- 1Laboratory of Basic an Applied Microbiology, Department of Biology, Faculty of Chemistry and Biology, University of Santiago, Santiago, Chile
- 2Program of Cellular and Molecular Biology, Institute of Biomedical Sciences, Faculty of Medicine, University of Chile, Santiago, Chile
Members of the genus Leptospirillum are aerobic iron-oxidizing bacteria belonging to the phylum Nitrospira. They are important members of microbial communities that catalyze the biomining of sulfidic ores, thereby solubilizing metal ions. These microorganisms live under extremely acidic and metal-loaded environments and thus must tolerate high concentrations of reactive oxygen species (ROS). Cobalamin (vitamin B12) is a cobalt-containing tetrapyrrole cofactor involved in intramolecular rearrangement reactions and has recently been suggested to be an intracellular antioxidant. In this work, we investigated the effect of the exogenous addition of cobalamin on oxidative stress parameters in Leptospirillum group II strain CF-1. Our results revealed that the external supplementation of cobalamin reduces the levels of intracellular ROSs and the damage to biomolecules, and also stimulates the growth and survival of cells exposed to oxidative stress exerted by ferric ion, hydrogen peroxide, chromate and diamide. Furthermore, exposure of strain CF-1 to oxidative stress elicitors resulted in the transcriptional activation of the cbiA gene encoding CbiA of the cobalamin biosynthetic pathway. Altogether, these data suggest that cobalamin plays an important role in redox protection of Leptospirillum strain CF-1, supporting survival of this microorganism under extremely oxidative environmental conditions. Understanding the mechanisms underlying the protective effect of cobalamin against oxidative stress may help to develop strategies to make biomining processes more effective.
Introduction
Acidophilic biomining bacteria from the genus Leptospirillum are of biotechnological interest due to their use in the recovery of economically important metals from sulfidic ores (Rawlings, 2002; Watling et al., 2014). Members of this genus are chemolithoautotrophic microorganisms that have the ability to oxidize ferrous (Fe2+) to ferric (Fe3+) iron, a reaction which is highly relevant for the leaching of ores and thus, for the recovery of metals.
Leptospirillum strains are able to live in acidic leaching solutions containing elevated concentrations of metals which are toxic to most living organisms. In aerobic environments, iron (Fe2+/Fe3+) is more soluble and hence, more available under acidic conditions than at neutral pH (Quatrini et al., 2007; Johnson et al., 2012), and thus its bioavailability is usually high. Iron is an essential micronutrient in bacteria and is important for several cellular processes by acting as part of redox centers of various proteins in central metabolism and in electron-transport chains (Carpenter and Payne, 2014). In addition, iron can be used as a primary energy source by many acidophilic microorganisms (Bonnefoy and Holmes, 2012). However, this element can induce damage to cell components due to its ability to generate reactive oxygen species (ROS) through the Fenton or the Haber–Weiss reactions (Papanikolaou and Pantopoulos, 2005; Valko et al., 2005). Besides iron, high concentrations of other heavy metals like copper, nickel and cobalt and of metalloids such as arsenic are usually present in acidic bioleaching environments and may result in the generation of highly oxidative conditions (Ercal et al., 2001; Valko et al., 2005; Slyemi and Bonnefoy, 2012).
Partially reduced ROSs are produced during aerobic respiration (Imlay, 2008). ROS include the superoxide anion (), the hydroxyl radical (), and the non-radical oxidant hydrogen peroxide (H2O2). In addition to its production during aerobic respiration, ROS can be generated in a number of different ways, including exposure to environmental factors such as light, oxidative chemical agents, and metals. In extremely acidic environments, it has been reported that ROS are produced spontaneously on mineral surfaces. Thus, ROS such as H2O2, , and can be formed on the surface of pyrite with kinetics directly proportional to the surface area of particles (Schoonen et al., 2010; Jones et al., 2013). ROS react with and damage all major molecular components of cells such as DNA, RNA, proteins, lipids and cofactors, thereby having a substantial impact on cell physiology (Cohn et al., 2010; Jones et al., 2013). Additionally, one study using an acidophilic microorganism showed that ROS negatively affect microbial cell growth and the rate of Fe2+ oxidation (Jones et al., 2013). These data illustrate that iron-oxidizing and most likely other acidophilic microorganisms are exposed to severe oxidizing conditions that have a negative effect for the cell, and the mechanisms for protection against oxidative stress are thus essential for adaptation and survival in these environments. Although some research has been conducted in this area (Ferrer et al., 2016b), the mechanisms involved in anti-oxidative protection in acidophilic microorganisms are not fully understood.
A meta-proteomic study carried out with the microbial community present in the biofilm of an acid mine drainage (AMD) system, where Leptospirillum group II was dominant, revealed that thioredoxins, chaperones and other proteins involved in the defense against oxidative radicals are highly represented, suggesting that they may play an important role in the protection against oxidants in members of the genus Leptospirillum (Ram et al., 2005). In addition, a transcriptomic study with material obtained from a natural site that had high concentrations of metals revealed that in L. ferrooxidans there is an increased expression of genes that code for proteins involved in DNA repair (RecG and DnaX), carotenoid biosynthesis, and of a putative regulator involved in the response to oxidative stress (Parro et al., 2007). In agreement with these data, the key role of the thioredoxin/thioredoxin reductase (Trx/TR) system in the protection against oxidative-stress was further confirmed for L. ferriphilum (Norambuena et al., 2012). Additionally, a bioinformatic analysis revealed that the genome of members of the genus Leptospirillum possess genes that encode for several peroxiredoxins, Dyp-peroxidase A, rubrerythrin A, and cytochrome c peroxidase (Cárdenas et al., 2012; Contreras et al., 2015). To the best of our knowledge, these bacteria do not carry genes coding for the ROS-scavenging enzymes superoxide dismutase (SOD), catalase (CAT), or for proteins that belong to a glutathione (GSH)-dependent system (Mi et al., 2011; Cárdenas et al., 2012).
Besides the proteins implicated in the response to oxidative-stress and repair of biomolecules, the meta-proteomic study mentioned above revealed the presence of high levels of proteins involved in cobalamin biosynthesis (Ram et al., 2005). It has been suggested that cobalamin could play a role in the adaptation of Leptospirillum to this extreme environment and for its establishment in it by increasing the competitiveness during colonization in the later stages of biofilm development (Denef et al., 2010). However, the underlying mechanisms involved in the protective role exerted by cobalamin have not been studied and are not yet understood.
Cobalamin is a cobalt-coordinated tetrapyrrole derived from uroporphyrinogen III, a precursor in the synthesis of heme, siroeheme, and chlorophylls (Raux et al., 2000). In nature, cobalamin is synthesized via a branch of the tetrapyrrole biosynthetic pathway, which involves around 30 enzymes (Martens et al., 2002), and in most bacteria the first intermediate is glutamyl-tRNA (Scott and Roessner, 2002). While many prokaryotes synthesize cobalamin either via oxygen-dependent or oxygen-independent pathways (Scott and Roessner, 2002), other organisms lack the ability to synthesize cobalamin and depend on its uptake from the environment.
In prokaryotes, cobalamin is mainly present in three classes of enzymes: adenosylcobalamin-dependent isomerases, methylcobalamin-dependent methyltransferases, and cobalamin-dependent reductive dehalogenases (Zhang et al., 2009). In addition to its function as an enzyme cofactor, studies using eukaryotic models have revealed that cobalamin also participates in anti-oxidative protection and inflammatory response (Scalabrino et al., 2008; Birch et al., 2009). Furthermore, a protective antioxidative and antiapoptotic role of cobalamin was reported for rat-liver cells exposed to arsenic, where cobalamin restored the activity of the ROS-scavenging enzymes SOD and CAT and increased the level of reduced gluthatione (GSH) (Majumdar et al., 2009, 2012; Chattopadhyay et al., 2012). Furthermore, different forms of cobalamin were shown to have a protective in vitro effect against oxidative stress increasing cell viability and reducing cellular damage of endothelial cells exposed to peroxide and superoxide (Birch et al., 2009; Moreira et al., 2011). The facts described above suggest that there is an important link between cobalamin and the response to oxidative-stress in eukaryotic cell lines and animal models. For prokaryotes, an antioxidative role of cobalamin has not been described. Nevertheless, in addition to being important for enzymatic functions, this vitamin directly regulates the transcription of the light-inducible car operon involved in carotenogenesis in the non-phototrophic bacterium Myxococcus xanthus (Ortiz-Guerrero et al., 2011). In both phototrophic and non-phototrophic organisms, carotenoids serve as protectors against photo-oxidative damage by scavenging harmful radicals, which are formed upon illumination (Ortiz-Guerrero et al., 2011). Thus, from these data an indirect role for cobalamin in the defense against oxidative radicals can be deduced in prokaryotic models. However, a direct effect of this vitamin in regulating ROS concentration and the redox status of the cell has not been demonstrated.
In the present study we investigated the protective effect of cobalamin in Leptospirillum strain CF-1 (Lo et al., 2007) in response to oxidative-stress induced by ferric ion, chromate, hydrogen peroxide, and diamide. We evaluated the impact of the exogenous addition of cobalamin on ROS content, oxidative cell damage, and on the activity of antioxidative enzymes. Furthermore, transcriptional expression of genes associated with cobalamin biosynthesis was assessed. Our data revealed the existence of a cobalamin-based mechanism to protect bacterial cells from oxidative damage, and they provide insights into the determinants involved in the tolerance to oxidative-stress in iron-oxidizing acidophilic bioleaching bacteria.
Materials and Methods
Culture Condition and Growth Measurement
Leptospirillum strain CF-1 (Lo et al., 2007) was obtained from J. Banfield. It was grown in 9K BR medium (Belnap et al., 2010). The cultures were grown aerobically at 37°C with constant stirring at 180 rpm. Bacterial growth was measured by direct microscopic counting by using a modified Neubauer chamber.
Oxidative Stress Induction
Two liter of Leptospirillum CF-1 culture was grown until late exponential phase. Cells were harvested by centrifugation at 9,000 × g for 20 min at 15°C. Then cells were resuspended in 25 mL of fresh medium and shaken for 30 min at 37°C. The cells were collected by centrifugation and washed with 10 mM H2SO4 and resuspended in 25 mL of fresh medium supplemented independently with either 260 mM Fe2(SO4)3 (Sigma–Aldrich), 1 mM H2O2 (Merck), 6 mM K2CrO4 (Merck), or 4 mM diamide [1,1′-azobis(N,N-dimethylformamide; Sigma–Aldrich)] as oxidative agent for 1 h at 37°C. Samples treated with cobalamin were pre-incubated with 5 nM of cyanocobalamin (Sigma–Aldrich) for 1 h at 37°C in darkness. Cyanocobalamin is by definition vitamin B12, and represents the form mainly manufactured by industry (Martens et al., 2002).
Determination of ROS Levels
The oxidant-sensitive probe H2DCFDA (2′,7′-dichlorodihydrofluorescein diacetate) (Davidson et al., 1996) was used to determine the intracellular level of total ROS. For ROS determination, Leptospirillum CF-1 cells were washed with 10 mM H2SO4 and incubated for 30 min in 100 mM potassium phosphate pH 7.4, containing 10 μM final concentration of H2DCFDA (from a 1 mM stock solution dissolved in dimethyl sulfoxide). After washing, the cells were suspended in the same buffer, disrupted by sonication, and centrifuged at 18,000 × g for 20 min. Aliquots of cell extracts (100 μL) were obtained and the fluorescence intensity was measured using a fluorescence reader (Synergy HT, BioTek) and an excitation at 498 nm. Emission values recorded at 522 nm were normalized to the respective protein concentration. Protein concentration was determined as described by Bradford (1976).
DNA Extraction
DNA purification was carried out according to standard procedures with some modifications. Cells were collected by centrifugation at 9,000 × g for 20 min, washed with 10 mM H2SO4, resuspended in TE buffer and treated with 2 mg/mL lysozyme (Sigma–Aldrich) for 1 h at 37°C. Chromosomal DNA extraction was carried out according to Sambrook and Russell (2001).
8-Hydroxy-2′-deoxyguanosine (8-OHdG) Content
Briefly, 10 μg of DNA were treated with 1 U of DNAse I (Invitrogen) at 37°C for 30 min. After digestion with DNase I, the DNA was denatured for 5 min at 95°C, quickly chilled on ice, and digested with 1 U of nuclease P1 (US Biologicals) for 2 h at 37°C in 20 mM sodium acetate, pH 5.2. After the incubation, the pH was adjusted to 7.5 with 1 M Tris-HCl pH 8.0, and the preparation was treated with 5 U of alkaline phosphatase (FastAP, Thermo Scientific) for 15 min at 37°C. The reaction mixture was centrifuged for 5 min at 6,000 × g and the supernatant was used for the measurement of 8-OHdG with the Oxiselect oxidative DNA damage ELISA-kit (Cell Biolabs) following the manufacturer’s instructions.
Cyclobutane Pyrimidine Dimer (CPD) Content
Cells of strain CF-1 were grown in 125 mL of medium until late exponential phase, collected by centrifugation at 9,000 × g for 20 min at 15°C, and incubated during 30 min in fresh medium. Cells were irradiated for 3 min using a UV lamp (256 nm, 8 Watts) at a distance of 30 cm at room temperature. After irradiation, cells were incubated again for 60 min in fresh medium. Then, genomic DNA was purified and CPD content was measured using the OxiSelectTM UV-Induced DNA Damage ELISA Kit (Cell Biolabs, INC) according to the manufacturer’s instructions. Absorbance was measured at 450 nm using a microplate reader (Synergy HT, BioTek).
Determination of Thiobarbituric Acid-Reactive Substances (TBARS)
Thiobarbituric acid-reactive substances in cell extracts of Leptospirillum CF-1 were determined using the Oxyselect TBARS kit (Cell Biolabs Inc.) which detects malondialdehyde (MDA), a byproduct of lipid peroxide oxidation. 2-thiobarbituric acid (TBA) forms adducts with MDA which were measured with a fluorimeter at an excitation of 540 nm and an emission of 590 nm. The concentration of MDA equivalents was determined by using an MDA standard curve.
Antioxidative Activities
Antioxidative activities were measured in whole-cell extracts prepared according to Norambuena et al. (2012) with some modifications. Bacterial extracts were prepared by ultrasonic disruption in buffer containing 30 mM Tris-HCl pH 8.0, 30 mM NaCl, 1 mM dithiothreitol, followed by centrifugation for 15 min at 30,000 × g at 4°C. As a negative control, the activities of a protein extract inactivated at 65°C for 15 min were followed.
Thioredoxin (Trx) Activity
Thioredoxin activity was assayed by reduction of disulfides of free chain insulin B by dithiothreitol and measured spectrophotometrically at 650 nm as turbidity formation from the protein precipitation according to Arnér and Holmgren (2001). The assay was carried out with minor modifications at room temperature as described by Norambuena et al. (2012).
Thioredoxin Reductase (TR)
Thioredoxin reductase activity was followed by monitoring the reduction of 5,5′-dithiobis-(2-nitrobenzoic acid) (DTNB) at 412 nm according to Lim and Lim (1995) with minor modification as previously described (Norambuena et al., 2012). The reaction was monitored every 30 s for 3 min. The negative control consisted of a protein extract inactivated by heating at 65°C for 15 min.
Cytochrome c Peroxidase (CcP)
This activity was assayed as described (Yonetani and Ray, 1966). Briefly, 50 mg of horse heart cytochrome c (Merck) were dissolved in 2 mL of 10 mM potassium phosphate pH 7.0 and 1 mM EDTA. To reduce ferricytochrome c, the reaction mixture was incubated with 10 mM sodium dithionite for 2 min. The salt excess was removed by gel filtration in Micro Bio-Spin columns (BioRad) packed with Bio-GelP6 (molecular exclusion limit of 1-6 kDa) (BioRad). Reduced cytochrome c was estimated spectrophotometrically at 550 nm. An aliquot of 10 μL was mixed with 490 μL of phosphate buffer pH 7.0 and absorbance was measured at 550 nm. The absorbance of a ferricyanide-oxidized cytochrome c was also determined. The percentage of cytochrome c reduction was estimated according to Matthis and Erman (1995) using an extinction coefficient (𝜀) of 27.7 mM-1 cm-1. To measure CcP activity, the reaction mixture (500 μL) contained 10 mM potassium phosphate pH 7.0, 25 mM ferrocytochrome c, and 50 μg protein extract. The reaction was started by adding 200 mM H2O2. The enzyme assay was performed by measuring the oxidation rate of ferrocytochrome c every 10 s for 3 min.
Superoxide Dismutase
This activity was measured as described by Oberley and Spitz (1984). Xanthine–xanthine oxidase was utilized to generate a superoxide flux. Reduction of nitro blue tetrazolium (NBT) by to blue formazan was followed at 560 nm, every 30 s for 3.5 min, at room temperature. The rate of NBT reduction in the absence of the extract was used as the reference rate. When increasing amounts of protein (with SOD activity) were added, the rate of NBT reduction was progressively inhibited. The degree of inhibition was expressed as a percentage of the reference rate of NBT reduction when SOD activity was not present. The data were plotted as percentage inhibition versus protein concentration. One unit of activity was defined as that amount of protein necessary to decrease the reference rate to 50% of maximum inhibition. To chelate redox cycling metal ions able to interfere with the reaction, the assay mixture also contained diethylenetriaminepentaacetic acid (DETAPAC). Each l-mL assay tube contained the final concentration of the following reagents: 50 mM potassium phosphate buffer pH 7.8, 1 mM DETAPAC, 60 μM NBT, 0.1 mM xanthine, enough xanthine oxidase to achieve the required reference rate, and 50 μg of protein extract of Leptospirillum CF-1. All data were expressed in units of SOD activity per milligram of protein.
Protein-Free Extract Preparation
Protein-free extract was obtained by filtering the total cell extract through Centricon tubes with a 3 kDa cutoff (Millipore) by centrifugation at 8,500 × g for 15 min at 4°C.
Relative Levels of RNA
RNA Isolation and cDNA Synthesis
RNA was isolated using the TRIsureTM reagent (Bioline). DNA was removed by DNase I treatment (Thermo Scientific) according to the manufacturer’s instructions. cDNA synthesis was carried out in 20-μL reaction mixtures containing 1 μg of RNA, 10 pmole of specific primers (Table 1), and M-MuLV reverse transcriptase (Thermo Scientific) following the instructions of the provider. cDNA was stored at -80°C until use.
qPCR Reaction
Primers for all reverse transcription and normal qPCR reactions (Table 1) were designed using the available gene sequences of Leptospirillum CF-1 (Ferrer et al., 2016a). Then, KAPA SYBR FAST qPCR kits (Kapabiosystems) was used for qPCR amplification according to manufacturer’s instructions. The qPCR conditions were an initial denaturation at 95°C for 5 min, followed by 40 cycles of denaturation (95°C for 30 s), annealing (58°C for 20 s) and extension (72°C for 10 s). All these reactions were performed in a StepOne Real-Time PCR system (Applied Biosystems). The relative abundance of each gene versus a constitutively expressed gene (16S rDNA) was determined. The results were expressed as means of two independent experiments.
Statistical Analysis
Statistical analysis was performed using the one-way ANOVA test followed by Turkey’s test in GraphPad Prism 5. The differences were considered to be significant at P < 0.05.
Results
Cobalamin Attenuates Oxidation-Induced ROS Generation
Ferric iron, hydrogen peroxide, and chromate have previously been shown to increase the content of intracellular ROS and to reduce the growth and cell viability of Leptospirillum ferriphilum (Cortés et al., 2011). We tested whether cobalamin has a suppressive effect on total ROS generation in Leptospirillum strain CF-1 treated with oxidative agents. As shown in Figure 1, cells exposed to 260 mM Fe2(SO4)3, 1 mM H2O2, or 6 mM K2CrO4 for 60 min showed significantly increased total ROS content (to 176, 172, 282%, respectively) as compared to control cells (100%). Interestingly, a significant reduction of total ROS generation was observed in cells pre-treated for 1 h with 5 nM cyanocobalamin. In cells exposed to ferric iron and hydrogen peroxide, ROS content decreased to levels similar to those detected under control conditions. It should be noted that the concentration of cobalamin required to exert a significant physiological effect was three orders of magnitude lower than those reported to be needed to achieve a similar effect in a eukaryotic cell line model (Birch et al., 2009). These results supported a role of cobalamin as an antioxidant in Leptospirillum CF-1 grown under oxidative stress.
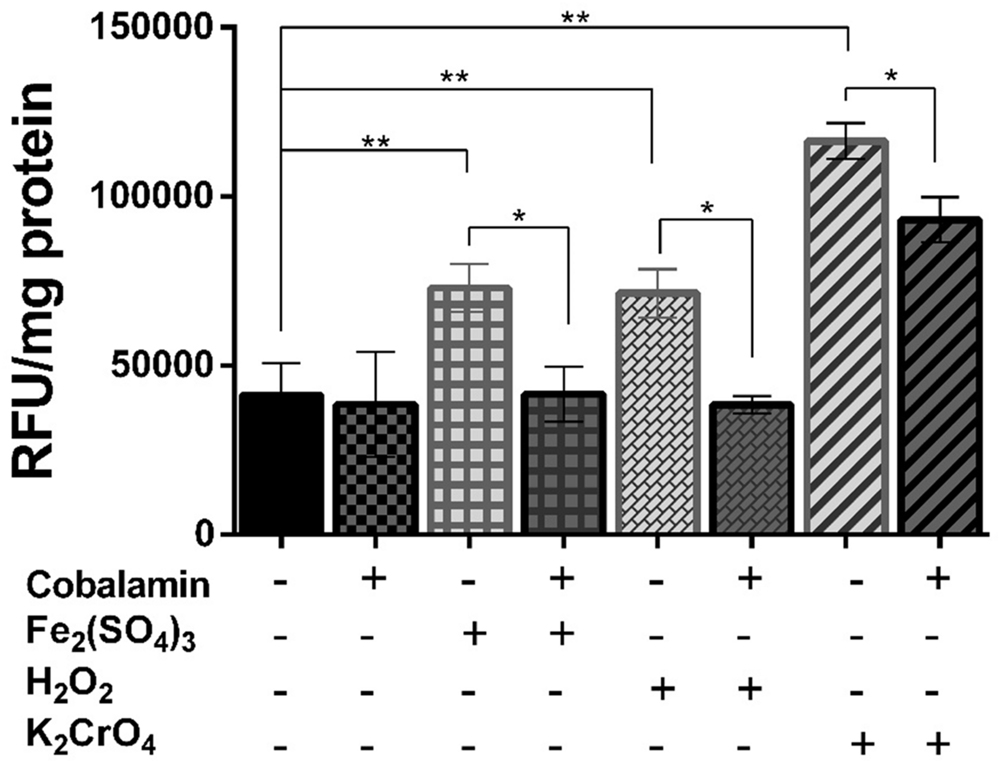
FIGURE 1. Effect of cobalamin on ROS generation. Cytoplasmic ROS content was estimated by measuring activation of the fluorescent-probe H2DCFDA in Leptospirillum CF-1 cells treated with oxidative agents as indicated under Section “Materials and Methods.” Fluorescence values were expressed as relative fluorescence units (RFU). Bars represent the average of three independent experiments ± standard deviations. ∗P < 0.05, ∗∗P < 0.01.
Consistent with these results, the decrease in the levels of intracellular ROS correlated with an increase in the growth of cells pre-incubated with 5 nM cyanocobalamin. For example, after 100 h of incubation, exposure to 260 mM Fe2(SO4)3 reduced growth to 28% of control cells, while in cells pre-treated with cobalamin and exposed to the same ROS-elicitor the recovery of cell densities were close (74%) to those of untreated cells. In addition, exposure of strain CF-1 to ferric iron for 60 min lead to a significant reduction in cell viability (45%) compared to non-stressed control cells (100%), while pre-treatment of cells with cobalamin before induction of oxidative stress limited the decrease in cell viability to 70% of the value for the control cells (data not shown).
Cobalamin Protects Lipids, but Not DNA against Oxidative Damage
To determine whether cobalamin plays a role in the protection of biomolecules, we evaluated oxidative damage to lipids and DNA in strain CF-1 exposed to oxidative-stress elicitors. As shown in Figure 2, exposure of cells to all of the different oxidative-stress elicitors led to a significant increase in the levels of MDA, as compared to control cells (100%). However, when cells were pre-treated with 5 nM cyanocobalamin for 60 min, the amount of MDA decreased significantly to levels similar or slightly lower (chromate and iron, respectively) than those observed under control conditions. These results are consistent with the ability of cobalamin to reduce the content of intracellular ROS (see above). However, the levels of 8-hydroxydeoxyguanosine, a signature of oxidized DNA, were not significantly different between cells treated with ferric iron in the presence or absence of cobalamin (∼125% of untreated cells, data not shown). Thus, it seems that the protective effect of cobalamin is not a general scavenging system for any ROS that is generated. Similarly, we did not detect a significant effect on the content of cyclobutane pyrimidine dimer (CPD) upon exposure to UV light, showing that cobalamin did not exert direct protection against oxidation of DNA.
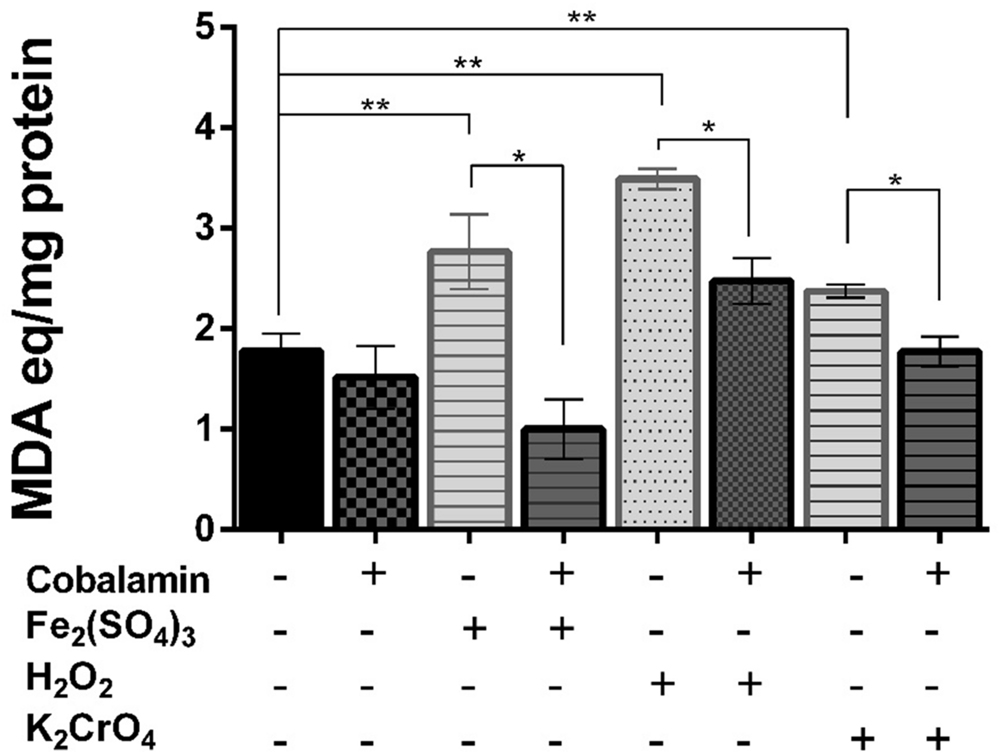
FIGURE 2. Effect of cobalamin on lipid peroxidation. Lipid peroxidation products, expressed as malondialdehyde (MDA) equivalents, were determined as thiobarbituric acid-reactive substances present in crude extracts in Leptospirillum CF-1 cells as indicated under Section “Materials and Methods.” Bars represent the average of three independent experiments ± standard deviations. ∗P < 0.05, ∗∗P < 0.01.
Cobalamin Increases the Activity of Protective Antioxidant Proteins
As previously described, Leptospirillum group II possesses a thioredoxin-based thiol/disulfide system (Ram et al., 2005; Norambuena et al., 2012). In addition, inspecting the genome sequence of Leptospirillum CF-1 allowed us to identify genes encoding for several peroxidases (cytochrome c peroxidase, rubrerythrin A, and Dyp peroxidase). In order to evaluate whether cobalamin exerts a role in the activation of anti-oxidative enzymes in Leptospirillum CF-1, we measured the activity of cytochrome c peroxidase (CcP) and of the thioredoxin/thioredoxin reductase (Trx/TR) system. Since superoxide scavenging enzymes are considered ubiquitous in aerobic organisms, we also tested cells for this enzymatic activity, although no canonical SOD gene was identified in the genome of strain CF-1.
Thioredoxin System
As is shown in Figure 3A, exposure of strain CF-1 to the disulfide-stress elicitor diamide led to an increase in thioredoxin activity, as compared to control cells. Interestingly, pre-treatment with 5 nM cyanocobalamin for 60 min led to a significant increase in thioredoxin activity in cells that were exposed to diamide (184%), but not in cells grown under the control condition. A similar trend was also observed when cells were exposed to ferric iron as an elicitor of oxidative-stress (data not shown).
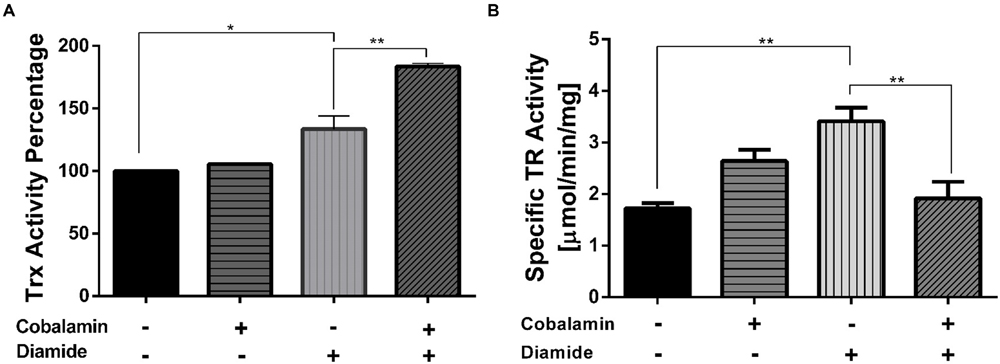
FIGURE 3. Effect of cobalamin on thioredoxin and thioredoxin reductase activity. To measure Trx activity (A), the reduction of the alpha-chain of insulin was monitored at 650 nm as described under Section “Materials and Methods.” TR activity (B) was monitored by following the reduction of 5,5′-dithiobis-(2-nitrobenzoic acid) (DTNB) at 412 nm. The activity in the control reaction corresponds to 100%. Data represent the average of two independent experiments (lines on top of bars indicate value ranges). ∗P < 0.05, ∗∗P < 0.01.
To evaluate whether the increase in Trx activity involved a coordinated increase in thioredoxin reductase activity (TR), TR activity was measured using the DTNB-reduction method in whole cellular extracts. Exogenous addition of cobalamin resulted in decreased TR activity in cells exposed to diamide for 30 min (Figure 3B). Thus, changes in TR activity could not directly explain the increase in Trx activity described above. Consequently, the marked effect on Trx activity upon treatment of cells with diamide seems to be the result of a different mechanism.
Cytochrome c Peroxidase
Cytochrome c peroxidase activity was measured in whole-cell extracts following the oxidation of ferrocytochrome c (Figure 4). In agreement with the bioinformatic analysis mentioned above, CcP activity was in fact detected in whole-cell extracts of this strain. In addition, CcP activity was significantly increased when cells were exposed to 1 mM hydrogen peroxide for 30 min (288%), as compared to control cells (100%). Pre-treatment of cells with 5 nM cyanocobalamin further increased CcP activity in cells exposed to hydrogen peroxide (394%). It is important to note that the activating effect of cobalamin was only observed in cells that had been exposed to elicitors of oxidative stress and not in cells treated solely with cobalamin. A similar trend was observed in Fe2(SO4)3-stressed cells (data not shown).
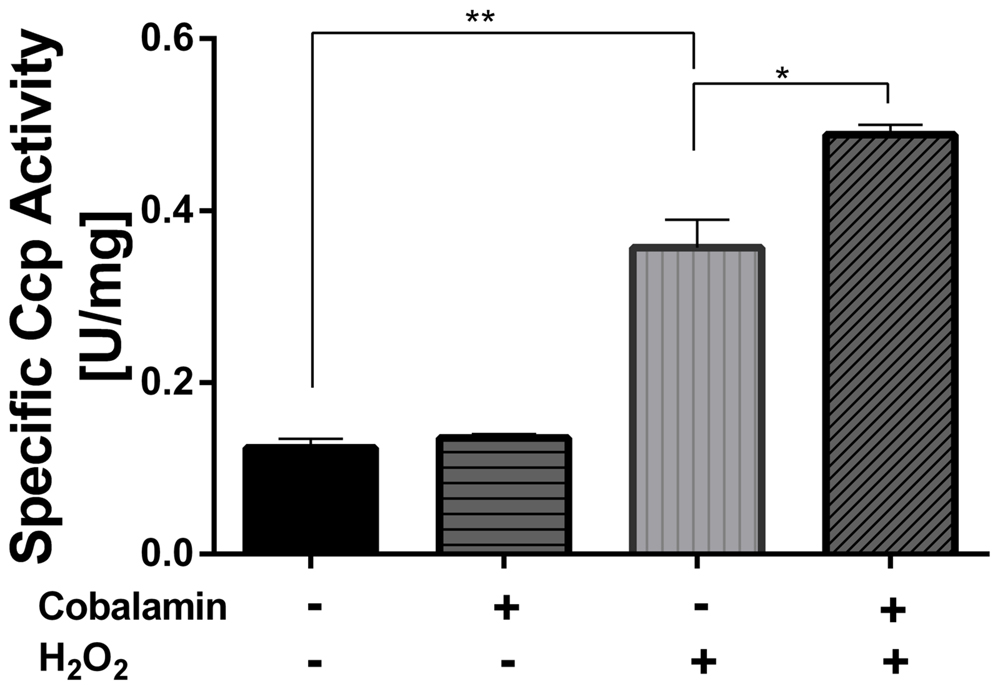
FIGURE 4. Effect of cobalamin on CcP activity. The activity was followed spectrophotometrically at 550 nm as indicated under Section “Materials and Methods.” Data represent the average of two independent experiments (lines on top of bars indicate value ranges). One unit (U) is defined as the amount of enzyme required to oxidize 1 μmol of ferrocytochrome c per min. ∗P < 0.05, ∗∗P < 0.01.
Superoxide Dismutase-Like Activity
The activity was evaluated using a xanthine oxidase-based superoxide generating system. After 30 min of exposure to 6 mM K2CrO4, SOD activity did not exhibit a significant change. In contrast, SOD activity increased dramatically after a 60 min exposure to the oxidative-stress elicitor (413%), as compared to the control (100%) (Figure 5). Like the other antioxidative activities that were analyzed, pre-treatment with cobalamin further increased SOD activity up to a 552% (P < 0.05). However, unlike Trx and CcP activities, SOD activity increased considerably in cells pre-incubated with cobalamin in both the presence or absence of oxidative stress elicitors. In addition, consistent with what was observed for CcP activity (see above), a similar activating effect of cyanocobalamin on SOD activity was observed in cells stressed with 260 mM Fe2(SO4)3 (data not shown).
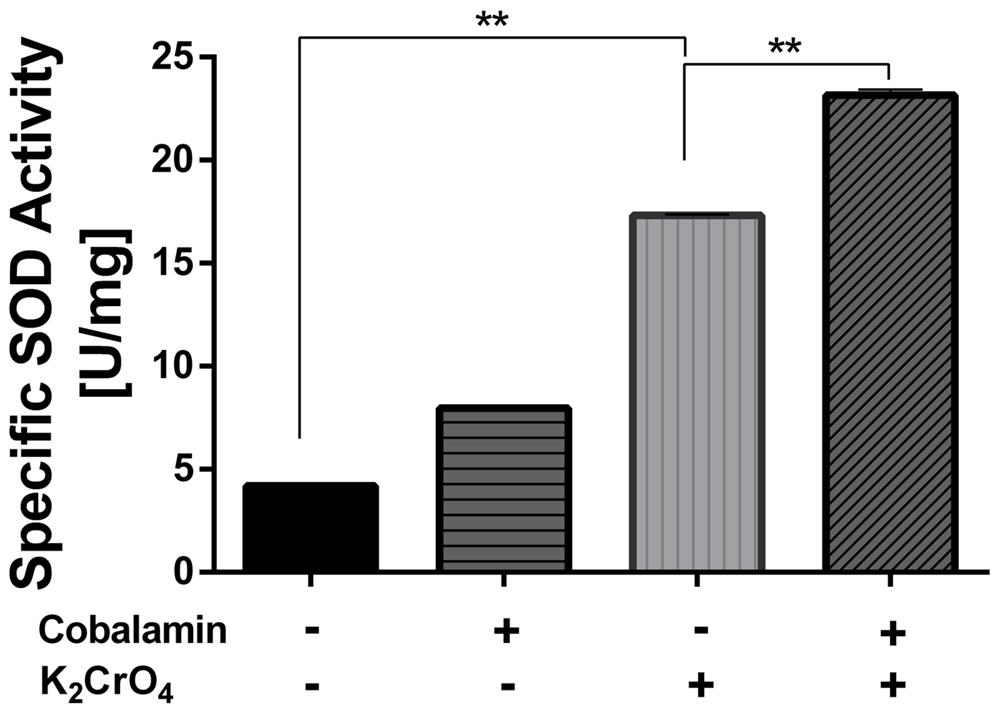
FIGURE 5. Effect of cobalamin on superoxide dismutase activity. The activity was measured by following the inhibition of NBT-reduction at 560 nm as described under Section “Materials and Methods.” Data represent the average of two independent experiments (lines on top of bars indicate value ranges). One unit (U) is defined as the amount of enzyme necessary to decrease the reference rate to 50% of maximum inhibition. ∗∗P < 0.01.
It is surprising that although superoxide-anion scavenger activity was detected, no genes encoding for SOD enzymes were found in the genome sequence of strain CF-1. This fact suggests the existence of an alternative repertoire of genes encoding either non-canonical catalysts of dismutation, or of enzymes involved in the biosynthesis of non-catalytic scavengers (Miriyala et al., 2012). Thus, we were interested in determining if the superoxide-anion radical-scavenger activity was present in the protein-enriched or protein–free fractions of cells exposed to oxidative conditions. In order to obtain these fractions, the extract derived from ferric iron-stressed cells was passed through a 3 kDa cut-off spin filter. The results (Figure 1, Supplementary Material) showed that the superoxide-anion scavenger activity is present in the protein-free fraction obtained after filtering the whole extract. Supporting this result, the activity was detected in whole-cell extracts after both protease and heat treatment (data not shown), strongly suggesting that the SOD-mimetic activity of strain CF-1 is determined by a non-proteinaceous metabolite.
Effect of Oxidative Stress on the Endogenous Cobalamin-Biosynthesis Pathway
Since cobalamin exerted a protective effect on cells exposed to oxidative-stress elicitors, it raised the question as to whether there is an increase in the activity of the cobalamin-biosynthetic pathway when Leptospirillum CF-1 is exposed to oxidative conditions. The inspection of the genome of strain CF-1 revealed the existence of 19 genes involved in the de novo non-oxygen-requiring biosynthetic route of cobalamin from 5-aminolevulinic acid (Supplementary Figure S2). In order to evaluate the activity of the biosynthetic pathway upon exposure to oxidative compounds, we determined the mRNA level of key genes associated with different parts of the pathway. Total RNA was isolated from strain CF-1 exposed to 260 mM Fe2(SO4)3 and the mRNA level of genes encoding for CobA, CbiG, CbiA, and CobU, as well as of one housekeeping gene (rrsB) was quantified by qRT-PCR. It should be noted that no significant changes were observed in the levels of the rrsB mRNA from strain CF-1 under any of the experimental conditions that were assayed (data not shown). The real-time PCR data showed that the four genes were, in fact, transcribed in strain CF-1 exposed to ferric iron. The genes cobA, cbiG, and cobU did not show significant changes in their transcript level between treated and untreated cells. However, the cbiA gene encoding for cobyrinic acid a,c-diamide synthase was significantly up-regulated in response to ferric iron-induced stress after a 30 min exposure (Figure 6). This result is in agreement with previous reports showing that the cbiA gene is upregulated in Escherichia coli, Salmonella Typhimurium, and Dehalococcoides mccartyi in response to short-term cobalamin deprivation (Rodionov et al., 2003; Men et al., 2014). Therefore, it can be presumed that in cells cultured under oxidative stress, the cobalamin biosynthetic route is activated as a strategy to contribute to alleviating the stress conditions.
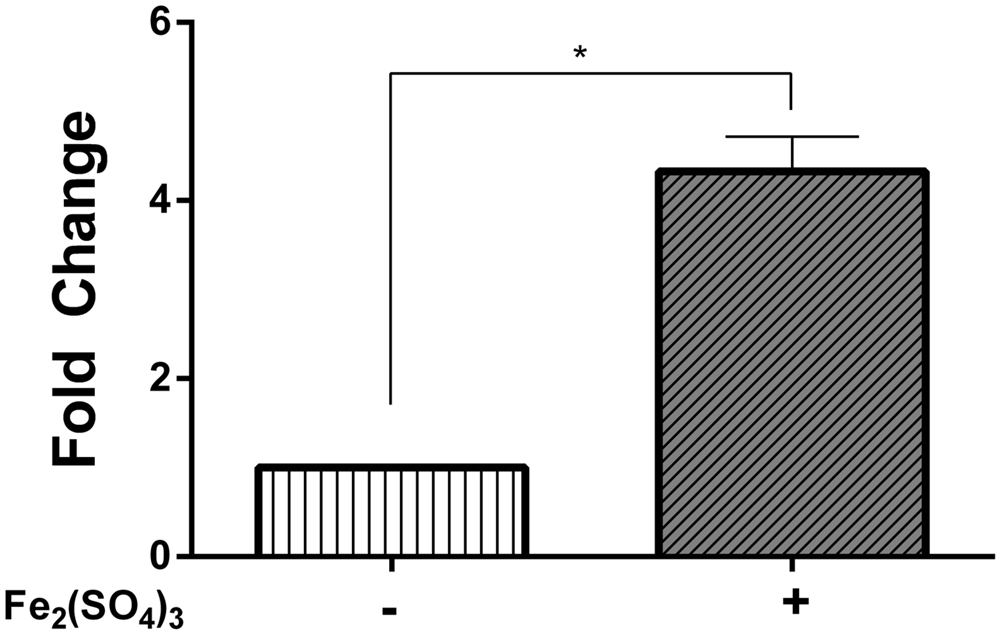
FIGURE 6. Relative mRNA levels of the cbiA gene in Leptospirillum CF-1. Bacteria were treated with 260 Fe2(SO4)3 for 30 min. Data were normalized by the 16S rRNA. Data represent the average of two independent experiments (bar indicates the value range). ∗P < 0.05.
Discussion
Acidic bioleaching environments are considered as highly oxidative where microorganisms are exposed to elevated concentrations of ROS (Cárdenas et al., 2012; Ferrer et al., 2016b). Thus, oxidative stress represents one of the greatest selective pressures imposed on acidophilic microorganisms. Members of the genus Leptospirillum grow in these extreme environments, however, they lack the main canonical enzymatic activities involved in antioxidant responses, namely SOD, CAT, and the glutathione system (Cárdenas et al., 2012), suggesting that alternative molecular mechanisms to maintain redox homeostasis might be present in these extremophilic microorganisms. In this study we showed that externally added cobalamin is able to mitigate oxidative stress in Leptospirillum CF-1, as judged by its effect on modulating the concentration of intracellular ROS and lipid damage of cells exposed to various oxidative-stress elicitors. In addition, cells exposed to cobalamin exhibited significantly enhanced cell density and viability under oxidative conditions. In agreement with these facts, the mRNA level of a gene associated with the de novo biosynthesis of cobalamin showed a significant increase after exposure to oxidative conditions, suggesting the up-regulation of this metabolic pathway. Therefore, we propose that cobalamin has a role in preventing oxidative-stress that could be relevant under the extreme conditions encountered in acidic environments.
Previously, it has been reported that reduced forms of cobalamin can directly dismutate the superoxide ion at rates approaching those exhibited by SOD enzymes (Suarez-Moreira et al., 2009). This finding is particularly significant in view of the observation that SOD-like activity could be detected in protein-free fractions of strain CF-1. For other organisms, a number of metabolites such as thiamine, pyrroloquinoline-quinone, or Mn/Fe-porphyrin derivatives with potent scavenger activity have been reported (Jung and Kim, 2003; Misra et al., 2004; Miriyala et al., 2012), and many of them are also potentially efficient scavengers of other reactive species such as peroxynitrite, the peroxyl radical, or H2O2 (Batinić-Haberle et al., 2010). It is tempting to speculate that in CF-1 cells, cobalamin may efficiently replace the SOD enzyme and play a major role in the detoxification of , and perhaps, of other reactive species. Based on our findings, this effect would be achieved at low concentrations compared to other molecules that have been reported as having a non-catalytic antioxidative activity, such as glutathione (Imlay, 2008). Thus, the potential cobalamin-based mechanism to scavenge could require the occurrence of molecular component(s) highly efficient in the maintenance of the redox status of the vitamin, allowing that its scavenging activity proceeds at high rates. However, at this point, the existence of other low molecular-weight compounds with SOD-mimetic activity in leptospirilli cannot be ruled out.
Cobalamin is known to be involved in the metabolism of methionine, a well-known antioxidant, by being a cofactor of methionine synthase (Levine et al., 2000; Halsted, 2013). The genome analysis of strain CF-1 revealed the presence of a gene that probably encodes a cobalamin-dependent methionine synthase (Ferrer et al., 2016a), suggesting that in this microorganism cobalamin could have an antioxidant activity through restoring methionine metabolism. Moreover, methionine is a precursor for S-adenosylmethionine which also has antioxidative properties as it is able to chelate Fe2+, thereby significantly preventing the occurrence of the Fenton reaction (Caro and Cederbaum, 2004). Thus, the mode of action of cobalamin in Leptospirillum CF-1 may proceed through different mechanisms that involve both a direct role as a ROS-scavenger system and indirect roles by participating in methionine metabolism.
In this work we observed an effect of cobalamin on restoring and even increasing the activity of antioxidative proteins such as CcP and thioredoxins. In agreement with these results, a similar effect was observed in rats, where pre-incubation with cobalamin restored the activity of SOD and CAT which were significantly inhibited in individuals treated with arsenic. Similarly, supplementing cobalamin to these rats could significantly restore the level of hepatic mitochondrial GSH as compared with an arsenic-treated group (Majumdar et al., 2012). Along the same line, it has been reported that lymphocytes from patients with cobalamin deficiency show a decrease in the pool of total and reduced glutathione (Pastore et al., 2012).
In prokaryotes, besides its primary role as an enzyme cofactor, cobalamin is also involved in the transcriptional regulation of genes related to carotenoid biosynthesis. In this process, gene regulation is based on the binding of cobalamin to the CarH repressor in a light-dependent manner (Ortiz-Guerrero et al., 2011). Interestingly, a role for cobalamin in the regulation of carotenogenesis has also been suggested in Streptomyces coelicolor (Takano et al., 2006) and in extremophilic bacteria of the genera Deinococcus and Thermus (Tian and Hua, 2010). Also, this metabolite may directly regulate gene expression via a riboswitch (Henkin, 2008). Some examples of genes regulated via a cobalamin-riboswitch include the genes for methionine synthase in Bacillus clausii and Mycobacterium tuberculosis, and genes of the btu operon in Escherichia coli, which are involved in cobalamin uptake. These data support the idea that regulation of antioxidative enzymes by cobalamin in strain CF-1 may occur at the level of mRNA abundance of the corresponding genes. Thus, further studies addressed to identify cobalamin-induced changes of the transcriptome and proteome of strain CF-1 would undoubtedly help us to better understand how cobalamin stimulates the activity of the antioxidative proteins.
Noticeably, the antioxidative role of cobalamin that was detected correlates with increased transcript levels of the cbiA gene after exposure to oxidative-stress elicitors. Therefore, we speculate that the redox status of cells may be involved in regulating the activity of the cobalamin-biosynthetic pathway. Whether this leads to an increased concentration of intracellular cobalamin should be addressed. On the other hand, it will also be interesting to address if special modifications of the axial ligand of cobalamin (Hannibal et al., 2008, 2013; Yan et al., 2015) are relevant and are determinants of the antioxidative properties that have been described for this vitamin.
Finally, it is a well-known fact that cobalamin biosynthesis is confined to Archaea and some Bacteria. Since it is a highly complex and energy-consuming process with about 30 enzymatic steps (Martens et al., 2002; Scott and Roessner, 2002), questions arise about the driving forces that contributed to select a cobalamin-based mechanism to restore the redox balance within the cell and to protect cells against oxidative damage. The evidence presented herein places cobalamin as part of the cellular oxidative-stress defense scheme of Leptospirillum CF-1, and likely of other members of this genus. In light of the facts discussed above, it is conceivable to postulate that the multi-target effect of cobalamin may contribute to maintain the redox balance under highly oxidizing conditions, leading to a global activation of cellular components that participate in the response to oxidative-stress. Thus, cobalamin might provide a specific advantage in extremely acidic and highly metal-loaded environments by increasing the tolerance and fitness of these microorganisms. Whether the cobalamin-based system is confined to leptospirilli or is a niche-specific adaptation of acidophilic microorganisms is still an open question.
Author Contributions
GL and OO: conceived and designed the experiments. AF, JR, CZ, JN, AS: performed the experiments. AF and RC: analyzed the data. GL, AF, and OO: wrote the paper.
Funding
This work was supported by Fondecyt Grant (1120746,) from the government of Chile, and Dicyt-Usach to GL. AF was recipient of a Doctoral Fellowship from CONICY, Chile.
Conflict of Interest Statement
The authors declare that the research was conducted in the absence of any commercial or financial relationships that could be construed as a potential conflict of interest.
Acknowledgments
We gratefully acknowledge Dr. Jill Banfield (University of California Berkeley, Berkeley, CA 94720, USA) for providing Leptospirillum strain CF-1 and Dr. Michael Schlömann. TUBAF, Freiberg, Germany, for comments on the manuscript.
Supplementary Material
The Supplementary Material for this article can be found online at: http://journal.frontiersin.org/article/10.3389/fmicb.2016.00748
References
Arnér, E., and Holmgren, A. (2001). Measurement of thioredoxin and thioredoxin reductase. Curr. Protoc. Toxicol. Chap. 7:Unit 7.4. doi: 10.1002/0471140856.tx0704s05
Batinić-Haberle, I., Rebouças, J. S., and Spasojević, I. (2010). Superoxide dismutase mimics: chemistry, pharmacology, and therapeutic potential. Antioxid. Redox Signal. 13, 877–918. doi: 10.1089/ars.2009.2876
Belnap, C. P., Pan, C., VerBerkmoes, N. C., Power, M. E., Samatova, N. F., Carver, R. L., et al. (2010). Cultivation and quantitative proteomic analyses of acidophilic microbial communities. ISME J. 4, 520–530. doi: 10.1038/ismej.2009.139
Birch, C. S., Brasch, N. E., McCaddon, A., and Williams, J. H. (2009). A novel role for vitamin B12: cobalamins are intracellular antioxidants in vitro. Free Radic. Biol. Med. 47, 184–188. doi: 10.1016/j.freeradbiomed.2009.04.023
Bonnefoy, V., and Holmes, D. S. (2012). Genomic insights into microbial iron oxidation and iron uptake strategies in extremely acidic environments. Environ. Microbiol. 14, 1597–1611. doi: 10.1111/j.1462-2920.2011.02626.x
Bradford, M. M. (1976). A rapid and sensitive method for the quantitation of microgram quantities of protein utilizing the principle of protein-dye binding. Anal. Biochem. 72, 248–254. doi: 10.1016/0003-2697(76)90527-3
Cárdenas, J. P., Moya, F., Covarrubias, P., Shmaryahu, A., Levicán, G., Holmes, D. S., et al. (2012). Comparative genomics of the oxidative stress response in bioleaching microorganisms. Hydrometallurgy 12, 162–167. doi: 10.1016/j.hydromet.2012.07.014
Caro, A. A., and Cederbaum, A. I. (2004). Antioxidant properties of S-adenosyl-L-methionine in Fe(2+)-initiated oxidations. Free Radic. Biol. Med. 36, 1303–1316. doi: 10.1016/j.freeradbiomed.2004.02.015
Carpenter, C., and Payne, S. M. (2014). Regulation of iron transport systems in Enterobacteriaceae in response to oxygen and iron availability. J. Inorg. Biochem. 133, 110–117. doi: 10.1016/j.jinorgbio.2014.01.007
Chattopadhyay, S., Deb, B., and Maiti, S. (2012). Hepatoprotective role of vitamin B12 and folic acid in arsenic intoxicated rats. Drug Chem. Toxicol. 35, 81–88. doi: 10.3109/01480545.2011.589439
Cohn, C. A., Fisher, S. C., Brownawell, B. J., and Schoonen, M. A. (2010). Adenine oxidation by pyrite-generated hydroxyl radicals. Geochem. Trans. 11, 2. doi: 10.1186/1467-4866-11-2
Contreras, M., Mascayano, M., Chávez, R., Ferrer, A., Paillavil, B., and Levicán, G. (2015). Dyp-type peroxidase (DypA) from the bioleaching acidophilic bacterium Leptospirillum ferriphilum DSM 14647. Adv. Mater. Res. 1130, 23–27. doi: 10.4028/AMR.1130.23
Cortés, A., Flores, R., Norambuena, J., Cárdenas, J. P., Quatrini, R., Chávez, R., et al. (2011). “Comparative study of redox stress response in the acidophilic bacteria Leptospirillum ferriphilum and Acidithiobacillus ferrooxidans,” in Proceedings of the 19th International Biohydrometallurgy Symposium, eds G. Qiu, T. Jiang, W. Qin, X. Liu, Y. Yang, and H. Wang (Changsha: Central South University Press), 354–357.
Davidson, J. F., Whyte, B., Bissinger, P. H., and Schiestl, R. H. (1996). Oxidative stress is involved in heat-induced cell death in Saccharomyces cerevisiae. Proc. Natl. Acad. Sci. U.S.A. 93, 5116–5121. doi: 10.1073/pnas.93.10.5116
Denef, V. J., Kalnejais, L. H., Mueller, R. S., Wilmes, P., Baker, B. J., Thomas, B. C., et al. (2010). Proteogenomic basis for ecological divergence of closely related bacteria in natural acidophilic microbial communities. Proc. Natl. Acad. Sci. U.S.A. 107, 2383–2390. doi: 10.1073/pnas.0907041107
Ercal, N., Gurer-Orhan, H., and Aykin-Burns, N. (2001). Toxic metals and oxidative stress part I: mechanisms involved in metal-induced oxidative damage. Curr. Top. Med. Chem. 1, 529–539. doi: 10.2174/1568026013394831
Ferrer, A., Bunk, B., Spröer, C., Biedendieck, R., Valdés, N., Jahn, M., et al. (2016a). Complete genome sequence of the bioleaching bacterium Leptospirillum sp. group II strain CF-1. J. Biotechnol. 222, 21–22. doi: 10.1016/j.jbiotec.2016.02.008
Ferrer, A., Orellana, O., and Levicán, G. (2016b). “Oxidative stress and metal tolerance,” in Acidophiles: Life in Extremely Acidic Environments, eds D. B. Jhonson and R. Quatrini (Norfolk: Horizon Scientific Press), 63–76.
Halsted, C. H. (2013). B-Vitamin dependent methionine metabolism and alcoholic liver disease. Clin. Chem. Lab. Med. 51, 457–465. doi: 10.1515/cclm-2012-0308
Hannibal, L., Axhemi, A., Glushchenko, A. V., Moreira, E. S., Brasch, N. E., and Jacobsen, D. W. (2008). Accurate assessment and identification of naturally occurring cellular cobalamins. Clin. Chem. Lab. Med. 46, 1739–1746. doi: 10.1515/CCLM.2008.356
Hannibal, L., DiBello, P. M., and Jacobsen, D. W. (2013). Proteomics of vitamin B12 processing. Clin. Chem. Lab. Med. 51, 477–488. doi: 10.1515/cclm-2012-0568
Henkin, T. M. (2008). Riboswitch RNAs: using RNA to sense cellular metabolism. Genes Dev. 22, 3383–3390. doi: 10.1101/gad.1747308
Imlay, J. A. (2008). Cellular defenses against superoxide and hydrogen peroxide. Annu. Rev. Biochem. 77, 755–776. doi: 10.1146/annurev.biochem.77.061606.161055
Johnson, D. B., Kanao, T., and Hedrich, S. (2012). Redox transformations of iron at extremely low pH: fundamental and applied aspects. Front. Microbiol. 3:96. doi: 10.3389/fmicb.2012.00096
Jones, G. C., Van Hille, R. P., and Harrison, S. T. L. (2013). Reactive oxygen species generated in the presence of fine pyrite particles and its implication in thermophilic mineral bioleaching. Appl. Microbiol. Biotechnol. 97, 2735–2742. doi: 10.1007/s00253-012-4116-y
Jung, I. L., and Kim, I. G. (2003). Thiamine protects against paraquat-induced damage: scavenging activity of reactive oxygen species. Environ. Toxicol. Pharmacol. 15, 19–26. doi: 10.1016/j.etap.2003.08.001
Levine, R. L., Moskovitz, J., and Stadtman, E. R. (2000). Oxidation of methionine in proteins: roles in antioxidant defense and cellular regulation. IUBMB Life 50, 301–307. doi: 10.1080/713803735
Lim, H., and Lim, J. L. (1995). Direct reduction of DTNB by E. coli thioredoxin reductase. J. Biochem. Mol. Biol. 28, 17–20.
Lo, I., Denef, V. J., Verberkmoes, N. C., Shah, M. B., Goltsman, D., DiBartolo, G., et al. (2007). Strain-resolved community proteomics reveals recombining genomes of acidophilic bacteria. Nature 446, 537–541. doi: 10.1038/nature05624
Majumdar, S., Maiti, A., Karmakar, S., Das, A. S., Mukherjee, S., Das, D., et al. (2012). Antiapoptotic efficacy of folic acid and vitamin B12 against arsenic-induced toxicity. Environ. Toxicol. 27, 351–363. doi: 10.1002/tox.20648
Majumdar, S., Mukherjee, S., Maiti, A., Karmakar, S., Das, A. S., Mukherjee, M., et al. (2009). Folic acid or combination of folic acid and vitamin B12 prevents short-term arsenic trioxide-induced systemic and mitochondrial dysfunction and DNA damage. Environ. Toxicol. 24, 377–387. doi: 10.1002/tox.20442
Martens, J. H., Barg, H., Warren, M. J., and Jahn, D. (2002). Microbial production of vitamin B12. Appl. Microbiol. Biotechnol. 58, 275–285. doi: 10.1007/s00253-001-0902-7
Matthis, A. L., and Erman, J. E. (1995). Cytochrome c peroxidase-catalyzed oxidation of yeast iso-1 ferrocytochrome c by hydrogen peroxide. Ionic strength dependence of the steady-state parameters. Biochemistry 34, 9985–9990. doi: 10.1021/bi00031a022
Men, Y., Seth, E. C., Yi, S., Allen, R. H., Taga, M. E., and Alvarez-Cohen, L. (2014). Sustainable growth of Dehalococcoides mccartyi 195 by corrinoid salvaging and remodeling in defined lactate-fermenting consortia. Appl. Environ. Microbiol. 80, 2133–2141. doi: 10.1128/AEM.03477-13
Mi, S., Song, J., Lin, J., Che, Y., Zheng, H., and Lin, J. (2011). Complete genome of Leptospirillum ferriphilum ML-04 provides insight into its physiology and environmental adaptation. J. Microbiol. 49, 890–901. doi: 10.1007/s12275-011-1099-9
Miriyala, S., Spasojevic, I., Tovmasyan, A., Salvemini, D., Vujaskovic, Z., St Clair, D., et al. (2012). Manganese superoxide dismutase, MnSOD and its mimics. Biochim. Biophys. Acta 1822, 794–814. doi: 10.1016/j.bbadis.2011.12.002
Misra, H. S., Khairnar, N. P., Barik, A., Indira Priyadarsini, K., Mohan, H., and Apte, S. K. (2004). Pyrroloquinoline-quinone: a reactive oxygen species scavenger in bacteria. FEBS Lett. 578, 26–30. doi: 10.1016/j.febslet.2004.10.061
Moreira, E. S., Brasch, N. E., and Yu, J. (2011). Vitamin B12 protects against superoxide-induced cell injury in human aortic endothelial cells. Free Radic. Biol. Med. 51, 876–883. doi: 10.1016/j.freeradbiomed.2011.05.034
Norambuena, J., Flores, R., Cárdenas, J. P., Quatrini, R., Chávez, R., and Levicán, G. (2012). Thiol/disulfide system plays a crucial role in redox protection in the acidophilic iron-oxidizing bacterium Leptospirillum ferriphilum. PLoS ONE 7:e44576. doi: 10.1371/journal.pone.0044576
Oberley, L. W., and Spitz, D. R. (1984). Assay of superoxide dismutase activity in tumor tissue. Methods Enzymol. 105, 457–464. doi: 10.1016/S0076-6879(84)05064-3
Ortiz-Guerrero, J. M., Polanco, M. C., Murillo, F. J., Padmanabhan, S., and Elias-Arnanz, M. (2011). Light-dependent gene regulation by a coenzyme B12-based photoreceptor. Proc. Natl. Acad. Sci. U.S.A. 108, 7565–7570. doi: 10.1073/pnas.1018972108
Papanikolaou, G., and Pantopoulos, K. (2005). Iron metabolism and toxicity. Toxicol. Appl. Pharmacol. 202, 199–211. doi: 10.1016/j.taap.2004.06.021
Parro, V., Moreno-Paz, M., and Gonzalez-Toril, E. (2007). Analysis of environmental transcriptomes by DNA microarrays. Environ. Microbiol. 9, 453–464. doi: 10.1111/j.1462-2920.2006.01162.x
Pastore, A., Martinelli, D., Piemonte, F., Tozzi, G., Boenzi, S., Di Giovamberardino, G., et al. (2012). Glutathione metabolism in cobalamin deficiency type C (cblC). J. Inherit. Metab. Dis. 37, 125–129. doi: 10.1007/s10545-013-9605-3
Quatrini, R., Lefimil, C., Veloso, F. A., Pedroso, I., Holmes, D. S., and Jedlicki, E. (2007). Bioinformatic prediction and experimental verification of Fur-regulated genes in the extreme acidophile Acidithiobacillus ferrooxidans. Nucleic Acids Res. 35, 2153–2166. doi: 10.1093/nar/gkm068
Ram, R. J., Verberkmoes, N. C., Thelen, M. P., Tyson, G. W., Baker, B. J., Blake, R. C. II, et al. (2005). Community proteomics of a natural microbial biofilm. Science 308, 1915–1920. doi: 10.1126/science.1109070
Raux, E., Schubert, H. L., and Warren, M. J. (2000). Biosynthesis of cobalamin (vitamin B12): a bacterial conundrum. Cell. Mol. Life Sci. 57, 1880–1893. doi: 10.1007/PL00000670
Rawlings, D. E. (2002). Heavy metal mining using microbes. Annu. Rev. Microbiol. 56, 65–91. doi: 10.1146/annurev.micro.56.012302.161052
Rodionov, D. A., Vitreschak, A. G., Mironov, A. A., and Gelfand, M. S. (2003). Comparative genomics of the vitamin B12 metabolism and regulation in prokaryotes. J. Biol. Chem. 278, 41148–41159. doi: 10.1074/jbc.M305837200
Sambrook, J., and Russell, D. W. (2001). Molecular Cloning. A Laboratory Manual. New York, NY: Cold Spring Harbor Laboratory Press.
Scalabrino, G., Veber, D., and Mutti, E. (2008). Experimental and clinical evidence of the role of cytokines and growth factors in the pathogenesis of acquired cobalamin-deficient leukoneuropathy. Brain Res. Rev. 59, 42–54. doi: 10.1016/j.brainresrev.2008.05.001
Schoonen, M. A. A., Harrington, A. D., Laffers, R., and Strongin, D. R. (2010). Role of hydrogen peroxide and hydroxyl radical in pyrite oxidation by molecular oxygen. Geochim. Cosmochim. Acta 74, 4971–4987. doi: 10.1016/j.gca.2010.05.028
Scott, A. I., and Roessner, C. A. (2002). Biosynthesis of cobalamin (vitamin B12). Biochem. Soc. Trans. 30, 613–620. doi: 10.1042/bst0300613
Slyemi, D., and Bonnefoy, V. (2012). How prokaryotes deal with arsenic. Environ. Microbiol. Rep. 4, 571–586. doi: 10.1111/j.1758-2229.2011.00300.x
Suarez-Moreira, E., Yun, J., Birch, C. S., Williams, J. H., McCaddon, A., and Brasch, N. E. (2009). Vitamin B(12) and redox homeostasis: cob(II)alamin reacts with superoxide at rates approaching superoxide dismutase (SOD). J. Am. Chem. Soc. 131, 15078–15079. doi: 10.1021/ja904670x
Takano, H., Beppu, T., and Ueda, K. (2006). The CarA/LitR-family transcriptional regulator: its possible role as a photosensor and wide distribution in non-phototrophic bacteria. Biosci. Biotechnol. Biochem. 70, 2320–2324. doi: 10.1271/bbb.60230
Tian, B., and Hua, Y. (2010). Carotenoid biosynthesis in extremophilic Deinococcus-Thermus bacteria. Trends Microbiol. 18, 512–520. doi: 10.1016/j.tim.2010.07.007
Valko, M., Morris, H., and Cronin, M. T. (2005). Metals, toxicity and oxidative stress. Curr. Med. Chem. 12, 1161–1208. doi: 10.2174/0929867053764635
Watling, H. R., Collinson, D. M., Li, J., Mutch, L., Perrot, F., Rea, S. M., et al. (2014). Bioleaching of a low-grade copper ore, linking leach chemistry and microbiology. Miner. Eng. 56, 35–44. doi: 10.1016/j.mineng.2013.10.023
Yan, J., Şimşir, B., Farmer, A. T., Bi, M., Yang, Y., Campagna, S. R., et al. (2015). The corrinoid cofactor of reductive dehalogenases affects dechlorination rates and extents in organohalide-respiring Dehalococcoides mccartyi. ISME J. 10, 1092–1101. doi: 10.1038/ismej.2015.197
Yonetani, T., and Ray, G. S. (1966). Studies on cytochrome c peroxidase. 3. Kinetics of the peroxidatic oxidation of ferrocytochrome c catalyzed by cytochrome c peroxidase. J. Biol. Chem. 241, 700–706.
Keywords: Leptospirillum group II CF-1, cobalamin, vitamin B12, oxidative stress, heavy metals, bioleaching
Citation: Ferrer A, Rivera J, Zapata C, Norambuena J, Sandoval Á, Chávez R, Orellana O and Levicán G (2016) Cobalamin Protection against Oxidative Stress in the Acidophilic Iron-oxidizing Bacterium Leptospirillum Group II CF-1. Front. Microbiol. 7:748. doi: 10.3389/fmicb.2016.00748
Received: 09 March 2016; Accepted: 03 May 2016;
Published: 23 May 2016.
Edited by:
Axel Schippers, Federal Institute for Geosciences and Natural Resources, GermanyReviewed by:
Wolfgang Sand, Universität Duisburg-Essen, GermanyMark Dopson, Linnaeus University, Sweden
Copyright © 2016 Ferrer, Rivera, Zapata, Norambuena, Sandoval, Chávez, Orellana and Levicán. This is an open-access article distributed under the terms of the Creative Commons Attribution License (CC BY). The use, distribution or reproduction in other forums is permitted, provided the original author(s) or licensor are credited and that the original publication in this journal is cited, in accordance with accepted academic practice. No use, distribution or reproduction is permitted which does not comply with these terms.
*Correspondence: Gloria Levicán, Z2xvcmlhLmxldmljYW5AdXNhY2guY2w=