- 1INRA, UMR 1347 Agroécologie, Dijon, France
- 2INRA, UR 1158 AgroImpact, Laon, France
Agriculture is the main source of terrestrial emissions of N2O, a potent greenhouse gas and the main cause of ozone layer depletion. The reduction of N2O into N2 by microorganisms carrying the nitrous oxide reductase gene (nosZ) is the only biological process known to eliminate this greenhouse gas. Recent studies showed that a previously unknown clade of N2O-reducers was related to the capacity of the soil to act as an N2O sink, opening the way for new strategies to mitigate emissions. Here, we investigated whether the agricultural practices could differently influence the two N2O reducer clades with consequences for denitrification end-products. The abundance of N2O-reducers and producers was quantified by real-time PCR, and the diversity of both nosZ clades was determined by 454 pyrosequencing. Potential N2O production and potential denitrification activity were used to calculate the denitrification gaseous end-product ratio. Overall, the results showed limited differences between management practices but there were significant differences between cropping systems in both the abundance and structure of the nosZII community, as well as in the [rN2O/r(N2O+N2)] ratio. More limited differences were observed in the nosZI community, suggesting that the newly identified nosZII clade is more sensitive than nosZI to environmental changes. Potential denitrification activity and potential N2O production were explained mainly by the soil properties while the diversity of the nosZII clade on its own explained 26% of the denitrification end-product ratio, which highlights the importance of understanding the ecology of this newly identified clade of N2O reducers for mitigation strategies.
Introduction
Nitrous oxide (N2O) is one of the six gases subject to restriction by the Kyoto Protocol, which aims at reducing anthropogenic greenhouse gas (GHG) emissions. N2O is both directly and indirectly of importance for the Earth's climate. Firstly, it is a potent greenhouse gas with a long life time of 110 years and a global warming potential 298 times that of carbon dioxide on a 100-year time scale and per unit of weight. Thus, N2O is the third most important GHG contributing to about 10% of annual global warming (Bates et al., 2008; Thomson et al., 2012). Secondly, after the success of the Montreal Protocol for phasing out chlorofluorocarbons (CFCs), N2O is today the dominant ozone-depleting substance (Ravishankara et al., 2009). The atmospheric concentration of N2O has been rising over the past 100 years resulting in a concentration 19% higher than pre-industrial levels (Montzka et al., 2011) with an estimated increase of N2O emissions of up to 60% by 2050 (relative to 1900 values) (Bouwman et al., 2013).
N2O emissions are, to a great extent, the result of microbial processes such as nitrification and denitrification. However, denitrification is also the only known sink for N2O. Denitrification is a microbial respiratory pathway through which soluble forms of nitrogen, i.e., nitrate (NO) and nitrite (NO), are sequentially transformed into NO, N2O and N2 gases via four enzymatic systems (Tiedje et al., 1982; Zumft, 1997). The reduction of soluble NO into NO and N2O is catalyzed by copper- or cd1- nitrite reductases and nitric oxide reductases, respectively (Zumft, 2005). Nitrous oxide reductase, whose catalytic subunit is encoded by the nosZ gene, is the last enzyme of the pathway. It converts the GHG N2O into inert N2, which accounts for 78% of the atmospheric gases, and is, therefore, the key enzyme involved in the N2O sink. It is now recognized that denitrification is a modular process (Graf et al., 2014). Thus, while some microorganisms harbor all denitrification enzymes and can potentially perform the complete pathway, others either lack the nitrous oxide reductase gene and produce only N2O as the denitrification end product (Philippot et al., 2011), or are only able to reduce N2O (Sanford et al., 2002).
Recent studies have identified a previously undescribed nosZ clade, herein after named nosZII, which is diverse and widespread in the environment (Sanford et al., 2012; Jones et al., 2013; Orellana et al., 2014). Genome analyses showed that an important fraction of the microorganisms that possess this nosZII gene also harbor a highly truncated version of the denitrification pathway without any nitrite reductase or N2O-producing nitric oxide reductase, and, therefore, can only consume N2O (Graf et al., 2014). The abundance and phylogenetic diversity of nosZII microorganisms was found to mediate the soil N2O sink capacity in European soils (Jones et al., 2014), showing the importance of understanding the ecology of this microbial guild for mitigating N2O emissions.
Agriculture accounts for about 60% of all N2O emissions from global anthropogenic sources (Syakila and Kroeze, 2011). A compilation of more than 1215 measurements of N2O emissions from agricultural and natural soils showed that agricultural practices, such as the N application rate, type of crop and type of fertilizer, affected the emissions (Stehfest and Bouwman, 2006). More recently, Shcherbak et al. (2014) reported that the response of soil N2O emissions to nitrogen fertilizer was nonlinear for synthetic fertilizers and most crop types. The effect of agricultural practices has often been described in terms of changes in soil substrates or environmental conditions, which can also affect soil microbial communities in various ways. For example, soil amendment with peat stimulated the relative abundance of the Alphaproteobacteria, but reduced the relative abundance of Firmicutes (Wessen et al., 2010). Organic farming increased richness, decreased evenness and shifted the structure of the soil microbial community when compared with conventionally managed soils amended with mineral fertilizers (Hartmann et al., 2014) Shifts in abundance and structure of the denitrifier community have also been reported in response to the fertilization regime (Hallin et al., 2009; Clark et al., 2012; Tatti et al., 2014) or in response to land use intensity (Meyer et al., 2013). However, very little is known about how soil management could affect microorganisms belonging to the newly described nosZII clade while agricultural practices that foster these microorganisms are of interest for mitigating N2O emissions.
This study was therefore set out to determine how the nosZI and nosZII N2O-reducing communities responded to various agricultural practices in two different arable farming systems. It also assessed the relationships between diversity, composition, and abundance of the N2O-reducing microbial communities and N-gas production (N2O and N2) by denitrification. The study was based on two randomized block experiments localized at the same site, one with an annual rotation with 5 different management practices (ORE) and one with a perennial crop system with 4 different management practices (BE).
Results
Potential N2O Production, Potential Denitrification Activity (N2O+N2) and Denitrification End-product Ratio [rN2O/r(N2O+N2)]
To assess the activity of the N2O reducing microbial communities, the potential N2O production and potential denitrification activity (PDA) were quantified and used to calculate the denitrification end-product ratio [rN2O/r(N2O+N2)]. The potential activity of denitrifying microorganisms varied in all cropping systems, ranging from 0.03 (CI95% = [0− 0.09]) to 0.85 (CI95% = [0.79−0.91]) and 0.17 (CI95% = [0.07−0.27]) to 1.51 (CI95% = [1.41−1.61]) μg N2O-N g−1 soil DW h−1 for potential N2O and PDA, respectively (Figures 1A,B). The [rN2O/r(N2O+N2)] ratio ranged between 0.18 (CI95% = [0.08–0.28]) and 1 (CI95% = [0.9–1.1]) (Figure 1C) and was significantly higher (P < 0.001) for BE than for ORE cropping system with an average of 0.65 (CI95% = 0.81–0.48) and 0.29 (CI95% = 0.24–0.34), respectively. There were significant differences in [rN2O/r(N2O+N2)] between the early (ME) and late harvest (ML) practices for plots planted with Miscanthus giganteus (P < 0.05) (Figure 1B) with the denitrification end product being mainly N2O in the early harvested plots with a [rN2O/r(N2O+N2)] close to 1 (Figure 1C). For switchgrass, there was the same tendency to have a higher [rN2O/r(N2O+N2)] with the early harvest practice, although this was not significant.
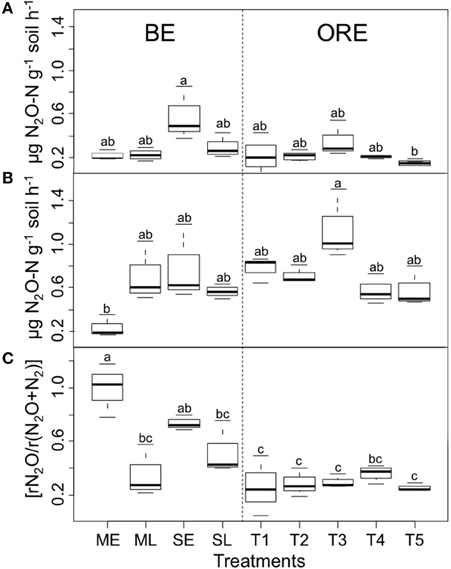
Figure 1. Potential N2O emissions (A), potential denitrification activity (B) and denitrification gaseous end-product ratio [rN2O/r(N2O+N2)] (C). Means ± sem per treatments within each experimental block are given. BE and ORE represent the respective cropping systems. Significant differences between treatments are indicated with different letters (anova followed by Tukey HSD test, P < 0.05).
Abundance of Total Bacteria, N2O-producers and N2O-reducers
The genes encoding catalytic enzymes involved in N2O production (nirK and nirS) and N2O reduction (nosZI and nosZII) were quantified by Real-Time quantitative PCR (qPCR) and used as proxies for the abundances of the corresponding functional communities. The 16S rRNA gene copy number, which was used to estimate the abundance of the total bacteria community, varied from 1.3 × 109 to 5.1 × 109 copy numbers g-1 oil DW without any significant difference between treatments (data not shown). The relative abundances of nirS and nirK communities were similar, ranging from 2.5 to 7.6% of the total bacterial community (Figure S1). The nosZI community was significantly (P < 0.05) more abundant than the nosZII community, ranging from 4.9 to 8.5% and 0.28 to 2.9% of the total bacteria, respectively (Figure S1). No significant differences in N2O-producers and N2O-reducers abundances were found between treatments (Figure S1). However, nosZII abundance was higher in BE than in ORE (P < 0.01).
N2O-reducer Diversity
To assess whether the agricultural practices could drive the composition and structure of the N2O-reducer community, the diversity of both nosZI and nosZII clades was characterized by 454 pyrosequencing. 123,130 nosZI and 121,500 nosZII sequences were obtained from samples after quality filtering. Similarity-based clustering of sequences gave an average of 162 (CI95% = 133–191) and 312 (CI95% = 279–345) OTUs for nosZI and nosZII respectively in BE, and 158 (CI95% = 136–180) and 355 (CI95% = 330–380) OTUs for nosZI and nosZII respectively in ORE. In both BE and ORE, the nosZII community had a significantly higher richness than nosZI (P < 0.0001). Within BE and ORE, the agricultural practices had no significant effect on the α-diversity of the N2O-reducing communities (Table S1). An analysis of nosZI and nosZII phylogenies showed that the most abundant sequences in ORE and BE were similar (Figures S2, S3), with nosZII sequences affiliated mainly to nosZ from Bacteroidetes while nosZI sequences were affiliated to nosZ from Alphaproteobacteria and Betaproteobacteria. Further examination of the β-diversity by non-metric multidimensional scaling (NMDS) and analysis of similarity (ANOSIM) showed (Figures 2A,B) no clustering of samples according to the agricultural practices. However, differences in both nosZ communities between annual rotation and perennial cropping systems were significant, but stronger for nosZII communities, (R = 0.43, P < 0.0001 and R = 0.77, P < 0.0001 for nosZI and nosZII respectively). Fitting the environmental variables onto the ordination plot showed that pH and calcium were significant explanatory variables (P<0.05) for the community structure of both guilds. The nosZI community structure was also related to the water content while the abundance of nitrite reductase genes (nirK) and sand content were related to the nosZII community structure (Figure 2B). The [rN2O/r(N2O+N2)] surface was fitted onto the ordination plot and showed a strong relationship between the nosZII community structure, its diversity and the denitrification end products with a lower proportion of N2O produced when the nosZII diversity increased (Figure 2B).
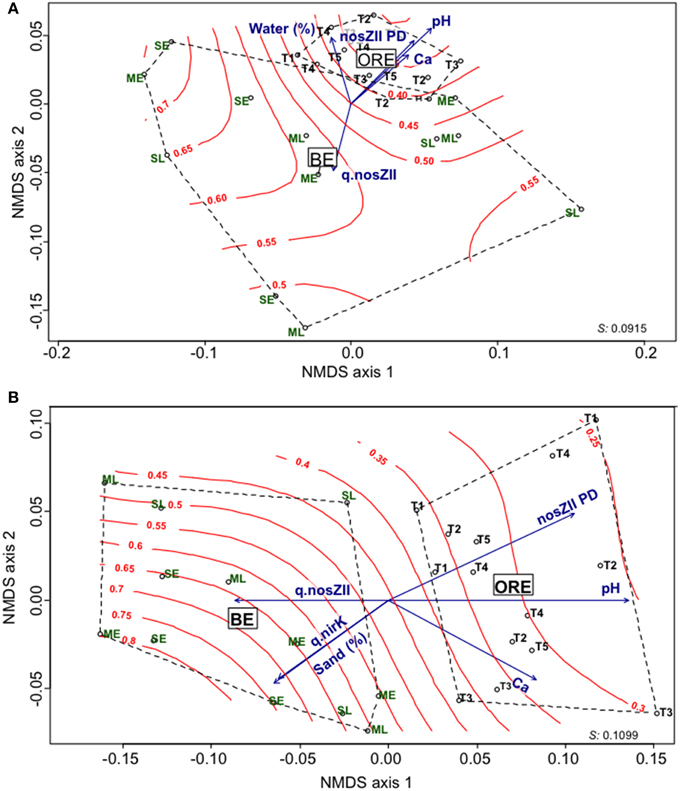
Figure 2. NMDS ordinations of nosZ weighted unifrac distance matrices. (A) Variation in nosZI community structure. (B) Variation in nosZII community structure. Red curves represent the final denitrification product [rN2O/r(N2O/N2)]. Significant explanatory variables are represented as blue vectors (P < 0.05), Ca (calcium g kg−1 dw soil), q.nosZII and q.nirK correspond to the quantification by qPCR of nitrous oxide reductase community (clade II) and copper nitrite reductase community, respectively (copy number g−1 dw soil); sand and water content are expressed in percentage. The lengths of the arrows are proportional to the strength of the correlation. Stress values are indicated at the bottom right of each panel.
Denitrification Activity and End Product Ratio as a Function of Soil Properties, Abundance and Diversity of the N2O-reducing Community
We used variance partitioning technique to quantify the relative contribution of the different groups of variables to the variation in N2 production by denitrification across samples (Figures 3A–D). The physical and chemical characteristics of the soil, the abundance of N2O producers and reducers, and the diversity of N2O reducers were used as explanatory variables. After model selection using multiple linear regressions (Table S2), the physical and chemical properties of the soil were found to be the variables that contributed most to the potential N2O production and PDA, explaining up to 29 and 45% of the variance, respectively (Figures 3B,C). In contrast, the [rN2O/r(N2O+N2)] was mostly explained by the diversity of the N2O-reducers (26%). Interactions between physical and chemical properties of the soil and the diversity of the N2O-reducing communities accounted for 26 and 17% of potential N2O production and [rN2O/r(N2O+N2)], respectively (Figures 3B,D). The importance of nosZII diversity for the end product ratio of denitrification was also suggested by the strong negative correlation between the (rN2O/r(N2O+N2)) and the nosZII diversity (r = -0.70, P < 0.0001) (Figure S4). The abundance of the communities studied made only a marginal contribution, explaining 2% of the variance in [rN2O/r(N2O+N2)].
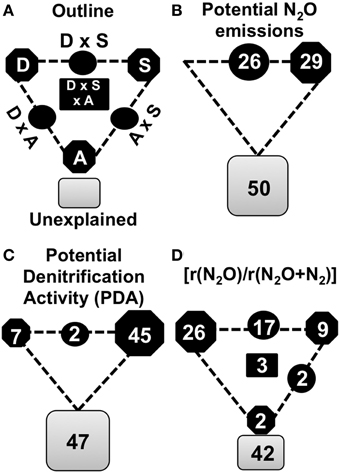
Figure 3. Variation partitioning of denitrification activities. (A) Variance in denitrification activities was partitionned into nosZ diversity (D), soil physicochemical properties (S), denitrifiers abundance (A) and by combinations of predictors. Geometric areas are proportional to the respective percentages of explained variation. The edges of the triangle depict the variation explained by each factor alone. Percentages of variation explained by interactions of two or all factors are indicated on the sides and in the middle of the triangles, respectively. (B) Variance partitioning of potential N2O emissions. (C) Variance partitioning of potential denitrification activity (PDA). (D) Variance partitioning of final denitrification product [r(N2O/r(N2O+N2)]. The variables used for each variation partitioning are indicated in the Table S2.
Discussion
The recent identification of a previously unknown clade of N2O reducers (Sanford et al., 2012; Jones et al., 2013), whose abundance and phylogenetic diversity are critical for the soil N2O sink capacity (Jones et al., 2014), raised the question of whether it would be possible to mitigate N2O GHG emissions by selecting agricultural practices that favor these microorganisms. A large body of literature shows that land management can drive microbial communities (Clark et al., 2012; Lauber et al., 2013; Hartmann et al., 2014). Here, our intent was not to compare the impact of individual agricultural practices between the two cropping systems but rather to assess how practices for a particular system, such as tillage, residue management, quantity and nature of N inputs in ORE and biomass crop species and harvest date in BE, affect N2O-reducing communities and N2 production. We did not observe any significant effect of the practices for either BE or ORE on either the diversity or the abundance of the N2O-reducing communities (Table S1 and Figure S1). In contrast, previous results showed differences in the denitrifier community under different fertilization regimes (Hallin et al., 2009) or tillage systems (Melero et al., 2011). Significant effects of the cropping systems on the total bacterial communities were also reported (Hartmann et al., 2014). One common feature of these studies is that they were based on long term experiments that had been running for up to 50 years. This discrepancy with our results could be due to the fact that the ORE and BE systems were established only in 2010 and 2006, respectively, which has not allowed a strong differentiation in soil properties between practices within a cropping system (Table 1). On the other hand, the comparison of ORE and BE did show differences in the N2O reducing communities with a higher nosZII abundance for ORE than for BE (P < 0.01). NosZII community richness estimated as OTU numbers was also significantly higher in ORE than in BE (Table S1). Furthermore, NMDS also showed significant clustering according to perennial and annual rotation cropping systems for nosZII and to a lesser extent for nosZI (Figures 2A,B). Since it is not possible to distinguish the effect of practices from soil legacy or age effects between ORE and BE, it cannot be concluded that the observed changes in the nosZII community were due to differences in agricultural practices. However, the stronger response of the nosZII community structure and abundance indicates that this recently identified clade is more sensitive to environmental changes than the nosZI clade and is therefore more likely to be driven by the land use type.
The agricultural practices studied also had little impact on PDA and N2O production. However, larger differences were observed when calculating the denitrification gaseous end-product ratio (rN2O/r(N2O+N2)) with, in particular, early harvest of M. giganteus giving a significantly higher proportion of N2O than late harvest. Previous studies at BE showed that late harvest of M. giganteus gave a significant net input and accumulation of organic matter due to senescence and leaf fall between early and late harvest (Amougou et al., 2011, 2012; Cadoux et al., 2014). This type of mulch is known to improve soil moisture by reducing soil surface evaporation, resulting in a lower partial pressure of oxygen (pO2). This is consistent with our results since previous studies have shown that production of N2O relative to N2 during denitrification in soils is strongly influenced by carbon availability and pO2 (Firestone et al., 1980; Murray and Knowles, 2004; Walker et al., 2008; Giles et al., 2012; Saggar et al., 2013) with, for example decreasing proportion of N2O produced during denitrification with decreasing O2 concentrations (Firestone et al., 1980). However, the harvesting date had no significant effect on the denitrification gaseous end-product ratio for switchgrass. This might be due to the fact there is no leaf deposition between late and early harvest for switchgrass. Similarly, to the N2O reducer community structure, significant differences in the N2O ratio were observed between the BE and ORE cropping systems with a lower end-product ratio in ORE (P < 0.001). In agreement with previous results (Jones et al., 2014), a strong negative correlation was found between the (rN2O/r(N2O+N2)) ratio and nosZII diversity (r = -0.70, P < 0.0001) (Figure S4), highlighting the importance of nosZII N2O reducers in the N2O-reducing capacity of a soil.
We found that the abundance of nosZII N2O reducers was significantly correlated with several soil properties such as pH, Ca concentration, soil moisture and total N. This was not the case for nosZI which was correlated solely with the C:N ratio, confirming that the nosZII clade is more sensitive to changes in environmental conditions than the nosZI clade. The analysis of the community structure showed that two N2O-reducer clades shared a certain number of explanatory variables but there were also distinct explanatory variables for each clade. The structure of both communities was driven by pH and calcium, while water content was related to clade I and sand to clade II (Figures 3A,B). To our knowledge, the difference in the response of the two N2O reducer clades to soil properties has only been assessed in one previous study (Jones et al., 2014). Using structural equation modeling, Jones et al. (2014) showed that soil texture was a more important driver of the abundance of the nosZI community whereas soil pH affected the abundance of the nosZII community only. Overall, our results, which showed that different factors influenced the nosZI and nosZII clades, confirm niche partitioning between the two N2O reducing communities. They also indicate that agricultural practices could affect nosZI and nosZII communities in different ways with consequences for N2O reduction.
Variation partitioning analysis was applied to disentangle the contribution of soil properties, the abundance of N2O producers and reducers, and the diversity of N2O reducing microbial communities to the production of N2O and N2 as the denitrification end products. Potential N2O production (29%) and PDA (45%) were mostly explained by soil properties. Water content, C/N ratio, Cation Exchange Capacity (CEC) and pH were the major soil properties explaining N2O production, while sand content, total nitrogen and the C/N ratio explained PDA. Accordingly, nitrate and carbon availability, pO2 and related variables such as water content and soil texture are well known proximal factors regulating N2O and PDA rates (Baggs, 2011; Giles et al., 2012). pH is also known to be a master regulator of biological processes in soils (Enwall et al., 2005; Giles et al., 2012; Petersen et al., 2012; Saggar et al., 2013; Liu et al., 2014) including the reduction of N2O into N2 by nitrous oxide reductase (Firestone et al., 1980). Unlike other studies (Hallin et al., 2009; Petersen et al., 2012; Jones et al., 2014), the abundance of the N2O producing and reducing communities did not explain variations in PDA nor in potential N2O production (Figures 3B,C). According to Petersen et al. (2012) the abundance of denitrifiers was correlated with PDA only when large variations in fluxes were observed, which was not the case in this study. In contrast to potential N2O production and PDA, the variance in the proportion of N2O emitted by denitrification [rN2O/r(N2O+N2)] was mostly explained by the diversity of the nosZII clade (26%), and the interaction between this diversity and soil properties (17%) (Figure 3D). This is in agreement with previous research showing a positive correlation between the ability of the soil to consume N2O and the phylogenetic diversity of the nosZII clade (Jones et al., 2014). It also illustrates the importance of the nosZII community in determining the nature of the denitrification gaseous end-products through their capacity to act as a N2O sink.
Overall, our results showed that the newly described nosZII clade is the strongest predictor of the [rN2O/r(N2O+N2)] ratio while soil properties are the main drivers of potential denitrification and N2O production. They also showed that the two clades of N2O reducers were not affected by the same soil properties, suggesting niche partitioning. The nosZII clade was more sensitive to environmental changes than the nosZI clade, which may make it easier to foster this group using agricultural practices as a new strategy for mitigating N2O emissions. Further studies are required to determine the effect of different agricultural practices on the abundance and diversity of the nosZII clade, in sites with different pedoclimatic conditions to provide more information on the ecology of this recently described functional guild. Moreover, due to the increasing evidence that fungi can produce N2O and N2 by denitrification and co-denitrification (Laughlin and Stevens, 2002; Wei et al., 2014; Maeda et al., 2015), respectively, the response of these microorganisms to agricultural practices should also be considered to circumvent any tradeoff.
Materials and Methods
Experimental Design and Sampling
Soil samples were collected in October 2013 from two randomized field experiments ORE (49°52′25.615″N, 3°1′53.914″E) and BE (49°52′19.29″N, 3°0′47.267″E) located at the same site near Estrées-Mons, France, which has both annual rotation and perennial crop systems, as well as various agricultural practices. A description of the practices can be found in Table 1. Replicated plots with the same practices were randomly distributed within each block experiment. Briefly, the ORE experiment, which consisted in 5 treatments (T1–T5), was set up in 2010 to study the effect of soil tillage, crop residue management, fertilization rate and substitution of mineral N input by fixation by legumes on biogeochemical cycles and soil biodiversity (www.soere-acbb.com). The BE experiment was set up in 2006 to compare the productivity and environmental impacts of various energy crop systems including perennial crops such as Miscanthus giganteus and Panicum virgatum and differences in management practices such as early or late harvest (ML, ME, SL, and SE). Three replicate samples were collected for each combination of cropping system and management practices, each being a composite sample of five subsamples (soil cores of 2.5 cm by 20 cm) from each plot. Samples were frozen (−20°C) until further analysis. The physical and chemical soil characteristics were measured for all samples (INRA Laboratory of Soil Analysis, Arras, France) (Table 1).
Potential Denitrification Activity (PDA) and Potential N2O production
Potential denitrification activity (N2O + N2) and potential nitrous oxide production (N2O) were measured using the acetylene inhibition technique (Yoshinari et al., 1977). For each sample 10 g of fresh weight soil was wetted with 20 ml of distilled water and was amended with a final concentration of 3 mM KNO3, 1.5 mM succinate, 1 mM glucose, and 3 mM acetate. To determine the potential denitrification activity, acetylene was added to reach 0.1 atm partial pressure followed by 30 min incubation at 25°C and agitation (175 rpm). Gas samples were taken every 30 min for 150 min (Pell et al., 1996). The N2O concentrations were determined using a gas chromatograph (Trace GC Ultra, Thermo Scientific) equipped with an EC-detector.
Nucleic Acid Extraction and Abundance of Bacterial Communities
DNA extraction for all samples was performed in accordance with ISO 11063 (Petric et al., 2011). 0.25 g of soil was homogenized with a 1 ml homogenization buffer for 30 s at 1600 rpm in a mini-bead beater cell disruptor (Mikro-Dismembrator S; B Braun Biotech International), followed by centrifugation at 14000 × g for 1 min to eliminate soil and cell debris. For protein precipitation, supernatant was incubated on ice for 10 min with 1/10 volume of 3M sodium acetate and centrifuged (14000 × g, 5 min, 4°C). The DNA was precipitated by adding one volume of cold isopropanol (−20°C) over 24 h. The mix was then centrifuged for 30 min at 14,000 g (4°C), the resulting pellet was washed with 70% ethanol, and the DNA was resuspended with 100 μL of TE buffer (pH 8). The DNA was purified in two steps: first using polyvinylpolypyrrolidone (PVPP) microbiospin columns (Bio-Rad, CA, USA), and then a Sepharose 4G column (Sigma-Aldrich, United Kingdom). The DNA quality was checked by electrophoresis on agarose gel and quantified by spectrofluorometer using the Quant-iT PicoGreen® dsDNA Assay Kit (Invitrogen, Cergy-Pontoise, France) following the manufacturer's instructions.
The abundance of denitrifiers was assessed by real-time quantitative PCR (qPCR) by targeting N2O-producers, nirK and nirS (Henry et al., 2004; Kandeler et al., 2006) and N2O-reducers nosZI (Henry et al., 2006; Jones et al., 2013) and nosZII (Table S3). Abundance of total bacteria was assed using 16S rRNA primers (Muyzer et al., 1993) as previously described (López-Gutiérrez et al., 2004). qPCR Reactions were carried out in a StepOnePlus Real time PCR System (Life Technologies, Carlsbad, CA, USA). The abundance was based on the increasing fluorescence intensity of the SYBR Green dye during amplification. The qPCR assay was carried out in a 15 μl reaction volume containing 1 ng of DNA, 7.5 μl of SYBRgreen PCR Master Mix (Absolute qPCR SYBR GreenRox, Thermo, Courtaboeuf, France), 1 μM of each primer, 250 ng of T4 gene 32 (QBiogene, Illkrich, France). Before assessing the abundance of the bacterial communities, an inhibition test was performed by mixing DNA extracts with a known amount of control plasmid DNA and no inhibition was detected. Three independent quantitative qPCR assays were performed for each gene. Controls and no-template controls giving null or negligible values were run for each quantitative qPCR assay. The qPCR efficiencies for the various genes ranged between 70 and 96%.
Phylogenetic Diversity of N2O-reducers
A diversity analysis of nosZI and nosZII was performed by 454 pyrosequencing as previously described in Jones et al. (2014). Briefly, the DNA was prepared using a two-step PCR procedure (Berry et al., 2012). In the first step, 20 PCR cycles were performed with primers nosZI and nosZII (Table S4) in a 25 μl reaction volume containing 5 μl 5 × Taq Buffer (GoTaq, Promega, Madison, U.S.A.), 2 μM of each primer, 250 ng of T4 gene 32 (QBiogene, Illkrich, France), 0.125 μl of DNA Polymerase (GoTaq, Promega, Madison, U.S.A.), 200 μM(each) deoxyribonucleoside triphosphate, and 1 ng of template DNA. In the second step, 4 μL of the PCR products of the first reaction were amplified in a 50 μl reaction volume containing 10 μl 5 × Taq Buffer (GoTaq, Promega, Madison, U.S.A.), 200 μM (each) deoxyribonucleoside triphosphate, 1 μM of each primer, 0.25 μl of DNA Polymerase (GoTaq G2, Promega, Madison, U.S.A.). In the second PCR 15 or 18 cycles PCR were performed using the forward primers preceded by 10 bp-long barcodes, the sequencing key and the forward sequencing adapter; the reverse primers being only preceded by the sequencing key and the reverse sequencing adapter as described in Jones et al. (2014) (Table S4). Because there were only very small amounts of products for nosZII after the first PCR, the second PCR for this gene was extended to 18 cycles. The product of 3 independent second PCR was then gel extracted and purified using the QIAEX II kit (Qiagen; France). Pyrosequencing was performed by Genoscreen (Lille, France) on a Roche's 454 FLX Genome Sequencer according to manufacturer's instructions.
Sequence Processing
The QIIME pipeline (Caporaso et al., 2010a) was used for quality trimming of raw 454 pyrosequencing data (QIIME version 1.8.0). The minimum and maximum sequence lengths were 230 and 410 bp respectively. Sequences with an average score below 25 using a sliding window of 50 bp were discarded. After quality checking, 123,130 sequences were found for nosZI and 121,500 sequences for nosZII. Sequences were then processed using the “pick_otus.py” script within QIIME, and the “usearch” option (Edgar, 2010) with reference-based and de novo chimera checking, and clustering of sequences at 97% similarity. Raw sequences were deposited at the NCBI under the accession number SRP058080. The process of raw sequence submission was greatly simplified by using the make.sra command of Mothur software (Schloss et al., 2009).
nosZ phylogeny
Reference sequences for nosZ were downloaded from all 4135 draft and completed microbial genome nucleotide sequences available in the National Center for Biology Information (NCBI) (Jones et al., 2014). These reference sequences were used as templates for aligning 454 reads with PYNAST (Caporaso et al., 2010b). Phylogenetic trees for nosZI and nosZII were constructed with fastree (Price et al., 2010) and ITOL was used to visualize and manipulate of the trees (Letunic and Bork, 2007).
Statistical Analysis
Statistical analysis and graphics were produced using the R statistical software, R version 3.0.3, (R Core Team, 2013) and the agricolae (Mendiburu, 2014) and vegan (Oksanen et al., 2015) packages. The effect of agricultural practices and cropping systems was determined by analysis of variance and post hoc Tukey HSD test. Collinearity between explaining variables within each group (soil properties, microbial community abundances, and denitrifiers diversity) was checked, and one of each pair of collinear variables was kept for subsequent analyses. Non-metric MultiDimensional Scaling (NMDS) of the Unifrac distance matrices (unweighted and weighted) was used to describe community structure. Ordinations with the lowest stress values were used. The soil properties, community abundances and diversity were plotted onto the ordination map as vectors. Permutation tests (n = 10000) were used to test the significance of vector fits and only significant ones were depicted (P < 0.05). Vector and surface fitting of variables within ordinations were performed using the envfit and ordisurf functions in the vegan package respectively. ANalysis Of SIMilarity (ANOSIM) was used to test for significant differences in community structure between cropping systems (permutations = 1999, P < 0.05).
Significant explanatory variables of [rN2O/r(N2O+N2)], potential N2O and PDA were chosen by linear regression and model selection (backward) and by minimizing the Akaike Information Criterion (AIC). The statistical significance was assessed by 1000 permutations of the reduced model. The resulting significant explanatory variables (Table S2) were used to access their contribution to explaining the variation of potential N2O, PDA and [rN2O/r(N2O+N2)], using the function varpart (Peres-Neto et al., 2006).
Conflict of Interest Statement
The authors declare that the research was conducted in the absence of any commercial or financial relationships that could be construed as a potential conflict of interest.
Acknowledgments
This research was supported by the European Union (Marie Curie ITN NORA, FP7- 316472) and the French Agency for the Environment and Energy (ADEME) thought the project EFEMAIR-N2O. We are grateful to Céline Peyrard and Bruno Mary for helpful discussions and suggestions.
Supplementary Material
The Supplementary Material for this article can be found online at: http://journal.frontiersin.org/article/10.3389/fmicb.2015.00971
References
Amougou, N., Bertrand, I., Cadoux, S., and Recous, S. (2012). Miscanthus x giganteus leaf senescence, decomposition and C and N inputs to soil. Glob. Change Biol. Bioen. 4, 698–707. doi: 10.1111/j.1757-1707.2012.01192.x
Amougou, N., Bertrand, I., Machet, J.-M., and Recous, S. (2011). Quality and decomposition in soil of rhizome, root and senescent leaf from Miscanthus x giganteus, as affected by harvest date and N fertilization. Plant Soil 338, 83–97. doi: 10.1007/s11104-010-0443-x
Baggs, E. M. (2011). Soil microbial sources of nitrous oxide: recent advances in knowledge, emerging challenges and future direction. Curr. Opin. Environ. Sustain. 3, 321–327. doi: 10.1016/j.cosust.2011.08.011
Bates, B., Kundzewicz, Z., Wu, S., and Palutikof, J. (2008). “Climate change and water,” in Technical Paper of the Intergovernmental Panel on Climate Change (Geneva: IPCC).
Berry, D., Ben Mahfoudh, K., Wagner, M., and Loy, A. (2012). Barcoded primers used in multiplex amplicon pyrosequencing bias amplification. Appl. Environ. Microbiol. 78, 612–612. doi: 10.1128/AEM.07448-11
Bouwman, A. F., Beusen, A. H. W., Griffioen, J., Van Groenigen, J. W., Hefting, M. M., Oenema, O., et al. (2013). Global trends and uncertainties in terrestrial denitrification and N2O emissions. Phil. Trans. R Soc. B. 368, 20130112. doi: 10.1098/rstb.2013.0112
Cadoux, S., Ferchaud, F., Demay, C., Boizard, H., Machet, J.-M., Fourdinier, E., et al. (2014). Implications of productivity and nutrient requirements on greenhouse gas balance of annual and perennial bioenergy crops. Glob. Change Biol. Bioeng. 6, 425–438. doi: 10.1111/gcbb.12065
Caporaso, J. G., Bittinger, K., Bushman, F. D., DeSantis, T. Z., Andersen, G. L., and Knight, R. (2010b). PyNAST: a flexible tool for aligning sequences to a template alignment. Bioinformatics 26, 266–267. doi: 10.1093/bioinformatics/btp636
Caporaso, J. G., Kuczynski, J., Stombaugh, J., Bittinger, K., Bushman, F. D., Costello, E. K., et al. (2010a). QIIME allows analysis of high-throughput community sequencing data. Nat. Methods 7, 335–336. doi: 10.1038/nmeth.f.303
Clark, I. M., Buchkina, N., Jhurreea, D., Goulding, K. W., and Hirsch, P. R. (2012). Impacts of nitrogen application rates on the activity and diversity of denitrifying bacteria in the Broadbalk Wheat Experiment. Phil. Trans. R. Soc. B Biol. Sci. 367, 1235–1244. doi: 10.1098/rstb.2011.0314
Edgar, R. C. (2010). Search and clustering orders of magnitude faster than BLAST. Bioinformatics 26, 2460–2461. doi: 10.1093/bioinformatics/btq461
Enwall, K., Philippot, L., and Hallin, S. (2005). Activity and composition of the denitrifying bacterial community respond differently to long-term fertilization. Appl. Environ. Microbiol. 71, 8335–8343. doi: 10.1128/AEM.71.12.8335-8343.2005
Firestone, M. K., Firestone, R. B., and Tiedje, J. M. (1980). Nitrous-oxide from soil denitrification - factors controlling its biological production. Science 208, 749–751. doi: 10.1126/science.208.4445.749
Giles, M., Morley, N., Baggs, E. M., and Daniell, T. J. (2012). Soil nitrate reducing processes drivers, mechanisms for spatial variation, and significance for nitrous oxide production. Front. Microbiol. 3:407. doi: 10.3389/fmicb.2012.00407
Graf, D. R., Jones, C. M., and Hallin, S. (2014). Intergenomic comparisons highlight modularity of the denitrification pathway and underpin the importance of community structure for N2O Emissions. PLoS ONE 9:e114118. doi: 10.1371/journal.pone.0114118
Hallin, S., Jones, C. M., Schloter, M., and Philippot, L. (2009). Relationship between N-cycling communities and ecosystem functioning in a 50-year-old fertilization experiment. ISME J. 3, 597–605. doi: 10.1038/ismej.2008.128
Hartmann, M., Frey, B., Mayer, J., Mäeder, P., and Widmer, F. (2014). Distinct soil microbial diversity under long-term organic and conventional farming. ISME J. 9, 1177–1194. doi: 10.1038/ismej.2014.210
Henry, S., Baudoin, E., López-Gutiérrez, J. C., Martin-Laurent, F., Brauman, A., and Philippot, L. (2004). Quantification of denitrifying bacteria in soils by nirK gene targeted real-time PCR. J. Microbiol. Methods 59, 327–335. doi: 10.1016/j.mimet.2004.07.002
Henry, S., Bru, D., Stres, B., Hallet, S., and Philippot, L. (2006). Quantitative detection of the nosZ gene, encoding nitrous oxide reductase, and comparison of the abundances of 16S rRNA, narG, nirK, and nosZ genes in soils. Appl. Environ. Microbiol. 72, 5181–5189. doi: 10.1128/AEM.00231-06
Jones, C. M., Graf, D. R., Bru, D., Philippot, L., and Hallin, S. (2013). The unaccounted yet abundant nitrous oxide-reducing microbial community: a potential nitrous oxide sink. ISME J. 7, 417–426. doi: 10.1038/ismej.2012.125
Jones, C. M., Spor, A., Brennan, F. P., Breuil, M.-C., Bru, D., Lemanceau, P., et al. (2014). Recently identified microbial guild mediates soil N2O sink capacity. Nat. Clim. Change 4, 801–805. doi: 10.1038/nclimate2301
Kandeler, E., Deiglmayr, K., Tscherko, D., Bru, D., and Philippot, L. (2006). Abundance of narG, nirS, nirK, and nosZ genes of denitrifying bacteria during primary successions of a glacier foreland. Appl. Environ. Microbiol. 72, 5957–5962. doi: 10.1128/AEM.00439-06
Lauber, C. L., Ramirez, K. S., Aanderud, Z., Lennon, J., and Fierer, N. (2013). Temporal variability in soil microbial communities across land-use types. ISME J. 7, 1641–1650. doi: 10.1038/ismej.2013.50
Laughlin, R. J., and Stevens, R. J. (2002). Evidence for fungal dominance of denitrification and codenitrification in a grassland soil. Soil Sci. Soc. Am. J. 66, 1540–1548. doi: 10.2136/sssaj2002.1540
Letunic, I., and Bork, P. (2007). Interactive Tree Of Life (iTOL): an online tool for phylogenetic tree display and annotation. Phylogenetics 23, 127–128. doi: 10.1093/bioinformatics/btl529
Liu, B., Frostegard, A., and Bakken, L. R. (2014). Impaired Reduction of N2O to N2 in acid soils is due to a posttranscriptional interference with the expression of nosZ. MBio 5:e01383-14. doi: 10.1128/mBio.01383-14
López-Gutiérrez, J. C., Henry, S., Hallet, S., Martin-Laurent, F., Catroux, G., and Philippot, L. (2004). Quantification of a novel group of nitrate-reducing bacteria in the environment by real-time PCR. J. Microbiol. Methods 57, 399–407. doi: 10.1016/j.mimet.2004.02.009
Maeda, K., Spor, A., Edel-Hermann, V., Heraud, C., Breuil, M. C., Bizouard, F., et al. (2015). N2O production, a widespread trait in fungi. Sci. Rep. 5:9697. doi: 10.1038/srep09697
Melero, S., Perez-de-Mora, A., Manuel Murillo, J., Buegger, F., Kleinedam, K., Kublik, S., et al. (2011). Denitrification in a vertisol under long-term tillage and no-tillage management in dryland agricultural systems: key genes and potential rates. Appl. Soil Ecol. 47, 221–225. doi: 10.1016/j.apsoil.2010.12.003
Mendiburu, F. (2014). agricolae: Statistical Procedures for Agricultural Research. R package version 1.2-1. Available online at: http://CRAN.R-project.org/package=agricolae
Meyer, A., Focks, A., Radl, V., Keil, D., Welzl, G., Schöening, I., et al. (2013). Different land use intensities in grassland ecosystems drive ecology of microbial communities involved in nitrogen turnover in soil. PLoS ONE 8:e73536. doi: 10.1371/journal.pone.0073536
Montzka, S. A., Dlugokencky, E. J., and Butler, J. H. (2011). Non-CO2 greenhouse gases and climate change. Nature 476, 43–50. doi: 10.1038/nature10322
Murray, R. E., and Knowles, R. (2004). Trace amounts of O-2 affect NO and N2O production during denitrifying enzyme activity (DEA) assays. Soil Biol. Biochem. 36, 513–517. doi: 10.1016/j.soilbio.2003.10.027
Muyzer, G., Dewaal, E. C., and Uitterlinden, A. G. (1993). Profiling of complex microbial-populations by denaturing gradient gel-electrophoresis analysis of polymerase chain reaction-amplified genes-coding for 16S ribosomal-RNA. Appl. Environ. Microbiol. 59, 695–700.
Oksanen, J., Blanchet, F. G., Kindt, R., Legendre, P., Minchin, R. P., O'Hara, R. B., et al. (2015). vegan: Community Ecology Package. R package version 2.2-1. Available online at: http://CRAN.R-project.org/package=vegan
Orellana, L. H., Rodriguez, -R. L. M., Higgins, S., Chee-Sanford, J. C., Sanford, R. A., Ritalahti, K. M., et al. (2014). Detecting Nitrous Oxide Reductase (nosZ) genes in soil metagenomes: method development and implications for the Nitrogen Cycle. MBio 5, e01193–e01114. doi: 10.1128/mBio.01193-14
Pell, M., Stenberg, B., Stenstrom, J., and Torstensson, L. (1996). Potential denitrification activity assay in soil - With or without chloramphenicol? Soil Biol. Biochem. 28, 393–398. doi: 10.1016/0038-0717(95)00149-2
Peres-Neto, P. R., Legendre, P., Dray, S., and Borcard, D. (2006). Variation partitioning of species data matrices: estimation and comparison of fractions. Ecology 87, 2614–2625. doi: 10.1890/0012-9658(2006)87[2614:VPOSDM]2.0.CO;2
Petersen, D. G., Blazewicz, S. J., Firestone, M., Herman, D. J., Turetsky, M., and Waldrop, M. (2012). Abundance of microbial genes associated with nitrogen cycling as indices of biogeochemical process rates acrolauss a vegetation gradient in Alaska. Environ. Microbiol. 14, 993–1008. doi: 10.1111/j.1462-2920.2011.02679.x
Petric, I., Philippot, L., Abbate, C., Bispo, A., Chesnot, T., Hallin, S., et al. (2011). Inter-laboratory evaluation of the ISO standard 11063 “Soil quality - Method to directly extract DNA from soil samples.” J. Microbiol. Methods 84, 454–460. doi: 10.1016/j.mimet.2011.01.016
Philippot, L., Andert, J., Jones, C. M., Bru, D., and Hallin, S. (2011). Importance of denitrifiers lacking the genes encoding the nitrous oxide reductase for N2O emissions from soil. Glob.Change. Biol. 17, 1497–1504. doi: 10.1111/j.1365-2486.2010.02334.x
Price, M. N., Dehal, P. S., and Arkin, A. P. (2010). FastTree 2-approximately maximum-likelihood trees for large alignments. PLoS ONE 5:e9490. doi: 10.1371/journal.pone,0.0009490
Ravishankara, A. R., Daniel, J. S., and Portmann, R. W. (2009). Nitrous Oxide (N2O): the dominant ozone-depleting substance emitted in the 21st Century. Science 326, 123–125. doi: 10.1126/science.1176985
R Core Team. (2013). R: A Language and Environment for Statistical Computing. Vienna: R Foundation for Statistical Computing.
Saggar, S., Jha, N., Deslippe, J., Bolan, N. S., Luo, J., Giltrap, D. L., et al. (2013). Denitrification and N2O:N-2 production in temperate grasslands: processes, measurements, modelling and mitigating negative impacts. Sci. Total Environ. 465, 173–195. doi: 10.1016/j.scitotenv.2012.11.050
Sanford, R. A., Cole, J. R., and Tiedje, J. M. (2002). Characterization and description of Anaeromyxobacter dehalogenans gen. nov., sp nov., an aryl-halorespiring facultative anaerobic myxobacterium. Appl. Environ. Microbiol. 68, 893–900. doi: 10.1128/AEM.68.2.893-900.2002
Sanford, R. A., Wagner, D. D., Wu, Q., Chee-Sanford, J. C., Thomas, S. H., Cruz-Garcia, C., et al. (2012). Unexpected nondenitrifier nitrous oxide reductase gene diversity and abundance in soils. Proc. Natl. Acad. Sci. U.S.A.109, 19709–19714. doi: 10.1073/pnas.1211238109
Schloss, P. D., Westcott, S. L., Ryabin, T., Hall, J. R., Hartmann, M., Hollister, E. B., et al. (2009). Introducing mothur: open-source, platform-independent, community-supported software for describing and comparing microbial communities. Appl. Environ. Microbiol. 75, 7537–7541. doi: 10.1128/AEM.01541-09
Shcherbak, I., Millar, N., and Robertson, G. P. (2014). Global metaanalysis of the nonlinear response of soil nitrous oxide (N2O) emissions to fertilizer nitrogen. Proc. Natl. Acad. Sci. U.S.A. 111, 9199–9204. doi: 10.1073/pnas.1322434111
Stehfest, E., and Bouwman, L. (2006). N2O and NO emission from agricultural fields and soils under natural vegetation: summarizing available measurement data and modeling of global annual emissions. Nutri. Cycl. Agroecosyst. 74, 207–228. doi: 10.1007/s10705-006-9000-7
Syakila, A., and Kroeze, C. (2011). The global nitrous oxide budget revisited. Greenh. Gas Measur. Managem. 1, 17–26. doi: 10.3763/ghgmm.2010.0007
Tatti, E., Goyer, C., Chantigny, M., Wertz, S., Zebarth, B. J., Burton, D. L., et al. (2014). Influences of over winter conditions on denitrification and nitrous oxide-producing microorganism abundance and structure in an agricultural soil amended with different nitrogen sources. Agric. Ecosyst. Environm. 183, 47–59. doi: 10.1016/j.agee.2013.10.021
Thomson, A. J., Giannopoulos, G., Pretty, J., Baggs, E. M., and Richardson, D. J. (2012). Biological sources and sinks of nitrous oxide and strategies to mitigate emissions. Phil. Trans. R. Soc. B. 367, 1157–1168. doi: 10.1098/rstb.2011.0415
Tiedje, J. M., Sexstone, A. J., Myrold, D. D., and Robinson, J. A. (1982). Denitrification - ecological niches, competition and survival. Antonie Van Leeuwenhoek J. Micrro. 48, 569–583. doi: 10.1007/BF00399542
Walker, J. K., Egger, K. N., and Henry, G. H. (2008). Long-term experimental warming alters nitrogen-cycling communities but site factors remain the primary drivers of community structure in high arctic tundra soils. ISME J. 2, 982–995. doi: 10.1038/ismej.2008.52
Wei, W., Isobe, K., Shiratori, Y., Nishizawa, T., Ohte, N., Otsuka, S., et al. (2014). N2O emission from cropland field soil through fungal denitrification after surface applications of organic fertilizer. Soil Biol. Biochem. 69, 157–167. doi: 10.1016/j.soilbio.2013.10.044
Wessen, E., Hallin, S., and Philippot, L. (2010). Differential responses of bacterial and archaeal groups at high taxonomical ranks to soil management. Soil Biol. Biochem. 42, 1759–1765. doi: 10.1016/j.soilbio.2010.06.013
Yoshinari, T., Hynes, R., and Knowles, R. (1977). Acetylene inhibition of nitrous-oxide reduction and measurement of denitrification and nitrogen fixation in soil. Soil Biol.Biochem. 9, 177–183. doi: 10.1016/0038-0717(77)90072-4
Zumft, W. G. (1997). Cell biology and molecular basis of denitrification. Microbiol. Mol. Biol. Rev. 61, 533–616.
Keywords: nosZ, greenhouse gas, agroecology, diversity, nitrous oxide, agricultural practices, nitrogen cycling
Citation: Domeignoz-Horta LA, Spor A, Bru D, Breuil M, Bizouard F, Léonard J and Philippot L (2015) The diversity of the N2O reducers matters for the N2O:N2 denitrification end-product ratio across an annual and a perennial cropping system. Front. Microbiol. 6:971. doi: 10.3389/fmicb.2015.00971
Received: 13 July 2015; Accepted: 01 September 2015;
Published: 24 September 2015.
Edited by:
Tim Daniell, The James Hutton Institute, UKReviewed by:
Gwen-Aelle Grelet, Landcare Research - Manaaki Whenua, New ZealandAdrian Langarica-Fuentes, The James Hutton Institute, UK
Copyright © 2015 Domeignoz-Horta, Spor, Bru, Breuil, Bizouard, Léonard and Philippot. This is an open-access article distributed under the terms of the Creative Commons Attribution License (CC BY). The use, distribution or reproduction in other forums is permitted, provided the original author(s) or licensor are credited and that the original publication in this journal is cited, in accordance with accepted academic practice. No use, distribution or reproduction is permitted which does not comply with these terms.
*Correspondence: Laurent Philippot, INRA, UMR 1347 Agroécologie, 17 Rue Sully, F-21065 Dijon, France,TGF1cmVudC5QaGlsaXBwb3RAZGlqb24uaW5yYS5mcg==