- 1Department of Environmental Protection, Estación Experimental del Zaidín-Consejo Superior de Investigaciones Científicas, Granada, Spain
- 2Section of Molecular Microbiology, Department of Molecular Cell Biology, VU University Amsterdam, Amsterdam, Netherlands
Gene regulation in bacteria is primarily controlled at the level of transcription initiation by modifying the affinity of the RNA polymerase (RNAP) for the promoter. This control often occurs through the substitution of the RNAP sigma (σ) subunit. Next to the primary σ factor, most bacteria contain a variable number of alternative σ factors of which the extracytoplasmic function group (σECF) is predominant. Pseudomonas aeruginosa contains nineteen σECF, including the virulence regulator σVreI. σVreI is encoded by the vreAIR operon, which also encodes a receptor-like protein (VreA) and an anti-σ factor (VreR). These three proteins form a signal transduction pathway known as PUMA3, which controls expression of P. aeruginosa virulence functions. Expression of the vreAIR operon occurs under inorganic phosphate (Pi) limitation and requires the PhoB transcription factor. Intriguingly, the genes of the σVreI regulon are also expressed in low Pi despite the fact that the σVreI repressor, the anti-σ factor VreR, is also produced in this condition. Here we show that although σVreI is partially active under Pi starvation, maximal transcription of the σVreI regulon genes requires the removal of VreR. This strongly suggests that an extra signal, probably host-derived, is required in vivo for full σVreI activation. Furthermore, we demonstrate that the activity of σVreI is modulated not only by VreR but also by the transcription factor PhoB. Presence of this regulator is an absolute requirement for σVreI to complex the DNA and initiate transcription of the PUMA3 regulon. The potential DNA binding sites of these two proteins, which include a pho box and −10 and −35 elements, are proposed.
Introduction
Regulation of gene expression allows bacteria to adapt rapidly to alterations in their environment. This regulation occurs primarily at the level of transcription initiation by modifying promoter recognition of the RNA polymerase (RNAP) holoenzyme. The RNAP holoenzyme of bacteria comprises a five-subunit core enzyme (RNAPc; subunit composition α2ββ′ω) and a dissociable sigma (σ) subunit (Murakami and Darst, 2003). The σ factor contains most promoter recognition determinants and confers promoter specificity to the RNAP. All bacteria contain a primary σ factor (i.e., σ70) that recognizes similar target promoter sequences and controls expression of genes required for general functions. Promoter recognition by σ70 is often modulated by transcription factors that either enhance or inhibit such recognition and therefore gene transcription (Ishihama, 2000; Martinez-Antonio et al., 2006). In addition, most bacteria contain several alternative σ factors that recognize alternative promoter sequences and activate expression of functions required only under specific circumstances (Ishihama, 2000). Therefore, the promoter recognition of the RNAP is modulated first by substitution of the σ subunit and secondly by the interaction with transcription factors.
The largest and most diverse group of bacterial alternative σ factors is the Group IV, which consists of the so-called extracytoplasmic function (ECF) σ factors (σECF). These σ factors control expression of important bacterial functions such as stress responses, iron uptake and pathogenicity (Lonetto et al., 1994; Helmann, 2002; Bastiaansen et al., 2012; Mascher, 2013). Both expression and activation of σECF are tightly regulated processes that usually occur in response to environmental signals. The post-translational control of σECF is carried out by anti-σ factors that bind to and sequester the σECF, which is only released and activated in the presence of an inducing signal. The functional unit of the σECF-dependent signaling is therefore formed by the σECF and its cognate anti-σ factor, and the genes encoding these two proteins are normally co-transcribed. This signal transduction cascade resembles that of the two-component systems in which a membrane bound histidine kinase controls the activity of a transcription factor (known as response regulator) that also mediates a cellular response through differential expression of target genes (Stock et al., 2000). However, whereas activation of two-components system involves phosphotransfer reactions, liberation and activation of the σECF in response to the inducing signal requires the targeted proteolysis of the anti-σ factor (Qiu et al., 2007; Ades, 2008; Draper et al., 2011; Bastiaansen et al., 2014, 2015).
A high number of σECF in a bacterial genome usually reflects the diversity of the bacterial living environment (Staroń et al., 2009). The human opportunistic pathogen Pseudomonas aeruginosa, which thrives in diverse habitats ranging from soil to the human airways, encodes nineteen σECF (Visca et al., 2002; Llamas et al., 2008, 2014). Most P. aeruginosa σECF belong to the iron-starvation group (Leoni et al., 2000) and initiate transcription of iron uptake functions. Expression of these σ factors is usually regulated by iron through the ferric-uptake regulator (Fur) repressor, and their function is normally activated by an iron carrier (i.e., siderophore) via a regulatory pathway known as cell-surface signaling (CSS) (Llamas et al., 2014). Apart from the σECF/anti-σ factor pair, the CSS cascade also involves an outer membrane receptor of the TonB-dependent family (Llamas et al., 2014). CSS receptors usually have a dual function: transduce the presence of the signal to the anti-σ factor which activates the σECF in the cytosol, and mediate the uptake of the inducing signal (i.e., siderophore) (Llamas et al., 2014). Moreover, P. aeruginosa contains two CSS σ factors that control expression of virulence genes (Llamas et al., 2014). This includes σPvdS, which responds to P. aeruginosa's own siderophore pyoverdine and regulates the production of exotoxin A (toxA) and PrpL endoprotease (prpL) (Lamont et al., 2002). The second example is σVreI, which regulates expression of several potential virulence factors, including secreted proteins and secretion systems (Figure 1A), and induces P. aeruginosa virulence (Llamas et al., 2009). σVreI is encoded by the second gene of the vreAIR operon, which also encodes a CSS-like receptor (VreA) and an anti-σ factor (VreR) (Llamas et al., 2009). These three proteins form the PUMA3 CSS system (Llamas et al., 2009, 2014). This system has a number of features that differentiate it from most CSS systems. First, the CSS receptor VreA lacks the C-terminal β-barrel domain typical of TonB-dependent receptors and seems to be located in the periplasm instead of in the outer membrane (Llamas et al., 2009). This suggests that this protein is only involved in signal transduction and not in the uptake of the signal molecule. In addition, expression of the vreAIR operon is not regulated by iron and Fur but by phosphate (Pi) and the PhoB transcription factor (Faure et al., 2013). In P. aeruginosa, the level of Pi in the environment is sensed by the phosphate-specific ABC transport Pst system, which under Pi starvation conditions mediates Pi transport and activates the PhoR-PhoB two-component system (Lamarche et al., 2008). Upon activation, the PhoR histidine kinase promotes phosphorylation of its cognate DNA-binding response regulator PhoB. Phosphorylated PhoB controls the expression of a large set of genes by binding as a dimer to a pho box, a 22-bp specific DNA sequence in the promoter region of the PhoB regulon genes (Blanco et al., 2002). Interestingly, the genes belonging to the PUMA3 regulon are also expressed in response to Pi starvation in a σVreI-dependent manner (Faure et al., 2013). This is an intriguing observation since in this condition the genes encoding both σVreI and its cognate repressor, the VreR anti-σ factor, are expressed. This study was conducted to elucidate how the activity of σVreI is modulated in Pi starvation conditions.
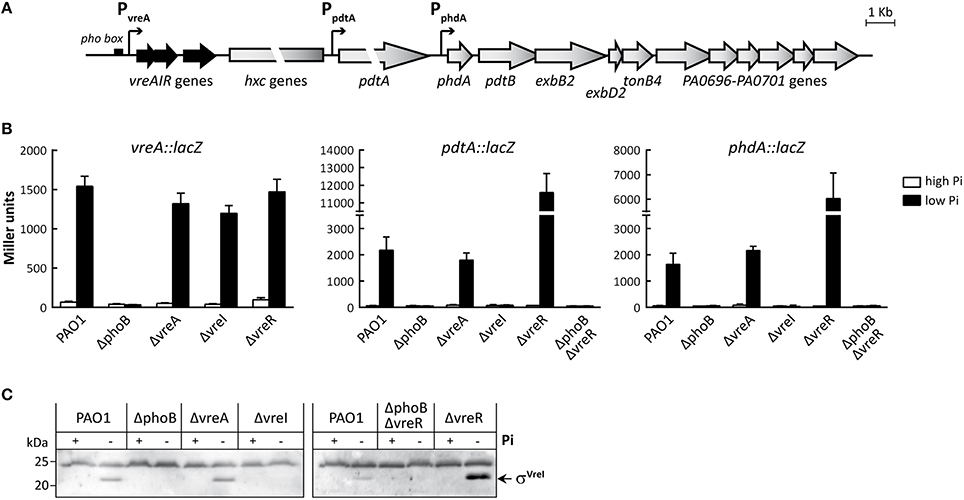
Figure 1. Genetic organization and expression of the PUMA3 regulon. (A) Transcriptional organization of the vreAIR locus encoding the PUMA3 CSS system (black) and the downstream PUMA3-regulated genes (gray). The big arrows represent the different genes, their relative sizes, and their transcriptional orientation. Below each arrow, the name of the gene, gene cluster or the PA number (http://www.pseudomonas.com) is indicated. Small arrows represent the promoters identified within the PUMA3 system (Llamas et al., 2009; Faure et al., 2013). The pho box present in the vreA promoter (Faure et al., 2013) is also indicated. (B) β-galactosidase activity of the P. aeruginosa PAO1 wild-type strain and the indicated mutants bearing the pMP220-derivated plasmids containing the indicated lacZ fusion (Table 1) after 18 h of growth in high or low Pi conditions. (C) Detection of σVreI in P. aeruginosa PAO1 wild-type strain and the indicated mutants. Proteins were detected by Western-blot using a polyclonal anti-VreI antibody. The positions of the molecular size marker (in kDa) and of the σVreI protein are shown.
Materials and Methods
Bacterial Strains and Growth Conditions
Strains used in this study are listed in Table 1. Bacteria were grown in liquid LB (Sambrook et al., 1989) or in 0.3% (w/v) proteose peptone (DIFCO) containing 100 mM HEPES, 20 mM NH4Cl, 20 mM KCl, 3.2 mM MgCl2, and 0.4% (w/v) glucose (pH 7.2), without (low Pi) or with 10 mM KH2PO4 (high Pi), on a rotatory shaker at 37°C and 200 rpm. When required, 1 mM isopropyl β-D-1-thiogalactopyranoside (IPTG) was added to the medium to induce full expression from the pMMB67EH Ptac promoter. Antibiotics were used at the following final concentrations (μg ml−1): ampicillin (Ap), 100; gentamicin (Gm), 20; kanamycin (Km), 50; nalidixic acid (Nal), 10; piperacillin (Pip), 25; rifampicin (Rif) 10; streptomycin (Sm), 100; tetracycline (Tc), 20.
Plasmids Construction and Molecular Biology
Plasmids used are described in Table 1 and primers listed in Table S1. PCR amplifications were performed using Phusion® Hot Start High-Fidelity DNA Polymerase (Finnzymes) or Expand High Fidelity DNA polymerase (Roche). Nucleotide substitutions or deletions in the pdtA and phdA promoters were generated by whole plasmid PCR site-directed mutagenesis (Fisher and Pei, 1997) with a pair of complementary mutagenic primers using the pTOPO-Pr0690 and pTOPO-Pr0691b plasmids (Table 1), respectively, as templates. After mutagenesis, the promoters were subcloned in the pMP220 plasmid as EcoRI-XbaI (PpdtA) or BglII-KpnI (PphdA) restriction fragments. All constructs were confirmed by DNA sequencing and transferred to P. aeruginosa by electroporation (Choi et al., 2006). Construction of null mutants was performed by allelic exchange using the suicide vector pKNG101 as described before (Bastiaansen et al., 2014). Southern blot analyses to confirm the chromosomal gene deletion were performed as described (Llamas et al., 2000).
Enzyme Assay
β-galactosidase activities in soluble cell extracts were determined using o-nitrophenyl-b-D-galactopyranoside (ONPG) (Sigma-Aldrich) as described before (Llamas et al., 2006). Each condition was tested in duplicate in at least three biologically independent experiments and the data given are the average with error bars representing standard deviation (SD). Activity is expressed in Miller units.
Production of α-VreI and α-VreR Antibodies
To obtain relatively pure protein recombinant, VreI and VreR were expressed as an insoluble protein in E. coli TOP10F' using an aggregation tag. Inclusion bodies were isolated as followed: bacterial cells were resuspended in 5 ml solution buffer (50 mM Tris-Hcl, 25% sucrose, 1 mM NaEDTA, 10 mM DTT, 0.4 mg/ml lysozyme, 20 μg/ml DNAse I and 2 mM MgCl2). Following sonication, 5 ml lysis buffer was added (50 mM Tris-HCl, 1% Triton X-100, 1% Na deoxycholate, 100 mM NaCl, 10 mM DTT) and the suspension was incubated on ice for 1 h. After a snap freezing and thawing cycle the total amount of NaEDTA and MgCl2 was increased to 15 mM and 6 mM, respectively. Inclusion bodies were pelleted at 11.000 × g for 20 min at 4°C and washed with a buffer containing 50 mM Tris-HCl, 1% Triton X-100, 100 mM NaCl, 1 mM NaEDTA and 1 mM DTT. Following sonication to obtain a homogenous suspension and another centrifugation step, washing was performed in the same buffer omitting Triton X-100. Subsequently, inclusion bodies were boiled in SDS-PAGE sample buffer. Proteins were analyzed by SDS-PAGE containing 12% (w/v) acrylamide and the VreI and VreR proteins were excised from the gel following an imidazole-zinc staining. The proteins were electroeluted out of the gel and purified VreI and VreR were sent to Innovagen (Sweden) for antibody production. Rabbits were immunized at day 0 and subsequently given boosters at days 14, 28, 49, and 70. At day 84 rabbits were sacrificed and serum was isolated. Prior Western-blot, serum was concentrated using 30K centrifugal filter units (Millipore) at 4000 rpm for 15 min.
SDS-PAGE and Western-Blot
Bacteria were grown until late log phase and pelleted by centrifugation. Samples were normalized according to the OD660 of the culture, solubilized in Laemmli buffer and heated for 10 min at 95°C. Proteins were separated by SDS-PAGE containing 12 or 15% (w/v) acrylamide and electrotransferred to nitrocellulose membranes. Ponceau S staining was performed as a loading control. Immunodetection was realized using polyclonal antibodies directed against the σVreI or the VreR proteins, or a monoclonal antibody directed against the influenza hemagglutinin epitope (HA.11, Covance). The second antibody, either the horseradish peroxidase-conjugated goat anti-rabbit IgG (Sigma-Aldrich) or the horseradish peroxidase-conjugated rabbit anti-mouse (DAKO), was detected using the SuperSignal® West Femto Chemiluminescent Substrate (Thermo Scientific). Blots were scanned and analyzed using the Quantity One version 4.6.7 (Bio-Rad).
RNA Preparation
P. aeruginosa cells were grown until late exponential phase in low or high phosphate medium. Total bacterial RNA was isolated by the hot phenol method using the TRI® Reagent protocol (Ambion) as described before (Llamas et al., 2008). RNA quantity and quality was assessed by UV absorption at 260 nm in a ND-1000 Spectrophotometer (NanoDrop Technologies, USA).
5′ RACE
The transcription start points were determined using the 5′ RACE System for Rapid Amplification of cDNA Ends (Invitrogen). RNA isolated from P. aeruginosa PAO1 or ΔvreR cells grown in low Pi was used as the template for 5′ RACE analysis. The primers used in this analysis are shown in Table S1. The 5′ RACE reactions were performed as recommended by the manufacturer and analyzed by agarose gel electrophoresis to assess purity and product size. Single cDNA bands were obtained for the reactions and, upon purification, were sequenced using a nested gene-specific primer to locate the 5′ end of the transcript. The sequencing results of the 5′ RACE product were aligned with the P. aeruginosa PAO1 genome sequence.
Primer Extension Analysis
Primer extension analyses were done basically as described by Marques et al. (1994) using 12 μg of total RNA in each reaction. About 105 cpm of [γ-32P]-labeled 5′-end oligonucleotides (Table S1) was used as primers in extension reactions. The cDNA products obtained after the reverse transcriptase reaction were separated and analyzed in urea-polyacrylamide sequencing gels. Visualization of the gels was performed using the Fujifilm imaging plate BAS-MS 2040.
PhoB and σVreI Protein Purification
His-tagged PhoB and σVreI proteins were produced in E. coli BL21 from the pET-phoB and pET-vreI plasmids, respectively, and purified by affinity chromatography. Cells were grown overnight at 18°C in LB supplemented with 0.1 mM IPTG and harvested by centrifugation. The pellet was resuspended in 30 ml of buffer A (20 mM Tris-HCl, 0.1 mM EDTA, 300 mM NaCl, 5% glycerol, 10 mM imidazole, 5 mM β-mercaptoethanol; pH 7.25) supplemented with 1x Complete protease inhibitor cocktail (Roche) and broken by repeated French Press passages at 1000 psi. Following centrifugation at 20.000 × g for 1 h the soluble fraction was passed through a 0.22 μm filter (Millipore) and loaded onto a 5 ml HisTrapHP chelating column (GE Healthcare) previously equilibrated in buffer A. PhoB and σVreI were eluted with a 10 mM to 500 mM imidazole gradient in buffer A and dialyzed against buffer B (50 mM Tris-HCl pH 7.5, 10 mM MgCl2, 1 mM DTT).
Electrophoretic Mobility Shift Assays
Two different EMSA methods were used in this work, the classic method using radioactive labeled DNA (Rojas et al., 2003) and a new method using fluorescein labeled DNA (Blanco et al., 2011). In both methods, phosphorylated PhoB protein was used. The protein was phosphorylated in 50 mM Tris-HCl, 10 mM MgCl2, 1 mM DTT, and 9 mM acetylphosphate reaction buffer at 37°C for 60 min as previously described (McCleary, 1996). For the radioactive method, dsDNA probe containing the promoter region of the P. aeruginosa pdtA gene was obtained by annealing non-labeled complementary oligonucleotides (Table S2). The pstC and fiuA promoter regions were amplified by PCR using genomic DNA from P. aeruginosa PAO1. These DNA fragments were then end-labeled with [γ-32P] deoxyadenosine triphosphate (ATP) using the T4 polynucleotide kinase. A 10 μl sample containing 0.002 pmols of labeled DNA (1.5 × 104 cpm) was incubated with increasing concentrations of phosphorylated PhoB and/or σVreI proteins for 20 min in binding buffer (12 mM Tris-HCl, pH 7, 23.6 mM NaCl, 0.12 M magnesium acetate, 0.24 mM EDTA, 0.24 mM DTT, 1.2% [v/v] glycerol, and 2.3 mM acetylphosphate) containing 20 μg/ml of polyd(IC) and 200 μg/ml of bovine serum albumin (BSA). DNA-protein complexes were resolved by electrophoresis on 4% (w/v) non-denaturing polyacrylamide gels in Tris/Glycine buffer (512 mM Tris, 58.6 mM glycine). For the second method, a 5′ end fluorescein-labeled oligonucleotide was annealed with the complementary strand (Table S2) to obtain dsDNA. In a final volume of 10 μl EMSA samples contained 0.025 nmols of fluorescein-labeled dsDNA and variable amounts of purified PhoB and/or σVreI proteins were incubated in the same binding buffer described above containing polyd(IC) but not BSA during 20 min at 37°C. In the competition experiment, increasing amounts of an unlabeled competitor dsDNA was added to the EMSA reaction. Samples were loaded onto 8% non-denaturing polyacrylamide gels prepared in Tris/Glycine buffer and run at 50 V at room temperature. The fluorescence signal was detected on a conventional UV transilluminator and pictures were taken with the gel-recoding apparatus Minilumi bio-imaging system (Bio-Imaging Systems Ltd).
Computer-Assisted Analyses
Sequence analyses of the Pseudomonas genomes were performed at http://www.pseudomonas.com (Winsor et al., 2011) and sequence alignments with ClustalW (Thompson et al., 1994).
Results
Effect of the PUMA3 Proteins on the Expression of the vreAIR Gene Cluster and the σVreI-Regulated Genes under Pi Starvation
To assess the expression of the PUMA3 genes, we used lacZ transcriptional fusions to three PUMA3 promoters: PvreA, PpdtA, and PphdA (Figure 1A). PvreA is the promoter of the vreAIR operon, PpdtA transcribes solely the pdtA gene, and PphdA transcribes the phdA, pdtB, exbB2D2, tonB4, and PA0696-PA0701 genes (Figure 1A; Faure et al., 2013). Activity of these three promoters was tested by β-galactosidase assay in the P. aeruginosa PAO1 wild-type strain and in the PUMA3 deletion mutants ΔvreA, ΔvreI, and ΔvreR upon growth in low and high Pi conditions. A ΔphoB mutant was also included in the assay. The PvreA was active in low Pi in a PhoB-dependent manner (Figure 1B), as reported previously (Faure et al., 2013). In the ΔvreA, ΔvreI and ΔvreR mutants this promoter reached wild-type levels (Figure 1B), showing that the PUMA3 CSS system is not involved in the regulation of its own expression. The PUMA3-regulated promoters PpdtA and PphdA were also active in low Pi and both the PhoB and σVreI proteins were required for such activation (Figure 1B). However, the activity of these promoters in the ΔvreA mutant reached wild-type levels (Figure 1B), which indicates that the VreA receptor is not involved in the expression of the PUMA3 regulon under Pi starvation. Expression from PpdtA and PphdA correlates with σVreI production, which occurs in the wild-type PAO1 and ΔvreA strains upon growth in low Pi but not in high Pi and does not occur in the ΔphoB mutant (Figure 1C). This confirms that PhoB is required for σVreI production. Interestingly, in the ΔvreR mutant the activity of PpdtA and PphdA in low Pi was considerably higher than in the PAO1 wild-type strain (5.3- and 3.7-fold higher, respectively) (Figure 1B). This indicates that full activation of σVreI requires the removal of VreR, which verifies the anti-σ role of this protein. In accordance, the amount of σVreI in the ΔvreR mutant was considerably higher than in the PAO1 wild-type strain (Figure 1C,-Pi). This supports previous results showing that an HA-tagged version of the σVreI protein is more stable in the absence of the anti-σ factor VreR (Llamas et al., 2009), and suggests that VreR promotes σVreI degradation. The high pdtA and phdA expression observed in the ΔvreR mutant was PhoB- and σVreI-dependent since activity of the lacZ fusions was completely abolished in a ΔphoB ΔvreR double mutant (Figure 1B) that lacks PhoB and in which σVreI is not produced (Figure 1C). Activity of the three promoters in the phoB mutants could be complemented by providing the phoB gene in trans (Figure S1). Complementation was only partial (~35–55% of the activity in wild-type conditions) since overproduction of PhoB from plasmid slightly diminished promoter activities, as observed in the PAO1 wild-type strain (Figure S1).
Role of the N-Terminus of VreR in the Regulation of σVreI Activity
To further analyse the role of VreR in the regulation of σVreI activity, we decided to focus on the N-terminal cytosolic tail (N-tail) of VreR. This anti-σ factor fragment (about 80–90 amino acids in length) is known to bind the σECF (Campbell et al., 2007). Although it was originally described as the domain that keeps the σECF sequestered and inactive in absence of the inducing signal, recent data have shown that the N-tail of some anti-σ factors has pro-sigma activity and is required for σECF functionality (Mettrick and Lamont, 2009; Bastiaansen et al., 2015). To analyse the effect of the N-tail of VreR on σVreI activity, fragments of VreR of different lengths were cloned in the pMMB67EH plasmid under the control of an IPTG-inducible Ptac promoter (Table 1). This includes VreR43 that contains the first 43 amino acids of the VreR protein, which constitutes only half of the cytosolic N-tail; VreR86 (amino acids 1–86), which contains the entire cytoplasmic portion of VreR (the N-tail); and VreR110 (amino acids 1–110), which contains the N-tail, the transmembrane domain and 4 periplasmic residues of VreR (Figure 2A). Activity of σVreI in the presence of these protein fragments was tested in the ΔvreR mutant bearing the σVreI-dependent phdA::lacZ transcriptional fusion upon growth in high and low Pi conditions. Expression of a full-length VreR protein in the mutant restored σVreI activity to wild-type levels (Figure 2B), showing that in trans production of VreR is able to complement the ΔvreR mutation. Amounts of σVreI in this strain were considerably lower than in the not complemented strain (Figure 2C), confirming previous observations indicating that the presence of VreR promotes σVreI degradation (Figure 1C and Llamas et al., 2009). Expression of the VreR43 fragment did not however affect σVreI activity (Figure 2B) or stability (Figure 2C), which were similar to those obtained in the not complemented ΔvreR mutant (Figure 2B). This suggests that the VreR43 fragment, which contains only half of the VreR N-tail, is unable to bind σVreI. In contrast, production of the VreR86 fragment containing the complete cytosolic N-tail of VreR significantly reduced expression from the phdA promoter, suggesting that this fragment interacts with σVreI and inhibits its activity (Figure 2B). Expression of VreR110 also reduced σVreI activity, but to a lesser extent than VreR86 (Figure 2B), likely because the presence of the transmembrane domain in VreR110 hinders the binding of the N-tail to σVreI. Interestingly, whereas expression of VreR110 results in a less stable σVreI protein when compared with the not complemented ΔvreR mutant, expression of VreR86 results in higher amounts of σVreI (Figure 2C). However, as described before, the σ factor is less active upon expression of VreR86 (Figure 2B), which implies that, in contrast to the full-length VreR and the VreR110 proteins, the VreR86-mediated inhibition of σVreI activity does not involve σVreI degradation. In accordance with the reported structures of other σECF/anti-σ pairs (Campbell et al., 2007), it is likely that the VreR86 fragment inhibits σVreI by binding to it and occluding its RNAPc binding determinants. All together these results show that overproduction of the N-tail of VreR inhibits σVreI activity, likely by interacting with this σ factor, and that therefore VreR does not contain pro-sigma activity.
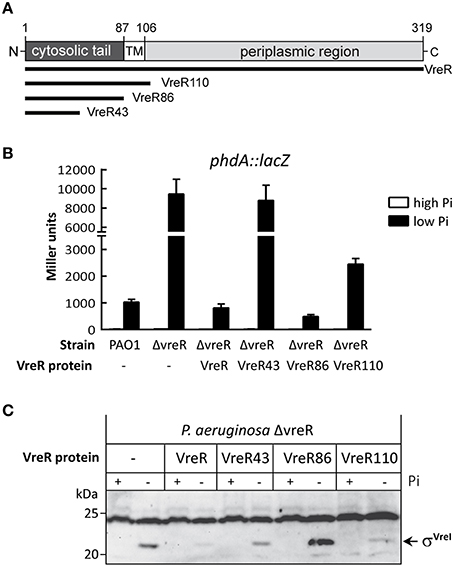
Figure 2. Effect of the N-tail of VreR on σVreI activity. (A) Schematic representation of the P. aeruginosa VreR protein. The VreR protein has been drawn to scale, and the cytosolic, transmembrane (TM), and periplasmic regions of the protein are shown. Numbers indicate amino acid positions. The produced VreR fragments are shown below the scheme. (B) β-galactosidase activity of the indicated P. aeruginosa strains bearing the transcriptional fusion phdA::lacZ and the pMMB67EH (-), pMMB-VreR, pMMB-VreR43, pMMB-VreR86 or pMMB-VreR110 plasmid expressing the indicated VreR fragment from the IPTG-inducible promoter Ptac (Table 1). Strains were grown in high or low Pi in the presence of 1 mM IPTG. (C) Detection of σVreI in P. aeruginosa ΔvreR mutant upon expression of the indicated VreR fragment in high (+) or low (−) Pi and 1 mM IPTG. Proteins were detected by Western-blot using a polyclonal anti-VreI antibody. The positions of the molecular size marker (in kDa) and the σVreI protein are shown.
Effect of σVreI Overproduction on Expression of σVreI-Regulated Genes
Several reports have shown that overproduction of σECF, including σVreI, allows expression of their target genes in absence of the inducing signal (Koster et al., 1994; Pradel and Locht, 2001; Llamas et al., 2006, 2008, 2009; Faure et al., 2013). To study the effect of σVreI overproduction in the different P. aeruginosa phoB and PUMA3 mutants we used the pMUM3 plasmid, which contains the vreI gene expressed from a IPTG-inducible promoter (Llamas et al., 2009; Table 1). Activity of both PpdtA and PphdA was null in high Pi when expression of vreI from pMUM3 was not induced by IPTG (Figure 3A). Upon IPTG induction, a significant increase in activity was observed in all strains tested, including the two phoB mutants (Figure 3A). This effect was considerably stronger in low Pi conditions (Figure 3A). The fact that there is promoter activity in high Pi and in the phoB mutants when vreI expression is induced by IPTG indicates that overproduction of σVreI can bypass the low Pi and PhoB requirements for PpdtA and PphdA activity, as observed previously (Llamas et al., 2009; Faure et al., 2013). In fact, σVreI is present in extremely high amounts when its expression from pMUM3 is induced by IPTG (Figure 3B). Activity of PpdtA and PphdA in low Pi without IPTG was similar to that observed in low Pi in absence of the pMUM3 plasmid: Maximal in the ΔvreR single mutant and null in both phoB mutants (Compare Figure 3A and Figure 1B). Moreover, the ΔvreI mutant could be complemented with pMUM3 in this condition (Figure 3A, low Pi -IPTG), which suggests that σVreI is also produced from the plasmid in absence of IPTG. This was confirmed by Western-blot (Figure 3B). Interestingly, the activity of PpdtA and PphdA in the complemented ΔvreI mutant was considerably higher than that obtained in the PAO1 wild-type strain in low Pi without IPTG (3.9- and 3-fold higher, respectively) and similar to that of the ΔvreR mutant (Figure 3A). Since the absence of the VreR anti-σ factor results in maximal σVreI activity (Figure 1B), the observed phenotype could be due either to a polar effect of the vreI mutation on the expression of the downstream vreR gene or to the instability of the VreR protein in absence of σVreI. To check these two possibilities, VreR production/stability was analyzed by Western-blot using an anti-VreR antibody that detects the chromosomally produced protein, and VreR stability was assayed using an anti-HAtag antibody that detects a C-terminally HA-tagged VreR protein constitutively produced from plasmid. This analysis showed that VreR is not produced in the ΔvreI mutant (Figure 3C, left panel), and that the stability of the protein is not affected in absence of vreI since even higher amount of the VreR-HA protein were detected in the ΔvreI mutant (Figure 3C, right panel). These results indicate that the vreI mutation exerts a polar effect on the expression of vreR. Therefore, both production of σVreI from pMUM3 in absence of IPTG and the lack of VreR explain the high promoter activity observed in the complemented ΔvreI mutant. Importantly, PpdtA and PphdA are not active in strains bearing the pMUM3 plasmid in absence of IPTG in high Pi—a condition in which PhoB is not active (Lamarche et al., 2008)—and in the two phoB mutants, despite the fact that σVreI is being produced and present in sufficient amount to target transcription (Figures 3A,B). This strongly suggests that PhoB is not only required for expression of the vreI gene but also to enhance the σVreI-mediated expression of the PUMA3 regulon genes.
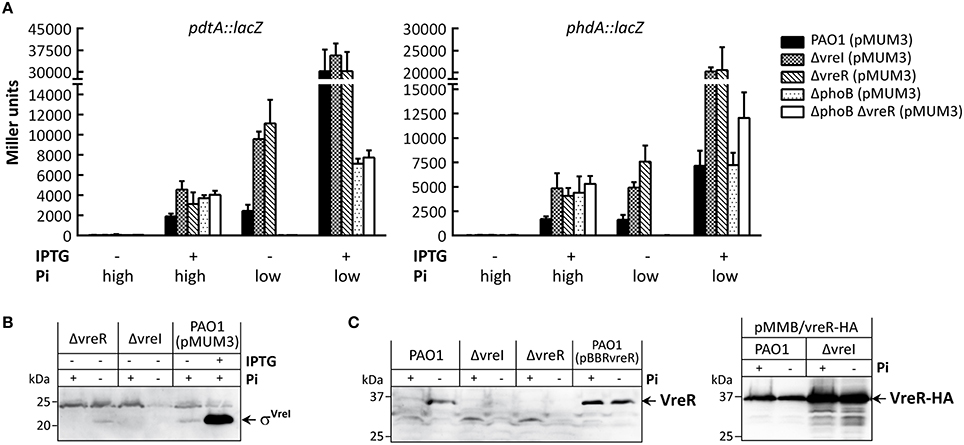
Figure 3. Effect of σVreI overproduction in the expression of the PUMA3 regulon. The indicated P. aeruginosa strains were grown 18h under high (+) or low Pi (−) conditions without (−) or with (+) 1 mM IPTG. (A) β-galactosidase activity of P. aeruginosa strains bearing the indicated lacZ fusion and the pMUM3 plasmid expressing the vreI gene from the IPTG-inducible promoter Ptac (Llamas et al., 2009) (Table 1). (B) Detection of σVreI by Western-blot using a polyclonal anti-VreI antibody. The positions of the molecular size marker (in kDa) and the σVreI protein are shown. (C) Detection of VreR by Western-blot using a polyclonal anti-VreR antibody (left panel) or a monoclonal anti-HA antibody (right panel). The production of the VreR-HA protein from plasmid (Table 1) was induced with 1 mM IPTG. The positions of the molecular size marker (in kDa) and the VreR proteins are shown.
Defining the Promoter Region of σVreI-Regulated Genes
In order to study the effect of the PhoB transcriptional regulator on the expression from PpdtA and PphdA, we decided to first define these promoter regions by locating the transcription initiation point of the pdtA and phdA genes (Figure 1A). Transcription start sites were mapped by 5′ RACE (Invitrogen) using RNA from the P. aeruginosa PAO1 wild-type strain or the ΔvreR mutant after growth in low Pi medium to induce maximal pdtA and phdA expression (Figure 1B). This strategy located the transcriptional start site of pdtA at an adenine residue situated 53-bp upstream the pdtA translational start codon and that of phdA at a thymine residue situated 198-bp upstream the phdA translational start codon (Figure 4A). In order to confirm these results and to rule out the possibility of the presence of other transcription initiation points not identified by 5′ RACE, we carried out primer extension analyses. Total RNA isolated from P. aeruginosa PAO1 cells was annealed to a 5′ -labeled oligonucleotide complementary to either the pdtA or the phdA gene (Table S1). A single cDNA product was obtained for each gene when the RNA was isolated from P. aeruginosa PAO1 or ΔvreR cells grown in low Pi, the amount of these products being considerably higher in the ΔvreR mutant (Figure 4B). In fact, the pdtA cDNA product could be detected only in the ΔvreR mutant (Figure 4B). This confirms the maximal lacZ activity of the transcriptional fusions observed in ΔvreR (Figure 1B). The sizes of the cDNA products (73-bp for pdtA and 178-bp for phdA) corresponded with the transcription initiation points identified by 5′ RACE. These bands were absent when total RNA was isolated from P. aeruginosa cells grown in high Pi or in the ΔvreI and phoB mutants (Figure 4B), confirming that expression of these genes occurs under Pi starvation in a σVreI- and PhoB-dependent manner (Figure 1B). An alignment of the DNA regions upstream the +1 site of pdtA and phdA genes allowed us to identify highly conserved DNA sequences centered within the −10 and −35 regions (Figure 4A). These sequences did not exhibit similarity to the consensus sequence recognized by σ70 (TATAAT at −10 and TTGACA at −35), and could therefore be an alternative promoter sequence recognized by the RNAP loaded with σVreI. Interestingly, a putative pho box was detected in both promoter regions. PhoB binds DNA as a dimer and recognizes a 22-bp region with two 7-bp direct repeats followed by an A/T-rich region of 4-bp (Blanco et al., 2002), a sequence that was present in PpdtA and PphdA (Figure 4A). The presence of a pho box further suggests the direct involvement of the PhoB regulator in the expression from these promoters.
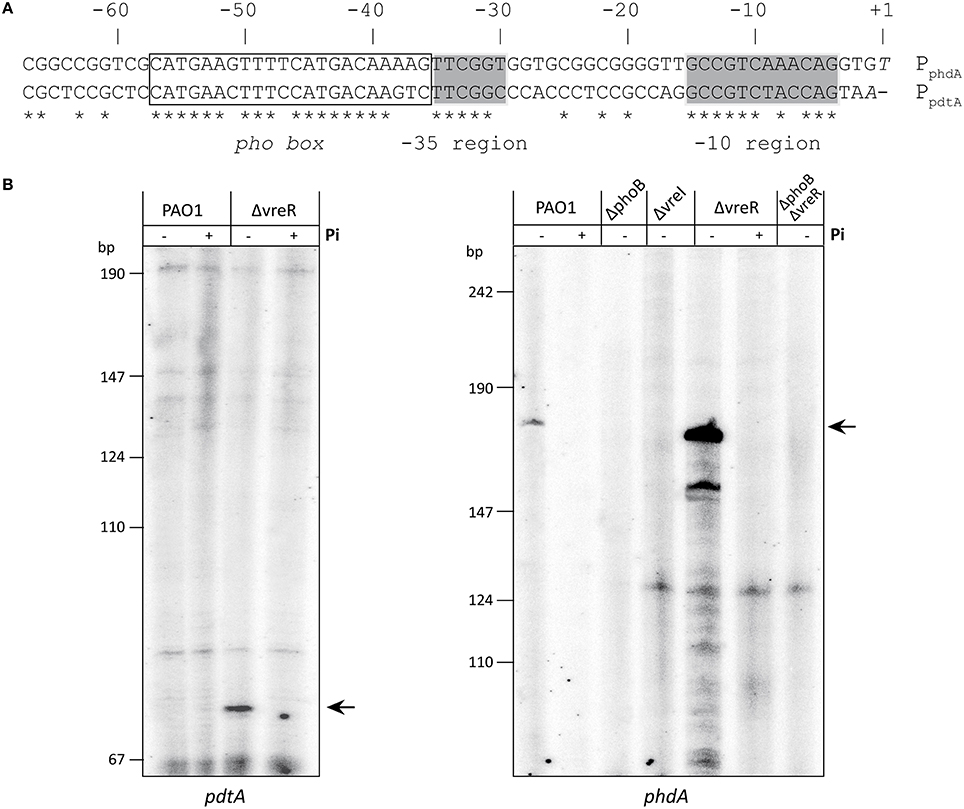
Figure 4. Determination of the transcription initiation points of the pdtA and phdA genes. (A) Identification of the +1 site by 5′ RACE and pdtA and phdA promoter analysis. The P. aeruginosa PAO1 genomic sequence corresponding to the region upstream of the pdtA and phdA gene is shown. Nucleotides in italic represent the proposed +1 site. The identified promoter elements (pho, −35 and −10 boxes) are indicated. Identical nucleotide residues in both promoter regions are marked with a star. (B) Primer extension analysis of pdtA and phdA mRNA. P. aeruginosa PAO1 cells and the indicated mutants were grown in low or high Pi medium, and samples were taken in stationary phase for total RNA isolation. The autoradiogram shows the cDNA products obtained after reverse transcription of 12 μg of total RNA with the 5′ -end-labeled PA0690R or PA0691R oligonucleotides (Table S1) hybridizing with the pdtA or the phdA mRNA, respectively.
Contribution of the −10 and −35 Regions and the pho box to the activity of the pdtA Promoter
To determine the contribution of the identified −10, −35 and pho box regions to the activity of the pdtA promoter, we made several constructs in which these sequences were disrupted by single or multiple substitutions (S), by insertions (I), or by deletions (Δ) (Table 2). These constructs were then fused to the lacZ reporter gene and transferred to the P. aeruginosa PAO1 wild-type strain and the ΔvreR mutant to test their activity upon growth in Pi starvation conditions. Activity of all constructs in high Pi conditions was null in both strains (data not shown), indicating that none of the mutations resulted in a constitutively active promoter. Importantly, the effect of the mutations in the promoter activity upon growth in Pi starvation was very similar when tested in the PAO1 and in the ΔvreR mutant (Table 2) in which σVreI activity is maximal, indicating that their activity depends on σVreI. Single and multiple mutations in the −10 region showed that changes in the nucleotides −5 to −11 had a dramatic effect on promoter activity, which was completely abolished (Table 2). However, mutation of the −3 and −4 nucleotides had little effect (70% of the activity retained); substitution of the −12, −13, and −14 nucleotides reduced, but did not abolish the activity (30–55% of the activity retained); and mutation of only the −13 and −14 nucleotides had no effect on promoter activity (Table 2). Substitutions within the region between the −10 and −35 boxes did not affect promoter activity (Table 2; S-19 and S-24), whereas changing the size of this region by either inserting or deleting one nucleotide did significantly affect expression (Table 2; I-22 and Δ-22). Within the −35 region, substitution of the nucleotides −30 to −34 and that of the –33 and –34 considerably reduced pdtA promoter activity (Table 2). In contrast, changing the –29 and −30 GC nucleotides into TA resulted in a more active promoter (Table 2). The contribution of the identified pho box to the pdtA promoter activity was also analyzed. Complete disruption of the pho box (S pho box) or disruption of only one of the two 7-bp direct repeat sequences (S-40 to −45 or S-50 to −56) completely abolished promoter activity (Table 2). This indicates that intact −10, −35 and pho boxes are required for pdtA expression.
The PhoB Transcription Factor Binds to the vreA, pdtA, and phdA Promoters
The results obtained here with the mutational analysis of the pho box of the pdtA promoter (Table 2) and those obtained previously with a similar analysis of the pho box of the vreA promoter (Faure et al., 2013), suggest that PhoB directly binds to these promoter regions. To confirm this, we performed electrophoretic mobility shift assays (EMSA) using a fixed amount of fluorescein-labeled dsDNA probes obtained by annealing oligonucleotides that contain the promoter region of the vreA, pdtA, or phdA genes (Table S2). Addition of increasing concentrations of purified and phosphorylated PhoB protein to the DNA fragments resulted in a slower complex that at higher protein concentration became the predominant (Figure 5A), showing that PhoB indeed binds the vreA, pdtA and phdA promoters. Since non-isotopic DNA labeling can alter the affinity and/or stoichiometry of the protein-DNA interaction, we also performed the EMSA using 32P-labeled dsDNA. In this condition, two retarded DNA bands were observed (Figure 5B, pdtA promoter). Since PhoB is known to bind to DNA as a dimer of which each monomer contacts one direct repeat of the pho box (Makino et al., 1996; Blanco et al., 2002), it is possible that these bands are the result of PhoB binding first as a monomer (complex I) and at higher concentrations as a dimer, generating the second retardation band (complex II). Two DNA retardation bands were also observed when the pstC promoter, which is known to contain a pho box (Nikata et al., 1996; Jensen et al., 2006), was used as a positive control (Figure S2). No band shifts were however detected when the fiuA promoter, which is not regulated by low Pi (Llamas et al., 2006) and does not have a pho box, was used as DNA probe (Figure S2). This confirms the specific binding of the purified PhoB protein to promoters containing a pho box. Moreover, addition of increasing amounts of an unlabeled competitor dsDNA to the EMSA reactions resulted in the complete disappearance of the second retardation band and in a considerably increase in the amount of free DNA (Figure S2). Although the complex I was still formed (probably because the amounts of unlabeled DNA did not reach the level needed for complex I to disappear), this indicates that there is competition between the DNAs and therefore that the retardation bands are the specific result of PhoB-DNA complexes formation. Interestingly, when a pdtA promoter containing mutations in the first direct repeat of the pho box was used as DNA probe, the binding of PhoB was considerably impaired and a higher concentration of the protein was needed for the formation of the PhoB-DNA complex (Figure 5C). Mutation of the two direct repeats of the pho box completely abolished PhoB binding (Figure 5C), which suggests that this mutated region contains the PhoB binding site. Altogether, our results show that PhoB binds to the promoter region of the vreAIR operon and, importantly, to that of the σVreI-dependent promoters pdtA and phdA.
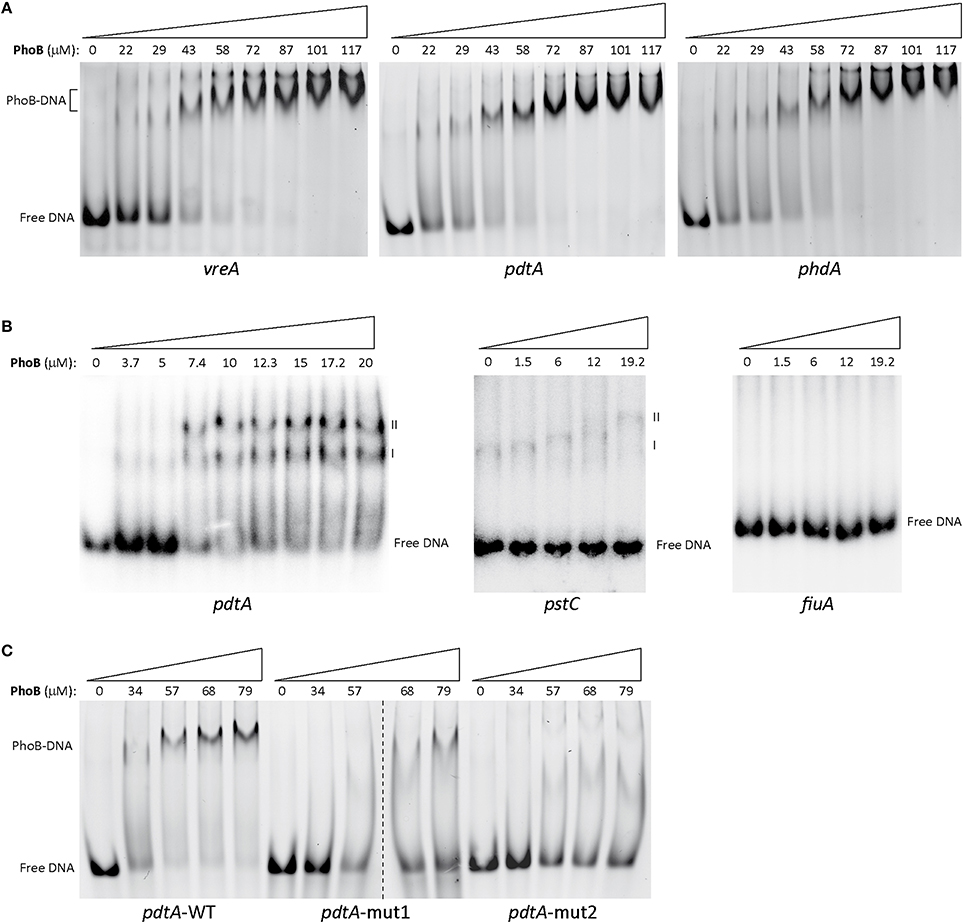
Figure 5. Binding of PhoB to the vreA, pdtA and phdA promoter regions. EMSA gels using fluorescein-labeled (A and C) or 32P-labeled (B) dsDNA probes (Table S2) containing the indicated P. aeruginosa promoter and increasing amounts of phosphorylated PhoB protein. Upper numbers indicate the concentration of PhoB used in the assay (in μM). In A and B wild-type (WT) promoter sequences were used as DNA probes. In C pdtA promoters with mutations in the first direct repeat (pdtA-mut1) or in both direct repeats (pdtA-mut2) of the pho box were used. The position of the free DNA and of the PhoB-DNA complexes (I and II) are indicated.
PhoB Is Required for the Binding of σVreI to the pdtA Promoter
Next, we assayed the binding of σVreI to the pdtA promoter by EMSA. Several attempts using the σVreI protein alone or reconstituted σVreI-RNAP holoenzyme did not result in DNA retardation bands (data not shown), suggesting that σVreI alone could not bind to this promoter region. Therefore, we tested the binding of σVreI to PhoB-DNA complexes. Addition of increasing amounts of σVreI in EMSA reactions containing the PhoB protein (in a concentration that results in the formation of the complex II) and the pdtA promoter resulted in the appearance of a new retarded DNA band (Figure 6). This change in mobility likely reflects the formation of a σVreI-PhoB-DNA complex, which was not formed in absence of σVreI or PhoB (Figure 6). This indicates that σVreI cannot interact with the promoter region of pdtA and phdA genes in the absence of PhoB, which is in agreement with the PhoB-requirement for expression of these genes even in conditions in which σVreI is present (Figure 3A, low Pi -IPTG). The σVreI-PhoB-DNA complex was not formed when the vreA promoter was used as DNA probe (Figure 6), in agreement with σVreI not being involved in the expression from this promoter (Figure 1B). This confirms the specific binding of the σ factor to σVreI-dependent promoters and shows the requirement of PhoB for this binding to occur.
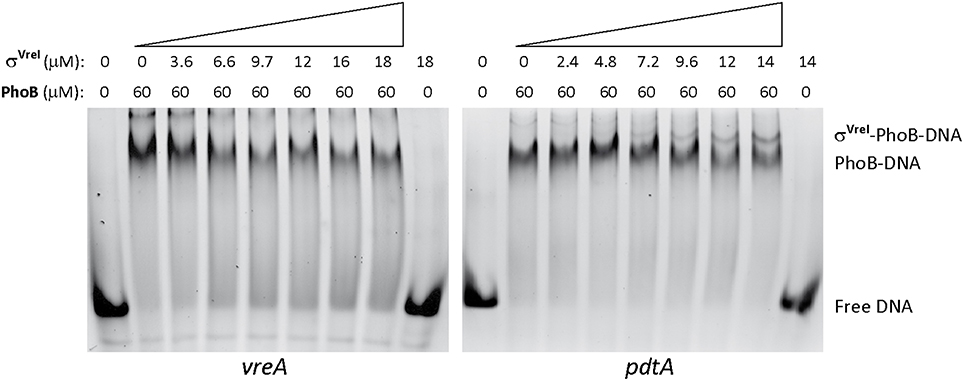
Figure 6. Binding of σVreI to the pdtA promoter. EMSA gels using fluorescein-labeled dsDNA probes containing the P. aeruginosa vreA or pdtA promoter (Table S2). Increasing amounts of σVreI were added to a preformed PhoB-DNA complex. Upper numbers indicate the concentration of phosphorylated PhoB and σVreI proteins used in the assay (in μM). The position of the free DNA and of the PhoB-DNA and σVreI-PhoB-DNA complexes are indicated.
Discussion
The PUMA3 system of P. aeruginosa is an unusual CSS cascade in various functional and architectural aspects. Importantly, this signal transduction system is directly involved in the regulation of virulence and, unlike most P. aeruginosa CSS systems, does not control iron uptake (Llamas et al., 2009, 2014). The architectural variations of the system mainly concern the VreA receptor-like component, which is smaller than regular CSS receptors and seems to function only in signaling and not in the transport of the signal molecule (Llamas et al., 2009). In addition, the genetic organization of the vreAIR genes encoding the PUMA3 system is different than that of most CSS pathways. While CSS σECF are generally co-transcribed with their cognate anti-σ factor and the receptor gene is located in a separate transcriptional unit (Koebnik, 2005; Llamas et al., 2014), the vreAIR genes form an operon (Figure 1A). In P. aeruginosa expression of most σECF/anti-σ operons is controlled by iron through the Fur regulator, which allows production of these proteins in iron depleted conditions (Llamas et al., 2014). In contrast, expression of the vreAIR operon is targeted by Pi starvation and requires the phosphate regulator PhoB. A pho box is present in the vreA promoter region (Faure et al., 2013), and direct binding of this transcription factor to this promoter region has been demonstrated in this work (Figure 5). The vreAIR gene products, including σVreI, are not involved in the expression from the vreA promoter, and in accordance, σVreI does not bind to this promoter (Figure 6). This indicates that another σ factor, likely the P. aeruginosa primary σ factor σ70, targets transcription of the vreAIR operon under Pi starvation and in a PhoB-dependent manner.
Interestingly, the genes belonging to the PUMA3 regulon are expressed in response to Pi starvation in a σVreI-dependent manner, despite the fact that in this condition the σVreI repressor VreR is also produced. A specific inducing signal is typically required to relieve the anti-σ-mediated inhibition of σECF activity. In presence of such a signal, the anti-σ factor protein is removed by regulated proteolysis allowing the σECF-mediated transcription (Qiu et al., 2007; Ades, 2008; Draper et al., 2011; Bastiaansen et al., 2014, 2015). We show here that deletion of the vreR anti-σ factor gene is required for maximal σVreI activity in low Pi (Figure 1B). This suggests that an additional stimulus not present in Pi starvation is needed to remove VreR and produce full σVreI activation. This hypothesis is supported by the fact that the VreA receptor, which by analogy with most CSS systems likely initiates the PUMA3 signaling cascade (Llamas et al., 2014), is not required for the transcription of the PUMA3 regulon genes in low Pi. Therefore, the detected σVreI activity in Pi starvation seems not to be the result of actual signaling through the PUMA3 CSS system, but represents “leaky” activity of σVreI. Previous studies have shown that σVreI-regulated genes are induced upon contact of P. aeruginosa with human airway epithelial cells (Frisk et al., 2004; Chugani and Greenberg, 2007). Moreover, antibodies against the PdtA and PA0697 proteins of the PUMA3 regulon (Figure 1A) have been detected in the serum of patients infected with P. aeruginosa (Llamas et al., 2009). This suggests that the molecule targeting PUMA3 signaling could be host-derived, and our current work aims at identifying such a signal.
The fact that VreR removal produces maximal activation of σVreI indicates that this anti-σ factor only has anti-σ function. Two divergent classes of CSS anti-σ factors have been reported, mere anti-σ factors and anti-σ factors with pro-sigma activity (also called sigma factor regulators) (Mettrick and Lamont, 2009; Llamas and Bitter, 2010; Llamas et al., 2014). Proteins within the first group only contain anti-σ activity and inhibit activity of their cognate σECF in absence of the CSS inducing signal. Deletion of these proteins results in signal-independent transcription of the σECF-regulated genes (Mettrick and Lamont, 2009). In contrast, deletion of anti-σ factors of the second group does not result in activation of its cognate σECF since these anti-σ factors are required for σECF activity. The pro-sigma activity of these proteins seems to reside within the short cytosolic N-terminal region (N-tail), since the expression of this domain alone induces σECF activity independently of the presence of the signal (Ochs et al., 1995; Ó Cuív et al., 2006; Mettrick and Lamont, 2009). Recently, we have shown that the N-tail of anti-σ factors is indeed produced in vivo in response to the inducing signal and that the transmembrane protease RseP is responsible for this process (Bastiaansen et al., 2015). Although still not experimentally determined, it has been proposed that the N-tail can protect the σECF from degradation and that this domain may be bound to the σECF-RNAP holoenzyme during the transcription process (Mahren and Braun, 2003). However, this does not seem to be the case for the N-tail of VreR since this protein fragment does not enhance σVreI activity. In fact, overexpression of the N-tail of VreR inhibits the activity of σVreI (Figure 2). This indicates that VreR does not contain pro-sigma activity, which is in accordance with the higher σVreI activity detected in the ΔvreR mutant. The role of VreR as a mere anti-σ factor is further supported by the fact that σVreI is more stable in absence of VreR. Our results suggest that VreR employs at least two mechanisms to inhibit σVreI activity: binding to the σ factor likely shielding the binding determinants of σVreI for the RNAPc, and promotion of σVreI degradation. The N-tail of VreR (aminoacids 1–86) seems to be sufficient to prevent binding of σVreI to the RNAPc, but this fragment alone does not promote σVreI degradation (Figure 2) and the complete protein seems to be required for this. Another P. aeruginosa CSS anti-σ factor, FvpR, has also been reported to induce degradation of its cognate σECF (Spencer et al., 2008), although the mechanism behind this process is still unknown. These observations further indicate that in vivo and upon sensing the PUMA3 inducing signal, VreR needs to be completely removed in order for σVreI to reach maximal activity.
Importantly, we show in this work that activity of σVreI is also modulated by a transcription factor, the phosphate regulator PhoB. This is an important finding since, while modulation of primary σ factors activity by trans-acting factors has been extensively reported, such modulation of σECF has not been extensively investigated yet. As demonstrated in this study, PhoB is not only required for σVreI production but also for the binding of σVreI to the promoter region of its target genes. In fact, the two proteins bind to the promoter of the σVreI-regulated genes and, in accordance, expression of these genes does not occur unless both proteins are present and active in the cell. Only extremely high levels of σVreI, which we obtained by overexpressing the vreI gene from an IPTG-inducible promoter, can bypass the PhoB requirement for the transcription of the σVreI target genes. However, these σVreI levels are not likely to be ever reached in vivo. As mentioned before, it is expected that upon sensing the PUMA3 inducing signal VreR is proteolytically degraded and σVreI released. Thus, the maximal σVreI amount expected in vivo upon induction of the PUMA3 cascade likely resembles the level obtained in the ΔvreR mutant, which is considerably lower than that obtained when production of σVreI from plasmid was induced with IPTG (Figure 3B). Therefore, both PhoB and σVreI are needed to target transcription of the PUMA3 regulon genes in vivo. The potential DNA binding sites for PhoB and σVreI in the promoter regions of PUMA3 regulon genes have been identified. A conserved pho box (Blanco et al., 2002) containing two 7-bp direct repeats is located upstream of the −35 region of both the pdtA and phdA promoters. Mutation of this region, either one of the direct repeats or the entire box, completely abrogates gene expression (Table 2), and, when the two direct repeats are mutated, also the binding of PhoB (Figure 5C). Based on these results, we propose that this region within the pdtA and pdhA promoters is the PhoB binding site. Although in E. coli the pho box is usually located near the σ70 –10 promoter region substituting the −35 region (Makino et al., 1986; Blanco et al., 2002), this does not seem to be the case for σVreI-dependent promoters. Downstream of the pho box, highly identical sequences centered within the −35 and −10 positions have been identified in the pdtA and phdA promoters (Figure 4A). Members of the σ70 family are known to recognize promoter sequences located at positions −35 and −10 from the transcriptional start point and regions 4.2 and 2.4, respectively, of the primary σ70 protein are involved in such recognition (Brooks and Buchanan, 2008). σECF are the smallest σ factors of the σ70 family and lack two of the four conserved domains of primary σ factors (domains 1 and 3) (Lonetto et al., 1994; Bastiaansen et al., 2012). However, promoter recognition by σECF involves the same σ factor regions (Enz et al., 2003; Wilson and Lamont, 2006). Interestingly, region 2.4, which recognizes the −10 promoter element, shows most variation within the σECF subfamily, which likely reflects differences in promoter binding specificity (Lonetto et al., 1994). This suggests that promoter specificity of σECF is predominantly determined by the −10 promoter element and the region 2.4 of the σECF. In agreement, single mutations within the −10 promoter sequence of pdtA (nucleotides −5 to −11) completely abrogated gene expression, in both the wild-type strain and the ΔvreR mutant, which strongly indicates that this region is essential for the σVreI-mediated transcription of this gene. Although σECF usually share a high degree of similarity in their −35 promoter element (Enz et al., 2003), which has a conserved AA motif that is important for DNA geometry and thus for σECF-DNA interaction (Lane and Darst, 2006), this is not the case for the σVreI-dependent promoters. The absence of this motif in the −35 region could impair the binding of σVreI to the DNA, which would be facilitated by the binding of the PhoB protein to the pho box. Our results strongly suggest a model in which PhoB recruits σVreI to the promoter region to trigger transcription, which is similar to the mechanism employs by PhoB with the primary σ70 factor (Makino et al., 1996; Blanco et al., 2011). Although studies focused on the structure of the PhoB-σVreI-DNA complex are required to fully understand the process, it is likely that the PhoB-σVreI interaction involves, as shown for σ70 (Blanco et al., 2011), the region 4 of σVreI, which is the region that contacts the −35 sequence and is potentially the closest to PhoB in the complex.
In summary, our results show that the activity of the P. aeruginosa σVreI in Pi starvation is modulated by both the anti-σ factor VreR and the transcription factor PhoB. Pi starvation is an important environmental cue that induces transcription of the so-called pho regulon, which in P. aeruginosa includes multiple potential virulence factors (Lamarche et al., 2008). It is therefore not surprising that Pi starvation enhances P. aeruginosa lethality in mice and nematodes, while providing excess phosphate protects from killing (Long et al., 2008; Zaborina et al., 2008; Zaborin et al., 2009, 2012). Overexpression of σVreI has been demonstrated to increase P. aeruginosa lethality in zebrafish embryos (Llamas et al., 2009) and preliminary results from our group indicate that Pi starvation enhances the virulence of this bacterium in this infection model (data not shown). Moreover, there are several indications that the P. aeruginosa pho regulon is induced in vivo during infection (Frisk et al., 2004; Datta et al., 2007; Long et al., 2008). Since the PUMA3 CSS system is produced under Pi starvation and the currently unknown inducing signal is likely host-derived, it will be of interest to determine the contribution of σVreI and the PUMA3 regulon proteins to the low Pi-induced virulence of P. aeruginosa.
Author Contributions
JQ and ML conceived and designed the study. JQ, JO, KB, and CC performed the experiments. JQ, KB, and ML analyzed and interpreted the data. ML wrote the manuscript.
Funding
This work has been supported by the EU Seventh Framework Programme through a Marie Curie CIG grant (3038130), and the Spanish Ministry of Economy with grants inside the Ramón&Cajal (RYC2011-08874 to ML) and the Plan Nacional for I+D+i (SAF2012-31919 and SAF2015-68873-P) programs.
Conflict of Interest Statement
The authors declare that the research was conducted in the absence of any commercial or financial relationships that could be construed as a potential conflict of interest.
Acknowledgments
We thank W. Bitter and S. Marqués for helpful discussions.
Supplementary Material
The Supplementary Material for this article can be found online at: http://journal.frontiersin.org/article/10.3389/fmicb.2016.01159
Abbreviations
CSS, cell-surface signaling; ECF, extracytoplasmic function; IPTG, isopropyl β-D-1-thiogalactopyranoside; RNAPc, RNA polymerase core enzyme; Pi, inorganic phosphate.
References
Ades, S. E. (2008). Regulation by destruction: design of the σE envelope stress response. Curr. Opin. Microbiol. 11, 535–540. doi: 10.1016/j.mib.2008.10.004
Bastiaansen, K. C., Bitter, W., and Llamas, M. A. (2012). “ECF sigma factors: from stress management to iron uptake,” in Bacterial Regulatory Networks, ed A. Filloux (Norfolk, VA: Caister Academic Press), 59–86.
Bastiaansen, K. C., Ibañez, A., Ramos, J. L., Bitter, W., and Llamas, M. A. (2014). The Prc and RseP proteases control bacterial cell-surface signaling activity. Environ. Microbiol. 16, 2433–2443. doi: 10.1111/1462-2920.12371
Bastiaansen, K. C., Otero-Asman, J. R., Luirink, J., Bitter, W., and Llamas, M. A. (2015). Processing of cell-surface signalling anti-sigma factors prior to signal recognition is a conserved autoproteolytic mechanism that produces two functional domains. Environ. Microbiol. 17, 3263–3277. doi: 10.1111/1462-2920.12776
Blanco, A. G., Canals, A., Bernues, J., Sola, M., and Coll, M. (2011). The structure of a transcription activation subcomplex reveals how σ70 is recruited to PhoB promoters. EMBO J. 30, 3776–3785. doi: 10.1038/emboj.2011.271
Blanco, A. G., Sola, M., Gomis-Ruth, F. X., and Coll, M. (2002). Tandem DNA recognition by PhoB, a two-component signal transduction transcriptional activator. Structure 10, 701–713. doi: 10.1016/S0969-2126(02)00761-X
Brooks, B. E., and Buchanan, S. K. (2008). Signaling mechanisms for activation of extracytoplasmic function (ECF) sigma factors. Biochim. Biophys. Acta 1778, 1930–1945. doi: 10.1016/j.bbamem.2007.06.005
Campbell, E. A., Greenwell, R., Anthony, J. R., Wang, S., Lim, L., Das, K., et al. (2007). A conserved structural module regulates transcriptional responses to diverse stress signals in bacteria. Mol. Cell 27, 793–805. doi: 10.1016/j.molcel.2007.07.009
Choi, K. H., Kumar, A., and Schweizer, H. P. (2006). A 10-min method for preparation of highly electrocompetent Pseudomonas aeruginosa cells: application for DNA fragment transfer between chromosomes and plasmid transformation. J. Microbiol. Methods 64, 391–397. doi: 10.1016/j.mimet.2005.06.001
Chugani, S., and Greenberg, E. P. (2007). The influence of human respiratory epithelia on Pseudomonas aeruginosa gene expression. Microb. Pathog. 42, 29–35. doi: 10.1016/j.micpath.2006.10.004
Datta, H. K., Malik, M., and Neely, R. D. (2007). Hepatic surgery-related hypophosphatemia. Clin. Chim. Acta 380, 13–23. doi: 10.1016/j.cca.2007.01.027
Draper, R. C., Martin, L. W., Beare, P. A., and Lamont, I. L. (2011). Differential proteolysis of sigma regulators controls cell-surface signalling in Pseudomonas aeruginosa. Mol. Microbiol. 82, 1444–1453. doi: 10.1111/j.1365-2958.2011.07901.x
Enz, S., Mahren, S., Menzel, C., and Braun, V. (2003). Analysis of the ferric citrate transport gene promoter of Escherichia coli. J. Bacteriol. 185, 2387–2391. doi: 10.1128/JB.185.7.2387-2391.2003
Faure, L. M., Llamas, M. A., Bastiaansen, K. C., De Bentzmann, S., and Bigot, S. (2013). Phosphate starvation relayed by PhoB activates the expression of the Pseudomonas aeruginosa σVreI ECF factor and its target genes. Microbiology 159, 1315–1327. doi: 10.1099/mic.0.067645-0
Fisher, C. L., and Pei, G. K. (1997). Modification of a PCR-based site-directed mutagenesis method. Biotechniques 23, 570–574.
Frisk, A., Schurr, J. R., Wang, G., Bertucci, D. C., Marrero, L., Hwang, S. H., et al. (2004). Transcriptome analysis of Pseudomonas aeruginosa after interaction with human airway epithelial cells. Infect. Immun. 72, 5433–5438. doi: 10.1128/IAI.72.9.5433-5438.2004
Fürste, J. P., Pansegrau, W., Frank, R., Blocker, H., Scholz, P., Bagdasarian, M., et al. (1986). Molecular cloning of the plasmid RP4 primase region in a multi-host-range tacP expression vector. Gene 48, 119–131. doi: 10.1016/0378-1119(86)90358-6
Hanahan, D. (1983). Studies on transformation of Escherichia coli with plasmids. J. Mol. Biol. 166, 557–580. doi: 10.1016/S0022-2836(83)80284-8
Helmann, J. D. (2002). The extracytoplasmic function (ECF) sigma factors. Adv. Microb. Physiol. 46, 47–110. doi: 10.1016/S0065-2911(02)46002-X
Herrero, M., De Lorenzo, V., and Timmis, K. N. (1990). Transposon vectors containing non-antibiotic resistance selection markers for cloning and stable chromosomal insertion of foreign genes in gram-negative bacteria. J. Bacteriol. 172, 6557–6567.
Ishihama, A. (2000). Functional modulation of Escherichia coli RNA polymerase. Annu. Rev. Microbiol. 54, 499–518. doi: 10.1146/annurev.micro.54.1.499
Jacobs, M. A., Alwood, A., Thaipisuttikul, I., Spencer, D., Haugen, E., Ernst, S., et al. (2003). Comprehensive transposon mutant library of Pseudomonas aeruginosa. Proc. Natl. Acad. Sci. U.S.A. 100, 14339–14344. doi: 10.1073/pnas.2036282100
Jensen, V., Lons, D., Zaoui, C., Bredenbruch, F., Meissner, A., Dieterich, G., et al. (2006). RhlR expression in Pseudomonas aeruginosa is modulated by the Pseudomonas quinolone signal via PhoB-dependent and -independent pathways. J. Bacteriol. 188, 8601–8606. doi: 10.1128/JB.01378-06
Jeong, H., Barbe, V., Lee, C. H., Vallenet, D., Yu, D. S., Choi, S. H., et al. (2009). Genome sequences of Escherichia coli B strains REL606 and BL21(DE3). J. Mol. Biol. 394, 644–652. doi: 10.1016/j.jmb.2009.09.052
Kaniga, K., Delor, I., and Cornelis, G. R. (1991). A wide-host-range suicide vector for improving reverse genetics in gram-negative bacteria: inactivation of the blaA gene of Yersinia enterocolitica. Gene 109, 137–141. doi: 10.1016/0378-1119(91)90599-7
Koebnik, R. (2005). TonB-dependent trans-envelope signalling: the exception or the rule? Trends Microbiol. 13, 343–347. doi: 10.1016/j.tim.2005.06.005
Koster, M., Van Klompenburg, W., Bitter, W., Leong, J., and Weisbeek, P. (1994). Role for the outer membrane ferric siderophore receptor PupB in signal transduction across the bacterial cell envelope. EMBO J. 13, 2805–2813.
Kovach, M. E., Elzer, P. H., Hill, D. S., Robertson, G. T., Farris, M. A., Roop, R. M., et al. (1995). Four new derivatives of the broad-host-range cloning vector pBBR1MCS, carrying different antibiotic-resistance cassettes. Gene 166, 175–176. doi: 10.1016/0378-1119(95)00584-1
Lamarche, M. G., Wanner, B. L., Crepin, S., and Harel, J. (2008). The phosphate regulon and bacterial virulence: a regulatory network connecting phosphate homeostasis and pathogenesis. FEMS Microbiol. Rev. 32, 461–473. doi: 10.1111/j.1574-6976.2008.00101.x
Lamont, I. L., Beare, P. A., Ochsner, U., Vasil, A. I., and Vasil, M. L. (2002). Siderophore-mediated signaling regulates virulence factor production in Pseudomonas aeruginosa. Proc. Natl. Acad. Sci. U.S.A. 99, 7072–7077. doi: 10.1073/pnas.092016999
Lane, W. J., and Darst, S. A. (2006). The structural basis for promoter -35 element recognition by the group IV sigma factors. PLoS Biol. 4:e269. doi: 10.1371/journal.pbio.0040269
Leoni, L., Orsi, N., De Lorenzo, V., and Visca, P. (2000). Functional analysis of PvdS, an iron starvation sigma factor of Pseudomonas aeruginosa. J. Bacteriol. 182, 1481–1491. doi: 10.1128/JB.182.6.1481-1491.2000
Llamas, M. A., and Bitter, W. (2010). “Cell-surface signalling in Pseudomonas,” in Pseudomonas: Molecular Microbiology, Infection and Biodiversity, eds J. L. Ramos and A. Filloux (Dordrecht; Heidelberg; London; New York, NY: Springer Science), 59–95.
Llamas, M. A., Imperi, F., Visca, P., and Lamont, I. L. (2014). Cell-surface signaling in Pseudomonas: stress responses, iron transport, and pathogenicity. FEMS Microbiol. Rev. 38, 569–597. doi: 10.1111/1574-6976.12078
Llamas, M. A., Mooij, M. J., Sparrius, M., Vandenbroucke-Grauls, C. M., Ratledge, C., and Bitter, W. (2008). Characterization of five novel Pseudomonas aeruginosa cell-surface signalling systems. Mol. Microbiol. 67, 458–472. doi: 10.1111/j.1365-2958.2007.06061.x
Llamas, M. A., Ramos, J. L., and Rodriguez-Herva, J. J. (2000). Mutations in each of the tol genes of Pseudomonas putida reveal that they are critical for maintenance of outer membrane stability. J. Bacteriol. 182, 4764–4772. doi: 10.1128/JB.182.17.4764-4772.2000
Llamas, M. A., Sparrius, M., Kloet, R., Jimenez, C. R., Vandenbroucke-Grauls, C., and Bitter, W. (2006). The heterologous siderophores ferrioxamine B and ferrichrome activate signaling pathways in Pseudomonas aeruginosa. J. Bacteriol. 188, 1882–1891. doi: 10.1128/JB.188.5.1882-1891.2006
Llamas, M. A., Van Der Sar, A., Chu, B. C., Sparrius, M., Vogel, H. J., and Bitter, W. (2009). A Novel extracytoplasmic function (ECF) sigma factor regulates virulence in Pseudomonas aeruginosa. PLoS Pathog. 5:e1000572. doi: 10.1371/journal.ppat.1000572
Lonetto, M. A., Brown, K. L., Rudd, K. E., and Buttner, M. J. (1994). Analysis of the Streptomyces coelicolor sigE gene reveals the existence of a subfamily of eubacterial RNA polymerase sigma factors involved in the regulation of extracytoplasmic functions. Proc. Natl. Acad. Sci. U.S.A. 91, 7573–7577. doi: 10.1073/pnas.91.16.7573
Long, J., Zaborina, O., Holbrook, C., Zaborin, A., and Alverdy, J. (2008). Depletion of intestinal phosphate after operative injury activates the virulence of P. aeruginosa causing lethal gut-derived sepsis. Surgery 144, 189–197. doi: 10.1016/j.surg.2008.03.045
Mahren, S., and Braun, V. (2003). The FecI extracytoplasmic-function sigma factor of Escherichia coli interacts with the beta' subunit of RNA polymerase. J. Bacteriol. 185, 1796–1802. doi: 10.1128/JB.185.6.1796-1802.2003
Makino, K., Amemura, M., Kawamoto, T., Kimura, S., Shinagawa, H., Nakata, A., et al. (1996). DNA binding of PhoB and its interaction with RNA polymerase. J. Mol. Biol. 259, 15–26. doi: 10.1006/jmbi.1996.0298
Makino, K., Shinagawa, H., Amemura, M., and Nakata, A. (1986). Nucleotide sequence of the phoB gene, the positive regulatory gene for the phosphate regulon of Escherichia coli K-12. J. Mol. Biol. 190, 37–44. doi: 10.1016/0022-2836(86)90073-2
Marques, S., Holtel, A., Timmis, K. N., and Ramos, J. L. (1994). Transcriptional induction kinetics from the promoters of the catabolic pathways of TOL plasmid pWW0 of Pseudomonas putida for metabolism of aromatics. J. Bacteriol. 176, 2517–2524.
Martinez-Antonio, A., Janga, S. C., Salgado, H., and Collado-Vides, J. (2006). Internal-sensing machinery directs the activity of the regulatory network in Escherichia coli. Trends Microbiol. 14, 22–27. doi: 10.1016/j.tim.2005.11.002
Mascher, T. (2013). Signaling diversity and evolution of extracytoplasmic function (ECF) sigma factors. Curr. Opin. Microbiol. 16, 148–155. doi: 10.1016/j.mib.2013.02.001
McCleary, W. R. (1996). The activation of PhoB by acetylphosphate. Mol. Microbiol. 20, 1155–1163. doi: 10.1111/j.1365-2958.1996.tb02636.x
Mettrick, K. A., and Lamont, I. L. (2009). Different roles for anti-sigma factors in siderophore signalling pathways of Pseudomonas aeruginosa. Mol. Microbiol. 74, 1257–1271. doi: 10.1111/j.1365-2958.2009.06932.x
Murakami, K. S., and Darst, S. A. (2003). Bacterial RNA polymerases: the wholo story. Curr. Opin. Struct. Biol. 13, 31–39. doi: 10.1016/S0959-440X(02)00005-2
Nikata, T., Sakai, Y., Shibat, K., Kato, J., Kuroda, A., and Ohtake, H. (1996). Molecular analysis of the phosphate-specific transport (pst) operon of Pseudomonas aeruginosa. Mol. Gen. Genet. 250, 692–698.
Ochs, M., Veitinger, S., Kim, I., Welz, D., Angerer, A., and Braun, V. (1995). Regulation of citrate-dependent iron transport of Escherichia coli: fecR is required for transcription activation by FecI. Mol. Microbiol. 15, 119–132. doi: 10.1111/j.1365-2958.1995.tb02226.x
Ó Cuív, P., Clarke, P., and O'connell, M. (2006). Identification and characterization of an iron-regulated gene, chtA, required for the utilization of the xenosiderophores aerobactin, rhizobactin 1021 and schizokinen by Pseudomonas aeruginosa. Microbiology 152, 945–954. doi: 10.1099/mic.0.28552-0
Pradel, E., and Locht, C. (2001). Expression of the putative siderophore receptor gene bfrZ is controlled by the extracytoplasmic-function sigma factor BupI in Bordetella bronchiseptica. J. Bacteriol. 183, 2910–2917. doi: 10.1128/JB.183.9.2910-2917.2001
Qiu, D., Eisinger, V. M., Rowen, D. W., and Yu, H. D. (2007). Regulated proteolysis controls mucoid conversion in Pseudomonas aeruginosa. Proc. Natl. Acad. Sci. U.S.A. 104, 8107–8112. doi: 10.1073/pnas.0702660104
Rojas, A., Segura, A., Guazzaroni, M. E., Teran, W., Hurtado, A., Gallegos, M. T., et al. (2003). In vivo and in vitro evidence that TtgV is the specific regulator of the TtgGHI multidrug and solvent efflux pump of Pseudomonas putida. J. Bacteriol. 185, 4755–4763. doi: 10.1128/JB.185.16.4755-4763.2003
Sambrook, J., Fritsch, E. F., and Maniatis, T. (1989). Molecular Cloning: A Laboratory Manual. Cold Spring Harbor, NY: Cold Spring Harbor Laboratory Press.
Spaink, H. P., Okker, R. J. H., Wijffelman, C. A., Pees, E., and Lugtenberg, B. J. J. (1987). Promoters in the nodulation region of the Rhizobium leguminosarum Syn plasmid pRL1JI. Plant Mol. Biol. 9, 27–39. doi: 10.1007/BF00017984
Spencer, M. R., Beare, P. A., and Lamont, I. L. (2008). Role of cell surface signaling in proteolysis of an alternative sigma factor in Pseudomonas aeruginosa. J. Bacteriol. 190, 4865–4869. doi: 10.1128/JB.01998-07
Staroń, A., Sofia, H. J., Dietrich, S., Ulrich, L. E., Liesegang, H., and Mascher, T. (2009). The third pillar of bacterial signal transduction: classification of the extracytoplasmic function (ECF) sigma factor protein family. Mol. Microbiol. 74, 557–581. doi: 10.1111/j.1365-2958.2009.06870.x
Stock, A. M., Robinson, V. L., and Goudreau, P. N. (2000). Two-component signal transduction. Annu. Rev. Biochem. 69, 183–215. doi: 10.1146/annurev.biochem.69.1.183
Thompson, J. D., Higgins, D. G., and Gibson, T. J. (1994). CLUSTAL W: improving the sensitivity of progressive multiple sequence alignment through sequence weighting, position-specific gap penalties and weight matrix choice. Nucleic Acids Res. 22, 4673–4680. doi: 10.1093/nar/22.22.4673
Visca, P., Leoni, L., Wilson, M. J., and Lamont, I. L. (2002). Iron transport and regulation, cell signalling and genomics: lessons from Escherichia coli and Pseudomonas. Mol. Microbiol. 45, 1177–1190. doi: 10.1046/j.1365-2958.2002.03088.x
Wilson, M. J., and Lamont, I. L. (2006). Mutational analysis of an extracytoplasmic-function sigma factor to investigate its interactions with RNA polymerase and DNA. J. Bacteriol. 188, 1935–1942. doi: 10.1128/JB.188.5.1935-1942.2006
Winsor, G. L., Lam, D. K., Fleming, L., Lo, R., Whiteside, M. D., Yu, N. Y., et al. (2011). Pseudomonas Genome Database: improved comparative analysis and population genomics capability for Pseudomonas genomes. Nucleic Acids Res. 39, D596–D600. doi: 10.1093/nar/gkq869
Zaborin, A., Gerdes, S., Holbrook, C., Liu, D. C., Zaborina, O. Y., and Alverdy, J. C. (2012). Pseudomonas aeruginosa overrides the virulence inducing effect of opioids when it senses an abundance of phosphate. PLoS ONE 7:e34883. doi: 10.1371/journal.pone.0034883
Zaborin, A., Romanowski, K., Gerdes, S., Holbrook, C., Lepine, F., Long, J., et al. (2009). Red death in Caenorhabditis elegans caused by Pseudomonas aeruginosa PAO1. Proc. Natl. Acad. Sci. U.S.A. 106, 6327–6332. doi: 10.1073/pnas.0813199106
Keywords: Pseudomonas aeruginosa, gene regulation, signal transduction, extracytoplasmic function sigma factor, phosphate starvation, PhoB
Citation: Quesada JM, Otero-Asman JR, Bastiaansen KC, Civantos C and Llamas MA (2016) The Activity of the Pseudomonas aeruginosa Virulence Regulator σVreI Is Modulated by the Anti-σ Factor VreR and the Transcription Factor PhoB. Front. Microbiol. 7:1159. doi: 10.3389/fmicb.2016.01159
Received: 04 May 2016; Accepted: 12 July 2016;
Published: 03 August 2016.
Edited by:
Dongsheng Zhou, Beijing Institute of Microbiology and Epidemiology, ChinaReviewed by:
Christopher Morton Thomas, University of Birmingham, UKGrzegorz Wegrzyn, University of Gdańsk, Poland
Copyright © 2016 Quesada, Otero-Asman, Bastiaansen, Civantos and Llamas. This is an open-access article distributed under the terms of the Creative Commons Attribution License (CC BY). The use, distribution or reproduction in other forums is permitted, provided the original author(s) or licensor are credited and that the original publication in this journal is cited, in accordance with accepted academic practice. No use, distribution or reproduction is permitted which does not comply with these terms.
*Correspondence: María A. Llamas, marian.llamas@eez.csic.es