and similar organisms.
Several researchers have isolated various yeasts from different sources for the ethanol production to reduce the overall cost. Yeast cells exhibit a complex mechanism with rapid molecular response, when exposed to elevated temperature (Cimpeanu et al., 2010; Ma and Liu, 2010; Stanley et al., 2010). Tofighi et al. (2014) isolated thermotolerant Saccharomyces cerevisiae from waste water samples with optimum growth temperatures ranging from 35 and 40°C and ethanol yield of 75% of its theoretical value in respect to glucose. Kaewkrajay et al. (2014) isolated thermotolerant yeast from the soil samples collected from sugarcane, cassava and pineapple plantations in five different provinces of Thailand. The yeast was used for ethanol production from cassava starch hydrolysate at 45°C with the maximum ethanol concentration of 42.4 g l−1 in 48 h, at a productivity of 0.88 g l−1 h−1 and a yield of 46% of the theoretical yield in respect to glucose. Similarly, Yuangsaard et al. (2013) isolated Pichia kudriavzevii DMKU 3-ET15 from the traditional fermented pork sausage, which produced ethanol concentration of 4% (w/v) with productivity of 1.27 g l−1 h−1 and yield of 42% of the theoretical yield in a cassava starch hydrolysate medium at pH 5.0 and 45°C. Dhaliwal et al. (2011) reported the isolation of P. kudriavzevii from sugarcane juice at 40°C, which produced 71.9 g/l of ethanol with a productivity of 4.0 g l−1 h−1. Hashem et al. (2013) isolated two thermotolerant yeasts Kluyveromyces sp. GU133329 and Kluyveromyces sp. GU133331 from plum fruit and cantaloupe, which produced 9.55 (w/v) and 11.72% (w/v) of ethanol, respectively at pH 5.5 and temperature of 35°C. These results imply that these isolated strains could be able to produce ethanol at high temperature.
Optimization of Growth Conditions
Physical and chemical parameters viz. temperature and pH play a noteworthy role in controlling the growth of microorganisms (Charoenchai et al., 1998). Growth and fermentation rate increases to a certain extent with increase of both temperature and pH, which decreases sharply, thereby lowering both cell and ethanol yields (Torija et al., 2003; Phisalaphong et al., 2005). According to Anusiem (2004), with every 10°C rise in temperature, the rate of reaction is doubled. However, in case of biochemical reactions, the inhibition of temperature on growth and fermentation beyond the optimized range can be endorsed due to denaturation of ribosomes and the enzymes as well as disruption of the cell membrane due to changes in the fluidity (McMeckin et al., 2002). Similarly, the deleterious effect of pH beyond the optimized range could influence the NADH to NAD+ ratio, which further affects the metabolic flux toward ethanol and biomass formation (Peña et al., 1972; Adnan et al., 2014). Hence, both these factors must be studied with respect to their interactive behavior and their influence on biomass and ethanol yields.
In the present study, both the variables were taken into account to study their effect on the specific growth rate of both the screened yeast isolates. FCCD matrix for both the variables (temperature and pH) with experimental and predicted values of maximum specific growth rates of the isolates K. marxianus NIRE-K1 and NIRE-K3 has been shown in Table 3. The significance of the quadratic model was further determined by the ANOVA tables through Fisher's “F”-test (Tables 4, 5). The regression models (quadratic) were found to be significant with F-value of 305.00 and 244.04 for K. marxianus NIRE-K1 and NIRE-K3, respectively. Moreover, for both the isolates, p-values were found to be less than 0.05. According to Rene et al. (2007), the high significance of the regression model is indicated by the F-value with a low probability “p”-value. For both the isolates, linear effect of temperature and squared effect of both temperature and pH were found to be significant model terms with p < 0.05 (Tables 4, 5). Though the variable, pH is insignificant in linear term but its significance in the squared term shows that any change in these variables significantly affects the specific growth rate. On the contrary, Serra et al. (2005) specified temperature as the main influencing factor in comparison to pH on maximum specific growth rate of wine yeasts, S. bayanus var. uvarum P3 and S. cerevisiae VL3c. The effect of both temperature and pH for ethanol production is also reported by Eiadpum et al. (2012), Singh and Bishnoi (2012), and Udhayaraja and Sriman (2012).
The “lack of fit” was found to be non-significant relative to the pure error with F-value of 3.24 and 3.30, respectively for both the isolates, K. marxianus NIRE-K1 and NIRE-K3. The equations based on the quadratic models for the isolates, K. marxianus NIRE-K1 (Equation 3) and NIRE-K3 (Equation 4), respectively, in terms of experimental factors has been represented below:
Where, Y is the maximum specific growth rate (h−1); A and B are temperature and pH, respectively.
The goodness of fit of the regression model is estimated by the coefficient of determination (R2) which measures the variability in the response values due to variation in the experimental factors and their interactions. The R-squared values close to 1 indicates the stronger model and better response prediction (Ohtani, 2000). However, a model can be accepted with R2 > 0.75 (Chauhan and Gupta, 2004). The model presented in Table 4 for K. marxianus NIRE-K1 exhibits high R-squared value of 0.9954 which explains 99.54% of the variation in the response, as well as high value of the adjusted determination coefficient (adjusted R2 = 0.9922) showing correlation between the observed and predicted values, suggesting a high significance of the model. Similar results were obtained for K. marxianus NIRE-K3 with determination coefficient (R2 = 0.9943) and adjusted determination coefficient (R2adj = 0.9902) as shown in Table 5. Singh et al. (2011) determined the accuracy of the RSM model by evaluating the correlation between the observed and predicted values, which was found to be ~0.9. However, the task of comparing the results of models from literature is quite exigent due to variation in operating conditions viz, type of inoculums, substrate, supplementary nutrients, type of reactor and its size.
The value of coefficient of variation (CV = 5.16 and 6.31% for K. marxianus NIRE-K1 and NIRE-K3) was low due to the small residue between actual and predicted maximum specific growth rate. Also, to measure the adequate precision of the model and reliability of the experimental part, ratio of signal to noise is determined, where a ratio greater than 4 is desirable (Montgomery, 2001). In the present study, the ratio of 41.292 and 37.079 in case of K. marxianus NIRE-K1 and NIRE-K3 indicates an adequate signal to use the model for prediction purposes. Figures 6A,B show the diagnostic plots between the experimental and predicted values for K. marxianus NIRE-K1 and NIRE-K3, respectively, wherein all the points lie along the diagonal line, again indicating a good fit for both the models. Contour and three dimensional plots for isolates K. marxianus NIRE-K1 and NIRE-K3 have been shown in Figures 7, 8, respectively. The interaction between the variables is indicated by the shape of the contour plot. Strong interactions between the variables are indicated by the elliptical plots whereas circular plots indicate weaker interactions (Prakash et al., 2008). However, the plots of both the isolates were found to be elliptical (Figure 7). In three dimensional plots, (Figure 8), the convex response surface suggested well-defined optimum variables (temperature and pH) and maximum specific growth rate increased to the peak with the increase of temperature and pH up to 45°C and 5.5 for both the isolates which declined beyond the values.
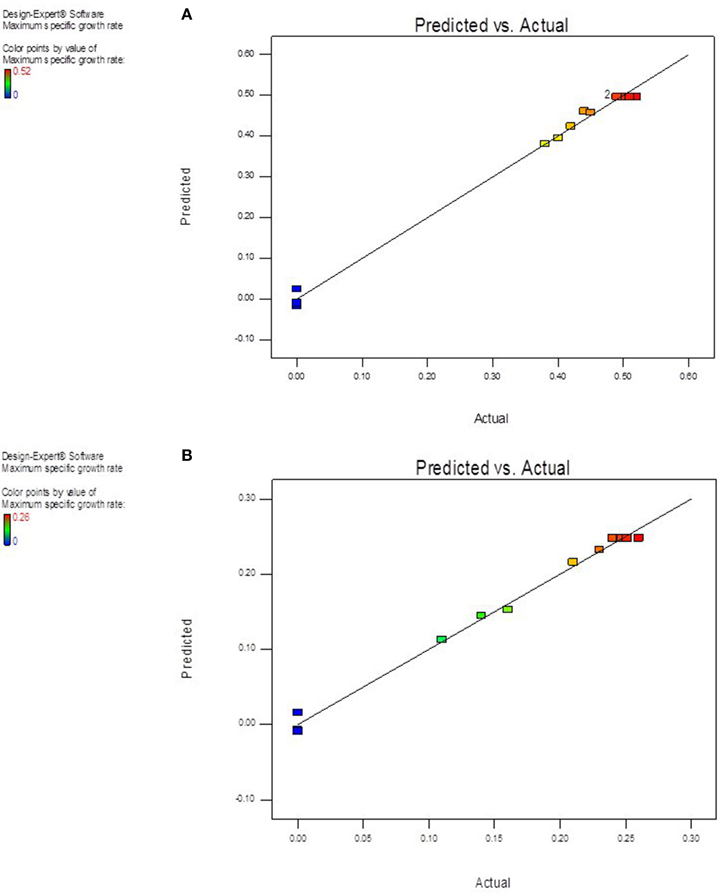
Figure 6. Diagnostic plot of the distribution of observed and predicted values of maximum specific growth rate (A) K. marxianus NIRE-K1 (B) K. marxianus NIRE-K3.
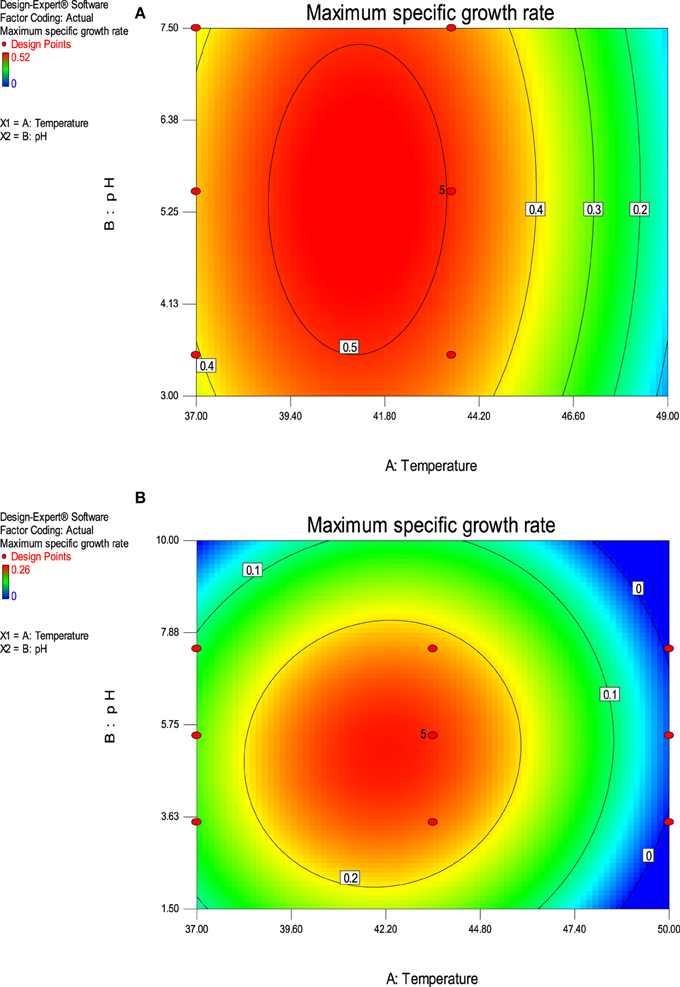
Figure 7. Contour plot of maximum specific growth rate as a function of temperature and pH (A) K. marxianus NIRE-K1 (B) K. marxianus NIRE-K3.
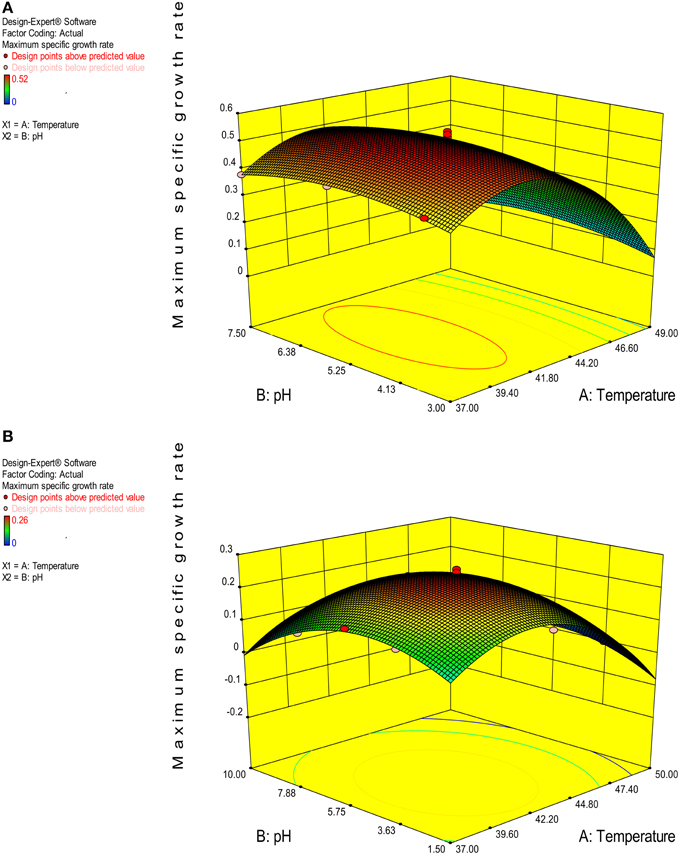
Figure 8. 3-D plot of maximum specific growth rate as a function of temperature and pH (A) K. marxianus NIRE-K1 (B) K. marxianus NIRE-K3.
The optimized values for both the variables were obtained by numerical optimization in the software with a desirability function values of 0.718 and 0.730 for K. marxianus NIRE-K1 and NIRE-K3, respectively. The optimized values of pH and temperature were found to be 5.49 and 45.17°C with a predicted μmax of 0.427 in case of isolate K. marxianus NIRE-K1 which was 5.24 and 45.41°C for K. marxianus NIRE-K3 with a predicted μmax of 0.214, keeping the goal of maximum temperature and specific growth rate. Similarly, Arroyo-López et al. (2009) reported 0.551 and 0.660 h−1 of maximum specific growth rates for yeasts T73 and hybrid W27 using optimized parameters of temperature 34.1°C and pH 4.76, respecively. Apart from the growth rate, many researchers have optimized the physical and chemical variables for ethanol fermentation using RSM. Man et al. (2010) reported maximum ethanol concentration of 24.17 g/L after optimizing the temperature of 38°C and pH 5.45. Dasgupta et al. (2013) optimized and reported a pH of 4.5 for K. marxianus IIPE453 to get maximum ethanol concentration.
Validation of the Model
To verify the accuracy of the model and reproduce the results predicted by the software, verification experiments were performed under optimized conditions for both the isolates and analyzed for their respective growth rates. The predicted growth rates suggested by the software under the optimized conditions were found to be 0.427 and 0.214 h−1 for K. marxianus NIRE-K1 and NIRE-K3, respectively. However, maximum specific growth rates of 0.413 and 0.209 h−1 were obtained after performing the experiments in triplicate for K. marxianus NIRE-K1 and NIRE-K3. The obtained values are in close agreement with the predicted values at a difference of only 3.3 and 2.3% for K. marxianus NIRE-K1 and NIRE-K3, respectively. According to Levin et al. (2008) differences between experimental and predicted values of less than 10% confirmed the validity of a model. Hence, the model developed from the response surface methodology in the present study is reliable and reproducible.
Batch Fermentation
Batch ethanol fermentation was performed under the optimized conditions of temperature and pH for fermentation with initial glucose concentration of 100 g l−1 by both the cultures of K. marxianus NIRE-K1 and K. marxianus NIRE-K3. The ethanol production and sugar utilization profile by these two cultures have been given in Figure 9. In the present study, ethanol production by both of the cells initiated in the log phage of the growth producing 5.26 ± 0.05 and 6.13 ± 0.18 g l−1 of ethanol using 16.89 ± 0.36 and 14.5 ± 0.33 g l−1 of sugar in 4 h of fermentation. The decrease in sugar concentration might be due to its utilization for initial growth and metabolism of the yeast in addition to its conversion into ethanol (Behera et al., 2011). For 8 and 12 h of fermentation, 55.26 and 74% of sugar was utilized with simultaneous increase in ethanol concentration to 23.44 ± 0.25 and 31.57 ± 0.48 g l−1, respectively with the cells of K. marxianus NIRE-K1 (Figure 9A). During 8 h of fermentation, 70.79% of sugar was utilized with simultaneous increase in ethanol concentration to 31.4 ± 0.40 g l−1 using the cells of K. marxianus NIRE-K3 (Figure 9B). Finally, maximum ethanol production of 39.12 ± 0.34 and 43.25 ± 0.36 g l−1 was achieved with 100% sugar utilization after 12 and 16 h of fermentation using the cells of K. marxianus NIRE-K1 and NIRE-K3, respectively.
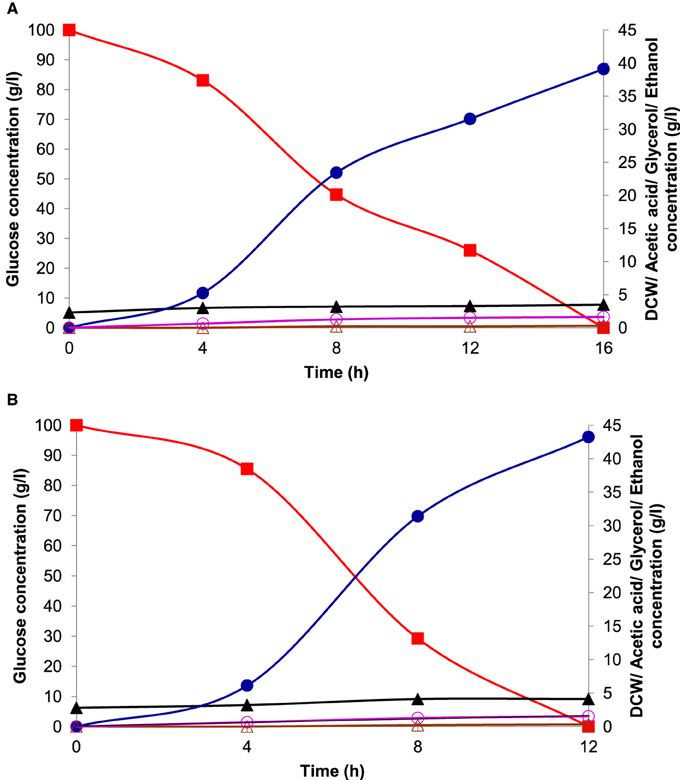
Figure 9. Fermentation profile of (A) K. marxianus NIRE-K1 and (B) K. marxianus NIRE-K3 in bench-scale bioreactor at optimized growth conditions, (■) Glucose; (▴) Dry cell weight; (•) Ethanol; (○) Glycerol; (△) Acetic acid.
The growth and fermentation kinetics of K. marxianus NIRE-K1 and NIRE-K3 were also studied which has been depicted in Table 6. The ethanol concentration (P) and volumetric substrate uptake (QS) obtained with the cells of K. marxianus NIRE-K3 (43.25 ± 0.36 g l−1 and 8.33 ± 0.07 g l−1 h−1) was 9.6 and 24.97% more than that of K. marxianus NIRE-K1 cells (39.12 ± 0.34 g l−1 and 6.25 ± 0.028 g l−1 h−1). The ethanol yield (YP∕S = 0.43 ± 0.05 g g−1) and volumetric product productivity (QP = 3.6 ± 0.11 g l−1 h−1) obtained with K. marxianus NIRE-K3 cells was found to be 9.3 and 31.94%, respectively, higher than that of YP∕S (0.39 ± 0.12 g g−1) and QP (2.45 ± 0.17 g l−1 h−1) of K. marxianus NIRE-K1 cells. Likewise, the final biomass concentration (X = 4.1 g l−1) and sugar to ethanol conversion rate (86.5 ± 0.34%) with the K. marxianus NIRE-K3 cells was 14.63 and 9.55% more than that of K. marxianus NIRE-K1 cells. However, specific product formation rate (qp = 0.798 ± 0.06 g g−1 h−1) and the specific sugar consumption rate (qs = 1.85 ± 0.09 g g−1 h−1) in the case of K. marxianus NIRE-K3 cells was considerably 5.9 and 14.75% lower than the K. marxianus NIRE-K1 cells (qp = 0.848 ± 0.05 g g−1 h−1 and qs = 2.17 ± 0.19 g g−1 h−1), which is useful during product separation and purification process (Behera et al., 2010). The isolates K. marxianus NIRE-K1 and NIRE-K3 showed 5.13 and 9.3% higher ethanol yield with optimized parameters as compared to without optimized one (YP∕S = 0.37 ± 0.05 and 0.39 ± 0.07 g g−1 in case on K. marxianus NIRE-K1 and NIRE-K3).
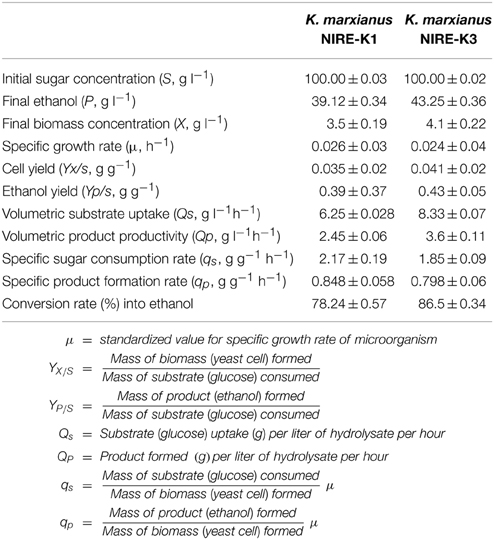
Table 6. Growth and fermentation kinetics of K. marxianus NIRE-K1 and NIRE-K3 at optimized conditions.
Several researchers fermented various types of sugars for the production of ethanol using different isolated yeast strains. Krishnan et al. (1999) reported an ethanol concentration of 47.9 g l−1 with 0.46 g g−1 ethanol yield after 36 h of incubation period by using recombinant Saccharomyces 1400 (pLNH33), which are comparable to the present study. This shows the efficiency of the isolates K. marxianus NIRE-K1 and NIRE-K3, which was comparable to Saccharomyces 1400 (pLNH33). In this study, specific growth rate of yeast on glucose-xylose mixture was found to lie between the specific growth rate on glucose and specific growth rate on xylose. Similarly, Behera et al. (2014c) carried out anaerobic fermentation of both glucose and xylose sugar using newly isolated NIRE-GX1 yeast at 40°C temperature which showed 7.1 ± 0.6 g l−1 maximum ethanol concentration with complete utilization of glucose (20 g l−1) in 24 h of incubation period. However, the strain was capable of simultaneously using glucose and xylose in a mixture of glucose concentration of 14 g l−1 and xylose concentration of 6 g l−1, achieving maximum ethanol and xylitol concentration of 5.3 ± 0.5 g l−1 and 0.95 ± 0.32 g l−1, respectively in 72 h fermentation time. In the present study, both the isolates K. marxianus NIRE-K1 and NIRE-K3 are capable of growing and fermenting in the presence of both glucose and xylose. Also, Kumar et al. (2009b) isolated a yeast strain Kluyveromyces sp. IIPE453 (MTCC 5314) from soil samples collected from dumping sites of crushed sugarcane bagasse in Sugar Mill, showing growth and fermentation efficiency at high temperatures ranging from 45 to 50°C. In batch fermentation, the strain showed maximum ethanol concentration of 82 ± 0.5 g l−1 (10.4% v/v) on initial glucose concentration of 200 g l−1, and ethanol concentration of 1.75 ± 0.05 g l−1 as well as xylitol concentration of 11.5 ± 0.4 g l−1 on initial xylose concentration of 20 g l−1 at 50°C of temperature. This study showed the efficiency of the Kluyveromyces sp. IIPE453 (MTCC 5314) for the utilization of both the sugars for the production of ethanol and xylitol. The use of K. marxianus NIRE-K1 and NIRE-K3 in the present study have also signified in aerobic production of xylitol (data not shown). Tanimura et al. (2012) isolated a yeast strain ATY839, which showed 99.5% identity to that of Candida shehatae was capable of producing a substantial amount of ethanol with 71.6% yield at 37°C temperature using 2% glucose or xylose sugar. The use of both the isolates K. marxianus NIRE-K1 and NIRE-K3 may have profound effect on economics of the process due to their thermotolerant nature. In ethanol production, cooling costs have great effect, which makes the process expensive. Hence, by using these thermotolerant yeasts, cooling and distillation costs can be reduced during process development. Besides, higher saccharification and fermentation rates, continuous ethanol removal and reduced contamination have stimulated a search for routes to thermotolerant yeasts. Therefore, thermotolerant microorganisms that are able to ferment both glucose and xylose are required for efficient bioconversion of biomass to ethanol which could overcome to the limitations of well-known ethanologens such as Saccharomyces cerevisiae or Zymomonas mobilis due to their metabolic inefficiency.
Conclusion
The screened and characterized thermotolerant isolates K. marxianus NIRE-K1 and NIRE-K3 has a great potential for bioethanol production in this context. The optimization of growth conditions w.r.t. temperature and pH using FCCD could increase ethanol yields to 0.39 and 0.43 g g−1 using K. marxianus NIRE-K1 and K3 yeast, respectively. The experimental results indicate that the pH and temperature exert significant effects on growth and bioethanol production yields. However, further studies on the physiology of the isolates using lignocellulosic hydrolysate, effect of fermentation inhibitors and metabolic flux analysis is required for the process development to exploit the potential of these isolates at commercial scale.
Notations
μ Specific growth rate (h−1)
μmaxMaximum specific growth rate (h−1)
S Rate limiting substrate concentration (g l−1)
Ks Saturation constant or half velocity constant or substrate utilization constant (g l−1)
P Final ethanol concentration (g l−1)
X Final biomass concentration (g l−1)
Yx∕s Cell yield (g g−1)
Yp∕s Ethanol yield (g g−1)
Qs Volumetric substrate uptake (g l−1h−1)
Qp Volumetric product productivity (g l−1h−1)
qs Specific sugar consumption rate (g g−1 h−1)
qp Specific product formation rate (g g−1 h−1)
Conflict of Interest Statement
The authors declare that the research was conducted in the absence of any commercial or financial relationships that could be construed as a potential conflict of interest.
Acknowledgments
We thank Prof. Y. K. Yadav, Director General, SSS-NIBE, Kapurthala, India for his valuable suggestions and encouragement to carry out this research work. We gratefully acknowledge the Ministry of New and Renewable Energy, Govt. of India for providing funds to carry out the research activities. The authors also acknowledge MTCC, Chandigarh for identification of the screened isolates. One of the authors (RA) also acknowledges I.K. Gujral Punjab Technical University, Kapurthala, India for her Ph.D. registration (Pro. Reg. No. 1315010).
References
Adnan, N. A. A., Suhaimi, S. N., Abd-aziz, S., Hassan, M. A., and Phang, L.-Y. (2014). Optimization of bioethanol production from glycerol by Escherichia coli SS1. Renew. Energy 66, 625–633. doi: 10.1016/j.renene.2013.12.032
Arora, R., Behera, S., and Kumar, S. (2015a). Bioprospecting thermophilic/thermotolerant microbes for production of lignocellulosic ethanol: a future perspective. Renew. Sust. Energy Rev. 51, 699–717. doi: 10.1016/j.rser.2015.06.050
Arora, R., Behera, S., Sharma, N. K., and Kumar, S. (2015b). Bioprospecting thermostable cellulosomes for efficient biofuel production from lignocellulosic biomass. Bioresour. Bioprocess. 2:38. doi: 10.1186/s40643-015-0066-4
Arora, R., Behera, S., Sharma, N. K., Singh, R., Yadav, Y. K., and Kumar, S. (2014). “Biochemical conversion of rice straw (Oryza sativa L.) to bioethanol using thermotolerant isolate K. marxianus NIRE-K3,” in Proceedings of Exploring & Basic sciences for Next Generation Frontiers, eds N. R. Sharma, R. C. Thakur, M. Sharma, L. Parihar, and G. Kumar (New Delhi: Elsevier), 143–146.
Arroyo-López, F. N., Orlic, S., Querol, A., and Barrio, E. (2009). Effects of temperature, pH and sugar concentration on the growth parameters of Saccharomyces cerevisiae, S. kudriavzevii and their interspecific hybrid. Int. J. Food Microbiol. 131, 120–127. doi: 10.1016/j.ijfoodmicro.2009.01.035
Bailey, J. E., and Ollis, D. F. (1986). Biochemical Engineering Fundamentals. New York, NY: McGraw-Hill.
Bas, D., and Boyaci, I. H. (2007). Modeling and optimization I: usability of response surface methodology. J. Food Eng. 78, 836–845. doi: 10.1016/j.jfoodeng.2005.11.024
Behera, S., Mohanty, R. C., and Ray, R. C. (2010). Ethanol fermentation of mahula (Madhuca latifolia L.) flowers using free and immobilized bacteria Zymomonas mobilis MTCC 92. Biologia 65, 416–421. doi: 10.2478/s11756-010-0041-7
Behera, S., Mohanty, R. C., and Ray, R. C. (2011). Ethanol production from mahula (Madhuca latifolia L.) flowers with immobilized cells of Saccharomyces cerevisiae in Luffa cylindrica L. sponge discs. Appl. Energy 88, 212–215. doi: 10.1016/j.apenergy.2010.07.035
Behera, S., and Ray, R. C. (2012). Comparison of Luffa cylindrica L. sponge discs and Ca-alginate gel beads as immobilized matrices of Saccharomyces cerevisiae for bio-ethanol production from sugarcane molasses. Afr. J. Biotechnol. 11, 16172–16176. doi: 10.5897/AJB12.830
Behera, S., Mohanty, R. C., and Ray, R. C. (2014a). Batch ethanol production from cassava (Manihot esculenta Crantz.) flour using Saccharomyces cerevisiae cells immobilized in calcium alginate. Ann. Microbiol. 65, 779–783. doi: 10.1007/s13213-014-0918-8
Behera, S., Arora, R., Nandhagopal, N., and Kumar, S. (2014b). Importance of chemical pretreatment for bioconversion of lignocellulosic biomass. Renew. Sust. Energy Rev. 36, 91–106. doi: 10.1016/j.rser.2014.04.047
Behera, S., Arora, R., Sharma, N. K., and Kumar, S. (2014c). “Fermentation of glucose and xylose sugar for the production of ethanol and xylitol by the newly isolated NIRE-GX1 yeast,” in Recent Advances in Bio-energy Research, Vol. 3, eds S. Kumar, A. K. Sarma, S. K. Tyagi, and Y. K. Yadav (Kapurthala: SSS-NIRE), 175–182.
Cadete, R. M., Santos, R. O., Melo, M. A., Mouro, A., and Goncalves, D. L. (2009). Spathaspora arborariae sp. nov., a D-xylose-fermenting yeast species isolated from rotting wood in Brazil. FEMS Yeast Res. 9, 1338–1342. doi: 10.1111/j.1567-1364.2009.00582.x
Charoenchai, C., Fleet, G., and Henschke, P. A. (1998). Effects of temperature, pH and sugar concentration on the growth rates and cell biomass of wine yeasts. Am. J. Enol. Vitic. 49, 283–288.
Chauhan, B., and Gupta, R. (2004). Application of statistical experimental design for optimization of alkaline protease production from Bacillus sp. RGR-14. Process Biochem. 39, 2115–2122. doi: 10.1016/j.procbio.2003.11.002
Cimpeanu, C., Campeanu, G., Begea, M., Vladescu, M., and Cornea, C. P. (2010). Bioethanol production by new thermotolerant Romanian yeast strains. Rom. Biotechnol. Lett. 15, 5310–5316.
Dasgupta, D., Suman, S. K., Pandey, D., Ghosh, D., Khan, R., Agrawal, D., et al. (2013). Design and optimization of ethanol production from bagasse pith hydrolysate by a thermotolerant yeast Kluyveromyces sp. IIPE453 using response surface methodology. SpringerPlus 2:159. doi: 10.1186/2193-1801-2-159
Dhaliwal, S. S., Oberoi, H. S., Sandhu, S. K., Nanda, D., Kumar, D., and Uppal, S. K. (2011). Enhanced ethanol production from sugarcane juice by galactose adaptation of a newly isolated thermotolerant strain of Pichia kudriavzevii. Bioresour. Technol. 102, 5968–5975. doi: 10.1016/j.biortech.2011.02.015
Dung, N. T. P., Thanonkeo, P., and Phong, H. X. (2012). Screening useful isolated yeasts for ethanol fermentation at high temperature. Int. J. Appl. Sci. Technol. 2, 65–71.
Eiadpum, A., Limtong, S., and Phisalaphong, M. (2012). High-temperature ethanol fermentation by immobilized coculture of Kluyveromyces marxianus and Saccharomyces cerevisiae. J. Biosci. Bioeng. 3, 325–329. doi: 10.1016/j.jbiosc.2012.04.004
El-Gendy, N. S., Madian, H. R., and Abu Amr, S. S. (2013). Design and optimization of a process for sugarcane molasses fermentation by Saccharomyces cerevisiae using Response surface Methodology. Int. J. Microbiol. 2013:815631. doi: 10.1155/2013/815631
Hashem, M., Zohri, A. N. A., and Ali, M. M. A. (2013). Optimization of the fermentation conditions for ethanol production by new thermotolerant yeast strains of Kluyveromyces sp. Afr. J. Microbiol. Res. 7, 4550–4561. doi: 10.5897/AJMR2013.5919
Jeffries, T. W. (2006). Engineering yeast for xylose metabolism. Curr. Opin. Biotechnol. 17, 320–326. doi: 10.1016/j.copbio.2006.05.008
Kaewkrajay, C., Dethoup, T., and Limtong, S. (2014). Ethanol production from cassava using a newly isolated thermotolerant yeast strain. ScienceAsia 40, 268–277. doi: 10.2306/scienceasia1513-1874.2014.40.268
Krishnan, M. S., Ho, N. W., and Tsao, G. T. (1999). Fermentation kinetics of ethanol production from glucose and xylose by recombinant Saccharomyces 1400 (pLNH33). Appl. Biochem. Biotechnol. 77–79, 373–388. doi: 10.1385/ABAB:78:1-3:373
Kumar, S., Singh, S. P., Mishra, I. M., and Adhikari, D. K. (2009a). Recent advances in production of bioethanol from lignocellulosic biomass. Chem. Eng. Technol. 32, 517–526. doi: 10.1002/ceat.200800442
Kumar, S., Singh, S. P., Mishra, I. M., and Adhikari, D. K. (2009b). Ethanol and xylitol production from glucose and xylose at high temperature by Kluyveromyces sp. IIPE453. J. Ind. Microbiol. Biotechnol. 36, 1483–1489. doi: 10.1007/s10295-009-0636-6
Kumar, S., Dheeran, P., Singh, S. P., Mishra, I. M., and Adhikari, D. K. (2013). Cooling system economy in ethanol production using thermotolerant yeast Kluyveromyces sp. IIPE453. Am. J. Microbiol. Res. 1, 39–44. doi: 10.12691/ajmr-1-3-1
Kumar, S., Dheeran, P., Singh, S. P., Mishra, I. M., and Adhikari, D. K. (2015). Bioprocessing of bagasse hydrolysate for ethanol and xylitol production using thermotolerant yeast. Bioprocess. Biosyst. Eng. 38, 39–47. doi: 10.1007/s00449-014-1241-2
Kwon, Y. J., Ma, A. Z., Li, Q., Wang, F., Zhuang, G. Q., and Liu, C. Z. (2011). Effect of lignocellulosic inhibitory compounds on growth and ethanol fermentation of newly-isolated thermotolerant Issatchenkia orientalis. Bioresour. Technol. 102, 8099–8104. doi: 10.1016/j.biortech.2011.06.035
Levin, L., Herrmann, C., and Papinutti, V. L. (2008). Optimization of lignocellulolytic enzyme production by white rot fungus Trametes trogii in solid state fermentation using response surface methodology. Biochem. Eng. J. 39, 207–214. doi: 10.1016/j.bej.2007.09.004
Lorliam, W., Akaracharanya, A., Jindamorakot, S., Suwannarangsee, S., and Tanasupawat, S. (2013). Characterization of xylose-utilizing yeasts isolated from herbivore faeces in Thailand. ScienceAsia 39, 26–35. doi: 10.2306/scienceasia1513-1874.2013.39.026
Ma, M., and Liu, Z. L. (2010). Mechanisms of ethanol tolerance in Saccharomyces cerevisiae. Appl. Microbiol. Biotechnol. 87, 829–845. doi: 10.1007/s00253-010-2594-3
Man, H. L., Behera, S. K., and Park, H. S. (2010). Optimization of operational parameters for ethanol production from Korean food waste leachate. Int. J. Environ. Sci. Technol. 7, 157–164. doi: 10.1007/BF03326127
Manikandan, K., and Viruthagiri, T. (2010). Kinetic and optimization studies on ethanol production from corn flour. Int. J. Chem. Biol. Eng. 3, 850–854. doi: 10.1155/2013/815631
Manivannan, A., and Narendhirakannan, R. T. (2014). Biodegradation of lignocellulosic residues of water hyacinth (Eichhornia crassipes) and response surface methodological approach to optimize bioethanol production using fermenting yeast Pachysolen tannophilus NRRL Y-2460. Int. J. Biol. Biomol. Agric. Food Biotechnol. Eng. 8, 153–158.
McMeckin, T. A., Olley, J., Ratkwsky, D. A., and Ross, T. (2002). Predictive microbiology: towards the interface and beyond. Int. J. Food Microbiol. 73, 395–407. doi: 10.1016/S0168-1605(01)00663-8
Narayanaswamy, N., Dheeran, P., Verma, S., and Kumar, S. (2013). “Biological pretreatment of lignocellulosic biomass for enzymatic saccharification,” in Pretreatment Techniques for Biofuels and Biorefineries, ed Z. Fang (Berlin; Heidelberg: Springer Verlag), 3–34. doi: 10.1007/978-3-642-32735-3_1
Nitiyon, S., Boonmak, C., Am-In, S., Jindamorakot, S., Kawasaki, H., Yongmanitchai, W., et al. (2011). Candida saraburiensis sp. nov. and Candida prachuapensis sp. nov., xylose-utilizing yeast species isolated in Thailand. Int. J. Syst. Evol. Microbiol. 61, 462–468. doi: 10.1099/ijs.0.023317-0
Ohtani, K. (2000). Bootstrapping R2 and adjusted R2 in regression analysis. Econ. Model. 17, 473–483. doi: 10.1016/S0264-9993(99)00034-6
Olofsson, K., Bertilsson, M., and Lidén, G. (2008). A short review on SSF–an interesting process option for ethanol production from lignocellulosic feedstocks. Biotechnol. Biofuels 1:7. doi: 10.1186/1754-6834-1-7
Orlygsson, J. (2012). Ethanol production from biomass by a moderate thermophile, Clostridium AK1. Icel. Agric. Sci. 25, 25–35.
Pakarinen, A., Maijala, P., Stoddard, F. L., Santanen, A., Tuomainen, P., Kymalainen, M., et al. (2011). Evaluation of annual bioenergy crops in the boreal zone for biogas and ethanol production. Biomass Bioenergy 35, 3071–3078. doi: 10.1016/j.biombioe.2011.04.022
Peña, A., Cinco, G., Gómez-Puyou, A., and Tuena, M. (1972). Effect of the pH of the incubation medium on glycolysis and respiration in Saccharomyces cerevisiae. Arch. Biochem. Biophys. 153, 413–425. doi: 10.1016/0003-9861(72)90359-1
Phisalaphong, M., Srirattana, N., and Tanthapanichakoon, W. (2005). Mathematical modeling to investigate temperature effect on kinetic parameters of ethanol fermentation. Biochem Eng. J. 28, 36–43. doi: 10.1016/j.bej.2005.08.039
Prakash, O., Talat, M., Hasan, S. H., and Pandey, R. K. (2008). Factorial design for the optimization of enzymatic detection of cadmium in aqueous solution using immobilized urease from vegetable waste. Bioresour. Technol. 99, 7565–7572. doi: 10.1016/j.biortech.2008.02.008
Rene, E. R., Jo, M. S., Kim, S. H., and Park, H. S. (2007). Statistical analysis of main and interaction effects during the removal of BTEX mixtures in batch conditions, using wastewater treatment plant sludge microbes. Int. J. Environ. Sci. Technol. 4, 177–182. doi: 10.1007/BF03326271
Riyanti, E. I., and Rogers, P. L. (2009). Kinetic evaluation of ethanol-tolerant thermophile Geobacillus thermoglucosidasius M10EXG for ethanol production. Indonesian J. Agric. Sci. 10, 34–41.
Scully, S. M., and Orlygsson, J. (2015). Recent advances in second generation ethanol production by thermophilic bacteria. Bioenergies 8, 1–30. doi: 10.3390/en8010001
Serra, A., Strehaiano, P., and Taillandier, P. (2005). Influence of temperature and pH on Saccharomyces bayanus var. uvarum growth; impact of a wine yeast interspecific hybridization on these parameters. Int. J. Food Microbiol. 104, 257–265. doi: 10.1016/j.ijfoodmicro.2005.03.006
Sigurbjornsdottir, M. A., and Orlygsson, J. (2012). Combined hydrogen and ethanol production from sugars and lignocellulosic biomass by Thermoanaerobacterium AK54, isolated from hot spring. Appl. Energy 97, 785–791. doi: 10.1016/j.apenergy.2011.11.035
Singh, A., Tuteja, S., Singh, N., and Bishnoi, N. R. (2011). Enhanced saccharification of rice straw and hulls by microwave-alkali pretreatment and lignocellulolytic enzyme production. Bioresour. Technol. 102, 1773–1782. doi: 10.1016/j.biortech.2010.08.113
Singh, A., and Bishnoi, N. R. (2012). Optimization of ethanol production from microwave alkali pretreated rice straw using statistical experimental designs by Saccharomyces cerevisiae. Ind. Crop. Prod. 37, 334–341. doi: 10.1016/j.indcrop.2011.12.033
Stanley, D., Bandara, A., Fraser, S., Chambers, P. J., and Stanley, G. A. (2010). The ethanol stress response and ethanol tolerance of Saccharomyces cerevisiae. J. Appl. Microbiol. 109, 13–24. doi: 10.1111/j.1365-2672.2009.04657.x
Tanimura, A., Nakamura, T., Watanabe, I., Ogawa, J., and Jun, S. (2012). Isolation of a novel strain of Candida shehatae for ethanol production at elevated temperature. Springer Plus 1:27. doi: 10.1186/2193-1801-1-27
Tofighi, A., Assadi, M. M., Asadirad, M. H. A., and Karizi, S. Z. (2014). Bio-ethanol production by a novel autochthonous thermo-tolerant yeast isolated from wastewater. J. Environ. Health Sci. Eng. 12, 1–6. doi: 10.1186/2052-336X-12-107
Tomás, A. F., Karagöz, P., Karakashev, D., and Angelidaki, I. (2013). Extreme thermophilic ethanol production from rapeseed straw: using the newly isolated Thermoanaerobacter pentosaceus and combining it with Saccharomyces cerevisiae in a two-step process. Biotechnol. Bioeng. 110, 1574–1582. doi: 10.1002/bit.24813
Torija, M. J., Rozès, N., Poblet, M., Guillamón, J. M., and Mas, A. (2003). Effects of fermentation temperature on the strain population of Saccharomyces cerevisiae. Int. J. Food Microbiol. 80, 47–53. doi: 10.1016/S0168-1605(02)00144-7
Udhayaraja, P., and Sriman, J. S. (2012). Optimization for production of bioethanol using Sorghum stovar by Saccharomyces cerevisiae. Int. J. Res. Pure Appl. Microbiol. 2, 64–67.
Uncu, O. N., and Cekmecelioglu, D. (2011). Cost-effective approach to ethanol production and optimization by response surface methodology. Waste Manage. 31, 636–643. doi: 10.1016/j.wasman.2010.12.007
Viikari, L., Vehmaanpera, J., and Koivula, A. (2012). Lignocellulosic ethanol: from science to industry. Biomass Bioenergy 46, 13–24. doi: 10.1016/j.biombioe.2012.05.008
Yuangsaard, N., Yongmanitchai, W., Yamada, M., and Limtong, S. (2013). Selection and characterization of a newly isolated thermotolerant Pichia kudriavzevii strain for ethanol production a high temperature from cassava starch hydrolysate. Antonie Van Leeuwenhoek 103, 577–588. doi: 10.1007/s10482-012-9842-8
Keywords: thermotolerant yeast, optimization, face-centered central composite design, bioethanol production, glucose, xylose
Citation: Arora R, Behera S, Sharma NK and Kumar S (2015) A new search for thermotolerant yeasts, its characterization and optimization using response surface methodology for ethanol production. Front. Microbiol. 6:889. doi: 10.3389/fmicb.2015.00889
Received: 20 June 2015; Accepted: 17 August 2015;
Published: 01 September 2015.
Edited by:
Vijai Kumar Gupta, National University of Ireland Galway, IrelandReviewed by:
Dayananda Chandrappa, University of Exeter, UKAnuj K. Chandel, Centro de Tecnologia Canavieviera, Brazil
Copyright © 2015 Arora, Behera, Sharma and Kumar. This is an open-access article distributed under the terms of the Creative Commons Attribution License (CC BY). The use, distribution or reproduction in other forums is permitted, provided the original author(s) or licensor are credited and that the original publication in this journal is cited, in accordance with accepted academic practice. No use, distribution or reproduction is permitted which does not comply with these terms.
*Correspondence: Sachin Kumar, Biochemical Conversion Division, Sardar Swaran Singh National Institute of Bio-Energy, Jalandhar-Kapurthala Road, Wadala Kalan, Kapurthala-144601, Punjab, India, sachin.biotech@gmail.com
†These authors have contributed equally to this work.