- 1Department of Botany, Government College University, Faisalabad, Pakistan
- 2Department of Bioinformatics and Biotechnology, Government College University, Faisalabad, Pakistan
Soil salinity severely affects plant nutrient use efficiency and is a worldwide constraint for sustainable crop production. Plant growth-promoting rhizobacteria, with inherent salinity tolerance, are able to enhance plant growth and productivity by inducing modulations in various metabolic pathways. In the present study, we reported the isolation and characterization of a salt-tolerant rhizobacterium from Kallar grass [Leptochloa fusca (L.) Kunth]. Sequencing of the 16S rRNA gene revealed its lineage to Staphylococcus sciuri and it was named as SAT-17. The strain exhibited substantial potential of phosphate solubilization as well as indole-3-acetic acid production (up to 2 M NaCl) and 1-aminocyclopropane-1-carboxylic acid deaminase activity (up to 1.5 M NaCl). Inoculation of a rifampicin-resistant derivative of the SAT-17 with maize, in the absence of salt stress, induced a significant increase in plant biomass together with decreased reactive oxygen species and increased activity of cellular antioxidant enzymes. The derivative strain also significantly accumulated nutrients in roots and shoots, and enhanced chlorophyll and protein contents in comparison with non-inoculated plants. Similar positive effects were observed in the presence of salt stress, although the effect was more prominent at 75 mM in comparison to higher NaCl level (150 mM). The strain survived in the rhizosphere up to 30 days at an optimal population density (ca. 1 × 106 CFU mL-1). It was concluded that S. sciuri strain SAT-17 alleviated maize plants from salt-induced cellular oxidative damage and enhanced growth. Further field experiments should be conducted, considering SAT-17 as a potential bio-fertilizer, to draw parallels between PGPR inoculation, elemental mobility patterns, crop growth and productivity in salt-stressed semi-arid and arid regions.
Introduction
The world has experienced an exponential increase in population within the last few decades leading to a reduced availability of quality food. The problem is worsened due to an increase in the salinization of agricultural lands (Ghafoor et al., 2002). High levels of soluble salt in soil cause deleterious effects on germination, seedling vigor, crop establishment, plant metabolism and reproductive growth (Zhu et al., 2004), which contribute to a reduced yield of agronomically important crops. Exposure of plants to high salinity stress inhibits water uptake by roots and also induces osmotic shock, which modulates cell division, cell expansion and stomatal closure (Flowers, 2004). Long-term exposure to salts causes the increased uptake of Na+ together with a decrease in the uptake of Ca2+ and K+ (Yildirim et al., 2006). Nutritional imbalances/deficiencies result in the senescence of leaves, reducing photosynthetic area necessary to maintain the optimum growth. In addition, uptake and accumulation of Cl- may disrupt photosynthetic function through the inhibition of nitrate reductase activity (Xu et al., 1999). Once the capacity of cells to store salts is exhausted, salts build up in the intercellular space, which results in cell dehydration and death (Sheldon et al., 2004). Moreover, at higher salinity, plants ultimately die due to reduced leaf production and expansion rates caused by oxidative damage (Kravchik and Bernstein, 2013). In Pakistan, 8.6 million hectares of arable land is saline and high yields of crops are usually not acquired because a great amount of time and energy is annually spent on reclamation strategies.
A possible strategy to cope with the low productivity of saline lands is microbial assisted amelioration of salt-induced damage (Dodd and Pérez-Alfocea, 2012). Among soil microbiota, plant growth-promoting rhizobacteria (PGPR) are potential candidates that are capable of colonizing the rhizosphere, penetrating the roots and triggering plant salinity-tolerance mechanisms (Tank and Saraf, 2010; Sheng et al., 2011). They influence plant physiology by releasing growth regulators, 1-aminocyclopropane-1-carboxylic acid (ACC) deaminase activity, enhanced soil phosphate solubilization and up-regulating the conserved salinity responsive mechanisms (Rajput et al., 2013; Kim et al., 2014). Many PGPR with inherent salt-tolerance have been isolated, characterized and applied to plants to increase crop productivity in saline regions (Rajput et al., 2013; Ahmad et al., 2014). There is evidence that PGPR regulate hormonal status (Sahoo et al., 2014) and initiate antioxidant defense mechanisms in plants exposed to high salt stress (Islam et al., 2015). Members of the Staphylococcus genus have been isolated from diverse environments and characterized as having salt-tolerance potential (Roohi et al., 2012; Nanjani and Soni, 2014). It has been reported that Staphylococcus mitigated the deleterious effects of salinity in radish (Yildirim et al., 2008), sweet cherry (Zhou et al., 2015) and strawberry (Karlidag et al., 2013). Sagar et al. (2012) reported that Staphylococcus arlettae strain Cr11 promoted plant growth via the reduction of hexavalent chromium.
Besides up-regulating stress responsive factors, PGPR also enhance the mobilization of fixed nutrients in salt-affected soils (Paul and Lade, 2014). A major soil-fixed nutrient is phosphorus (P) which is bound to cations (Ca2+, Al2+, Fe2+) and thus remains unavailable to plants (Bhattacharyya and Jha, 2012). Farmers apply phosphate-based fertilizers where a very limited amount is used by the plants and a large amount of fertilizers are converted into insoluble complexes in the soil (Zaidi et al., 2009). The excessive use of fertilizers adversely affects the environment as these are a potential source of environmental contamination (Savci, 2012). Moreover, phosphate fertilizers often leach from the soil and cause the eutrophication of surface and groundwater sources (Sharpley, 1999; He et al., 2003). Alternatively, a trend toward the use of slow-release phosphate (rock phosphate) fertilizers has been reported (Duponnois et al., 2005). Furthermore, efforts have also been made to explore PGPR as fertilizer supplements with the objective of substantially reducing the use of synthetic fertilizers (Hanif et al., 2015; Shahid et al., 2015). The issues of low nutrient availability and oxidative damage in saline lands severely affect the growth and physiology of Maize (Zea mays L.), which is one of the important domesticated cereal crops grown widely throughout the world. It is a nutritious rich source of human food and animal feed, and also provides raw material for industrial products.
The present work was designed to explore the potential of a salt-tolerant PGPR strain S. sciuri SAT-17 to boost maize growth under saline environments. To the best of our knowledge, this is the first report elucidating the physiological and phenotypic responses of maize after inoculation with a phyto-beneficial S. sciuri strain. The study will raise attention toward the establishment of long-term programs involving PGPR-based bio-formulations for the efficient utilization of salt-affected soils.
Materials and Methods
Sampling Site and Bacterial Isolation
The roots and rhizospheric soil surrounding Kallar grass [Leptochloa fusca (L.) Kunth] was collected from salt rich fields located at/near Pakka Anna (31°13′60 N and 72°48′0 E), Punjab, Pakistan. The samples were transported to the laboratory in sterilized polythene bags. The roots were shaken gently in sterile distilled water to remove the loosely adhering soil. One gram of strictly adhered soil was added in 9 mL of 0.85% (w/v) NaCl solution and serially diluted, as described by Somasegaran and Hoben (1994). An amount of 100 μL from three dilutions (10-4, 10-5, and 10-6) was spread on nutrient agar, amended with 8% NaCl, and plates were incubated at 28 ± 2°C for 48 h. Purification of the culture was achieved through repeated-streaking and pure culture (designated as SAT-17) was stored in 20% (v/v) glycerol at -80°C. Colony morphology, cell shape, motility and Gram’s reaction was performed under a light microscope (Olympus, Tokyo, Japan) as described earlier (Vincent, 1970). Catalase (CAT) activity was determined by pouring H2O2 on the culture on a glass slide. The physical and chemical analysis of rhizospheric and bulk soil samples was carried out at Ayub Agriculture Research Institute, Faisalabad, Punjab, Pakistan.
Molecular Identification and Phylogenetic Analysis
Total genomic DNA of the isolate “SAT-17” was isolated by the alkaline lysis method (Maniatis et al., 1982), quantified by the NanoDropTM 2000/2000c (Thermo Fisher Scientific, Waltham, MA, USA) and used to amplify the 16S rRNA gene using primers fD1 (5′ AGAGTTTGATCCTGGCTCAG 3′) and rD1 (5′ AAGGAGGTGATCCAGCC 3′) (Weisburg et al., 1991). The reaction mixture and thermocycler conditions were set as described earlier by Shahid et al. (2015). Subsequently, the amplicon was cloned in pTZ57R/T and sequencing of the 16S rRNA gene was carried out by Macrogen, South Korea. Trimming of raw sequences, BLASTn analysis and phylogenetic studies were conducted using the methods and software packages described previously by Shahid et al. (2016).
Characterization of Salt-Tolerance and Plant-Beneficial Traits
Salt-Tolerance Studies
The pure culture of SAT-17 was streaked on nutrient agar media with various NaCl concentrations (0, 0.5, 1, 1.5, 2, 2.5, 3, 3.5, or 4 M) and the salt-tolerance level was determined by measuring the minimum inhibitory concentration (MIC) of NaCl. Additionally, SAT-17 was re-exposed to NaCl concentrations (0, 0.5, 1, 1.5, 2, 2.5 M) in nutrient broth and subjected to the serial dilution method (Somasegaran and Hoben, 1994) to determine the bacterial cell density up to the MIC.
Phosphate Solubilization
A single purified colony of isolate SAT-17 was inoculated in 100 mL Pikovskaya’s broth (Pikovskaya, 1948) medium supplemented with different levels of NaCl (0, 0.5, 1, 1.5, 2 or 2.5 M) and incubated at 30 ± 2°C for 240 h in an orbital shaker (150 rpm). Twenty mililiter of bacterial culture was harvested and centrifuged at 13,000 g for 10 min. The quantitative measurement of phosphate solubilization was performed according to phosphomolybdate blue color method (Murphy and Riley, 1962) using a UV-visible spectrophotometer (Shimadzu UV/VIS, Kyoto, Japan) at 882 nm.
Indole-3-Acetic Acid (IAA) Production
The method described by Gordon and Weber (1951) was used to estimate IAA synthesis potential of the strain SAT-17. A single colony of SAT-17 was inoculated to 100 mL nutrient broth media with (100 mg L-1) or without tryptophan, each amended with NaCl (0, 0.5, 1, 1.5, 2, or 2.5 M). The cultures were grown on an orbital shaker (150 rpm) at 30 ± 2°C for 48 h. Thereafter, the cultures were harvested and centrifuged at 13,000 g. An amount of 1 mL supernatant was mixed in 2 mL of Salkowisk’s reagent. The tubes were kept in the dark for 30 min for color development. Quantification was carried out through a spectrophotometer at 540 nm. The IAA solutions (0, 5, 10, 50, 100, 200, or 500 μg mL-1) were used to draw standard curve for comparative measurements.
1-Aminocyclopropane-1-Carboxylic Acid (ACC) Deaminase Activity
The ability of isolate SAT-17 to use ACC as a sole nitrogen source was assessed in 5 mL DF salt minimal medium (Penrose and Glick, 2003) containing 3 μL of 0.5 M ACC and supplemented with different concentrations (0, 0.5, 1, 1.5, 2, or 2.5 M) of NaCl. Cultures were grown at 30 ± 2°C for 24 h in a shaker. To determine ACC deaminase activity, the turbidity of the inoculated cultures was compared to that of the non-inoculated control.
Greenhouse Experiment
Comparative Fitness Studies and Inoculum Preparation
Rifampicin-resistant derivatives of strain SAT-17 (SAT-17rif) were constructed, followed by comparative growth studies with its wild-type (SAT-17w) as described earlier by Shahid et al. (2012). For inoculum preparation, SAT-17rif was grown up to 109 CFU mL-1 cell density. The culture was centrifuged at 8,000 g and washed twice with ddH2O. The cells were re-suspended in equal volume of ddH2O and diluted to 108 CFU mL-1.
Experimental Soil
The soil with textural class clay loam (available P: 8.3 mg kg-1, total N: 0.89 g kg-1, available K: 112 mg kg-1, organic matter 1.6% and pH 7.1) was obtained from the botanical garden of Government College University, Faisalabad, Pakistan. The soil was pre-inoculated by mixing 7 mL of SAT-17rif inoculum per 100 g of soil (inoculated soil) or by mixing 7 mL of ddH2O per 100 g of soil (non-inoculated soil). A total of 18 pots were filled (each with 600 g soil) where nine pots received the inoculated and nine the non-inoculated soil.
Plant Material and Experimental Design
Maize seeds (FH-992) were surface-sterilized by immersing in 5% (w/v) sodium hypochlorite for 10 min and subsequently washed thrice with ddH2O. The seeds were submerged in SAT-17rif inoculum and ddH2O separately for 20 min. The SAT-17rif-inoculated seeds were sown in pots containing inoculated soil, while ddH2O-dipped seeds were sown in non-inoculated pots. Seed rate was set at eight seeds per pot. The plants were thinned to five plants per pot after seedling emergence. Initially, the pots were irrigated, with a 3 days interval, using canal water. When seedlings emerged, these were watered periodically with equal volumes of half strength Hoagland solution (Arnon and Hoagland, 1940) with 3 NaCl levels, i.e., 0, 75 or 100 mM (Batool et al., 2013; Kim et al., 2014). The total treatments were named as follows:
Control0: non-inoculated soil with 0 mM NaCl
Control75: non-inoculated soil with 75 mM NaCl
Control150: non-inoculated soil with 150 mM NaCl
SAT-170: SAT-17rif inoculated soil with 0 mM NaCl
SAT-1775: SAT-17rif inoculated soil with 75 mM NaCl
SAT-17150: SAT-17rif inoculated soil with 150 mM NaCl
The experiment was conducted in a greenhouse (day/night temperature 25/20°C, light/dark periods 16/8) with a completely randomized design (CRD) and three replications for each treatment.
Bacterial Recovery and Growth Data
Before seed sowing, three random samples from inoculated and non-inoculated soil were serially diluted (Somasegaran and Hoben, 1994) and spread on nutrient-agar plates amended with (50 μg mL-1) rifampicin to determine initial soil population density of SAT-17rif. Thereafter, one plant from each inoculated and non-inoculated pot was randomly uprooted, at 10, 20, and 30 days after sowing (DAS) and a survival rate of SAT-17rif was determined, as described by Shahid et al. (2012). Plant growth attributes (length as well as fresh and dry weights) were recorded for the remaining plants at 30 DAS.
Analysis of Physiological Parameters and Nutrient Acquisition Patterns of Maize Plants
Lipid Peroxidation
The malondialdehyde (MDA) content of plant tissue was determined by thiobarbituric acid (TBA) reaction (Heath and Packer, 1968) to estimate the level of lipid peroxidation. The shoots homogenized with 0.1% trichloroacetic acid (TCA) were centrifuged and the supernatant was added to 20% TCA containing 0.5% TBA. The reaction mixture was heated (100°C) for 30 min and subsequently cooled to stop the reaction. The samples were centrifuged again and the absorbance of the supernatant was measured at 532 nm using Ultrospec 3000 (Biochrom Ltd, Cambridge, England) and adjusted for non-specific absorbance at 600 nm. The extinction coefficient was 155 mM cm-1.
Proline Content
The method of Bates et al. (1973) was used to determine the proline concentrations. Fresh leaves (ca. 0.5 g) were homogenized in sulfo-salicylic acid (3%, w/v) and the filtrate (2 mL) was mixed with 2 mL of acid ninhydrin reagent and 2 mL of glacial acetic acid. The mixture was incubated at 100°C for 60 min followed by cooling. Toluene (4 mL) was added to the solution and the contents were mixed well. The absorbance of the lower layer (chromophore-containing toluene) was observed spectrophotometrically at 520 nm.
Catalase Activity
Leaf material was homogenized in phosphate buffer (50 mM and pH 7.8) and centrifuged at 10,000 g for 10 min. The CAT activity of the supernatant was measured (Aebi, 1984) where the sample (ca. 100 μL) was mixed with H2O2 (0.75 M) and a decrease in absorbance was recorded at 240 nm for 20 s with the Ultrospec 3000 (Biochrom Ltd, Cambridge, England). Extinction coefficient was 0.039 mM cm-1.
Peroxidase (POD) Activity
The protocol described by Chance and Maehly (1955) with some modifications was used for the determination of POD activity. The plant samples were ground in phosphate buffer (pH 7.8) and centrifugation was performed at 8,000 g at 25°C. The absorbance of the reaction mixture [(2.7 mL phosphate buffer (pH 5), 0.1 mL Guiacol (20 mM), 0.1 mL H2O2 (40 mM) and 0.1 mL plant extract] was measured every 20 s for 2 min at 470 nm in a spectrophotometer. One unit of POD activity was considered as change of absorbance of 0.01 units min-1.
Total Phenolics Content
A 0.5 g of fresh leaf tissue was homogenized in 80% (v/v) acetone solution and centrifuged at 10,000 g for 10 min at 4°C. The supernatant (100 μL) was diluted with 2 mL of water plus 1 mL of Folin–Ciocalteau’s phenol reagent. Five mL of 20% (w/v) Na2CO3 was then added and the volume was made up to 10 mL with ddH2O. The absorbance was read at 750 nm and the results were expressed as mg g-1 FW of leaf (Julkunen-Tiitto, 1985) by comparison with standards of known concentrations.
Determination of Plant Nutrient Elements
The plant roots and shoots were oven-dried at 105°C for 24 h. The oven-dried ground plant material (ca. 0.5 g) was taken in digestion flasks containing 5 mL conc. H2SO4 (Wolf, 1982). The flasks were incubated overnight at room temperature. A 0.5 mL solution of 35% (v/v) H2O2 was poured and the flasks were then placed over a hot plate (350°C). The digestion flasks remained on a hot plate until no fumes were produced. Afterward, these were removed from the hot plate and allowed to cool. The step was repeated until the digestion mixture became fully transparent. The cooled mixtures were then diluted up to 50 mL, filtered and stored at 4°C. The N, P, K, Ca, and Mg contents of the dried shoot and root samples were determined using an atomic absorption spectrophotometer (Hitachi, Model 7JO-8024, Tokyo, Japan) with flame spectrophotometry. To minimize the matrix affect during plant metal analysis, standard reference materials (SRM) and standard solutions were used.
Plant Chlorophyll Content
The method of Arnon (1949) was used for the determination of plant chlorophyll contents. An 80% (v/v) solution of acetone was used for homogenization followed by centrifugation and filtration. The absorbance of the supernatant was recorded using a spectrophotometer at three different wavelengths, i.e., 663, 645, and 480 nm.
Plant Protein Content
Fresh plant material was homogenized, centrifuged (at 10,000 g for 15 min at 4°C) and mixed with Bradford reagent (Bradford, 1976). The mixture was incubated for 15–20 min and the absorbance was measured spectrophotometrically at 595 nm. Total soluble proteins were estimated by comparison with a standard curve of bovine serum albumin (BSA).
Data Analysis
Data for green house experiments were analyzed statistically by analysis of variance (Steel et al., 1997) using Statistix (ver. 8.1) software. The least significant difference test (Fisher’s LSD) at 5% probability was used to compare the differences between treatment means. The phylogenetic tree was constructed with MEGA (ver. 6) software (Tamura et al., 2013).
Results
Soil Physicochemical Analysis
The physical and chemical properties of bulk and Kallar grass rhizospheric soil samples collected from Pakka Anna were determined and are presented in Table 1. Soil texture was sandy loam for bulk soil and sandy clay loam for rhizospheric soil. Both soils exhibited high EC and pH but were deficient in organic matter contents; this is an indication of saline nature. The examined soils were also low in total N and available P contents.
Molecular Identification of SAT-17
The isolate was identified as S. sciuri SAT-17 on the basis of 16S rRNA gene sequence analysis. BLASTn analysis of 1388 bp sequence (submitted to NCBI GenBank as Acc. # KU672729) of SAT-17 showed 99% sequence identity with S. sciuri PS25 (Acc. # KM276789) and S. sciuri DHAN01 (Acc. # KT270573) (Supplementary Figure S1). Furthermore, strain SAT-17 was placed in a cluster of S. sciuri ATCC 29062T (Acc. # S83569), S. sciuri PS25 (Acc. # KM276789) and S. sciuri DHAN01 (Acc. # KT270573) in a phylogenetic tree (Figure 1).
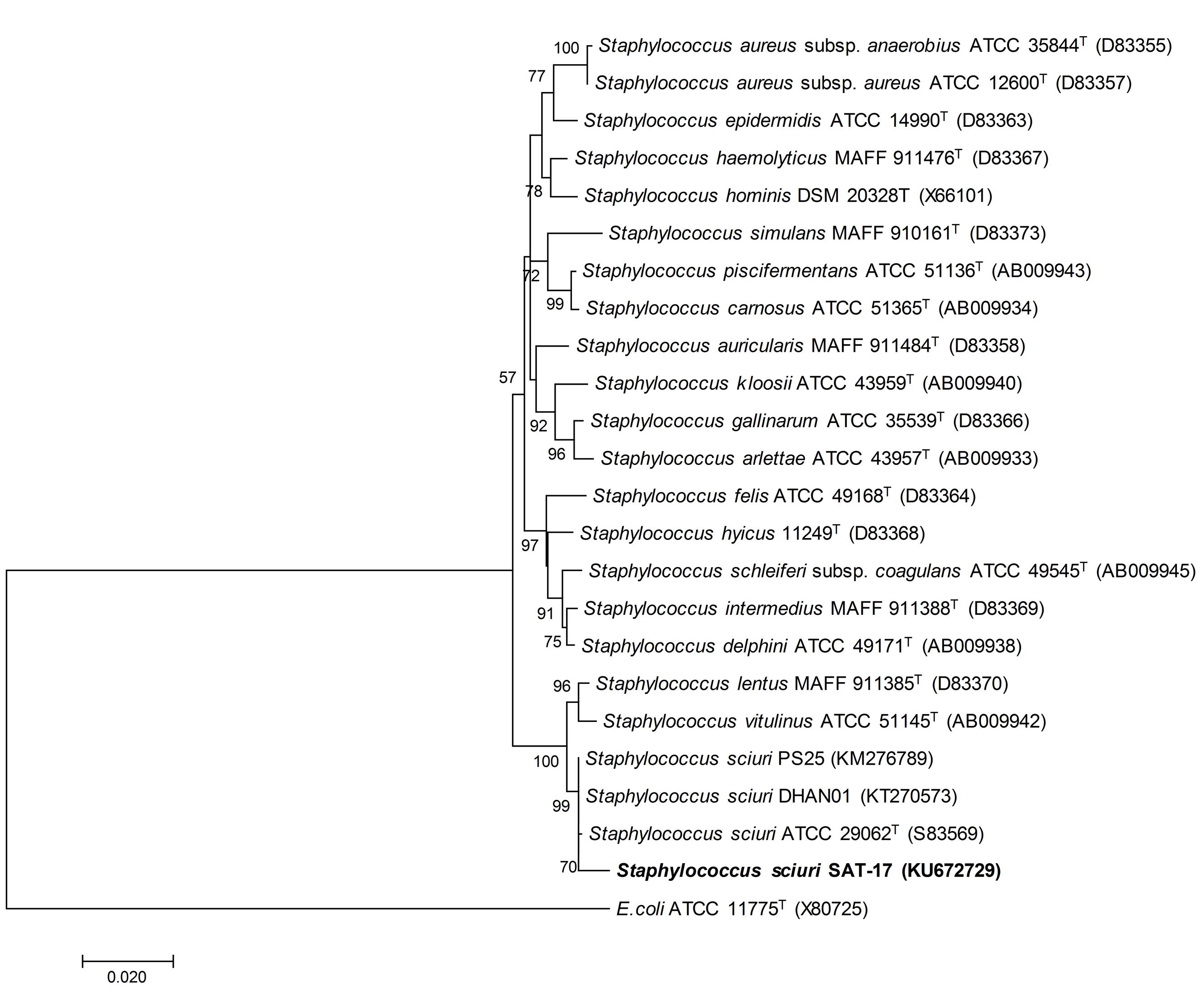
FIGURE 1. Phylogenetic analysis of S. sciuri SAT-17 with type strains of genus Staphylococcus and the closest GenBank matches. The evolutionary history was inferred using the Neighbor-Joining method. The optimal tree with the sum of branch length = 0.39351674 is shown. The percentages (≥50%) of replicate trees in which the associated taxa clustered together in the bootstrap test (1000 replicates) are shown. The evolutionary distances were computed using the Maximum Composite Likelihood method and are in the units of the number of base substitutions per site. Codon positions of 24 nucleotide sequences included were 1st + 2nd + 3rd + Non-coding.
Physiological Characterization
Staphylococcus sciuri SAT-17 was able to grow in culture medium amended with 2.5 M NaCl and this level of in vitro salinity stress was considered the MIC for the strain. Moreover, the bacterial cell density (Log CFU mL-1 24 h-1) was measured in the following descending order at 0, 0.5, 1, 1.5, and 2 M NaCl concentration: 10.6 ± 0.7 > 10.5 ± 0.8 > 9.3 ± 0.4 > 7.2 ± 0.5 > 5.4 ± 0.3 > 2.9 ± 0.3 (Table 2).
The tricalcium phosphate (TCP) solubilizing ability of the strain was found to be up to 40.8 ± 5.2 μg mL-1 in the presence of 0.5 M salt. This ability decreased together with an increase in salt level and only 7.6 ± 1.1 μg mL-1 TCP was solubilized at a concentration of 2 M NaCl. The strain synthesized IAA (3.2 ± 0.23 μg mL-1) up to 2 M NaCl addition in the presence of tryptophan, while in the absence of tryptophan, 11.9 ± 0.73 μg mL-1 IAA was measured up to 1.0 M NaCl stress. S. sciuri SAT-17 was also found to be positive for in vitro ACC deaminase activity with the addition of up to 1.5 M NaCl (Table 2).
Comparative Growth Studies and Bacterial Recovery from Rhizosphere
The wild type strain (SAT-17w) and its derivative (SAT-17rif) showed similar growth behaviors in plate count method and spectrophotometric OD methods, as presented in Figure 2. The growth of both SAT-17w and SAT-17rif was found to be optimal (ca. 1 × 1010 CFU mL-1) and a normal bacterial growth curve pattern was obtained after plotting the measurements obtained at different time intervals.
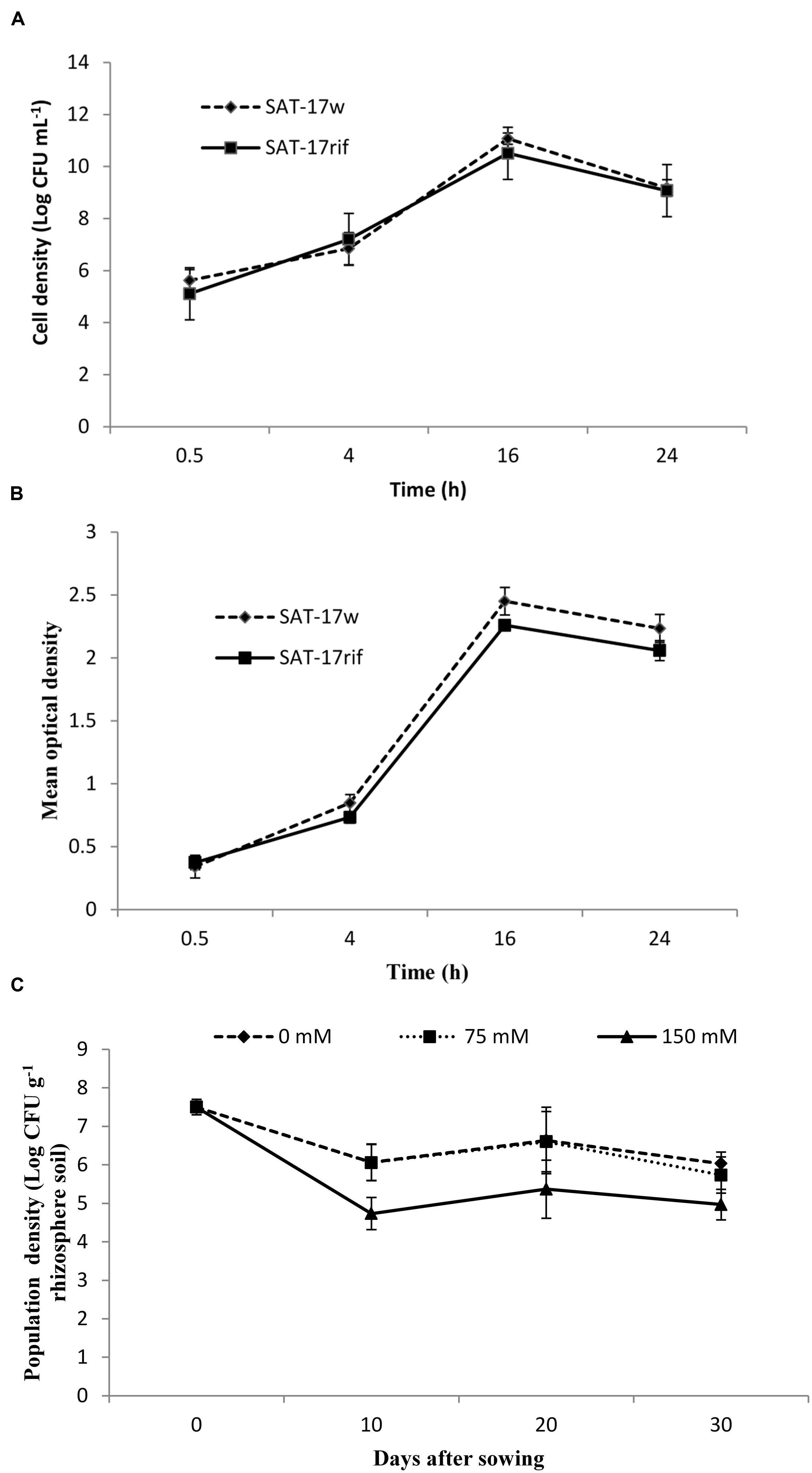
FIGURE 2. Comparative growth curve of wild-type and rifampicin-resistant derivative of S. sciuri SAT-17 constructed with the plate-count method (A) and by measuring OD (B). Population density of S. sciuri SAT-17 recovered from maize rhizosphere at different time intervals (C). Error bars represent standard errors (n = 3).
The population density of SAT-17rif, on rifampicin-amended agar plants before seed sowing, was found to be 3.5 × 107 CFU g-1 of soil. The strain survived in the maize rhizosphere up to 30 DAS at an optimal population density of 6 × 106 CFU g-1 soil (in treatment SAT-170) and 4.9 × 105 CFU g-1 soil (in treatment SAT-1775). The population density of SAT17rif decreased to 8.9 × 104 CFU g-1 in the treatment SAT-17150. We did not observe any bacterial growth on agar plates for the treatments control0, control75 and control150 (Figure 2C).
Effect of Salt Stress and SAT-17 Inoculation on Maize Growth
In the absence of SAT-17 inoculation, a decrease in root length was observed with increasing salt level. The SAT-17 inoculation resulted in enhanced root length, with a maximum (9.98 cm) in the absence of NaCl. At 75 mM salt stress, the percentage difference between the SAT-17 treated and non-treated plants was 55.9%, where the treated ones exhibited greater root length (8.83 cm). Similarly, at 150 mM salt stress, we observed a percentage difference of 42.6% with higher root length in plants that received the treatment, i.e., SAT-17150 (Table 3).
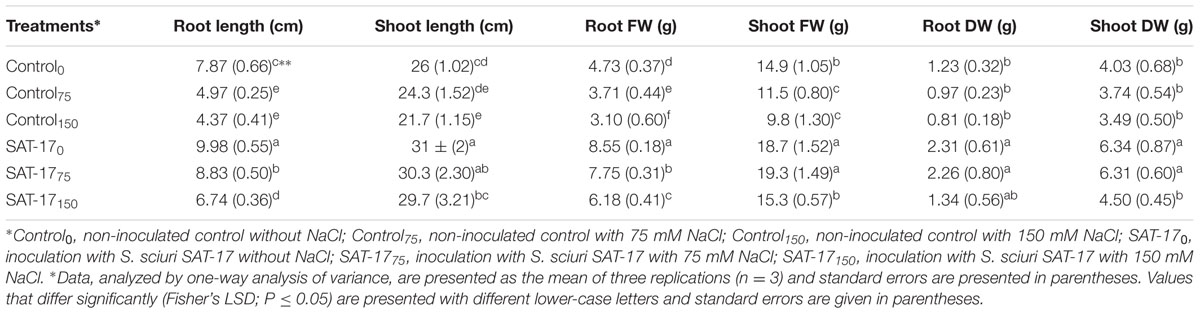
TABLE 3. Effect of S. sciuri SAT-17 inoculation on various growth parameters of maize with or without salt stress.
The control plants exhibited a shoot length of 26 cm and the addition of salt reduced the shoot length, significantly at 150 mM (19.2%). The inoculation with SAT-17 increased the shoot length both in the absence (0 mM) and presence (75 or 150 mM) of NaCl. The SAT-17 treatment, in the presence of 75 mM salt, resulted in a 24.6% increase in shoot length compared to the control, while, at this level of stress, there was 31.1% difference in shoot length between SAT-17 treated (29.7 cm shoot length) and non-treated (21.7 cm shoot length) plants (Supplementary Figure S3). The control plants and those grown in the presence of 150 mM salt, without SAT-17 treatment, exhibited 34% difference for the root fresh weight, where a lower weight was recorded in the latter group (3.1 g). The SAT-17 application increased the root FW, irrespective of the level of imposed stress. A similar trend was observed for root DW as well as shoot biomass.
Effect of Salt Stress and SAT-17 Inoculation on Antioxidants
The control plants exhibited a phenolics content of 26.8 mg g-1 FW. When no SAT-17 treatment was applied, a reduction of 25 and 31.3% was observed, in comparison to the control, at 75 and 150 mM stress, respectively. When no salt stress was present, the SAT-17 treatment enhanced the content up to 50.3% (40.3 mg g-1 FW). Plants grown at a salt level of 75 mM along with SAT-17 treatment exhibited higher phenolic content (37.6 mg g-1 FW) compared to plants that received 75 mM salt stress but no SAT-17 inoculation (20.1 mg g-1 FW). There was a 42.8% difference between the SAT-17 treated and non-treated plants at 150 mM salt stress, with the former resulting in higher phenolics content (30.1 mg g-1 FW). Without SAT-17 application, we observed a 46.2% (at 75 mM salt) and 153% (at 150 mM salt) increase in the MDA content compared to the control plants (1.75 nmole/g-1 DW). The plants that received SAT-17 treatment, in the absence of salt, exhibited a 13.7% decrease in MDA content. At 75 mM salt stress, the MDA contents were 61.2% different in SAT-17 treated (1.3 nmole/g-1 DW) and non-treated plants (2.56 nmole/g-1 DW). A similar trend was observed at a salt level of 150 mM, where treated plants exhibited less MDA than the non-treated ones.
An H2O2 level of 13.8 ng g-1 DW was observed in the control plants. The presence of salt, without SAT-17rif treatment, increased the H2O2 level, significantly (26.0%) at 150 mM salt stress. The plants that received 75 mM salt stress exhibited higher H2O2 levels (14.2 ng g-1 DW) than those which were grown with salt and SAT-17 treatment (12.5 ng g-1 DW). A similar trend was observed for plants grown with 150 mM salt stress alone and those that received salt stress as well as SAT-17 treatment. Without SAT-17 treatment, the maximum proline content (2.97 μg g-1 DW) was observed in plants grown with 150 mM salt level, with results that were 10.8 and 8.3% higher than for those plants grown with 0 and 75 mM salt levels, respectively. The application of SAT-17 (at 0 mM salt level) reduced the proline content in comparison to control plants, while at 75 mM, the SAT-17 treatment significantly increased (11.0%) the proline content in comparison to the plants that did not receive the treatment. There were no significant differences in proline content of the plants that received (2.96 μg g-1 DW) or did not receive (2.97 μg g-1 DW) SAT-17 treatment at the salt level of 150 mM. However, the observed values were significantly higher than those reported in the control plants (2.68 μg g-1 DW proline).
Without SAT17rif inoculation, the 75 mM NaCl level increased the CAT activity (32.5 U/mg protein), while the higher stress level (150 mM) resulted in reduced (19.8 U/mg protein) CAT activity in comparison to control plants (28 U/mg protein). The imposition of salt stress (75 or 150 mM) combined with SAT-17 treatment (SAT-1775 and SAT-17150) resulted in higher CAT activity compared to the plants that received salt stress but not the SAT-17 application (Control75 and Control150). The maximum POD activity (38.2 U/mg protein) was observed in plants grown under 150 mM salt stress without SAT17rif inoculation (control150 treatment). This POD activity was 70.5 and 101% higher than the activity observed in control0 (22.4 U/mg protein) and SAT-170 (19.0 U/mg protein) plants, respectively. At 75 mM salt level, we observed a 9.9% difference in POD activity between the SAT-17 treated and non-treated plants. However, at the 150 mM salt level, the SAT-17 treated and non-treated plants exhibited a similar POD activity (Table 4).
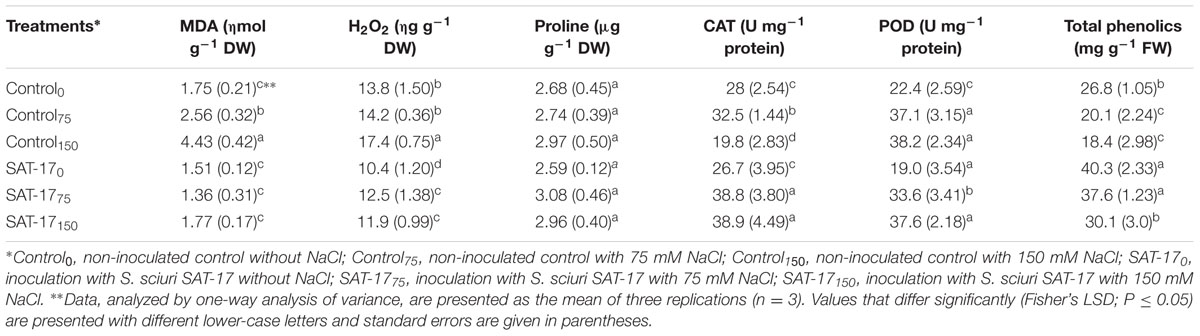
TABLE 4. Oxidative stress responsive species and antioxidants as affected by inoculation of S. sciuri SAT-17 at different salinity stress levels.
Effect of Salt Stress and SAT-17 Inoculation on Maize Nutrient Physiology
The addition of NaCl hindered the uptake of soil N and a decrease in root N content was observed together with an increase in the imposed salt stress. Without SAT-17 treatment, a decrease of 13.6% in N content was observed in plants grown in the presence of 150 mM salt in comparison to control plants (30 mg g-1 DW). The SAT-17 treatment, at 75 mM salt, resulted in increased N content (30.5 mg g-1 DW) in comparison to plants that received only salt stress (Control75). However, at higher salt stress (150 mM), the difference between SAT-17 treated and non-treated plants was non-significant, with the former resulting in 26.1 mg g-1 DW and the latter 25.9 mg g-1 DW root N content. The SAT-17 treatment, in the absence or presence of salt stress, increased the shoot N content where the maximum shoot N content (28.7 mg g-1 DW) was observed in plants grown without salt stress (SAT-170). At 75 mM stress, the difference between the SAT-17 treated and non-treated group was 9.64%, where the treated group showed higher shoot N. However, at a salt level of 150 mM, the difference between SAT-17 treated (SAT-17150) and non-treated (Control150) plants was not significant, with 20.2 mg g-1 DW and 19.6 mg g-1 DW shoot N, respectively (Table 5).
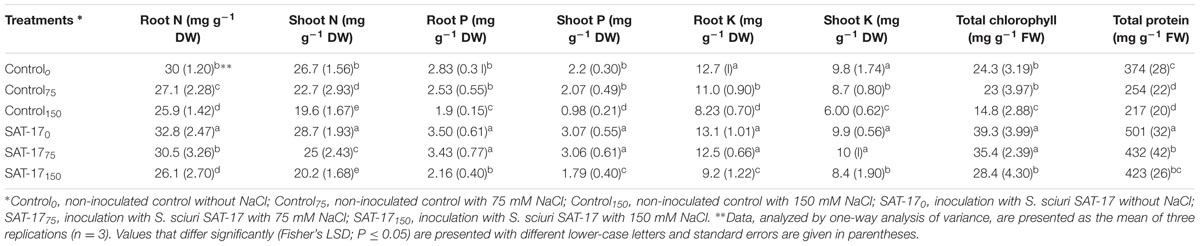
TABLE 5. Effect of S. sciuri SAT-17 inoculation on maize nutrient, chlorophyll and protein contents at different levels of salt-stress.
The control plants exhibited 2.83 mg g-1 DW root P content. The addition of salt reduced the P uptake, significantly at the salt level of 150 mM (1.9 mg g-1 DW root P content). The SAT-17 treatment increased the uptake of P, at 0 and 75 mM NaCl levels, as indicated by higher root P content. At the higher salt level (150 mM), the reduction in P uptake was 2.16 mg g-1 DW and 1.9 mg g-1 DW with and without SAT-17 treatment, respectively. Without SAT-17 inoculation, the imposed salt stress reduced the shoot P content either non-significantly (at 75 mM salt) or significantly (at 150 mM salt) compared to control plants (2.2 mg g-1 DW shoot P content). The SAT-17 treatment increased the shoot P content, significantly, at all of the applied salt levels.
The root K contents were decreased together with an increase in the salt stress level, where 150 mM NaCl resulted in a 35.1% decrease in root K in comparison to the control (12.7 mg g-1 DW). The control plants exhibited 9.8 mg g-1 DW shoot K content. Without SAT-17 treatment, 150 mM salt stress resulted in a 38.7% decrease, while, at this level, the SAT-17 treated plants exhibited only a 14.2% decrease in comparison to control. The effect of strain SAT-17rif inoculation on the uptake and translocation of Ca and Mg was found to be non-significant compared to non-inoculated plants exposed to different salinity levels (data not shown).
The control maize plants showed 24.3 mg g-1 FW total chlorophyll (Chl) content while SAT-17rif inoculation, in the absence of salt, resulted in a 38.1% increase (39.3 mg g-1 FW). The salt stress, without SAT-17 application, resulted in a reduction of maize Chl contents, which was significant (64.1%) at 150 mM. A difference of 42.4% was observed between the treated and non-treated plants grown with 75 mM salt stress, where the former showed a higher Chl content (35.4 mg g-1 FW). A similar trend was observed between the treated and non-treated plants at a salt level of 150 mM.
Discussion
The present work reported the identification and characterization of a PGPR strain isolated from the rhizosphere of a halophytic plant “Kallar grass [Leptochloa fusca (L.) Kunth].” The 16 s gene sequence identity (99%) and its clustering with S. sciuri ATCC 29062T (S83569) (Figure 1) confirmed its molecular identity as S. sciuri and the strain was designated as SAT-17 (Supplementary Figure S2). Most strains of S. sciuri have been reported to form commensal associations with animals (Nemeghaire et al., 2014). Members of the genus Staphylococcus can tolerate high salt concentrations (Roohi et al., 2012; Khan et al., 2015) and exhibit plant growth-promoting properties (Yildirim et al., 2008). Zhou et al. (2015) reported the increased growth of sweet cherry plants after inoculation with a PGPR strain S. sciuri subspecies sciuri, grown in sterilized soil.
The in vitro phosphate solubilization ability of the strain was comparable with that reported previously (Oliveira et al., 2009). Solubilization of bound soil phosphate by PGPR triggered soil acidification by the production of organic acids, depending upon the number of carboxylic groups carried. The rhizospheric acidosis promoted the release of cations (Al2+, Fe2+, Ca2+) associated with phosphate, thereby making it available for plant uptake (Mullen, 2005; Trivedi and Sa, 2008). The strain SAT-17 produced a significant amount of IAA up to a salt treatment level of 2 M with the addition of tryptophan (Table 2). Spaepen et al. (2007) reported that IAA is one of the best characterized traits of many PGPR and it is an important phyto-hormone involved in the regulation of plant growth and development. The hormone is also thought to be involved in plant stress responses as a signaling molecule (Spaepen and Vanderleyden, 2011). The SAT-17 also exhibited ACC deaminase activity, a key factor for a PGPR strain to induce stress tolerance in host plants by controlling ethylene concentrations (Glick et al., 2007). Furthermore, the salt treatment level of 2.5 M was found to be the MIC for the isolate SAT-17, making it physiologically more competent than the previously reported PGPR strains.
The rifampicin-resistant derivatives of strain SAT-17 (S. sciuri SAT-17rif) were constructed so that the strain could successfully be recovered and identified after inoculation. Rifampicin was selected as a selectable marker due to the susceptibility of most soil bacteria against rifampicin (Shahid et al., 2012). The comparative growth curves of SAT-17w and SAT-17rif revealed that the derivative strain was healthy enough to inoculate the maize seedlings. It survived in the rhizosphere, with an optimal population density of 6 × 106 CFU g-1 rhizospheric soil, up to 30 DAS, suggesting that the strain was rhizospherically competent (Shahid et al., 2012). Many PGPR exhibiting in vitro beneficial traits have failed to induce positive effects, in vivo, due to poor root colonization and antagonism with the native soil microorganisms (Benizri et al., 2001). The SAT-17rif density was initially declined in the soil but later the strain adapted well and survived. Fischer et al. (2010) also reported an initial decrease in the density of the rifampicin-resistant derivative of Pseudomonas sp. SF4c in the rhizosphere of wheat which later became stable and induced beneficial effects.
Plant growth-promoting rhizobacteria can relieve plants from the deleterious effects of salinity and enhance plant growth and productivity through a variety of mechanisms (Rajput et al., 2013; Kim et al., 2014; Islam et al., 2015). S. sciuri SAT-17rif, when used as an inoculum, significantly promoted the growth and biomass of maize plants grown in the absence and presence of 75 mM salt stress, each compared with the non-inoculated ones (Table 3). Adverse effects of salt stress on plant growth are mainly attributed to limited water uptake as a result of ion osmotic effect, which in turn affect the photosynthetic rate, cell function, nutrient balance and several other metabolic functions (Kumar et al., 2005). In the present study, it seems likely that SAT-17rif modulated the plants’ ability to uptake water more efficiently, probably by modulating the root system architecture as the root hairs and lateral roots are the main sites for PGPR colonization in members of the family Poaceae (Combes-Meynet et al., 2011; Couillerot et al., 2011). Moreover, PGPR supply may enhance nutrient uptake by stimulating root formation (Yildirim et al., 2011) and inhibiting the salt-ion accumulation (Mayak et al., 2004), which in turn promotes plant growth (Supplementary Figure S3). Furthermore, PGPR inoculation might altered the source-sink relations as Capsicum annuum plants, co-inoculated with Azospirillum brasilense and Pantoea dispersa, exhibited the higher plant dry matter accumulation associated with a higher source activity, stomatal conductance and photosynthesis (del Amor and Cuadra-Crespo, 2012). As the SAT-17 produced optimum IAA in vitro, it was suggested that IAA acted as a negative feedback signal to temporarily repress cytokinin synthesis in the roots and their transport to the shoot (Rahayu et al., 2005) leading to increased root elongation. The observed effects on plant biomass may also be attributed to modulated regulation of ethylene, as SAT-17 exhibited significant ACC deaminase activity (Dodd and Pérez-Alfocea, 2012; Kim et al., 2014). Our results coincide well with the findings of Rajput et al. (2013) who reported an increase in the growth and yield of wheat under salt stress conditions after treating the wheat plants with Planococcus rifietoensis strain SAL-15. In another study, Ahmad et al. (2014) reported that the integrated use of PGPR, biogas slurry and chemical nitrogen enhanced maize growth and productivity. The PGPR strains Erwinia persicinus RA2 (with ACC deaminase activity) and Bacillus pumilus WP8 (without ACC deaminase activity) significantly enhanced tomato growth and quality under seawater irrigation (Cha-Um and Kirdmanee, 2009).
In non-inoculated plants, salt stress increased the MDA content and triggered the production of H2O2; this is an indication of oxidative damage to membranes, as reported for various crops (Cui and Wang, 2006; Nasraoui-Hajaji et al., 2012). Increased MDA contents at the cellular level resulted in oxidative injury as well as a disruption of nutrient ion balance (Azooz et al., 2009). Ashraf (2010) reported that reduced leaf water potential was observed due to lipid peroxidation and ionic leakage induced by salt stress. Inoculation of maize seedlings with S. sciuri SAT-17rif significantly prevented salt-induced lipid peroxidation of membranes, as evidenced by the decreased MDA contents in the present study.
Proline may act as an enzyme protectant and stabilize the structure of other macromolecules (Mahajan and Tuteja, 2005).
In the present study, increased proline accumulation in the inoculated plants alleviated the adverse effects of salt stress. It is well known that proline accumulation and other compatible solutes under stress conditions might help with the osmotic adjustment at a cellular level. PGPR are well known for producing resistance in plants against various stresses like salinity through the enhanced production of antioxidants (Younesi and Moradi, 2014).
The adverse environmental conditions triggered the synthesis of hydrogen peroxide (H2O2), superoxide (O2-), and/or hydroxyl (OH-) radicals (Shigeoka et al., 2002); all of these ROS pose potential hazards if they are not detoxified by the plants. The anti-oxidative defense system, comprised of enzymatic and non-enzymatic components, provides a mechanism against the deleterious actions of ROS in plant cells (Ali and Ashraf, 2011). Inoculated maize plants under salinity treatments (75 and 150 mM) showed significantly reduced ROS levels and elevated levels of antioxidant enzymes (CAT, POD), suggesting the induction of oxidative damage repair mechanisms (Table 4). Our results were in agreement with earlier findings that PGPR strains under salinity stress environments triggered the stress responsive non-enzymatic (Kim et al., 2014; Islam et al., 2015) and enzymatic anti-oxidative defense systems (comprised of CAT and POD) (Ali and Ashraf, 2011; Masood et al., 2012). The PGPR-induced salt alleviation may be attributed to bacterial exopolysaccharides which adhere to soil Na+ and prevent its transfer to shoots/leaves (Ashraf et al., 2004). In addition, PGPR also confer salt-tolerance in plants by the tissue-specific regulation of the Na transporter HKT1 (Zhang et al., 2008). Under saline conditions, PGPR regulate the enzymes of important metabolic pathways (tricarboxylic acid cycle, glyoxylate cycle, glycolysis) and improved the energetic status of the plant (Sheng et al., 2011), which in turn help to sustain ionic homeostasis by maintaining the Na+ exclusion capacity in the roots and delay the incidence of toxic ionic effects (Pérez-Alfocea et al., 2010). Sahoo et al. (2014) reported that Azotobacter vinellandii (SRIAz3), isolated from rice rhizosphere, improved rice productivity by inducing stress tolerance and altering plant endogenous hormonal levels.
Salt stress negatively affected the uptake of N, P and K in the roots and shoots of maize, probably due to the increased uptake of Na and Cl ions (Table 5). Earlier works reported that K+ uptake in tomato plants decreased due to the antagonistic relationship of Na+ and K+ at the root surface under NaCl stress (Bastias et al., 2010). Furthermore, the root membrane structure and selectivity for ions has also been reported to be negatively affected under salt stress due to the interference of Na+ with K+ (Grattan and Grieve, 1999). Inoculation of maize seedlings with S. sciuri SAT-17rif significantly increased the uptake of N, P, and K+ in roots (and translocation to the shoots) compared to the non-inoculated control. S. sciuri SAT-17 was a potential phosphate-solubilizing strain; hence it enhanced the mobilization of P from the soil to the roots/shoots. Similarly, the plant growth-promoting effect of the strain favored the maize plants accumulating more macronutrients in the biomass. Our results are in accordance with the findings of Mohamed and Gomaa (2012), who reported a significant increase in macronutrient (N, P, K+, Ca2+ and Mg2+) concentrations in radish when the seeds were inoculated with PGPR (Bacillus subtilis and Pseudomonas fluorescens). Yildirim et al. (2011) reported that the increased nutrient concentrations in broccoli plants were due to the increased root surface area as well as root exudation. The enhanced root exudation up-regulates microbial activity, leading to increased soil nutrient solubility and, later, higher influx into the plant roots (Adesemoye et al., 2008). The enhanced nutrient uptake by maize roots, after SAT-17 inoculation, may be attributed to a low rhizospheric pH which increased the bioavailable fraction of cationic nutrients as reported earlier (Abou-Shanab et al., 2006). However, the underlying mechanisms are not yet completely elucidated. A similar trend was also determined for total chlorophyll and protein contents of the plants. Earlier works with PGPR have also been shown to alleviate NaCl stress by increasing the leaf chlorophyll contents. The increase in leaf chlorophyll contents under PGPR inoculation was attributed to the increased nutrient availability (Karlidag et al., 2013). The observed effect is also likely due to root proliferation and enhanced water absorption, which in turn increases the leaf numbers and leaf surface area for photosynthesis. Hence, increased chlorophyll contents, after S. sciuri SAT-17rif inoculation, were responsible for the shoot growth enhancement under salt stress which offered new binding sites for nutrient ions. Under salt stress, the protein contents were significantly reduced, probably due to biological degradation, as the toxic ions can activate the mechanism of protein denaturation in maize plants (Gadd and Griffith, 1978). Alterations in protein contents under saline conditions may modulate the enzyme activities responsible for the anti-oxidative defense to cope with salt-mediated production of ROS (Hossain et al., 2012). Inoculation of maize seedlings with S. sciuri SAT-17rif significantly increased the protein contents of maize, enabling the plants to synthesize anti-oxidative enzymes and better withstand the salt stress.
Conclusion
The salt-tolerant rhizobacterium S. sciuri strain SAT-17, characterized in the current study, exhibited substantial phosphate solubilization as well as indole-3-acetic acid production and 1-aminocyclopropane-1-carboxylic acid deaminase activity. The inoculation of maize with SAT-17 improved plant growth alongside decreasing the reactive oxygen species levels and increasing the cellular antioxidant enzyme activities (CAT, POD and proline). Moreover, the inoculation increased the uptake of N, P and K to maintain optimum nutrient, chlorophyll and protein levels in maize plants, thereby preventing drought-induced lipid peroxidation of membranes under salt stress. It has been concluded that the use of S. sciuri SAT-17 could serve as an efficient approach for enhancing crop tolerance to salinity in arid and semi-arid regions. This study opens the future directions for researchers to investigate about the genetic mechanism involved in salinity tolerance induction by S. sciuri SAT-17 in maize. The study on expression profiling of some stress-responsive genes of maize in response to S. sciuri SAT-17 inoculation might be helpful to understand about molecular cross-talk between plant and bacterial strain.
Author Contributions
MSA: designed experiments, data analysis, manuscript preparation. MT: designed experiments, manuscript preparation. MS: designed experiments, manuscript preparation, data analysis. MA: manuscript preparation, data analysis. MTJ: critical revision of manuscript, data analysis. SS: student who practically performed experiments, manuscript preparation. SR: student who practically performed experiments, manuscript preparation.
Conflict of Interest Statement
The authors declare that the research was conducted in the absence of any commercial or financial relationships that could be construed as a potential conflict of interest.
Acknowledgments
The present work was solely funded by Government College University, Faisalabad, Pakistan under annual departmental (BNB research grant) research fund. We gratefully acknowledge Dr. Qasim Ali, Assistant Professor, Department of Botany, Government College University, Faisalabad for assistance in macro- and micro-nutrient analysis through atomic absorption spectrophotometry.
Supplementary Material
The Supplementary Material for this article can be found online at: http://journal.frontiersin.org/article/10.3389/fmicb.2016.00867
References
Abou-Shanab, R. A. I., Angle, J. S., and Chaney, R. L. (2006). Bacterial inoculants affecting nickel uptake by Alyssum murale from low, moderate and high Ni soils. Soil Biol. Biochem. 38, 2882–2889. doi: 10.1016/j.soilbio.2006.04.045
Adesemoye, A., Torbert, H., and Kloepper, J. (2008). Enhanced plant nutrient use efficiency with PGPR and AMF in an integrated nutrient management system. Can. J. Microbiol. 54, 876–886. doi: 10.1139/w08-081
Aebi, H. (1984). Catalase in vitro. Methods Enzymol. 105, 121–126. doi: 10.1016/S0076-6879(84)05016-3
Ahmad, M., Zahir, Z. A., Jamil, M., Nazli, F., Latif, M., and Akhtar, M. (2014). Integrated use of plant growth promoting rhizobacteria, biogas slurry and chemical nitrogen for sustainable production of maize under salt–affected conditions. Pak. J. Bot. 46, 375–382.
Ali, Q., and Ashraf, M. (2011). Induction of drought tolerance in maize due to exogenous application of trehalose: growth, photosynthesis, water relation and oxidative defence mechanism. Agron. J. Agron. Crop Sci. 197, 258–271. doi: 10.1111/j.1439-037X.2010.00463.x
Arnon, D. I. (1949). Copper enzymes in isolated chloroplasts: polyphenoloxidase in Beta vulgaris. Plant Physiol. 24, 1–15. doi: 10.1104/pp.24.1.1
Arnon, D. I., and Hoagland, D. R. (1940). Crop production in artificial culture solution, sand and soil with special reference to factors influencing yield and absorption of inorganic nutrient. Soil Sci. 50, 463–483.
Ashraf, M. (2010). Inducing drought tolerance in plants: recent advances. Biotechnol. Adv. 28, 169–183. doi: 10.1016/j.biotechadv.2009.11.005
Ashraf, M., Hasnain, S., Berge, O., and Mahmood, T. (2004). Inoculating wheat seedlings with exopolysaccharide-producing bacteria restricts sodium uptake and stimulates plant growth under salt stress. Biol. Fertil. Soils 40, 157–162. doi: 10.1007/s00374-004-0766-y
Azooz, M. M., Ismail, A. M., and Elhamd, M. A. (2009). Growth, lipid peroxidation and antioxidant enzyme activities as a selection criterion for the salt tolerance of maize cultivars grown under salinity stress. Int. J. Agric. Biol. 11, 21–26.
Bastias, E., Alcaraz-López, C., Bonilla, I., Martínez-Ballesta, M. C., Bolaños, L., and Carvajal, M. (2010). Interactions between salinity and boron toxicity in tomato plants involve apoplastic calcium. J. Plant Physiol. 167, 54–60. doi: 10.1016/j.jplph.2009.07.014
Bates, L., Waldren, R., and Teare, I. (1973). Rapid determination of free proline for water-stress studies. Plant Soil 39, 205–207. doi: 10.1016/j.dental.2010.07.006
Batool, A., Ashraf, M., Akram, N., and Al-Qurainy, F. (2013). Salt-induced changes in the growth, key physicochemical and biochemical parameters, enzyme activities, and levels of non-enzymatic anti-oxidants in cauliflower (Brassica oleracea L.). J. Hortic. Sci. Biotechnol. 88, 231–241. doi: 10.1080/14620316.2013.11512961
Benizri, E., Baudoin, E., and Guckert, A. (2001). Root colonization by inoculated plant growth-promoting rhizobacteria. Biocontrol. Sci. Technol. 11, 557–574. doi: 10.1080/09583150120076120
Bhattacharyya, P. N., and Jha, D. K. (2012). Plant growth-promoting rhizobacteria (PGPR): emergence in agriculture. World J. Microbiol. Biotechnol. 28, 1327–1350. doi: 10.1007/s11274-011-0979-9
Bradford, M. M. (1976). A rapid and sensitive method for the quantitation of microgram quantities of protein utilizing the principle of protein-dye binding. Anal. Biochem. 72, 248–254. doi: 10.1016/0003-2697(76)90527-3
Chance, B., and Maehly, A. (1955). Assay of catalases and peroxidases. Methods Enzymol. 2, 764–775. doi: 10.1016/S0076-6879(55)02300-8
Cha-Um, S., and Kirdmanee, C. (2009). Effect of salt stress on proline accumulation, photosynthetic ability and growth characters in two maize cultivars. Pak. J. Bot. 41, 87–98.
Combes-Meynet, E., Pothier, J. F., Moënne-Loccoz, Y., and Prigent-Combaret, C. (2011). The Pseudomonas secondary metabolite 2, 4-diacetylphloroglucinol is a signal inducing rhizoplane expression of Azospirillum genes involved in plant-growth promotion. Mol. Plant-Microbe Interact. 24, 271–284. doi: 10.1094/MPMI-07-10-0148
Couillerot, O., Combes-Meynet, E., Pothier, J. F., Bellvert, F., Challita, E., Poirier, M.-A., et al. (2011). The role of the antimicrobial compound 2, 4-diacetylphloroglucinol in the impact of biocontrol Pseudomonas fluorescens F113 on Azospirillum brasilense phytostimulators. Microbiology 157, 1694–1705. doi: 10.1099/mic.0.043943-0
Cui, Y., and Wang, Q. (2006). Physiological responses of maize to elemental sulphur and cadmium stress. Plant Soil Environ. 52, 523–529.
del Amor, F. M., and Cuadra-Crespo, P. (2012). Plant growth-promoting bacteria as a tool to improve salinity tolerance in sweet pepper. Funct. Plant Biol. 39, 82–90. doi: 10.1071/FP11173
Dodd, I. C., and Pérez-Alfocea, F. (2012). Microbial amelioration of crop salinity stress. J. Exp. Bot. 63, 3415–3428. doi: 10.1093/jxb/ers033
Duponnois, R., Colombet, A., Hien, V., and Thioulouse, J. (2005). The mycorrhizal fungus Glomus intraradices and rock phosphate amendment influence plant growth and microbial activity in the rhizosphere of Acacia holosericea. Soil Biol. Biochem. 37, 1460–1468. doi: 10.1016/j.soilbio.2004.09.016
Fischer, S. E., Jofré, E. C., Cordero, P. V., Gutiérrez Mañero, F. J., and Mori, G. B. (2010). Survival of native Pseudomonas in soil and wheat rhizosphere and antagonist activity against plant pathogenic fungi. Antonie Leeuwenhoek 97, 241–251. doi: 10.1007/s10482-009-9405-9
Flowers, T. (2004). Improving crop salt tolerance. J. Exp. Bot. 55, 307–319. doi: 10.1093/jxb/erh003
Gadd, G. M., and Griffith, A. J. (1978). Microorganisms and heavy metal toxicity. Microb. Ecol. 4, 303–317. doi: 10.1007/BF02013274
Ghafoor, A., Qadir, M., and Murtaza, G. (2002). Agriculture in the Indus plains: sustainability of land and water resources: a review. Int. J. Agric. Biol. 4, 428–437.
Glick, B. R., Todorovic, B., Czarny, J., Cheng, Z., Duan, J., and McConkey, B. (2007). Promotion of plant growth by bacterial ACC deaminase. Crit. Rev. Plant Sci. 26, 227–242. doi: 10.1080/07352680701572966
Gordon, S. A., and Weber, R. P. (1951). Colorimetric estimation of indoleacetic acid. Plant Physiol. 26, 192–195. doi: 10.1104/pp.26.1.192
Grattan, S. R., and Grieve, C. M. (1999). “Mineral nutrient acquisition and responses by plants grown in saline environments,” in Handbook of Plant and Crop Stress, 2nd Edn, ed. M. Pessarakli (Boca Raton: CRC Press), 203–229.
Hanif, K., Hameed, S., Imran, A., Naqqash, T., Shahid, M., and Van Elsas, J. D. (2015). Isolation and characterization of a β-propeller gene containing phosphobacterium Bacillus subtilis strain KPS-11 for growth promotion of potato (Solanum tuberosum L.). Front. Microbiol. 6:583. doi: 10.3389/fmicb.2015.00583
He, Z., Zhang, M., Calvert, D., Stoffella, P., and Li, Y. (2003). “Loading of phosphorus in surface runoff in relation to management practices and soil properties,” in Proceedings - Soil and Crop Science Society of Florida, (Florida, FL: Soil and Crop Science Society Florida), 12–20.
Heath, R. L., and Packer, L. (1968). Photoperoxidation in isolated chloroplasts: I. Kinetics and stoichiometry of fatty acid peroxidation. Arch. Biochem. Biophys. 125, 189–198. doi: 10.1016/0003-9861(68)90654-1
Hossain, M. A., Piyatida, P., Jaime, A., Silva, T. D., and Fujita, M. (2012). Molecular mechanism of heavy metal toxicity and tolerance in plants: central role of glutathione in detoxification of reactive oxygen species and methylglyoxal and in heavy metal chelation. J. Bot. 13, 872–875.
Islam, F., Yasmeen, T., Arif, M. S., Ali, S., Ali, B., Hameed, S., et al. (2015). Plant growth promoting bacteria confer salt tolerance in Vigna radiata by up-regulating antioxidant defense and biological soil fertility. Plant Growth Regul. 42, 565–572. doi: 10.1007/s10725-10015-10142-y
Julkunen-Tiitto, R. (1985). Phenolic constituents in the leaves of northern willows: methods for the analysis of certain phenolics. J. Agric. Food Chem. 33, 213–217. doi: 10.1021/jf00062a013
Karlidag, H., Yildirim, E., Turan, M., Pehluvan, M., and Donmez, F. (2013). Plant growth promoting rhizobacteria mitigate deleterious effects of salt stress on strawberry plants (Fragaria ananassa). Hortic. Sci. 48, 563–567.
Khan, M. U., Sessitsch, A., Harris, M., Fatima, K., Imran, A., Arslan, M., et al. (2015). Cr-resistant rhizo-and endophytic bacteria associated with Prosopis juliflora and their potential as phytoremediation enhancing agents in metal-degraded soils. Front. Plant Sci. 5:755. doi: 10.3389/fpls.2014.00755
Kim, K., Jang, Y.-J., Lee, S. M., Oh, B.-T., Chae, J.-C., and Lee, K. J. (2014). Alleviation of salt stress by Enterobacter sp. EJ01 in tomato and Arabidopsis is accompanied by up-regulation of conserved salinity responsive factors in plants. Mol. Cells 37, 109–117. doi: 10.14348/molcells.2014.2239
Kravchik, M., and Bernstein, N. (2013). Effects of salinity on the transcriptome of growing maize leaf cells point at cell-age specificity in the involvement of the antioxidative response in cell growth restriction. BMC Genomics 14:24. doi: 10.1186/1471-2164-14-24
Kumar, R., Goyal, V., and Kuhad, M. S. (2005). Influence of fertility-salinity interactions on growth, water status and yield of Indian mustard (Brassica Juncea). Indian J. Plant Physiol. 10, 139–144.
Mahajan, S., and Tuteja, N. (2005). Cold salinity and drought stresses an overview. Arch. Biochem. Biophys. 444, 139–158. doi: 10.1016/j.abb.2005.10.018
Maniatis, T., Fritsch, E. F., and Sambrook, J. (1982). Molecular Cloning: A Laboratory Manual. New York, NY: Cold Spring Harbor Laboratory, 45.
Masood, S., Saleh, L., Witzel, K., Plieth, C., and Mühling, K. H. (2012). Determination of oxidative stress in wheat leaves as influenced by boron toxicity and NaCl stress. Plant Physiol. Biochem. 56, 56–61. doi: 10.1016/j.plaphy.2012.04.011
Mayak, S., Tirosh, T., and Glick, B. R. (2004). Plant growth-promoting bacteria that confer resistance to water stress in tomatoes and peppers. Plant Sci. 166, 525–530. doi: 10.1016/j.plantsci.2003.10.025
Mohamed, H. I., and Gomaa, E. Z. (2012). Effect of plant growth promoting Bacillus subtilis and Pseudomonas fluorescens on growth and pigment composition of radish plants (Raphanus sativus) under NaCl stress. Photosynthetica 50, 263–272. doi: 10.1007/s11099-012-0032-8
Mullen, M. D. (2005). Phosphorus in soils: biological interactions. Encycl. Soils Environ. 3, 210–215. doi: 10.1016/B0-12-348530-4/00161-2
Murphy, J., and Riley, J. P. (1962). Modified solution method for determination of phosphate in natural water. Anal. Chim. Acta 27, 31–36. doi: 10.1016/S0003-2670(00)88444-5
Nanjani, S. G., and Soni, H. P. (2014). Characterization of an extremely halotolerant Staphylococcus arlettae HPSSN35C isolated from Dwarka Beach. India. J. Basic Microbiol. 53, 1–8.
Nasraoui-Hajaji, A., Gouia, H., Carrayol, E., and Haouari-Chaffei, C. (2012). Ammonium alleviates redox state in solanum seedlings under cadmium stress conditions. J. Environ. Anal. Toxicol. 2, 116–120.
Nemeghaire, S., Argudín, M. A., Feßler, A. T., Hauschild, T., Schwarz, S., and Butaye, P. (2014). The ecological importance of the Staphylococcus sciuri species group as a reservoir for resistance and virulence genes. Vet. Microbiol. 171, 342–356. doi: 10.1016/j.vetmic.2014.02.005
Oliveira, C. A., Alves, V. M. C., Marriel, I. E., Gomes, E. A., Scotti, M. R., Carneiro, N. P., et al. (2009). Phosphate solubilizing microorganisms isolated from rhizosphere of maize cultivated in an oxisol of the Brazilian Cerrado Biome. Soil Biol. Biochem. 41, 1782–1787. doi: 10.1016/j.soilbio.2008.01.012
Paul, D., and Lade, H. (2014). Plant-growth-promoting rhizobacteria to improve crop growth in saline soils: a review. Agron. Sustain. Dev. 34, 737–752. doi: 10.1007/s13593-014-0233-6
Penrose, D. M., and Glick, B. R. (2003). Methods for isolating and characterizing ACC deaminase-containing plant growth-promoting rhizobacteria. Physiol. Plant 118, 10–15. doi: 10.1034/j.1399-3054.2003.00086.x
Pérez-Alfocea, F., Albacete, A., Ghanem, M. E., and Dodd, I. C. (2010). Hormonal regulation of source–sink relations to maintain crop productivity under salinity: a case study of root-to-shoot signalling in tomato. Funct. Plant Biol. 37, 592–603. doi: 10.1071/FP10012
Pikovskaya, R. I. (1948). Metabolism of phosphorous in soil in connection with vital activity of some microbial species. Microbiologia 17, 362–370.
Rahayu, Y. S., Walch-Liu, P., Neumann, G., Römheld, V., von Wirén, N., and Bangerth, F. (2005). Root-derived cytokinins as long-distance signals for NO3–induced stimulation of leaf growth. J. Exp. Bot. 56, 1143–1152. doi: 10.1093/jxb/eri107
Rajput, L., Imran, A., Mubeen, F., and Hafeez, F. Y. (2013). Salt-tolerant PGPR strain Planococcus rifietoensis promotes the growth and yield of wheat (Triticum aestivum L.) cultivated in saline soil. Pak. J. Bot. 45, 1955–1962.
Roohi, A., Ahmed, I., Iqbal, M., and Jamil, M. (2012). Preliminary isolation and characterization of halotolerant and halophilic bacteria from salt mines of Karak, Pakistan. Pak. J. Bot. 44, 365–370.
Sagar, S., Dwivedi, A., Yadav, S., Tripathi, M., and Kaistha, S. D. (2012). Hexavalent chromium reduction and plant growth promotion by Staphylococcus arlettae strain Cr11. Chemosphere 86, 847–852. doi: 10.1016/j.chemosphere.2011.11.031
Sahoo, R. K., Ansari, M. W., Pradhan, M., Dangar, T. K., Mohanty, S., and Tuteja, N. (2014). A novel Azotobacter vinellandii (SRI Az 3) functions in salinity stress tolerance in rice. Plant Signal. Behav. 9, 511–523. doi: 10.4161/psb.29377
Savci, S. (2012). An agricultural pollutant: chemical fertilizer. Int. J. Environ. Sci. Dev. 3, 77–80.
Shahid, M., Hameed, S., Imran, A., Ali, S., and van Elsas, J. D. (2012). Root colonization and growth promotion of sunflower (Helianthus annuus L.) by phosphate solubilizing Enterobacter sp. Fs-11. World J. Microbiol. Biotechnol. 28, 2749–2758. doi: 10.1007/s11274-012-1086-2
Shahid, M., Hameed, S., Tariq, M., Zafar, M., Ali, A., and Ahmad, N. (2015). Characterization of mineral phosphate-solubilizing bacteria for enhanced sunflower growth and yield-attributing traits. Ann. Microbiol. 65, 1525–1536. doi: 10.1007/s13213-014-0991-z
Shahid, M., Hussain, B., Riaz, D., Khurshid, M., Ismail, M., and Tariq, M. (2016). Identification and partial characterization of potential probiotic lactic acid bacteria in freshwater Labeo rohita and Cirrhinus mrigala. Aquac. Res. doi: 10.1111/are.13006
Sharpley, A. N. (1999). Agricultural Phosphorus and Eutrophication, 2nd Edn. Washington, DC: Agricultural Research Service, 149.
Sheldon, A., Menzies, N., So, H. B., and Dalal, R. (2004). “The effect of salinity on plant available water,” in Supersoil 2004: Proceedings for the 3rd Australian New Zealand Soils Conference, ed. B. Singh (Sydney, NSW: The Regional Institute), 1–5.
Sheng, M., Tang, M., Zhang, F., and Huang, Y. (2011). Influence of arbuscular Mycorrhiza on organic solutes in maize leaves under salt stress. Mycorrhiza 21, 423–430. doi: 10.1007/s00572-010-0353-z
Shigeoka, S., Ishikawa, T., Tamoi, M., Miyagawa, Y., Tekeda, T., Yabuta, Y., et al. (2002). Regulation and function of ascorbate peroxidase isoenzymes. J. Exp. Bot. 53, 1305–1319. doi: 10.1093/jexbot/53.372.1305
Somasegaran, P., and Hoben, H. J. (1994). Handbook for Rhizobia: Methods in Legume-Rhizobium Technology. Heidelberg, NY: Springer.
Spaepen, S., and Vanderleyden, J. (2011). Auxin and plant-microbe interactions. Cold Spring Harbor Perspect. Biol. 3:4. doi: 10.1101/cshperspect.a001438
Spaepen, S., Vanderleyden, J., and Remans, R. (2007). Indole-3-acetic acid in microbial and microorganism-plant signaling. FEMS Microbiol. Rev. 31, 425–448. doi: 10.1111/j.1574-6976.2007.00072.x
Steel, R. G. D., Torrie, J. H., and Dickey, D. A. (1997). Principles and Procedures of Statistics, A Biometrical Approach, 3rd Edn. New York, NY: McGraw Hill Book Int. Co, 172–177.
Tamura, K., Stecher, G., Peterson, D., Filipski, A., and Kumar, S. (2013). MEGA6: molecular evolutionary genetics analysis version 6.0. Mol. Biol. Evol. 30, 2725–2729. doi: 10.1093/molbev/mst197
Tank, N., and Saraf, M. (2010). Salinity-resistant plant growth promoting rhizobacteria ameliorates sodium chloride stress on tomato plants. J. Plant Interact. 5, 51–58. doi: 10.1080/17429140903125848
Trivedi, P., and Sa, T. (2008). Pseudomonas corrugata (NRRL B-30409) mutants increased phosphate solubilization, organic acid production, and plant growth at lower temperatures. Curr. Microbiol. 56, 140–144. doi: 10.1007/s00284-007-9058-8
Vincent, J. M. (1970). “A manual for the practical study of the root nodule bacteria,” in International Biological Programme, Vol. 15, (Oxford: Blackwell).
Weisburg, W. G., Barns, S. M., Pelletier, D. A., and Lane, D. J. (1991). 16S ribosomal DNA amplification for phylogenetic study. J. Bacteriol. 173, 697–703.
Wolf, B. (1982). A comprehensive system of leaf analyses and its use for diagnosing crop nutrient status. Commun. Soil Sci. Plant Anal. 13, 1035–1059. doi: 10.1080/00103628209367332
Xu, G., Magen, H., Tarchitzky, J., and Kafkafi, U. (1999). Advances in chloride nutrition of plants. Adv. Agron. 68, 97–150. doi: 10.1016/S0065-2113(08)60844-5
Yildirim, E., Karlidag, H., Turan, M., Dursun, A., and Goktepe, F. (2011). Growth, nutrient uptake, yield promotion of Broccoli by plant growth promoting rhizobacteria with manure. Hort. Sci. 46, 932–936.
Yildirim, E., Taylor, A., and Spittler, T. (2006). Ameliorative effects of biological treatments on growth of squash plants under salt stress. Sci. Hortic. 111, 1–6. doi: 10.1016/j.scienta.2006.08.003
Yildirim, E., Turan, M., and Donmez, M. F. (2008). Mitigation of salt stress in radish (Raphanus sativus L.) by plant growth promoting rhizobacteria. Roumanian Biotechnol. Lett. 13, 3933–3943.
Younesi, O., and Moradi, A. (2014). Effects of plant growth promoting rhizobacterium (PGPR) and arbuscular mycorrhizal fungus (AM) on antioxidant enzyme activities in salt stressed bean (Phaseolus vulgaris L.). Agriculture 60, 10–21.
Zaidi, A., Khan, M., Ahemad, M., and Oves, M. (2009). Plant growth promotion by phosphate solubilizing bacteria. Acta Microbiol. Immunol. Hung. 56, 263–284. doi: 10.1556/AMicr.56.2009.3.6
Zhang, H., Kim, M.-S., Sun, Y., Dowd, S. E., Shi, H., and Paré, P. W. (2008). Soil bacteria confer plant salt tolerance by tissue-specific regulation of the sodium transporter HKT1. Mol. Plant-Microbe Interact. 21, 737–744. doi: 10.1094/MPMI-21-6-0737
Zhou, W., Qin, S., Lyu, D., and Zhang, P. (2015). Soil sterilisation and plant growth-promoting rhizobacteria promote root respiration and growth of sweet cherry rootstocks. Arch. Agron. Soil. Sci. 61, 361–370. doi: 10.1080/03650340.2014.935346
Keywords: antioxidants, biofertilizer, reactive oxygen species, salinity, Staphylococcus sciuri Zea mays
Citation: Akram MS, Shahid M, Tariq M, Azeem M, Javed MT, Saleem S and Riaz S (2016) Deciphering Staphylococcus sciuri SAT-17 Mediated Anti-oxidative Defense Mechanisms and Growth Modulations in Salt Stressed Maize (Zea mays L.). Front. Microbiol. 7:867. doi: 10.3389/fmicb.2016.00867
Received: 19 February 2016; Accepted: 23 May 2016;
Published: 09 June 2016.
Edited by:
Khaled Masmoudi, Center of Biotechnology of Sfax, TunisiaReviewed by:
Biswapriya Biswavas Misra, University of Florida, USALei Zhang, Washington State University, USA
Copyright © 2016 Akram, Shahid, Tariq, Azeem, Javed, Saleem and Riaz. This is an open-access article distributed under the terms of the Creative Commons Attribution License (CC BY). The use, distribution or reproduction in other forums is permitted, provided the original author(s) or licensor are credited and that the original publication in this journal is cited, in accordance with accepted academic practice. No use, distribution or reproduction is permitted which does not comply with these terms.
*Correspondence: Muhammad Shahid, c2hhaGlkbXBnQHlhaG9vLmNvbQ==