- 1Department of Microbiology, Immunobiology and Genetics, Max F. Perutz Laboratories, University of Vienna, Vienna Biocenter, Vienna, Austria
- 2Department of Pharmaceutical Technology and Biochemistry, Faculty of Chemistry, Gdańsk University of Technology, Gdańsk, Poland
Formation of glucosamine-6-phosphate (GlcN6P) by enzyme GlcN6P synthase (GlmS) represents the first step in bacterial cell envelope synthesis. In Escherichia coli, expression of glmS is controlled by small RNAs (sRNAs) GlmY and GlmZ. GlmZ activates the glmS mRNA by base-pairing. When not required, GlmZ is bound by adapter protein RapZ and recruited to cleavage by RNase E inactivating the sRNA. The homologous sRNA GlmY activates glmS indirectly. When present at high levels, GlmY sequesters RapZ by an RNA mimicry mechanism suppressing cleavage of GlmZ. The interplay of both sRNAs is believed to adjust GlmS synthesis to the needs of the cell, i.e., to achieve GlcN6P homeostasis. Bacilysin (tetaine) and Nva-FMDP are dipeptide antibiotics that impair cell envelope synthesis by inhibition of enzyme GlmS through covalent modification. However, although taken up efficiently, these antibiotics are less active against E. coli for reasons unknown so far. Here we show that the GlmY/GlmZ circuit provides resistance. Inhibition of GlmS causes GlcN6P deprivation leading to activation of GlmY and GlmZ, which in turn trigger glmS overexpression in a dosage-dependent manner. Mutation of glmY or glmZ disables this response and renders the bacteria highly susceptible to GlmS inhibitors. Thus, E. coli compensates inhibition of GlmS by increasing its synthesis through the GlmY/GlmZ pathway. This mechanism is also operative in Salmonella indicating that it is conserved in Enterobacteriaceae possessing these sRNAs. As GlmY apparently responds to GlcN6P, co-application of a non-metabolizable GlcN6P analog may prevent activation of the sRNAs and thereby increase the bactericidal activity of GlmS inhibitors against wild-type bacteria. Initial experiments using glucosamine-6-sulfate support this possibility. Thus, GlcN6P analogs might be considered for co-application with GlmS inhibitors in combined therapy to treat infections caused by pathogenic Enterobacteriaceae.
Introduction
The continuous increase and spread of antibiotic resistance mechanisms among bacterial pathogens represents a major threat for public health. Of particular concern is the recent emergence of extended spectrum β-lactamases and carbapenemases in pathogenic Enterobacteriaceae limiting therapeutic treatment options for infections caused by these bacteria. Thus, there is an urgent need for novel therapies, which may not only include the discovery of novel antibacterial drugs, but also revision of known compounds that were previously neglected (Brown and Wright, 2016; Mühlen and Dersch, 2016). Many clinically relevant antibiotics interfere with the biochemical machinery for peptidoglycan biosynthesis (Silver, 2013; Borisova et al., 2014). However, the initial steps in this pathway collectively referred to as hexosamine pathway, have been rarely considered as drug targets. The hexosamine pathway generates UDP–N-acetyl-glucosamine (UDP–GlcNAc) and is initiated by key enzyme glucosamine-6-phosphate (GlcN6P) synthase GlmS, which synthesizes GlcN6P from L-glutamine and fructose-6-phosphate (Figure 1A; Milewski, 2002; Teplyakov et al., 2002). GlmS is essential unless exogenous amino sugars such as glucosamine (GlcN) are available in adequate amounts. GlcN can be taken up and converted to GlcN6P, thereby bypassing GlmS (Figure 1A) (Plumbridge, 2015). The GlcN concentration in human bodily fluids is too low to sustain growth of glmS mutants making GlmS essential for enteric bacteria colonizing the human host (Persiani et al., 2007; Kim et al., 2013; Bennett et al., 2016).
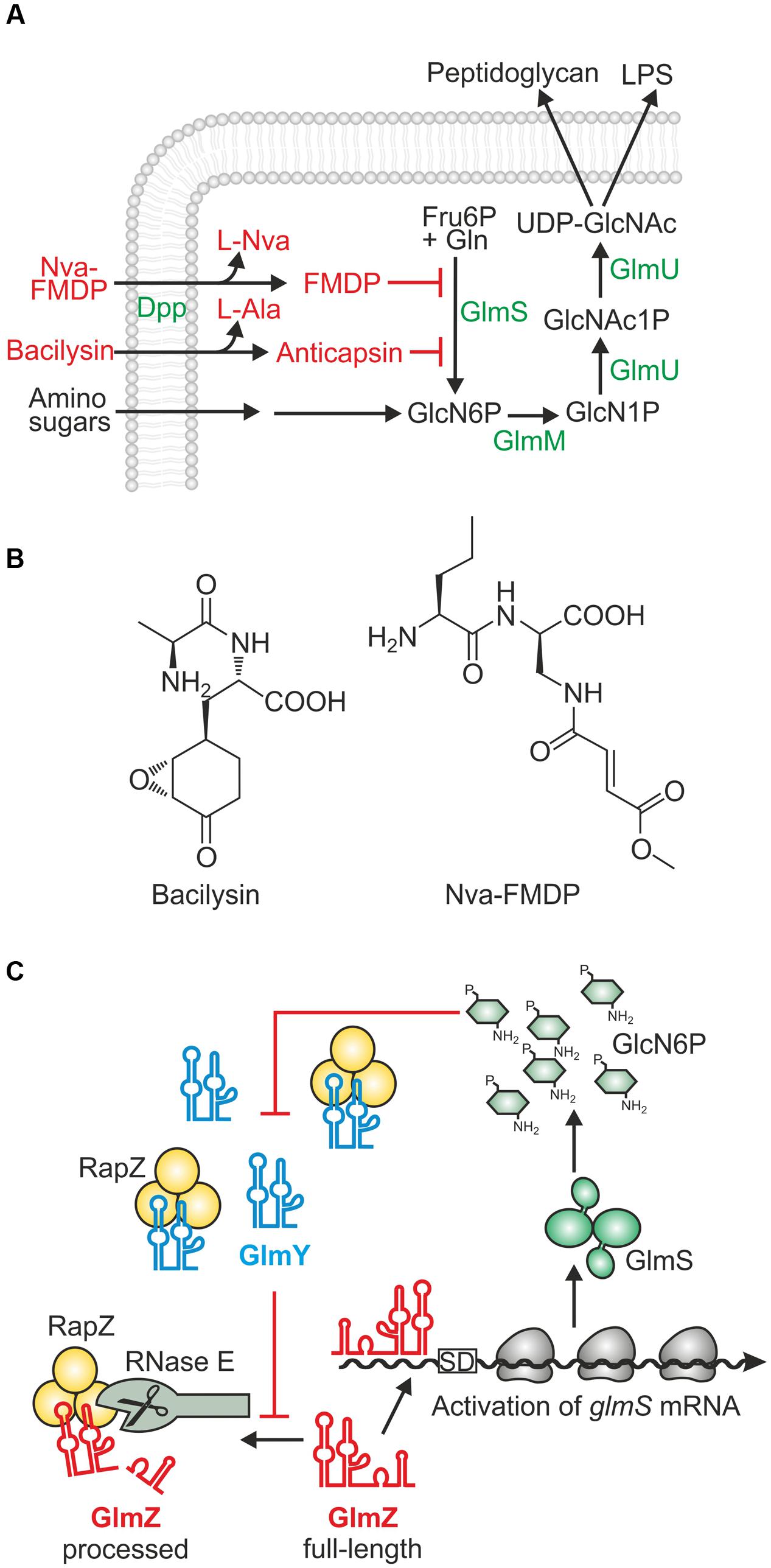
FIGURE 1. Role, regulation and inhibitors of enzyme GlmS in Escherichia coli. (A) Key role of enzyme GlmS in the hexosamine pathway and its inhibition by antibiotics. GlmS catalyzes synthesis of GlcN6P from fructose-6-phosphate and glutamine. When available, amino sugars such as GlcN can be taken up and converted to GlcN6P bypassing the need for GlmS. GlcN6P is further converted by enzymes GlmM and GlmU to UDP-GlcNAc, which is the last common precursor for synthesis of peptidoglycan and lipopolysaccharides (LPS). The dipeptide antibiotics Nva-FMDP and bacilysin require peptide transporters such as Dpp for uptake. Intracellular hydrolysis releases the active C-terminal moieties FMDP and anticapsin, which inhibit GlmS through covalent binding to a residue in the glutamine binding pocket. (B) Structures of the dipeptide antibiotics bacilysin and Nva-FMDP. (C) Feedback regulation of GlmS synthesis by small RNAs GlmY and GlmZ and adapter protein RapZ in E. coli (Göpel et al., 2013, 2016). GlmZ base-pairs with the glmS 5′-UTR enhancing translation and stabilizing the transcript. Alternatively, GlmZ is bound by adapter protein RapZ and recruited to cleavage by RNase E. The decision on the fate of GlmZ is made by the homologous decoy sRNA GlmY. Upon GlcN6P scarcity, GlmY accumulates and sequesters RapZ thereby counteracting cleavage of GlmZ by RNase E.
Several naturally produced antibiotics that inhibit GlmS enzymatic activity have been identified including bacilysin and compound A 19009 synthesized by Bacillus subtilis and Streptomyces collinus, respectively (Molloy et al., 1972; Kenig and Abraham, 1976). These antibiotics are dipeptides of exotic amino acids and rely on peptide transporters for uptake and on intracellular peptidases for release of the warhead moiety, which is anticapsin in case of bacilysin (Figures 1A,B; Milewski, 2002; Özcengiz and Ögülür, 2015). A systematic survey of synthetic derivatives related to A 19009 identified the corresponding methylester N3-(4-methoxyfumaroyl)-(S)-2,3-diaminopropanionic acid (FMDP) as most efficient and selective inhibitor of purified GlmS from various organisms including Escherichia coli and Salmonella (Chmara et al., 1986; Badet et al., 1988). Among various tested FMDP peptides, L-norvalyl-FMDP (Nva-FMDP; Figures 1A,B) exhibited the strongest growth inhibitory effect on bacteria (Andruszkiewicz et al., 1987; Chmara et al., 1998). FMDP as well as anticapsin act as glutamine analogs and covalently bind to the glutamine binding domain of GlmS causing its irreversible inhibition (Milewski et al., 1986; Kucharczyk et al., 1990). As a result, GlcN6P production is blocked leading to exhaustion of nucleotide precursors for peptidoglycan biosynthesis and ultimately to bacteriolysis. Cell death can be prevented by co-administration of amino sugars demonstrating that these antibiotics are specific for GlmS and lack off-target activity (Kenig and Abraham, 1976; Chmara et al., 1998). Nva-FMDP is highly effective against Gram-positive bacteria, but shows only weak activity against E. coli [minimal inhibitory concentration (MIC) ≥ 100 μg/ml; Andruszkiewicz et al., 1987; Chmara et al., 1998], although it is taken up rapidly and efficiently by the Dpp dipeptide ATP binding cassette (ABC) transporter (Marshall et al., 2003). So far, the reason for this weak efficacy remained mysterious.
Synthesis of GlmS is feed-back regulated by GlcN6P, thereby achieving homeostasis of this metabolite. The underlying mechanisms employ regulatory RNA elements, but differ remarkably between Gram-positive and Gram-negative bacteria. The glmS mRNA of Gram-positive species contains a ribozyme in its 5′-untranslated region (5′-UTR), which upon binding of GlcN6P triggers self-cleavage leading to down-regulation of glmS expression (Winkler et al., 2004). In contrast, E. coli and presumably most species of the Gram-negative Enterobacteriaceae employ two trans-encoded homologous small RNAs (sRNAs), GlmY and GlmZ, and adapter protein RapZ to regulate GlmS synthesis (Figure 1C) (Reichenbach et al., 2008; Urban and Vogel, 2008; Göpel et al., 2013, 2016). Assisted by RNA chaperone Hfq, GlmZ base-pairs with the 5′-UTR of the glmS transcript and stimulates translation concomitantly stabilizing the mRNA. In an alternative fate, GlmZ is bound by protein RapZ, which recruits RNase E to inactivate the sRNA through processing. The path to be taken by GlmZ is ultimately determined by the level of sRNA GlmY. GlmY accumulates when GlcN6P decreases in the cell and sequesters RapZ through molecular mimicry. As a result, GlmZ remains un-cleaved and upregulates glmS expression to replenish GlcN6P. In addition, in enterohemorrhagic E. coli GlmY and GlmZ were recruited to regulate horizontally acquired virulence genes (Gruber and Sperandio, 2014, 2015).
In the present study, we investigated the roles of GlmY and GlmZ for susceptibility to GlmS inhibitors. We show that these sRNAs provide intrinsic resistance by countervailing inhibition of GlmS with its increased synthesis. Beyond E. coli, this mechanism also operates in Salmonella indicating that it applies to a wider range of enterobacterial species in which these sRNAs are conserved. Our findings indicate that compounds suppressing GlmY and/or GlmZ would increase the efficacy of GlmS inhibitors when applied in combination. Experiments using the non-metabolizable GlcN6P analog glucosamine-6-sulfate (GlcN6SO4) are in favor of this possibility.
Materials and Methods
Bacterial Strains, Culture Conditions, and Chemicals
Bacterial strains used in this study are listed in Table 1. Derivatives of E. coli MG1655 were used to test the bacteriocidal effects of Nva-FMDP and bacilysin. In these strains the lacZ gene is deleted allowing monitoring of lacZ reporter gene fusions. General transduction by phage T4GT7 (Wilson et al., 1979) was used to introduce established ΔglmY::cat, ΔglmZ::cat alleles and the spectinomycin resistance tagged glmS’–lacZ fusion into strain S4197, respectively. The temperature-sensitive Flippase (FLP)-recombinase plasmid pCP20 was used to remove the chloramphenicol resistance genes (Datsenko and Wanner, 2000). Additional experiments were performed using Salmonella enterica serovar Typhimurium strain SL1344 and its ΔglmY and ΔglmZ derivatives JVS112 and JVS122, respectively (Papenfort et al., 2008). Bacteria were grown at 37°C in Luria-Bertani (LB) Lennox, Mueller Hinton broth (Merck), MacConkey lactose (Roth), and M9 minimal medium (Miller, 1972). M9 medium was supplemented with 1% [w/v] glucose as carbon source and in case of Salmonella strains additionally with 0.002% L-histidine. Antibiotics were added when required: chloramphenicol (15 μg/ml), spectinomycin (75 μg/ml), neomycin (4 μg/ml), erythromycin (0.5 μg/ml). Nva-FMDP was synthesized as described previously (Andruszkiewicz et al., 1987) and solved in H2O to yield a stock solution of 1 mg/ml. D-GlcN6SO4 was purchased from Santa Cruz Biotechnology. B. subtilis strain TI304, which overproduces bacilysin, was used to prepare bacilysin enriched culture supernatant as previously described (Inaoka et al., 2009). Bacilysin-free supernatant, which served as negative control, was prepared using B. subtilis strain TI398. The latter strain is unable to synthesize bacilysin due to inactivation of gene ywfE (bacD) encoding alanine–anticapsin ligase (Inaoka et al., 2009). The culture supernatants were concentrated 20-fold using a centrifugal evaporator and subsequently stored at -20°C until further use.
Large Scale Liquid Culture Experiments to Study the Effects of Nva-FMDP and Bacilysin
Large scale cultures were grown to assess the effect of Nva-FMDP and Bacilysin on cell viability and the regulatory GlmY/GlmZ/glmS circuit. To test Nva-FMDP, strains were inoculated from overnight cultures in 35 ml LB medium to an OD600 ~ 0.1 and grown until an OD600 ~ 0.3. Subsequently, cultures were split into three 10 ml subcultures that were supplemented either with 100 μg/ml Nva-FMDP, 100 μg/ml Nva-FMDP + 0.2% GlcN or with the equivalent volume of H2O (mock control), respectively. Growth was continued for 6 h and 1.5 ml samples were taken in hourly intervals for RNA extraction and to determine the OD600, CFU numbers and β-galactosidase activities. A similar setup was used to test bacilysin-containing culture supernatant but as a difference cultures were split at an OD600 ~ 0.2 and supplemented either with 1.5 ml concentrated culture supernatant of B. subtilis strains TI304 or TI398 or with H2O (mock control), respectively.
Determination of CFU Numbers
To determine CFU numbers, serial dilutions of the cultures were prepared in Mg saline (145 mM NaCl, 10 mM MgSO4) and appropriate dilutions were plated in duplicate on non-selective LB agar. Following incubation at 37°C overnight, colonies were counted from plates containing 30–300 colonies using a Stuart SC6 colony counter (Bibby Scientific).
Extraction of Total RNA and Northern Blotting
Total RNA was extracted from 1 ml samples using the RNeasy mini kit (Qiagen) according to the instructions of the manufacturer. The RNAs of interest were detected in subsequent Northern experiments using DIG labeled RNA probes that were generated by in vitro transcription using the DIG labeling kit (Roche diagnostics). The corresponding DNA templates were obtained by PCR using previously described oligonucleotide primers (Reichenbach et al., 2008, 2009). To detect sRNAs GlmY and GlmZ, 2.5 μg total RNA per lane were separated on 8% polyacrylamide gels containing 7 M urea and 1 × TBE. Subsequently, RNA was transferred to a nylon membrane (Hybond N+, GE healthcare) by electro-blotting and cross-linked by 254 nm UV radiation. RNA hybridization and detection was performed according the supplier’s instruction (Roche Diagnostics). To obtain 5S loading controls, sRNA probes were stripped by incubating the membrane 2× for 60 min in 50% formamide, 5% SDS, 50 mM Tris-HCl pH 7.5. Subsequently, the membrane was re-probed using a probe directed against the rrfD transcript. For detection of glmS mRNA, 5 μg total RNA per lane were separated by formaldehyde agarose gel electrophoresis (1% agarose, 18% formaldehyde, 20 mM MOPS, 5 mM sodium acetate, 1 mM EDTA, pH 7.0) and subsequently blotted onto a Hybond N+ nylon membrane using the VacuGene XL vacuum blotting system (Amersham Biosciences) at 80 mbar for 4 h. To obtain loading controls, the 23S and 16S rRNAs were visualized by ethidium bromide prior to blotting.
β-Galactosidase Assays
β-Galactosidase enzyme activities were determined as described previously (Miller, 1972). Enzyme activities are expressed in Miller units and represent the average of at least two measurements using independent cultures.
Disk Diffusion Assay
Disk diffusion assays (Bauer et al., 1966) were carried out on various media including Mueller Hinton, LB Lennox, MacConkey lactose and M9 agar. Cells were grown at 37°C in LB to an OD600 ~ 0.5, pelleted and re-suspended in the medium subsequently used in the assay. A volume containing ~4 × 107 cells was mixed with 4 ml thin agar layer (medium containing 7.5% agarose) that was kept molten at 44°C and subsequently spread over 15 ml solid agar base of the same medium prepared in 100 mm Petri dishes. The compounds to be tested were adsorbed onto 6 mm cellulose disks (Rotilabo), which were subsequently placed on the seeded agar surface in the Petri dishes. Growth inhibition zones were recorded following incubation for 16 h at 37°C. Assays were performed at least twice using independent cultures.
Determination of MICs
Minimal inhibitory concentrations were determined in various media according the guidelines of the European society of clinical microbiology and infectious diseases using the broth microdilution method (EUCAST, 2003). Briefly, serial dilutions of the compounds to be tested were prepared in 96 well plates (Sterilin) and subsequently inoculated with 5 × 105 CFU/ml of an overnight culture grown in the same medium as used for MIC determination. Following incubation at 37°C for 12 h growth was monitored spectrophotometrically by measuring the OD595 using a microplate reader (iMark plate reader, BioRad). MIC was defined by the lowest drug concentration preventing visible growth (OD595 < 0.1). MIC assays were performed at least twice using independent cultures.
Results
Escherichia coli Responds to Nva-FMDP by Overexpression of glmS
Nva-FMDP exerts only a moderate growth inhibitory effect on E. coli cells (Andruszkiewicz et al., 1987). Our preliminary results suggested that E. coli responds to this drug by upregulation of GlmS synthesis (Reichenbach et al., 2008). To gain further insight, we first studied the effect of Nva-FMDP at sub-MIC concentrations. To this end, we used strain Z854, a derivative of E. coli K-12 reference strain MG1655 carrying an ectopic glmS’–lacZ fusion on the chromosome. Strain Z854 was grown in LB Lennox supplemented with either 100 μg/ml Nva-FMDP, or 100 μg/ml Nva-FMDP + 0.2% GlcN or with the corresponding volume of water (mock control). OD600 readings detected no growth impairment by Nva-FMDP (Supplementary Figure S1A). However, the CFU number was diminished ~twofold as compared to the mock control (Figure 2A). Addition of GlcN cured this growth defect, corroborating that Nva-FMDP causes cell death by depletion of intracellular GlcN6P resulting from GlmS inhibition. To determine glmS expression levels, total RNA was extracted from cells harvested during the first 3 h of growth and analyzed by Northern blotting. In parallel, the β-galactosidase activities were determined. Both assays consistently detected a strong upregulation of glmS expression in the Nva-FMDP treated cells, which was suppressed by the addition of GlcN (Figures 2B, bottom panel and 4B, columns left). To determine whether GlmY and GlmZ are involved in upregulation of glmS expression in response to Nva-FMDP, we performed Northern blotting. Indeed, high levels of GlmY and of full-length GlmZ were observed in the Nva-FMDP treated cells but not in the other samples (Figure 2B).
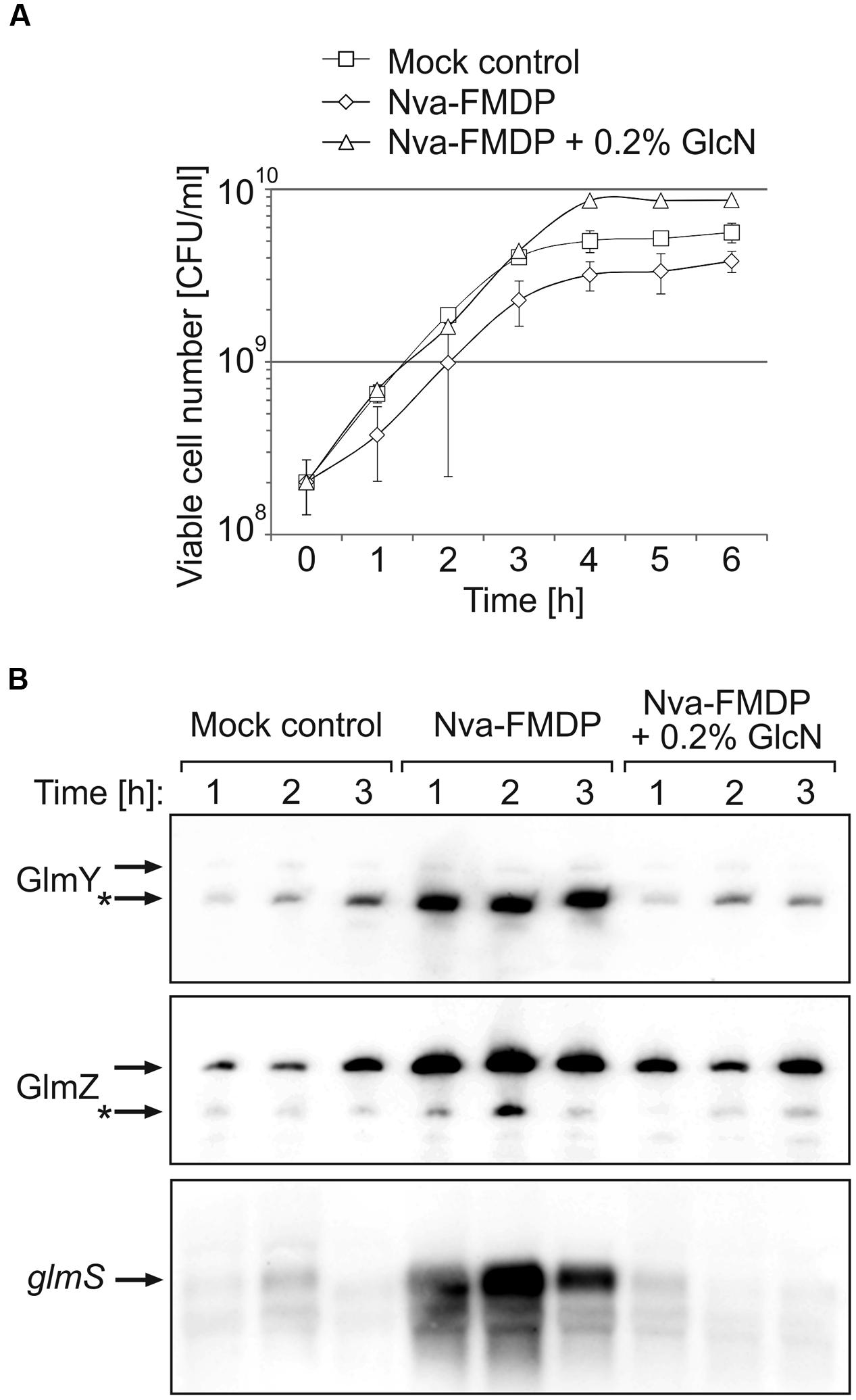
FIGURE 2. Nva-FMDP triggers accumulation of GlmY, GlmZ and glmS RNAs in E. coli and co-administration of GlcN suppresses this effect. (A) CFU numbers of cultures of E. coli strain Z854 grown in LB supplemented either with 100 μg/ml Nva-FMDP (diamonds) or 100 μg/ml Nva-FMDP + 0.2% glucosamine (triangles) or the equivalent volume of H2O (mock control; squares). The corresponding OD600 readings are provided in Supplementary Figure S1A. (B) Northern analysis of GlmY, GlmZ, and glmS RNAs in the cultures analyzed in (A). Total RNA was extracted from samples harvested at hourly intervals during the first 3 h after inoculation and subsequently probed for GlmY (top panel), GlmZ (medium panel) and glmS RNAs (bottom panel). Loading controls are provided in Supplementary Figure S1B. The various RNA species are indicated by arrows. Processed variants are denoted by asterisks.
Activation of the GlmY/GlmZ/glmS Regulatory Cascade Is Not Restricted to Nva-FMDP But Applies to GlmS Inhibitors in General
Next, we determined whether upregulation of the GlmY/GlmZ/glmS regulatory cascade is specific to Nva-FMDP or may also apply to other GlmS inhibitors such as bacilysin. Since bacilysin is commercially unavailable, we used concentrated culture supernatant of B. subtilis strain TI304, which overproduces and secretes bacilysin into the medium (Inaoka et al., 2009). Culture supernatant of the bacilysin-negative B. subtilis mutant TI398 served as negative control. E. coli strain Z854 was grown in LB Lennox supplemented with supernatant of B. subtilis strain TI304 or TI398 or with water (mock control). Presence of the bacilysin containing supernatant resulted in slight growth retardation, which was not observable in the culture treated with supernatant of the bacilysin-negative mutant (Figure 3A). This observation indicated that the growth defect was specifically caused by bacilysin present in the culture supernatant of TI304. Northern blot analysis of RNA extracted from samples harvested at various times detected accumulation of the glmS transcript and of the GlmY/GlmZ sRNAs in cells treated with supernatant of TI304, similar to cells treated with Nva-FMDP, which was tested in parallel (Figure 3B). Upregulation of glmS in response to the bacilysin-containing culture supernatant was also confirmed by β-galactosidase assays (Figure 3C, columns left). In contrast, the culture supernatant of strain TI398 had no effect on GlmY, GlmZ, and glmS as judged from comparison with the mock control (Figures 3B,C). Taken together, the data indicate that activation of the GlmY/GlmZ system and overexpression of glmS is not a specific response to Nva-FMDP but applies to GlmS inhibitors in general.
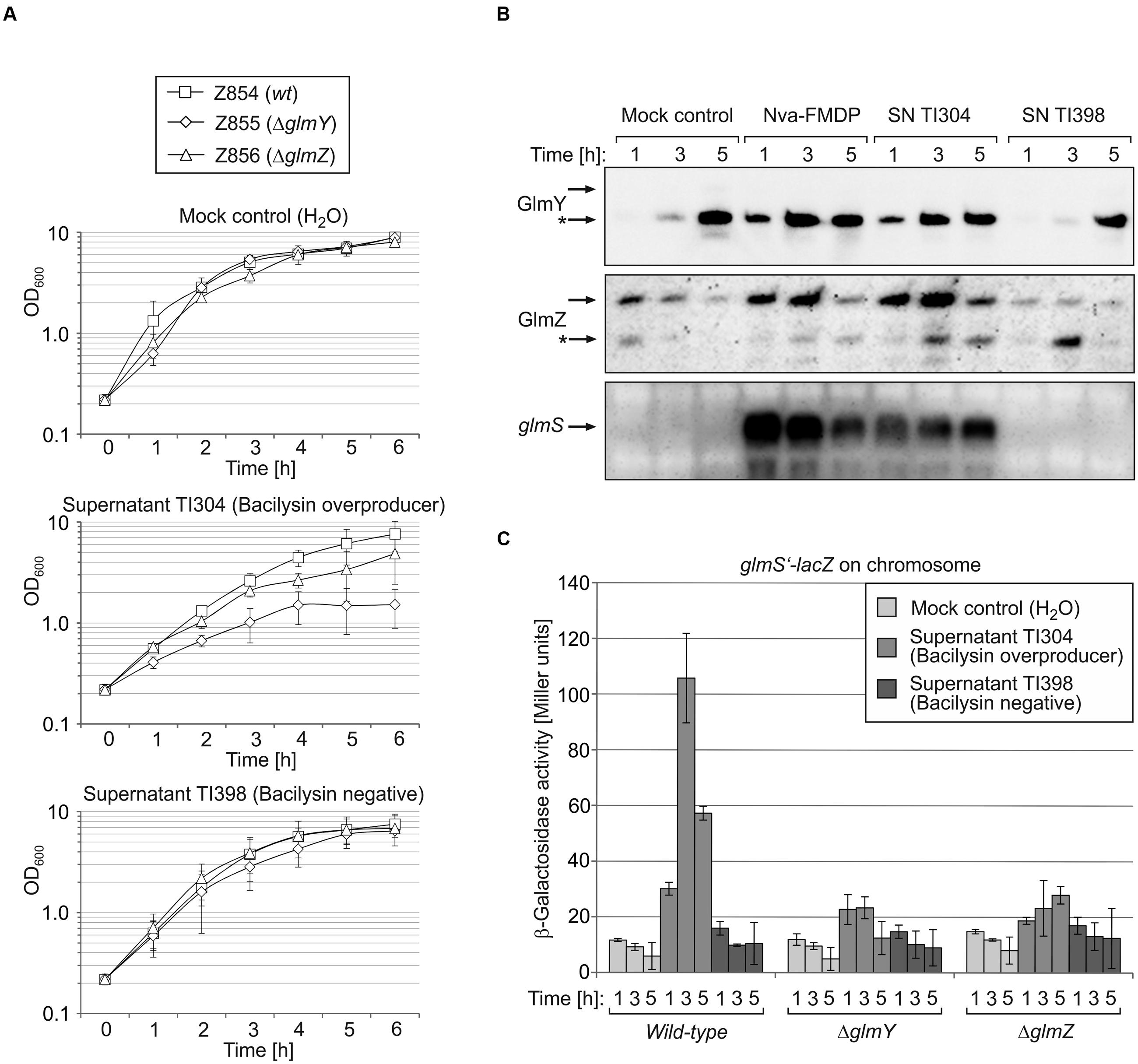
FIGURE 3. Bacilysin causes accumulation of GlmY, GlmZ and glmS RNAs in E. coli recapitulating the effects of Nva-FMDP. E. coli strains Z854 (wild-type), Z855 (ΔglmY) and Z856 (ΔglmZ) were grown in LB supplemented either with culture supernatant (SN) of B. subtilis strain TI304 overproducing bacilysin or with SN derived from the bacilysin-negative B. subtilis mutant TI398. Cultures treated with the equivalent volume of H2O served as mock control. (A) Growth curves of the various cultures as determined by OD600 recording. (B) Northern blot analysis for detection of GlmY, GlmZ and glmS in the cultures of E. coli wild-type strain Z854. Samples for isolation of total RNA were harvested 1, 3, and 5 h after inoculation of the cultures. For comparison, a culture of strain Z854 treated with Nva-FMDP was analyzed in parallel. Loading controls are provided in Supplementary Figure S2. It should be noted that expression of glmY is generally upregulated in E. coli when entering the stationary phase (Göpel et al., 2013) explaining accumulation of GlmY in the mock control culture following 5 h of growth. However, the high GlmY levels are not transduced to glmS due to the low expression levels of GlmZ under these conditions. (C) β-Galactosidase assays monitoring expression of a glmS’–lacZ reporter fusion in the various cultures. Enzyme activities were determined from samples harvested after 1, 3, and 5 h of growth.
E. coli Mutants Lacking GlmY or GlmZ Fail to Upregulate glmS in Response to GlmS Inhibitors
Northern analyses showed that Nva-FMDP and bacilysin trigger accumulation of sRNAs GlmY and GlmZ concomitantly with the glmS mRNA and additional presence of GlcN suppressed this effect (Figures 2B and 3B). This observation suggested that intracellular depletion of GlcN6P caused by GlmS inhibition led to activation of the GlmY/GlmZ sRNAs, which in turn stimulated glmS expression. To prove this hypothesis, we studied the effect of Nva-FMDP on isogenic E. coli ΔglmY and ΔglmZ mutants, respectively. The strains were grown in the absence or presence of 100 μg/ml Nva-FMDP and samples were harvested at hourly intervals and analyzed. Northern blot analysis as well as determination of β-galactosidase activities revealed that both mutants failed to activate glmS expression in response to Nva-FMDP, whereas glmS strongly accumulated in the wild-type strain as observed before (Figures 4B,C). In the ΔglmY mutant treated with Nva-FMDP, stabilization of the full-length variant of GlmZ did not occur, explaining the inability to activate glmS (Figure 4C, middle). Vice versa, GlmY still accumulated in the ΔglmZ mutant upon exposure to Nva-FMDP, but remained without effect on glmS, which is explained by the absence of GlmZ (Figure 4C, right). As judged from glmS’–lacZ fusion data, the ΔglmY and ΔglmZ mutants also failed to activate glmS in response to bacilysin (Figure 3C). Therefore, these data show that antibiotics inhibiting GlmS induce glmS overexpression through activation of the GlmY/GlmZ regulatory sRNA cascade. Interestingly, growth monitoring suggested that both sRNA mutants are more susceptible to growth inhibition by Nva-FMDP and bacilysin as compared to the wild-type (Figures 3A and 4A).
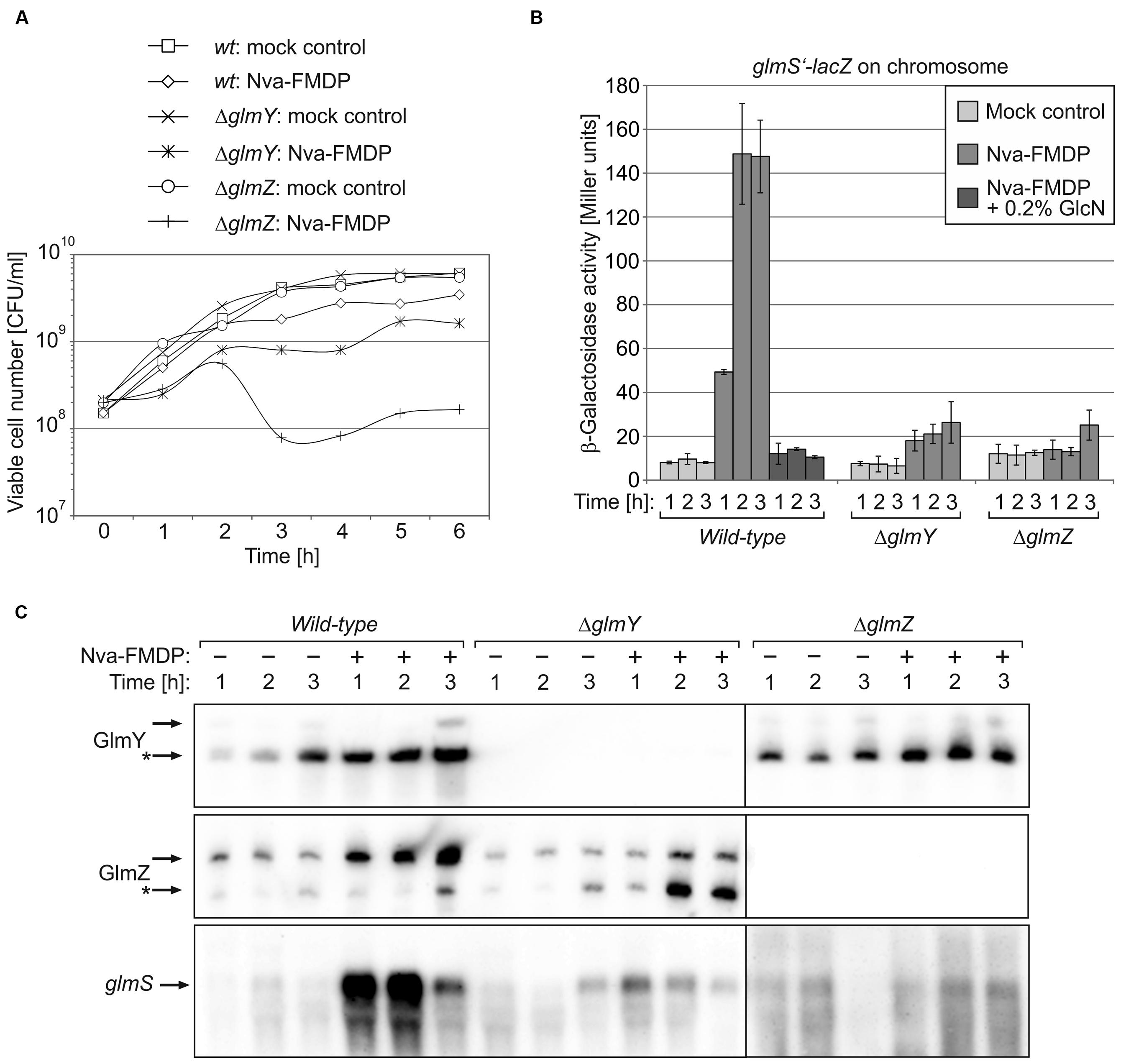
FIGURE 4. Escherichia coli mutants lacking GlmY or GlmZ do not respond to Nva-FMDP by upregulation of glmS mRNA levels. E. coli strains Z854 (wild-type), Z855 (ΔglmY) and Z856 (ΔglmZ) were grown in LB supplemented either with Nva-FMDP or the equivalent volume of water (mock control), respectively. (A) CFU numbers of the various cultures. (B) β-Galactosidase assays monitoring expression of a glmS’–lacZ reporter fusion in the various cultures during the first 3 h following inoculation. (C) Northern analysis for detection of GlmY, GlmZ and glmS RNAs in the various cultures. For details, see legend of Figure 2B. Loading controls are provided in Supplementary Figure S3.
Mutation of GlmY or GlmZ Attenuates Intrinsic Resistance of E. coli to Nva-FMDP
To investigate the possibly higher sensitivity of ΔglmY and ΔglmZ mutants to GlmS inhibitors, we first studied the effect of Nva-FMDP more thoroughly using the broth microdilution method. Serial dilutions of Nva-FMDP in LB medium were inoculated with the wild-type strain and the ΔglmY and ΔglmZ mutants, respectively. Following 12 h incubation, the OD595 of the cultures and the β-galactosidase activities were determined. Growth of the wild-type strain was slightly impaired only at highest Nva-FMDP concentrations (Figure 5A; MIC > 500 μg/ml). In contrast, growth of the mutant strains was completely inhibited at Nva-FMDP concentrations above 62.5 μg/ml. This higher susceptibility of the mutants can be attributed to the incapability to increase glmS expression as indicated by constantly low glmS’–lacZ expression levels regardless of the applied Nva-FMDP concentration (Figure 5B). In contrast, the wild-type strain exhibited glmS’–lacZ expression levels that gradually increased with incremental Nva-FMDP concentrations (Figure 5B). Thus, GlmY and GlmZ enable E. coli to activate glmS expression in a dosage-dependent manner, thereby overcoming inhibition of GlmS enzymatic activity by Nva-FMDP.
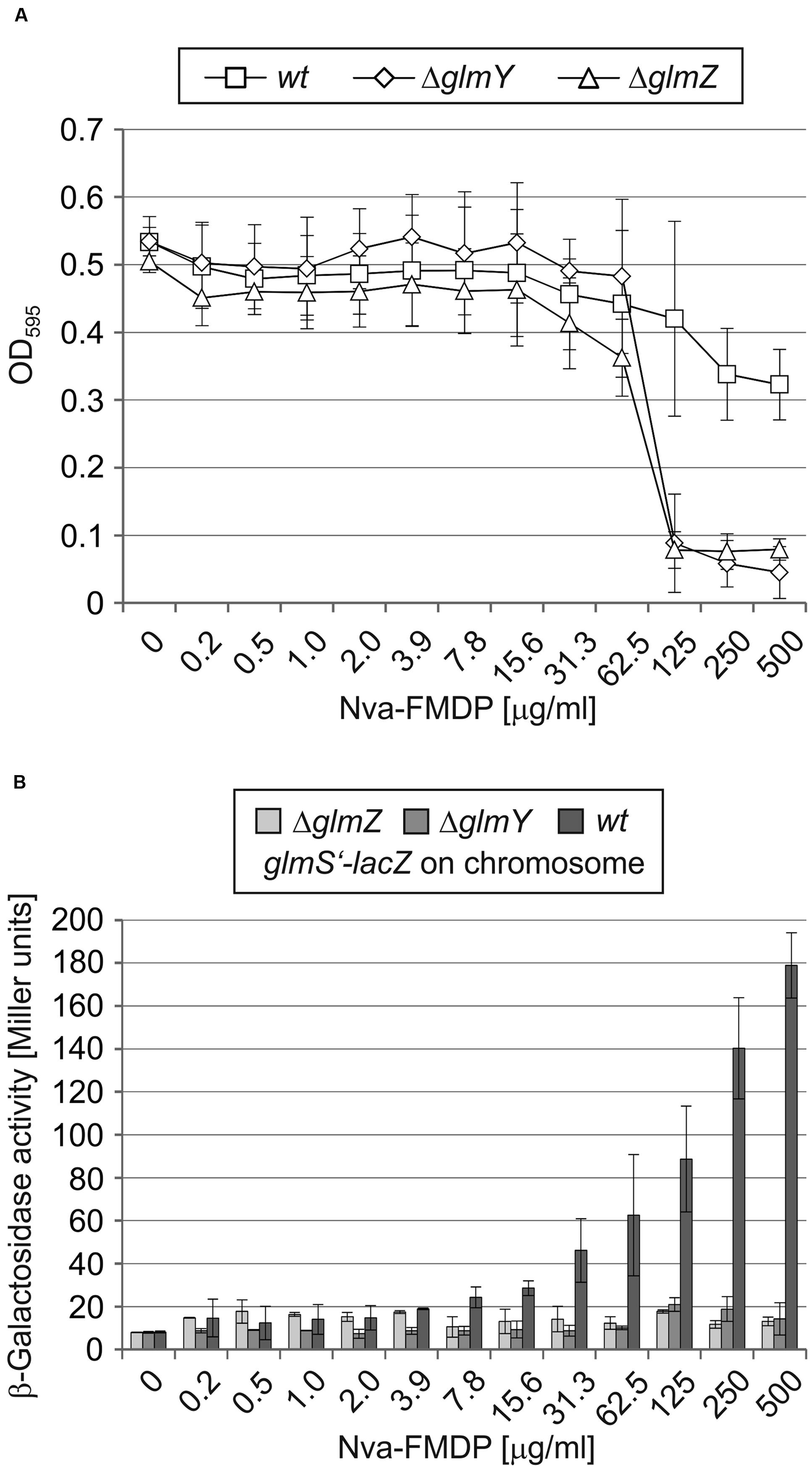
FIGURE 5. GlmY and GlmZ enable E. coli to resist the bactericidal effect of Nva-FMDP by upregulation of glmS expression in a dosage-dependent manner. Serial dilutions of Nva-FMDP in LB medium were inoculated with 5 × 105 CFU/ml of E. coli strains Z854 (wild-type), Z855 (ΔglmY) and Z856 (ΔglmZ), respectively. (A) Cell densities of the cultures as determined by OD595 measurements after 12 h incubation. (B) β-Galactosidase activities monitoring expression of a chromosomal glmS’–lacZ fusion in the various cultures.
Next, we characterized susceptibility of the various E. coli strains to Nva-FMDP more systematically. To this end, we performed disk diffusion assays and MIC determinations in various media (Tables 2 and 3; Supplementary Figures S4A,B). Nva-FMDP showed no significant activity against the wild-type strain when grown in LB or Mueller Hinton medium. However, reasonable activity was detectable when the bacteria grew in MacConkey or M9 minimal medium, indicating that the antibacterial activity of Nva-FMDP is modulated by composition of the growth medium. In contrast to the wild-type, the ΔglmY and ΔglmZ mutants were growth-inhibited by Nva-FMDP in all media. Larger inhibition zones as compared to the wild-type were observed on the various disk diffusion plates and MIC values of the mutants were roughly fourfold lower demonstrating that absence of GlmY or GlmZ increases susceptibility to Nva-FMDP significantly (Tables 2 and 3, Supplementary Figure S4A,B). Again, susceptibility of the mutants to Nva-FMDP varied with the growth medium increasing in the order Mueller Hinton < LB < MacConkey lactose < M9 minimal medium. Co-administration of GlcN abolished growth inhibition by Nva-FMDP on the disk diffusion plates, regardless whether GlcN was included in the growth medium or directly administered on the filter disk (Supplementary Figures S4B,D), re-emphasizing that Nva-FMDP acts via depletion of metabolites of the amino sugar pathway.
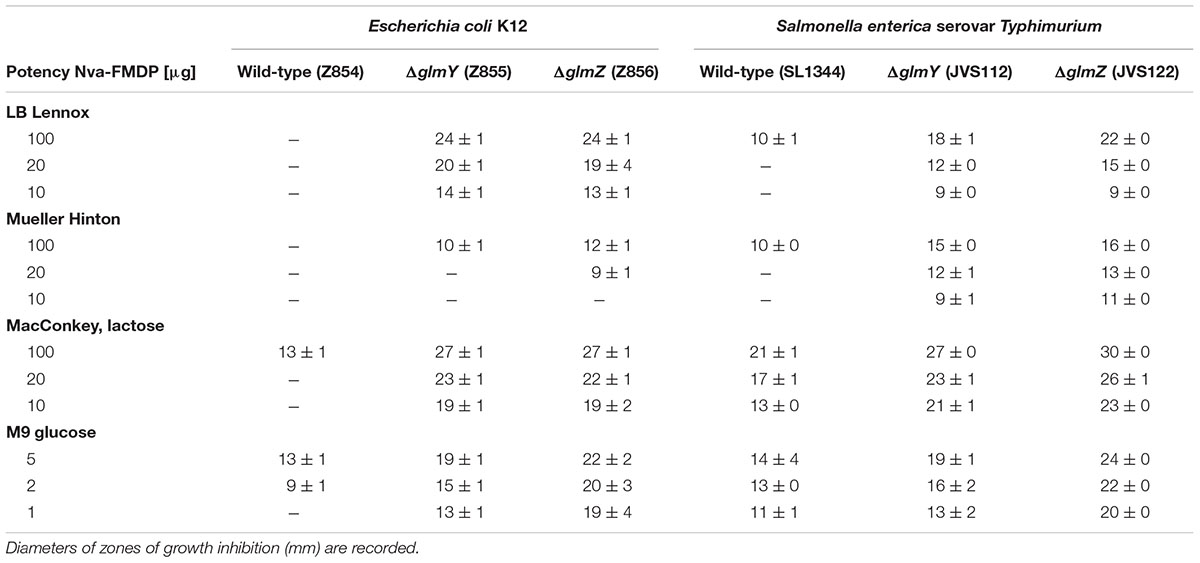
TABLE 2. Disk diffusion assays addressing susceptibility of Escherichia coli and Salmonella strains to Nva-FMDP on various media.

TABLE 3. Susceptibility [minimal inhibitory concentration (MIC) in μg/ml] of E. coli and Salmonella strains to Nva-FMDP in various growth media.
The Mechanism of Intrinsic Resistance to GlmS Inhibitors Procured by GlmY and GlmZ Is Also Operative in Salmonella
GlmY and GlmZ are conserved in Enterobacteriaceae and sequence analysis suggests that this even applies to GlmZ/glmS base-pairing (Fröhlich and Vogel, 2009; Göpel et al., 2011). This raises the possibility that GlmY and GlmZ may also bestow other Enterobacteriaceae with intrinsic resistance to GlmS inhibitors. To test this hypothesis, we investigated the effect of Nva-FMDP on the virulent Salmonella enterica serovar Typhimurium strain SL1344 and its isogenic ΔglmY and ΔglmZ mutants. First, we repeated the experiment shown in Figure 2, but used the Salmonella wild-type strain rather than E. coli. Indeed, Nva-FMDP elicited concomitant accumulation of GlmY and of the full-length variant of GlmZ (Figure 6B, top and medium panel). As expected, this resulted in upregulation of glmS mRNA (Figure 6B, bottom panel). The additional presence of GlcN suppressed accumulation of the various RNAs, recapitulating the observations in E. coli (compare Figures 2B and 6B). Nva-FMDP had a significant negative effect on the viable cell number (Figure 6A), although this was not reflected by the OD600 readings (Supplementary Figure S5A), as observed for E. coli (Figure 2A; Supplementary Figure S1A).
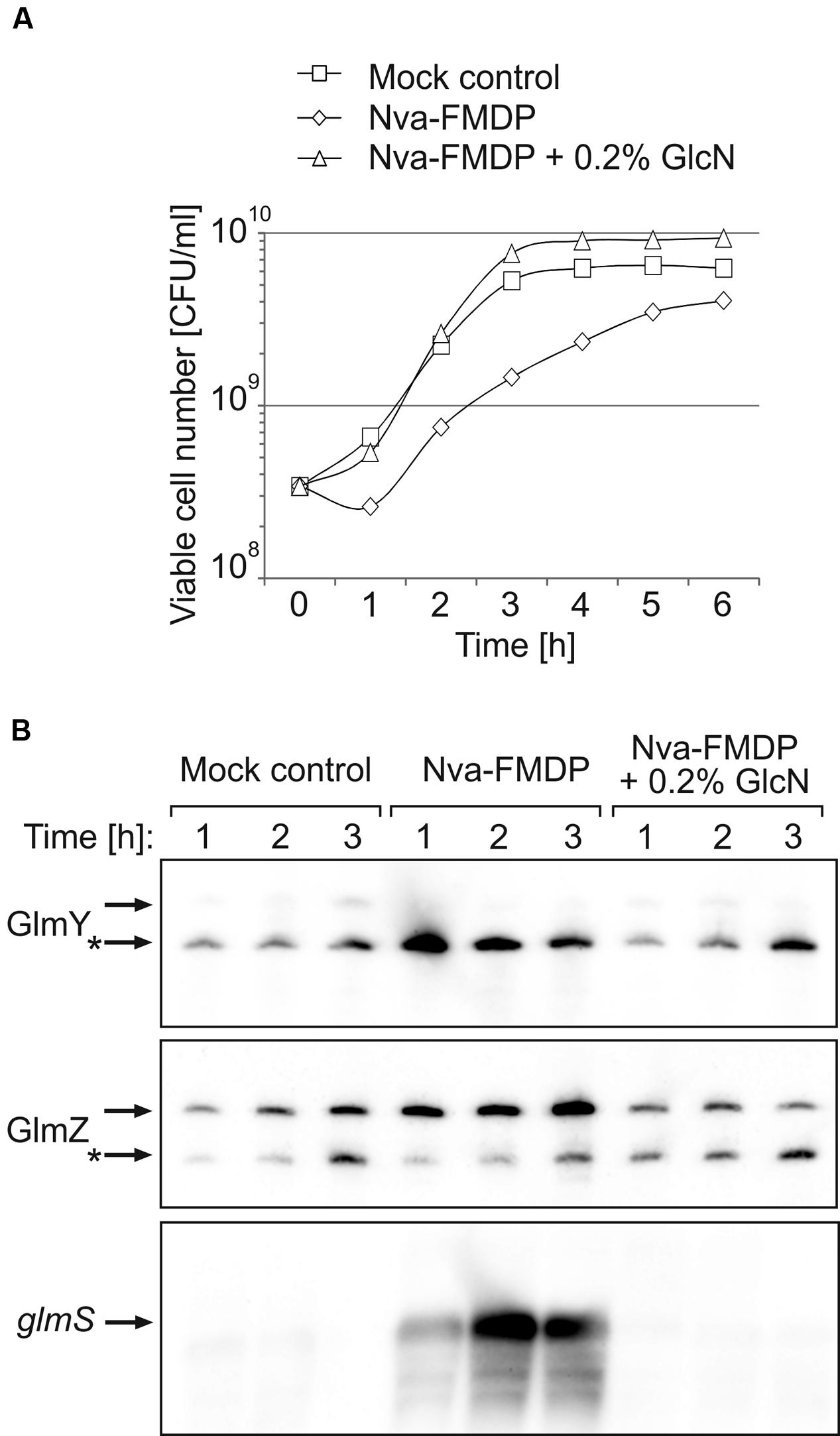
FIGURE 6. Upregulation of GlmY, GlmZ, and glmS RNAs by Nva-FMDP also occurs in Salmonella. (A) CFU numbers of cultures of Salmonella strain SL1344 grown in LB containing either 100 μg/ml Nva-FMDP (diamonds) or 100 μg/ml Nva-FMDP + 0.2% GlcN (triangles) or the equivalent volume of H2O (mock control; squares). The corresponding OD600 readings are provided in Supplementary Figure S5A. (B) Northern analysis of GlmY, GlmZ and glmS RNAs in the cultures analyzed in (A). Total RNA was extracted from samples harvested in hourly intervals during the first 3 h after inoculation and subsequently probed for detection of GlmY (top panel), GlmZ (medium panel) and glmS RNAs (bottom panel). Loading controls are provided in Supplementary Figure S5B. The various RNA species are indicated by arrows. Processed variants are denoted by asterisks.
Next, we studied susceptibility of Salmonella to Nva-FMDP and the roles of sRNAs GlmY and GlmZ in this process by carrying out disk diffusion assays and MIC determinations using wild-type strain SL1344 as well as the sRNA deletion strains (Tables 2 and 3; Supplementary Figures S4A,C). Globally, the results resembled those obtained for E. coli, but with some remarkable differences. First, Nva-FMDP had a stronger growth inhibitory effect on the Salmonella wild-type strain as compared to the E. coli wild-type. Zones of growth inhibition could be detected in all media (Table 2) and MIC values were generally lower for Salmonella decreasing in the order LB > Mueller Hinton > MacConkey lactose > M9 glucose (Table 3). Second, the absence of GlmY and GlmZ strongly increased susceptibility to Nva-FMDP confirming that these sRNAs provide intrinsic resistance even in Salmonella. Apparently, the sRNAs had a stronger impact on drug susceptibility in Salmonella: Depending on the medium, mutation of GlmY decreased the MIC 4- to 15-fold and mutation of GlmZ even 16- to 64-fold, whereas in E. coli a ~4-fold effect was seen (Table 3). In conclusion, GlmY and GlmZ are key players for the intrinsic resistance to antibiotics inhibiting GlmS, not only in E. coli but also in Salmonella.
Combination with a Non-metabolizable GlcN6P Analog May Increase Efficacy of Nva-FMDP against the E. coli Wild-Type
As GlmY and GlmZ are crucial for overcoming the deleterious effect of GlmS inhibitors, we reasoned whether suppression of the sRNAs could increase efficacy of these drugs against wild-type strains. Since the GlmY/GlmZ system apparently senses and responds to intracellular GlcN6P levels, we considered that a non-metabolizable analog of GlcN6P could potentially prevent the GlmY/GlmZ-mediated upregulation of GlmS, thereby increasing the efficacy of GlmS inhibition by Nva-FMDP. To provide a proof of concept, we used GlcN6SO4, which was shown to activate the B. subtilis glmS ribozyme to some extent in vitro indicating that it may act as GlcN6P analog in biological systems (Winkler et al., 2004). First, we tested GlcN6SO4 using disk diffusion assays. A small inhibition zone of ~8 mm diameter was observed when a filter disk loaded with 50 μg Nva-FMDP was applied onto a MacConkey lactose plate seeded with the E. coli wild-type strain Z854. Interestingly, the zone of growth inhibition gradually increased up to 13 mm upon co-administration of incremental amounts of GlcN6SO4 (Figure 7A). GlcN6SO4 showed no growth inhibitory properties on its own, indicating that it acts specifically by enhancing the antibacterial potential of Nva-FMDP (Supplementary Figure S6). Interestingly, this enhancing effect could not be observed for the ΔglmY and ΔglmZ mutant strains. In this case, constant inhibition zones were observed, regardless of the co-applied GlcN6SO4 potency, indicating that GlcN6SO4 requires the small RNAs to act (Supplementary Figure S7). To provide further evidence, we tested the combined effect of Nva-FMDP and GlcN6SO4 in liquid culture using the broth microdilution method. Serial dilutions of a solution containing 250 μg/ml Nva-FMDP and 2.5 mg/ml GlcN6SO4 were prepared in LB medium and subsequently inoculated with the E. coli wild-type strain Z854. As controls, serial dilutions containing only Nva-FMDP or GlcN6SO4 were tested in parallel. Indeed, following 12 h incubation, the cultures treated with the combination of both compounds showed a slightly stronger growth defect as compared to the cultures treated with Nva-FMDP alone (Figure 7B). Albeit weak, this difference was detectable in each individual experiment. The cultures that were solely supplemented with GlcN6SO4 exhibited no growth impairment confirming that this GlcN6P analog is nontoxic for the bacteria. Taken together, these results suggest that a GlcN6P analog such as GlcN6SO4 may have the potential to increase the antimicrobial activity of antibiotics targeting GlmS.
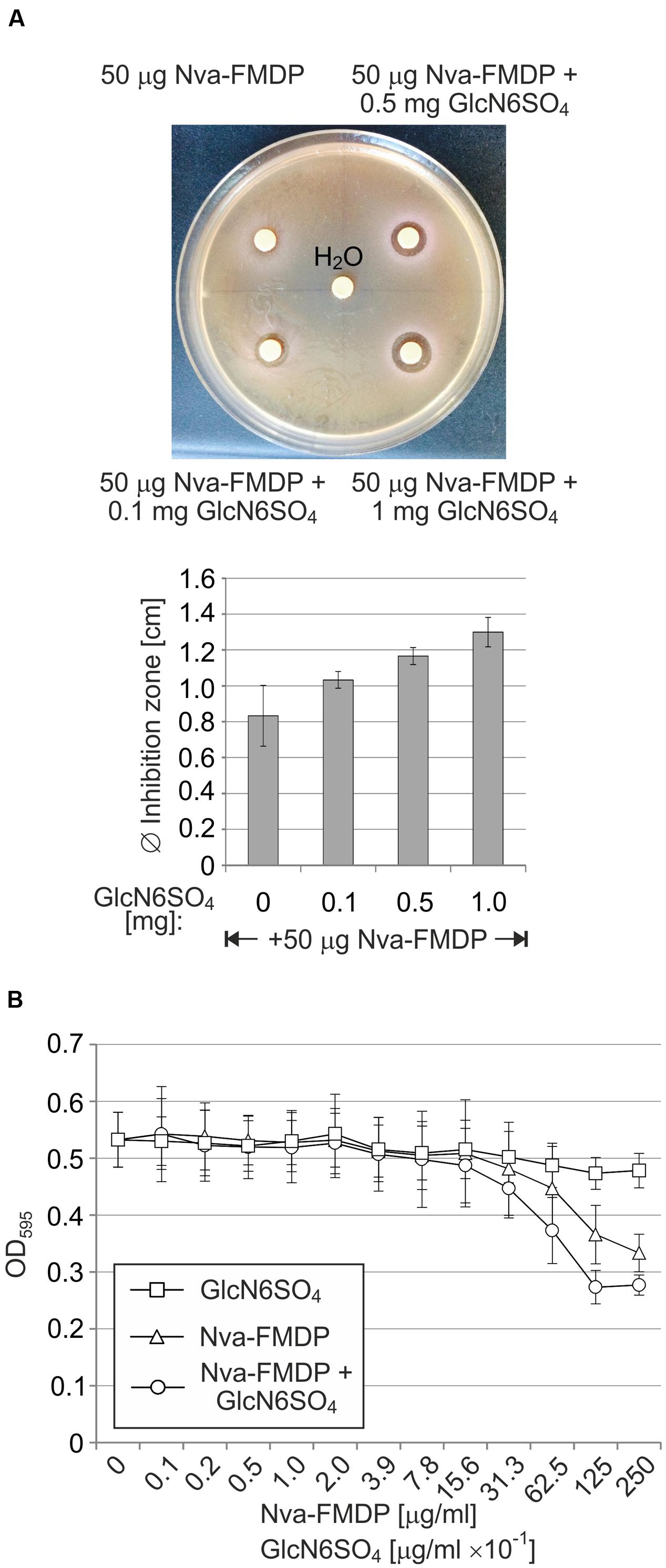
FIGURE 7. Co-administration of GlcN6SO4 increases susceptibility of wild-type E. coli towards Nva-FMDP. (A) Disk diffusion assay to address the combined effect of Nva-FMDP and GlcN6SO4 on E. coli. Filter disks containing 50 μg Nva-FMDP and various GlcN6SO4 amounts as indicated were applied to MacConkey lactose plates that were seeded with E. coli wild-type strain Z854. An exemplary experiment is shown at the top. A diagram depicting the results of three independent experiments is shown below. (B) Effect of GlcN6SO4 on susceptibility of E. coli to Nva-FMDP in LB liquid medium. Serial dilutions of Nva-FMDP or GlcN6SO4 or a mixture of both compounds were prepared in LB medium and subsequently inoculated with 5 × 105 CFU/ml of E. coli strain Z854. Cell densities were recorded following 12 h incubation.
Discussion
Peptide antibiotics targeting enzyme GlmS at the heart of bacterial cell envelope synthesis, are known for a long time. Structurally related oligopeptides such as Nva-FMDP have been developed, which lack the toxic effects exhibited by bacilysin on eukaryotic cells (Bontemps-Gracz et al., 1991). However, although readily taken up by peptide transporters, Gram-negative bacteria, such as E. coli, possess high intrinsic resistance towards these drugs, limiting their potential clinical use. Here, we reveal the mechanism underlying this intrinsic resistance. We show that both E. coli as well as Salmonella respond to these antibiotics by increased glmS expression, which compensates for inhibition of GlmS enzymatic activity (Figures 2 and 6). Cells respond in a dosage-dependent manner and adjust the level of glmS expression exactly to the level required to overcome growth inhibition by the GlmS inhibitor (Figure 5).
Responsible for this upregulation are two sRNAs which jointly regulate the glmS mRNA. Inhibition of GlmS by antibiotics leads to GlcN6P deprivation, which is sensed by sRNA GlmY triggering its accumulation (Figures 2 and 4). As expected, higher GlmY levels counteract degradation of sRNA GlmZ consequently activating glmS expression by base-pairing. We have recently shown that GlmY acts by sequestration of adapter protein RapZ, which otherwise binds GlmZ and recruits RNase E to inactivate the latter sRNA (Göpel et al., 2013, 2016). As these two sRNAs act hierarchically to control glmS expression, inactivation of either of them results in the inability to respond to GlcN6P deprivation and consequently increases susceptibility to GlmS inhibitors significantly, i.e., up to eightfold for E. coli and up to 64-fold for Salmonella depending on the growth medium (Tables 2 and 3). The basis for the different impact of the sRNAs on drug susceptibility in these two species is unknown, but could be caused by differences in basal glmS expression levels remaining in the absence of GlmY or GlmZ. The reason for the strong variation of efficacy of Nva-FMDP by the growth medium also remains unclear. A reasonable explanation might be provided by competition of Nva-FMDP with externally available peptides for uptake (Marshall et al., 2003) and/or by the known repression of the dipeptide transporter component DppA by amino acids (Olson et al., 1991). Regardless of the growth medium, GlmY/GlmZ endow both species with intrinsic resistance against GlmS inhibitors. Thus, this mechanism might be conserved in all species possessing GlmY/GlmZ, i.e., in Enterobacteriaceae.
Our data indicate that suppression of the GlmY/GlmZ circuit would substantially increase susceptibility of wild-type bacteria to GlmS inhibitors. As the GlmY/GlmZ cascade is sensing and responding to GlcN6P, a non-metabolizable analog should accomplish this task. Initial experiments using GlcN6SO4 support this possibility as this compound increases efficacy of Nva-FMDP in a GlmY/GlmZ-dependent manner, albeit weakly (Figure 7; Supplementary Figure S7). The reason for the weak activity of GlcN6SO4 is unclear, but hydrolysis by so far unknown sulfatases or inefficient uptake is a likely explanation. Thus, a future task will be the identification of a stable GlcN6P analog that suppresses the GlmY/GlmZ system efficiently. Testing the numerous artificial GlcN6P mimics that have been synthesized to serve as artificial actuators of the Gram-positive glmS ribozyme (Fei et al., 2014), might provide a good starting point. Finally, it might also be possible to design this analog on a rational basis, once the GlcN6P sensor and the way how it interacts with the amino sugar has been identified. As it sits at the top of the regulatory cascade, sRNA GlmY is the most likely candidate, a possibility that is currently addressed in our laboratory.
The contribution of RNA regulatory elements to antibiotic resistance and their potential value as drug targets is appreciated only recently. Traditionally, screens for novel antimicrobial targets or factors mediating drug resistance have focused on proteins (Martinez and Rojo, 2011; Cox and Wright, 2013). A first systematic screen investigating the roles of various Hfq-dependent sRNAs for susceptibility to different classes of antibiotics has been reported recently (Kim et al., 2015). Several sRNAs were shown to alter antibiotic susceptibility in E. coli and Salmonella when deleted and/or overproduced. The underlying mechanisms remain largely unknown, but in two cases regulation of efflux pump components by sRNAs might be involved (Nishino et al., 2011; Kim et al., 2015; Parker and Gottesman, 2016). In sum, there is increasing evidence that sRNAs have crucial roles in genetic networks that provide intrinsic resistance to antibiotics, either by acting via more unspecific mechanisms (e.g., drug efflux pumps) and involving multiple targets (Schnorpfeil et al., 2013; Kim et al., 2015) or by impacting specifically on a single gene whose product is selectively targeted by a cognate antibiotic (this study). It remains to be investigated, whether sRNAs and other regulatory RNA elements indeed represent druggable targets proving useful for antimicrobial chemotherapy. In this regard, a recent study is encouraging that identified a compound, which binds to an RNA riboswitch and thereby suppresses synthesis of the essential vitamin riboflavin (Howe et al., 2015). Future work must determine whether the same holds true for the GlmY/GlmZ system, i.e., whether a compound could be identified that interferes with the sRNAs and inhibits their response to GlcN6P deprivation, thereby increasing susceptibility to GlmS inhibitors in combined therapy.
Author Contributions
BG designed the study. BG and MK conceived the experiments. MK performed the experiments with assistance of YG. All authors analyzed the data and discussed the results. SM contributed material. BG wrote the paper. All authors read, commented on and approved the final manuscript.
Funding
This work was supported by the ‘Austrian Science Fund’ (FWF; grant numbers P 26681-B22, F4317 to BG).
Conflict of Interest Statement
The authors declare that the research was conducted in the absence of any commercial or financial relationships that could be construed as a potential conflict of interest.
Acknowledgments
We thank Takashi Inaoka for providing B. subtilis strains TI304 and TI398 and Jörg Vogel for Salmonella strains SL1344, JVS112 and JVS122. We are grateful to Dr. Ryszard Andruszkiewicz (Gdańsk University of Technology) for synthesis of Nva-FMDP.
Supplementary Material
The Supplementary Material for this article can be found online at: http://journal.frontiersin.org/article/10.3389/fmicb.2016.00908
References
Andruszkiewicz, R., Chmara, H., Milewski, S., and Borowski, E. (1987). Synthesis and biological properties of N3-(4-methoxyfumaroyl)-L-2,3-diaminopropanoic acid dipeptides, a novel group of antimicrobial agents. J. Med. Chem. 30, 1715–1719. doi: 10.1021/jm00393a005
Badet, B., Vermoote, P., and Le Goffic, F. (1988). Glucosamine synthetase from Escherichia coli: kinetic mechanism and inhibition by N3-fumaroyl-L-2,3-diaminopropionic derivatives. Biochemistry 27, 2282–2287. doi: 10.1021/bi00407a006
Bauer, A. W., Kirby, W. M., Sherris, J. C., and Turck, M. (1966). Antibiotic susceptibility testing by a standardized single disk method. Am. J. Clin. Pathol. 45, 493–496.
Bennett, A. M., Shippy, D. C., Eakley, N., Okwumabua, O., and Fadl, A. A. (2016). Functional characterization of glucosamine-6-phosphate synthase (GlmS) in Salmonella enterica serovar Enteritidis. Arch. Microbiol. doi: 10.1007/s00203-016-1212-x [Epub ahead of print].
Bontemps-Gracz, M., Milewski, S., and Borowski, E. (1991). The influence of L-norvalyl-N3-4-methoxyfumaroyl-L-2,3-diaminopropanoic acid, an antifungal agent, on mammalian cells in tissue culture. Acta Biochim. Pol. 38, 229–239.
Borisova, M., Gisin, J., and Mayer, C. (2014). Blocking peptidoglycan recycling in Pseudomonas aeruginosa attenuates intrinsic resistance to fosfomycin. Microb. Drug Resist. 20, 231–237. doi: 10.1089/mdr.2014.0036
Brown, E. D., and Wright, G. D. (2016). Antibacterial drug discovery in the resistance era. Nature 529, 336–343. doi: 10.1038/nature17042
Chmara, H., Andruszkiewicz, R., and Borowski, E. (1986). Inactivation of glucosamine-6-phosphate synthetase from Salmonella typhimurium LT2 by fumaroyl diaminopropanoic acid derivatives, a novel group of glutamine analogs. Biochim. Biophys. Acta 870, 357–366. doi: 10.1016/0167-4838(86)90240-2
Chmara, H., Milewski, S., Andruszkiewicz, R., Mignini, F., and Borowski, E. (1998). Antibacterial action of dipeptides containing an inhibitor of glucosamine-6-phosphate isomerase. Microbiology 144, 1349–1358. doi: 10.1099/00221287-144-5-1349
Cox, G., and Wright, G. D. (2013). Intrinsic antibiotic resistance: mechanisms, origins, challenges and solutions. Int. J. Med. Microbiol. 303, 287–292. doi: 10.1016/j.ijmm.2013.02.009
Datsenko, K. A., and Wanner, B. L. (2000). One-step inactivation of chromosomal genes in Escherichia coli K-12 using PCR products. Proc. Natl. Acad. Sci. U.S.A. 97, 6640–6645. doi: 10.1073/pnas.120163297
EUCAST (2003). EUCAST Discussion Document E. Dis 5.1: determination of minimum inhibitory concentrations (MICs) of antibacterial agents by broth dilution. Clin. Microbiol. Infect. 9, 1–7. doi: 10.1042/BA20090219
Fei, X., Holmes, T., Diddle, J., Hintz, L., Delaney, D., Stock, A., et al. (2014). Phosphatase-inert glucosamine 6-phosphate mimics serve as actuators of the glmS riboswitch. ACS Chem. Biol. 9, 2875–2882. doi: 10.1021/cb500458f
Fröhlich, K. S., and Vogel, J. (2009). Activation of gene expression by small RNA. Curr. Opin. Microbiol. 12, 674–682. doi: 10.1016/j.mib.2009.09.009
Göpel, Y., Khan, M. A., and Görke, B. (2016). Domain swapping between homologous bacterial small RNAs dissects processing and Hfq binding determinants and uncovers an aptamer for conditional RNase E cleavage. Nucleic Acids Res. 44, 824–837. doi: 10.1093/nar/gkv1161
Göpel, Y., Lüttmann, D., Heroven, A. K., Reichenbach, B., Dersch, P., and Görke, B. (2011). Common and divergent features in transcriptional control of the homologous small RNAs GlmY and GlmZ in Enterobacteriaceae. Nucleic Acids Res. 39, 1294–1309. doi: 10.1093/nar/gkq986
Göpel, Y., Papenfort, K., Reichenbach, B., Vogel, J., and Görke, B. (2013). Targeted decay of a regulatory small RNA by an adaptor protein for RNase E and counteraction by an anti-adaptor RNA. Genes Dev. 27, 552–564. doi: 10.1101/gad.210112.112
Gruber, C. C., and Sperandio, V. (2014). Posttranscriptional control of microbe-induced rearrangement of host cell actin. MBio 5:e1025-13. doi: 10.1128/mBio.01025-13
Gruber, C. C., and Sperandio, V. (2015). Global analysis of posttranscriptional regulation by GlmY and GlmZ in enterohemorrhagic Escherichia coli O157:H7. Infect. Immun. 83, 1286–1295. doi: 10.1128/IAI.02918-14
Hoiseth, S. K., and Stocker, B. A. (1981). Aromatic-dependent Salmonella typhimurium are non-virulent and effective as live vaccines. Nature 291, 238–239. doi: 10.1038/291238a0
Howe, J. A., Wang, H., Fischmann, T. O., Balibar, C. J., Xiao, L., Galgoci, A. M., et al. (2015). Selective small-molecule inhibition of an RNA structural element. Nature 526, 672–677. doi: 10.1038/nature15542
Inaoka, T., Wang, G., and Ochi, K. (2009). ScoC regulates bacilysin production at the transcription level in Bacillus subtilis. J. Bacteriol. 191, 7367–7371. doi: 10.1128/JB.01081-09
Kalamorz, F., Reichenbach, B., März, W., Rak, B., and Görke, B. (2007). Feedback control of glucosamine-6-phosphate synthase GlmS expression depends on the small RNA GlmZ and involves the novel protein YhbJ in Escherichia coli. Mol. Microbiol. 65, 1518–1533. doi: 10.1111/j.1365-2958.2007.05888.x
Kenig, M., and Abraham, E. P. (1976). Antimicrobial activities and antagonists of bacilysin and anticapsin. J. Gen. Microbiol. 94, 37–45. doi: 10.1099/00221287-94-1-37
Kim, K., Jeong, J. H., Lim, D., Hong, Y., Yun, M., Min, J. J., et al. (2013). A novel balanced-lethal host-vector system based on glmS. PLoS ONE 8:e60511. doi: 10.1371/journal.pone.0060511
Kim, T., Bak, G., Lee, J., and Kim, K. S. (2015). Systematic analysis of the role of bacterial Hfq-interacting sRNAs in the response to antibiotics. J. Antimicrob. Chemother. 70, 1659–1668. doi: 10.1093/jac/dkv042
Kucharczyk, N., Denisot, M. A., Le Goffic, F., and Badet, B. (1990). Glucosamine-6-phosphate synthase from Escherichia coli: determination of the mechanism of inactivation by N3-fumaroyl-L-2,3-diaminopropionic derivatives. Biochemistry 29, 3668–3676. doi: 10.1021/bi00467a012
Marshall, N. J., Andruszkiewicz, R., Gupta, S., Milewski, S., and Payne, J. W. (2003). Structure-activity relationships for a series of peptidomimetic antimicrobial prodrugs containing glutamine analogues. J. Antimicrob. Chemother. 51, 821–831. doi: 10.1093/jac/dkg170
Martinez, J. L., and Rojo, F. (2011). Metabolic regulation of antibiotic resistance. FEMS Microbiol. Rev. 35, 768–789. doi: 10.1111/j.1574-6976.2011.00282.x
Milewski, S. (2002). Glucosamine-6-phosphate synthase–the multi-facets enzyme. Biochim. Biophys. Acta 1597, 173–192. doi: 10.1016/S0167-4838(02)00318-7
Milewski, S., Chmara, H., and Borowski, E. (1986). Anticapsin: an active site directed inhibitor of glucosamine-6-phosphate synthetase from Candida albicans. Drugs Exp. Clin. Res. 12, 577–583.
Miller, J. (1972). Experiments in Molecular Genetics. Cold Spring Harbor, NY: Cold Spring Harbor Laboratory Press.
Molloy, B. B., Lively, D. H., Gale, R. M., Gorman, M., and Boeck, L. D. (1972). A new dipeptide antibiotic from Streptomyces collinus. Lindenbein. J. Antibiot. (Tokyo) 25, 137–140. doi: 10.7164/antibiotics.25.137
Mühlen, S., and Dersch, P. (2016). Anti-virulence strategies to target bacterial infections. Curr. Top. Microbiol. Immunol. doi: 10.1007/82_2015_490 [Epub ahead of print].
Nishino, K., Yamasaki, S., Hayashi-Nishino, M., and Yamaguchi, A. (2011). Effect of overexpression of small non-coding DsrA RNA on multidrug efflux in Escherichia coli. J. Antimicrob. Chemother. 66, 291–296. doi: 10.1093/jac/dkq420
Olson, E. R., Dunyak, D. S., Jurss, L. M., and Poorman, R. A. (1991). Identification and characterization of dppA, an Escherichia coli gene encoding a periplasmic dipeptide transport protein. J. Bacteriol. 173, 234–244.
Özcengiz, G., and Ögülür, I. (2015). Biochemistry, genetics and regulation of bacilysin biosynthesis and its significance more than an antibiotic. New Biotechnol. 32, 612–619. doi: 10.1016/j.nbt.2015.01.006
Papenfort, K., Pfeiffer, V., Lucchini, S., Sonawane, A., Hinton, J. C., and Vogel, J. (2008). Systematic deletion of Salmonella small RNA genes identifies CyaR, a conserved CRP-dependent riboregulator of OmpX synthesis. Mol. Microbiol. 68, 890–906. doi: 10.1111/j.1365-2958.2008.06189.x
Parker, A., and Gottesman, S. (2016). Small RNA regulation of TolC, the outer membrane component of bacterial multidrug transporters. J. Bacteriol. 198, 1101–1113. doi: 10.1128/JB.00971-15
Persiani, S., Rotini, R., Trisolino, G., Rovati, L. C., Locatelli, M., Paganini, D., et al. (2007). Synovial and plasma glucosamine concentrations in osteoarthritic patients following oral crystalline glucosamine sulphate at therapeutic dose. Osteoarthr. Cartil. 15, 764–772. doi: 10.1016/j.joca.2007.01.019
Plumbridge, J. (2015). Regulation of the utilization of amino sugars by Escherichia coli and Bacillus subtilis: same genes, different control. J. Mol. Microbiol. Biotechnol. 25, 154–167. doi: 10.1159/000369583
Reichenbach, B., Göpel, Y., and Görke, B. (2009). Dual control by perfectly overlapping sigma 54- and sigma 70- promoters adjusts small RNA GlmY expression to different environmental signals. Mol. Microbiol. 74, 1054–1070. doi: 10.1111/j.1365-2958.2009.06918.x
Reichenbach, B., Maes, A., Kalamorz, F., Hajnsdorf, E., and Görke, B. (2008). The small RNA GlmY acts upstream of the sRNA GlmZ in the activation of glmS expression and is subject to regulation by polyadenylation in Escherichia coli. Nucleic Acids Res. 36, 2570–2580. doi: 10.1093/nar/gkn091
Schnorpfeil, A., Kranz, M., Kovacs, M., Kirsch, C., Gartmann, J., Brunner, I., et al. (2013). Target evaluation of the non-coding csRNAs reveals a link of the two-component regulatory system CiaRH to competence control in Streptococcus pneumoniae R6. Mol. Microbiol. 89, 334–349. doi: 10.1111/mmi.12277
Silver, L. L. (2013). Viable screening targets related to the bacterial cell wall. Ann. N. Y. Acad. Sci. 1277, 29–53. doi: 10.1111/nyas.12006
Teplyakov, A., Leriche, C., Obmolova, G., Badet, B., and Badet-Denisot, M. A. (2002). From Lobry de Bruyn to enzyme-catalyzed ammonia channelling: molecular studies of D-glucosamine-6P synthase. Nat. Prod. Rep. 19, 60–69. doi: 10.1039/b103713g
Urban, J. H., and Vogel, J. (2008). Two seemingly homologous noncoding RNAs act hierarchically to activate glmS mRNA translation. PLoS Biol. 6:e64. doi: 10.1371/journal.pbio.0060064
Venkatesh, G. R., Kembou Koungni, F. C., Paukner, A., Stratmann, T., Blissenbach, B., and Schnetz, K. (2010). BglJ-RcsB heterodimers relieve repression of the Escherichia coli bgl operon by H-NS. J. Bacteriol. 192, 6456–6464. doi: 10.1128/JB.00807-10
Wilson, G. G., Young, K. Y., Edlin, G. J., and Konigsberg, W. (1979). High-frequency generalised transduction by bacteriophage T4. Nature 280, 80–82. doi: 10.1038/280080a0
Keywords: glucosamine-6-phosphate synthase GlmS, Nva-FMDP, Bacilysin, intrinsic resistance, small RNA, GlmY, GlmZ, antimicrobial chemotherapy
Citation: Khan MA, Göpel Y, Milewski S and Görke B (2016) Two Small RNAs Conserved in Enterobacteriaceae Provide Intrinsic Resistance to Antibiotics Targeting the Cell Wall Biosynthesis Enzyme Glucosamine-6-Phosphate Synthase. Front. Microbiol. 7:908. doi: 10.3389/fmicb.2016.00908
Received: 11 May 2016; Accepted: 27 May 2016;
Published: 15 June 2016.
Edited by:
Jose L. Martinez, Centro Nacional de Biotecnología, SpainReviewed by:
Antonio Oliver, Hospital Son Dureta, SpainMarco Rinaldo Oggioni, University of Leicester, UK
Copyright © 2016 Khan, Göpel, Milewski and Görke. This is an open-access article distributed under the terms of the Creative Commons Attribution License (CC BY). The use, distribution or reproduction in other forums is permitted, provided the original author(s) or licensor are credited and that the original publication in this journal is cited, in accordance with accepted academic practice. No use, distribution or reproduction is permitted which does not comply with these terms.
*Correspondence: Boris Görke, Ym9yaXMuZ29lcmtlQHVuaXZpZS5hYy5hdA==