- 1Tianjin Key Laboratory of Biomedical Material, Institute of Biomedical Engineering, Peking Union Medical College – Chinese Academy of Medical Sciences, Tianjin, China
- 2Department of Materials Chemistry, Nankai University, Tianjin, China
- 3Tianjin Medical University Cancer Institute and Hospital, Tianjin, China
Lysine-porphyrin conjugate 4i has potent photosensitive antibacterial effect on clinical isolated bacterial strains such as Methicillin-resistant Staphylococcus aureus (MRSA), Escherichia coli, and Pseudomonas aeruginosa. The mechanism of photodynamic antibacterial chemotherapy of 4i (4i-PACT) in vitro and the treatment effect in vivo was investigated in this paper. Atomic force microscopy (AFM) revealed that 4i-PACT can effectively destroy membrane and wall of bacteria, resulting in leakage of its content. This was confirmed by dual fluorescent staining with acridine orange/ethidium bromide and measuring materials absorption at 260 nm. Agarose gel electrophoresis measurement showed that 4i-PACT can damage genomic DNA. Healing of wound in rat infected by mixed bacteria showed that the efficiency of 4i-PACT is dependent on the dose of light. These results showed that 4i-PACT has promising bactericidal effect both in vitro and in vivo.
Introduction
The antibiotics for treating common infections have become less effective in recent years. Nearly 1000 of resistance-related β-lactamases, which inactivate the antibiotics, have been identified, a 10 times increase since 1990 (Davies and Davies, 2010; Laxminarayan et al., 2013). This situation made us at the dawn of post antibiotic era (Ryono, 2014). So, it is urgent to find alternative approaches against antibiotics-resistant bacteria. Generally, there are two solutions to this problem. One is to develop novel antibacterial agents, the other is to develop novel physical sterilization. The latter includes radiation sterilization (Nusbaum and Rose, 1979), high pressure electric field sterilization (Sasagawa et al., 2006), microwave sterilization (Dovigo et al., 2009), magnetic sterilization (Haile et al., 2008), and pulse light sterilization (Krishnamurthy et al., 2004). Chemical agents and physical approaches are combined in Photodynamic antimicrobial chemotherapy (PACT) (Wainwright, 1998), which consists of a non-toxic photosensitizer (PS), illumination light of an appropriate wavelength, and ambient molecular oxygen. However, not all of the PS can be used in PACT treatment of bactrerial infection. Only special designed PS can match the PACT demand (Meng et al., 2015). So from this point of view, PACT falls between novel antibacterial agents and novel physical sterilization approaches, namely, a physicochemical treatment. With the advantage of circumventing the bacterial multidrug resistance in the treatment of infection, PACT has received great attention in recent years.
The PS is the crutial element in PACT. In the past few decades, several types of PSs have been reported, such as porphyrin, heme, phthalocyanine, phenothiazinium dyes, BODIPY, methylene blue, conjugated polyelectrolytes, cationic functionalized fullerene, as well as nanoparticals (Wainwright and Crossley, 2004; Wainwright, 2010; Fu et al., 2013; Sperandio et al., 2013). As an ideal PS candidate for photodynamic therapies (Stojiljkovic et al., 2001), Porphyrin can efficiently kill Gram-positive bacteria. Meanwhile, in combination with agents that permeabilize the highly organized outer membrane of Gram-negative bacteria, only cationic PSs, or noncationic PSs are able to kill Gram-negative species (Mbakidi et al., 2013; Prasanth et al., 2014).
In a previous paper, we have reported the synthesis, characterization, and in vitro photodynamic antimicrobial activity of basic amino acid-porphyrin conjugates (Meng et al., 2015), a new class of PSs. Among them, compared with other PSs, 4i exhibits a broader spectrum of photoinactivation in vitro, improved biocompatibility and stability (Bertolini et al., 1990; Nitzan et al., 1992; Tomé et al., 2004; Liu et al., 2012; Gomes et al., 2013; Mbakidi et al., 2013; Prasanth et al., 2014). This paper reported the mechanism of bacterial inactivation by 4i-PACT and its effectiveness in vivo on experimental mixed bacterial infected rat wounds. The results showed that 4i-PACT had potent bactericidal effect both in vitro and in vivo.
Materials and Methods
Bacterial Culture
In this experiment, three clinical bacterial strains, MRSA, Escherichia Coli, and Pseudomonas aeruginosa were isolated at the Tianjin Armed Police Hospital. These bacterial strains were cultured in Luria Bertani (LB) medium. A single colony was used to inoculate 10 mL of liquid medium. The bacteria was grown under aerobic condition at 37°C in a shaking incubator (200 rpm) for 18 h. The bacteria was then collected by centrifugation and resuspended in an equal volume of PBS (Zhao et al., 2014).
Chemicals and Instruments
Porphyrin derivative 4i was synthesized and characterized with the procedure reported by Meng et al. (2015). For clarity, synthetic procedure and characterization detail are omitted. The chemical structure of porphyrin derivative 4i is shown in Figure 1.
Light from a semiconductor laser (7404, Intense, USA) with a wavelength of 650 nm was delivered on the sample via an optic fiber. The energy density of light spot was measured by a light power meter (LM1; Carl Zeiss).
MIC and MBC Determinations
Three bacterial strains were treated with the same procedure. As an example of all of the strains, the treatment of MRSA is described in detail. Experiments were performed in 96-well flat bottom plates. Twenty microliter of MRSA suspension and 180 μL of the compounds were added to each well. The final concentration of the bacterial in the mixture was 106 colony-forming units/mL (CFU/mL). 4i with different mole concentrations were prepared, e.g., 0.98, 1.95, 3.91, 7.8, 15.6, 31.25, 62.5, 125, 250, and 500 μM, respectively. Plates were kept in the dark for 30 min at 37°C and then exposed to light illumination for 30 min or kept in the dark to provide dark control samples. After light exposure, the samples were incubated in dark at 37°C and CFU was evaluated after 18 h. Three sets of independent experiments were performed (Rodrigues et al., 2013).
Dose-Dependent Photoinactivation Effects
Suspensions of bacteria (107 CFU/mL) were incubated at 37°C for 30 min in the dark with 4i (3.91, 7.81, 15.63, 31.25, and 62.50 μM). The suspensions were then loaded into a 96-well plate and illuminated with light with energy density of 6 J/cm2 for the photoreaction. After illumination, 100 μL mixture from each well was taken to determine the CFU. The mixtures were serially diluted to 101, 102, 103, 104, and 105 times of the initial concentrations using PBS. 100 μL of each dilution was plated onto LB agar plates and incubated for 18 h at 37°C in dark. The CFUs were counted, and each experiment was performed in triplicate. The surviving fractions of bacteria were expressed as ratios of CFU produced by bacteria treated with PSs and light (Grinholc et al., 2008).
Atomic Force Microscopy (AFM) Imaging
The bacteria were examined with atomic force microscope (Veeco Multi Mode 8/B0021, Veeco German) equipped with Antimony doped silicon of conical shape (Veeco). The force constant is 3 N/m. Bacterial samples in a concentration of 107 CFU/mL were dropped on to the mica plate surface (about 0.5 cm2), naturally dried at ambient temperature, then were scanned in intelligent mode (Wu et al., 2014).
Fluorescence Imaging
In order to study 4i-PACT effect on the permeability of the bacterial membrane, the samples after 4i-PACT treatment were stained with 4 μL of the acridine orange/ethidium bromide (AO/EB) double fluorescent dyes mixture (100 mg/L of AO and 100 mg/L of EB) for 5 min in dark. Then 5 μL of the stained mixture was dropped on a microscopic glass slide with cover glass and immediately checked by the fluorescent microscope (Nikon Eclipse Ti/B0004, Nikon, Japan) at 200× magnification (Sui et al., 2013).
Genomic DNA Extraction and Electrophoresis
Bacterial suspensions (1 × 107 CFU/mL) were treated with 10 μM 4i as mentioned in experiment 2.4 above. After exposing to the laser light, the genomic DNA was extracted from bacteria immediately using a Genomic DNA Purification Kit (Solarbio, Beijing). DNA samples were gently mixed with 6x loading buffer (Solarbio, Beijing). DNA was analyzed by electrophoresis with 0.8% agarose gel in 1x TAE buffer at 70 V for 30 min. DNA green was incorporated into the agarose gel. The 15000 bp DNA Marker (TAKARA) was used as a molecular weight marker with DNA fragments between 250 and 15000 bp. The light dose had been optimized in vitro in our experiments. Light dose higher than 6 J/cm2 did not improve the efficacy, while the light with lower dose results in a lower bactericidal ability. Without PS 4i, light exposure alone on bacteria in vitro did not have significant bactericidal effect.
Exudation Study for Measuring Materials Absorption at 260 nm
DNA and RNA have absorbance at 260 nm, so exudation study was conducted by measuring the materials absorption at 260 nm for bacteria treated by 10 μM 4i-PACT, 10 μM 4i alone, and PBS, respectively. After treatment, 400 μL of the bacterial suspensions (1 × 108) were filtered to remove the bacteria. OD at 260 nm (OD260) of the supernatant was recorded for treated and pre-treatment control groups.
Excisional Wound Model and Establishment of Infection
The wound healing characteristic of the 4i-PACT was evaluated using a rat model (Dash et al., 2001). The Animal Care and Use Committee of PUMC approved all experimental protocols involving rats. Sprague–Dawley rats from Beijing HFK Bioscience CO.,LTD, weighing about 220 g, were housed at one rat one cage and maintained in dark except PACT treatment. The rats were first anesthetized via intraperitoneal injection of chloral hydrate (300 mg/kg). Then their back was shaved with an electric razor, followed by a depilatory agent. Four circular wounds (1.5 cm in diameter) with a depth of the skin layers were prepared along both sides of the spine. Immediately after that, suspension (50 μL) of mixed bacteria (109 CFU/mL MRSA, 5 × 108 CFU/mL E. coli, 5 × 108 CFU/mL P. aeruginosa) in sterile PBS was inoculated onto the surface of each wound with a pipet tip and then smeared onto the wound surface with an inoculating loop. Next, a bandage was wrapped around each rat to protect the shaved skin and the wounds from other harm. This state was kept for 1 day. Then these rats were used as the wounds model for mixed bacterial infection.
4i-PACT In Vivo
Thirty rats with wound infected by mixed bacteria were divided into five groups: (A) no treatment control; (B) 4i + 100 J/cm2 Light; (C) 4i + 50 J/cm2 Light; (D) 4i + 25 J/cm2 Light; (E) 4i + 12.5 J/cm2 Light. Since light illumination doses alone in this study do not have significant effect on the wound healing, we did not provide light illumination doses alone groups.
Twenty four hours after infection, 50 μL of 4i solution (40 μM in PBS) was injected under eschar of the wound of groups B–E. Then after 30 min for the PS uptaken by the bacteria, rats in groups B–E were illuminated with 650 nm laser (100 mW/cm2), the light dose was kept at 100, 50, 25, 12.5 J/cm2 for group B–E, respectively. On the next day, the same light dose was given to increase the antibacterial effect of 4i-PACT. The above treatment was repeated three times.
The day, on which rats were infected, was regarded as day 1, the widths and lengths of the wounds were measured using a vernier caliper on days 1, 2, 3, 4, 5, 6, 7, 8, 10, and 12. The viability of each group of rats after infection was recorded, respectively. Meanwhile bacterial CFUs in the wound were calculated in the following ways. Swabs collected from the rat wounds were cultured in PBS. Viable bacteria in the PBS were counted by making 10-fold serial dilutions and culturing the dilutions on LB agar for 18 h at 37°C. Then CFUs were counted manually (Kumar et al., 2011; Sudheesh Kumar et al., 2012).
Statistical Analysis
All data are presented as mean ± SEM. The significance of differences between sample means was determined by Student’s t-test using SPSS 19.0.
Results and Discussion
MIC and MBC Determinations
The minimum inhibitory and bactericidal concentration (MIC and MBC) of 4i toward bacteria was studied. Bacterial suspensions (106 CFU/mL) were incubated with the 4i in dark for 30 min at 37°C and then exposed to light illumination (650 nm, 6 J/cm2). The concentration of 4i required to make the suspensions (106 CFU/mL) change visibly from turbid to clear was regarded as the minimum inhibitory concentration (MIC), while the concentration at which no more than five colonies were observed on the plates was regarded as the minimum bactericidal concentration (MBC). As shown in Table 1, compound 4i had high bacterial photoinactivation ability with MIC values of 3.91 μM against MRSA, 1.95 μM against E. coli, and 7.81 μM against P. aeruginosa, respectively; MBC values of 7.81 μM against MRSA, 3.91 μM against E. coli, and 15.60 μM against P. aeruginosa. Meanwhile the dark toxicity of the 4i is relatively weak, with MIC > 62.5 μM, MBC > 125 μM for these three strains. From the values of MBC, it can be concluded that 4i-PACT is very effective toward E coli, P. aeruginosa is the most resistant strain and MRSA is at the middle of them. So 4i-PACT seemly shows higher bactericidal activity toward Gram-negative strains than that of Gram-positive strains. This trend is different from the PACT of other positive charged PS (Sahu et al., 2009; Kharkwal et al., 2011; Ke et al., 2014), which show higher photoinactivation toward the Gram-positive strains than the Gram-negative counterparts. The latter possess a highly organized outer membrane which imparts a higher permeability barrier for the PSs to penetrate into the bacteria. In this study, 4i-PACT shows higher bactericidal effect toward E. coli than MRSA and P. aeruginosa. This unexpected result can be interpreted based upon the chemical structure of 4i, π–π interaction of large planar porphyrin rings, hydrogen bonding interaction and charge interaction from four lysine moieties. The ensemble interaction and difference of the outside membrane structure of these strains lead to the unexpected bactericidal effect of 4i-PACT.
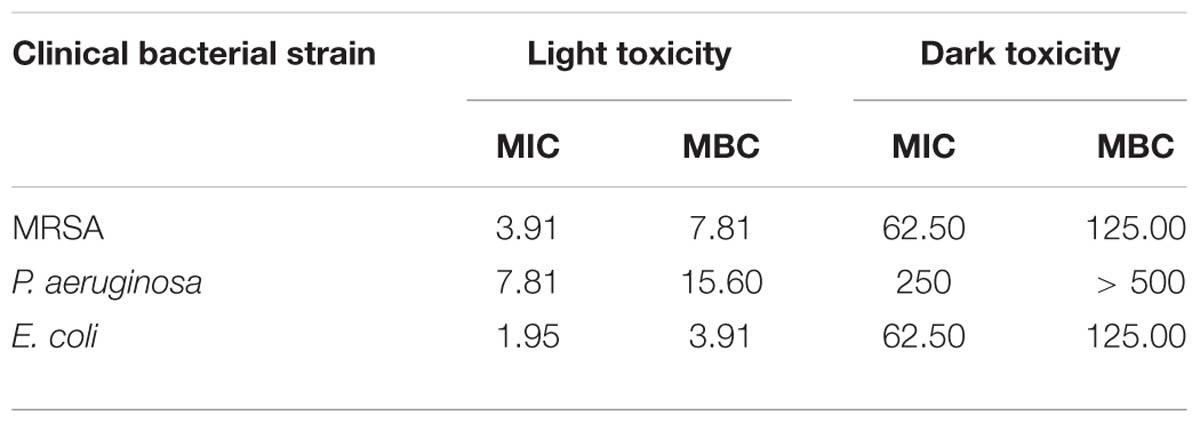
TABLE 1. Minimal inhibitory concentration (MIC, μM) and minimal bactericidal concentration (MBC, μM) of 4i against MRSA, Pseudomonas aeruginosa, Escherichia coli.
Dose-Dependent Photoinactivation Effects
The photoinactivation effect of 4i against MRSA, E. coli and P. aeruginosa strains was dose-dependent (Figure 2). A sharp decrease in bacterial survival fraction with increase of the concentration was observed. These three strains were incubated with 3.9 μM 4i in the dark for 30 min at 37°C, then illuminated by the 650 nm laser with power of 6 J/cm2. 4.35, 6.96, and 3.56 log10 reduction in bacterial survival fraction was observed for the clinical isolated MRSA, E. coli and P. aeruginosa, respectively. As a control, 4i did not show any bactericidal effect to these strains in dark at less than 10 μM.
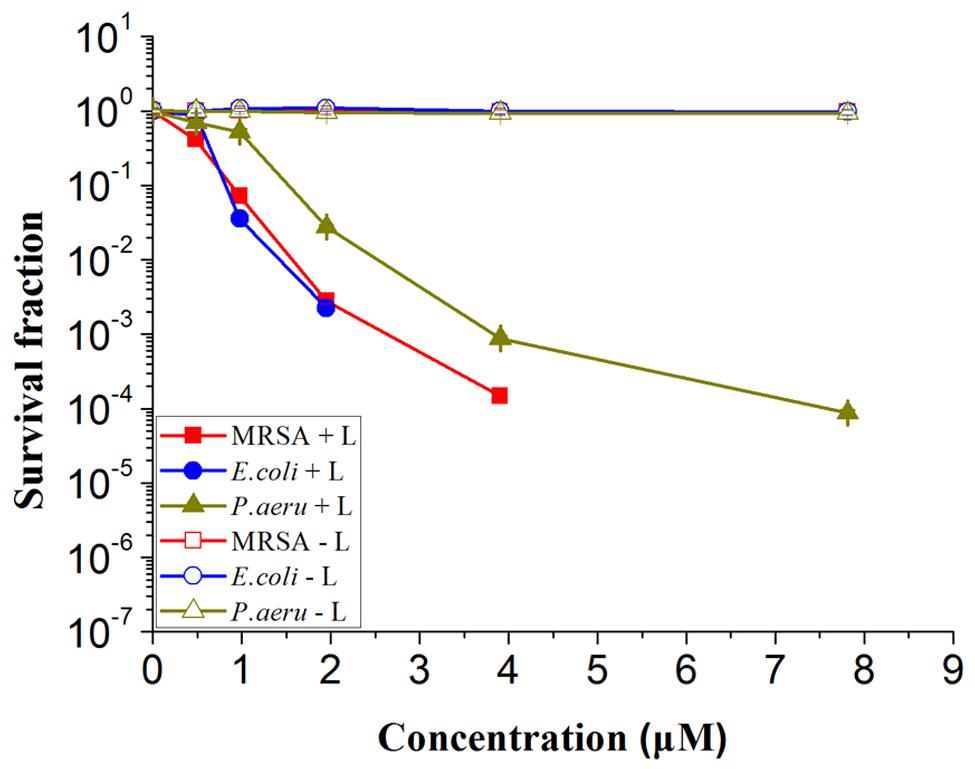
FIGURE 2. Survival fraction of three bacterial strains treated by different concentration of 4i with and without illumination. Light toxicity marked by MRSA + L, Escherichia coli + L, Pseudomonas aeruginosa + L and dark toxicity marked by MRSA – L, E. coli – L, P. aeruginosa – L respectively.
Morphology Changes
Changes of the bacterial structure can be clearly revealed at morphological level by AFM. The representative results are shown in Figure 3. The normal ball shape structure of MRSA (Figure 3g), the rod-like shape of P. aeruginosa (Figure 3h) and E. coli (Figure 3i) was observed without any treatment. The size and shape of the bacteria agree with that reported in previous study (Eaton et al., 2008). For these three strains treated by 4i in the dark (Figures 3d-f), it can be seen that the bacterial structure is complete. Particles scattered at the surface of bacteria may be the aggregates of 4i. In the groups treated by 4i-PACT (Figures 3a–c), it was found that bacteria is damaged and fragmented. Some irregular aggregation of the dead corpse was detected. Researchers have found that the roughness, size and shape of bacterial strains are slightly altered after PACT treatment with Toluidine Blue O (Sahu et al., 2009; Jin et al., 2010; Lin et al., 2012). The leakage from Staphylococcus aureus cell suspension is greatly increased (Sahu et al., 2009), suggesting that cytoplasmic materials are lost through the damaged bacterial membrane. Results in this study showed that bacterial strains are completely broken into pieces. Thus, 4i-PACT treatment appeared to have potent effect on the bacterial envelope, including damaging the bacterial wall and membrane.
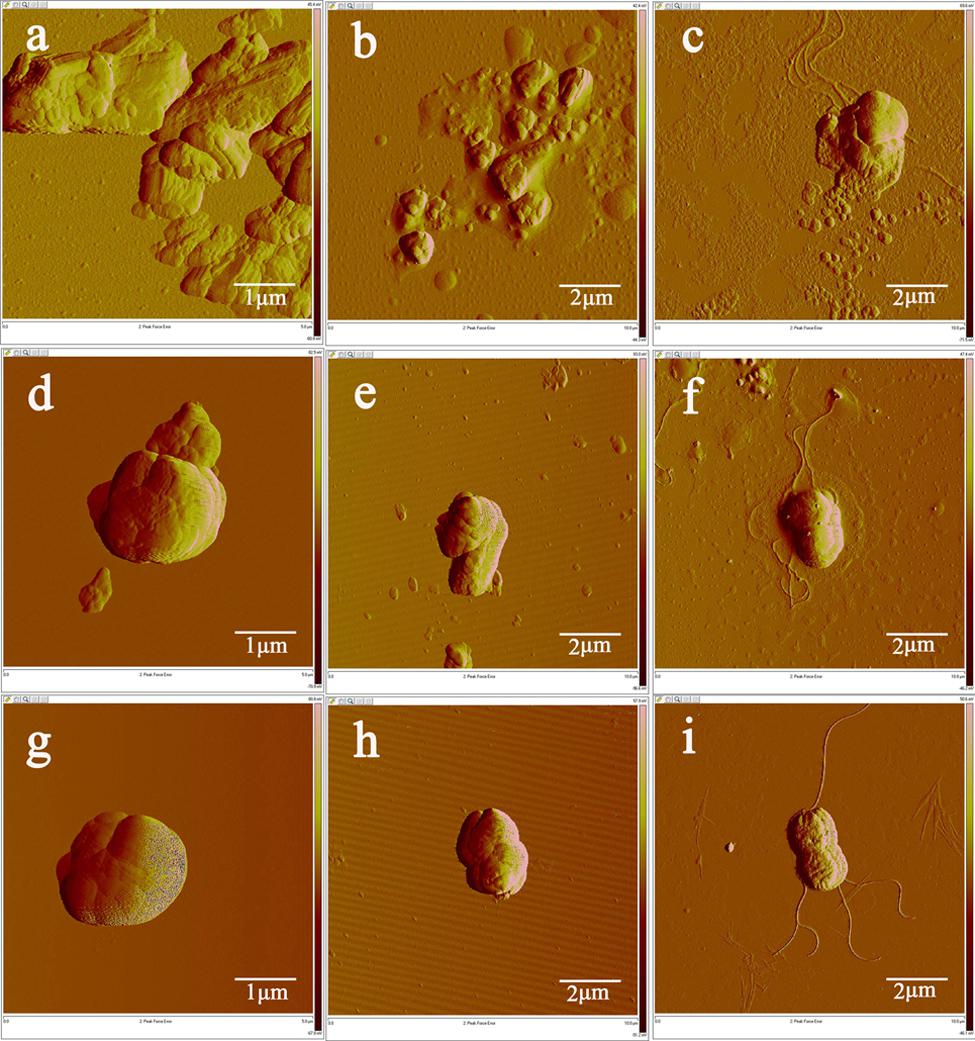
FIGURE 3. Fluorescence microscopy image of three bacterial strains treated by 10 μM 4i with and without illumination. (a) MRSA treated by 4i-PACT, (d) 4i with no light, (g) control; (b) P. aeruginosa treated by 4i-PACT, (e) 4i with no light, (h) control; (c) E. coli treated by the 4i-PACT, (f) 4i with no light, (i) control.
Membrane Integrity
The fluorescence microscopy was used to investigate the permeability of bacterial membrane before and after 4i-PACT. Fluorescent dyes (AO/EB), as the indicator of cell membrane damage, were used to assess the antibacterial activity of 4i-PACT. Living bacteria was observed as green and dead as red, respectively. Figures 4g–i show fluorescence microscopy images of the control samples, where no 4i was added, the bacteria is dispersed and alive with green color. Figures 4a–c are the fluorescence microscopy images of MRSA, P. aeruginosa and E. coli treated by 4i-PACT (10 μM, 6J/cm2, 650 nm), respectively, where most of the bacteria is dead with red color. On the other hand, fluorescence microscopy images of MRSA (Figure 4d), P. aeruginosa (Figure 4e), and E. coli (Figure 4f) treated by10 μM 4i without illumination showed little bactericidal effect. The above results mean that 4i-PACT can damage the membrane of bacteria and change the permeability of the bacterial membrane. This again explains why the antimicrobial effect of 4i-PACT is so strong.
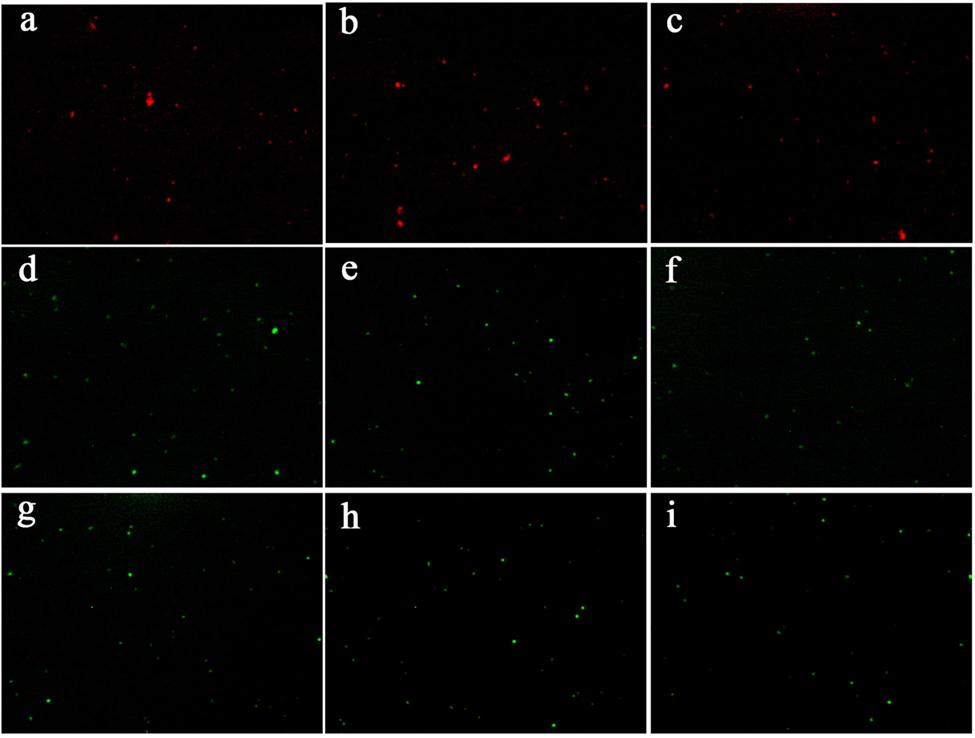
FIGURE 4. AFM image of three bacterial strains treated by 10 μM 4i with and without illumination. (a) MRSA treated by 4i-PACT, (d) 4i with no light, (g) control; (b) P. aeruginosa treated by 4i-PACT, (e) 4i with no light, (h) control; (c) E. coli treated by 4i-PACT, (f) 4i with no light, (i) control.
Photodynamic Effect on Genomic DNA
The influence of 4i-PACT on bacteria was further studied from a genomic point of view such as DNA leakage or damage. The electrophoresis graph (Figure 5) shows that the DNA bands, extracted from the processed bacterial cells, disappear after 4i-PACT (10 μM, 6 J/cm2, 650 nm), while a clear DNA band is detected in the control groups with no 4i-PACT or treated by 4i alone. This result is consistent with the AFM morphological study as well as the fluorescent staining, which reveal that the 4i-PACT breaks the bacterial membrane and wall and causes the leakage of genomic DNA. The absence of DNA bands can be attributed to either the leakage of bacteria membrane or the break of the DNA. So we conducted another independent experiment. The naked DNA extracted from each strains was treated by 4i-PACT. The agarose gel electrophoresis graph still shows no DNA bands. This result indicated that 4i-PACT can break DNA into pieces. This phenomenon is consistent with the previous results (Menezes et al., 1990; Choi et al., 2010; Lin et al., 2012). Generally, the reason for multidrug resistance in cellular level is due to the drug only blocked or weakened the partial binding site or signal pathway, while the whole cellular bodies were kept, so it is easy to develop multidrug resistance. Double effects of 4i-PACT, destroying bacterial membrane and damaging the DNA, can account for the vanish of DNA band in electrophoresis graph. This is the reason why bacterial strains have no resistant to 4i-PACT.
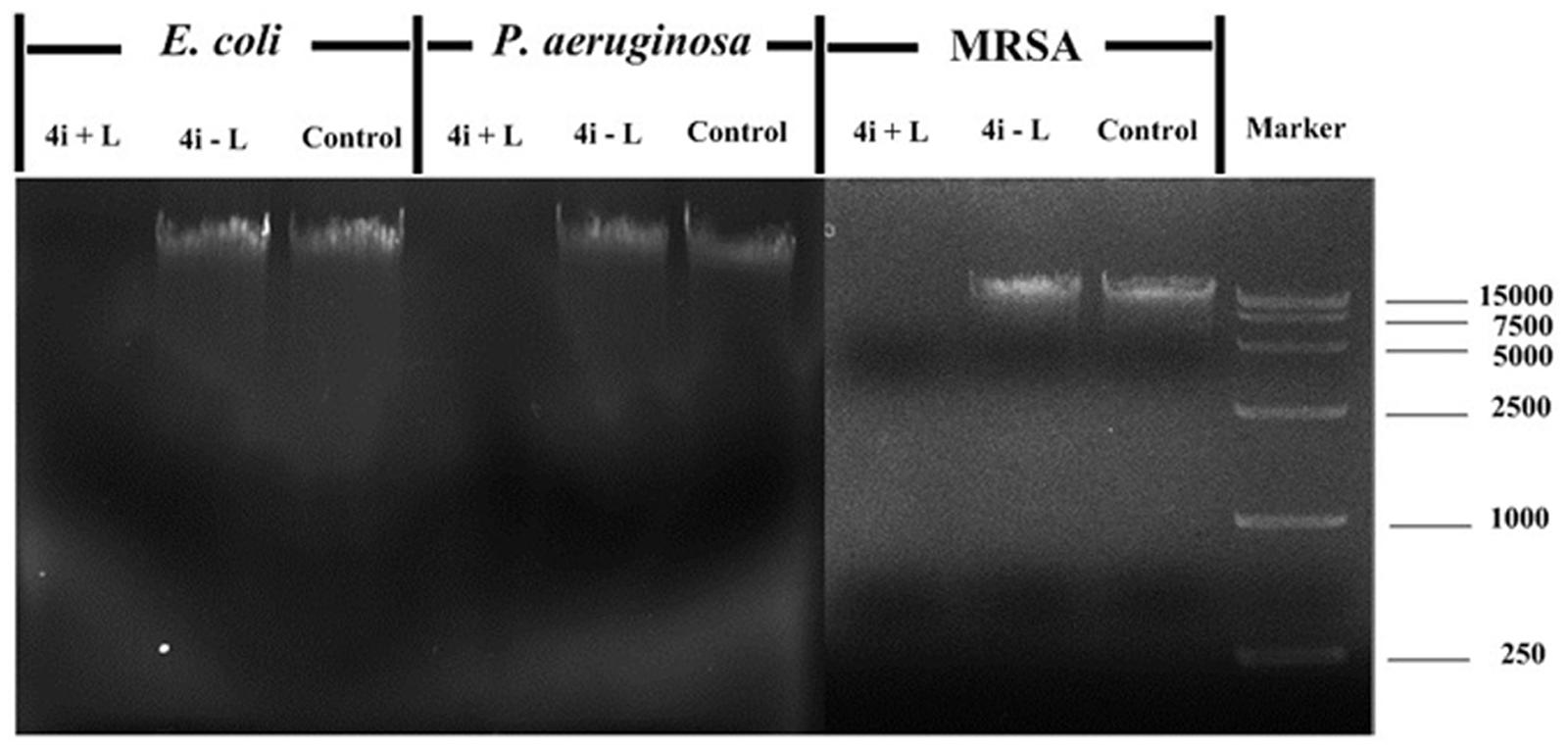
FIGURE 5. Agarose gel electrophoresis of three bacterial strains after treated by 10 μM 4i with and without illumination. Bacteria treated with 4i-PACT (4i + L), treated with 4i in the dark (4i–L) and no treatment (control).
Bacterial Content Exudation
Release of intracellular components is a good indicator of membrane integrity. Small ions such as potassium and phosphate tend to exude first, followed by large molecules such as DNA, RNA, and other materials. Since these nucleotides have strong UV absorption at 260 nm, measuring the absorbance at 260 nm can reveal the information of membrane integrity (Denyer and Hugo, 1991; Chen and Cooper, 2002). As shown in Table 2, after 10 μM 4i-PACT, the bacterial content exudation from MRSA, P. aeruginosa, and E. Coli are increased by 247.87, 188.98, 336.67%, respectively, compared with pre-treatment samples, while changes less than 10% was observed in the control or 4i group. This result is consistent with that of AFM and fluorescent images. These data are larger than that reported by Sahu et al. (2009), which were only 20–60% increase compared with the control groups.
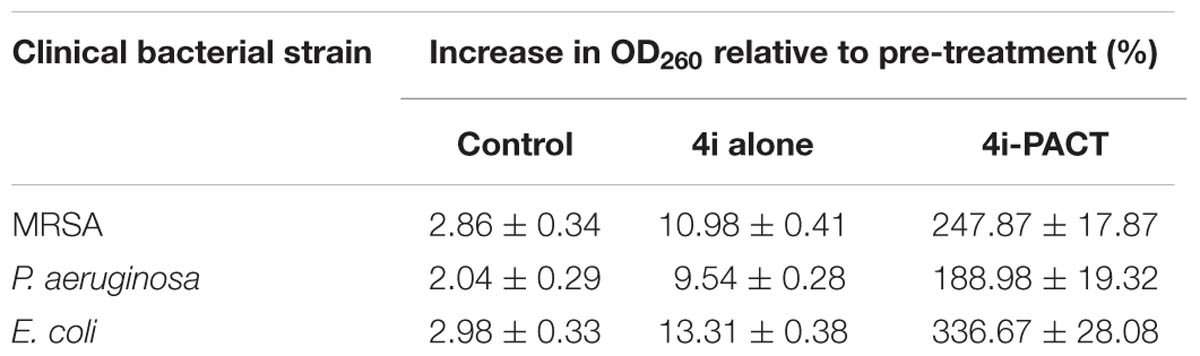
TABLE 2. Bacterial content exudation in the control (PBS), 4i alone, and 4i-PACT treatment groups (n = 4, mean ± SD) measured at 260 nm.
In Vivo Antibacterial Activity and Wound-Healing Effect
In vivo antibiotics experiment, generally 5 or 10 times MIC was chosen as the working concentration. 40 μM of 4i, five times of MIC (Singh et al., 2006; Dai et al., 2009) was chosen as the working dose. The antibacterial effect of 4i-PACT with different light dose was studied. The wound healing rate is highly light dose dependent (Figure 6). Control group A, with no treatment, showed less wound healing at each observation time point. A rapid wound healing was observed in the treatment of groups C (50 J/cm2) and D (25 J/cm2) during the day 4 to 8 after infection (p < 0.05). After day 8, wound healing became slow till completely healed on day 12. However, in the groups B (100 J/cm2) and E (12.5 J/cm2), rapid wound closure happened from day 7 to 10. On the day 8, mean wound area ratios of B–E were 0.4252 ± 0.0512, 0.1515 ± 0.0312, 0.1823 ± 0.0777, and 0.2273 ± 0.0239, respectively, but in the control group wound area ratio was 0.6619 ± 0.0834, indicating B–E groups had better wound healing ratio compared with the control group. Almost total wound closure was observed by the day 12 post infection in all treated groups.
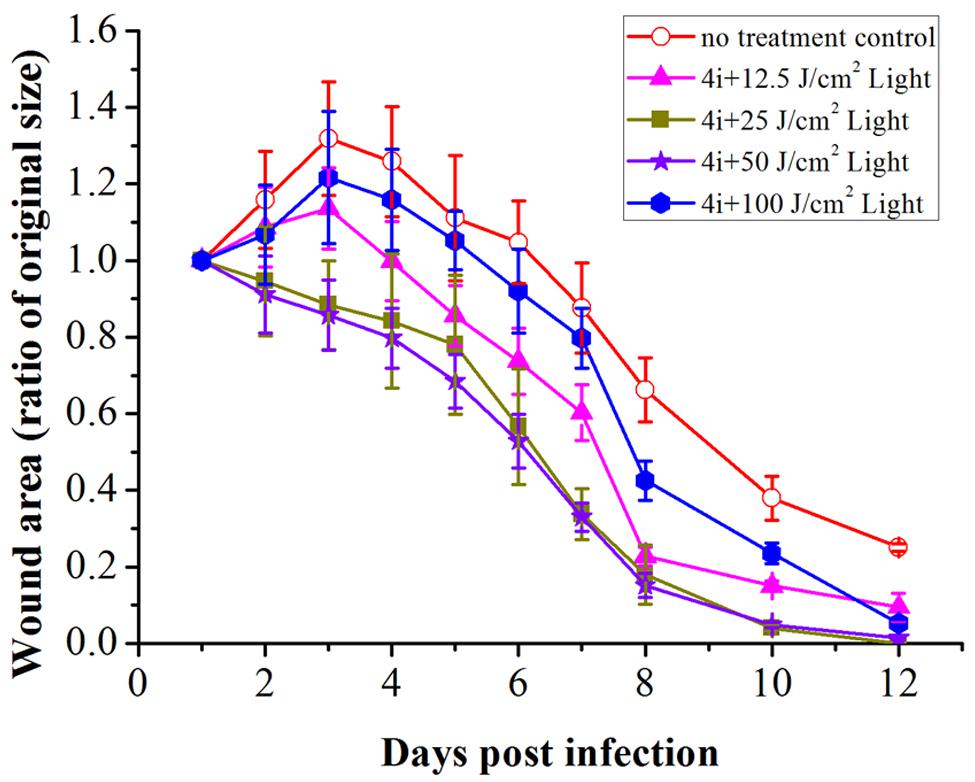
FIGURE 6. Wound healing ratio over time in bacterial infected rats with and without 4i-PACT. Each point represents the mean ratio of the area of the original wound. Data is expressed as mean ± SEM. N = 6 in each group.
The viable bacteria in wound tissue were counted as an index of the bactericidal effect of 4i-PACT. On days 1, 2, 3, 4, 5, 6, 7, 8 post infection, PACT-treated groups exhibited obvious reduction in bacterial viability compared with the no treatment control group (Figure 7). At the same time point, the decrease of viable bacterial count is in the order of light illumination dose, B > C > D > E. This suggests that 4i-PACT accelerates wound healing via bactericidal effect against MRSA, P. aeruginosa, and E. coli. However, the wound healing ratio is in the order of C > D > E > B, instead of paralleling of the bactericidal effect. This indicates that 4i-PACT effect on normal tissue should be considered. A rationalized interpretation can be given based upon the multiple function of 4i-PACT in vivo: 4i-PACT not only photoinactivates bacteria, but also causes the normal tissue damaged and repaired. The observed effect is the balance of the ensemble results. For either a weaker 4i-PACT with a lower illumination dose (12.5 J/cm2 in group E), or a stronger 4i-PACT with a higher illumination dose (100 J/cm2 in group B), the total results are not good. The best result is from treatment with medium light dose like group C (50 J/cm2). So for the practical application of PACT further study is requires.
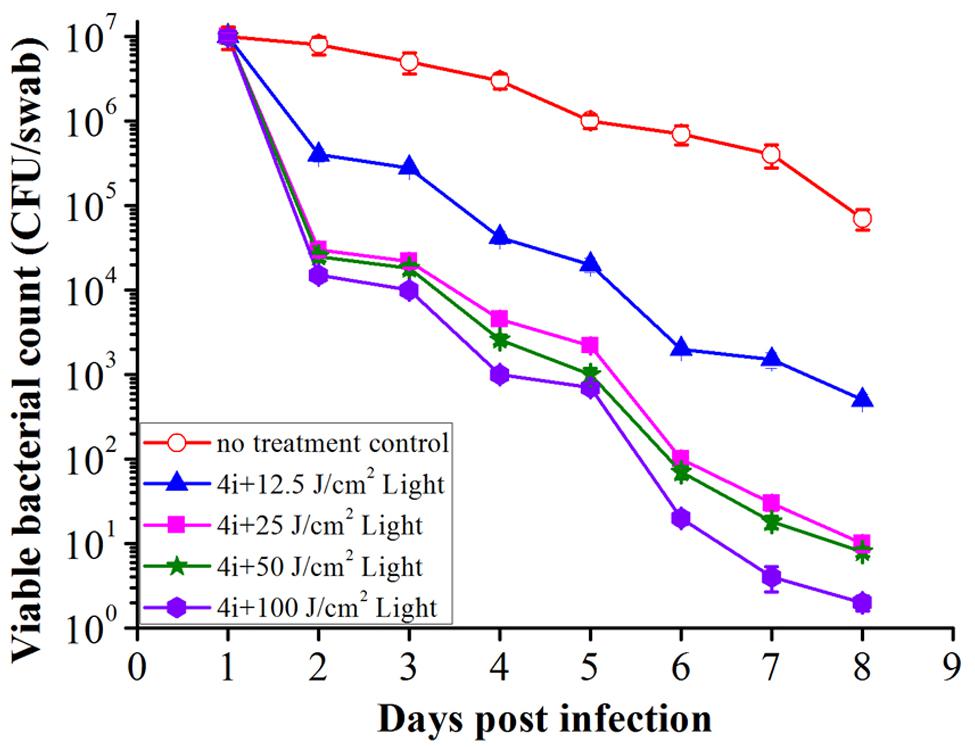
FIGURE 7. The mean bacterial counts of the swabs collected from the rat wounds during the 8 days post infection. Data is expressed as mean ± SEM. N = 6 in each group.
In the no treatment control group, two rats died on day 2 and day 4, rats in other groups all survived the experiment. Therefore, the viability of rats post treatment indicates that 4i-PACT is more efficient in the treatment of mixed bacterial infection (Figure 8).
Conclusion
This paper studied the photoinactivation mechanism of 4i-PACT in vitro and its wound healing effect on wound rat model infected by mixed bacteria. 4i-PACT (3.91 μM, 6J/cm2, 650 nm) had a broad potent antibacterial spectrum: 4.35, 6.96, and 3.56 log10 reduction in bacterial survival for MRSA, E. coli, and P. aeruginosa, respectively. AFM revealed that 4i-PACT can destroy bacterial wall and membrane. This is further confirmed by Dual fluorescent staining with AO/EB. Agarose gel electrophoresis indicated that 4i-PACT can damage the genomic DNA of bacteria. Absorbance at 260 nm reveals that 4i-PACT could cause bacterial content leakage. 4i-PACT breaks not only bacterial wall but also the genomic DNA. That is why the bacterial strains have no resistance to 4i-PACT. Wound healing effect on mixed bacterial infected rat model revealed that 4i-PACT in vivo is highly potent and light-dose dependent. The ensemble effect is the balanced effect of 4i-PACT both to normal tissue and bacterial strains. The best light dose is 50 J/cm2. Overall, 4i-PACT is a highly efficient treatment modality for bacterial infection in vitro or in vivo.
Author Contributions
TL designed experiments; SM, ZX, LP, and YG carried out experiments; YG and ZX analyzed experimental results, CW analyzed sequencing data. TL, ZX, and YG wrote the manuscript. TL designed experiments; SM, ZX, LP, and YG carried out experiments; YG and ZX analyzed experimental results, CW analyzed sequencing data. TL, ZX, and YG wrote the manuscript.
Conflict of Interest Statement
The authors declare that the research was conducted in the absence of any commercial or financial relationships that could be construed as a potential conflict of interest.
Acknowledgments
This work was supported by the key technologies R & D program of Tianjin (12ZCDZSY11900), Peking Union Medical College Peking Union Youth Fund grant to ZX (NO.3332015030) and the National Natural Science Foundation of China (81202415).
References
Bertolini, G., Rossi, F., Valduga, G., Jori, G., and Van Lier, J. (1990). Photosensitizing activity of water-and lipid-soluble phthalocyanines on Escherichia coli. FEMS Microbiol. Lett. 71, 149–155. doi: 10.1111/j.1574-6968.1990.tb03814.x
Chen, C. Z., and Cooper, S. L. (2002). Interactions between dendrimer biocides and bacterial membranes. Biomaterials 23, 3359–3368. doi: 10.1016/S0142-9612(02)00036-4
Choi, S. S., Lee, H. K., and Chae, H. S. (2010). In vitro photodynamic antimicrobial activity of methylene blue and endoscopic white light against Helicobacter pylori 26695. J. Photochem. Photobiol. B Biol. 101, 206–209. doi: 10.1016/j.jphotobiol.2010.07.004
Dai, T., Tegos, G. P., Lu, Z., Huang, L., Zhiyentayev, T., Franklin, M. J., et al. (2009). Photodynamic therapy for Acinetobacter baumannii burn infections in mice. Antimicrob. Agents Chemother. 53, 3929–3934. doi: 10.1128/AAC.00027-09
Dash, G., Suresh, P., and Ganapaty, S. (2001). Studies on hypoglycaemic and wound healing activities of Lantana camara Linn. J. Nat. Remedies 1, 105–110.
Davies, J., and Davies, D. (2010). Origins and evolution of antibiotic resistance. Microbiol. Mol. Biol. Rev. 74, 417–433. doi: 10.1128/MMBR.00016-10
Denyer, S. P., and Hugo, W. B. (1991). Mechanisms of Action of Chemical Biocides. Their Study and Exploitation. Oxford: Blackwell Scientific Publications.
Dovigo, L. N., Pavarina, A. C., Ribeiro, D. G., De Oliveira, J. A., Vergani, C. E., and Machado, A. L. (2009). Microwave disinfection of complete dentures contaminated in vitro with selected bacteria. J. Prosthodont. 18, 611–617. doi: 10.1111/j.1532-849X.2009.00489.x
Eaton, P., Fernandes, J. C., Pereira, E., Pintado, M. E., and Malcata, F. X. (2008). Atomic force microscopy study of the antibacterial effects of chitosans on Escherichia coli and Staphylococcus aureus. Ultramicroscopy 108, 1128–1134. doi: 10.1016/j.ultramic.2008.04.015
Fu, X.-J., Fang, Y., and Yao, M. (2013). Antimicrobial photodynamic therapy for methicillin-resistant Staphylococcus aureus infection. Biomed Res. Int. 2013, 159157. doi: 10.1155/2013/159157
Gomes, M. C., Silva, S., Faustino, M. A., Neves, M. G., Almeida, A., Cavaleiro, J. A., et al. (2013). Cationic galactoporphyrin photosensitisers against UV-B resistant bacteria: oxidation of lipids and proteins by 1 O 2. Photochem. Photobiol. Sci. 12, 262–271. doi: 10.1039/c2pp25149c
Grinholc, M., Szramka, B., Kurlenda, J., Graczyk, A., and Bielawski, K. P. (2008). Bactericidal effect of photodynamic inactivation against methicillin-resistant and methicillin-susceptible Staphylococcus aureus is strain-dependent. J. Photochem. Photobiol. Biol. 90, 57–63. doi: 10.1016/j.jphotobiol.2007.11.002
Haile, M., Pan, Z., Gao, M., and Luo, L. (2008). Efficacy in microbial sterilization of pulsed magnetic field treatment. Int. J. Food Eng. 4, 1–14. doi: 10.2202/1556-3758.1177
Jin, H., Huang, X., Chen, Y., Zhao, H., Ye, H., Huang, F., et al. (2010). Photoinactivation effects of hematoporphyrin monomethyl ether on Gram-positive and-negative bacteria detected by atomic force microscopy. Appl. Microbiol. Biotechnol. 88, 761–770. doi: 10.1007/s00253-010-2747-4
Ke, M.-R., Eastel, J. M., Ngai, K. L. K., Cheung, Y.-Y., Chan, P. K. S., Hui, M., et al. (2014). Photodynamic inactivation of bacteria and viruses using two monosubstituted zinc (II) phthalocyanines. Eur. J. Med. Chem. 84, 278–283. doi: 10.1016/j.ejmech.2014.07.022
Kharkwal, G. B., Sharma, S. K., Huang, Y. Y., Dai, T., and Hamblin, M. R. (2011). Photodynamic therapy for infections: clinical applications. Lasers Surg. Med. 43, 755–767. doi: 10.1002/lsm.21080
Krishnamurthy, K., Demirci, A., and Irudayaraj, J. (2004). Inactivation of Staphylococcus aureus by pulsed UV-light sterilization. J. Food Prot. 67, 1027–1030.
Kumar, A., Srivastava, K., Kumar, S. R., Siddiqi, M., Puri, S. K., Sexana, J. K., et al. (2011). 4-Anilinoquinoline triazines: a novel class of hybrid antimalarial agents. Eur. J. Med. Chem. 46, 676–690. doi: 10.1016/j.ejmech.2010.12.003
Laxminarayan, R., Duse, A., Wattal, C., Zaidi, A. K., Wertheim, H. F., Sumpradit, N., et al. (2013). Antibiotic resistance—the need for global solutions. Lancet Infect. Dis. 13, 1057–1098. doi: 10.1016/S1473-3099(13)70318-9
Lin, S. L., Hu, J. M., Tang, S. S., Wu, X. Y., Chen, Z. Q., and Tang, S. Z. (2012). Photodynamic inactivation of methylene blue and tungsten-halogen lamp light against food pathogen Listeria monocytogenes. Photochem. Photobiol. 88, 985–991. doi: 10.1111/j.1751-1097.2012.01154.x
Liu, F., Soh Yan Ni, A., Lim, Y., Mohanram, H., Bhattacharjya, S., and Xing, B. (2012). Lipopolysaccharide neutralizing peptide–porphyrin conjugates for effective photoinactivation and intracellular imaging of Gram-negative bacteria strains. Bioconjug. Chem. 23, 1639–1647. doi: 10.1021/bc300203d
Mbakidi, J.-P., Herke, K., Alvès, S., Chaleix, V., Granet, R., Krausz, P., et al. (2013). Synthesis and photobiocidal properties of cationic porphyrin-grafted paper. Carbohydr. Polym. 91, 333–338. doi: 10.1016/j.carbpol.2012.08.013
Menezes, S., Capella, M., and Caldas, L. (1990). Photodynamic action of methylene blue: repair and mutation in Escherichia coli. J. Photochem. Photobiol. B Biol. 5, 505–517. doi: 10.1016/1011-1344(90)85062-2
Meng, S., Xu, Z., Hong, G., Zhao, L., Zhao, Z., Guo, J., et al. (2015). Synthesis, characterization and in vitro photodynamic antimicrobial activity of basic amino acid–porphyrin conjugates. Eur. J. Med. Chem. 92, 35–48. doi: 10.1016/j.ejmech.2014.12.029
Nitzan, Y., Gutterman, M., Malik, Z., and Ehrenberg, B. (1992). Inactivation of gram-negative bacteria by photosensitized porphyrins. Photochem. Photobiol. 55, 89–96. doi: 10.1111/j.1751-1097.1992.tb04213.x
Nusbaum, H., and Rose, R. (1979). The effects of radiation sterilization on the properties of ultrahigh molecular weight polyethylene. J. Biomed. Mater. Res. 13, 557–576. doi: 10.1002/jbm.820130404
Prasanth, C. S., Karunakaran, S. C., Paul, A. K., Kussovski, V., Mantareva, V., Ramaiah, D., et al. (2014). Antimicrobial photodynamic efficiency of novel cationic porphyrins towards periodontal Gram-positive and Gram-negative pathogenic bacteria. Photochem. Photobiol. 90, 628–640. doi: 10.1111/php.12198
Rodrigues, G. B., Dias-Baruffi, M., Holman, N., Wainwright, M., and Braga, G. U. (2013). In vitro photodynamic inactivation of Candida species and mouse fibroblasts with phenothiazinium photosensitisers and red light. Photodiagnosis Photodyn. Ther. 10, 141–149. doi: 10.1016/j.pdpdt.2012.11.004
Ryono, R. (2014). Carbapenem-resistant Enterobacteriaceae (CRE) distribution within the veterans affairs healthcare system: 2013. Open Forum Infect. Dis. 1(Suppl. 1), S140–S141. doi: 10.1093/ofid/ofu052.221
Sahu, K., Bansal, H., Mukherjee, C., Sharma, M., and Gupta, P. K. (2009). Atomic force microscopic study on morphological alterations induced by photodynamic action of Toluidine Blue O in Staphylococcus aureus and Escherichia coli. J. Photochem. Photobiol. B Biol. 96, 9–16. doi: 10.1016/j.jphotobiol.2009.03.008
Sasagawa, A., Yamazaki, A., Kobayashi, A., Hoshino, J., Ohshima, T., Sato, M., et al. (2006). Inactivation of Bacillus subtilis spores by a combination of hydrostatic high-pressure and pulsed electric field treatments. High Press. Sci. Technol. 16, 45–53. doi: 10.4131/jshpreview.16.45
Singh, M., Govindarajan, R., Nath, V., Rawat, A. K. S., and Mehrotra, S. (2006). Antimicrobial, wound healing and antioxidant activity of Plagiochasma appendiculatum Lehm. et Lind. J. Ethnopharmacol. 107, 67–72. doi: 10.1016/j.jep.2006.02.007
Sperandio, F. F., Huang, Y.-Y., and Hamblin, M. R. (2013). Antimicrobial photodynamic therapy to kill Gram-negative bacteria. Recent Pat. Antiinfect. Drug Discov. 8, 108–120. doi: 10.2174/1574891X113089990012
Stojiljkovic, I., Evavold, B. D., and Kumar, V. (2001). Antimicrobial properties of porphyrins. Expert Opin. Investig. Drugs 10, 309–320. doi: 10.1517/13543784.10.2.309
Sudheesh Kumar, P., Lakshmanan, V.-K., Anilkumar, T., Ramya, C., Reshmi, P., Unnikrishnan, A., et al. (2012). Flexible and microporous chitosan hydrogel/nano ZnO composite bandages for wound dressing: in vitro and in vivo evaluation. ACS Appl. Mater. Interfaces 4, 2618–2629. doi: 10.1021/am300292v
Sui, M., Zhang, L., Sheng, L., Huang, S., and She, L. (2013). Synthesis of ZnO coated multi-walled carbon nanotubes and their antibacterial activities. Sci. Total Environ. 452, 148–154. doi: 10.1016/j.scitotenv.2013.02.056
Tomé, J. P., Neves, M. G., Tomé, A. C., Cavaleiro, J. A., Soncin, M., Magaraggia, M., et al. (2004). Synthesis and antibacterial activity of new poly-S-lysine-porphyrin conjugates. J. Med. Chem. 47, 6649–6652. doi: 10.1021/jm040802v
Wainwright, M. (1998). Photodynamic antimicrobial chemotherapy (PACT). J. Antimicrob. Chemother. 42, 13–28. doi: 10.1093/jac/42.1.13
Wainwright, M. (2010). ‘Safe’photoantimicrobials for skin and soft-tissue infections. Int. J. Antimicrob. Agents 36, 14–18. doi: 10.1016/j.ijantimicag.2010.03.002
Wainwright, M., and Crossley, K. B. (2004). Photosensitising agents—circumventing resistance and breaking down biofilms: a review. Int. Biodeterior. Biodegradation 53, 119–126. doi: 10.1016/j.ibiod.2003.11.006
Wu, M., He, J., Ren, X., Cai, W.-S., Fang, Y.-C., and Feng, X.-Z. (2014). Development of functional biointerfaces by surface modification of polydimethylsiloxane with bioactive chlorogenic acid. Colloids Surf. B Biointerfaces 116, 700–706. doi: 10.1016/j.colsurfb.2013.11.010
Keywords: lysine-porphyrin conjugate, photodynamic antimicrobial chemotherapy, antibiotic resistance, photosensitizer, mixed bacterial-infected wounds
Citation: Xu Z, Gao Y, Meng S, Yang B, Pang L, Wang C and Liu T (2016) Mechanism and In Vivo Evaluation: Photodynamic Antibacterial Chemotherapy of Lysine-Porphyrin Conjugate. Front. Microbiol. 7:242. doi: 10.3389/fmicb.2016.00242
Received: 22 October 2015; Accepted: 15 February 2016;
Published: 02 March 2016.
Edited by:
Gilberto Igrejas, University of Trás-os-Montes and Alto Douro, PortugalReviewed by:
Martin Picard, CNRS-Institut de Biologie Physico-Chimique, FranceAbiola Olumuyiwa Olaitan, Aix-Marseille University, France
Copyright © 2016 Xu, Gao, Meng, Yang, Pang, Wang and Liu. This is an open-access article distributed under the terms of the Creative Commons Attribution License (CC BY). The use, distribution or reproduction in other forums is permitted, provided the original author(s) or licensor are credited and that the original publication in this journal is cited, in accordance with accepted academic practice. No use, distribution or reproduction is permitted which does not comply with these terms.
*Correspondence: Tianjun Liu, bGl1dGlhbmp1bkBob3RtYWlsLmNvbQ==