- Institute for Water Research, and Department of Microbiology, University of Granada, Granada, Spain
A collection of desiccation-tolerant xeroprotectant-producing microorganisms was screened for their ability to protect plants against drought, and their role as plant growth-promoting rhizobacteria was investigated in two different crops (tomato and pepper). The most commonly described biochemical mechanisms for plant protection against drought by microorganisms including the production of phytohormones, antioxidants and xeroprotectants were analyzed. In particular, the degree of plant protection against drought provided by these microorganisms was characterized. After studying the findings and comparing them with results of the closest taxonomic relatives at the species and strain levels, we propose that trehalose produced by these microorganisms is correlated with their ability to protect plants against drought. This proposal is based on the increased protection of plants against drought by the desiccation-sensitive microorganism Pseudomonas putida KT2440, which expresses the otsAB genes for trehalose biosynthesis in trans.
Introduction
Drought, the main limiting factor for crop yields worldwide (Sharp et al., 2004), is considered the abiotic stress with the greatest effect on plants, since 45% of global farming lands are subjected to continuous or frequent droughts (Long and Ort, 2010). The Intergovernmental Panel on Climate Change (IPCC) has projected that the land area affected by drought will increase while water resources in the affected areas could decline by as much as 30% by mid-century (Christensen et al., 2007).
In this context, ethylene, abscisic acid (ABA) and indoleacetic acid (IAA) are phytohormones often associated with plant senescence or root control (Stella et al., 1996) and have also been associated with how plants respond to water deficit (Yamaguchi-Shinozaki and Shinozaki, 1994; Kawakami et al., 2010). Ethylene is synthesized from 1-amino cyclopropane-1-carboxylic acid (ACC) by the enzyme ACC oxidase (ACO or ethylene-forming enzyme; EC:1.14.17.4), and regulates a number of physiological mechanisms. At low concentrations, it promotes the development of adventitious roots and root hairs (Nadeem et al., 2010), and at high concentrations it inhibits root growth under stress conditions such as drying soil (Glick et al., 2007). With regard to ABA, this key phytohormone causes stomatal closure to prevent further decreases in water content, promotes root growth and the accumulation of compatible solutes, and regulates the synthesis of dehydrins as well as late embryogenesis abundant (LEA) proteins, thus coordinating various facets of the plant’s response to low water potential (ψw) (Pierce and Raschke, 1980; Zhu, 2002; Raschke, 2006). Some auxins, including IAA, when present in low concentrations, increase cell elongation, resulting in greater root growth and the generation of lateral roots. However, at higher concentrations IAA, inhibits root growth either directly or indirectly via the promotion of ethylene synthesis. The effect of ethylene is intertwined with the production of IAA, since on the one hand ethylene has been shown to lower endogenous IAA levels in different plant tissues, and on the other hand IAA stimulates ethylene production by promoting ACC synthase activity (Glick, 2003; Dimkpa et al., 2009).
The accumulation of xeroprotectants such as trehalose by some microorganisms and some plants enables them to withstand extreme abiotic stress such as desiccation (Chaplin, 2006; Julca et al., 2012). Efforts have been made to bolster plant drought tolerance by developing transgenic plants with altered phytohormone production (Sobeih et al., 2004) and greater trehalose concentrations (Goddijn and Smeekens, 1998; Pilon-Smits et al., 1998). In addition to transgenic plants, plant-growth-promoting rhizobacteria (PGPR) have great potential in agriculture since their presence in the roots increases crop production by enhancing the plant’s tolerance to drought and other types of environmental stress (Vilchez and Manzanera, 2011). According to Timmusk et al. (2013), these bacteria are called rhizobacterial drought-tolerance enhancers (RDTE). Over the last decade, RDTE have received particular attention (Glick et al., 2007), and since Mayak et al. (2004) reported that the strain Achromobacter piechaudii ARV8 was useful in protecting plants against drought, many other microorganisms have been described with similar properties. In general, these microorganisms are able to counteract the negative effects of drought stress in plants by producing ACC deaminase (ACCd), lowering ABA, or raising IAA production (Mayak et al., 2004). See Kaushal and Wani (2016) for a recent review on this subject.
Water stress also affects the viability of microorganisms in different ways (Manzanera et al., 2002) by compromising protein structure, stability, activity, folding, and assembly by disrupting the physical architecture and functions of nucleic acids; and by altering membrane structure and function (Chaplin, 2006; Raschke, 2006; Julca et al., 2012). A large number of RDTE have been isolated in samples from dry or drought-affected locations (Mayak et al., 2004; Dimkpa et al., 2009; Roca et al., 2013), thus suggesting that this type of microorganism might be well adapted to water stress.
Drought conditions would therefore be expected to produce the simultaneous selection of tolerant plants and microorganisms in the environment, assuming that the selection of desiccation-tolerant microorganisms counteracts the deleterious effect of drought on plants (Lau and Lennon, 2012). However, no study available has drawn a clear connection between a microbial phenotype and the ability to protect plants from drying. The present study establishes a correlation between tolerance to desiccation in a set of highly desiccation-tolerant PGPR and their closest taxonomic relatives at the species and strain level, and the level of plant protection against drought in two different crops (tomato and pepper plants). This correlation between desiccation tolerance of the microorganism and its ability to protect some plants from drought seems to depend on the microorganism’s capacity to finely regulate the concentration of trehalose in the plant as a signal of drying damage, since trehalose triggers the plant-defense system to counteract the damage caused by drought. To demonstrate the role of microbial trehalose in protecting the plant against desiccation, we isolated the otsAB genes, coding for alpha, alpha-trehalose-phosphate synthase and trehalose-6-phosphate phosphatase, from the highly desiccation-tolerant strain Microbacterium sp. 3J1, and inserted them into the desiccation-sensitive microorganism P. putida KT2440, generating P. putida KT2440 (pUCP22:otsAB) with a significant increase in the intracellular concentration of trehalose under water stress conditions. In addition, plants inoculated with the resulting trehalose-overexpressing P. putida KT2440 (pUCP22:otsAB) showed a significantly greater tolerance to drought.
Materials and Methods
Microorganisms, Media, and Culture Conditions
The strains used in this study are shown in Table 1. The organisms described here will be made available upon request. Bacteria were grown in tryptic soy broth (TSB) or M9 Minimal Medium (Sigma M6030) with glucose or fructose (50 mM) as the sole carbon source at 30°C (Manzanera et al., 2004). To prepare hypersaline minimal medium (HMM), we added NaCl (6 M) to M9 medium at the concentration specified by Manzanera et al. (2002). To generate hyperosmotic conditions, we added 5% or 50% (wt/vol) polyethylene glycol (PEG) 6000 was added to the media (Sandhya et al., 2009).
Air Drying: Determination of Survival Rates
A colony of each pure culture containing 107–109 cells was suspended in 1 mL M9 Minimal Medium. Aliquot fractions (100 μL) were placed on sterile Petri dishes and dried under a current of sterile air for 24 h. Cells were suspended in 1 mL sterile saline buffer, and serial dilutions of the cells before and after drying were plated on trypticase soy agar (TSA) plates. All the experiments were performed at room temperature. The survival rate was calculated as CFU/mL after drying with reference to the pre-drying CFU/mL, expressed as a percentage. The assays were performed in triplicate following to Narváez-Reinaldo et al. (2010).
Plant Material and Growth Conditions
Tomato (Lycopersicum esculentum Mill cv. F144) and green pepper (Capsicum annuum L. cv. Maor) seedlings were started from sterile seeds that were sown in plastic trays in wet vermiculite accordingly to Mayak et al. (2004). After 1 week, uniform-sized seedlings (shoot height approximately 3 cm) were selected and planted in non-sterile soil composed of a mixture of plant substrate (black peat, vegetable compost, white peat, and coconut pH 7.2 and 56% organic matter) and vermiculite (1:1), one per pot, using 0.4 L pots filled with approx. 0.26 L of soil mixture. The pots were incubated in a Climates Ing GROW growth chamber at constant relative humidity (50–60%). The chamber was lit with a 12-h day/night cycle and gradual dimming/brightening of the light to simulate dawn and dusk. The day cycle consisted of 200 μmol photons⋅m-2⋅s-1, and the dawn–dusk cycle consisted of 150 μmol photons⋅m-2⋅s-1. The temperature was programmed to change from 18 to 20°C for the night cycle to 20–25°C in the diurnal cycle. Seedlings were regularly watered during the first 2 weeks. On day 7, after being transferred to the pots, the plants were fertilized with basal salt Murashige and Skoog medium diluted 1/10 (vol/vol) (Murashige and Skoog, 1962) and on day 14, they were inoculated with the bacterial strains. This time was considered experimental day 0 for plant growth-promotion tests.
At inoculation, seedlings were treated with 40 mL of bacterial suspension (108–109 CFU/mL) in sterile M9 buffer, and non-inoculated controls were watered with sterile M9 buffer. Inoculated and non-inoculated plants were watered for 2 weeks after the seedlings were transplanted.
Monitoring of Plant Growth
After 2 weeks, watering with the buffer was stopped, and this time was considered time 0 for the drought-tolerance-enhancement tests. Thus, dry weight (DW), fresh weight (FW), fully turgid weight (FTW) of the whole plants free from soil were measured four times after inoculation at 7, 14, 21, and 33 days. The relative water content (RWC) was calculated according to Mayak et al. (2004) as follows: RWC = (FW-DW) × (TW-DW)-1. In addition, root length (RL) and stem length (SL) were recorded.
Trehalose Production by Bacterial Strains
Intracellular trehalose was determined following to Manzanera et al. (2002) with slight modifications. Samples of 1 mL were aliquoted from cultures in the exponential growth phase, and were then centrifuged and washed in 1 × M9 minimal medium without a carbon source. The bacterial pellets were lysed with FastPrep, and the trehalose concentration was determined by ion chromatography in a high-performance 940 Professional IC Vario 2 chromatography system (Metrohm, Switzerland) with a MetroSTEP Carb 2 copolymer polystyrene-divinylbenzene column (with quaternary ammonium groups) measuring 250 mm by 4.0 mm (Metrohm, Switzerland). The column temperature was set at 30°C at a pressure of 130 atmospheres (1911 psi). Trehalose concentrations were calculated relative to a reference standard curve, and efficiency of the process relative to sucrose detected was calculated with reference to the viable cells counted in the initial cultures.
16S rRNA Gene Analysis
To identify the most closely related bacterial species to the desiccation-tolerant strain collection, the nearly complete sequence of 16S rRNA from the different isolates was aligned with the sequences in closely related species. A phylogenetic tree of alignments was generated with the CLUSTAL X 2 program (Larkin et al., 2007), and phylogenetic trees were inferred with the neighbor-joining and maximum-likelihood methods using the MEGA 5.0 software package (Tamura et al., 2011). Bootstrap analysis was based on 1,000 resamplings. The distances were calculated according to Kimura’s two-parameter model.
Construction of the Plasmid-Expressing otsAB
To construct an otsAB-expressing plasmid, a 2.291-kb otsAB gene fragment from Microbacterium sp. 3J1 was amplified with the oligonucleotides OtsAB-F (5′AGGAATTCCACCCATGCCAGCCGCA 3′) and OtsAB-R (5′TCAAGCTTGGACATGACGAGAGAGTCTATTCCCG 3′). Oligonucleotides were designed with the help of Clone Manager software, and the 30-cycles PCR program included 30 s denaturation steps at 94°C, followed by 30 s annealing steps at 55°C and 150 s extension steps at 72°C. The EcoRI and HindIII sites, which cut into the polylinker of the vector, but not into the insert sequence, were included in the oligonucleotides (underlined). The plasmid was then cloned into pUCP22 to obtain the vector pUCP22:otsAB in Escherichia coli DH5α (West et al., 1994). The sequence of the resulting construct was analyzed by Sanger sequencing, and P. putida KT2440 was transformed with both plasmids (pUCP22 and pUCP22:otsAB) by electroporation, as described previously (Enderle and Farwell, 1998). Trehalose production by the transformed strains was analyzed as described in the preceding section (Trehalose production by bacterial strains).
Superoxide Dismutase and Catalase Activity of Bacterial Strains
Superoxide dismutase activity was determined according to Beauchamp and Fridovich (1971).
In addition, CAT activity of the strains was determined with the method of Aebi (1984) by measuring the decomposition of H2O2 directly as the decrease in absorbance at 240 nm.
Indoleacetic Acid, Abscisic Acid, and Gibberellic Acid Production by Strains
The production of IAA by bacterial strains was determined following to Bano and Musarrat (2003). Abscisic acid and gibberellic acid (GA3) production were detected and measured with thin-layer chromatography (TLC) and quantified by high-performance liquid chromatography (HPLC) as in Karadeniz et al. (2006), with slight modifications taken from Ross et al. (1987). Strains were grown in TSB at 120 rpm and 30°C until they reached the exponential growth phase. Next, 100 mL of the cultures were extracted and centrifuged at 4,800 × g for 15 min, and the samples were filtered to sterilize the supernatants. They were supplemented with 1 mL butylated hydroxytoluene (BHT) to prevent hormone oxidation. Extraction was done with ethyl acetate and concentration in a rotary evaporator prior to TLC. Chromatography was performed in a mixture of isopropanol:ammonia:distilled water (10:1:1, vol/vol/vol) on silica gel TLC plates (60F254 TLC, Merck).
Salicylic Acid Production and Aminocyclopropane Carboxylic Acid Consumption by Strains
Qualitative determination of salicylic acid (SA) by TLC followed to the protocol of Buysens et al. (1996), and quantitative determinations were performed by colorimetry. The consumption of ACC by bacterial strains was determined as in Penrose and Glick (2003).
Data Analyses
Analysis of covariance (ANCOVA) was used to determine whether desiccation tolerance of plants determined as FW, DW, FTW, RWC and the size of plants subjected to drought considered as plant responses (continuous predictive variables or covariates) was influenced by bacterial activity. This activity was measured as the concentration of phytohormones, antioxidants, and trehalose (dependent variables) produced by the different microorganisms. Prior to the ANCOVA test, Levene’s test for equality of variances was performed. When Levene’s test was positive (P < 0.05) the variances in the groups were considered different, and therefore the assumptions for the ANCOVA were not met. Pearson correlation coefficients were calculated to investigate the relationship between plant variables and microbial activity.
Results and Discussion
Pepper and tomato plants were used to test the ability of five highly desiccation-tolerant microorganisms to protect plants against drought. All five bacterial strains were previously isolated in our laboratory: Microbacterium sp. 3J1, Arthrobacter koreensis 5J12A, A. siccitolerans 4J27, Rhodococcus sp. 4J2A2, and Leucobacter sp. 4J7B1 (Narváez-Reinaldo et al., 2010). For a negative control, we used the desiccation-sensitive PGPR P. putida KT2440, and for a positive control, we used the well-characterized RDTE A. piechaudii 366-5 (Manzanera et al., 2002; Dos Santos et al., 2004; Mayak et al., 2004). An additional set of non-inoculated plants was used as a negative control. Plants were inoculated and subjected to drying conditions as described in the experimental procedures section. After 33 days in the absence of water, both non-inoculated pepper plants and plants inoculated with desiccation-sensitive P. putida KT2440 showed signs of dying (Figure 1A). Similar results were seen when the plants were inoculated with A. siccitolerans 4J27, Leucobacter sp. 4J7B1 and Rhodococcus sp. 4J2A2. However, pepper plants inoculated with the most desiccation-tolerant isolates, Microbacterium sp. 3J1 and A. koreensis 5J12A, as well as with A. piechaudii 366-5, appeared healthy and yielded the highest values of DW (Figure 1B), FW (Figure 1C), FTW (Figure 1D), and RWC (Figure 1E), and had the longest roots (Figure 1F) and stems (Figure 1G) at the end of the experiment. Soil moisture was reduced from 71.52 to 13.75%. No statistical differences were found in the soil moisture regardless of the applied strain applied. Analogous results were found when tomato plants were used, thus validating these findings in a different plant species (Supplementary Figure S1). However, some minor but significant protection against drought was detected in tomato plants when Rhodococcus sp. 4J2A2 was used as the inoculant. These results suggest that some plant species are more sensitive than others to certain xerotolerant strains, since Rhodococcus sp. 4J2A2 showed a xeroprotectant effect in tomato but not in pepper plants under our assay conditions. In addition, these results suggest that only the strains showing the highest desiccation tolerance protect certain plants against drought.
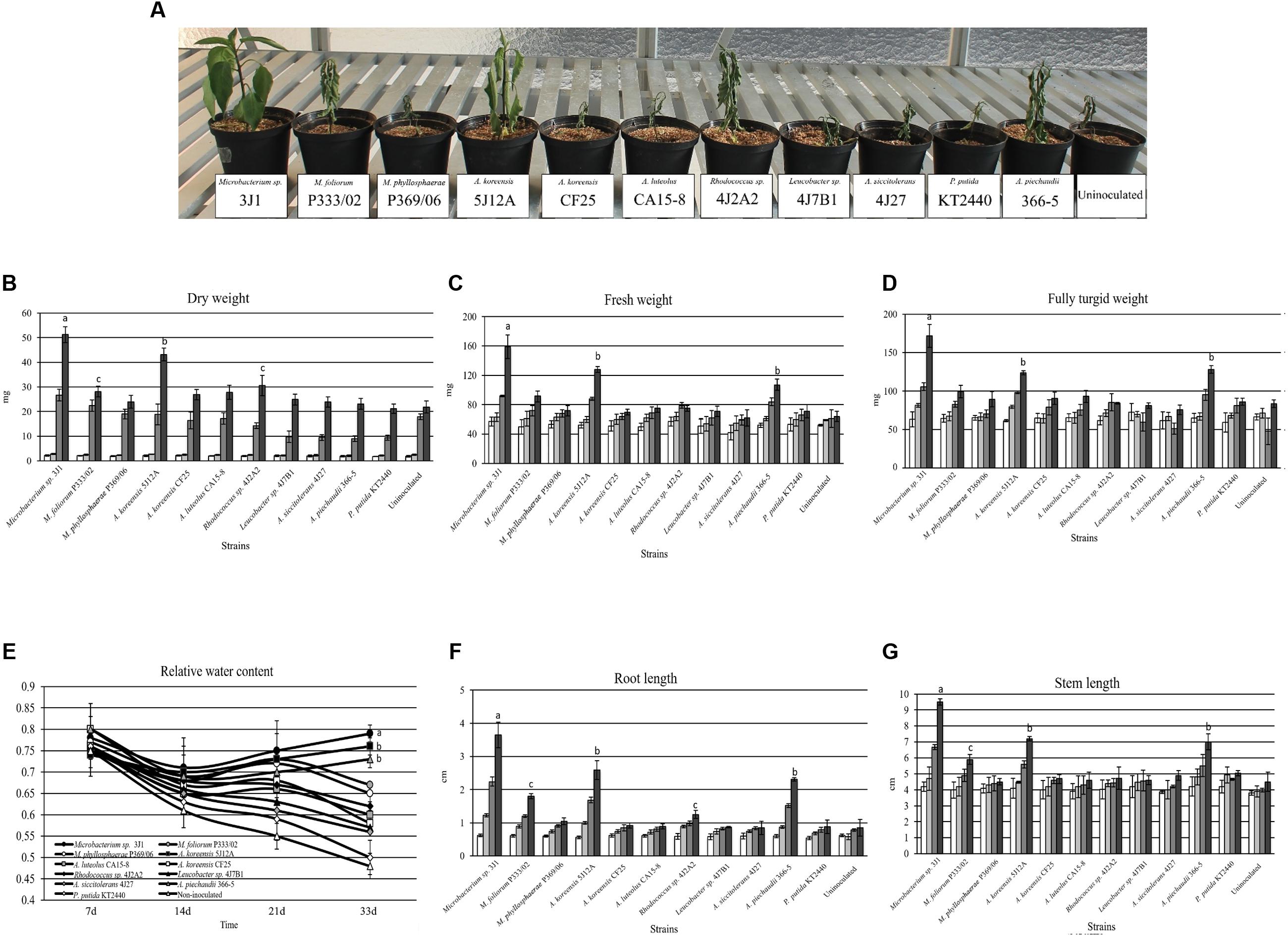
FIGURE 1. The effect of desiccation-tolerant microorganisms on pepper plants subjected to water stress. (A) Shows the physical appearance of pepper plants after 33 days without watering. (B,C) show the DW and FW (mg), respectively, of whole pepper plants free from soil. (D–G) show the FTW (mg), RWC, RL, and SL, respectively, of whole pepper plants free from soil. White bars correspond to day 7, light-gray bars correspond to day 14, dark bars correspond to day 21 and black bars correspond to day 33. Values are the means of three replicates ±SD.
To determine whether this protective effect was a specific characteristic of the isolate itself or was a property shared by strains that are closely related taxonomically, we compared the sequence for the 16S rRNA gene in strains Microbacterium sp. 3J1 (GenBank accession number GU815136) and A. koreensis 5J12A (GenBank accession number GU815140; Narváez-Reinaldo et al., 2010) with the sequence from the EzTaxon server corresponding to the same gene1 in order to identify the most closely related strains. The nearly complete sequence for the 16S rRNA gene in strains Microbacterium sp. 3J1 and A. koreensis 5J12A (approximately 1,500 bp) was aligned with the sequences in closely related species of the genera Microbacterium and Arthrobacter, including A. piechaudii ATCC 43552 as an outgroup. The resulting neighbor-joining trees are shown in Figure 2.
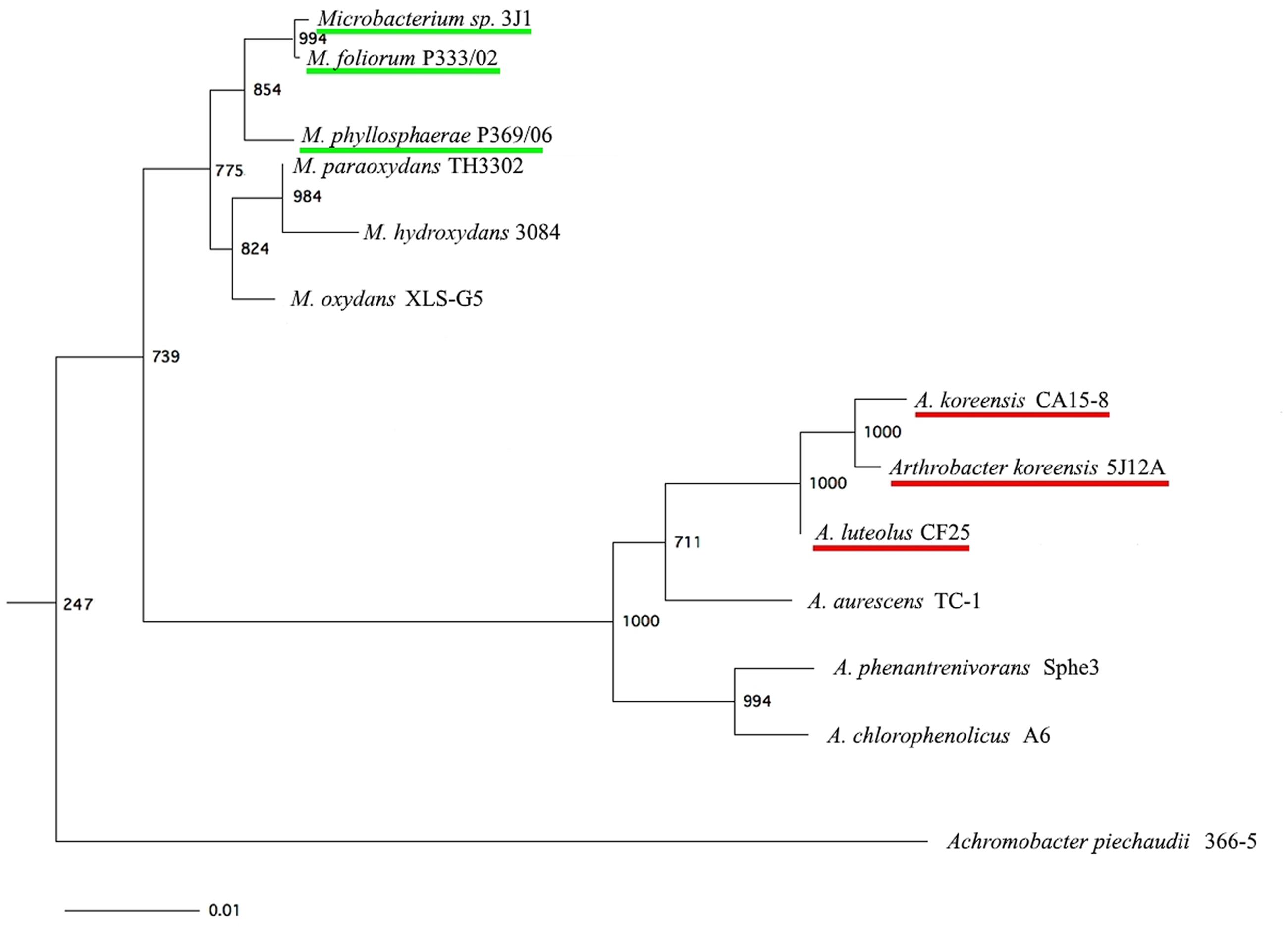
FIGURE 2. Neighbor-joining phylogenetic tree based on the 16S rRNA sequence of strains Microbacterium sp. 3J1 and A. koreensis 5J12A (both with the latter’s closest relative strains), and A. piechaudii 366-5, used as outgroup. Numbers at the bifurcations indicate how many times each species occurred at this position. Bar, 0.01 changes per nucleotide position.
Once, we determined their taxonomic affiliations, the bacterial strains identified as most closely related to Microbacterium sp. 3J1 were M. foliorum P333/02 (Behrendt et al., 2001) and M. phyllosphaerae P369/06 (Behrendt et al., 2001), while the strains found to be most closely related to A. koreensis 5J12A proved to be A. koreensis CA15-8 (Lee et al., 2003) and A. luteolus CF25 (Wauters et al., 2000). Further comparisons showed that the desiccation tolerance provided by Microbacterium sp. 3J1 and A. koreensis 5J12A appeared to correlate with the degree of drought protection they provided to the plant. In other words, plants were most tolerant to drought when they were inoculated with Microbacterium sp. 3J1 and A. koreensis 5J12A, according to the high values, we found for desiccation-tolerance parameters. The intermediate levels of desiccation tolerance provided by M. foliorum P333/02, M. phyllospherae P369/06, A. koreensis CA15-8, and A. luteolus CF25 were translated into intermediate levels of protection of the plant against drought.
In light of our results and earlier findings in the genera studied here, we suggest a possible correlation between the degree of desiccation tolerance in a given PGPR and the level of protection against drought it confers to the plant. To identify the molecule responsible for plant protection, we tested the strategies most commonly used by RDTE. Our panel of assays tested the in vitro production of phytohormones by the microorganisms involved in protection against desiccation (IAA, GA3, ABA, and SA) as well as ACC consumption (Table 2), antioxidant molecules involved preventing and repairing damage caused by reactive oxygen species (ROS) [superoxide dismutase (SOD) and catalase (CAT)] (Figure 3), and trehalose as a xeroprotectant involved in the conservation of essential biomolecules (Figure 4). Our ANCOVA (one-way ANOVA, p < 0.05) results showed a correlation between plant-growth parameters, e.g., DW, FW and FTW, RWC, RL, and shoot length (SL), and the concentration of trehalose produced by the microbial cells (Figure 5). This analysis indicates that the highest trehalose production by the strain Microbacterium sp. 3J1 correlated strongly with the highest values of DW, FW, RWC, and RL in plants inoculated with this strain, as documented by coefficients of determination, R2 above 0.8. Moreover, trehalose production by P. putida KT2440 (a desiccation-sensitive strain) and the rest of the analyzed strains analyzed showed similar correlations, which indicate that the values of all these parameters depend on trehalose production. This molecule which can stabilize essential biomolecules in plant cells, has been linked to the modulation of plant metabolism via the induction of stress-response genes, mostly transcriptional factors and protein kinases (Schluepmann et al., 2004). Bae et al. (2005) found that 30 mM trehalose, when added exogenously, was linked to the regulation of ethylene- and jasmonate-signaling pathways. The presence of trehalose thus appears to lead to cross-talk with ABA signaling in different physiological processes, including the regulation of stomatal aperture size (Gómez et al., 2010). Inoculation of Medicago truncatula with an IAA-overproducing strain of Sinorhizobium meliloti led to the accumulation of trehalose in the bacterial cell, and re-modulation of the plant’s phytohormone profile (Bianco and Defez, 2009). These results are evidence of a two-way interaction system between trehalose as the elicitor produced by the bacteria and the phytohormone regulatory map of the plant. In addition, exogenously added trehalose has been associated with protection against oxidative damage by reducing ROS accumulation, increasing non-enzymatic antioxidants, and co-activating the antioxidative and glyoxalase system (Mostofa et al., 2014). A more in-depth discussion of these bi-directional regulatory mechanisms was published by Lunn et al. (2014) in a recent review of trehalose metabolism in plants (Lunn et al., 2014).
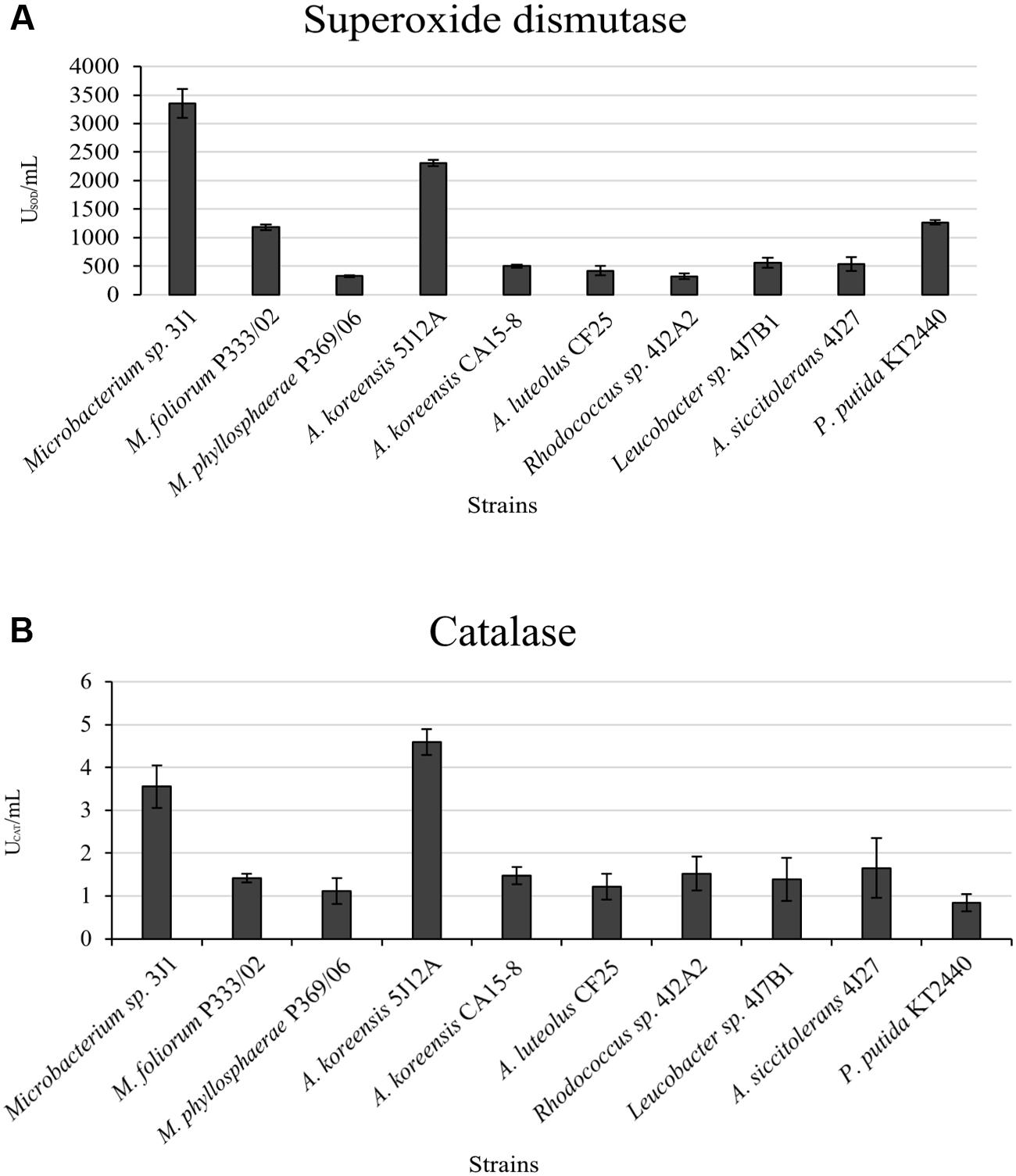
FIGURE 3. Antioxidant enzyme activity in different strains. (A) shows SOD in USOD/mL; and (B) shows CAT in UCAT/mL (units of enzyme activity, U). Pseudomonas putida KT2440 was used as the control strain. Values are the means of three replicates ±SD.
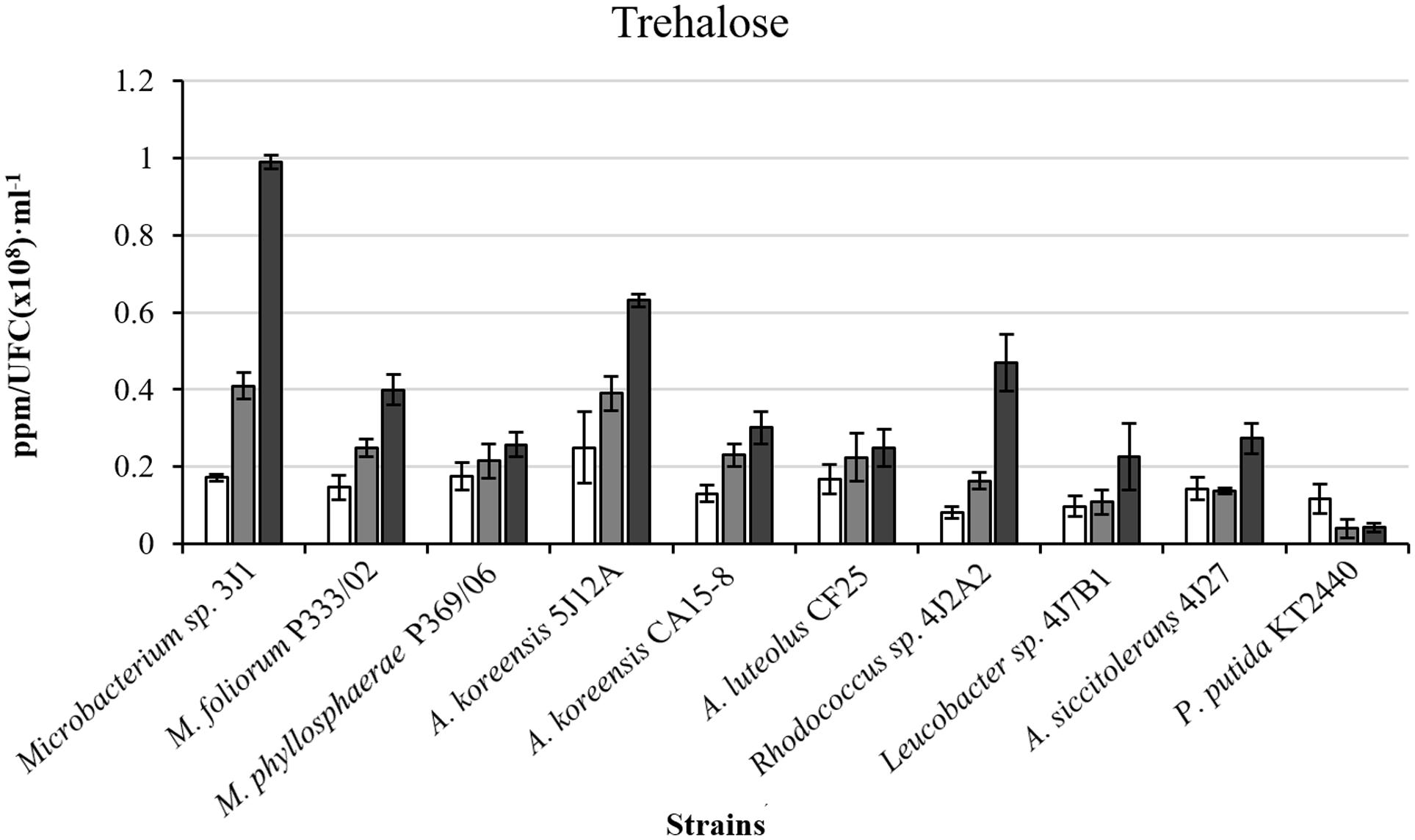
FIGURE 4. Intracellular trehalose production in ppm/UFC (×108)⋅mL-1 by bacterial strains in different polyethylene glycol (PEG) concentrations. White bars correspond to cultures in the absence of PEG (0% PEG); gray bars, correspond to cultures supplemented with 5% PEG; and black bars, to cultures supplemented with 50% PEG. P. putida KT2440 was used as the control strain. Values are the means of three replicates ±SD.
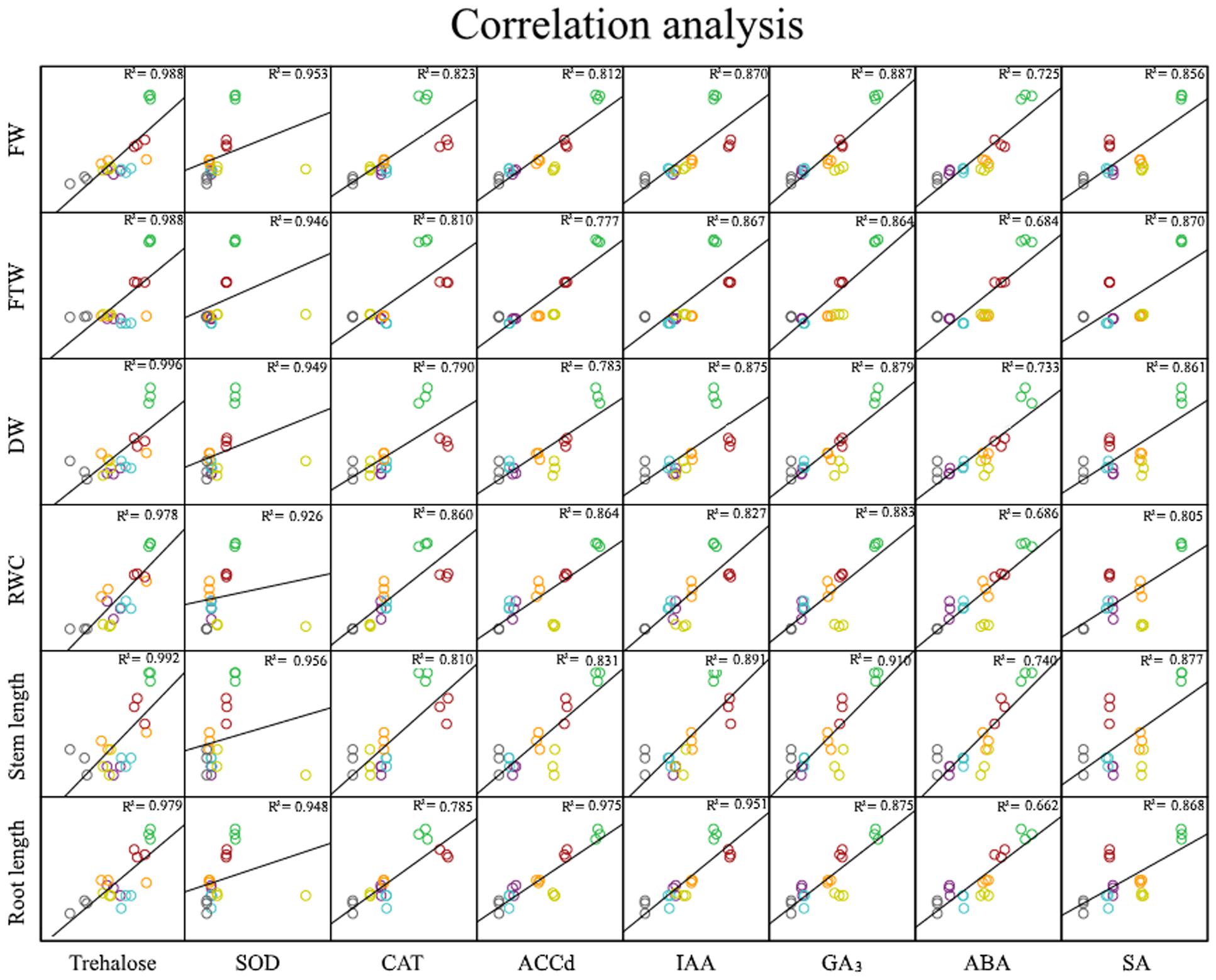
FIGURE 5. Analysis of statistical correlation. One-way ANCOVA for the correlations between production values by each strain of trehalose, SOD, CAT, ACCd, IAA, GA3, ABA, SA, and values for plant DW, FW, FTW, RWC, shoot length, and root length. Green circles correspond to Microbacterium sp. 3J1; red circles correspond to A. koreensis 5J12A; orange circles correspond to Rhodococcus sp. 4J2A2; violet circles correspond to Leucobacter sp. 4J7B1; blue circles correspond to A. siccitolerans 4J27; yellow circles correspond to P. putida KT2440, and gray circles correspond to non-inoculated samples.
Salt stress in plants is known to induce the accumulation of ROS species such as H2O2. To avoid or alleviate the risk of ROS accumulation, plants respond by activating different scavenging pathways (Fujita et al., 2006). Among the scavenging systems, SOD is the primary scavenger in the detoxification of ROS induced by oxidative stress in plants. Other scavenging enzymes, including the ascorbate–glutathione cycle enzymes (ascorbate peroxidase and glutathione reductase) and CAT, also play an important role in detoxifying of the toxic cellular products of SOD (i.e., H2O2) in plant cells (Mittler, 2002). Moreover, exogenous trehalose is able to differentially modulate antioxidant enzymes and the expression of related plant genes (Nounjan et al., 2012). To elucidate the role of trehalose production by the microorganisms in protecting the plant against desiccation applied to the plant, we assayed the effect of otsAB genes from Microbacterium sp. 3J1 that were expressed in P. putida KT2440 under the constitutive expression of the Plac promoter in the broad-host-range vector pUCP22 in order to generate the pUCP22:otsAB vector (West et al., 1994). The trehalose concentration in P. putida KT2440 (pUCP22:otsAB) increased more than one order of magnitude (from 12.12 to 134.14 ppm/CFU(108)⋅ml-1) compared to the concentration in P. putida KT2440 (pUCP22), and was nearly half the trehalose production by Microbacterium sp. 3J1 when grown in the presence of PEG 50% (-2.64 MPa). In addition, when we compared the desiccation tolerance of pepper plants inoculated with the trehalose-overproducing P. putida KT2440 (pUCP22:otsAB) to plants inoculated with P. putida KT2440 (pUCP22), we found a significant increase in desiccation tolerance in the former based on the ANOVA test (p < 0.05). DW of the plants inoculated with P. putida KT2440 (pUCP22:otsAB) was 1.13- and 1.2-fold higher than for plants inoculated with P. putida KT2440 (pUCP22) and uninoculated plants, respectively, while we observed an increase in RWC of 1.21-fold compared to plants inoculated with P. putida KT2440 (pUCP22) and 1.68-fold compared to non-inoculated plants (Figure 6).
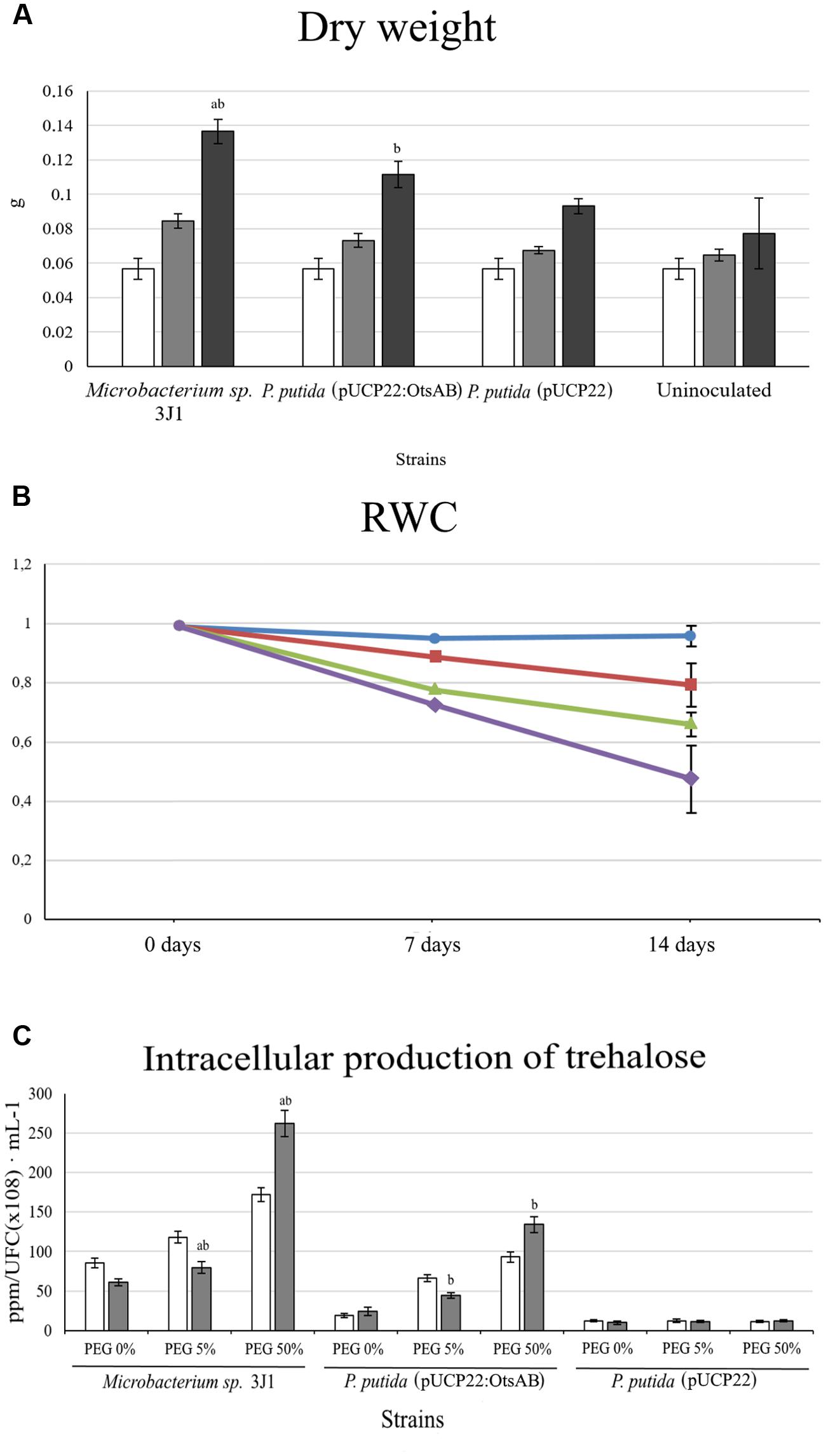
FIGURE 6. Trehalose production by P. putida KT2440 containing the otsAB genes from Microbacterium sp. 3J1 and effect over pepper plants. (A) Shows the DW of pepper plants inoculated with P. putida KT2440 (pUCP22), P. putida (pUCP22:otsAB) and Microbacterium sp. 3J1. Where white bars correspond to day 0, light-gray bars correspond to day 7, black bars correspond to day 14. Values are the means of three replicates ±SD. (B) Shows the relative water content of pepper plants inoculated with P. putida KT2440 (pUCP22; triangles), P. putida (pUCP22:otsAB; squares), and Microbacterium sp. 3J1 (circles), as well as non-inoculated plants (diamonds) at time 0, 7, and 14 days. Values are the means of three replicates ±SD. (C) Shows the intracellular concentration of trehalose in the absence of PEG and in presence of 5 and 50% PEG. White bars correspond to cultures grown on M9 minimal medium, and gray bars to cultures grown on TSB medium. All bars show SD; abindicates a significant difference with respect to all other results; bindicates a significant difference with respect to control.
These results point to a possible link between the degree of desiccation tolerance imparted by trehalose production by a given PGPR and the level of protection against drought that the organism confers to plants. Isolation assays have been performed to select RDTE from different locations such as quarry sand and riverside sand. All genera isolated in these studies belonged to Actinobacteria, one of the groups with the highest desiccation-tolerance values. This characteristic may reflect their natural abundance in soils, which may be explained by the broader tolerance of this genus to abiotic stress such as drought, extreme cold and starvation (Deutch and Perera, 1992; Zevenhuizen, 1992). We speculate that the high desiccation tolerance of these microorganisms enables the survival of a sufficient number of cells to colonize and establish interactions with the plant prior to the establishment of the symbiotic protection of the plant.
This is the first report available to link desiccation tolerance of a microorganism to the protection they confer to plants against drought. Plant-survival rate seems to correlate with the ability of the microbial cell to produce trehalose. The trehalose produced by the microorganism may control most of the plant’s enzymatic and non-enzymatic responses by favoring the production of the plant’s repertoire of phytohormones.
Conclusion
We propose that the ability of microorganisms to produce trehalose under drying conditions can facilitate their own survival and that of the plant being colonized. This makes the selection of highly desiccation-tolerant PGPR potentially useful in agriculture as biostimulants to protect plants against drought.
Author Contributions
JV, CG-F, and DR-N performed the experimental assays. JV, JG-L, and MM have performed the statistical analysis. JV and MM have designed and written the article.
Conflict of Interest Statement
The authors declare that the research was conducted in the absence of any commercial or financial relationships that could be construed as a potential conflict of interest.
Acknowledgments
This research was funded by the Spanish Ministry of Science and Innovation under the aegis of research project CTM2009-09270 and the Andalusian Regional Government under the aegis of research project P11-RNM-7844.
Supplementary Material
The Supplementary Material for this article can be found online at: http://journal.frontiersin.org/article/10.3389/fmicb.2016.01577
FIGURE S1 | The effect of desiccation-tolerant microorganisms on tomato plants subjected to water stress. (A) Shows the physical appearance of pepper plants after 33 days without watering. (B–D) show the DW, FW and FTW (mg), respectively, of whole tomato plants free from soil. (E–G) show the RWC, RL and SL, respectively, of whole tomato plants free from soil. White bars correspond to day 7, light-gray bars correspond to day 14, dark bars correspond to day 21 and black bars correspond to day 33. Values are the means of 3 replicates ± SD.
Footnotes
References
Aebi, H. (1984). Catalase in vitro. Methods Enzymol. 105, 121–126. doi: 10.1016/S0076-6879(84)05016-3
Bae, H., Herman, E., Bailey, B., Bae, H.-J., and Sicher, R. (2005). Exogenous trehalose alters Arabidopsis transcripts involved in cell wall modification, abiotic stress, nitrogen metabolism, and plant defense. Physiol. Plant. 125, 114–126. doi: 10.1111/j.1399-3054.2005.00537.x
Bano, N., and Musarrat, J. (2003). Isolation and characterization of phorate degrading soil bacteria of environmental and agronomic significance. Lett. Appl. Microbiol. 36, 349–353. doi: 10.1046/j.1472-765X.2003.01329.x
Beauchamp, C., and Fridovich, I. (1971). Superoxide dismutase: improved assays and an assay applicable to acrylamide gels. Anal. Biochem. 44, 276–287. doi: 10.1016/0003-2697(71)90370-8
Behrendt, U., Ulrich, A., and Schumann, P. (2001). Description of Microbacterium foliorum sp. nov. and Microbacterium phyllosphaerae sp. nov., isolated from the phyllosphere of grasses and the surface litter after mulching the sward, and reclassification of Aureobacterium resistens (Funke et al. 1998) as Microbacterium resistens comb. nov. Int. J. Syst. Evol. Microbiol. 51, 1267–1276.
Bianco, C., and Defez, R. (2009). Medicago truncatula improves salt tolerance when nodulated by an indole-3-acetic acid-overproducing Sinorhizobium meliloti strain. J. Exp. Bot. 60, 3097–3107. doi: 10.1093/jxb/erp140
Buysens, S., Heungens, K., Poppe, J., and Hofte, M. (1996). Involvement of pyochelin and pyoverdin in suppression of pythium-induced damping-off of tomato by Pseudomonas aeruginosa 7NSK2. Appl. Environ. Microbiol. 62, 865–871.
Chaplin, M. (2006). Do we underestimate the importance of water in cell biology? Nat. Rev. Mol. Cell. Biol. 7, 861–866. doi: 10.1038/nrm2021
Christensen, J. H., Hewitson, B., Busuioc, A., Chen, A., Gao, X., Held, R., et al. (2007). “Regional climate projections,” in Climate Change: The Physical Science Basis Contribution of Working Group I to the Fourth Assessment Report of the Intergovernmental Panel on Climate Change, eds S. Solomon, D. Qin, M. Manning, Z. Chen, M. Marquis, K. B. Averyt, et al. (Cambridge: University Press).
Deutch, C. E., and Perera, G. S. (1992). Myceloid cell formation in Arthrobacter globiformis during osmotic stress. J. Appl. Bacteriol. 72, 493–499. doi: 10.1111/j.1365-2672.1992.tb01865.x
Dimkpa, C., Weinand, T., and Asch, F. (2009). Plant-rhizobacteria interactions alleviate abiotic stress conditions. Plant Cell Environ. 32, 1682–1694. doi: 10.1111/j.1365-3040.2009.02028.x
Dos Santos, V. A., Heim, S., Moore, E. R., Stratz, M., and Timmis, K. N. (2004). Insights into the genomic basis of niche specificity of Pseudomonas putida KT2440. Environ. Microbiol. 6, 1264–1286. doi: 10.1111/j.1462-2920.2004.00734.x
Enderle, P. J., and Farwell, M. A. (1998). Electroporation of freshly plated Escherichia coli and Pseudomonas aeruginosa cells. BioTechniques 25, 954–956.
Fujita, M., Fujita, Y., Noutoshi, Y., Takahashi, F., Narusaka, Y., Yamaguchi-Shinozaki, K., et al. (2006). Crosstalk between abiotic and biotic stress responses: a current view from the points of convergence in the stress signaling networks. Curr. Opin. Plant Biol. 9, 436–442. doi: 10.1016/j.pbi.2006.05.014
Glick, B. R. (2003). Phytoremediation: synergistic use of plants and bacteria to clean up the environment. Biotechnol. Adv. 21, 383–393. doi: 10.1016/S0734-9750(03)00055-7
Glick, B. R., Todorovic, B., Czarny, J., Cheng, Z., Duan, J., and McConkey, B. (2007). Promotion of plant growth by bacterial ACC deaminase. Crit. Rev. Plant Sci. 26, 227–242. doi: 10.1080/07352680701572966
Goddijn, O., and Smeekens, S. (1998). Sensing trehalose biosynthesis in plants. Plant J. 14, 143–146. doi: 10.1046/j.1365-313X.1998.00140.x
Gómez, L. D., Gilday, A., Feil, R., Lunn, J. E., and Graham, I. A. (2010). AtTPS1-mediated trehalose 6-phosphate synthesis is essential for embryogenic and vegetative growth and responsiveness to ABA in germinating seeds and stomatal guard cells. Plant J. 64, 1–13. doi: 10.1111/j.1365-313X.2010.04312.x
Julca, I., Alaminos, M., Gonzalez-Lopez, J., and Manzanera, M. (2012). Xeroprotectants for the stabilization of biomaterials. Biotechnol. Adv. 30, 1641–1654. doi: 10.1016/j.biotechadv.2012.07.002
Karadeniz, A., Topcuoğlu,Ş. F., and İnan, S. (2006). Auxin, gibberellin, cytokinin and abscisic acid production in some bacteria. World J. Microbiol. Biotechnol. 22, 1061–1064. doi: 10.1007/s11274-005-4561-1
Kaushal, M., and Wani, S. P. (2016). Plant-growth-promoting rhizobacteria: drought stress alleviators to ameliorate crop production in drylands. Ann. Microbiol. 66, 35–42. doi: 10.1007/s13213-015-1112-3
Kawakami, E., Oosterhuis, D., and Snider, J. (2010). Physiological effects of 1-methylcyclopropene on well-watered and water-stressed cotton plants. J. Plant Growth Regul. 29, 280–288. doi: 10.1007/s00344-009-9134-3
Kiredjian, M., Holmes, B., Kersters, K., Guilvout, I., and De Ley, J. (1986). Alcaligenes piechaudii, a new species from human clinical specimens and the environment. Int. J. Syst. Evol. Microbiol. 36, 282–287.
Larkin, M. A., Blackshields, G., Brown, N. P., Chenna, R., McGettigan, P. A., McWilliam, H., et al. (2007). Clustal W and Clustal X version 2.0. Bioinformatics 23, 2947–2948. doi: 10.1093/bioinformatics/btm404
Lau, J. A., and Lennon, J. T. (2012). Rapid responses of soil microorganisms improve plant fitness in novel environments. Proc. Natl. Acad. Sci. U.S.A. 109, 14058–14062. doi: 10.1073/pnas.1202319109
Lee, J. S., Lee, K. C., Pyun, Y. R., and Bae, K. S. (2003). Arthrobacter koreensis sp. nov., a novel alkalitolerant bacterium from soil. Int. J. Syst. Evol. Microbiol. 53, 1277–1280. doi: 10.1099/ijs.0.02492-0
Long, S. P., and Ort, D. R. (2010). More than taking the heat: crops and global change. Curr. Opin. Plant Biol. 13, 241–248. doi: 10.1016/j.pbi.2010.04.008
Lunn, J. E., Delorge, I., Figueroa, C. M., Van Dijck, P., and Stitt, M. (2014). Trehalose metabolism in plants. Plant J. 79, 544–567. doi: 10.1111/tpj.12509
Manzanera, M., Garcia, de Castro, A., Tondervik, A., Rayner-Brandes, M., Strom, A. R., et al. (2002). Hydroxyectoine is superior to trehalose for anhydrobiotic engineering of Pseudomonas putida KT2440. Appl. Environ. Microbiol. 68, 4328–4333. doi: 10.1128/AEM.68.9.4328-4333.2002
Manzanera, M., Vilchez, S., and Tunnacliffe, A. (2004). Plastic encapsulation of stabilized Escherichia coli and Pseudomonas putida. Appl. Environ. Microbiol. 70, 3143–3145. doi: 10.1128/AEM.70.5.3143-3145.2004
Mayak, S., Tirosh, T., and Glick, B. R. (2004). Plant growth-promoting bacteria confer resistance in tomato plants to salt stress. Plant Physiol. Biochem. 42, 565–572. doi: 10.1016/j.plaphy.2004.05.009
Mittler, R. (2002). Oxidative stress, antioxidants and stress tolerance. Trends Plant Sci. 7, 405–410. doi: 10.1016/S1360-1385(02)02312-9
Mostofa, M. G., Yoshida, N., and Fujita, M. (2014). Spermidine pretreatment enhances heat tolerance in rice seedlings through modulating antioxidative and glyoxalase systems. Plant Growth Regul. 73, 31–44. doi: 10.1007/s10725-013-9865-9
Murashige, T., and Skoog, F. (1962). A revised medium for rapid growth and bio assays with tobacco tissue cultures. Physiol. Plant. 15, 473–497. doi: 10.1111/j.1399-3054.1962.tb08052.x
Nadeem, S. M., Zahir, Z. A., Naveed, M., Asghar, H. N., and Arshad, M. (2010). Rhizobacteria capable of producing ACC-deaminase may mitigate salt stress in sheat. Soil Sci. Soc. Am. J. 74, 533–542. doi: 10.2136/sssaj2008.0240
Narváez-Reinaldo, J. J., Barba, I., Gonzalez-Lopez, J., Tunnacliffe, A., and Manzanera, M. (2010). Rapid method for isolation of desiccation-tolerant strains and xeroprotectants. Appl. Environ. Microbiol. 76, 5254–5262. doi: 10.1128/AEM.00855-10
Nelson, K. E., Weinel, C., Paulsen, I. T., Dodson, R. J., Hilbert, H., Martins dos Santos, V. A. P., et al. (2002). Complete genome sequence and comparative analysis of the metabolically versatile Pseudomonas putida KT2440. Environ. Microbiol. 4, 799–808. doi: 10.1046/j.1462-2920.2002.00366.x
Nounjan, N., Nghia, P. T., and Theerakulpisut, P. (2012). Exogenous proline and trehalose promote recovery of rice seedlings from salt-stress and differentially modulate antioxidant enzymes and expression of related genes. J. Plant Physiol. 169, 596–604. doi: 10.1016/j.jplph.2012.01.004
Penrose, D. M., and Glick, B. R. (2003). Methods for isolating and characterizing ACC deaminase-containing plant growth-promoting rhizobacteria. Physiol. Plant. 118, 10–15. doi: 10.1034/j.1399-3054.2003.00086.x
Pierce, M., and Raschke, K. (1980). Correlation between loss of turgor and accumulation of abscisic acid in detached leaves. Planta 148, 174–182. doi: 10.1007/BF00386419
Pilon-Smits, E. A. H., Terry, N., Sears, T., Kim, H., Zayed, A., Hwang, S., et al. (1998). Trehalose-producing transgenic tobacco plants show improved growth performance under drought stress. J. Plant Physiol. 152, 525–532. doi: 10.1016/S0176-1617(98)80273-3
Raschke, T. M. (2006). Water structure and interactions with protein surfaces. Curr. Opin. Struct. Biol. 16, 152–159. doi: 10.1016/j.sbi.2006.03.002
Roca, A., Pizarro-Tobias, P., Udaondo, Z., Fernandez, M., Matilla, M. A., Molina-Henares, M. A., et al. (2013). Analysis of the plant growth-promoting properties encoded by the genome of the rhizobacterium Pseudomonas putida BIRD-1. Environ. Microbiol. 15, 780–794. doi: 10.1111/1462-2920.12037
Ross, G. S., Elder, P. A., McWha, J. A., Pearce, D., and Pharis, R. P. (1987). The development of an indirect enzyme linked immunoassay for abscisic acid. Plant Physiol. 85, 46–50. doi: 10.1104/pp.85.1.46
Sandhya, V., Ali, S. K. Z., Grover, M., Reddy, G., and Venkateswarlu, B. (2009). Alleviation of drought stress effects in sunflower seedlings by the exopolysaccharides producing Pseudomonas putida strain GAP-P45. Biol. Fertil. Soils 46, 17–26. doi: 10.1007/s00374-009-0401-z
Schluepmann, H., van Dijken, A., Aghdasi, M., Wobbes, B., Paul, M., and Smeekens, S. (2004). Trehalose mediated growth inhibition of Arabidopsis seedlings is due to trehalose-6-phosphate accumulation. Plant Physiol. 135, 879–890. doi: 10.1104/pp.104.039503
Sharp, R. E., Poroyko, V., Hejlek, L. G., Spollen, W. G., Springer, G. K., Bohnert, H. J., et al. (2004). Root growth maintenance during water deficits: physiology to functional genomics. J. Exp. Bot. 55, 2343–2351. doi: 10.1093/jxb/erh276
Sobeih, W. Y., Dodd, I. C., Bacon, M. A., Grierson, D., and Davies, W. J. (2004). Long-distance signals regulating stomatal conductance and leaf growth in tomato (Lycopersicon esculentum) plants subjected to partial root-zone drying. J. Exp. Bot. 55, 2353–2363. doi: 10.1093/jxb/erh204
Stella, L., Wouters, S., and Baldellon, F. (1996). Chemical and biochemical aspects of the biosynthesis of ethylene, a plant hormone. Bull. Soc. Chim. Fr. 133, 441–455.
Tamura, K., Peterson, D., Peterson, N., Stecher, G., Nei, M., and Kumar, S. (2011). MEGA5: molecular evolutionary genetics analysis using maximum likelihood, evolutionary distance, and maximum parsimony methods. Mol. Biol. Evol. 28, 2731–2739. doi: 10.1093/molbev/msr121
Timmusk, S., Timmusk, K., and Behers, L. (2013). Rhizobacterial plant drought stress tolerance enhancement: towards sustainable water resource management and food security. J. Food Sec. 1, 6–10.
Vilchez, S., and Manzanera, M. (2011). Biotechnological uses of desiccation-tolerant microorganisms for the rhizoremediation of soils subjected to seasonal drought. Appl. Microbiol. Biotechnol. 91, 1297–1304. doi: 10.1007/s00253-011-3461-6
Wauters, G., Charlier, J., Janssens, M., and Delmee, M. (2000). Identification of Arthrobacter oxydans, Arthrobacter luteolus sp. nov., and Arthrobacter albus sp. nov., isolated from human clinical specimens. J. Clin. Microbiol. 38, 2412–2415.
West, S. E., Schweizer, H. P., Dall, C., Sample, A. K., and Runyen-Janecky, L. J. (1994). Construction of improved Escherichia-Pseudomonas shuttle vectors derived from pUC18/19 and sequence of the region required for their replication in Pseudomonas aeruginosa. Gene. 11, 81–86. doi: 10.1016/0378-1119(94)90237-2
Yamaguchi-Shinozaki, K., and Shinozaki, K. (1994). A novel cis-acting element in an Arabidopsis gene is involved in responsiveness to drought, low-temperature, or high-salt stress. Plant Cell 6, 251–264. doi: 10.2307/3869643
Zevenhuizen, L. P. (1992). Levels of trehalose and glycogen in Arthrobacter globiformis under conditions of nutrient starvation and osmotic stress. Antonie Van Leeuwenhoek 61, 61–68. doi: 10.1007/BF00572124
Keywords: desiccation tolerance, rhizobacterial drought-tolerance enhancers (RDTE), plant-growth-promoting rhizobacteria (PGPR), trehalose, Pseudomonas putida KT2440
Citation: Vílchez JI, García-Fontana C, Román-Naranjo D, González-López J and Manzanera M (2016) Plant Drought Tolerance Enhancement by Trehalose Production of Desiccation-Tolerant Microorganisms. Front. Microbiol. 7:1577. doi: 10.3389/fmicb.2016.01577
Received: 04 July 2016; Accepted: 21 September 2016;
Published: 30 September 2016.
Edited by:
Pierre-Emmanuel Courty, University of Fribourg, SwitzerlandReviewed by:
David Dowling, Institute of Technology Carlow, IrelandAli SkZ, Agri Biotech Foundation, India
Catalina Cabot, Universitat de les Illes Balears, Spain
Copyright © 2016 Vílchez, García-Fontana, Román-Naranjo, González-López and Manzanera. This is an open-access article distributed under the terms of the Creative Commons Attribution License (CC BY). The use, distribution or reproduction in other forums is permitted, provided the original author(s) or licensor are credited and that the original publication in this journal is cited, in accordance with accepted academic practice. No use, distribution or reproduction is permitted which does not comply with these terms.
*Correspondence: Maximino Manzanera, manzanera@ugr.es