- Department of Applied Biology, Graduate School of Science and Technology, Kyoto Institute of Technology, Kyoto, Japan
Vanillin is a potent fermentation inhibitor derived from the lignocellulosic biomass in biofuel production, and high concentrations of vanillin result in the pronounced repression of bulk translation in Saccharomyces cerevisiae. Studies on genes that are efficiently translated even in the presence of high concentrations of vanillin will be useful for improving yeast vanillin tolerance and fermentation efficiency. The BDH1 and BDH2 genes encode putative medium-chain alcohol dehydrogenase/reductases and their amino acid sequences are very similar to each other. Although BDH2 was previously suggested to be involved in vanillin tolerance, it has yet to be clarified whether Bdh1/Bdh2 actually contribute to vanillin tolerance and reductions in vanillin. Therefore, we herein investigated the effects of Bdh1 and Bdh2 on vanillin tolerance. bdh2Δ cells exhibited hypersensitivity to vanillin and slower reductions in vanillin than wild-type cells and bdh1Δ cells. Additionally, the overexpression of the BDH2 gene improved yeast tolerance to vanillin more efficiently than that of BDH1. Only BDH2 mRNA was efficiently translated under severe vanillin stress, however, both BDH genes were transcriptionally up-regulated. These results reveal the importance of Bdh2 in vanillin detoxification and confirm the preferential translation of the BDH2 gene in the presence of high concentrations of vanillin. The BDH2 promoter also enabled the expression of non-native genes under severe vanillin stress and furfural stress, suggesting its availability to improve of the efficiency of bioethanol production through modifications in gene expression in the presence of fermentation inhibitors.
Introduction
Biomass conversion inhibitors including vanillin, furans, and organic acids are inevitably formed during the saccharification pretreatment of the lignocellulosic biomass. They are significant barriers to decreasing the production cost of bioethanol because of their toxicity to yeast cells (Palmqvist and Hahn-Hägerdal, 2000; Helle et al., 2003; Klinke et al., 2004; Jonsson et al., 2013). Because vanillin is one of the most potent fermentation inhibitors in lignocellulose hydrolysates (Klinke et al., 2004), breeding robust yeast strains with a high tolerance to vanillin has become increasingly important for improving the productive efficiency of bioethanol from the lignocellulosic biomass. In order to improve yeast tolerance against vanillin, its physiological effects on yeast cells and cellular responses to vanillin need to be clarified in more detail.
Previous studies reported that several types of stress such as glucose starvation and ethanol stress cause a rapid reduction in overall protein synthesis and the formation of cytosolic mRNP granules including P-bodies and stress granules (SGs) in Saccharomyces cerevisiae (Ashe et al., 2000; Kato et al., 2011). We also showed that severe vanillin stress caused translational repression and the formation of cytosolic mRNP granules, leading to a reduction in overall protein synthesis levels and the limited translation of mRNAs (Iwaki et al., 2013b; Nguyen et al., 2014). It is conceivable that mRNAs encoding the proteins that play a role in stress tolerance are efficiently translated even under severe stress conditions. Indeed, small heat shock protein mRNAs are efficiently translated overcoming translation repression caused by glucose starvation (Zid and O’Shea, 2014). We also recently reported that ADH7 mRNA, which encodes a NADPH-dependent medium-chain alcohol dehydrogenase, was efficiently translated under severe vanillin stress conditions (Larroy et al., 2002; Nguyen et al., 2015). Adh7 and Adh6 reduce vanillin to vanillyl alcohol (Larroy et al., 2002). However, other mRNAs are considered to be efficiently translated during severe vanillin stress, except for ADH7.
Shen et al. (2014) recently demonstrated that a vanillin tolerant yeast strain (EMV-8) strongly expressed the BDH2 gene, which also encodes a putative medium-chain alcohol dehydrogenase/reductase (MDR; Nordling et al., 2002), suggesting the importance of Bdh2 in vanillin tolerance in yeast cells. The amino acid sequence of Bdh2 is 51% identical to that of Bdh1 (González et al., 2010). Although Bdh1 exhibits butanediol-dehydrogenase activity (González et al., 2010), Bdh2 is devoid of this activity and its physiological function remains unclear. The BDH1 (YAL060w) gene is adjacent to the BDH2 (YAL061w) gene in the same chromosome and their transcription is reciprocally regulated (González et al., 2010).
In order to evaluate the in vivo roles of Bdh1/Bdh2 in response to vanillin stress, we examined the expression of the BDH1 and BDH2 genes under severe vanillin stress conditions in addition to their contribution to the detoxification of vanillin. bdh2Δ cells grew slowly in the presence of vanillin with a slower rate of vanillin reduction than wild-type and bdh1Δ cells, suggesting that Bdh2 significantly contributes to the detoxification of vanillin in vivo. We found that although BDH2 mRNA was efficiently translated, BDH1 and BDH2 mRNA levels were both up-regulated in the presence of severe vanillin stress. We also showed that the BDH2 promoter enabled the protein synthesis of non-native genes such as ADH6 and GFP under severe vanillin stress, indicating that the BDH2 promoter is useful for improving the stress tolerance and fermentation efficiency of yeast cells by modifying gene expression in lignocellulose hydrolysates.
Materials and Methods
Strains and Medium
Saccharomyces cerevisiae strain BY4742 (MATα his3Δ1 ura3Δ0 leu2Δ0 lys2Δ0) and its isogenic bdh2Δ and bdh1Δ null mutants (Open Biosystems Inc., Huntsville, AL, USA) were used in the present study. Since the BDH1 (YAL060w) gene is adjacent to the BDH2 (YAL061w) gene in the same chromosome, the double knockout mutant bdh1Δbdh2Δ was constructed using the sequential integrative transformation method (Kitada et al., 1995; González et al., 2010). The DNA fragment (1.8 kb) encoding the bdh1Δbdh2Δ::cgHIS3 region was amplified using the plasmid pSHB1805 (Kitada et al., 1995) as a template and the primer set bdhKO-F/R (Table 1), and was introduced into BY4742. The disruption of the BDH1 and BDH2 genes was confirmed by PCR. Cells were cultured in 50 ml of SD medium (2% glucose, 0.67% yeast nitrogen base w/o amino acids, 20 mg/L uracil, 30 mg/L L-lysine HCl, 100 mg/L L-leucine, and 20 mg/L L-histidine HCl) at 28°C with reciprocal shaking (120 rpm) in Erlenmeyer flasks (200 ml). Cell growth in the presence of vanillin was monitored by measuring optical density at 600 nm (OD600).
Plasmids
The oligonucleotide sequences of the primers used for plasmid construction are listed in Table 1. Genomic DNA from BY4742 was used as a template to amplify yeast genes by PCR. YIp-ADH7-FLAG and pRS316-ADH7Pro-ADH6-FLAG-ADH7Ter were described previously (Nguyen et al., 2015).
YIp-BDH1-GFP and YIp-BDH1-FLAG
A 0.9-kbp fragment encoding part of the open reading frame (ORF) of the BDH1 gene was amplified using the primer set BDH1-F1/R1. The amplicon was digested with NotI/XhoI, and then cloned into the NotI/XhoI sites of pJK67 (Kahana et al., 1998) in order to construct YIp-BDH1-GFP. The plasmid was linearized by SphI and integrated at the chromosomal BDH1 locus.
The integrate-type plasmid YIp-BDH1-FLAG was constructed to estimate the protein levels of Bdh1. This plasmid contained a FLAG tag sequence (encoded by 24 nt) immediately upstream of the stop codon and 3′-flanking region of BDH1. A 0.4-kbp fragment encoding a FLAG tag sequence, stop codon, and the 3′-flanking region of BDH1 was amplified using the primer set BDH1-F2/R2. The amplicon was digested with XhoI/BamHI and cloned into the XhoI/BamHI sites of YIp-BDH1-GFP to construct YIp-BDH1-FLAG. In order to integrate the BDH1-FLAG gene at the chromosomal BDH1 locus, YIp-BDH1-FLAG was linearized through its digestion with SphI and was then introduced into yeast cells.
YIp-BDH2-GFP and YIp-BDH2-FLAG
A 0.8-kbp fragment encoding part of the ORF of the BDH2 gene was amplified using the primer set BDH2-F1/R1. The amplicon was digested with SpeI/XhoI, and then cloned into the XbaI/XhoI sites of pJK67 to construct YIp-BDH2-GFP. The plasmid was linearized by NarI and integrated at the chromosomal BDH2 locus.
The integrate-type plasmid YIp-BDH2-FLAG was constructed to estimate the protein levels of Bdh2. A 0.35-kbp fragment encoding a FLAG tag sequence, stop codon, and the 3′-flanking region of BDH2 was amplified using the primer set BDH2-F2/R2. The amplicon was digested with XhoI/KpnI and cloned into the XhoI/KpnI sites of YIp-BDH2-GFP to construct YIp-BDH2-FLAG. In order to integrate the BDH2-FLAG gene at the chromosomal BDH2 locus, YIp-BDH2-FLAG was linearized by digesting with NarI and was then introduced into yeast cells.
pRS423-BDH1 and pRS426-BDH2
A DNA fragment containing the promoter region (0.7 kbp), ORF, and terminator region (0.5 kbp) of the BDH1 gene was amplified using the primer set BDH1-F3/R3 and cloned into the BamHI/XhoI sites of pRS423 (Sikorski and Hieter, 1989) to construct pRS423-BDH1. A DNA fragment containing the promoter region (0.7 kbp), ORF, and terminator region (0.5 kbp) of the BDH2 gene was amplified using the primer set BDH2-F4/R4 and cloned into the BamHI/XhoI sites of pRS426 (Sikorski and Hieter, 1989) to construct pRS426-BDH2.
pRS316-BDH2Pro/FLAG/Ter
The promoter region (0.7 kbp) and terminator region with the FLAG tag (0.35 kbp) of the BDH2 gene were amplified using the primer sets BDH2-F3/R3 and BDH2-F2/R2, respectively. They were cloned into the SacI/XbaI and XhoI/KpnI sites of pRS316 (Sikorski and Hieter, 1989) to construct pRS316-BDH2Pro/FLAG/Ter. The ORF of the GFP gene was amplified using the primer set GFP-F/R, and cloned into the XbaI/XhoI sites of pRS316-BDH2Pro/FLAG/Ter to construct pRS316-BDH2Pro-GFP-FLAG-BDH2Ter. In order to construct pRS316-BDH2Pro-ADH6-FLAG-BDH2Ter, the ORF of the ADH6 gene was amplified using the primer set ADH6Orf-F2/R (Nguyen et al., 2015), and cloned into the EcoRI/XhoI sites of pRS316-BDH2Pro/FLAG/Ter.
pRS316-BDH2Pro-ADH6-FLAG-ADH6Ter
A 0.5-kbp fragment encoding a FLAG tag sequence, stop codon, and the 3′-flanking region of ADH6 was amplified using the primer set ADH6Ter-F/R (Nguyen et al., 2015). The amplicon was digested with XhoI/KpnI and cloned into the XhoI/KpnI sites of pRS316-BDH2Pro-ADH6-FLAG-BDH2Ter to construct pRS316-BDH2Pro-ADH6-FLAG-ADH6Ter.
pRS316-BDH2Pro-ADH6-FLAG-BDH1Ter
A 0.4-kbp fragment encoding a FLAG tag sequence, stop codon, and the 3′-flanking region of BDH1 was amplified using the primer set BDH1-F2/R4. The amplicon was digested with XhoI/KpnI and cloned into the XhoI/KpnI sites of pRS316-BDH2Pro-ADH6-FLAG-BDH2Ter to construct pRS316-BDH2Pro-ADH6-FLAG-BDH1Ter.
Quantitative Real-time PCR
The relative mRNA levels of the BDH1 and BDH2 genes were measured by quantitative real time-PCR (qRT-PCR). Total RNA was extracted by the method of Schmitt et al. (1990). cDNA was synthesized using ReverTra Ace qPCR RT Master Mix FSQ-201 (Toyobo, Osaka, Japan), and an analysis was performed using SYBR® Premix Ex TaqTMII (Takara Bio Inc., Shiga, Japan) and the sequence detection system (Thermal Cycler Dice Real Time System Lite, Takara Bio Inc., Shiga, Japan). The oligonucleotide sequences of the primers used for qRT-PCR were verified in previous studies (Subramanian et al., 2005; Erasmus and van Vuuren, 2009; Takahashi et al., 2011) and are listed in Table 2.
Chemicals and Analysis Methods
Vanillin, furfural, and dimethyl sulfoxide (DMSO) were obtained from Wako (Osaka, Japan). Cycloheximide (CHX) was purchased from Nacalai Tesque, Kyoto, Japan. Stock solutions of 2 M vanillin, 2 M furfural, and 20 mg/ml CHX were prepared in DMSO and stored at -30°C. Vanillin levels in SD medium were assayed by the methods of Fitzgerald et al. (2003). Briefly, after spinning down cells, the supernatant was separated using a linear gradient elution of 10–45% acetonitrile in 0.1% trifluoroacetic acid by high performance liquid chromatography (HPLC; Shimadzu, Kyoto, Japan) equipped with a C18 reversed-phase column, TSKgel ODS-100S (4.6 mm × 150 mm; Tosoh, Tokyo, Japan). The column was maintained at 40°C. The eluted compounds were detected at 280 nm. A Leica AF6500 (Leica Microsystems Vertrieb GmbH, Germany) fluorescence microscopic system was used for the microscopic analysis. Cells treated with vanillin were immediately observed without fixation. All experiments were repeated at least three times.
Western Blot Analysis
A cell-free extract (CFE) in 50 mM potassium phosphate buffer (pH 6.8) was prepared after the stress treatment. The total protein concentrations of CFE were measured using the Protein Assay CBB Solution kit (Nacalai Tesque, Kyoto, Japan) and 36 micrograms of total protein was applied to each lane of a 12.5% polyacrylamide gel for the SDS-PAGE analysis. The levels of FLAG-tagged proteins (Bdh1, Bdh2, Adh6, and Adh7) were monitored using a monoclonal anti-FLAG M2 antibody (Sigma–Aldrich, St. Louis, MO, USA). GFP levels were monitored with an anti-GFP antibody (mFX75; Wako, Osaka, Japan). Pgk1 was used as a loading control, and its levels were monitored with a monoclonal anti-PGK antibody (22C5D8; Life Technologies, Frederick, MD, USA). The bands of the Western blot were quantified using Image Studio Digits Ver 4.0 software (LI-COR Biotechnology, Lincoln, NE, USA).
Results
bdh2Δ Mutants Were Sensitive to Vanillin
In order to investigate the importance of Bdh1 and Bdh2 for tolerance to vanillin, the growth of the null mutants of BDH genes in SD medium containing vanillin (the initial vanillin concentration was 8 mM) was investigated (Figure 1A). Although the growth curves of wild-type and the mutants (bdh1Δ, bdh2Δ, and bdh1Δbdh2Δ) were almost identical under non-stressed conditions, the presence of vanillin led to slower growth rates for the bdh2Δ and bdh1Δbdh2Δ mutants than those for the wild-type and bdh1Δ mutant. This result strongly indicates the importance of Bdh2 for vanillin tolerance.
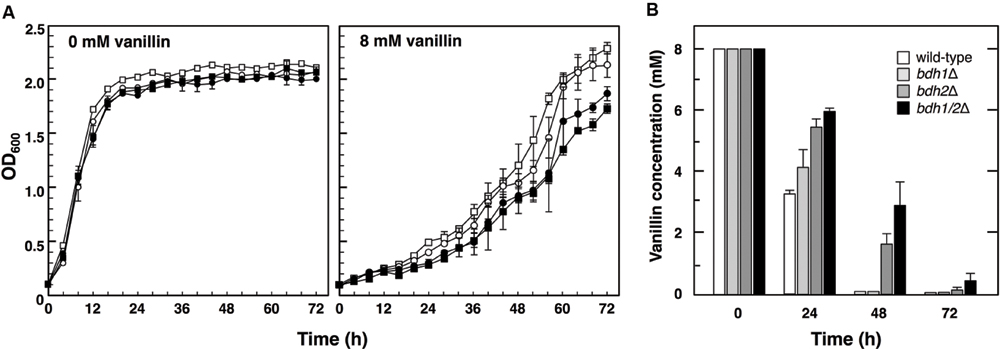
FIGURE 1. Growth of bdh1Δ, bdh2Δ, and bdh1Δbdh2Δ mutants in the presence of vanillin. (A) Cells were cultured at 28°C in SD medium containing 8 mM vanillin. Growth was monitored by measuring the optical density at 600 nm (OD600). Data are shown as the mean ± SE of three independent experiments. The strains examined were as follows: open squares, wild-type; open circles, bdh1Δ; closed circles, bdh2Δ; closed squares, bdh1Δbdh2Δ. (B) Changes in vanillin concentrations in culture media. The concentration of vanillin was determined by high performance liquid chromatography (HPLC). Yeast cells were cultured in SD medium containing 8 mM vanillin, and supernatants of the culture media were collected. Data are shown as the mean ± SE of three independent experiments.
In an attempt to further confirm the importance of Bdh1 and Bdh2, we examined changes in vanillin concentrations in medium throughout the cultivation (Figure 1B). After a 24-h cultivation, all mutants were less efficient at reducing vanillin levels than wild-type cells, indicating that Bdh1 and Bdh2 contribute to reductions in vanillin. While almost no vanillin was detected in the media of wild-type and bdh1Δ cells after a 48-h cultivation, approximately 2–3 mM vanillin remained in the media of bdh2Δ and bdh1Δbdh2Δ cells. These results indicate that Bdh2 more effectively contributes to reductions in vanillin levels than Bdh1.
Synthesis of the Bdh2 Protein Was Inducible with Severe Vanillin Stress
Since Bdh1 and Bdh2 both appear to be involved in reductions in vanillin and vanillin tolerance, we next examined whether vanillin affects the transcription of the BDH1 and BDH2 genes using quantitative real time-polymerase chain reaction (qRT-PCR). BDH1 and BDH2 mRNA levels were both significantly increased by the treatment with vanillin (6–15 mM; Figure 2A), indicating that both BDH genes were transcriptionally up-regulated by vanillin stress. Notably, the induction of the transcription of BDH2 by vanillin was markedly greater than that of BDH1 (Figure 2A).
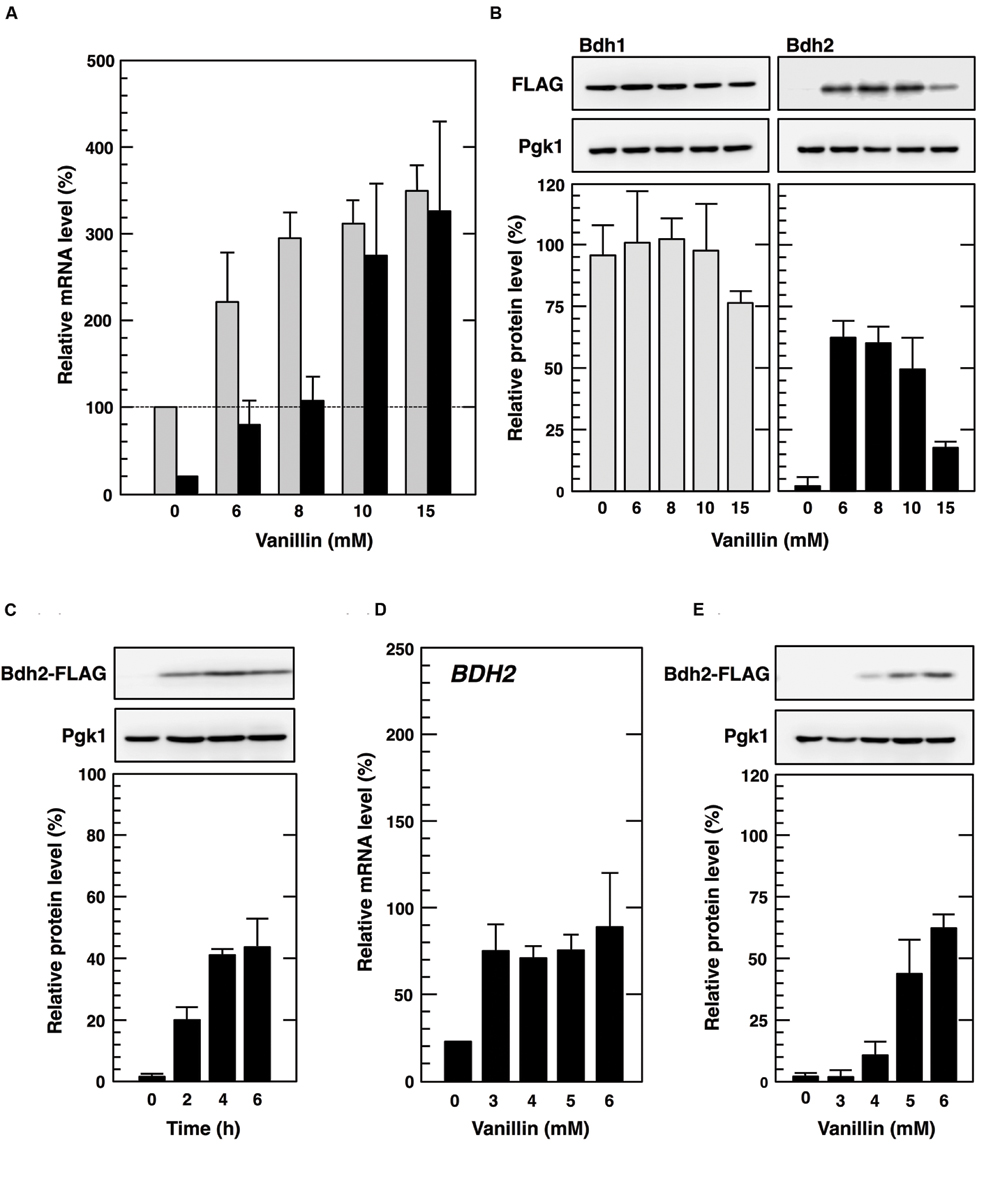
FIGURE 2. Expression of BDH1 and BDH2 genes under vanillin stress. (A,D) Cells in the exponential phase of growth were treated with vanillin for 1 h. BDH1 and BDH2 mRNA levels were analyzed by qRT-PCR. In order to compare mRNA expression levels, the mRNA level of each gene was normalized to that of ACT1. The BDH1 mRNA level in cells not treated with vanillin was considered to be 100%. Data are shown as the mean ± SE of three independent experiments. Gray bars, BDH1 mRNA; black bars, BDH2 mRNA. (B,C,E) Cells carrying a FLAG-tagged chromosomal copy of the BDH1 or BDH2 gene in the exponential phase of growth were treated with 6–15 mM vanillin for 1 h (B), 15 mM vanillin for 2–6 h (C), or 3–6 mM vanillin for 1 h (E). Bdh1-FLAG and Bdh2-FLAG levels were determined by Western blot analysis using an anti-FLAG antibody. Pgk1 was used as a loading control. The protein levels of Bdh1-FLAG and Bdh2-FLAG were normalized to that of Pgk1, and the intensity of the Pgk1 band of each lane was considered to be 100%. Data are shown as the mean ± SE of three independent experiments.
We reported that severe vanillin stress (greater than 7.5 mM) causes a rapid reduction in overall protein synthesis (Nguyen et al., 2014), and a previous study also verified the translational repression caused by vanillin (Nguyen et al., 2015). Therefore, we investigated the effects of severe vanillin stress on the protein synthesis of Bdh1 and Bdh2. Protein levels of Bdh1 were constitutive in the presence of varying concentrations of vanillin. In contrast, the Bdh2 protein was synthesized at levels lower than the detection limit under non-stressed conditions, whereas clear bands representing Bdh2 protein were detected after the treatments with vanillin (Figure 2B). Protein synthesis of Bdh2 was still detected following the treatment with 15 mM vanillin, which severely repressed bulk translation activity (Nguyen et al., 2014, 2015). Additionally, a prolonged treatment with 15 mM vanillin elevated Bdh2 protein levels (Figure 2C). These results clearly suggest that BDH2 mRNA can be translated even in the presence of high concentrations of vanillin, despite the repression of overall protein synthesis. On the other hand, Bdh2 protein synthesis was hardly up-regulated in the presence of low concentrations of vanillin (less than 4 mM) despite the increased mRNA levels of BDH2 (Figures 2D,E), clearly suggesting that the expression of the BDH2 gene was mainly inducible with severe, but not mild vanillin stress.
To verify that translation of BDH1 mRNA was repressed by vanillin, we also investigated protein levels of Bdh1 in cells treated with vanillin and CHX, a strong inhibitor of protein biosynthesis (Obrig et al., 1971), simultaneously. CHX had almost no effect on the transcriptional activation of BDH1 and BDH2 under vanillin stress (Figures 2A and 3A). Since CHX inhibits protein synthesis during the treatment with vanillin, induction of Bdh2 protein synthesis under vanillin stress was almost completely repressed (Figure 3B). On the other hand, CHX had almost no effect on the protein levels of Bdh1 after the treatment with vanillin for 1 h (Figures 2B and 3B). Since blocking the supply of newly synthesized protein had little effect on the Bdh1 levels, these results clearly indicate that translation of BDH1 mRNA was repressed under vanillin stress and also suggest that Bdh1 was relatively stable and its protein turnover was not so fast at least under our experimental conditions.
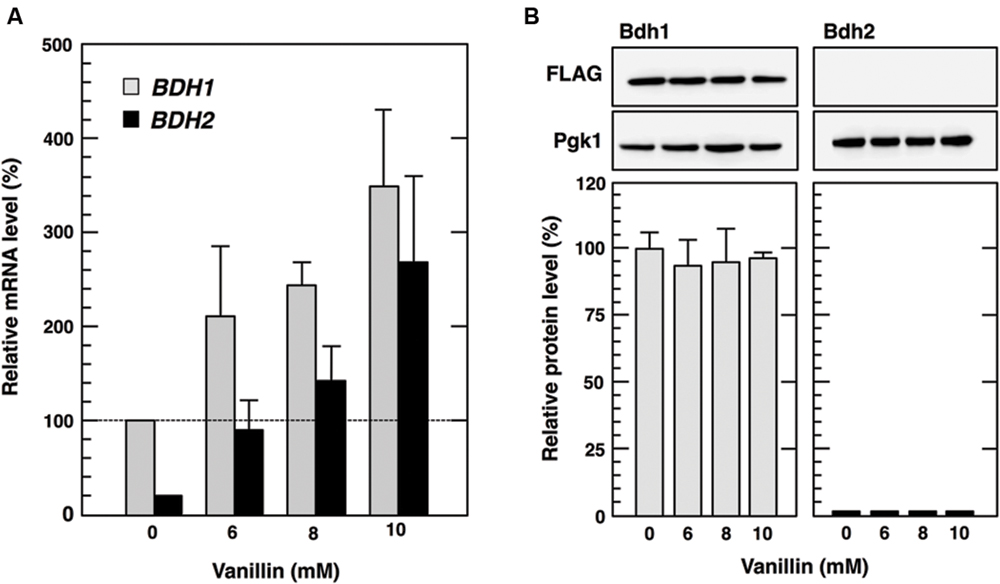
FIGURE 3. Cycloheximide had little effect on Bdh1 protein levels under vanillin stress. (A) Cells in the exponential phase of growth were treated with 6–10 mM vanillin and 0.1 mg/ml cycloheximide (CHX) for 1 h. BDH1 and BDH2 mRNA levels were analyzed by qRT-PCR. In order to compare mRNA expression levels, the mRNA level of each gene was normalized to that of ACT1. The BDH1 mRNA level in cells not treated with vanillin was considered to be 100%. Data are shown as the mean ± SE of three independent experiments. Gray bars, BDH1 mRNA; black bars, BDH2 mRNA. (B) Cells carrying a FLAG-tagged chromosomal copy of the BDH1 or BDH2 gene in the exponential phase of growth were treated with 6–10 mM vanillin and 0.1 mg/ml CHX for 1 h. Bdh1-FLAG and Bdh2-FLAG levels were determined by Western blot analysis using an anti-FLAG antibody. Pgk1 was used as a loading control. The protein levels of Bdh1-FLAG and Bdh2-FLAG were normalized to that of Pgk1, and the intensity of the Pgk1 band of each lane was considered to be 100%. Data are shown as the mean ± SE of three independent experiments.
Elimination of Vanillin Did Not Maintain the Synthesis of the Bdh2 Protein
We next examined the effects of the elimination of vanillin on the increases in Bdh2 protein levels caused by severe vanillin stress. After the treatment with 8 mM vanillin for 1 h, cells were transferred to fresh medium without vanillin. Changes in Bdh1 and Bdh2 protein levels were monitored during the recovery process (Figure 4). Although Bdh1 protein levels remained almost constant after the elimination of vanillin, those of Bdh2 were not maintained and gradually decreased during the recovery process and were finally lower than the detection limit after 5 h. These results suggest that the expression of BDH2 is repressed under non-severe vanillin stress conditions.
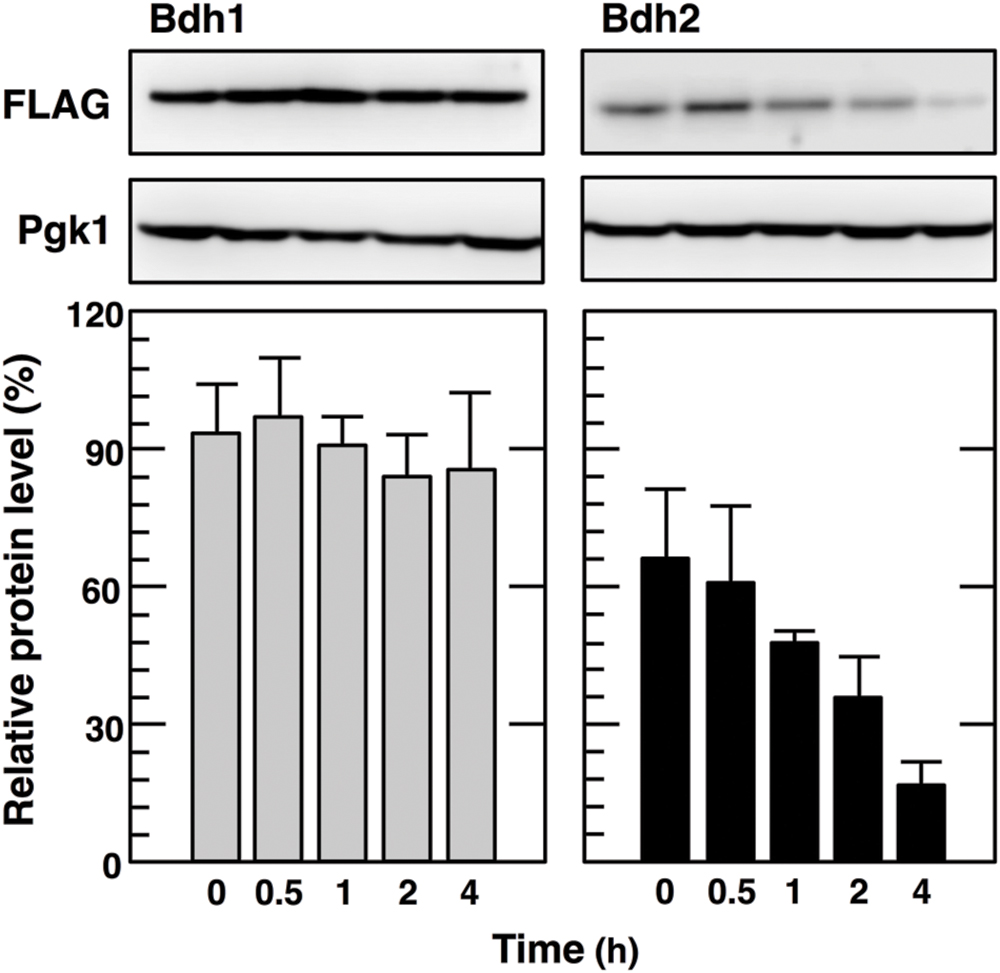
FIGURE 4. Bdh2 protein levels gradually decreased with the elimination of vanillin stress. Cells carrying a FLAG-tagged chromosomal copy of the BDH1 or BDH2 gene were treated with 8 mM vanillin for 1 h, washed with 50 mM potassium phosphate buffer (pH 6.8) twice, and then transferred to fresh SD medium without vanillin. Cells were incubated further for the indicated periods of time. Bdh1-FLAG and Bdh2-FLAG levels were determined by Western blot analysis using anti-FLAG antibody. Pgk1 was used as a loading control. The protein level of Bdh2-FLAG was normalized to that of Pgk1, and the intensity of the Pgk1 band in each lane was considered to be 100%. Data are shown as the mean ± SE of three independent experiments.
Overexpression of the BDH2 Gene Improved Yeast Vanillin Tolerance
We also investigated the effects of the overexpression of the BDH2 gene on tolerance to severe vanillin stress. Cells carrying the BDH2-overexpression plasmid (pRS426-BDH2) showed better growth in medium with very severe vanillin stress (the initial concentration of 12 mM) than cells carrying the control plasmid (pRS426; Figure 5A). On the other hand, the growth of cells carrying pRS423-BDH1 and pRS426 was slightly better than that of cells carrying empty plasmids only (pRS423 and pRS426) in the presence of 12 mM vanillin, indicating that the overexpression of the BDH1 gene only has a little effect on tolerance to severe vanillin stress. Additionally, vanillin levels in the culture medium were determined by HPLC (Figure 5B). The overexpression of BDH2 efficiently reduced of vanillin levels in the medium. The overexpression of BDH1 also facilitated reductions in vanillin levels; however, its effects were less than those of BDH2. These results strongly indicate that Bdh2 plays a very important role in vanillin detoxification and tolerance.
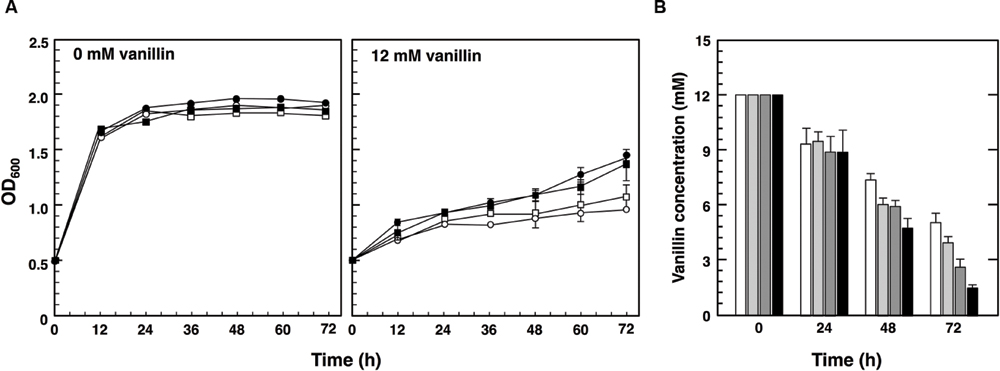
FIGURE 5. A high-copy plasmid containing the BDH2 gene improved vanillin tolerance in yeast cells. Cells were cultivated in SD medium containing 0 or 12 mM vanillin at 28°C. The plasmids introduced into cells were as follows: open squares and white bars, pRS423 and pRS426; open circles and light gray bars, pRS423-BDH1 and pRS426; closed circles and dark gray bars, pRS423 and pRS426-BDH2; closed squares and black bars, pRS423-BDH1 and pRS426-BDH2. (A) Growth was monitored by measuring optical density at 600 nm (OD600). Data are shown as the mean ± SE of three independent experiments. (B) Vanillin in SD medium was monitored by HPLC. Data are shown as the mean ± SE of three independent experiments.
The BDH2 Promoter Enabled Protein Synthesis under Severe Vanillin Stress
Previous studies reported that the promoters of small heat shock protein genes are sufficient for efficient protein synthesis upon glucose depletion, which causes the repression of bulk translation activity (Ashe et al., 2000; Zid and O’Shea, 2014). We also recently demonstrated that the ADH7 promoter enables effective protein synthesis, even under severe vanillin stress (Nguyen et al., 2015). Furthermore, we examined whether the BDH2 promoter enables protein synthesis under severe vanillin stress. The BDH2 promoter and terminator regions were fused to other ORFs such as GFP and ADH6. Cells carrying pRS316-BDH2Pro-GFP-BDH2Ter showed a clear GFP signal after the treatment with vanillin, however, the signal was hardly detected under non-stressed conditions (Figure 6A). Increases in the protein levels of GFP were verified by Western blot analysis (Figure 6B). Furthermore, Adh6-FLAG protein levels were increased by severe vanillin stress in cells carrying pRS316-BDH2Pro-ADH6-FLAG-BDH2Ter (Figure 6C). These results strongly indicate that the BDH2 promoter region enables the expression of its regulated genes under severe vanillin stress. Since the increased protein levels of Adh6-FLAG were also caused by severe vanillin stress in cells carrying pRS316-BDH2Pro-ADH6-FLAG-ADH6Ter or pRS316-BDH2Pro-ADH6-FLAG-BDH1Ter (Figure 6C), the BDH2 terminator region appears to have a negligible effect on preferential translation upon severe vanillin stress.
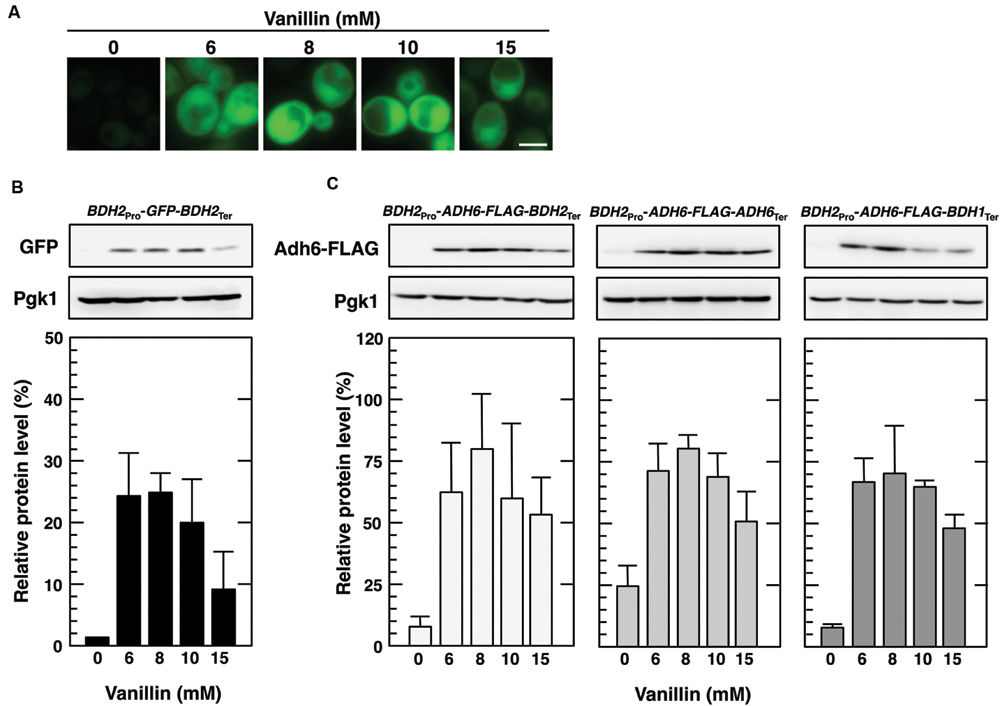
FIGURE 6. The BDH2 promoter region enabled protein synthesis of non-native genes in the presence of high concentrations of vanillin. (A,B) Cells carrying pRS316-BDH2Pro-GFP-FLAG-BDH2Ter in the exponential phase of growth were treated with vanillin for 1 h. (A) A fluorescence microscopic analysis was performed to monitor the synthesis of GFP. The exposure times were the same for all conditions. White bar indicates 3 μm. (B) GFP protein levels were determined by Western blot analysis using an anti-GFP antibody. (C) Cells carrying pRS316-BDH2Pro-ADH6-FLAG-BDH2Ter, pRS316-BDH2Pro-ADH6-FLAG-ADH6Ter, or pRS316-BDH2Pro-ADH6-FLAG-BDH1Ter in the exponential phase of growth were treated with vanillin for 1 h. Protein levels of Adh6-FLAG were determined by Western blot analysis using an anti-FLAG antibody. Pgk1 was used as a loading control. Protein levels of GFP and Adh6-FLAG were normalized to those of Pgk1, and the intensity of the Pgk1 band in each lane was considered to be 100%. Data are shown as the mean ± SE of three independent experiments.
Furfural also Induced Protein Synthesis under the Control of the BDH2 Promoter
During the process of bioethanol production from the lignocellulosic biomass, yeast cells are exposed to various inhibitory compounds in lignocellulose hydrolysates. Furfural is one of the major biomass conversion inhibitors from the lignocellulosic biomass and affects yeast fermentation activity (Klinke et al., 2004; Lu et al., 2009). Additionally, high concentrations of furfural have been shown to repress translation activity (Iwaki et al., 2013a). Therefore, we examined whether the protein synthesis of Bdh2 was induced under furfural stress conditions. After a treatment with 5–15 mM furfural for 3 h, BDH2 mRNA levels and the protein synthesis of Bdh2 were increased (Figures 7A,B). Furthermore, we investigated whether the BDH2 promoter enabled protein synthesis under furfural stress conditions. Cells carrying pRS316-BDH2Pro-ADH6-FLAG-BDH2Ter showed increased protein levels of Adh6-FLAG in the presence of furfural (Figure 7C). These results indicate that the BDH2 promoter is useful for efficient gene expression under furfural stress conditions as well as severe vanillin stress. On the other hand, the protein synthesis of Adh7 and ADH7 promoter-driven Adh6-FLAG (Nguyen et al., 2015) was not significantly induced by furfural (Figure 7).
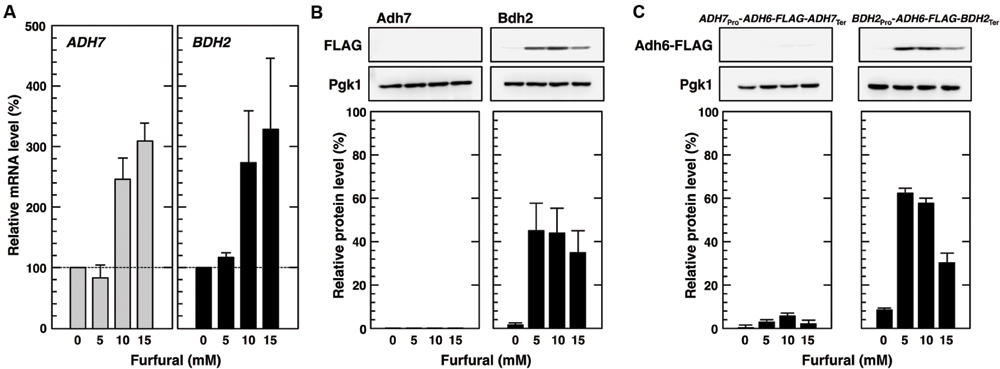
FIGURE 7. Furfural induced the expression of BDH2 promoter-driven genes. (A) Cells in the exponential phase of growth were treated with furfural (5–15 mM) for 3 h. ADH7 and BDH2 mRNA levels were analyzed by qRT-PCR. In order to compare mRNA expression levels, the mRNA level of each gene was normalized to that of ACT1. The mRNA level in cells not treated with furfural was considered to be 100%. Data are shown as the mean ± SE of three independent experiments. (B,C) Cells carrying a FLAG-tagged chromosomal copy of the ADH7 or BDH2 gene (B), and cells carrying pRS316-BDH2Pro-ADH6-FLAG-BDH2Ter or pRS316-ADH7Pro-ADH6-FLAG-ADH7Ter (C) in the exponential phase of growth were treated with furfural (5–15 mM) for 3 h. Adh7-FLAG, Bdh2-FLAG, and Adh6-FLAG protein levels were determined by Western blot analysis using an anti-FLAG antibody. Pgk1 was used as a loading control. FLAG-tagged protein levels were normalized to those of Pgk1, and the intensity of the Pgk1 band of each lane was considered to be 100%. Data are shown as the mean ± SE of three independent experiments.
Discussion
We herein demonstrated the importance of BDH1 and BDH2 genes in tolerance to vanillin stress even though they were not detected as genes involved in vanillin tolerance in previous genome-wide studies (Endo et al., 2008; Pereira et al., 2011). The null mutants of BDH genes were more sensitive to vanillin than the wild-type. bdh2Δ cells showed hyper-sensitivity to vanillin and reduced vanillin less efficiently than wild-type cells and bdh1Δ cells (Figure 1), thereby verifying the significant contribution of Bdh2 to vanillin tolerance. Therefore, cells carrying the BDH2-overexpression plasmid (pRS426-BDH2) showed a significant improvement in growth under very severe vanillin stress (12 mM; Figure 5). Bdh1 and Bdh2 as well as Adh6 and Adh7 belong to the superfamily of MDR (Ehsani et al., 2009). Since Adh6 and Adh7 catalyze the reduction of vanillin to vanillyl alcohol (Larroy et al., 2002), it appears to be quite feasible that Bdh1 and Bdh2 also catalyze the reduction of vanillin to vanillyl alcohol.
We demonstrated that the BDH1 and BDH2 genes exhibited different protein expression patterns in response to vanillin stress. Bdh1 protein levels were almost constitutive and did not reflect the increased levels of BDH1 mRNA upon severe vanillin stress (Figure 2). Since blocking protein synthesis using CHX had almost no effect on the Bdh1 protein levels (Figure 3), it is likely that translation of BDH1 mRNA was repressed under severe vanillin stress. On the other hand, BDH2 mRNA could be translated upon severe vanillin stress, and Bdh2 levels were increased even in the presence of a very high concentration of vanillin (15 mM; Figures 2B,C). These results revealed that the expression of the BDH2 gene was inducible upon severe vanillin stress. The synthesis of the Bdh2 protein was not induced by low concentrations of vanillin (1–4 mM) in spite of the transcriptional activation of BDH2 (Figures 2D,E), suggesting that the expression of BDH2 under 1–4 mM vanillin stress is repressed in a posttranscriptional manner, which still remains to be clarified. Since Bdh2 levels were gradually reduced after the elimination of vanillin stress (Figure 4), protein degradation may also be important to maintain the low levels of Bdh2 under non-stress and mild vanillin stress conditions.
We recently reported that the synthesis of the Adh7 protein but not the Adh6 protein was induced by severe vanillin stress, despite both ADH genes being transcriptionally up-regulated (Nguyen et al., 2015). Additionally, the expression of ADH7 was not induced by mild vanillin stress (1–4 mM; Nguyen et al., 2015). Therefore, it is clear that the expression pattern of BDH2 closely resembles that of ADH7 in response to vanillin stress. The induction of BDH2 and ADH7 expression by severe vanillin stress appears to be crucial for yeast tolerance to high concentrations of vanillin. On the other hand, the constitutive expression of BDH1 and ADH6 may play a role in the initial stage of the response to vanillin, and yeast cells are presumably able to cope with mild vanillin stress without the expression of BDH2 and ADH7. Adh6 and Adh7 are NADP(H)-dependent MDRs, while Bdh1 and Bdh2 are NAD(H)-dependent MDRs (González et al., 2000; Ehsani et al., 2009). The use of both kinds of reductants for vanillin reduction appears to be a strong advantage for maintaining the robustness of the yeast response to vanillin stress.
It has been previously reported that specific promoter sequences affected the efficiency of translation under glucose depletion, which causes severe bulk translation repression (Zid and O’Shea, 2014). They demonstrated that the transcripts of genes containing heat shock elements (HSEs) in their promoter regions may be preferentially translated during glucose starvation. It is also conceivable that BDH2 and ADH7 also have specific promoter sequences that enable preferential translation under severe vanillin stress. HSEs (5′-NGAANNTTCN-3′ or 5′-NTTCNNGAAN-3′) have been detected in BDH2 promoter sequences (from positions -389 to -378 and -119 to -108, relative to the translation initiation ATG codon) and ADH7 promoter sequences (-473 to -464; Bonner et al., 1994). On the other hand, no HSE has been detected in the promoter regions of BDH1 and ADH6. HSEs in the promoters of BDH2 and ADH7 may also contribute to preferential translation under severe vanillin stress as well as glucose depletion conditions. In mammalian cells, the recruitment of the translation elongation factor eEF1A to the HSEs of the HSP70 gene was found to promote the nuclear export and translation of HSP70 mRNA during heat shock (Vera et al., 2014). Similar to mammalian eEF1A, some kinds of factor(s) that facilitate translation under severe stress conditions may be recruited to HSEs in yeast cells.
In addition to the ADH7 promoter, the ability of the BDH2 promoter to overcome vanillin-induced translation repression appears to be useful for improving the efficiency of bioethanol production. Since the expression of BDH2 but not ADH7 was also induced by furfural (Figure 7), the BDH2 promoter may be more practical than the ADH7 promoter for efficient bioethanol production. The manipulation of gene expression using the BDH2 promoter may represent an effective approach in the breeding of robust and optimized yeast strains for bioethanol production. For example, the expression of UDP-glucoside transferase using a BDH2 promoter may efficiently reduce vanillin levels through the conversion of vanillin to vanillin β-D-glucoside (Hansen et al., 2009; Brochado et al., 2010; Brochado and Patil, 2013; Gallage and Møller, 2015), and vanillin β-D-glucoside may be collected and re-converted into vanillin after the completion of fermentation. Although further studies are needed in order to construct an ideal high-performance yeast for bioethanol production from the lignocellulosic biomass, the results of the present study provide useful information on yeast responses to vanillin and insights in order to improve the vanillin tolerance and fermentation efficiency of yeast cells.
Author Contributions
YI did most of experiments and prepared the manuscript. TN and SK did several important experiments. SI did several experiments and mainly prepared the manuscript.
Funding
Fundings from the Japan Society for the Promotion of Science 26292039, 15K0737, and 20260614 are for SI, and 15J08781 is for TN.
Conflict of Interest Statement
The authors declare that the research was conducted in the absence of any commercial or financial relationships that could be construed as a potential conflict of interest.
Acknowledgment
We are grateful to A. Iwaki for her technical support.
References
Ashe, M. P., De Long, S. K., and Sachs, A. B. (2000). Glucose depletion rapidly inhibits translation initiation in yeast. Mol. Biol. Cell 11, 833–848. doi: 10.1091/mbc.11.3.833
Bonner, J. J., Ballou, C., and Fackenthal, D. L. (1994). Interactions between DNA-bound trimers of the yeast heat shock factor. Mol. Cell. Biol. 14, 501–508. doi: 10.1128/MCB.14.1.501
Brochado, A. R., Matos, C., Møller, B. L., Hansen, J., Mortensen, U. H., and Patil, K. R. (2010). Improved vanillin production in baker’s yeast through in silico design. Microb. Cell Fact. 9, 84–98. doi: 10.1186/1475-2859-9-84
Brochado, A. R., and Patil, K. R. (2013). Overexpression of O-methyltransferase leads to improved vanillin production in baker’s yeast only when complemented with model-guided network engineering. Biotechnol. Bioeng. 110, 656–659. doi: 10.1002/bit.24731
Ehsani, M., Fernández, M. R., Biosca, J. A., and Dequin, S. (2009). Reversal of coenzyme specificity of 2,3-butanediol dehydrogenase from Saccharomyces cerevisiae and in vivo function analysis. Biotechnol. Bioeng. 104, 381–389. doi: 10.1002/bit.22391
Endo, A., Nakamura, T., Ando, A., Tokuyasu, K., and Shima, J. (2008). Genome-wide screening of the genes required for tolerance to vanillin, which is a potential inhibitor of bioethanol fermentation, in Saccharomyces cerevisiae. Biotechnol. Biofuels 1, 3. doi: 10.1186/1754-6834-1-3
Erasmus, D. J., and van Vuuren, H. J. J. (2009). Genetic basis for osmosensitivity and genetic instability of the wine yeast Saccharomyces cerevisiae VIN7. Am. J. Enol. Vitic. 60, 145–154.
Fitzgerald, D. J., Stratford, M., and Narbad, A. (2003). Analysis of the inhibition of food spoilage yeasts by vanillin. Int. J. Food Microbiol. 86, 113–122. doi: 10.1016/S0168-1605(03)00059-X
Gallage, N. J., and Møller, B. L. (2015). Vanillin-bioconversion and bioengineering of the most popular plant flavor and its de novo biosynthesis in the vanilla orchid. Mol. Plant 8, 40–57. doi: 10.1016/j.molp.2014.11.008
González, E., Fernández, M. R., Larroy, C., Solà, L., Pericàs, M. A., Parés, X., et al. (2000). Characterization of a (2R,3R)-2,3-butanediol dehydrogenase as the Saccharomyces cerevisiae YAL060W gene product. Disruption and induction of the gene. J. Biol. Chem. 275, 35876–35885. doi: 10.1074/jbc.M003035200
González, E., Fernández, M. R., Marco, D., Calam, E., Sumoy, L., Parés, X., et al. (2010). Role of Saccharomyces cerevisiae oxidoreductases Bdh1p and Ara1p in the metabolism of acetoin and 2,3-butanediol. Appl. Environ. Microbiol. 76, 670–679. doi: 10.1128/AEM.01521-09
Hansen, E. H., Møller, B. L., Kock, G. R., Bünner, C. M., Kristensen, C., Jensen, O. R., et al. (2009). De novo biosynthesis of vanillin in fission yeast (Schizosaccharomyces pombe) and baker’s yeast (Saccharomyces cerevisiae). Appl. Environ. Microbiol. 75, 2765–2774. doi: 10.1128/AEM.02681-08
Helle, S., Cameron, D., Lam, J., White, B., and Duff, S. (2003). Effect of inhibitory compounds found in biomass hydrolysates on growth and xylose fermentation by a genetically engineered strain of S. cerevisiae. Enzyme Microb. Technol. 33, 786–792. doi: 10.1016/S0141-0229(03)00214-X
Iwaki, A., Kawai, T., Yamamoto, Y., and Izawa, S. (2013a). Biomass conversion inhibitors furfural and 5-hydroxymethylfurfural induce formation of messenger RNP granules and attenuate translation activity in Saccharomyces cerevisiae. Appl. Environ. Microbiol. 79, 1661–1667. doi: 10.1128/AEM.02797-12
Iwaki, A., Ohnuki, S., Suga, Y., Izawa, S., and Ohya, Y. (2013b). Vanillin inhibits translation and induces messenger ribonucleoprotein (mRNP) granule formation in Saccharomyces cerevisiae: application and validation of high-content, image-based profiling. PLoS ONE 8:e61748. doi: 10.1371/journal.pone.0061748
Jonsson, L. J., Alriksson, B., and Nilvebrant, N. O. (2013). Bioconversion of lignocellulose: inhibitors and detoxicification. Biotechnol. Biofuels 6, 16. doi: 10.1186/1754-6834-6-16
Kahana, J. A., Schlenstedt, G., Evanchuk, D. M., Geiser, J. R., Hoyt, M. A., and Silver, P. A. (1998). The yeast dynactin complex is involved in partitioning the mitotic spindle between mother and daughter cells during anaphase B. Mol. Biol. Cell 9, 1741–1756. doi: 10.1091/mbc.9.7.1741
Kato, K., Yamamoto, Y., and Izawa, S. (2011). Severe ethanol stress induces assembly of stress granules in Saccharomyces cerevisiae. Yeast 28, 339–347. doi: 10.1002/yea.1842
Kitada, K., Yamaguchi, E., and Arisawa, M. (1995). Cloning of the Candida glabrata TRP1 and HIS3 genes, and construction of their disruptant strains by sequential integrative transformation. Gene 165, 203–206. doi: 10.1016/0378-1119(95)00552-H
Klinke, H. B., Thomsen, A. B., and Ahring, B. K. (2004). Inhibition of ethanol-producing yeast and bacteria by degradation products produced during pre-treatment of biomass. Appl. Microbiol. Biotechnol. 66, 10–26. doi: 10.1007/s00253-004-1642-2
Larroy, C., Parés, X., and Biosca, J. A. (2002). Characterization of a Saccharomyces cerevisiae NADP(H)-dependent alcohol dehydrogenase (ADHVII), a member of the cinnamyl alcohol dehydrogenase family. Eur. J. Biochem. 269, 5738–5745. doi: 10.1046/j.1432-1033.2002.03296.x
Lu, X., Yamauchi, K., Phaiiboonsilpa, N., and Saka, S. (2009). Two-step hydrolysis of Japanese beech as treated by semi-flow hot-compressed water. J. Wood Sci. 55, 367–375. doi: 10.1007/s10086-009-1040-6
Nguyen, T. T. M., Iwaki, A., and Izawa, S. (2015). The ADH7 promoter of Saccharomyces cerevisiae is vanillin inducible and enables mRNA translation under severe vanillin stress. Front. Microbiol. 6:1390. doi: 10.3389/fmicb.2015.01390
Nguyen, T. T. M., Kitajima, S., and Izawa, S. (2014). Importance of glucose-6-phosphate dehydrogenase (G6PDH) for vanillin tolerance in Saccharomyces cerevisiae. J. Biosci. Bioeng. 118, 263–269. doi: 10.1016/j.jbiosc.2014.02.025
Nordling, E., Jörnvall, H., and Persson, B. (2002). Medium-chain dehydrogenases/reductases (MDR) family characterizations including genome comparisons and active site modeling. Eur. J. Biochem. 269, 4267–4276. doi: 10.1046/j.1432-1033.2002.03114
Obrig, T. G., Culp, W. J., McKeehan, W. L., and Hardesty, B. (1971). The mechanism by which cycloheximide and related glutarimide antibiotics inhibit peptide synthesis on reticulocyte ribosomes. J. Biol. Chem. 246, 174–181.
Palmqvist, E., and Hahn-Hägerdal, B. (2000). Fermentation of lignocellulosic hydrolysates. I: inhibition and detoxification. Bioresour. Technol. 74, 17–24. doi: 10.1016/S0960-8524(99)00160-1
Pereira, F. B., Guimaraes, P. M., Gomes, D. G., Mira, N. P., Teixeira, M. C., Sá-Correia, I., et al. (2011). Identification of candidate genes for yeast engineering to improve bioethanol production in very high gravity and lignocellulosic biomass industrial fermentations. Biotechnol. Biofuels 4, 57. doi: 10.1186/1754-6834-4-57
Schmitt, M. E., Brown, T. A., and Trumpower, B. L. (1990). A rapid and simple method for preparation of RNA from Saccharomyces cerevisiae. Nucleic Acids Res. 18, 3091–3092. doi: 10.1093/nar/18.10.3091
Shen, Y., Li, H., Wang, X., Zhang, Z., Hou, J., Wang, L., et al. (2014). High vanillin tolerance of an evolved Saccharomyces cerevisiae strain owing to its enhanced vanillin reduction and antioxidative capacity. J. Ind. Microbiol. Biotechnol. 41, 1637–1645. doi: 10.1007/s10295-014-1515-3
Sikorski, R. S., and Hieter, P. (1989). A system of shuttle vectors and yeast host strains designed for efficient manipulation of DNA in Saccharomyces cerevisiae. Genetics 122, 19–27.
Subramanian, M., Qiao, W., Khanam, N., Wilkins, O., Der, S. D., Lalich, J. D., et al. (2005). Transcriptional regulation of the one-carbon metabolism regulon in Saccharomyces cerevisiae by Bas1p. Mol. Microbiol. 57, 53–69. doi: 10.1111/j.1365-2958.2005.04663.x
Takahashi, T., Satake, S., Hirose, K., Hwang, G.-W., and Naganuma, A. (2011). A screening for essential cell growth-related genes involved in arsenite toxicity in Saccharomyces cerevisiae. J. Toxicol. Sci. 36, 859–861. doi: 10.2131/jts.36.859
Vera, M., Pani, B., Griffiths, L. A., Muchardt, C., Abbott, C. M., Singer, R. H., et al. (2014). The translation elongation factor eEF1A1 couples transcription to translation during heat shock response. Elife 3:e03164. doi: 10.75554/eLife.03164
Keywords: BDH1, BDH2, ADH7, vanillin, furfural, translation repression, lignocellulosic biomass, bioethanol production
Citation: Ishida Y, Nguyen TTM, Kitajima S and Izawa S (2016) Prioritized Expression of BDH2 under Bulk Translational Repression and Its Contribution to Tolerance to Severe Vanillin Stress in Saccharomyces cerevisiae. Front. Microbiol. 7:1059. doi: 10.3389/fmicb.2016.01059
Received: 27 April 2016; Accepted: 23 June 2016;
Published: 06 July 2016.
Edited by:
Vijai Kumar Gupta, NUI Galway, IrelandReviewed by:
Jun Ishii, Kobe University, JapanTomoyuki Nakagawa, Gifu University, Japan
Daisuke Watanabe, Nara Institute of Science and Technology, Japan
Copyright © 2016 Ishida, Nguyen, Kitajima and Izawa. This is an open-access article distributed under the terms of the Creative Commons Attribution License (CC BY). The use, distribution or reproduction in other forums is permitted, provided the original author(s) or licensor are credited and that the original publication in this journal is cited, in accordance with accepted academic practice. No use, distribution or reproduction is permitted which does not comply with these terms.
*Correspondence: Shingo Izawa, dGhpb3JlZG94aW5Aa2l0LmFjLmpw