- Division of Infectious Diseases, University Medical Center Freiburg, Freiburg, Germany
Sporulation is the most enduring survival strategy developed by several bacterial species. However, spore development of the model organism Bacillus subtilis has mainly been studied by means of media or conditions optimized for the induction of sporogenesis. Here, I show that during prolonged growth during stationary phase in minimal medium, B. subtilis undergoes an asymmetric cell division that produces small and round-shaped, DNA containing cells. In contrast to wild-type cells, mutants harboring spo0A or spoIIIE/sftA double mutations neither sporulate nor produce this special cell type, providing evidence that the small round cells emerge from the abortion of endospore formation. In most cases observed, the small round cells arise in the presence of sigma H but absence of sigma F activity, different from cases of abortive sporulation described for rich media. These data suggest that in minimal media, many cells are able to initiate but fail to complete spore development, and therefore return to normal growth as rods. This work reveals that the continuation of asymmetric cell division, which results in the formation of the small round cells, is a way for cells to delay or escape from—unsuccessful—sporulation. Based on these findings, I suggest to name the here described cell type as “dwarf cells” to distinguish them from the well-known minicells observed in mutants defective in septum placement or proper chromosome partitioning.
Introduction
In response to nutrient deprivation, some bacterial species within the Firmicutes phylum differentiate into dormant and non-reproductive cells called endospores. This highly resistant form of bacteria can survive harsh conditions that are usually lethal to their vegetative counterparts. The model organism, Bacillus subtilis, is to date the best-studied organism in terms of spore development. Numerous genes are up- or down-regulated or even switched on or off in the process of endospore formation. The process initially involves asymmetric cell division near one of the cell poles, which results in the formation of a smaller cell, the prespore or forespore, and a larger cell, the mother cell (stages I–II). The latter cell subsequently engulfs the forespore (stages II–III), ensures its proper development to the endospore [cortex synthesis (stage IV); spore coat synthesis and spore maturation (stages V–VI)], and finally undergoes autolysis releasing a mature spore (stage VII) (Errington, 1993, 2003; Stragier and Losick, 1996; Eijlander et al., 2014).
B. subtilis cells grown to stationary phase are heterogenic as they display a multitude of cell types associated with distinct differentiation pathways (Kearns and Losick, 2005). This heterogeneity is in part due to the bistable expression/activation (“ON” or “OFF”) states of regulatory proteins (Dubnau and Losick, 2006). In B. subtilis, the entry into sporulation is subject to bistability (Veening et al., 2005), which is regulated by the expression and phosphorylation level of the transcription factor and response regulator Spo0A~P (Hoch, 1993). Spore development is initiated when the cellular level of Spo0A~P reaches the threshold level (Fujita et al., 2005). Indeed, the level of Spo0A~P increases gradually as the cell enters the stationary phase (Fujita and Losick, 2005). The level of Spo0A~P is tightly regulated through a phosphorelay system. Environmental stimuli, cell cycle cues or metabolic factors trigger the autophosphorylation of KinA, the first kinase of the system. Thereafter, a flux of phosphate follows through the phosphorelay to the final acceptor Spo0A via intermediate kinases Spo0F and Spo0B, respectively (Burbulys et al., 1991; Grossman, 1991; Trach et al., 1991; Sonenshein, 2000). Sublevels of Spo0A~P are proposed to mediate the regulation of alternative developmental fates such as cannibalism and biofilm formation (Fujita and Losick, 2005). The decision of the cell to sporulate is thus the ultimate adaptive response to starvation. The success of the spore development resides in the compartmentalization of the gene expression established by two sigma factors σF in the forespore and σE in the mother cell (Errington and Illing, 1992). Both sigma factors become active only after the completion of the septum. This later event is reported to set the commitment to sporulation: The “point of no return” (Losick and Stragier, 1992; Parker et al., 1996; Dworkin and Losick, 2005). However, the activation of both σF and σE depends on subthreshold levels of Spo0A activity. At some subthreshold levels of Spo0A activity, cells that activate σF may fail to activate σE causing the abortion of sporulation, while most of the cells with active σE produce spores (Narula et al., 2012). This finding rather sets the σE activation as the ultimate sporulation decision point.
Most studies investigating spore development rely on three major protocols involving chemical induction (i.e., by the addition of decoyinine) (Grossman and Losick, 1988), resuspension method (Sterlini and Mandelstam, 1969) or the exhaustion in Difco sporulation medium (DSM) (Schaeffer et al., 1965b). These methods aim at maximizing sporulation efficiency and therefore do only poorly reflect sporulation under undisturbed growth conditions. Because of this experimental bias, only little is known on the sporulation behavior of wild-type B. subtilis grown in standard growth media. To address this issue, I set out to investigate stationary phase cells, which were grown in S750 minimal medium, conventionally used for B. subtilis lab cultures.
In this medium, spore development is not an intrinsically robust process since many cells do not follow this pathway after asymmetric division. Instead, the unequally sized cells separate by generating an unusually small round and a normal cell. These findings reveal that the formation of small round cells by spore forming bacteria could provide an escape route from unsuccessful sporulation, or, alternatively, provide a strategy to avoid the costly spore development process under minimal medium growth condition.
Materials and Methods
Growth Conditions
Strains were grown at room temperature (25°C) with shaking at 200 rpm in Lysogeny Broth (LB) (Bertani, 2004), DSM (Schaeffer et al., 1965b), or S750 minimal medium containing 50 mM MOPS (adjusted to pH 7.0 with KOH), 10 mM (NH4)2SO4, 5 mM potassium phosphate (pH 7.0), 2 mM MgCl2, 0.7 mM CaCl2, 50 μM MnCl2, 1 μM ZnCl2, 1 μg/ml thiamine-HCl, 20 μM HCl, 5 μM FeCl3 and 1% glucose supplemented with 0.1% glutamate and 0.004% casamino acids (Grossman and Losick, 1988; Harwood and Cutting, 1990). Spectinomycin (100 μg/ml), chloramphenicol (5 μg/ml), macrolide-lincosamide-streptogramin (MLS) (1 μg/ml erythromycin and 25 μg/ml lincomycin), or kanamycin (10 μg/ml) were added to the growth medium where appropriate. The growth medium was supplemented with 1 μM IPTG when required. For comparative analysis of growth, small round cells formation and sporulation, samples were collected at regular time intervals for OD600 measurement and evaluation of the % of small round cells and sporulation. The % of small round cells was determined by cell counts on acquired images. The evaluation of the % of sporulation is described below (see the “heat kill assay” method). Unless stated otherwise, samples were collected after 90–100 h of growth for microscopy. To investigate the viability of small round cells, cultures of B. subtilis generating small round cells were diluted 1:10 into fresh growth medium and grown for 60 min prior to the time-lapse experiments. Table 1 lists all the strains used in this work.
Heat Kill Assay
Samples were collected in regular time intervals and serially diluted with sterile double distilled water (ddH2O). The number of spores was measured as heat-resistant (20 min at 80°C) colony-forming units (CFU) on LB plates. In parallel, viable cells were measured as total colony-forming units (CFU) on LB plates. In this case, serial dilutions were performed in sterile 0.9% NaCl. % sporulation = [spores/ml)/(cells/ml)] × 100% (Grossman and Losick, 1988). However, because the numbers of live vegetative cells decline after the start of the stationary phase, the percentage of sporulation during stationary phase was calculated relative to the number of viable cells reaching the stationary phase.
Image Acquisition
Cells were mounted on agarose gel pads containing S750 medium (Harwood and Cutting, 1990) on microscope slides. Fluorescence microscopy was performed on a Zeiss Axio Imager A1 or a Zeiss Axio Observer Z1 microscope equipped with a CoolSnap HQ CCD camera (Photometrics) or pco.edge camera (scientific CMOS camera) respectively. The electronic data processing was conducted with Metamorph 6.3-Software (Meta Imaging Software) or VisiView Test-Version 2.1.1 (Visitron Systems GmbH). Fluorescence intensities were measured using the ROI manager tools of ImageJ (v1.48; Rasband,W.S., ImageJ, U.S. National Institutes of Health, Bethesda, Maryland, USA, http://imagej.nih.gov/ij/, 1997-2014) (Schneider et al., 2012).
Results
B. subtilis Wild-Type Cells Generate Small Round Cells Containing DNA during Prolonged Growth in Minimal S750 Medium
Endospore formation is the preferred response of B. subtilis cells to different nutrient-limited conditions in minimal medium (Chubukov and Sauer, 2014). However, the extent to which this bacterium can adjust its structure and physiology during extended stationary phase in optimum growth condition is less known.
To follow sporulation behavior of wild-type B. subtilis cells during prolonged stationary growth under laboratory conditions, minimal S750 medium (Grossman and Losick, 1988; Harwood and Cutting, 1990) was chosen for this study, which is frequently used to grow B. subtilis. For comparative analysis Lysogeny Broth (LB) (Bertani, 2004) and sporulation medium DSM (Schaeffer et al., 1965b) were also used. Cells were grown at room temperature (25°C) with shaking at 200 rpm and samples were collected at regular time intervals for OD600 measurement, microscopy analysis and evaluation of the % of sporulation. Whenever B. subtilis was grown in S750 minimal medium, LB broth or DSM medium, sporulation occurred earlier in S750 and DSM media (~ 12 h) compared to LB broth (~ 21 h). In all media, the percentage of spores in relation to the total viable population increased with time and yielded 0.94 and 0.21% in S750 medium and LB broth, respectively, after 126 h (Figures 1A,B; the sporulation data are shown in Tables S1,S2). Meanwhile, spores readily accumulated in DSM medium and reached 14.5% of total viable counts after 126 h (Figure 1C; the sporulation data are shown in Table S3). Spores were formed already during exponential growth phase in S750 medium (Figure 1A), in contrast to DSM medium and LB broth, in which spores appeared only when cell density declined (Figures 1B,C). These observations indicate that spore development in DSM medium and LB broth arose upon nutrient exhaustion, while in S750 medium the heterogeneity of the cell population with respect to sporogenesis started earlier during growth.
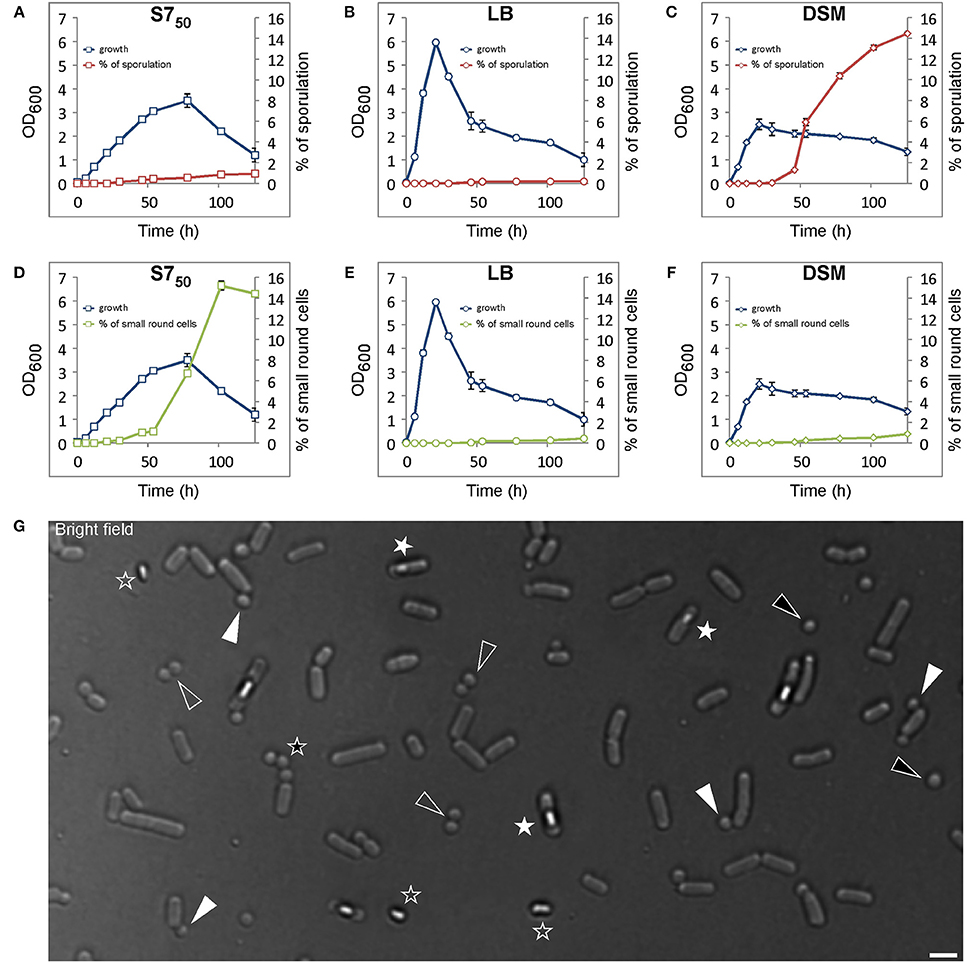
Figure 1. Wild-type B. subtilis cells generate small round cells during prolonged growth. Comparative analysis of growth and spore formation (A–C) and comparative analysis of growth and small round cells formation (D–F) in different media over time. Error bars represent standard deviations of the means of two independent experiments. Small round cells counts and sporulation data are shown in Supplemental Data (Table S1–S4). (G) Representative view of cells acquired at time point 100 h grown in S750 at room temperature. White triangles indicate small round cells still attached to their larger siblings, black triangles indicate free small round cells, white asterisks indicate sporulated cells, black asterisk or empty triangles indicate a triplet or doublets of small round cells respectively from consecutive polar divisions. Empty asterisks indicate released spores. Scale bars 2 μm.
Interestingly, in addition to spores, the formation of small round cells was observed that appeared in a way similar to the well-characterized minicells (Adler et al., 1967; Reeve et al., 1973) (Figures 1D–G). Appearance of this cell type could be detected in all media, but was most prominent in S750, in which a rapid increase was noted at the transition between logarithmic growth and stationary phase. The small round cells eventually constituted 15.2% of the total cell counts (1750 cells counted; small round cells counts data are shown in Table S4) in S750 (Figure 1D) compared to 0.45 or 0.9% in LB or DSM, respectively (Figures 1E,F, the small round cells counts data are shown in Tables S5,S6). The small round cells exhibited diameters varying from 0.7 to 1.15 μm (150 cells measured) (Figure 1G). The undomesticated B. subtilis strain (NCIB 3610) as well as other species such as Bacillus atrophaeus, pumilus, and sphaericus (Figure S1) also produced small round cells, suggesting that this phenotype is not a trait of the lab strain PY79 and may be conserved among endospore-forming bacteria.
Small Round Cells Evolve from the Abortion of Sporulation
A brief analysis of the small round cells showed that they were significantly different from minicells because of the presence of DNA in these cells visualized by DAPI staining (Figure 2A), which was absent in minicells from a mutant defective in proper placement of the cell division machinery (Figure 2B).
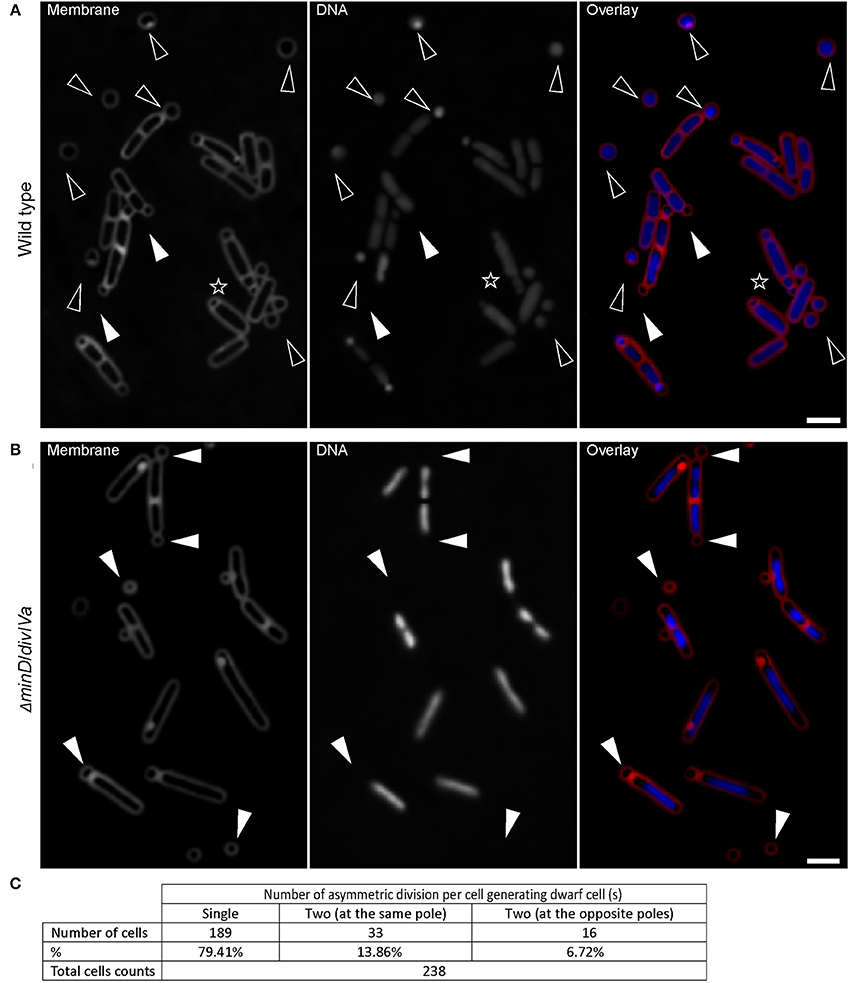
Figure 2. Wild-type B. subtilis cells generate small round cells containing DNA. (A) Wild-type cells showing that almost all small round cells contain DNA (black triangles). White triangles indicate two small round cells devoid of DNA. 98.33 ± 0.58% (mean ± SD of three independent experiments; > 250 cells counted each) of small round cells generated from wild-type cells contain DNA, while minicells generated from a B. subtilis minD/divIV double mutant (B) are all devoid of DNA. White triangles point out some minicells. The black asterisk in panel (A) indicates a cell with multiple attempts of sporulation. Cells were grown in S750 for about 100 and 28 h for wild-type and minD/divIV double mutant cells respectively. (C) Quantification of the frequency of the number of asymmetric divisions occurring per cell generating dwarf cell (s). Scale bars 2 μm.
The question that immediately arose was to find out the origin of these small round cells. The accumulation of small round cells in the growth medium as the culture reached the stationary phase where sporulation events should have happened more frequently suggests that the occurrence of small round cells in S750 could be the result of failed spore development.
B. subtilis has different developmental states that are mostly controlled by Spo0A, the master regulator of the stationary phase. To further confirm that there is a link between sporogenesis and small round cells formation, the expression level of Spo0A using a gfp fusion to the spoIIG promoter was monitored. spoIIG is a sporulation gene which is controlled by Spo0A (Satola et al., 1992). Spo0A is the sporulation initiator protein and its intracellular level determines the entry of cells into the sporulation process. High levels of Spo0A are required for the activation of sporulation-specific genes (Fujita et al., 2005). Figures 3A,B show that the intensity of GFP (expressed from spoIIG promoter from an ectopic locus) fluorescence signals in cells producing small round cells (54171.84 ± 30261.937 arb. Units; mean ± SD of 178 measurements) was similar to that of sporulating cells (60386.68 ± 26532.14 arb. Units; mean ± SD of 193 measurements). This suggests that the Spo0A concentration in cells producing the small round cells was sufficient to trigger sporulation. Moreover, all cells producing the small round cells exhibited GFP fluorescence suggesting that small round cells formation requires the activity of Spo0A. Indeed, I also investigated a spo0A mutant which is known to arrest sporulation at stage 0 (Piggot and Coote, 1976). No single small round cells (observation from three independent experiments, 300 cells analyzed each) could be detected in this strain (Figure 3C). Cells lacking spo0H gene, which codes for σH that directs genes expression at an early stage of sporulation, also did not generate small round cells (observation from two independent experiments, 350 cells analyzed each). However, for unknown reasons spo0H deletion strains investigated in this work exhibited elongated shaped cells (Figure 3D). Because sporulation is the unique developmental state requiring an asymmetric septation, these findings are consistent with the idea that the small round cells can only be formed upon initiation of spore development. Thus, the small round cells evolve from the inability of cells to complete sporulation. Of the cells generating the small round cells (the small round cells still being attached to their larger siblings), 20% (Figure 2C, 238 cells counted) underwent more than one asymmetric division at the same pole or opposite poles (Figures 1G, 2A, black asterisk), indicating that a failure to develop a spore does not imply that the cell returns to the vegetative state, and thereby allowing a subsequent reinitiation of sporulation. Thus, the initial sporulation signals are rather strong and remain stable in cells producing the small round cells.
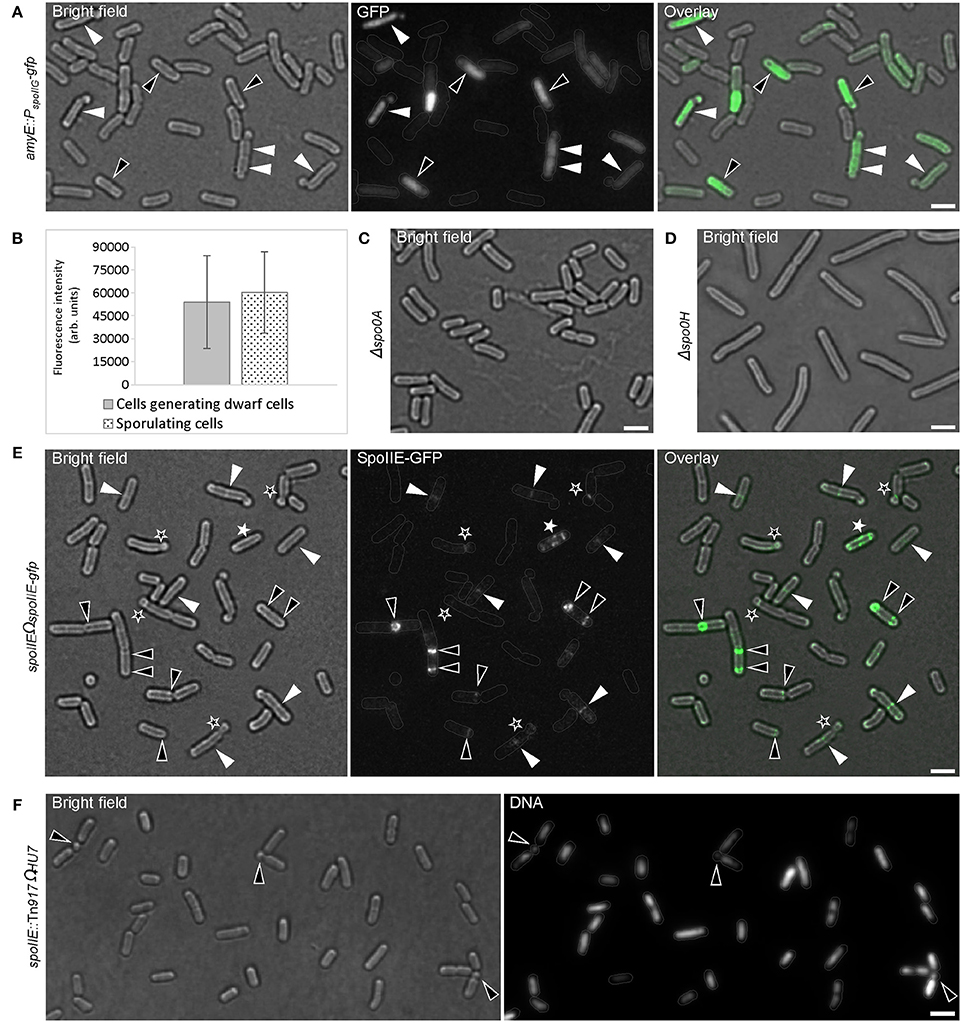
Figure 3. Early sporulation genes (onset of sporulation) are active during formation of small round cells. (A) Cells expressing a fusion of gfp to the Spo0A-controlled spoIIG gene to visualize the activity of Spo0A. Black and white triangles indicate sporulating cells and cells generating small round cells respectively. (B) Analysis of GFP intensity in cells shown in panel (A). The difference in the GFP intensity of sporulating cells compared with cells generating small round cells was evaluated by the Mann-Whitney test and a p-value of > 0.05 (0.1765) was considered statically non-significant. 178 and 193 cells were measured for cells generating small round cells and sporulating cells respectively. Error bars represent standard deviations of the means. Fluorescence intensities are in arbitrary units (arb. units). Error bars represent standard deviations of the means. spo0A (C) or spo0H (D) mutants are unable to generate small round cells. (E) Cells expressing SpoIIE fused to GFP. Black triangles indicate polar E-rings. White asterisks indicate the helical distribution of SpoIIE-GFP. White triangles indicate the presence of E-rings at mid-cell position. Black asterisks indicate E-rings at the septum of the small round cells. (F) spoIIE mutant cells also generate small round cells (black triangles), but less frequently than the wild-type strain. Gray lines show the contour of the cells. Scale bars 2 μm.
B. subtilis Mutant Defective in Proper Polar Septation Forms Fewer Small Round Cells
Asymmetric cell division in B. subtilis is the second major morphological change observed during sporulation (Schaeffer et al., 1965a). My observations indicated that the development of the spore septum must be required for the small round cells formation. To test whether the ability to form proper polar septa affects the occurrence of the small round cells, the bifunctional SpoIIE protein was examined. SpoIIE is involved in asymmetric division and is required for the establishment of compartment-specific activation of transcription factors (Barák et al., 1996). SpoIIE is proposed to play a role in the switch from one Z-ring observed during vegetative growth to two polar Z-rings when cells enter sporulation (Khvorova et al., 1998). Consequently, SpoIIE also assembles into rings (E-ring) that are dependent on Z-ring formation (Arigoni et al., 1995; Levin et al., 1997; King et al., 1999). Figure 3E shows rings and helical patterns of SpoIIE-GFP localization. 92% of small round cells (146 cells analyzed) had E-rings at their septum. The remaining small round cells showed no or very faint SpoIIE-GFP signals at their septum (Figure 3E). Interestingly, an E-ring also frequently formed at the mid cell position indicating that the interaction of SpoIIE with the vegetative Z-ring precedes the polar relocalization of the Z/E-rings via the helical pattern distribution of both proteins (Ben-Yehuda and Losick, 2002) (Figure 3E).
The precise role of SpoIIE in this dynamic relocalization of FtsZ is still unclear. However, in the absence of SpoIIE, sporulating cells are still able to assemble polar Z-rings and to form asymmetrically positioned septa (Levin et al., 1997; Wu et al., 1998). These septa are rather thick and similar to those formed during vegetative growth. The literature suggests that SpoIIE may regulate cell wall synthesis at the asymmetric division sites (Piggot and Coote, 1976; Illing and Errington, 1991; Barák and Youngman, 1996) and may play a role in peptidoglycan remodeling during engulfment (Campo et al., 2008). Indeed, a recent study indicated that SpoIIE recruits the morphogenic protein RodZ at the asymmetric septum during sporulation where both proteins might be involved in peptidoglycan thinning during engulfment (Muchova et al., 2016). The second distinct function of SpoIIE during sporulation is the activation of σF in the forespore due to its phosphatase activity (Duncan et al., 1995; Arigoni et al., 1996; Feucht et al., 1996). Therefore, a spoIIE mutant arrests spore development at stage II (Margolis et al., 1991). The spoIIE null mutant analyzed in this work also generated the small round cells (Figure 3F), but only up to 3.0 ± 0.6% (mean ± SD of three independent experiments; 247 cells counted each); a frequency that is 5-fold less than that observed in wild-type strain. This finding is consistent with the previous report that spoIIE null mutants have a noticeably reduced frequency of polar septum formation (Barák and Youngman, 1996) and shows a correlation between the ability to form polar septa and the generation of small round cells. Taken together, these findings support the idea that the small round cells formation requires the asymmetric septa involved in sporulation.
σE or σF Are Not Active during Formation of Small Round Cells
Because E-ring signals could be also visualized at the small round cells septa (Figure 3E), I investigated whether σE or σF were indeed expressed during the formation of small round cells in the former mother cell or forespore respectively. The activity of σE or σF was visualized using strains bearing gfp fusions to spoIID or spoIIQ promoter of whose expression is controlled by σE or σF, respectively (Rong et al., 1986; Londoño-Vallejo et al., 1997). Figure 4 shows that σE and σF were active in cells undergoing sporulation, but not in those generating small round cells. Sporulating cells showed discrete curved septa indicating the engulfment of the forespore at the cell pole while a true polar cell division was often marked by a clear invagination of the cell wall (Figures 4A,B). In some cases, cells turned on σE or σF following the formation of the small round cell (Figures 4A,B, black asterisks). This observation is consistent with the above finding that cells generating the small round cells retain sporulation signals and can attempt another round of sporulation initiation. Unexpectedly, the forespores were always formed at poles where small round cells had been generated, suggesting the presence of specific markers at the cell poles. These markers would eventually drive a subsequent sporulation event at the same pole. Thus, the formation of the small round cells does not require the activities of σE or σF but σH controlling the early stationary phase genes involved in the initiation of sporulation.
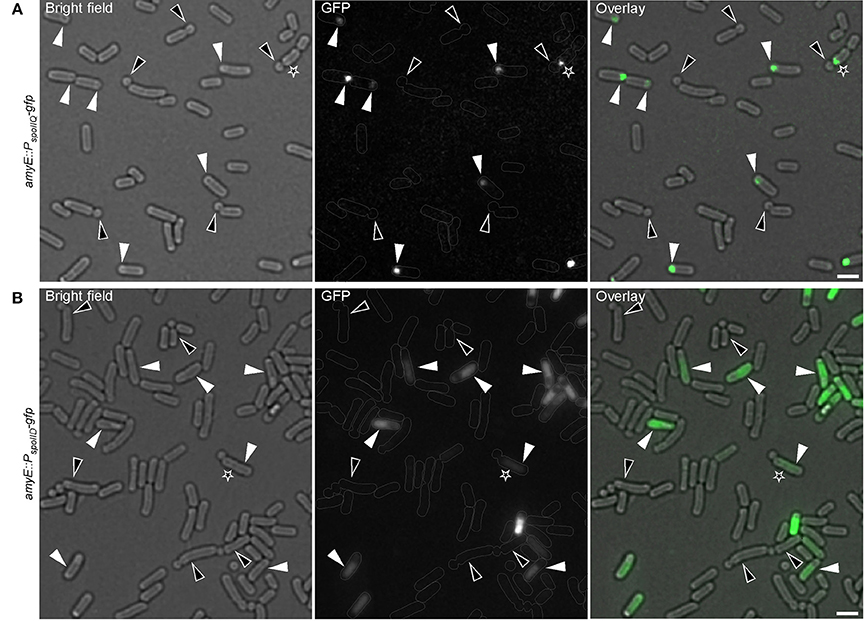
Figure 4. Compartmentalized activation of sporulation-specific sigma factors is not established during formation of small round cells. Cells expressing a fusion of gfp to the σF-controlled spoIIQ (A) or to σE-controlled spoIID (B) genes were applied to visualize the activity of σF or σE, respectively. White triangles indicate sporulating cells with active σF or σE. Black triangles indicate cells dividing asymmetrically with no apparent activity of any sigma factor. Black asterisks indicate cells that had abortive sporulation followed by a successful attempt as judged by the attached small round cells. Gray lines show the contour of the cells. Scale bars 2 μm.
Chromosomal DNA Is Actively Translocated into the Small Round Cells
At the onset of sporulation, as cells possess two chromosomes, the origins of replication are targeted to the opposite cell poles by RacA in a DivIVA-dependent manner (Ben-Yehuda et al., 2003). This mechanism ensures that the future sporangium gets a copy of the chromosome at both poles. When the asymmetric division is taking place, SpoIIIE, a DNA translocase assembles at the closing septum and actively pumps the remaining chromosome from the mother cell into the forespore (Wu et al., 1995; Wu and Errington, 1997; Bath et al., 2000).
This raises the question whether DNA is actively transported into the small round cells or simply pinched-off from the bulk nucleoid by the closing septum. To explore this question, I examined a strain expressing SpoIIIE fused to YFP as a sole source of protein during the formation of small round cells. SpoIIIE-YFP accumulated at the asymmetric septum, as previously reported (Wu et al., 1995; Wu and Errington, 1997; Bath et al., 2000) (Figure 5A). Interestingly, a SpoIIIE-YFP foci was also present at the asymmetric septum of most of the small round cells containing DNA (90% of 213 cells counted), but absent at the septum of all empty small round cells (empty small round cells represent about 1.67% of the small round cells) (Figure 5A). Thus, similar to the sporulation process, SpoIIIE is also required for DNA translocation during the formation of small round cells. Surprisingly, up to 60% (180 cells counted) of small round cells produced by a spoIIIE null strain contained DNA (Figure 5B). This observation suggests that another cellular factor is implicated in this process. Such factor could be the B. subtilis DNA translocase SftA (septum-associated FtsK-like translocase of DNA). SftA belongs to the SpoIIIE/FtsK-like protein family found in B. subtilis, but lacks the transmembrane domain (Biller and Burkholder, 2009; Kaimer et al., 2009). It has been proposed that SftA acts first at the cell division site by clearing the sister chromosomes and that SpoIIIE only comes into play when chromosomes get trapped at the closing septum (Biller and Burkholder, 2009; Kaimer et al., 2009; Wu, 2009). However, recent investigations using super-resolution microscopy revealed that SpoIIIE is consistently present at the invaginating septum (Fiche et al., 2013), suggesting that both proteins are synchronously recruited to the division machinery. A spoIIIE/sftA double null mutant neither produced small round cells nor sporulated (Figure 5C), a finding consistent with the correlation between spore and the small round cells formation. Thus, similar to their respective function during vegetative growth, SpoIIIE, and SftA act synergistically to ensure proper chromosome partitioning/translocation during sporulation or subsequent small round cells formation when the sporulation process fails.
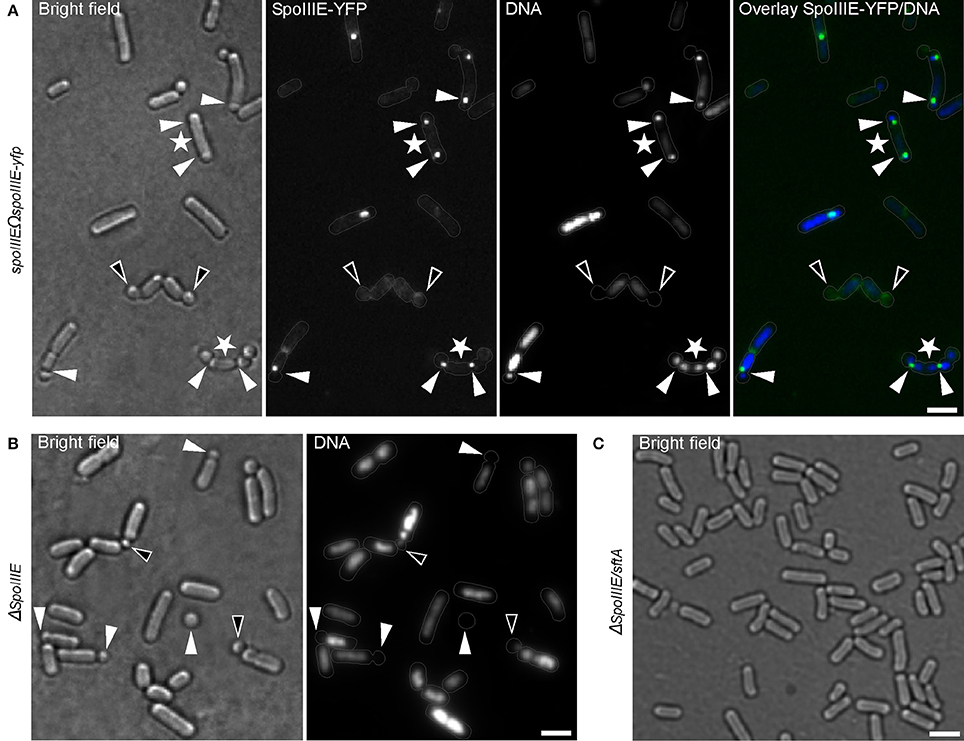
Figure 5. Chromosomal DNA is actively translocated into the small round cells. (A) B. subtilis cells expressing DNA translocase SpoIIIE fused to YFP. White triangles indicate SpoIIIE-YFP focus at the asymmetric septum. White asterisks indicate few cases where bipolar asymmetric septa persist and generate two small round cells from a single cell. Black triangles indicate small round cells without DNA lacking SpoIIIE-YFP foci at their septa. (B) spoIIIE mutant cells showing an increased number of small round cells without DNA (white triangles). Black triangles indicate small round cells apparently containing only residual amounts of DNA. (C) Cells bearing null mutations in both spoIIIE and sftA are unable to generate small round cells. Gray lines show the contour of the cells. Scale bars 2 μm.
Small Round Cells Contain a Full Chromosome
It was interesting to find out if the translocation of DNA into the emerging small round cells might be affected while abortion of the sporulation occurs. In other words, do the small round cells contain full chromosomes? Assuming that 2/3 of the forespore chromosome, which remains in the mother cell (Wu and Errington, 1994), is translocated in an ordered manner (i.e., the terminus of the chromosome being translocated last), this question was examined by visualizing the presence of the terminus (terC) region of the chromosome in the small round cells. Figure 6A shows that about 50% (103 cells counted) of the small round cells contained the terC region while 98.33% of the small round cells contained DNA (Figure 2A). The discrepancy between the number of cells containing terC and the total number of cells containing DNA could be due to incomplete translocation of the chromosome into the forespore. In agreement with this, about 99% (68 cells counted) of the small round cells were found to contain oriC (Figure 6B). Therefore, the ~2% of anucleate small round cells may suggest a release of the oriC region from the forespore pole and subsequent retraction of the chromosome into the mother cell compartment. This could happen when opposing signals to commitment are sensed earlier in the developmental process. However, although there is no evidence that B. subtilis can reverse commitment to sporulation by retracting the chromosome, it should be noted that the chromosome will be retracted if the origin is not in the forespore (Becker and Pogliano, 2007). In any case, the high probability that the small round cells inherit a full copy of the chromosomal DNA increases the chances of viability for these cells.
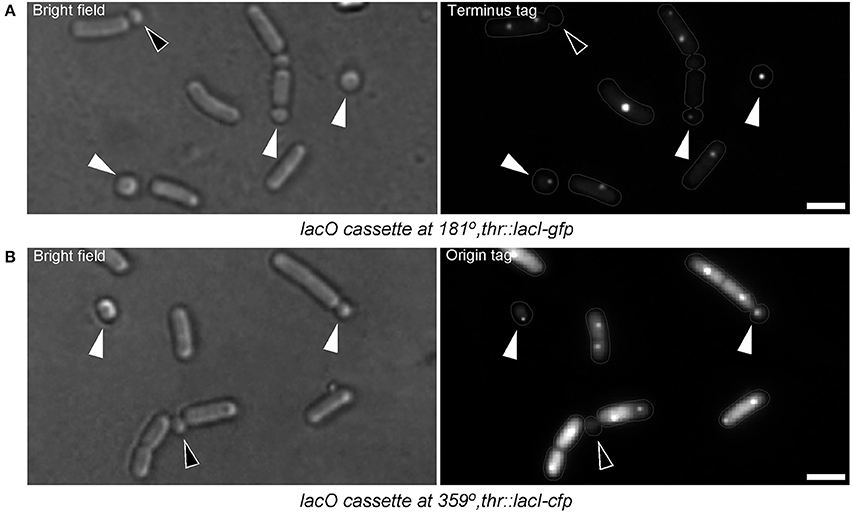
Figure 6. Small round cells contain full chromosomes. (A) B. subtilis cells harboring a lacO cassette at 181° (terminus region of the chromosome). White triangles indicate small round cells with terminus region signal, which is a proof that the cells possess the full chromosome. The black triangle indicates a small round cell which has not completed DNA translocation (both terminus regions are still in the larger cell compartment). (B) B. subtilis cells harboring a lacO cassette at 359° (origin region of the chromosome). Nearly all small round cells contain the origin region (white triangles). Black triangle indicates a small round cell lacking origin signal. Terminus region tagged cells were grown in S750. For unknown reasons, origin region tagged cells hardly sporulated or generated small round cells in S750. However, when grown in S750 diluted 1:1 with LB, sporulation and small round cells formation could be observed (but less frequently compared with the wild-type cells grown in S750). Somehow, in this medium, cells exhibited high level of diffused fluorescence probably due to the higher expression of GFP-LacI. Gray lines show the contour of the cells. Scale bars 2 μm.
Small Round Cells Resume Growth upon Addition of Fresh Growth Medium
Sporulating cells lacking σF are unable to complete spore development and end up with an empty mid-cell compartment flanked by two opposite forspores containing each a full copy of the chromosome. These forespores are able to resume vegetative growth when shifted into rich medium (Dworkin and Losick, 2005). A key question of this work was whether the small round cells were dead-end structures or whether they could resume normal growth upon addition of fresh medium. To test this, I monitored the growth of small round cells by time-lapse microscopy. Cultures of B. subtilis generating small round cells were diluted into fresh growth media and grown for 60 min at room temperature before time-lapse imaging. Strikingly, the small round cells were able to return to the rod shaped form (Figures 7A–C, Figure S2, and Movie S1). Different sizes of small round cells were monitored for growth. Out of 49 cells analyzed, 47 cells extended into rods. The small round cells observed did not extend at the same speed. The speed of growth was dependent on the size of the small round cells at the beginning of the experiment. The larger the diameter size of small round cells, the faster they reached their first division. Among the 47 cells that extended into rods, 16 cells that had diameter sizes ranging from 0.95 to 1.15 μm cells divided after 2.5–3 h and 21 cells that had diameter sizes ranging from 0.8 to 0.9 divided after 3.5–4 h. The rest of the cells (10 cells) with smaller diameter sizes (~0.7 μm) did not did divide during the time course of the time-lapse experiment, which was ~4 h.
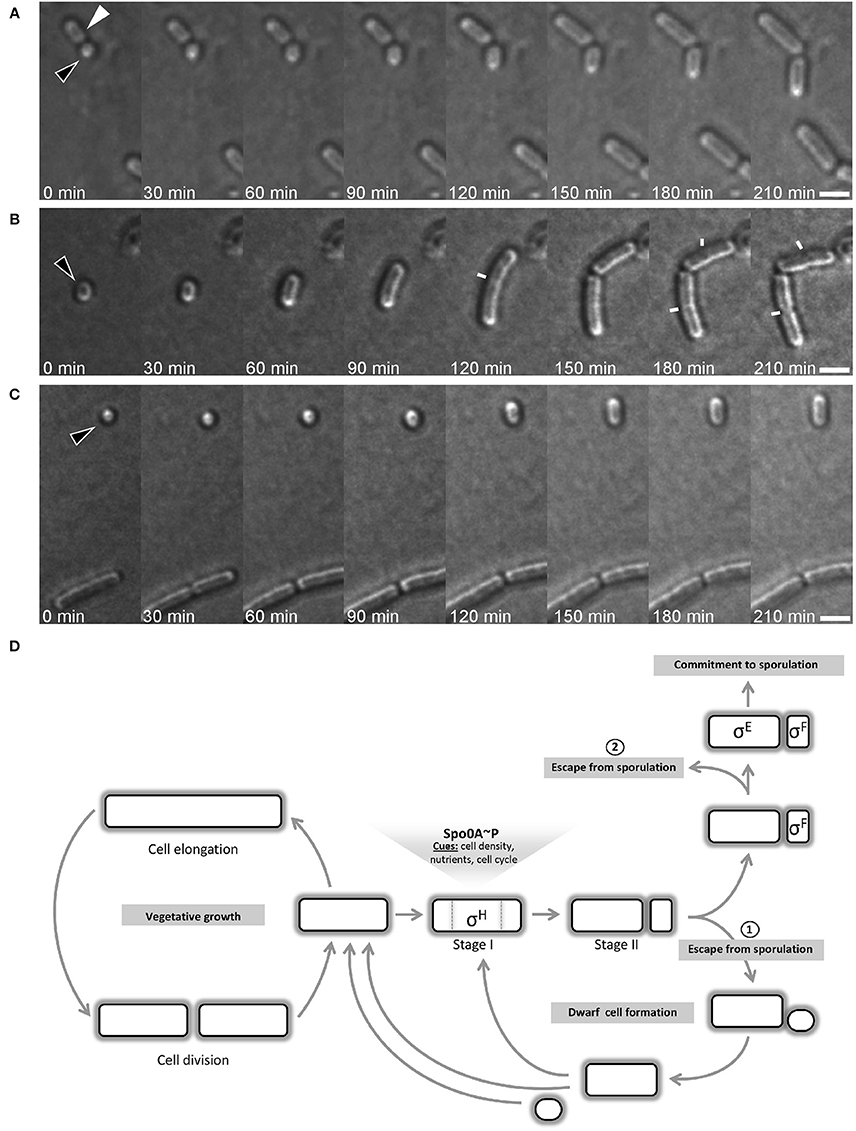
Figure 7. Time-lapse microscopy of growing small round cells. Black triangles indicate small round cells that grow back to rod shape and white triangle indicate a former mother cell resuming growth. Images were acquired every 30 min. Small round cells are of different size at the start of the time-lapse: “medium” (A), “large” (B), and “tiny” (C). In (B), white lines indicate the septum of dividing cells. Scale bars 2 μm. (D) B. subtilis cell cycle depicting the bifurcation in the spore development process. After the initiation phase of sporulation and the establishment of the asymmetric septum, the cell can escape from sporulation by generating a small round cell, which I name “dwarf” cell as it is generated by wild-type cells. However, the sporulation signals can remain stable in the former mother cell and a second round of the process is often initiated. Dwarf cells and former mother cells are able to return to rod shape and resume vegetative growth. The commitment to sporulate requires that both sigma factors are activated. The activation of sigma factors is not simultaneous and the activation of σF occurs first. Before σE is activated, cell can still abort the sporulation process, as reported by Narula et al. (2012).
Taken together, these experiments suggest that the here-described small round cells could provide an escape route for cells that are unable to continue the sporulation process.
Discussion
Although spore development is generally assumed to be a well-studied process, sporulation has not yet been stringently assessed in conditions that are not optimized to induce sporulation. In this work, I have monitored the sporulation behavior of wild-type B. subtilis in standard minimal S750 medium during extended stationary-phase growth. For comparative analysis, I have used the sporulation DSM and the nutritionally rich LB media. In DSM, as expected, the major response of B. subtilis cells to stationary-phase growth was the formation of spores due to nutrient limitation (Schaeffer et al., 1965b).
In LB, B. subtilis cells yielded poor sporulation efficiency. What then can cause inhibition of sporulation in LB? It was shown that in contrary to the conventional thinking, LB medium is a rather carbon-source limited (Sezonov et al., 2007). Tryptone and yeast extract which are the main components of LB are mostly composed of oligopeptides (Bertani, 1951). The catabolizable amino acids recovered from these oligopeptides represent the main carbon source. Their degradation during growth results in excretion of the excess ammonium, which alkalinizes the growth medium. Thus, the inhibition of sporulation observed in LB is neither due to catabolite repression nor the acidification of the culture medium and therefore, remains enigmatic.
The major finding comes from the growth in minimal medium. One could expect that the sporulation would readily occur, but this was not the case. I show that instead of spores, cells frequently generate small round shaped offsprings as the culture reaches the stationary phase. The small round cells are morphologically similar to spherical cell morphology generated by Escherichia coli during stationary-phase growth. However, in contrast to small round cells that are generated by asymmetric septation, small spherical E. coli cells arise from mid cell division of cells that have decreased their size and changed their shape in response to starvation or stationary-phase conditions (Lange and Hengge-Aronis, 1991).
The small round cells are also morphologically similar to minicells. Minicells are mostly described as originating from cells lacking the MinCD components of the cell division machinery or mutants unable to properly segregate their chromosome (Adler et al., 1967; Reeve et al., 1973; de Boer et al., 1989; Levin et al., 1992; Varley and Stewart, 1992; Wu and Errington, 2004; Bernhardt and de Boer, 2005). An mreBH (mreB paralog in B. subtilis) mutant has been described to generate minicells containing DNA (Soufo and Graumann, 2010) similar to spoVG mutant cells during sporulation (Matsuno and Sonenshein, 1999). Recently, B. subtilis cells expressing the cytosolic part of RodZ was shown to generate comparable type of minicells (Muchova et al., 2013). However, the small round cells observed here originate from wild-type cells and no mutations were employed. This implies that the small round cells examined in this work are unprecedented. Therefore, to distinguish them from the well-known minicells, I propose to name them “dwarf cells.” The observation of dwarf cells in several different, spore-forming bacteria suggests that their appearance is not limited to B. subtilis, but might be general to spore formers.
This work clearly shows that cells generating dwarf cells are physiologically similar to cells initiating sporulation. Indeed, both types of cell expressed the same level of Spo0A. I could show that cells generating dwarf cells have well initiated sporulation, but for yet unknown reasons, the process aborts and dwarf cells are produced. Therefore, it seems that dwarf cells evolve from former forespores. The spore developmental process seems to be perturbed after the asymmetric division has taken place. Interestingly, cells can undergo one or two re-initiations as judged by multiple dwarf cell formations showing that the initial decision to sporulate persists despite failure.
The presence of SpoIIE—which is thought to regulate the thickness of the septum during sporulation (Piggot and Coote, 1976; Illing and Errington, 1991; Barák and Youngman, 1996)—at the septum of the dwarf cells raises the question whether their septum could be thin like the sporulation septum or thick like the septum of vegetative cells. Because dwarf cells separate from the former mother cells, it seems likely that their septum is similar to that of the vegetative cells. In this case, SpoIIE would not be needed for the synthesis of the septum of the dwarf cells. Indeed, the data show that some dwarf cells do not harbor SpoIIE-GFP signal at their septum. This observation suggests that SpoIIE might be removed from the septum of the dwarf cells either by the degradation of the protein or by the disassembly of the E-ring and its reassembly at the medial position in the former mother cell in case of re-initiation of sporulation. In any case, it will be interesting to characterize the exact nature of the septum built during the formation of dwarf cells.
Narula and collaborators previously reported abortion events during sporulation. They noticed that, until the σE is activated in the mother cell, sporulating cells can fail to engulf the forespore and either become dormant or resume vegetative growth (Narula et al., 2012). However, neither dwarf cell formation nor multiple initiations of sporulation events were reported. My experiments suggest that cells can escape from sporogenesis very early in the process. Frequently, cells are unable to activate σE and σF after polar division and rather generate dwarf cells, which can re-enter normal growth as rod shaped cells (Figure 7D). The inability of cells lacking Spo0A (the master regulator of sporulation initiation) or σH to generate dwarf cells, the detection of Spo0A activity in all cells generating dwarf cells and the ability of dwarf cells to grow back to rod shape cells strongly suggest that dwarf cell formation is a new escape route from sporulation. Thus, when cells cannot further proceed with the sporulation process after the polar septation, a “true” asymmetric cell division appears to be a proper rescue mechanism for the pre-divisional sporangium.
This cellular event, which is described here for the first time, adds a further layer of complexity to the complex bacterial developmental pathways. Further investigations are needed to characterize environmental and physiological cues that cause the early abortion of sporulation (i.e., before the expression of sigma factors) described in this work. On the other hand, dwarf cells could be an interesting tool for the investigation of cellular components involved in the determination of rod shape in bacteria.
Author Contributions
HJDS conceived, designed and performed the experiments, analyzed the data, and wrote the paper.
Conflict of Interest Statement
The author declares that the research was conducted in the absence of any commercial or financial relationships that could be construed as a potential conflict of interest.
Acknowledgments
I thank Peter Graumann for helpful discussions and Richard Losick for valuable suggestions. I am grateful to the following people for providing me with Bacillus strains: Masaya Fujita [MF248, SB201 (MF1029), MF248, and PE128 (MF1027)], Imrich Barák [PY180 (IB206)] and Daniel Jonas (B. atrophaeus and B. pumilus). I further thank Sonja Albers for giving me access to her lab imaging facility. I am grateful to Peter Graumann, Elisabeth Grohmann, Christian Reimold, Thomas Rösch, and Jihad El Andari for critical reading of the manuscript. I am also very grateful to Gert Bange, whose comments and suggestions have helped to improve the last version of the manuscript. I am very indebted to Prof. Winfried V. Kern for providing me hospitality at the University Medical Center of Freiburg. The preliminary work of this manuscript was supported by the FOR 929 grant of the Deutsche Forschungsgemeinschaft (DFG).
Supplementary Material
The Supplementary Material for this article can be found online at: http://journal.frontiersin.org/article/10.3389/fmicb.2016.01810/full#supplementary-material
References
Adler, H. I., Fisher, W. D., Cohen, A., and Hardigree, A. A. (1967). Miniature Escherichia coli cells deficient in DNA. Proc. Natl. Acad. Sci. U.S.A. 57, 321–326. doi: 10.1073/pnas.57.2.321
Arigoni, F., Duncan, L., Alper, S., Losick, R., and Stragier, P. (1996). SpoIIE governs the phosphorylation state of a protein regulating transcription factor sigma F during sporulation in Bacillus subtilis. Proc. Natl. Acad. Sci. U.S.A. 93, 3238–3242. doi: 10.1073/pnas.93.8.3238
Arigoni, F., Pogliano, K., Webb, C. D., Stragier, P., and Losick, R. (1995). Localization of protein implicated in establishment of cell type to sites of asymmetric division. Science 270, 637–640.
Barák, I., Behari, J., Olmedo, G., Guzmán, P., Brown, D. P., Castro, E., et al. (1996). Structure and function of the Bacillus SpoIIE protein and its localization to sites of sporulation septum assembly. Mol. Microbiol. 19, 1047–1060. doi: 10.1046/j.1365-2958.1996.433963.x
Barák, I., and Youngman, P. (1996). SpoIIE mutants of Bacillus subtilis comprise two distinct phenotypic classes consistent with a dual functional role for the SpoIIE protein. J. Bacteriol. 178, 4984–4989.
Bath, J., Wu, L. J., Errington, J., and Wang, J. C. (2000). Role of Bacillus subtilis SpoIIIE in DNA transport across the mother cell-prespore division septum. Science 290, 995–997. doi: 10.1126/science.290.5493.995
Becker, E. C., and Pogliano, K. (2007). Cell-specific SpoIIIE assembly and DNA translocation polarity are dictated by chromosome orientation. Mol. Microbiol. 66, 1066–1079. doi: 10.1111/j.1365-2958.2007.05992.x
Ben-Yehuda, S., and Losick, R. (2002). Asymmetric cell division in B. subtilis involves a spiral-like intermediate of the cytokinetic protein FtsZ. Cell 109, 257–266. doi: 10.1016/S0092-8674(02)00698-0
Ben-Yehuda, S., Rudner, D. Z., and Losick, R. (2003). RacA, a bacterial protein that anchors chromosomes to the cell poles. Science 299, 532–536. doi: 10.1126/science.1079914
Bernhardt, T. G., and de Boer, P. A. (2005). SlmA, a nucleoid-associated, FtsZ binding protein required for blocking septal ring assembly over chromosomes in E. coli. Mol. Cell 18, 555–564. doi: 10.1016/j.molcel.2005.04.012
Bertani, G. (1951). Studies on lysogenesis. I. The mode of phage liberation by lysogenic Escherichia coli. J. Bacteriol. 62, 293–300.
Bertani, G. (2004). Lysogeny at mid-twentieth century: P1, P2, and other experimental systems. J. Bacteriol. 186, 595–600. doi: 10.1128/JB.186.3.595-600.2004
Biller, S. J., and Burkholder, W. F. (2009). The Bacillus subtilis SftA (YtpS) and SpoIIIE DNA translocases play distinct roles in growing cells to ensure faithful chromosome partitioning. Mol. Microbiol. 74, 790–809. doi: 10.1111/j.1365-2958.2009.06893.x
Burbulys, D., Trach, K. A., and Hoch, J. A. (1991). Initiation of sporulation in Bacillus subtilis is controlled by a multicomponent phosphorelay. Cell 64, 545–552. doi: 10.1016/0092-8674(91)90238-T
Campo, N., Marquis, K. A., and Rudner, D. Z. (2008). SpoIIQ anchors membrane proteins on both sides of the sporulation septum in Bacillus subtilis. J. Biol. Chem. 283, 4975–4982. doi: 10.1074/jbc.M708024200
Chubukov, V., and Sauer, U. (2014). Environmental dependence of stationary-phase metabolism in Bacillus subtilis and Escherichia coli. Appl. Environ. Microbiol. 80, 2901–2909. doi: 10.1128/AEM.00061-14
de Boer, P. A., Crossley, R. E., and Rothfield, L. I. (1989). A division inhibitor and a topological specificity factor coded for by the minicell locus determine proper placement of the division septum in E. coli. Cell 56, 641–649. doi: 10.1016/0092-8674(89)90586-2
Soufo, H. J. D., and Graumann, P. L. (2010). Bacillus subtilis MreB paralogues have different filament architectures and lead to shape remodelling of a heterologous cell system. Mol. Microbiol. 78, 1145–1158. doi: 10.1111/j.1365-2958.2010.07395.x
Dubnau, D., and Losick, R. (2006). Bistability in bacteria. Mol. Microbiol. 61, 564–572. doi: 10.1111/j.1365-2958.2006.05249.x
Duncan, L., Alper, S., Arigoni, F., Losick, R., and Stragier, P. (1995). Activation of cell-specific transcription by a serine phosphatase at the site of asymmetric division. Science 270, 641–644.
Dworkin, J., and Losick, R. (2005). Developmental commitment in a bacterium. Cell 121, 401–409. doi: 10.1016/j.cell.2005.02.032
Eichenberger, P., Fawcett, P., and Losick, R. (2001). A three-protein inhibitor of polar septation during sporulation in Bacillus subtilis. Mol. Microbiol. 42, 1147–1162. doi: 10.1046/j.1365-2958.2001.02660.x
Eijlander, R. T., de Jong, A., Krawczyk, A. O., Holsappel, S., and Kuipers, O. P. (2014). SporeWeb: an interactive journey through the complete sporulation cycle of Bacillus subtilis. Nucleic Acids Res. 42(Database issue), D685–D691. doi: 10.1093/nar/gkt1007
Errington, J. (1993). Bacillus subtilis sporulation: regulation of gene expression and control of morphogenesis. Microbiol. Rev. 57, 1–33.
Errington, J. (2003). Regulation of endospore formation in Bacillus subtilis. Nat. Rev. Microbiol. 1, 117–126. doi: 10.1038/nrmicro750
Errington, J., and Illing, N. (1992). Establishment of cell-specific transcription during sporulation in Bacillus subtilis. Mol. Microbiol. 6, 689–695. doi: 10.1111/j.1365-2958.1992.tb01517.x
Fawcett, P., Eichenberger, P., Losick, R., and Youngman, P. (2000). The transcriptional profile of early to middle sporulation in Bacillus subtilis. Proc. Natl. Acad. Sci. U.S.A. 97, 8063–8068. doi: 10.1073/pnas.140209597
Feucht, A., and Lewis, P. J. (2001). Improved plasmid vectors for the production of multiple fluorescent protein fusions in Bacillus subtilis. Gene 264, 289–297. doi: 10.1016/S0378-1119(01)00338-9
Feucht, A., Magnin, T., Yudkin, M. D., and Errington, J. (1996). Bifunctional protein required for asymmetric cell division and cell-specific transcription in Bacillus subtilis. Genes Dev. 10, 794–803. doi: 10.1101/gad.10.7.794
Fiche, J. B., Cattoni, D. I., Diekmann, N., Langerak, J. M., Clerte, C., Royer, C. A., et al. (2013). Recruitment, assembly, and molecular architecture of the SpoIIIE DNA pump revealed by superresolution microscopy. PLoS Biol. 11:e1001557. doi: 10.1371/journal.pbio.1001557
Fujita, M., González-Pastor, J. E., and Losick, R. (2005). High- and low-threshold genes in the Spo0A regulon of Bacillus subtilis. J. Bacteriol. 187, 1357–1368. doi: 10.1128/JB.187.4.1357-1368.2005
Fujita, M., and Losick, R. (2003). The master regulator for entry into sporulation in Bacillus subtilis becomes a cell-specific transcription factor after asymmetric division. Genes Dev. 17, 1166–1174. doi: 10.1101/gad.1078303
Fujita, M., and Losick, R. (2005). Evidence that entry into sporulation in Bacillus subtilis is governed by a gradual increase in the level and activity of the master regulator Spo0A. Genes Dev. 19, 2236–2244. doi: 10.1101/gad.1335705
Garti-Levi, S., Hazan, R., Kain, J., Fujita, M., and Ben-Yehuda, S. (2008). The FtsEX ABC transporter directs cellular differentiation in Bacillus subtilis. Mol. Microbiol. 69, 1018–1028. doi: 10.1111/j.1365-2958.2008.06340.x
Grossman, A. D. (1991). Integration of developmental signals and the initiation of sporulation in B. subtilis. Cell 65, 5–8. doi: 10.1016/0092-8674(91)90353-Z
Grossman, A. D., and Losick, R. (1988). Extracellular control of spore formation in Bacillus subtilis. Proc. Natl. Acad. Sci. U.S.A. 85, 4369–4373. doi: 10.1073/pnas.85.12.4369
Harwood, C. R., and Cutting, S. M. (1990). Molecular Biological Methods for Bacillus. New York, NY: John Wiley and Sons.
Hoch, J. A. (1993). Regulation of the phosphorelay and the initiation of sporulation in Bacillus subtilis. Annu. Rev. Microbiol. 47, 441–465. doi: 10.1146/annurev.mi.47.100193.002301
Illing, N., and Errington, J. (1991). Genetic regulation of morphogenesis in Bacillus subtilis: roles of sigma E and sigma F in prespore engulfment. J. Bacteriol. 173, 3159–3169.
Kaimer, C., González-Pastor, J. E., and Graumann, P. L. (2009). SpoIIIE and a novel type of DNA translocase, SftA, couple chromosome segregation with cell division in Bacillus subtilis. Mol. Microbiol. 74, 810–825. doi: 10.1111/j.1365-2958.2009.06894.x
Kearns, D. B., and Losick, R. (2005). Cell population heterogeneity during growth of Bacillus subtilis. Genes Dev. 19, 3083–3094. doi: 10.1101/gad.1373905
Khvorova, A., Zhang, L., Higgins, M. L., and Piggot, P. J. (1998). The spoIIE locus is involved in the Spo0A-dependent switch in the location of FtsZ rings in Bacillus subtilis. J. Bacteriol. 180, 1256–1260.
King, N., Dreesen, O., Stragier, P., Pogliano, K., and Losick, R. (1999). Septation, dephosphorylation, and the activation of sigmaF during sporulation in Bacillus subtilis. Genes Dev. 13, 1156–1167. doi: 10.1101/gad.13.9.1156
Lange, R., and Hengge-Aronis, R. (1991). Growth phase-regulated expression of bolA and morphology of stationary-phase Escherichia coli cells are controlled by the novel sigma factor sigma S. J. Bacteriol. 173, 4474–4481.
Levin, P. A., Losick, R., Stragier, P., and Arigoni, F. (1997). Localization of the sporulation protein SpoIIE in Bacillus subtilis is dependent upon the cell division protein FtsZ. Mol. Microbiol. 25, 839–846. doi: 10.1111/j.1365-2958.1997.mmi505.x
Levin, P. A., Margolis, P. S., Setlow, P., Losick, R., and Sun, D. (1992). Identification of Bacillus subtilis genes for septum placement and shape determination. J. Bacteriol. 174, 6717–6728.
Londoño-Vallejo, J. A., Fréhel, C., and Stragier, P. (1997). SpoIIQ, a forespore-expressed gene required for engulfment in Bacillus subtilis. Mol. Microbiol. 24, 29–39. doi: 10.1046/j.1365-2958.1997.3181680.x
Losick, R., and Stragier, P. (1992). Crisscross regulation of cell-type-specific gene expression during development in B. subtilis. Nature 355, 601–604. doi: 10.1038/355601a0
Margolis, P., Driks, A., and Losick, R. (1991). Establishment of cell type by compartmentalized activation of a transcription factor. Science 254, 562–565.
Marston, A. L., and Errington, J. (1999). Selection of the midcell division site in Bacillus subtilis through MinD-dependent polar localization and activation of MinC. Mol. Microbiol. 33, 84–96. doi: 10.1046/j.1365-2958.1999.01450.x
Mascarenhas, J., Soppa, J., Strunnikov, A. V., and Graumann, P. L. (2002). Cell cycle-dependent localization of two novel prokaryotic chromosome segregation and condensation proteins in Bacillus subtilis that interact with SMC protein. EMBO J. 21, 3108–3118. doi: 10.1093/emboj/cdf314
Matsuno, K., and Sonenshein, A. L. (1999). Role of SpoVG in asymmetric septation in Bacillus subtilis. J. Bacteriol. 181, 3392–3401.
Muchová, K., Chromiková, Z., and Barák, I. (2013). Control of Bacillus subtilis cell shape by RodZ. Environ. Microbiol. 15, 3259–3271. doi: 10.1111/1462-2920.12200
Muchova, K., Chromiková, Z., Bradshaw, N., Wilkinson, A. J., and Barák, I. (2016). Morphogenic protein RodZ interacts with sporulation specific SpoIIE in Bacillus subtilis. PLoS ONE 11:e0159076. doi: 10.1371/journal.pone.0159076
Narula, J., Devi, S. N., Fujita, M., and Igoshin, O. A. (2012). Ultrasensitivity of the Bacillus subtilis sporulation decision. Proc. Natl. Acad. Sci. U.S.A. 109, 3513–3522. doi: 10.1073/pnas.1213974109
Parker, G. F., Daniel, R. A., and Errington, J. (1996). Timing and genetic regulation of commitment to sporulation in Bacillus subtilis. Microbiology 142, 3445–3452. doi: 10.1099/13500872-142-12-3445
Piggot, P. J., and Coote, J. G. (1976). Genetic aspects of bacterial endospore formation. Bacteriol. Rev. 40, 908–962.
Pogliano, K., Hofmeister, A. E., and Losick, R. (1997). Disappearance of the sigma E transcription factor from the forespore and the SpoIIE phosphatase from the mother cell contributes to establishment of cell-specific gene expression during sporulation in Bacillus subtilis. J. Bacteriol. 179, 3331–3341.
Reeve, J. N., Mendelson, N. H., Coyne, S. I., Hallock, L. L., and Cole, R. M. (1973). Minicells of Bacillus subtilis. J. Bacteriol. 114, 860–873.
Rong, S., Rosenkrantz, M. S., and Sonenshein, A. L. (1986). Transcriptional control of the Bacillus subtilis spoIID gene. J. Bacteriol. 165, 771–779.
Sandman, K., Losick, R., and Youngman, P. (1987). Genetic analysis of Bacillus subtilis spo mutations generated by Tn917-mediated insertional mutagenesis. Genetics 117, 603–617.
Satola, S. W., Baldus, J. M., and Moran, C. P. Jr. (1992). Binding of Spo0A stimulates spoIIG promoter activity in Bacillus subtilis. J. Bacteriol. 174, 1448–1453.
Schaeffer, P., Ionesco, H., Ryter, A., and Balassa, G. (1965a). Mécanismes De Régulation Des Activités Cellulaires Chez Les Microorganismes. Marseille: Centre national de la recherche scientifique.
Schaeffer, P., Millet, J., and Aubert, J. P. (1965b). Catabolic repression of bacterial sporulation. Proc. Natl. Acad. Sci. U.S.A. 54, 704–711.
Schneider, C. A., Rasband, W. S., and Eliceiri, K. W. (2012). NIH Image to ImageJ: 25 years of image analysis. Nat. Methods 9, 671–675. doi: 10.1038/nmeth.2089
Sezonov, G., Joseleau-Petit, D., and D'Ari, R. (2007). Escherichia coli physiology in Luria-Bertani broth. J. Bacteriol. 189, 8746–8749. doi: 10.1128/JB.01368-07
Sonenshein, A. L. (2000). Control of sporulation initiation in Bacillus subtilis. Curr. Opin. Microbiol. 3, 561–566. doi: 10.1016/S1369-5274(00)00141-7
Sterlini, J. M., and Mandelstam, J. (1969). Commitment to sporulation in Bacillus subtilis and its relationship to development of actinomycin resistance. Biochem. J. 113, 29–37. doi: 10.1042/bj1130029
Stragier, P., and Losick, R. (1996). Molecular genetics of sporulation in Bacillus subtilis. Annu. Rev. Genet. 30, 297–341. doi: 10.1146/annurev.genet.30.1.297
Teleman, A. A., Graumann, P. L., Lin, D. C., Grossman, A. D., and Losick, R. (1998). Chromosome arrangement within a bacterium. Curr. Biol. 8, 1102–1109. doi: 10.1016/S0960-9822(98)70464-6
Trach, K., Burbulys, D., Strauch, M., Wu, J. J., Dhillon, N., Jonas, R., et al. (1991). Control of the initiation of sporulation in Bacillus subtilis by a phosphorelay. Res. Microbiol. 142, 815–823. doi: 10.1016/0923-2508(91)90060-N
Varley, A. W., and Stewart, G. C. (1992). The divIVB region of the Bacillus subtilis chromosome encodes homologs of Escherichia coli septum placement (minCD) and cell shape (mreBCD) determinants. J. Bacteriol. 174, 6729–6742.
Veening, J. W., Hamoen, L. W., and Kuipers, O. P. (2005). Phosphatases modulate the bistable sporulation gene expression pattern in Bacillus subtilis. Mol. Microbiol. 56, 1481–1494. doi: 10.1111/j.1365-2958.2005.04659.x
Webb, C. D., Teleman, A., Gordon, S., Straight, A., Belmont, A., Lin, D. C., et al. (1997). Bipolar localization of the replication origin regions of chromosomes in vegetative and sporulating cells of B. subtilis. Cell 88, 667–674. doi: 10.1016/S0092-8674(00)81909-1
Wu, L. J. (2009). It takes two DNA translocases to untangle chromosomes from the division septum. Mol. Microbiol. 74, 773–776. doi: 10.1111/j.1365-2958.2009.06892.x
Wu, L. J., and Errington, J. (1994). Bacillus subtilis SpoIIIE protein required for DNA segregation during asymmetric cell division. Science 264, 572–575.
Wu, L. J., and Errington, J. (1997). Septal localization of the SpoIIIE chromosome partitioning protein in Bacillus subtilis. EMBO J. 16, 2161–2169. doi: 10.1093/emboj/16.8.2161
Wu, L. J., and Errington, J. (2004). Coordination of cell division and chromosome segregation by a nucleoid occlusion protein in Bacillus subtilis. Cell 117, 915–925. doi: 10.1016/j.cell.2004.06.002
Wu, L. J., Feucht, A., and Errington, J. (1998). Prespore-specific gene expression in Bacillus subtilis is driven by sequestration of SpoIIE phosphatase to the prespore side of the asymmetric septum. Genes Dev. 12, 1371–1380. doi: 10.1101/gad.12.9.1371
Keywords: sporulation, minimal growth medium, cell division, minicell, dwarf cell
Citation: Defeu Soufo HJ (2016) A Novel Cell Type Enables B. subtilis to Escape from Unsuccessful Sporulation in Minimal Medium. Front. Microbiol. 7:1810. doi: 10.3389/fmicb.2016.01810
Received: 29 August 2016; Accepted: 27 October 2016;
Published: 11 November 2016.
Edited by:
Ivan Mijakovic, Chalmers University of Technology, SwedenReviewed by:
Emma Denham, University of Warwick, UKDennis Claessen, Leiden University, Netherlands
Imrich Barak, Slovak Academy of Sciences, Slovakia
Copyright © 2016 Defeu Soufo. This is an open-access article distributed under the terms of the Creative Commons Attribution License (CC BY). The use, distribution or reproduction in other forums is permitted, provided the original author(s) or licensor are credited and that the original publication in this journal is cited, in accordance with accepted academic practice. No use, distribution or reproduction is permitted which does not comply with these terms.
*Correspondence: Hervé Joël Defeu Soufo, hjdefeusoufo@gmail.com