- 1Institute for Bio-Medical Convergence, International St. Mary's Hospital and College of Medicine, Catholic Kwandong University, Incheon, Korea
- 2Institute of Life Science and Biotechnology, Sungkyunkwan University, Suwon, Korea
- 3College of Pharmacy, Chung-Ang University, Seoul, Korea
Upon hyperosmotic stress, the High Osmolarity Glycerol (HOG) pathway is activated, resulting in phosphorylation of the stress-activated protein kinasei Hog1 (O'Rourke et al., 2002; Saito and Posas, 2012; Brewster and Gustin, 2014). The Hog1/mitogen-activated protein kinase-mediated signaling cascades are essential for sensing hyperosmotic stress and for transmitting these signals to the nucleus to modulate gene expression (O'Rourke et al., 2002; Saito and Posas, 2012; Brewster and Gustin, 2014). MAPK-mediated cascade pathways are composed of three serine/threonine protein kinases (MAPK kinase kinase, MAPK kinase, and MAPK). This signal transduction pathway is well-conserved in eukaryotes (O'Rourke et al., 2002; Saito and Posas, 2012; Brewster and Gustin, 2014).
Phosphorylated Hog1 stimulates expression of genes encoding enzymes involved in glycerol production and uptake (O'Rourke et al., 2002; Saito and Posas, 2012; Gomar-Alba et al., 2013; Lee et al., 2013; Babazadeh et al., 2014; Brewster and Gustin, 2014). The glycerol production starts with the reduction of the glycolytic intermediate di-hydroxyl-acetone phosphate (DHAP) to glycerol-3-phosphate (G3P) catalyzed by the NAD+-dependent glycerol-3-phospate dehydrogenase (GPD; Ansell et al., 1997; Pahlman et al., 2001; Valadi et al., 2004). Increased expression of GPD1 enhances glycerol production under hyperosmotic stress (Albertyn et al., 1994; Rep et al., 1999). In addition, Hog1 appears to stimulate the 6-phosphofructo-2-kinase Pfk26, which produces fructose-2,6-diphosphate (F26DP), an allosteric activator of the glycolytic enzyme phosphofructokinase (Pfk1; Dihazi et al., 2004). Hog1 is rapidly phosphorylated upon curcumin treatment, an active polyphenol derived from the spice turmeric (Azad et al., 2014). Trichosporonoides oedocephalis is known to produce large amounts of polyols (erythritol and glycerol). Tohog1 null mutation increased erythritol production and decreased glycerol production, respectively (Li et al., 2016). Therefore, Hog1 is considered to have an important role in the metabolic control, however little is known regarding the changes of metabolites induced by Hog1 from a metabolic perspective. Nuclear magnetic resonance (NMR) spectroscopy is a rapid method that requires minimal sample preparation (Beckonert et al., 2007; Kim et al., 2010). In addition, NMR is a useful technique for structure elucidation due to its various two-dimensional measurements, which makes NMR an ideal tool for the identification and quantification of metabolites (Beckonert et al., 2007; Kim et al., 2010).
Materials and Methods
Materials
The Beauveria bassiana strain EFCC783 was grown on sterilized cellophane (Bio-Rad) in SDAY medium. The Saccharomyces cerevisiae wild-type (W303) and hog1Δ strains were grown on YPD medium.
Complementation Assay of BbHog1 in hog1Δ Strain
BbHog1 was sub-cloned into the yeast expression vector pD1218 (DNA2.0). Each pD1218 construct was then transformed into the hog1Δ mutants. Positive transformants were selected in YPD medium containing geneticin (200 μg/ml), and confirmed using PCR. To evaluate the ability of BbHog1 to rescue cell growth under hyperosmotic stress, growth was compared on YPD versus YPD containing 0.8 M NaCl. Plates were incubated for 24 h at 30°C.
Western Blot Analysis
Yeast cells were treated with 0.8 M NaCl for 20 min. Their protein extracts (30 μg) were separated by SDS-PAGE using 10% polyacrylamide gel and analyzed by Western blotting using the anti-p-Hog1/p38 or anti-Hog1/p38 antibodies (Cell Signaling) and a secondary horseradish peroxidase-conjugated anti-IgG antibody (Sigma-Aldrich). Enhanced chemiluminescent reagent (Bio-Rad) was used to detect the proteins.
Proton Nuclear Magnetic Resonance (1H-NMR)
Yeast cells were treated with 0.8 M NaCl for 40 min, and then washed following the rapid quenching procedure [60% MeOH and 0.85% AMBIC (pH 7.4)]. The quenched cells were dissolved in 90 mM phosphate buffer in D2O, containing 0.01 % TSP as an internal standard. After cell lysis, the supernatants were clarified by centrifuging at 15,000 rpm for 30 min at 4°C, filtered by 3 kDa cutoff filters (Millipore), and collected into 1.5 ml tubes. Then, 600 μl of each filtered extract was loaded into 5 mm NMR tubes. 1H-NMR spectra were acquired at 300 K on a 600.13-MHz Bruker Avance Spectrometer (Bruker) using the standard zgpr pulse sequence (Kim et al., 2010). In total, 128 transients were gathered into 32 K data points with a relaxation delay of 2 s, an acquisition time of 1.70 s per scan, and a spectra width of 12.0 ppm. The NMR spectra were analyzed using Chenomx NMR suite software v8.1 (Fan, 1996).
1H-NMR Raw Data Processing
1H-NMR raw files (.fid) produced by a 600.13-MHz Bruker Advance spectrometer were imported and analyzed using Chenomx NMR suite software v8.1 (Chenomx). The Chenomx NMR suite software v8.1 (Chenomx) converted 1H-NMR raw files (.fid) to Chenomx file format (.cnx). Each spectral intensity dataset was normalized to the assigned chemical compounds according to total sum of the spectral regions, converted to Microsoft Office format, and imported into Web-based MetaboAnalyst v3.0 software to carry out our normalization, scaling, and multivariate analysis. Multivariate statistical analyses were performed by one-way ANOVA using PASW Statistics 22 software (IBM) followed by a Tukey's significant difference test. Significance was determined with a p-value threshold (<0.05). Metabolites levels were normalized using the log2 function and then, mean centering and Pareto scaling was applied for all PCA and Heatmaps by MetaboAnalyst v3.0. The hierarchical clustering analysis (HCA) also shows clustering based on the replicate values of averaged appropriate and Ward's method on Euclidean distance matrix.
Results
Beauveria bassiana is an important entomopathogenic fungus with wide application for biological control of harmful insects in the world (Posada et al., 2004). We isolated a gene for the Hog1 through searching the genome database of B. bassiana. The predicted molecular weight of BbHog1 was 40.9 kDa and its pI was 5.45, respectively. Our initial attempt at producing BbHog1 knockout mutants was unsuccessful. Moreover, we failed to get the Bbhog1Δ (Zhang et al., 2009). Alternatively, we employed complementation strategies using S. cerevisiae hog1Δ mutant. The hog1Δ strain was transformed with plasmids driving the expression of BbHog1. The viability of transformed yeast cells was then determined in medium containing 0.8 M NaCl. BbHog1 successfully complemented the hog1Δ phenotype under hyperosmotic stress (Figure S1). In addition, BbHog1 can be phosphorylated in yeast under hyperosmotic stress (Figure S2). Therefore, our results indicate that BbHog1 is functional in S. cerevisiae.
To explore metabolic changes during hyperosmotic stress response in hog1Δ/vector and hog1Δ/BbHog1, we profiled the metabolites in each strain using 1H-NMR spectroscopy. Chemical shifts of signals were assigned to the metabolites in the area of amino acids, organic acids, carbohydrates, and nucleotide derivatives. According to the 1H-NMR spectra, we identified 32 metabolites from the whole yeast extracts with chemical shifts and coupling patterns (Figures S3, S4). The main variations are summarized in the form of a heatmap shown in Figure 1A and Figure S5. 1H-NMR spectra of hog1Δ/vector and hog1Δ/BbHog1 showed differential resonance spectra under hyperosmotic stress.
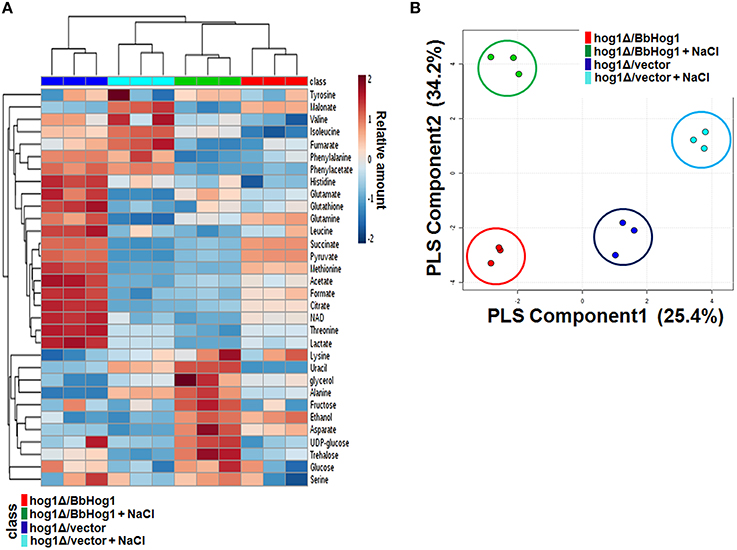
Figure 1. Heatmap of main metabolite variations (A) and PLS-DA score plot (B) in hog1Δ/vector and hog1Δ/BbHog1 under hyperosmotic stress.
Multivariate data analysis is used to identify differences or similarities among the samples. Each point of the score plot in multivariate analysis represents an individual sample. Grouping and outliers of samples can be easily observed in a score plot. Partial least squares-discriminant analysis (PLS-DA) was used to investigate intrinsic variation in 1H-NMR data. In the PLS-DA score plot, two principal components, PC1 and PC2, were calculated with the R2Y and Q2Y parameters of 0.990 and 0.955 (Figure 1B). As shown in Figure 1B, the PLS-DA score plot of 1H-NMR spectra showed a clear separation between hog1Δ/vector and hog1Δ/BbHog1 under hyperosmotic stress.
Glycerol and trehalose function as representative osmolytes in order to cope with changes in hyperosmotic stress (Hounsa et al., 1998; Hohmann, 2002). According to 1H-NMR data, cellular concentrations of glucose, glycerol (Albertyn et al., 1994), and trehalose were increased under hyperosmotic stress in hog1Δ/BbHog1, but not in hog1Δ/vector (Figure 2A). Glucose is the main energy source in yeast. Maintenance of ATP, the main energy source, homeostasis is critical for all cells. In response to hyperosmotic stress, the higher energy demands required for hyperosmotic stress tolerance are allotted by a rapid alteration in cellular ATP metabolism (Olz et al., 1993; Oren, 1999). Therefore, the increased levels of glucose induced by Hog1 might be required to satisfy the higher energy requirements, as well as increased production of osmolytes such as glycerol and trehalose during hyperosmotic stress signaling.
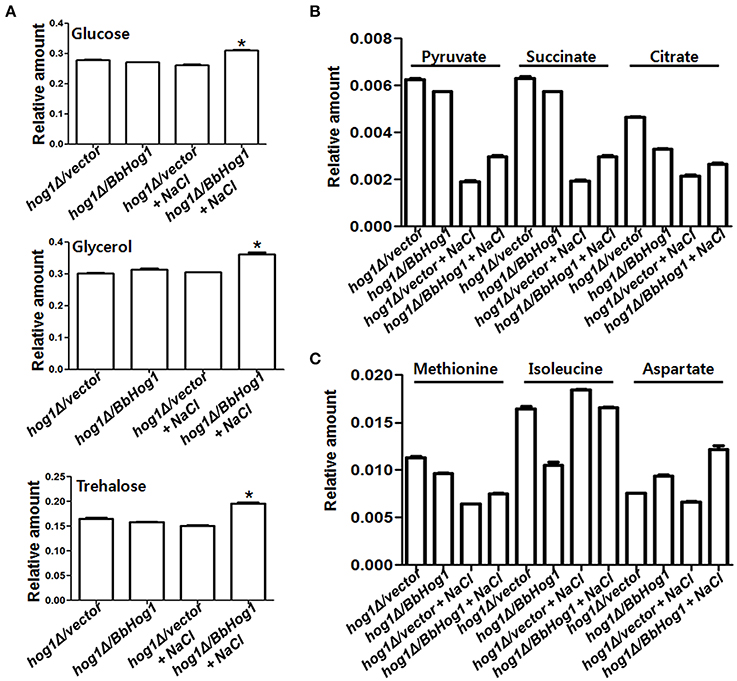
Figure 2. Hog1 regulates cellular concentrations of a variety of metabolites under hyperosmotic stress. Cellular concentrations of glucose, glycerol, and trehalose (A), and succinate, citrate and pyruvate (B), and Met, Ile, and Asp (C) are differentially regulated in hog1Δ/vector and hog1Δ/BbHog1 under hyperosmotic stress. Experimental values are means of three independent experiments with standard deviation (Student's t-test, *p < 0.01).
In general, glucose inhibits the expression of genes involved in respiration (Hardie et al., 1998). In addition, multiple stress environments suppress the expression of genes associated with the tricarboxylic acid (TCA) cycle (Diano et al., 2009). Through, 1H-NMR data, we investigated whether Hog1 regulates cellular concentrations of TCA cycle intermediates under hyperosmotic stress. As shown in Figure 2B, the cellular concentrations of succinate and citrate were slightly reduced in hog1Δ/BbHog1 under normal conditions. Succinate and citrate were largely reduced in hog1Δ/vector in response to hyperosmotic stress, but these reductions were significantly reduced in hog1Δ/BbHog1. Pyruvate can be made from glucose through glycolysis, or converted back to glucose via gluconeogenesis. Under hyperosmotic stress, the cellular level of pyruvate had a similar pattern with those of succinate and citrate (Figure 2B). Therefore, our results suggest that hyperosmotic stress lead to reductions in pyruvate and TCA cycle intermediates, and that Hog1 plays a role in mediating these metabolic changes under hyperosmotic stress.
Hyperosmotic stress alters cellular concentration of amino acids as the cell attempts to survive against hyperosmotic stress (Kovács et al., 2012). As shown in Figure 2C, the cellular concentration of methionine (Met) was largely reduced in hog1Δ/vector in response to hyperosmotic stress, but these reductions were significantly reduced in hog1Δ/BbHog1. Cellular concentration of isoleucine (Ile) was largely reduced under normal conditions in hog1Δ/BbHog1, but not in hog1Δ/vector. In case of aspartate (Asp), its cellular concentration was increased under hyperosmotic stress in hog1Δ/BbHog1, not in hog1Δ/vector (Figure 2C). Therefore, our results suggest that hyperosmotic stress induces metabolic changes of amino acids such as Met, Ile, and Asp, and Hog1 plays a role in these metabolic regulations during hyperosmotic stress.
In summary, this work represents that MAP kinase Hog1 regulates cellular concentrations of a variety of metabolites during hyperosmotic stress signaling.
Author Contributions
JK and GS designed the study. JK and JO performed the experiments. JK, JO, and GS analyzed data. JK and GS wrote the paper.
Data Access
Our 1H-NMR source files were deposited in figshare (https://dx.doi.org/10.6084/m9.figshare.2066538.v1).
Conflict of Interest Statement
The authors declare that the research was conducted in the absence of any commercial or financial relationships that could be construed as a potential conflict of interest.
Acknowledgments
This research was supported by the Convergence of Conventional Medicine and Traditional Korean Medicine R&D project funded by the Ministry of Health & Welfare through the Korea Health Industry Development Institute (HI15C0094) and a research fund from Catholic Kwandong University (CKURF-201407040001).
Supplementary Material
The Supplementary Material for this article can be found online at: http://journal.frontiersin.org/article/10.3389/fmicb.2016.00732/full
Figure S1. BbHog1 is functional in S. cerevisiae. Yeast cells were spotted on YPD plates and YPD plates containing 0.8 M NaCl at 30°C for 24 h.
Figure S2. BbHog1 phosphorylation under hyperosmotic stress. Yeast cells were treated with 0.8 M NaCl for 20 min, and their protein extracts were then subjected to Western blot analysis with anti-p-Hog1 or anti-Hog1 antibodies.
Figure S3. 1H-NMR spectra of the metabolites of S. cerevisiae.
Figure S4. Chemical shifts of metabolite compounds according to 1H-NMR.
Figure S5. Relative metabolite contents of S. cerevisiae according to 1H-NMR. Experimental values are the means of three independent experiments with standard deviation.
References
Albertyn, J., Hohmann, S., Thevelein, J. M., and Prior, B. A. (1994). GPD1, which encodes glycerol-3-phosphate dehydrogenase, is essential for growth under osmotic stress in Saccharomyces cerevisiae, and its expression is regulated by the highosmolarity glycerol response pathway. Mol. Cell. Biol. 14, 4135–4144. doi: 10.1128/MCB.14.6.4135
Ansell, R., Granath, K., Hohmann, S., Thevelein, J. M., and Adler, L. (1997). The two isoenzymes for yeast NAD+-dependent glycerol 3-phosphate dehydrogenase encoded by GPD1 and GPD2 have distinct roles in osmoadaptation and redox regulation. EMBO J. 16, 2179–2187. doi: 10.1093/emboj/16.9.2179
Azad, G. K., Singh, V., Thakare, M. J., Baranwal, S., and Tomar, R. S. (2014). Mitogen-activated protein kinase Hog1 is activated in response to curcumin exposure in the budding yeast Saccharomyces cerevisiae. BMC Microbiol. 14:317. doi: 10.1186/s12866-014-0317-0
Babazadeh, R., Furukawa, T., Hohmann, S., and Furukawa, K. (2014). Rewiring yeast osmostress signalling through the MAPK network reveals essential and non-essential roles of Hog1 in osmoadaptation. Sci. Rep. 4:4697. doi: 10.1038/srep04697
Beckonert, O., Keun, H. C., Ebbels, T. M., Bundy, J., Holmes, E., Lindon, J. C., et al. (2007). Metabolic profiling, metabolomic and metabonomic procedures for NMR spectroscopy of urine, plasma, serum and tissue extracts. Nat. Protoc. 2, 2692−2703. doi: 10.1038/nprot.2007.376
Brewster, J. L., and Gustin, M. C. (2014). Hog1: 20 years of discovery and impact. Sci. Signal. 7, re7. doi: 10.1126/scisignal.2005458
Diano, A., Peeters, J., Dynesen, J., and Nielsen, J. (2009). Physiology of Aspergillus niger in oxygen-limited continuous cultures: influence of aeration, carbon source concentration and dilution rate. Biotechnol. Bioeng. 103, 956–965. doi: 10.1002/bit.22329
Dihazi, H., Kessler, R., and Eschrich, K. (2004). High osmolarity glycerol (HOG) pathway-induced phosphorylation and activation of 6-phosphofructo-2-kinase are essential for glycerol accumulation and yeast cell proliferation under hyperosmotic stress. J. Biol. Chem. 279, 23961–23968. doi: 10.1074/jbc.M312974200
Fan, T. M. T. (1996). Metabolite profiling by one- and two-dimensional NMR analysis of complex mixtures. Prog. Nucl. Magn. Reson. Spectrosc. 28, 161–219. doi: 10.1016/0079-6565(96)90002-3
Gomar-Alba, M., Alepuz, P., and del Olmo, M. L. (2013). Dissection of the elements of osmotic stress response transcription factor Hot1 involved in the interaction with MAPK Hog1 and in the activation of transcription. Biochim. Biophys. Acta 1829, 1111–1125. doi: 10.1016/j.bbagrm.2013.07.009
Hardie, D. G., Carling, D., and Carlson, M. (1998). The AMP-activated/SNF1 protein kinase subfamily: metabolic sensors of the eukaryotic cell? Annu. Rev. Biochem. 67, 821–855.
Hohmann, S. (2002). Osmotic adaptation in yeast–control of the yeast osmolyte system. Int. Rev. Cytol. 215, 149–187. doi: 10.1016/S0074-7696(02)15008-X
Hounsa, C. G., Brandt, E. V., Thevelein, J., Hohmann, S., and Prior, B. A. (1998). Role of trehalose in survival of Saccharomyces cerevisiae under osmotic stress. Microbiology 144, 671–680. doi: 10.1099/00221287-144-3-671
Kim, H. K., Choi, Y. H., and Verpoorte, R. (2010). NMR-based metabolomic analysis of plants. Nat. Protoc. 5, 536−549. doi: 10.1038/nprot.2009.237
Kovács, Z., Simon-Sarkadi, L., Vashegyi, I., and Kocsy, G. (2012). Different accumulation of free amino acids during short- and long-term osmotic stress in wheat. Sci. World J. 2012, 216521. doi: 10.1100/2012/216521
Lee, J., Reiter, W., Dohnal, I., Gregori, C., Beese-Sims, S., Kuchler, K., et al. (2013). MAPK Hog1 closes the S. cerevisiae glycerol channel Fps1 by phosphorylating and displacing its positive regulators. Genes Dev. 27, 2590–2601. doi: 10.1101/gad.229310.113
Li, L., Yang, T., Guo, W., Ju, X., Hu, C., Tang, B., et al. (2016). Construction of an efficient mutant strain of Trichosporonoides oedocephalis with HOG1 gene deletion for production of erythritol. J. Microbiol. Biotechnol. 26, 700–709. doi: 10.4014/jmb.1510.10049
O'Rourke, S. M., Herskowitz, I., and O'shea, E. K. (2002). Yeast go the whole HOG for the hyperosmotic response. Trends Genet. 18, 405–412. doi: 10.1016/S0168-9525(02)02723-3
Olz, R., Larsson, K., Adler, L., and Gustafsson, L. (1993). Energy flux and osmoregulation of Saccharomyces cerevisiae grown in chemostats under NaCl stress. J. Bacteriol. 175, 2205–2213.
Pahlman, A. K., Granath, K., Ansell, R., Hohmann, S., and Adler, L. (2001). The yeast glycerol 3-phosphatases Gpp1p and Gpp2p are required for glycerol biosynthesis and differentially involved in the cellular responses to osmotic, anaerobic, and oxidative stress. J. Biol. Chem. 276, 3555–3563. doi: 10.1074/jbc.M007164200
Posada, F., Vega, F. E., Rehner, S. A., Blackwell, M., Weber, D., Suh, S. O., et al. (2004). Syspastospora parasitica, a mycoparasite of the fungus Beauveria bassiana attacking the Colorado potato beetle Leptinotarsa decemlineata: a tritrophic association. J. Insect Sci. 4, 24. doi: 10.1093/jis/4.1.24
Rep, M., Albertyn, J., Thevelein, J. M., Prior, B. A., and Hohmann, S. (1999). Different signalling pathways contribute to the control of GPD1 gene expression by osmotic stress in Saccharomyces cerevisiae. Microbiology 145, 715–727. doi: 10.1099/13500872-145-3-715
Saito, H., and Posas, F. (2012). Response to hyperosmotic stress. Genetics 192, 289–318. doi: 10.1534/genetics.112.140863
Valadi, A., Granath, K., Gustafsson, L., and Adler, L. (2004). Distinct intracellular localization of Gpd1p and Gpd2p, the two yeast isoforms of NAD+-dependent glycerol-3-phosphate dehydrogenase, explains their different contributions to redox-driven glycerol production. J. Biol. Chem. 279, 39677–39685. doi: 10.1074/jbc.M403310200
Keywords: Hog1/MAP kinase, hyperosmotic stress, 1H-nuclear magnetic resonance, Beauveria bassiana, Saccharomyces cerevisiae
Citation: Kim J, Oh J and Sung G-H (2016) MAP Kinase Hog1 Regulates Metabolic Changes Induced by Hyperosmotic Stress. Front. Microbiol. 7:732. doi: 10.3389/fmicb.2016.00732
Received: 21 January 2016; Accepted: 02 May 2016;
Published: 18 May 2016.
Edited by:
Dongsheng Zhou, Beijing Institute of Microbiology and Epidemiology, ChinaCopyright © 2016 Kim, Oh and Sung. This is an open-access article distributed under the terms of the Creative Commons Attribution License (CC BY). The use, distribution or reproduction in other forums is permitted, provided the original author(s) or licensor are credited and that the original publication in this journal is cited, in accordance with accepted academic practice. No use, distribution or reproduction is permitted which does not comply with these terms.
*Correspondence: Jiyoung Kim, jiyoungk1972@gmail.com;
Gi-Ho Sung, sung97330@gmail.com
†These authors have contributed equally to this work.