- Department of Animal Science, The University of Tennessee, Knoxville, TN, USA
Beta-lactam antibiotics are an important class of antibiotics for treating bacterial infections. Despite prevalent β-lactam resistance in Campylobacter jejuni, the leading bacterial cause of human diarrhea in developed countries, molecular mechanism of β-lactam resistance in C. jejuni is still largely unknown. In this study, C. jejuni 81–176 was used for random transposon mutagenesis. Screening of a 2,800-mutant library identified 22 mutants with increased susceptibility to ampicillin. Of these mutants, two mutants contains mutations in Cj0843c (a putative lytic transglycosylase gene) and in its upstream gene Cj0844c, respectively. Complementation experiment demonstrated that the Cj0843 contributes to β-lactam resistance. The Cj0843c insertional mutation was subsequently introduced to diverse C. jejuni clinical strains for MIC test, showing that Cj0843c contributes to both intrinsic and acquired β-lactam resistance of C. jejuni. Consistent with this finding, inactivation of Cj0843c also dramatically reduced β-lactamase activity. Genomic examination and PCR analysis showed Cj0843c is widely distributed in C. jejuni. High purity recombinant Cj0843c was produced for generation of specific antiserum. The Cj0843 was localized in the periplasm, as demonstrated by immunoblotting using specific antibodies. Turbidimetric assay further demonstrated the capability of the purified Cj0843c to hydrolyze cell walls. Inactivation of Cj0843c also significantly reduced C. jejuni colonization in the intestine. Together, this study identifies a mechanism of β-lactam resistance in C. jejuni and provides insights into the role of cell wall metabolism in regulating β-lactamase activity.
Introduction
Beta-lactam antibiotics, which inhibit biosynthesis of bacterial cell wall, are the most commercially available antibiotics in the market. For instance, in 2009, beta-lactam antibiotics account for more than half of the total antibiotic sales globally (Hamad, 2010). However, the efficacy of the β-lactam based therapy is severely threatened by a variety of β-lactam resistance mechanisms in bacteria, such as production of beta-lactamases (Korfmann and Wiedemann, 1988; Iaconis and Sanders, 1990; Jacoby, 2009), expression of the penicillin-binding proteins (PBPs) with reduced affinity for β-lactam antibiotics (Fuda et al., 2004), and extrusion of β-lactam using multi-drug efflux pumps (Nikaido, 1998; Vila and Martinez, 2008). Production of β-lactamase has been a major and threatening β-lactam resistance mechanism. Recently, increasing evidence demonstrate a direct link between cell wall metabolism and regulation of β-lactamase activity in Gram-negative bacteria, which provides insights into the development of innovative strategies to counteract β-lactam resistance in pathogenic bacteria (Zeng and Lin, 2013).
Campylobacter jejuni is the leading bacterial cause of human gastroenteritis in the developed countries (Humphrey et al., 2007). Clinical symptoms include fever and watery diarrhea in humans. In rare case, campylobacteriosis can cause severe sequelae such as Guillain–Barré syndrome, an acute flaccid paralysis that may lead to respiratory muscle compromise and death (Nachamkin et al., 1998; Hughes and Cornblath, 2005; Nyati and Nyati, 2013). The annual incidence of campylobacteriosis in the United States is approximately 1% (Poly and Guerry, 2008), resulting in 1.7 billion dollars cost in medical practice and productivity (Hoffmann et al., 2012). In parallel to its increased prevalence, Campylobacter is increasingly resistant to macrolides and fluoroquinolones, the two major clinical antibiotics (Luo et al., 2005; Caldwell et al., 2008; Koningstein et al., 2011). Recently, it has been proposed that an oral β-lactam, such as co-amoxiclav, may provide an alternative therapy for Campylobacter infection (Elviss et al., 2009; Griggs et al., 2009). However, β-lactam resistant C. jejuni strains have also been observed in epidemiological studies (Lachance et al., 1991; Griggs et al., 2009). Thus, understanding the mechanisms of β-lactam resistance in C. jejuni would help us develop effective therapeutic strategy that will allow the use of existing β-lactams against resistant C. jejuni. In Campylobacter, two beta-lactam resistance mechanisms have been characterized, which include the presence of CmeABC multi-drug efflux pump (Lin et al., 2002) and the production β-lactamase OXA-61 (Alfredson and Korolik, 2005; Griggs et al., 2009). Despite these progresses, the findings from recent studies strongly suggest the existence of novel β-lactamases and/or regulatory system in C. jejuni for β-lactam resistance (Griggs et al., 2009; Zeng et al., 2014).
In this study, using random mutagenesis, we identified a putative lytic transglycosylase (LT) Cj0843c that is required for intrinsic and acquired β-lactam resistance in C. jejuni. This finding was further validated by a series of molecular, biochemical and genomic examination. Consistent with this finding, inactivation of Cj0843c, which is highly conserved in C. jejuni, dramatically reduced β-lactamase activity. The role of Cj0843c in peptidoglycan metabolism was validated by a standard LT assay using purified Cj0843c. This study provides compelling evidence that Cj0843c, a putative LT, plays a critical role in regulating β-lactamase-mediated β-lactam resistance in C. jejuni.
Materials And Methods
Bacterial Strains, Plasmids, and Culture Conditions
The major bacterial strains and plasmids used in this study are listed in Table 1. The C. jejuni strains were grown routinely in Müller-Hinton (MH) broth or agar at 42°C in tri-gas incubator (85% N2, 10% CO2, 5% O2). Escherichia coli strains were grown routinely in Luria-Bertani (LB) broth with shaking (250 rpm) or on agar at 37°C overnight. When needed, culture media were supplemented with ampicillin (100 μg/ml), kanamycin (50 μg/ml), and chloramphenicol (Cm; 20 μg/ml).
Random Transposon Mutagenesis
We have successfully used C. jejuni 81–176 as a host strain for in vivo random transposon mutagenesis using EZ-Tn5 < KAN-2 > Tnp Transposome system (Epicentre; Lin et al., 2009; Hoang et al., 2011, 2012; Zeng et al., 2013b). In this study, the wild-type C. jejuni 81–176 (ampicillin Minimum inhibitory concentration (MIC) = 1 μg/ml) was subjected to in vivo random transposon mutagenesis; the procedure and screening strategy are detailed in previous publications (Lin et al., 2009; Hoang et al., 2011, 2012; Zeng et al., 2013b). The mutants with growth defects in MH broth containing kanamycin (50 μg/ml) and ampicillin (0.25 μg/mL) were identified. To determine transposon insertion site, genomic DNA extracted from the selected mutant was subjected to direct sequencing as described previously (Lin et al., 2009; Hoang et al., 2011; Zeng et al., 2013b).
Antimicrobial Susceptibility Test
The susceptibilities of C. jejuni strains to different antimicrobials (Fisher Bioreagents) were determined by a standard microtiter broth dilution method with an inoculum of 106 CFU/ml as described previously (Lin et al., 2002; Zeng et al., 2014). MIC for specific antimicrobial was defined by the lowest concentration of the antimicrobial showing complete inhibition of bacterial growth after 2 days of incubation at 42°C under microaerophilic condition.
Beta-lactamase Activity Assay
To measure β-lactamase activity in C. jejuni strains, standard assay was performed on cell lysates of different strains by following previous procedure (Griggs et al., 2009; Zeng et al., 2014) with minor modifications. Briefly, late-log phase bacterial cells were harvested from MH plates, washed with PBS, and adjusted to an optical density at 600 nm of approximately 1.2 in PBS. Bacterial suspensions were sonicated and centrifuged at 13,000 rpm for 5 min. The supernatant was subjected to protein concentration measurement using BCA Protein Assay kit (Pierce). One hundred microliter of supernatant containing 40 μg of protein was mixed with 0.8 ml of ice-chilled reaction buffer (0.1 M phosphate; 1 mM EDTA, pH 7.0). Then 100 μl of nitrocefin (500 μg/ml) was added to the above reaction mix followed by incubation at 37°C for 30 min. The activity was determined by spectrometric at 486 nm. The molar extinction coefficient of hydrolyzed nitrocefin at 486 nm is 20,500 M-1 cm-1. The lysate from C. jejuni 81–176 was used as negative control. The β-lactamase activity was expressed as hydrolyzed nitrocefin (μM) per hr per mg of protein.
Complementation of C. jejuni 81–176 Transposon Mutants
The long fragment (containing the genes Cj0843c, Cj0844c, and Cj0845c) and the short fragment (containing the genes Cj0844c and Cj0845c) were PCR amplified from C. jejuni 81–176 using primer pairs of Cj0843c_F/Cj0843c_R and Cj0843c_F/Cj0844c_R (Table 2), respectively, with PfuUltra fusion II DNA polymerase (Stratagene). The resulting fragments were ligated into SmaI-digested shuttle vector pRY111 (Yao et al., 1993) and ligation mix was introduced into E. coli DH5α by heat shock transformation. The E. coli transformants containing the plasmid that bears the three-gene (named pCj843c) or the two-gene fragment (named pCj0844c) were identified and confirmed by sequencing. Both constructs were mobilized into C. jejuni 81–176 transposon mutants by triparental conjugation as described previously (Zeng et al., 2013a, 2014).
Natural Transformation
Natural transformation (biphasic method) was performed following standard procedure as described by Wang and Taylor (1990). Approximately 4 μg of C. jejuni genomic DNA was used for natural transformation. The insertional Cj0843c mutations in different strains were confirmed by PCR using primer pair Cj0843c_F1 and Cj0843c_R1 (Table 2).
Production of Recombinant Cj0843c and Generation of Polyclonal Antiserum
N-terminal histidine-tagged recombinant Cj0843c was produced in E. coli by using pET-28b(+) vector. Briefly, approximately 1568-bp fragment of Cj0843c was PCR amplified from C. jejuni NCTC 81–176 using primer pairs rCj0843c_F1(NdeI) and rCj0843c-pET28b_R(SalI; Table 2). The amplified product and the vector pET-28b(+) were both digested with NdeI and SalI and ligated with each other. Cloning, expression, and purification of rCj0843c were performed using the procedures described in our previous publication (Lin et al., 2005; Zeng et al., 2013a). The plasmid pET28b-Cj0843c in the E. coli BL21(DE3) clone (JL985) producing rCj0843c was sequenced to confirm the correct insertion. The fractions containing pure rCj0843c were pooled and dialyzed against 1 x PBS buffer (Fisher Bioreagents). The concentration of the purified rCj0843c was determined using BCA Protein Assay Kit (PierceTM). Approximately 4 mg of highly purified rCj0843c was used for the production of rabbit polyclonal antisera by Pacific Immunology Corp (Ramona, CA). Pre- and post-immune serum samples were analyzed by immunoblotting against pure rCj0843c to confirm the generation of specific Cj0843c antibody.
Enzymatic Activity of rCj0843c
The LT activity of rCj0843c was evaluated using turbidimetric assay as reported before with minor modification (Caldentey and Bamford, 1992). In brief, the isogenic Cj0843c mutant of C. jejuni 81–176 was harvested from three overnight-cultured MH agar plates and resuspended in 50 ml Tris-HCl buffer (50 mM, pH 7.5). Then 3 ml of chloroform was added in the suspension, which was kept at room temperature for 30 min with gently shaking. Subsequently, the chloroform-extracted cells (the crude cell walls) were pelleted by centrifugation at 13,000 rpm for 5 min, washed once with 50 mM Tris-HCl buffer (pH7.5), and resuspended in 50 ml of 50 mM Tris-HCl buffer (pH 7.5).
To perform turbidimetric assay, the crude cell walls were adjusted to final OD450 nm of approximately 0.45 using 50 mM Tris-HCl buffer. Then the purified rCj0843c was added to 1 ml of such cell wall suspension with final concentration of 24 μg/ml; the cell wall suspension containing PBS instead of rCj0843c served as control. The decrease in turbidity at 450 nm was determined after 2.5 h of incubation at 37°C. The turbidimetric assay was performed with triplicate and the statistical analysis of a Student’s t test was carried out using SAS (v9.4). A P-value less than 0.05 was considered significant.
Localization of the Cj0843c
To determine cellular localization of Cj0843c, the periplasmic and spheroplastic fractions were prepared using the PeriPrepsTM Periplasting Kit (Epicentre) as detailed in our recent publication (Zeng et al., 2013a). Different cellular fractions were subsequently subjected to SDS-PAGE and immunoblotting analysis as described in our previous publications (Lin et al., 2002; Zeng et al., 2009, 2013a). Briefly, the protein samples were separated by SDS-PAGE with a 12% (wt/vol) polyacrylamide separating gel. After SDS-PAGE, proteins in the gels were then electrophoretically transferred to nitrocellulose membranes (Bio-Rad) at 60 V for 1 h at 4°C. The membranes were incubated with blocking buffer (5% Nestle skim milk powder in PBS) for 16 h at 4°C prior to incubation with primary antibodies (Rabbit anti-Cj0843c at 1:5000 dilution in the blocking buffer). To ensure the quality of periplasmic and spheroplastic fractions, antibodies directed against CmeR (cytoplasmic control, 1:2000 dilution) were also used as primary antibodies. After incubation at 25°C for 1 h, the blots were washed three times with PBS containing 0.05% Tween 20 and subsequently incubated with secondary antibodies (1:50,000 dilution of goat anti-rabbit IgG-HRP; Kirkegaard and Perry) at 25°C for 1 h. After washing, the blots were developed with the SuperSignal West Dura Chemiluminescent Substrate (Thermo Scientific). Prestained molecular weight markers (Bio-Rad) were coelectrophoresed and blotted to allow estimation of the sizes of the proteins.
Prevalence of Cj0843c in C. jejuni
Total 30 C. jejuni strains from different hosts and geographically diverse areas were grown microaerophilically to late exponential phase and harvested by centrifugation. The cell pellets were suspended in 100 μL of water and lysed by boiling for 5 min. After centrifugation, the supernatants were used as template in PCR assay with highly Cj0843c-specific primers Cj0843c_F1 and Cj0843c_R1 (Table 2). For bioinformatic analysis of Cj0843c prevalence among available genome sequences, total 103 C. jejuni genomes were extracted from Integrated Microbial Genomes1 and the multi-sequence alignment was analyzed using Clustal Omega2. In addition, presence of Cj0843c homolog was also examined in the genomes of 49 Campylobacter coli and 298 Helicobacter pylori strains.
Chicken Colonization Experiment
Campylobacter jejuni 81–176 wild-type strain together with its isogenic Cj0843c mutant (JL916) and the complementation strain (JL926; Table 2) were used for colonization assay in a chicken model system as described in previous studies (Lin et al., 2002; Lin and Martinez, 2006; Hoang et al., 2011; Zeng et al., 2013a). The chicken study was approved by the Institutional Animal Care and Use Committee at The University of Tennessee (Protocol 1387 under oversight of the IACUC Chair Carla Sommardahl). Briefly, 1-day-old broiler chickens were obtained from a commercial hatchery (Hubbard Hatchery, Pikesville, TN, USA). The chickens were negative for Campylobacter as determined by culturing cloacal swabs prior to use in this study. Three groups of 4-days-old chickens (10 birds/group) were inoculated with different bacteria via oral gavage using a dose approximately 105 CFU of fresh late log-phase C. jejuni per bird. Notably, the motility of parent strain and its Cj0843c mutant was confirmed to be at a comparable level prior to challenge. The isogenic Cj0843c mutant displayed reduced growth in MH broth when compared to its parent strain. However, the fresh C. jejuni cultures with similar growth phase, reflected by the final OD600 nm measurement, were used to challenge chickens; the bacteria in such cultures were assumed to be in the same physiological state. For each group, 5 birds were euthanized and cecal samples were collected at 6 and 10 days post-inoculation. The cecal contents from each bird were weighed and diluted in MH broth. The diluted samples were plated onto MH agar plates with Campylobacter specific selective supplements (Oxoid, UK). The number of CFU per gram of cecal contents was calculated for each chicken and was used as an indicator of the colonization level. The detection limit of the plating methods was 102 CFU/g of cecal contents. A bird from which no Campylobacter colonies were detected was assigned a conservative value of 99 CFU/g of cecal contents for the purpose of calculating means and for statistical analysis. One-way analysis of variance (ANOVA) followed by a least-significant difference test was used to analyze the significant differences in colonization level (log transformed). A P-value less than 0.05 was considered significant.
Results
Cj0843c, a Putative Lytic Transglycosylase, is Required for Ampicillin Resistance
The C. jejuni 81–176 strain was used as a host strain to generate a library consisting of 2,800 Tn5-insertional mutants. Individual mutant grown in microtiter plates was replicated into screening plates containing MH broth with sublethal concentration of ampicillin (0.25 μg/ml). A total of 22 mutants displayed increased susceptibility to ampicillin. The insertional sites of these mutants were mapped by direct sequencing. It’s not surprising that most of them (20 mutants) contains transposon insertion in cmeABC locus which encodes CmeABC multidrug efflux pump system which was previously shown to be involved in ampicillin resistance (Lin et al., 2002, 2003). Specifically, transposon insertional mutations were identified in cmeA (two different sites with four mutants), cmeB (two different sites with four mutants), and cmeC (2 different sites with 12 mutants). Interestingly, the other two mutants have transposon insertion identified in two adjacent genes, the CJJ81176_0859 and CJJ81176_0860 (hereinafter, the corresponding universal locus names in C. jejuni NCTC 11168, Cj0843c and Cj0844c, were used). The Cj0843c was annotated as a putative secreted LT. Specifically, Cj0843c contains a highly conserved domain (COG0741, E-value is 3.53e-27) represented by MltE, an endo-specific LT from E. coli that catalyzes hydrolysis of glycan chains to generate short chains with 1,6-anhydro-MurNAc ends (Fibriansah et al., 2012). Cj0844c was annotated as putative integral membrane protein. Both mutants displayed fourfold MIC reduction for ampicillin when compared to that of wild type 81–176 (Table 3).

TABLE 3. Susceptibilities of two representative C. jejuni strains and their isogenic mutants to ampicillin.
Genome analysis showed that the start codon of Cj0843c overlapped the stop codon of Cj0844c by 4 bp nucleotides. In addition, there is only 2 bp nucleotide gap between the start codon of Cj0844c and the stop codon of its upstream gene Cj0845c. Therefore, the Cj0845c, Cj0844c, and Cj0843c may form an operon. The transposon insertion into Cj0844c may cause polar effect, affecting the expression of downstream gene Cj0843c. To test this, two constructs with different length (pCj0843c and pCj0844c) was constructed for complementation (Table 1). The pCj0843c covers the whole three-gene operon while pCj0844c only contains two upstream genes Cj0845c and Cj0844c. As shown in Table 3, complementation with pCj0843c restored the MIC of both mutants to the level of wild type strain. However, complementation of the Cj0844c mutant with pCj0844c failed to restore MIC of ampicillin to wild-type level, indicating that the Cj0843c, not Cj0844c, is involved in ampicillin resistance in C. jejuni 81–176.
To examine the role of Cj0843c in ampicillin resistance in different strains, the Cj0843c transposon mutation was also introduced into C. jejuni NCTC 11168 via natural transformation. As shown in Table 3, the isogenic Cj0843c mutant of NCTC 11168 also displayed increased susceptibility to ampicillin (eightfold reduction) while the MIC of the complementation strain was the same as that of wild type strain.
Cj0843c is Involved in the Resistance to Various β-lactam Antibiotics in Campylobacter
We also examined if inactivation of Cj0843c affected susceptibilities of C. jejuni to different β-lactam antibiotics. As shown in Table 4, susceptibilities of Cj0843c mutant to various β-lactam antibiotics (penicillin G, ticarcillin, carbenicillin, and cloxacillin) significantly increased (fourfold) when compared to wild-type 81–176 strain. Complementation of the isogenic Cj0843c mutant with pCj0843c restored MIC of ampicillin to wild type level (Table 4). In terms of other types of antimicrobials, such as bile salts (cholic acid and deoxycholic acid), ciprofloxacin and ethidium bromide (Table 4), the susceptibilities of Cj0843c mutant did not change when compared to wild-type strain.
Cj0843c also Plays an Important Role in Acquired Ampicillin Resistance
Although C. jejuni 81–176 lacks beta-lactamase gene blaOXA-61 in the genome (Griggs et al., 2009), it could acquire high-level ampicillin resistance (Ampr) due to the acquisition of active beta-lactamase blaOXA-61 from C. jejuni 21190 via natural transformation (Zeng et al., 2014). The MIC of ampicillin for the Ampr derivative of 81–176 was 128 μg/mL, which is 128-fold higher than the MIC of wild-type 81–176 (Figure 1), as reflected by the dramatic change of the beta-lactamase activity (Figure 1). However, inactivation of Cj0843c in the Ampr derivative of 81–176 dramatically reduced MIC (8 μg/mL) as well as the beta-lactamase activity in cell lysates (Figure 1). In contrast, although mutation in multidrug efflux pump gene cmeB (Lin et al., 2002) in the Ampr derivative led to reduced MIC of ampicillin (16 μg/mL), the beta-lactamase activity was not changed (Figure 1). This finding clearly indicated that Cj0843c also plays an important role in β-lactamase-mediated ampicillin resistance by modulating the activity of β-lactamase BlaOXA-61 in C. jejuni.
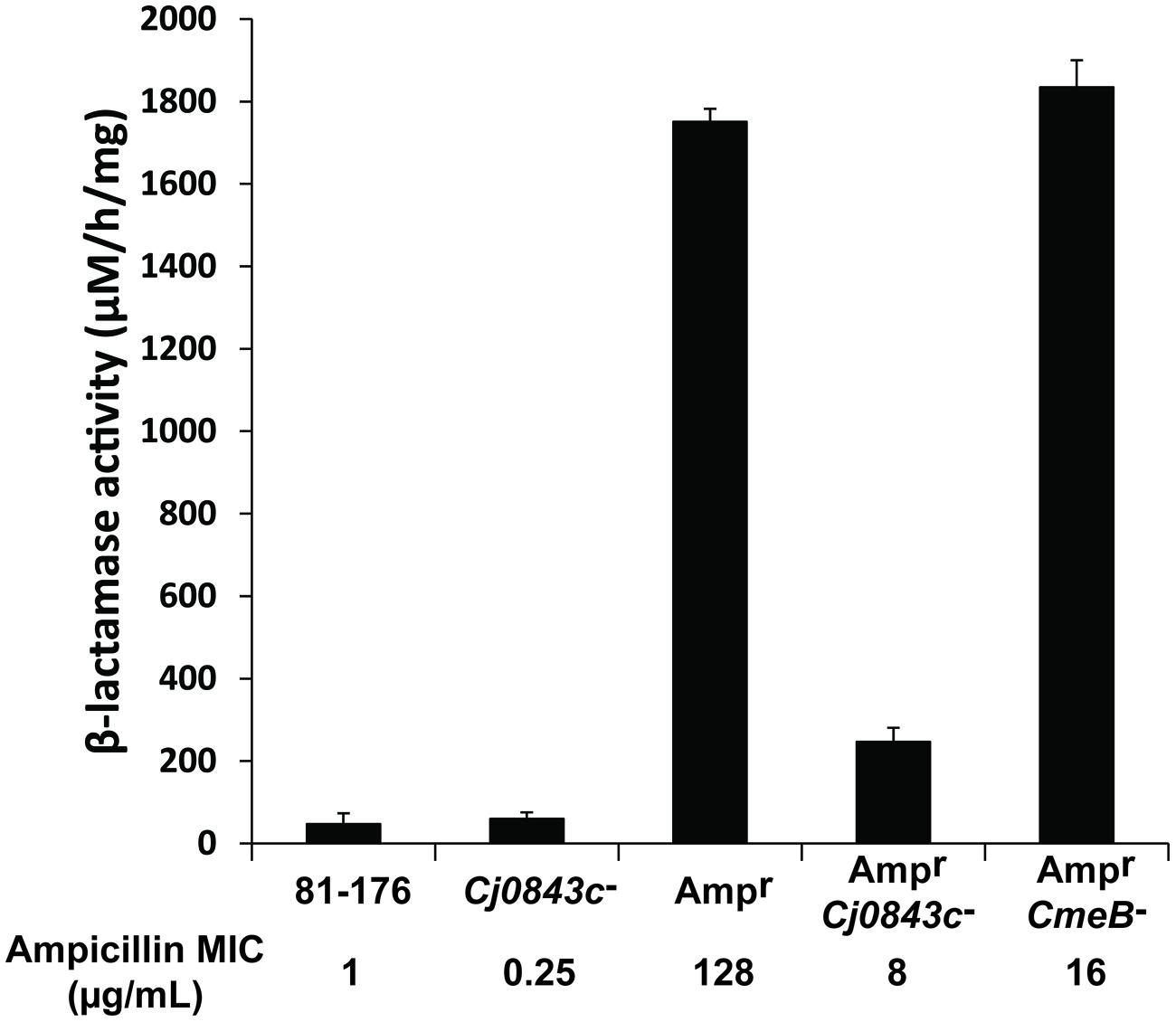
FIGURE 1. Effect of Cj0843c mutation on intracellular β-lactamase activities and ampicillin resistance in Campylobacter jejuni. The ampicillin MIC values were listed below each corresponding strain. Ampr, the 81–176 derivative with β-lactamase gene blaOXA-61 introduced into chromosome via natural transformation using genomic DNA from ampicillin resistant C. jejuni 21190 (Zeng et al., 2014). The procedure for nitrocefin-based β-lactamase activity assay was detailed in Materials and Methods. The activity was determined by spectrometric change at 486 nm. The molar extinction coefficient of hydrolyzed nitrocefin at 486 nm is 20,500 M-1 cm-1. The β-lactamase activity was expressed as hydrolyzed nitrocefin (μM) per hr per mg of protein.
To determine the role of Pgp1, an identified gene involved cell wall metabolism in C. jejuni (Frirdich et al., 2012), in ampicillin resistance, the pgp1::kan mutation was introduced into the wild-type 81–176 and its Ampr derivative in this study via natural transformation using genomic DNA of the pgp1 mutant, creating isogenic pgp1 mutant JL995 and JL996, respectively (Table 1). The MICs for JL995 and JL996 are 1 and 128 μg/mL, respectively, which are the same as those for their corresponding parent strains. Consistent with this finding, the beta-lactamase activity was not changed due to the inactivation of pgp1.
The Prevalent and Highly Conserved Cj0843c is Required for Ampicillin Resistance in Diverse C. jejuni Isolates
It’s well known that the genome of C. jejuni species displayed considerable plasticity (de Boer et al., 2002; Poly et al., 2008; Duong and Konkel, 2009). In this study, a PCR survey was performed, demonstrating that the Cj0843c was present in 30 diverse C. jejuni isolates (data not shown). The representative isolates were listed in Table 5. Regardless of the level of β-lactamase activity and the level of ampicillin resistance, introduction of Cj0843c mutation into these isolates all resulted in the decrease of MIC of ampicillin by more than fourfold (Table 5).
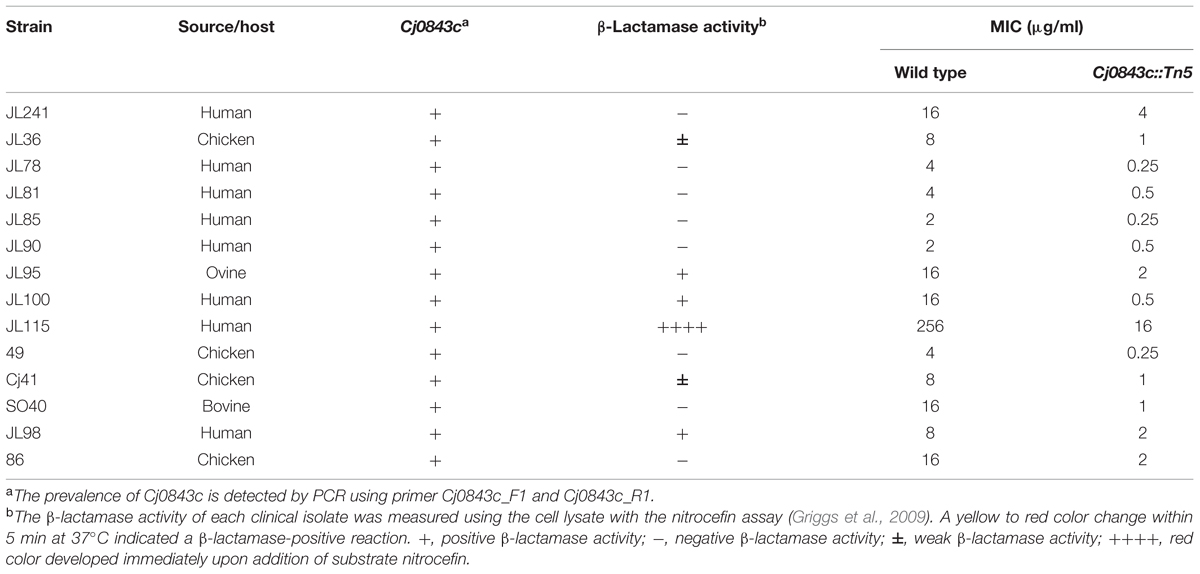
TABLE 5. Cj0843c is prevalent and involved in intrinsic and acquired ampicillin resistance in different clinical C. jejuni isolates.
In addition to this PCR survey, genome analysis also provided compelling evidence showing Cj0843c was highly conserved in C. jejuni and other closely related species. Specifically, Cj0843c was present in all 101 currently available C. jejuni genomes deposited in the database. Notably, the three-gene operon containing Cj0843c is also highly conserved in the genomes of different 𝜀-proteobacteria, including 101 C. jejuni, 49 C. coli, and 298 H. pylori (Supplementary Figure S1 and detailed in Supplementary Table S1).
The multi-sequence alignment data show the percentage aa identity among C. jejuni strains ranges from 93 to 100%. The across-species alignment shows that the aa identity and homology between C. jejuni NCTC 11168 and C. coli RM2228 are 79 and 89%, respectively, and those between C. jejuni NCTC 11168 and H. pylori 26695 are 31 and 51%, respectively.
The Cj0843c is Localized in Periplasm
Based on annotation, Cj0843c is predicted to interact with the glycan strand of the periplasmic peptidoglycan. To confirm this, we first cloned Cj0843c into expression vector pET-28b and produced N-terminal 6xHis tagged recombinant Cj0843c (rCj0843c). Majority of rCj0843c is in the soluble fraction and was successfully purified by Ni-NTA affinity column (Figure 2A).
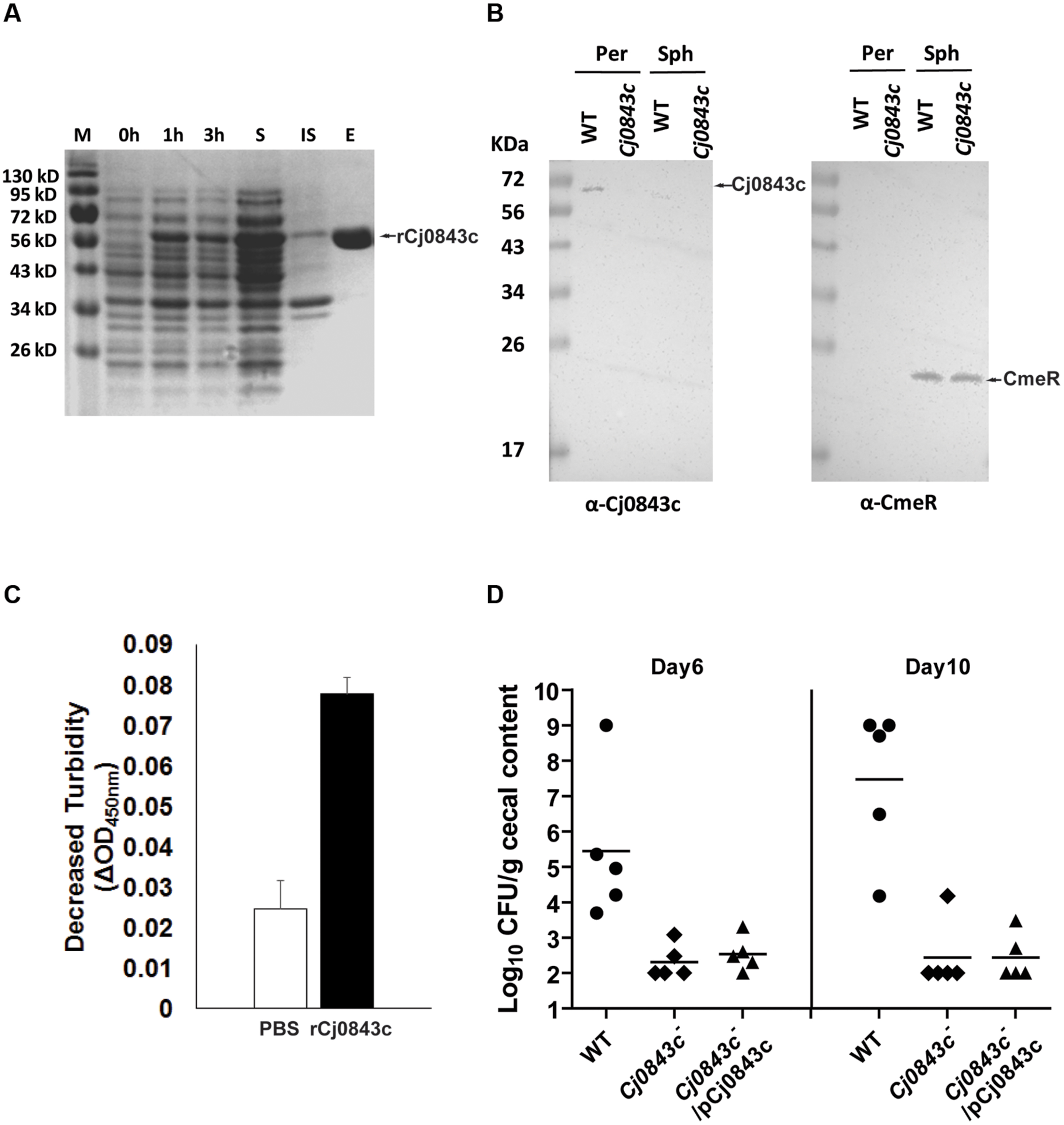
FIGURE 2. Characterization of Cj0843c. (A) Expression and purification of rCj0843c. Cj0843 fragment was cloned into pET-28b vector. Expression of rCj0843c was induced in the presence of 0.5 mM IPTG. 0, 1 and 3 h, the cell lysates from pre-induction, 1 and 3 h induced cells; S, soluble fraction; IS, insoluble fraction; E, elution fraction. (B) Cj0843c is localized in periplasm. The intracellular regulator CmeR was used as cytoplasm control. Per, periplasm; Sph, spheroplast; WT, wild type. (C) Turbidimetric assay of rCj0843c. Chloroform-treated Cj0843c- mutant cells (crude cell walls) were mixed with rCj0843c or PBS control solution. The decrease of absorbance at 450 nm was measured after 2.5 h incubation. Each bar represents the mean ± SD from three independent measurements. (D) Critical role of Cj0843c in colonization of C. jejuni in chicken. The chickens in each group (10 birds) were inoculated with C. jejuni 81–176 (close circle), its isogenic Cj0843c mutant (diamond), and complementation strain (triangle) at dose of 105 CFU/chicken. At days 6 and 10 post-challenge, five chickens from each group were killed and cecal contents were collected for C. jejuni numeration. The detection limit is 102 CFU/gram fecal sample. Each horizontal line segment denotes the mean value of log transformed CFU of different strains at the indicated days post-inoculation. The detection limit is 102 CFU/gram fecal sample.
The specific Cj0843c antiserum was successfully raised and was used for immunoblotting to determine localization of Cj0843c in C. jejuni. As shown in Figure 2B, Cj0843c is detected in the periplasm fraction but not in the spheroplast fraction of wild-type cells (left panel). The Cj0843c was not detected in the periplasm fraction of the isogenic Cj0843c mutant either (left panel in Figure 2B). As a control, CmeR, an intracellular regulator, is only detected in the spheroplast fractions in both wild type and Cj0843c mutants (right panel in Figure 2B). This immunoblotting analysis provides compelling evidence that that Cj0843c is localized in the periplasm, the place where peptidoglycan resides in Gram-negative bacteria.
Lytic Transglycosylase Activity of rCj0843c
The chloroform-treated Cj0843c mutant cells was used as cell wall substrates because the rCj0843c action sites on cell walls from this mutant are possibly intact. As shown in Figure 2C, the decrease in OD450nm (ΔOD450nm) in the reaction solution containing the rCj0843c is significantly larger than PBS control (P < 0.05), indicating the capability of rCj0843c as LT to hydrolyze cell wall.
Cj0843c is Required for C. jejuni Colonization
The role of Cj0843c in in vivo survival of C. jejuni was evaluated using chicken model system. As shown in Figure 2D, wild-type C. jejuni 81–176 could colonize chicken efficiently on days 6 and 10 post-challenge with colonization level as high as 109 CFU per gram of feces. In contrast, the isogenic Cj0843c mutant displayed significantly reduced colonization in the intestine (P < 0.05); the mutant was not detected in most of chickens. Complementation with the plasmid did not rescue the impaired colonization of the Cj0843c mutant, likely due to instability of the plasmid in the complemented strain. To test this, an in vitro plasmid stability assay was performed by subculturing complemented strain every 2–3 days in fresh antibiotic-free MH broth (1:400 dilution) for 10 days; by day 10, majority of the cells (85%) were observed to lose the complemented plasmid.
Discussion
In this study, random transposon mutagenesis was used to identify novel genes contributing to beta-lactam resistance in C. jejuni, which led to the discovery of a putative LT Cj0843c that plays an important role in the intrinsic and acquired beta-lactam resistance in C. jejuni. Notably, the mutation of Cj0843c significantly reduced beta-lactamase activity and MIC for ampicillin (Table 3 and Figure 1). The periplasmic Cj0843c was prevalent and highly conserved in C. jejuni and other 𝜀-proteobacteria, such as C. coli and H. pylori (Supplementary Figure S1 and Supplementary Table S1), indicating the critically conserved role of Cj0843c in cell wall metabolism. Cj0843c is also required for C. jejuni colonization in the intestine (Figure 2D). Mutation of another cell wall metabolism-related gene pgp1 was also observed to impair C. jejuni colonization (Frirdich et al., 2012). However, at this stage, it is still premature to speculate how Cj0843c is involved in colonization. Mutation of Cj0843c may affect C. jejuni physiology and exert pleiotropic effects that are related to C. jejuni in vivo colonization.
The LT also have been observed to be involved in beta-lactam resistance in other bacteria. For instance, single (ΔMltB), double (ΔMltA/ΔMltB; Kraft et al., 1999) or sextuple (Slt70, MltA, MltB, MltC, MltD, and EmtA; Korsak et al., 2005) LT mutants in an E. coli MC1061 displayed significantly decreased beta-lactamase activity. Specific inhibition of the LT Slt70 by bulgecin A suppressed the activity of beta-lactamase AmpC in E. coli (Kraft et al., 1999). Notably, not all PG-degrading enzymes are involved in beta-lactam resistance. For instance, the deletion of two amidases (cleaving the amide bond between N-acetylmuramic acid and L-Ala) and three DD-endopeptidases (acting on the peptide cross-links) had little effect on beta-lactam resistance (Korsak et al., 2005). In C. jejuni, inactivation of Pgp1, which is involved in cleaving monomeric tripeptides to dipeptides (Frirdich et al., 2012), did not affect beta-lactamase activity and the susceptibility of C. jejuni to ampicillin as shown in this study.
The LT-mediated beta-lactam resistance identified in this study indicates LT is a promising target for combinational antimicrobial chemotherapy in C. jejuni, even in 𝜀-proteobacteria. Our genome survey suggested the three-gene operon containing Cj0843c is conserved across 𝜀-proteobacteria. The LT-mediated beta-lactam resistance mechanism might be also prevalent in 𝜀-proteobacteria. For example, the H. pylori homolog (Slt) shares high homology to the Cj0843c (31% aa identity and 51% aa similarity). Recently, Slt also has been found to contribute to amoxicillin resistance in Helicobacter (Bonis et al., 2012). Bulgecin A, the specific inhibitor of H. pylori Slt, could enhance the antimicrobial effect of amoxicillin (Bonis et al., 2012). Given amoxicillin is an important β-lactam component of the triple therapy to eradicate H. pylori infection in human (Tepes et al., 2012), LT inhibitor may serve as a good combination agent (e.g., together with beta-lactam/beta-lactamase inhibitors) to maximize the clinical efficacy of beta-lactam antibiotics.
The decreased β-lactamase activity in LT mutants might be correlated with altered metabolism of peptidoglycan (Kraft et al., 1999). In Gram-negative bacteria, the LT-degraded PG fragments, also called muropeptides, not only serve as cell wall recycling materials during cell growth, but also serve as a signal to turn on the production of β-lactamase through an intracellular transcriptional regulator (Jacobs et al., 1997; Boudreau et al., 2012; Zeng and Lin, 2013). Specifically, muropeptides was either transported into cytoplasm through inner membrane transport AmpG and bound by the regulator AmpR, or directly bound by response regulators of the BlrAB-like two-component regulatory system, to activate the expression of β-lactamase AmpC (Boudreau et al., 2012; Zeng and Lin, 2013). Mutation of the putative LT Cj0843c likely disrupt such intracellular signaling pathway, therefore interfering the induced expression of beta-lactamase and leading to significantly reduced beta-lactamase activity as well as ampicillin MIC observed in this study (Figure 1). This speculation needs to be examined in future studies.
Author Contributions
JL is the project leader who oversights all the experiments described in this manuscript. XZ and JL contributed with the conception and design of the study. XZ and BG were involved in the collection of data. XZ and JL were involved in the analysis and interpretation of data; drafting and revision of the article; and final approval of the article.
Conflict of Interest Statement
The authors declare that the research was conducted in the absence of any commercial or financial relationships that could be construed as a potential conflict of interest.
Acknowledgments
We thank Erin C. Gaynor (University of British Columbia, Canada) for providing genomic DNA of pgp1 mutant, Dr. Patricia Guerry for providing C. jejuni BH-01-0142, and Samantha Brown for providing technical support. This work is supported by The University of Tennessee AgResearch.
Supplementary Material
The Supplementary Material for this article can be found online at: http://journal.frontiersin.org/article/10.3389/fmicb.2015.01292
Footnotes
References
Alfredson, D. A., and Korolik, V. (2005). Isolation and expression of a novel molecular class D beta-lactamase, OXA-61, from Campylobacter jejuni. Antimicrob. Agents Chemother. 49, 2515–2518. doi: 10.1128/AAC.49.6.2515-2518.2005
Black, R. E., Levine, M. M., Clements, M. L., Hughes, T. P., and Blaser, M. J. (1988). Experimental Campylobacter jejuni infection in humans. J. Infect. Dis. 157, 472–479. doi: 10.1093/infdis/157.3.472
Bonis, M., Williams, A., Guadagnini, S., Werts, C., and Boneca, I. G. (2012). The effect of bulgecin A on peptidoglycan metabolism and physiology of Helicobacter pylori. Microb. Drug Resist. 18, 230–239. doi: 10.1089/mdr.2011.0231
Boudreau, M. A., Fisher, J. F., and Mobashery, S. (2012). Messenger functions of the bacterial cell wall-derived muropeptides. Biochemistry 51, 2974–2990. doi: 10.1021/bi300174x
Caldentey, J., and Bamford, D. H. (1992). The lytic enzyme of the Pseudomonas phage phi 6. Purification and biochemical characterization. Biochim. Biophys. Acta 1159, 44–50. doi: 10.1016/0167-4838(92)90073-M
Caldwell, D. B., Wang, Y., and Lin, J. (2008). Development, stability, and molecular mechanisms of macrolide resistance in Campylobacter jejuni. Antimicrob. Agents Chemother. 52, 3947–3954. doi: 10.1128/AAC.00450-08
de Boer, P., Wagenaar, J. A., Achterberg, R. P., Van Putten, J. P., Schouls, L. M., and Duim, B. (2002). Generation of Campylobacter jejuni genetic diversity in vivo. Mol. Microbiol. 44, 351–359. doi: 10.1046/j.1365-2958.2002.02930.x
Duong, T., and Konkel, M. E. (2009). Comparative studies of Campylobacter jejuni genomic diversity reveal the importance of core and dispensable genes in the biology of this enigmatic food-borne pathogen. Curr. Opin. Biotechnol. 20, 158–165. doi: 10.1016/j.copbio.2009.03.004
Elviss, N. C., Williams, L. K., Jorgensen, F., Chisholm, S. A., Lawson, A. J., Swift, C., et al. (2009). Amoxicillin therapy of poultry flocks: effect upon the selection of amoxicillin-resistant commensal Campylobacter spp. J. Antimicrob. Chemother. 64, 702–711. doi: 10.1093/jac/dkp277
Fibriansah, G., Gliubich, F. I., and Thunnissen, A. M. (2012). On the mechanism of peptidoglycan binding and cleavage by the endo-specific lytic transglycosylase MltE from Escherichia coli. Biochemistry 51, 9164–9177. doi: 10.1021/bi300900t
Frirdich, E., Biboy, J., Adams, C., Lee, J., Ellermeier, J., Gielda, L. D., et al. (2012). Peptidoglycan-modifying enzyme Pgp1 is required for helical cell shape and pathogenicity traits in Campylobacter jejuni. PLoS Pathog 8:e1002602. doi: 10.1371/journal.ppat.1002602
Fuda, C., Suvorov, M., Vakulenko, S. B., and Mobashery, S. (2004). The basis for resistance to beta-lactam antibiotics by penicillin-binding protein 2a of methicillin-resistant Staphylococcus aureus. J. Biol. Chem. 279, 40802–40806. doi: 10.1074/jbc.M403589200
Griggs, D. J., Peake, L., Johnson, M. M., Ghori, S., Mott, A., and Piddock, L. J. (2009). Beta-lactamase-mediated beta-lactam resistance in Campylobacter species: prevalence of Cj0299 (blaOXA-61) and evidence for a novel beta-Lactamase in C. jejuni. Antimicrob. Agents Chemother. 53, 3357–3364. doi: 10.1128/AAC.01655-08
Hoang, K. V., Stern, N. J., Saxton, A. M., Xu, F., Zeng, X., and Lin, J. (2011). Prevalence, development, and molecular mechanisms of bacteriocin resistance in Campylobacter. Appl. Environ. Microbiol. 77, 2309–2316. doi: 10.1128/AEM.02094-10
Hoang, K. V., Wang, Y., and Lin, J. (2012). Identification of genetic loci that contribute to Campylobacter resistance to fowlicidin-1, a chicken host defense peptide. Front. Cell Infect. Microbiol. 2:32. doi: 10.3389/fcimb.2012.00032
Hoffmann, S., Batz, M. B., and Morris, J. G. Jr. (2012). Annual cost of illness and quality-adjusted life year losses in the United States due to 14 foodborne pathogens. J. Food Prot. 75, 1292–1302. doi: 10.4315/0362-028X.JFP-11-417
Hughes, R. A., and Cornblath, D. R. (2005). Guillain-barré syndrome. Lancet 366, 1653–1666. doi: 10.1016/S0140-6736(05)67665-9
Humphrey, T., O’brien, S., and Madsen, M. (2007). Campylobacters as zoonotic pathogens: a food production perspective. Int. J. Food Microbiol. 117, 237–257. doi: 10.1016/j.ijfoodmicro.2007.01.006
Iaconis, J. P., and Sanders, C. C. (1990). Purification and characterization of inducible beta-lactamases in Aeromonas spp. Antimicrob. Agents Chemother. 34, 44–51. doi: 10.1128/AAC.34.1.44
Jacobs, C., Frere, J. M., and Normark, S. (1997). Cytosolic intermediates for cell wall biosynthesis and degradation control inducible beta-lactam resistance in gram-negative bacteria. Cell 88, 823–832. doi: 10.1016/S0092-8674(00)81928-5
Jacoby, G. A. (2009). AmpC beta-lactamases. Clin. Microbiol. Rev. 22, 161–182. doi: 10.1128/CMR.00036-08
Koningstein, M., Simonsen, J., Helms, M., Hald, T., and Molbak, K. (2011). Antimicrobial use: a risk factor or a protective factor for acquiring campylobacteriosis? Clin. Infect. Dis. 53, 644–650. doi: 10.1093/cid/cir504
Korfmann, G., and Wiedemann, B. (1988). Genetic control of beta-lactamase production in Enterobacter cloacae. Rev. Infect. Dis. 10, 793–799. doi: 10.1093/clinids/10.4.793
Korsak, D., Liebscher, S., and Vollmer, W. (2005). Susceptibility to antibiotics and beta-lactamase induction in murein hydrolase mutants of Escherichia coli. Antimicrob. Agents Chemother. 49, 1404–1409. doi: 10.1128/AAC.49.4.1404-1409.2005
Kraft, A. R., Prabhu, J., Ursinus, A., and Holtje, J. V. (1999). Interference with murein turnover has no effect on growth but reduces beta-lactamase induction in Escherichia coli. J. Bacteriol. 181, 7192–7198.
Lachance, N., Gaudreau, C., Lamothe, F., and Lariviere, L. A. (1991). Role of the beta-lactamase of Campylobacter jejuni in resistance to beta-lactam agents. Antimicrob. Agents Chemother. 35, 813–818. doi: 10.1128/AAC.35.5.813
Lin, J., Akiba, M., Sahin, O., and Zhang, Q. (2005). CmeR functions as a transcriptional repressor for the multidrug efflux pump CmeABC in Campylobacter jejuni. Antimicrob. Agents Chemother. 49, 1067–1075. doi: 10.1128/AAC.49.3.1067-1075.2005
Lin, J., and Martinez, A. (2006). Effect of efflux pump inhibitors on bile resistance and in vivo colonization of Campylobacter jejuni. J. Antimicrob. Chemother. 58, 966–972. doi: 10.1093/jac/dkl374
Lin, J., Michel, L. O., and Zhang, Q. (2002). CmeABC functions as a multidrug efflux system in Campylobacter jejuni. Antimicrob. Agents Chemother. 46, 2124–2131. doi: 10.1128/AAC.46.7.2124-2131.2002
Lin, J., Sahin, O., Michel, L. O., and Zhang, Q. (2003). Critical role of multidrug efflux pump CmeABC in bile resistance and in vivo colonization of Campylobacter jejuni. Infect. Immun. 71, 4250–4259. doi: 10.1128/IAI.71.8.4250-4259.2003
Lin, J., Wang, Y., and Hoang, K. V. (2009). Systematic identification of genetic loci required for polymyxin resistance in Campylobacter jejuni using an efficient in vivo transposon mutagenesis system. Foodborne Pathog. Dis. 6, 173–185. doi: 10.1089/fpd.2008.0177
Luo, N., Pereira, S., Sahin, O., Lin, J., Huang, S., Michel, L., et al. (2005). Enhanced in vivo fitness of fluoroquinolone-resistant Campylobacter jejuni in the absence of antibiotic selection pressure. Proc. Natl. Acad. Sci. U.S.A. 102, 541–546. doi: 10.1073/pnas.0408966102
Nachamkin, I., Allos, B. M., and Ho, T. (1998). Campylobacter species and Guillain-Barré syndrome. Clin. Microbiol. Rev. 11, 555–567.
Nikaido, H. (1998). Antibiotic resistance caused by gram-negative multidrug efflux pumps. Clin. Infect. Dis. 27(Suppl. 1), S32–S41. doi: 10.1086/514920
Nyati, K. K., and Nyati, R. (2013). Role of Campylobacter jejuni infection in the pathogenesis of Guillain-Barré syndrome: an update. Biomed. Res. Int. 2013:852195. doi: 10.1155/2013/852195
Parkhill, J., Wren, B. W., Mungall, K., Ketley, J. M., Churcher, C., Basham, D., et al. (2000). The genome sequence of the food-borne pathogen Campylobacter jejuni reveals hypervariable sequences. Nature 403, 665–668. doi: 10.1038/35001088
Poly, F., and Guerry, P. (2008). Pathogenesis of Campylobacter. Curr. Opin. Gastroenterol. 24, 27–31. doi: 10.1097/MOG.0b013e3282f1dcb1
Poly, F., Read, T. D., Chen, Y. H., Monteiro, M. A., Serichantalergs, O., Pootong, P., et al. (2008). Characterization of two Campylobacter jejuni strains for use in volunteer experimental-infection studies. Infect. Immun. 76, 5655–5667. doi: 10.1128/IAI.00780-08
Tepes, B., O’connor, A., Gisbert, J. P., and O’morain, C. (2012). Treatment of Helicobacter pylori infection 2012. Helicobacter 17(Suppl. 1), 36–42. doi: 10.1111/j.1523-5378.2012.00981.x
Vila, J., and Martinez, J. L. (2008). Clinical impact of the over-expression of efflux pump in nonfermentative Gram-negative bacilli, development of efflux pump inhibitors. Curr. Drug Targets 9, 797–807. doi: 10.2174/138945008785747806
Wang, Y., and Taylor, D. E. (1990). Natural transformation in Campylobacter species. J. Bacteriol. 172, 949–955.
Yao, R., Alm, R. A., Trust, T. J., and Guerry, P. (1993). Construction of new Campylobacter cloning vectors and a new mutational cat cassette. Gene 130, 127–130. doi: 10.1016/0378-1119(93)90355-7
Zeng, X., Brown, S., Gillespie, B., and Lin, J. (2014). A single nucleotide in the promoter region modulates the expression of the beta-lactamase OXA-61 in Campylobacter jejuni. J. Antimicrob. Chemother. 69, 1215–1223. doi: 10.1093/jac/dkt515
Zeng, X., and Lin, J. (2013). Beta-lactamase induction and cell wall metabolism in Gram-negative bacteria. Front. Microbiol. 4:128. doi: 10.3389/fmicb.2013.00128
Zeng, X., Mo, Y., Xu, F., and Lin, J. (2013a). Identification and characterization of a periplasmic trilactone esterase, Cee, revealed unique features of ferric enterobactin acquisition in Campylobacter. Mol. Microbiol. 87, 594–608. doi: 10.1111/mmi.12118
Zeng, X., Xu, F., and Lin, J. (2013b). Specific TonB-ExbB-ExbD energy transduction systems required for ferric enterobactin acquisition in Campylobacter. FEMS Microbiol. Lett. 347, 83–91. doi: 10.1111/1574-6968.12221
Keywords: beta-lactamase, peptidoglycan, random transposon mutagenesis, lytic transglycosylase
Citation: Zeng X, Gillespie B and Lin J (2015) Important Role of a Putative Lytic Transglycosylase Cj0843c in β-Lactam Resistance in Campylobacter jejuni. Front. Microbiol. 6:1292. doi: 10.3389/fmicb.2015.01292
Received: 20 July 2015; Accepted: 04 November 2015;
Published: 17 November 2015.
Edited by:
Gilberto Igrejas, University of Trás-os-Montes and Alto Douro, PortugalReviewed by:
Elaine Allan, University College London, UKAlessandra Polissi, Università degli Studi di Milano-Bicocca, Italy
Copyright © 2015 Zeng, Gillespie and Lin. This is an open-access article distributed under the terms of the Creative Commons Attribution License (CC BY). The use, distribution or reproduction in other forums is permitted, provided the original author(s) or licensor are credited and that the original publication in this journal is cited, in accordance with accepted academic practice. No use, distribution or reproduction is permitted which does not comply with these terms.
*Correspondence: Jun Lin, amxpbjZAdXRrLmVkdQ==