- 1Research Division for Biotechnology, Korea Atomic Energy Research Institute, Jeongeup, South Korea
- 2Radiation Microbiology Laboratory, Department of Life Sciences, Faculty of Life Sciences, Toyo University, Gunma, Japan
Escherichia coli is a representative microorganism that is frequently used for industrial biotechnology; thus its cellular robustness should be enhanced for the widespread application of E. coli in biotechnology. Stress response genes from the extremely radioresistant bacterium Deinococcus radiodurans have been used to enhance the stress tolerance of E. coli. In the present study, we introduced the cold shock domain-containing protein PprM from D. radiodurans into E. coli and observed that the tolerance to hydrogen peroxide (H2O2) was significantly increased in recombinant strains (Ec-PprM). The overexpression of PprM in E. coli elevated the expression of some OxyR-dependent genes, which play important roles in oxidative stress tolerance. Particularly, mntH (manganese transporter) was activated by 9-fold in Ec-PprM, even in the absence of H2O2 stress, which induced a more than 2-fold increase in the Mn/Fe ratio compared with wild type. The reduced production of highly reactive hydroxyl radicals (·OH) and low protein carbonylation levels (a marker of oxidative damage) in Ec-PprM indicate that the increase in the Mn/Fe ratio contributes to the protection of cells from H2O2 stress. PprM also conferred H2O2 tolerance to E. coli in the absence of OxyR. We confirmed that the H2O2 tolerance of oxyR mutants reflected the activation of the ycgZ-ymgABC operon, whose expression is activated by H2O2 in an OxyR-independent manner. Thus, the results of the present study showed that PprM could be exploited to improve the robustness of E. coli.
Introduction
Escherichia coli (E. coli) is one of the most frequently used bacterial hosts for the industrial production of recombinant proteins (Gopal and Kumar, 2013), biofuels (Clomburg and Gonzalez, 2010), and pharmaceuticals (Ferrer-Miralles et al., 2009). Rapid growth rates, high cell-density fermentation, and the availability of various genetic tools are crucial factors that facilitate the industrial use of E. coli (Makino et al., 2011). However, the production and accumulation of recombinant proteins, fuels, and chemicals can induce a variety of stresses in E. coli, such as an unsuitable pH, temperature, osmotic pressure, and oxidative stress, all of which reduce the production from this bacteria (Han et al., 2001; Hoffmann and Rinas, 2004; Rutherford et al., 2010). Oxidative stress induced through reactive oxygen species (ROS), such as superoxide radical ( ·), hydroxyl radical (·OH), and hydrogen peroxide (H2O2), is common because ROS inevitably results from aerobic growth in a fermenter (Li et al., 2009). ROS has harmful effects on cells, including DNA mutations, metabolic pathway disruption, and growth inhibition (Imlay, 2013). Therefore, oxidative stress tolerance is a key characteristic for industrial host strains, and various methods have been explored to enhance the tolerance to oxidative stress (Basak and Jiang, 2012; Lee et al., 2014; Zhao et al., 2014).
Deinococcus radiodurans (D. radiodurans) is well known for its ability to survive multiple extreme stresses, including gamma radiation (γ-radiation), UV light, ROS, and other DNA-damaging agents (Slade and Radman, 2011). Various enzymatic and non-enzymatic anti-oxidative systems, redundancy in DNA repair genes with a unique DNA repair machinery called extended synthesis dependent strand annealing (ESDSA), and some physical characteristics of nucleoids, which are advantageous to DNA repair, contribute to the extreme resistance of D. radiodurans (Ishino and Narumi, 2015; Munteanu et al., 2015). However, the precise mechanisms governing the multiple resistance characteristics of this organism remain unclear. Stress responsive genes from D. radiodurans have been used to enhance the stress tolerance of E. coli. Indeed, the heterologous expression of a pyrrolquinoline-quinone (PQQ) synthase, a manganese (Mn) transporter protein (MntH), a small heat shock protein (Hsp20), and a response regulator (DR1558) in E. coli improved oxidative stress tolerance (Khairnar et al., 2003; Haiyan and Baoming, 2010; Singh et al., 2014; Appukuttan et al., 2016).
Deinococcus-specific ppr (pleiotropic protein promoting DNA repair) genes are essential for the extreme resistance of this organism (Hua et al., 2003; Narumi et al., 2004). A global regulator, PprI (also named IrrE), serves as a general switch for downstream DNA repair and protection pathways (Lu et al., 2009). The introduction of native and engineered PprI has been effective in not only enhancing the tolerance of E. coli against abiotic stresses, including oxidative stress (Gao et al., 2003), but also in improving ethanol production in ethanologenic E. coli (Pan et al., 2009; Ma et al., 2011). PprA, which plays a role in DNA damage resistance and the genome maintenance of D. radiodurans (Devigne et al., 2013; Selvam et al., 2013; Kota et al., 2014), also enhanced tolerance against oxidative stress when expressed in E. coli (Kota and Misra, 2006). PprM, a cold shock protein (CSP) homolog in D. radiodurans, functions as a novel modulator of PprA in PprI-dependent signal transduction pathways, and the deletion of pprM reduces resistances to γ-radiation (Ohba et al., 2009) and H2O2 stress (Jeong et al., 2016b). Taken together, these observations prompted us to investigate the effect of PprM on oxidative stress tolerance in E. coli.
In the present study, we observed that E. coli cells expressing PprM exhibited improved tolerance to hydrogen peroxide (H2O2) through an increased intracellular Mn/Fe ratio and ycgZ-ymgABC operon expression.
Materials and Methods
Construction of Plasmids and Strains
The pprM gene (dr0907) was PCR amplified from D. radiodurans R1 (ATCC13939) genomic DNA using pprM-F and pprM-R primers (Table S1). The amplified product was digested with BsaI and ligated into the BsaI site of the pASK-IBA3plus plasmid (IBA, Germany), which carries the inducible tetracycline promoter/operator for the regulated expression of proteins. The resulting plasmid, named pPprM, was transformed into E. coli EPI300 cells (F− λ− mcrA Δ(mrr-hsdRMS-mcrBC) φ80dlacZΔM15 ΔlacX74 recA1 endA1 araD139 Δ(ara, leu)7697 galU galK rpsL(StrR) nupG trfA dhfr) (Epicentre, USA) to generate the Ec-PprM strain. The E. coli strain carrying the empty vector, pASK-IBA3, was designated as Ec-pASK. The E. coli ycgZ, ymgA, ymgB, and ymgC genes were PCR amplified using forward and reverse primers specific for each gene, as detailed in Table S1. The entire ycgZ-ymgABC operon was PCR amplified using ycgZ-F and ymgC-R primers (Table S1). Each PCR product was cloned into EcoRI and PstI sites in pASK-IBA3. The resulting plasmids, pYcgZ, pYmgA, pYmgB, pYmgC, and pYZYC (carrying the entire ycgZ-ymgABC operon) were verified through nucleotide sequencing and transformed into EPI300. To construct the E. coli oxyR mutant strain, a one-step gene inactivation method (i.e., λ-Red recombination system) was used (Datsenko and Wanner, 2000). Briefly, the RED helper plasmid, pKD46, was transformed into EPI300 to generate EPI300-pKD46. A chloramphenicol cassette from pKD3 was PCR amplified using oxyR-MF and oxyR-MR primers (Table S1), and the resulting PCR product was transformed into EPI300-pKD46 through electroporation. The oxyR mutation was confirmed by PCR using the diagnostic primers, oxyR-DF and oxyR-DR (Table S1), followed by nucleotide sequencing.
Growth Conditions
The E. coli recombinant strains carrying pASK-IBA3 and its derivatives were routinely cultivated in LB medium (1% tryptone, 0.5% yeast extract, and 0.5% NaCl) at 37°C with shaking or on LB agar supplemented with 1.5% Bacto-agar. A stationary-phase culture grown for 18 h was used as the seed culture. The seed culture was inoculated into fresh LB broth at a 1:100 dilution and grown to mid-log phase (OD600 ≈ 0.5) at 37°C. For protein expression, the mid-log cultures of E. coli were further incubated for 2 h in the presence of anhydrotetracycline (AHT), an inducer of the tetracycline promoter of pASK-IBA vectors. Ampicillin (100 μg/ml) and chloramphenicol (25 μg/ml) were used when necessary.
Preparation of Cell-Free Extracts
After centrifugation, the cell pellet was resuspended in lysis buffer [50 mM Tris-HCl (pH 8.0), 300 mM NaCl, 4 mM 2-mercaptoethaol, 1 mM phenylmethylsulfonyl fluoride (PMSF) and 10% glycerol] and lysed using a VP-15S homogenizer (TAITEC, Japan). The cell lysates were subjected to centrifugation at 12,000 g for 20 min at 4°C. The protein concentration in the clarified supernatant was determined using the Bradford colorimetric assay with bovine serum albumin (BSA) as the standard.
Western Blot Analysis
The pprM gene was PCR amplified using pprM-WF and pprM-WR primers (Table S1) and subsequently cloned into the NdeI and XhoI sites of the pET-22b expression vector. The resulting plasmid was used to transform E. coli BL21 (DE3). When the recombinant strain reached an OD600 of 0.5 at 30°C, 1 mM isopropyl β-D-1-thiogalactopyranoside (IPTG) was added to the cultures. Following PprM expression for 4 h at 30°C, PprM was purified using a Ni-NTA column as previously described (Jeong et al., 2016a). The purified PprM protein was used for immunizing CD-1 mice to obtain a polyclonal antibody against PprM, which was subsequently used in Western blot analysis. Following 2-h incubation with AHT, Ec-pASK and Ec-PprM were harvested for lysis (see “Preparation of cell-free extracts”). Equivalent amounts of cell-free extract (10 μg) were resolved on a 12% Bis-Tris gel with MOPS buffer and subsequently transferred onto a polyvinylidene fluoride (PVDF) membrane. The PVDF membranes were incubated with the primary antibody to PprM (1:5000) and sequentially probed with peroxidase-conjugated goat anti-mouse antibody (1:5000) (Sigma, USA). The secondary antibody was detected using a Pierce ECL Western Blotting Substrate according to the manufacturer's instructions (Thermo Scientific, USA). The chemiluminescent signals on the PVDF membrane were visualized using a ChemiDoc Touch Imaging System (Bio-Rad, USA).
Survival Studies
Survival studies were performed 2 h after the addition of AHT (200 ng/ml). The E. coli cultures were harvested, washed, and resuspended in phosphate buffer (20 mM, pH 7.4). The cells were incubated with different concentrations of H2O2 (ranged from 0 to 40 mM) for 1 h. Acid stress survival studies were performed as described previously (Lee et al., 2007b). The cells was diluted 40-fold in LB acidified to pH 2.5 and incubated at 37°C for different time intervals. The stressed cells were serially diluted with phosphate buffer, and 10 μl of each dilution was spotted on LB-ampicillin agar plates. For continuous exposure to H2O2, the cells were adjusted to give ~107 CFU/ml (OD600 ≈ 0.1) and then serially diluted 10-fold in phosphate buffer from 107 to 102 CFU/ml. A 10-μl volume (105 to 100 CFU) from each diluted suspension was spotted on the LB agar plates containing H2O2 (ranged from 0 to 0.6 mM). The plates were incubated at 37°C for 18 h prior to the enumeration of the colonies. The survival fraction was calculated by dividing the number of colonies of stressed cells by the number of colonies of non-stressed cells.
Quantitative Real-Time PCR (qRT-PCR)
Following the 2 h incubation with AHT (200 ng/ml), the cells were exposed to 10 mM of H2O2 for 15 min and immediately mixed with 10% volume (v/v) of ice-cold phenol-ethanol mixture (5% phenol and 95% ethanol) to preserve RNA integrity. Total RNA was isolated both from non-stressed and stressed cells using a RiboEX reagent (GeneAll, Korea), treated with DNase, and purified using a RNeasy Mini Kit (Qiagen, Germany) according to the manufacturer's instructions. cDNA was synthesized from 1 μg of RNA from each of the samples using a PrimeScript 1st strand cDNA Synthesis Kit (Takara Bio, Japan). For qRT-PCR analysis, 20 μl of the reaction solution containing 10 μl of 2 × SYBR Green PCR Mix (Takara Bio), 1 μl of template cDNA, 0.5 μl of each primer, and 8 μl of RNase-free water was prepared. The housekeeping gap gene encoding glyceraldehyde-3-phosphate dehydrogenase (GAPDH) was used as an internal control. The qRT-PCR was run at 95°C for 10 min, followed by 40 cycles of 95°C for 15 s, 60°C for 15 s, and 72°C for 15 s. Amplification, data acquisition, and analysis were performed on an Eco™ Real-Time PCR System (Illumina, USA). Data expressed as means ± standard errors were compared for statistical significance by unpaired t-test. The primer sequences used for qRT-PCR are listed in Table S2.
Catalase Activity Assay
The cell-free extracts were prepared as mentioned in “Preparation of cell extracts.” Catalase activity was measured using a spectrophotometric assay as previously described (Jeong et al., 2016a). Ten micrograms of the cell extract were mixed with 1 ml of 50 mM phosphate buffer (pH 7.0) containing 10 mM H2O2 and incubated for 5 min at 25°C. Catalase activity was calculated from the rate of decrease in absorbance at 240 nm resulting from the decomposition of H2O2. After the rate of decrease in the absorbance was calculated, the catalase activities were expressed as a percentage relative to wild type (set to 100%).
Determination of Intracellular Mn/Fe Ratio
Total amounts of intracellular iron and manganese were measured using an Inductively Coupled Plasma Atomic Emission Spectrometer (ICP-AES). Following 2 h incubation with AHT (200 ng/ml), one-liter cultures of Ec-PprM and Ec-pASK strains were centrifuged and washed twice with ice-cold 10 mM Tris-HCl containing 1 mM EDTA and once with ice-cold 10 mM Tris-HCl. After lyophilization, the metal contents in the samples were determined using an Optima 4300 DV ICP spectrometer (Perkin Elmer, USA) at the Korea Basic Science Institute (KBSI, Gwangju, South Korea).
EPR Spin Trapping
The amounts of hydroxyl radical (·OH) in Ec-PprM and Ec-pASK cells were detected using electron paramagnetic resonance (EPR) spectroscopy with the specific probe ethanol/a-(4-pyridyl-1-oxide)-N-tert-butylnitrone (4-POBN) as previously described (Lee et al., 2009). Following 2 h incubation with AHT (200 ng/ml), the cells were washed twice with ice-cold Chelex-treated Hank's balanced salt solution (HBSS) and resuspended in ice-cold HBSS. The cell suspension was added to a mixture containing 100 mM diethylenetriaminepentaacetic acid (DTPA), 10 mM 4-POBN, 170 mM ethanol, 1 mM H2O2, and HBSS. The final cell densities were equivalent in all samples (OD600 of ~20). The EPR spectra were measured on a JES-FA200 ESR spectrometer (JEOL, Japan). The spectra were recorded at room temperature with a 9.4 GHz microwave frequency, 10 mW microwave power, 0.2 mT modulation amplitude, 100 kHz modulation frequency, and 3.0 × 10 amplification.
Detection of Carbonylated Proteins
Following the 2 h incubation with AHT (200 ng/ml), the cells were exposed to 10 and 20 mM H2O2 for 1 h. The cell-free extracts (50 μg) were prepared as mentioned in “Preparation of cell extracts,” and protein carbonylation was detected as previously described (Daly et al., 2007). The carbonyl groups in the E. coli protein samples were derivatized to 2,4-dinitrophenylhydrazone by reaction with 2,4-dinitrophenylhydrazine (DNPH) using a OxyBlot Protein Oxidation Detection Kit (Millipore, USA) according to the manufacturer's instructions. The protein samples were resolved on a 12% Bis-Tris gel and subsequently transferred to PVDF membrane. The dinitrophenol (DNP) adduct of the carbonyls were probed using the primary and secondary antibodies provided in the Oxyblot Kit (Millipore). The chemiluminescent signals produced using the Pierce ECL Western Blotting Substrate were visualized using the ChemiDoc Touch Imaging System (Bio-Rad).
Results
Overexpression of pprM in E. coli Enhances H2O2 Tolerance
To determine whether PprM protects E. coli cells from oxidative stress, E. coli cells expressing PprM (Ec-PprM) or carrying an empty vector (Ec-pASK) were grown to early log phase, followed by incubation for 2 h with anhydrotetracycline (AHT) to produce PprM. Subsequently, these cells were treated with different amounts of H2O2 (0–40 mM) for 1 h. The surviving fraction of Ec-pASK was dramatically decreased in a H2O2 concentration-dependent manner, whereas Ec-PprM maintained the survival rate (Figure 1A). The incubation of Ec-pASK with 40 mM of H2O2 decreased cell survival for approximately 4 log cycles, but Ec-PprM easily survived incubation with up to 40 mM of H2O2 with less than 1 log cycle of reduction (Figure 1A). Next, to determine the PprM effect at low concentration of H2O2 (below 1 mM), Ec-PprM cells were spotted on the plates containing H2O2 and incubated for an extended period of time (18 h) because exposure time is inversely related to concentration. The survival fraction of Ec-PprM grown in the presence of H2O2 was reduced but still was 0.5 and 1-log cycle higher at 0.4 and 0.6 mM H2O2, respectively, relative to Ec-pASK (Figure 1B). To investigate the relevance of H2O2 tolerance according to the PprM expression level, we monitored the cell survival rate under different AHT concentrations, followed by treatment with 20 mM H2O2. Western blot analysis showed that the cellular levels of PprM protein were increased in an AHT concentration-dependent manner (Figure 1C), and cell survival was also gradually increased in a PprM dependent-manner (Figure 1D). Taken together, these results indicate that the heterologous expression of PprM enhances the oxidative stress tolerance of E. coli, and the level of tolerance is directly associated with the amount of PprM in the cell.
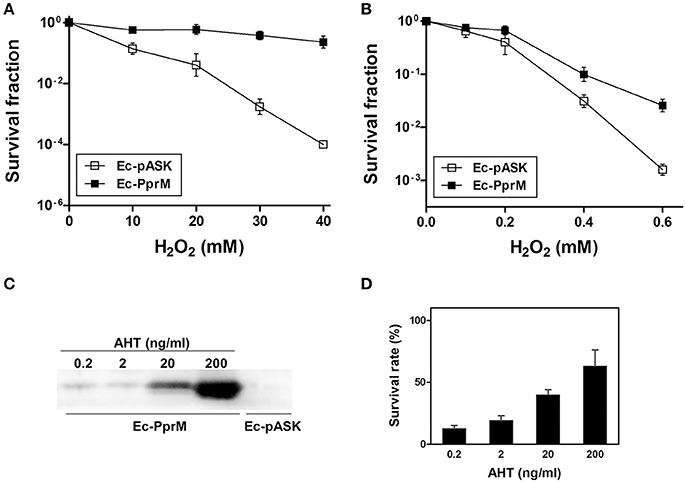
Figure 1. Survival of E. coli expressing pprM under H2O2 stress. Following the 2 h incubation with AHT (200 ng/ml), cells expressing pprM (Ec-PprM) and carrying an empty vector (Ec-pASK) were exposed to H2O2 (10, 20, 30, and 40 mM) for 1 h (A) or were spotted on the plates containing H2O2 (0.1, 0.2, 0.4, and 0.6 mM) (B). The survival fraction was calculated by dividing the colony-forming units (CFUs) of H2O2-treated cells by the CFUs of non-treated cells. The error bars represent the standard deviation of three independent experiments (n = 3). (C) Ec-PprM grown to early log phase was incubated at the indicated concentrations of AHT for 2 h, and Ec-pASK was incubated in 200 ng/ml AHT. Equivalent amounts of cell-free extract (10 μg) were loaded in each lane. The PprM levels were measured through Western blotting using anti-PprM antibodies. (D) Ec-PprM was incubated in different concentrations of AHT for 2 h and subsequently exposed to 20 mM H2O2 for 1 h. The survival rate was measured through comparison to non-treated cells, with the CFUs of non-treated cells set to 100%. The error bars represent the standard deviation of three independent experiments (n = 3).
Oxidative Stress Response Genes Are Activated in Ec-PprM
The OxyR transcription factor is one of the major regulators activated during oxidative stress in E. coli. OxyR immediately senses the presence of H2O2 and induces the antioxidant system (Chiang and Schellhorn, 2012). To examine the mechanism of how PprM confers H2O2 tolerance to E. coli, we initially determined the mRNA levels of OxyR-dependent genes, including grxA (glutaredoxin 1), dps (nonspecific DNA binding protein), sufB (Fe-S cluster assembly protein), ahpCF (alkyl hydroperoxide reductase), fur (ferric uptake regulator), mntH (Mn transporter), hemH (ferrochelatase), and katG (bifunctional hydroperoxidase I, HPI) using quantitative RT-PCR (qRT-PCR) analysis. Although the expression of oxyR was down-regulated, we observed that most of the OxyR-dependent genes tested, except katG, were increased in Ec-PprM prior to H2O2 treatment compared with Ec-pASK (Figure 2). Thus, it is likely that the pre-activation of these genes prepares the cells for oxidative stress by increasing their antioxidant capacity prior to H2O2 exposure. When the gene expression levels were compared between Ec-PprM and Ec-pASK cells treated with H2O2, the expression levels of sufB, ahpCF, fur, mntH, and hemH were higher in Ec-PprM+H2O2 than in Ec-pASK+H2O2, and grxA and dps expressions were comparable in both strains (Figure 2). Particularly, the fold increases (from 9- to 16-fold) of mntH and hemH expression were much higher than other OxyR-dependent genes tested, regardless of H2O2 treatment (Figure 2). These results suggest that mntH and hemH play more important roles than other genes in conferring H2O2 tolerance to Ec-PprM.
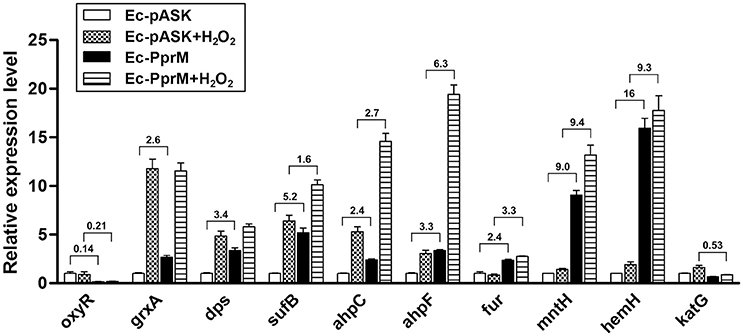
Figure 2. qRT-PCR assay of OxyR-dependent genes in Ec-PprM. Following the 2 h incubation with AHT (200 ng/ml), cells were exposed to 10 mM H2O2 for 15 min. The relative expression values were determined by defining the mRNA levels of each gene from non-treated Ec-pASK cells as 1. The error bars represent the standard error of three independent experiments conducted in duplicate (n = 3). Fold changes of genes showing a statistically significant difference (p < 0.05) in expression between Ec-pASK and Ec-PprM are indicated.
Increased Intracellular Manganese Protects Proteins in Ec-PprM
The modulation of the cellular metal ion content is an important and widespread component of bacterial adaptive responses to H2O2. Particularly, a high manganese to iron (Mn/Fe) ratio has been correlated with ROS resistance (Faulkner and Helmann, 2011). One of the distinct features observed in the qRT-PCR results was the up-regulation of mntH, which encodes the Mn transporter (Figure 2). Because the enhanced expression of mntH might increase the intracellular Mn concentration, we compared the Mn concentration between Ec-pASK and Ec-PprM. Ec-PprM showed more than 2 times higher Mn levels than Ec-pASK, and the Fe level decreased by approximately 25% in Ec-PprM compared with Ec-pASK. Notably, the Mn/Fe ratio was at least 2.5 times higher in Ec-PprM (Table 1). In a spontaneous reaction catalyzed by ferrous iron (Fenton reaction), H2O2 can be converted to highly reactive ·OH (Lisher and Giedroc, 2013). Intracellular ·OH is trapped by ethanol/4-POBN, forming hydroxyethyl-POBN adducts, which can be detected by electron paramagnetic resonance (EPR) spin trapping (Lee et al., 2009). The relative intensity of the six-line EPR signal, which is characteristically exhibited by POBN radical adducts, was markedly decreased in Ec-PprM compared with Ec-pASK (Figure 3), demonstrating that the ·OH level is lower in Ec-PprM than in Ec-pASK. This result is consistent with the decreased Fe level in Ec-PprM (Table 1).
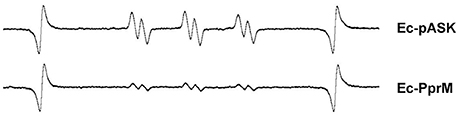
Figure 3. EPR absorption of the POBN-hydroxyethyl adduct diagnostic for ·OH. Following the 2 h incubation with AHT (200 ng/ml), the cell suspension of Ec-pASK and Ec-PprM was mixed with 4-POBN and ethanol. The presence of POBN-adducts was detected using EPR spectroscopy.
Bacteria with high Mn/Fe ratios are resistant to protein oxidation, whereas bacteria with low Mn/Fe ratios are sensitive to protein oxidation (Daly, 2009). The level of carbonyl groups in proteins, detected through Western blot analysis, has been widely used as a marker of oxidative protein damage (Daly et al., 2007). The proteins extracted from Ec-PprM exhibited substantially less carbonyl groups compared with Ec-pASK (Figure 4). After H2O2 treatments, high levels of protein oxidation occurred in Ec-pASK, whereas low levels of protein carbonylation were observed in Ec-PprM, suggesting that the high Mn/Fe ratio in Ec-PprM renders E. coli cells more tolerant to oxidative stress through the protection of proteins from ROS.
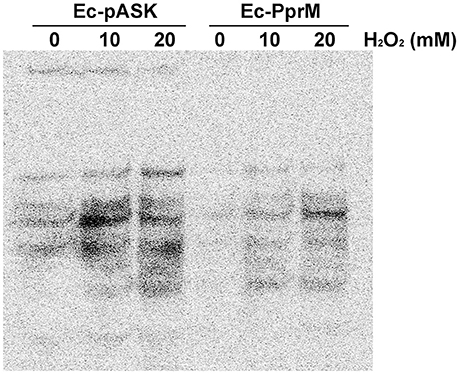
Figure 4. Protein carbonylation assay of Ec-PprM. Following the 2 h incubation with AHT (200 ng/ml), cells were exposed to the indicated concentrations of H2O2 for 1 h. Equivalent amounts of cell-free extract (50 μg) were loaded in each lane. The protein carbonyl groups (a marker of oxidative damage) were derivatized with DNPH and detected using an antibody to DNP.
Ec-PprM Shows H2O2 Tolerance in the Absence of oxyR
Because oxyR expression was not induced in Ec-PprM (Figure 2), an E. coli oxyR mutant strain was constructed to investigate whether OxyR is necessary for the enhanced H2O2 tolerance of Ec-PprM. As expected, E. coli cells became sensitive to H2O2 stress in the absence of oxyR. The oxyR mutant strain carrying an empty vector (ΔoxyR-pASK) could not survive under 40 mM H2O2 (Figure 5). Interestingly, when pprM was expressed in oxyR mutants (ΔoxyR-PprM), ΔoxyR-PprM showed a greater H2O2 tolerance than Ec-pASK. The survival rate of ΔoxyR-PprM was approximately 2 log cycles higher than that of Ec-pASK under 40 mM H2O2 (Figure 5). In addition, the expression of the OxyR-dependent genes (sufB, dps, and mntH) tested was not increased in ΔoxyR-PprM (data not shown). These results suggest that OxyR-independent genes contribute to PprM-mediated H2O2 tolerance in the oxyR deletion background.
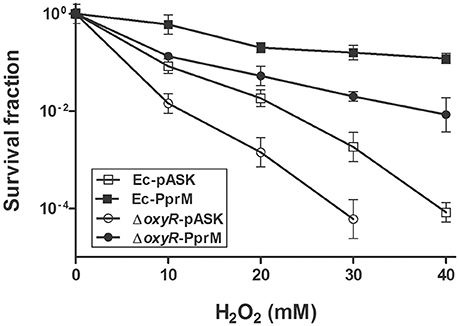
Figure 5. Survival of oxyR mutants under H2O2 stress. Following the 2 h incubation with AHT (200 ng/ml), the wild-type and oxyR mutant strains expressing pprM (Ec-PprM and ΔoxyR-PprM, respectively) and carrying an empty vector (Ec-pASK and ΔoxyR-pASK, respectively) were exposed to H2O2 at the indicated concentrations for 1 h. The survival fraction was calculated by dividing the CFUs of H2O2-treated cells by the CFUs of non-treated cells. The error bars represent the standard deviation of three independent experiments (n = 3).
Activation of ycgZ-ymgABC Leads to Elevated H2O2 Tolerance
A previous study has reported the activation of a set of genes by H2O2 in ΔoxyR (Zheng et al., 2001). Some of these genes were elevated in Ec-PprM in the absence of H2O2 stress (Figure S1). Among the 29 genes tested, ymgA and ymgB showed the highest expression levels (Figure S1). In E. coli, ycgZ, ymgA, ymgB, and ymgC constitute an operon (ycgZ-ymgABC) that encodes small proteins of 78–90 amino acids (Tschowri et al., 2009, 2012). We confirmed that all four genes were expressed more than 100-fold in Ec-PprM, regardless of H2O2 treatments (Figure 6). Next, we constructed E. coli strains overexpressing each gene of the ycgZ-ymgABC operon and examined the viability of strains subjected to H2O2 stress. Ec-YcgZ and Ec-YmgB showed robust H2O2 stress tolerance (Figure 7A): the survival rates of both strains were approximately 3 log cycles higher than that of Ec-pASK at 40 mM H2O2, which is comparable to that of Ec-PprM (Figure 1A). Ec-YmgA was less tolerant to H2O2 than Ec-YcgZ and Ec-YmgB but still showed an approximately 1-log cycle increase in the survival rate compared with Ec-pASK at 30 and 40 mM H2O2 (Figure 7A). Although Ec-YmgC was more sensitive to H2O2 than Ec-pASK, the E. coli strain overexpressing the ycgZ-ymgABC operon (Ec-YZYC) exhibited the highest H2O2 stress tolerance (Figure 7A). The cell survival rate of the E. coli strain overexpressing the ycgZ-ymgAB region was not different from that of Ec-YZYC (data not shown), suggesting that the negative effect of YmgC overexpression on cell survival can be masked by the simultaneous expression of the ycgZ, ymgA, and ymgB genes in Ec-YZYC. The overexpression of the entire operon markedly increased the cell survival rate of ΔoxyR under H2O2 stress conditions, which was higher than those of not only ΔoxyR-YcgZ and ΔoxyR-YmgB but also ΔoxyR-PprM (Figure 7B). These results suggest that the activation of the ycgZ-ymgABC operon through PprM enhances the H2O2 stress tolerance of E. coli in the absence of OxyR.
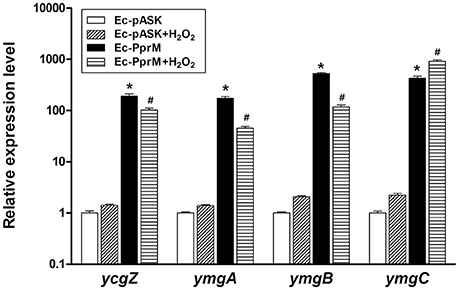
Figure 6. qRT-PCR assay of the ycgZ-ymgABC operon. Following the 2 h incubation with AHT (200 ng/ml), cells were exposed to 10 mM H2O2 for 15 min. The relative expression values were determined by defining the mRNA levels of each gene from non-treated Ec-pASK cells as 1. The error bars represent the standard error of three independent experiments conducted in duplicate (n = 3). Data were analyzed by Student's t-test. *p < 0.01 compared with Ec-pASK; #p < 0.01 compared Ec-pASK+H2O2.
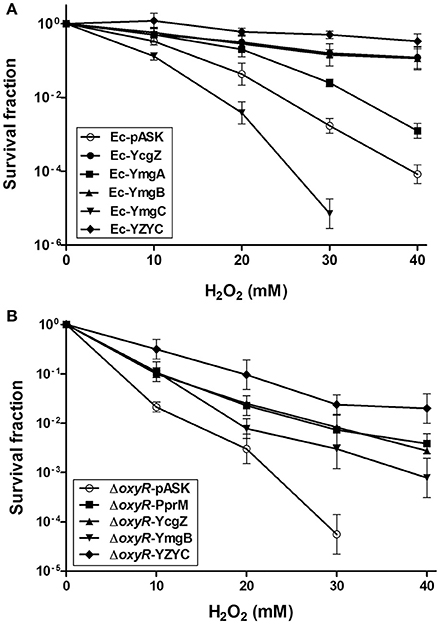
Figure 7. Survival of E. coli expressing the ycgZ and ymg genes under H2O2 stress. Following the 2 h incubation with AHT (200 ng/ml), the recombinant strains were exposed to H2O2 at the indicated concentrations for 1 h. The survival fraction was calculated by dividing the CFUs of H2O2-treated cells by the CFUs of non-treated cells. The error bars represent the standard deviation of three independent experiments (n = 3). (A) The wild-type strains expressing ycgZ, ymgA, ymgB, ymgC, and the entire ycgZ-ymgABC operon were designated Ec-YcgZ, Ec-YmgA, Ec-YmgB, Ec-YmgC, and Ec-YZYC, respectively. (B) The oxyR mutant strains expressing pprM, ycgZ, ymgB, and the entire ycgZ-ymgABC operon were designated ΔoxyR-PprM, ΔoxyR-ycgZ, ΔoxyR-YmgB, and ΔoxyR-YZYC, respectively.
Discussion
Stress tolerance and cellular robustness are key characteristics for industrial microbes. In an effort to improve the robustness of E. coli as an industrial host, many strategies have been used, including stress-induced mutagenesis, combinatorial gene deletion, engineering of transcriptional regulators, and synthetic biology (Zhu et al., 2012; Lin et al., 2013; Lennen and Herrgård, 2014; Zhu et al., 2014). The introduction or overexpression of specific proteins, such as heat shock proteins (HSPs), also enhances resistance at the cellular level (Jia et al., 2014). Particularly, microbes living in extreme environments, known as extremophiles, provide genetic resources for developing robust hosts (Lin et al., 2013; Jia et al., 2014; Appukuttan et al., 2016). Here, we enhanced the oxidative stress (H2O2) tolerance of E. coli through the heterologous expression of PprM, a CSP homolog of the extraordinary γ-radiation-resistant bacterium D. radiodurans (Figure 1).
The E, coli OxyR transcription factor is one of the major regulators activated during oxidative stress. OxyR controls a regulon of approximately 40 genes induced through H2O2 and protects E. coli from H2O2 toxicity. Upon exposure to H2O2, OxyR is activated by the formation of an intramolecular disulfide bond that induces a conformational change, and the oxidized OxyR binds to the promoter regions of target genes to activate transcription (Chiang and Schellhorn, 2012). Although most of the OxyR regulon genes examined in this study were activated in Ec-PprM, oxyR transcription was decreased (Figure 2). Because OxyR acts as a repressor of its own transcription (Pomposiello and Demple, 2001), it is likely that OxyR oxidation occurs in Ec-PprM, thereby activating OxyR regulon genes and repressing oxyR expression. The thioredoxin (Trx) system and the glutathione (GSH)-glutaredoxin system can deactivate OxyR through the reduction of disulfide bonds in OxyR (Lu and Holmgren, 2014). During aerobic growth, the disruption of both GSH metabolism and Trx partially activates OxyR in the absence of added H2O2 (Pomposiello and Demple, 2001), and E. coli strains without Trx proteins are more resistant to oxidative stress, reflecting the defective OxyR reduction (Lu and Holmgren, 2014), suggesting that multiple systems contribute to the maintenance of OxyR in the reduced state. There is no direct evidence that PprM participates in OxyR activation, but the OxyR reduction systems might be hampered as a result of PprM overexpression.
Catalases protect cells through catalysis of H2O2 decomposition into oxygen and water (Imlay, 2013). In E. coli, deinococcal Ppr proteins PprI and PprA stimulated KatG (bifunctional catalase-peroxidase) and KatE (monofunctional hydroperoxidase II), respectively, thereby improving tolerance against oxidative stress (Gao et al., 2003; Kota and Misra, 2006). However, katG was not activated in Ec-PprM (Figure 2), and the total catalase activity of Ec-PprM was not significantly altered following AHT addition (Figure S2). E. coli possesses nine CSP homologs, CspA to CspI: CspA, CspB, CspG, and CspI are cold inducible, whereas CspE and CspC are constitutively expressed at physiological temperatures, and CspD is induced under nutrient deprivation (Horn et al., 2007). CSPs function as RNA chaperones that maintain the single-stranded state of RNA by melting the secondary structure for efficient transcription and/or translation (Horn et al., 2007). katG requires the melting function of CspE for optimal expression at physiological temperatures (37°C) (Phadtare et al., 2006). PprM also exerted an effect under physiological temperatures (Ohba et al., 2009). Thus, it is likely that katG expression might not be activated by the competition of exogenous deinococcal CSP PprM and endogenous E. coli CSPs, suggesting that catalase makes a little, if any, contribution to the H2O2 tolerance of Ec-PprM.
The OxyR regulon contributes to the suppression of the Fenton reaction, and Mn import via MntH is a key element of the OxyR response to H2O2 in E. coli (Anjem et al., 2009). E. coli is capable of shifting from metabolism based on Fe2+ to metabolism based on Mn2+ to protect key enzymes from inactivation by ROS (Lisher and Giedroc, 2013). mntH was up-regulated by approximately 9-fold in Ec-PprM (Figure 2), and Ec-PprM showed increased intracellular Mn/Fe levels of more than two times compared with wild type (Table 1). Mn acts as a chemical scavenger of through the formation of low molecular weight Mn complexes, such as Mn-phosphate and Mn-carbonate, and metalloenzyme cofactor substitution to prevent Fenton-based protein damage and subsequent enzyme inactivation (Lisher and Giedroc, 2013). Mn has also been implicated in the metalation of the Mn-cofactor superoxide dismutase (SodA), which catalyzes the conversion of to H2O2 and water (Imlay, 2013). In this context, the low levels of intracellular ·OH and protein oxidation compared with wild type (Figures 3, 4) suggests the possibility that Mn plays an important role in the oxidative stress tolerance of Ec-PprM.
PprM overexpression activated the expression of the ycgZ-ymgABC operon (Figure 6), which enhanced the H2O2 stress tolerance of Ec-PprM, even in the absence of OxyR (Figure 7). The ycgZ-ymgABC operon is located immediately adjacent to the ycgF-ycgE region separated by a divergently transcribing control region (Tschowri et al., 2009, 2012). The expression of the ycgZ-ymgABC operon is repressed by YcgE (BluR), and YcgF (BluF) directly antagonizes YcgE, leading to the expression of the ycgZ-ymgABC operon in a light-dependent manner (Tschowri et al., 2009, 2012). When we examined the expression of ycgE and ycgF, both genes showed increased expression in Ec-PprM, but the expression of ycgE was higher than that of ycgF (Figure S1). Thus, the activation of the ycgZ-ymgABC operon in Ec-PprM might not be dependent on YcgF. It has recently been reported that the promoter region of the ycgZ-ymgABC operon is bound by apo-Fur (iron-free Fur) under iron starvation, and this binding increases transcript levels (Seo et al., 2014). Considering the increased fur expression (Figure 2) and decreased Fe levels (Table 1), apo-Fur might activate the ycgZ-ymgABC operon expression in Ec-PprM. Ferrochelatase, encoded by hemH, completes heme synthesis through the insertion of a ferrous iron into protoporphyrin IX (Mancini and Imlay, 2015). Notably, hemH was markedly induced in Ec-PprM (Figure 2), suggesting the possibility that the increased induction of hemH could facilitate apo-Fur formation through the reduction of intracellular unincorporated iron. In E. coli, mntH transcription is repressed in the presence of iron in a Fur-dependent manner (Patzer and Hantke, 2001), but mntH was induced in Ec-PprM, similar to hemH (Figure 2). This result suggests that Fur lacked the ferrous iron cofactor and thus did not exert a negative effect. Indole is an extracellular signal in E. coli, and this compound represses the ycgZ-ymgABC operon 2- to 5-fold (Lee et al., 2007a). We observed that the tnaA gene, encoding tryptophanase, which converts tryptophan into indole, was repressed approximately 50-fold in Ec-PprM (Figure S1). Further systematic studies are needed to elucidate the mechanisms underlying the up-regulation of the ycgZ-ymgABC operon in Ec-PprM.
YmgB is important for the survival of E. coli against H2O2 (Lee et al., 2007b), but the molecular functions of the YcgZ and Ymg proteins are not yet clearly understood. Ymg proteins have been implicated in biofilm formation as mutations in ymgA, ymgB, and ymgC increased biofilm formation (Lee et al., 2007b), and YcgZ partially counteracts the effects of YmgB (Tschowri et al., 2009). However, ymgC expression resulted in an effect opposite to that of ycgZ and ymgB in the present study: the overexpression of ycgZ and ymgB (and to a lesser extent YmgA) enhanced H2O2 tolerance, whereas ymgC expression decreased H2O2 tolerance (Figure 7). Of the ycgZ, ymgA, ymgB, and ymgC mutants, only the ymgB mutant showed approximately 40-fold less survival in an acidic LB medium (pH 2.5 for 1 h) (Lee et al., 2007b). Thus, the E. coli strains overexpressing the ycgZ and/or ymg genes were examined for acid stress tolerance in the same conditions (LB medium, pH 2.5). Not only YmgB but also YcgZ rendered E. coli more resistant to low-pH stress, and the co-expression of the ycgZ-ymgABC genes enhanced acid tolerance more than 10-fold compared with Ec-pASK. However, Ec-YmgC showed an approximately 1-log cycle decrease in survival relative to Ec-pASK after 2 h incubation under acidic conditions (Figure S3). Taken together, these results suggest that YmgC might play a different role depending on the types of environmental stressors. Although Northern blot analysis indicated that all four genes are expressed in a single polycistronic mRNA (Tschowri et al., 2009), ycgZ, ymgA, and ymgB, but not ymgC, are under the control of the general stress σ factor σS (RpoS) (Weber et al., 2005). The fact that the ycgZ-ymgAB region (without ymgC) is conserved in various bacteria shows that this region is a functional genetic unit (Tschowri et al., 2012). Of the acid resistance (AR) systems in E. coli, AR1 referred to as oxidative system is dependent on σS (Chung et al., 2006). Because the overexpression of ycgZ and/or ymgAB expression protected cells from oxidative and acid stress, it is thus possible that the contribution of YcgZ and YmgB to acid stress tolerance lies in the protection against oxidative damage. The investigation of the roles of the YcgZ and Ymg proteins in stress response system sheds light on the stress tolerance mechanisms of not only Ec-PprM but also E. coli itself.
Because exposure to low pH leads to oxidative stress (Lund et al., 2014), and increased expression of OxyR-dependent genes are observed by transcriptional profiling of acid tolerant phenotypes (Chung et al., 2006), metabolic and genetic engineering of Ec-PprM for organic acid production can increase productivity at low pH. Ec-PprM can also be applied to the wastewater treatment system like Advanced Oxidation Process (AOP), where high levels of H2O2 are used as oxidizing agents (Ramteke and Gogate, 2016; Son et al., 2016). The removal efficiency of chemical oxygen demand by the Fenton oxidation-based AOP (FAOP) was significantly enhanced by the addition of 1.5 g/L (approximately 44 mM) of H2O2 (Ramteke and Gogate, 2016). It has been reported that subsequent biological oxidation using microorganism shows the synergistic effect in oxidizing organic pollutants present in the wastewater pretreated by FAOP (Mandal et al., 2010; Meenatchisundaram et al., 2014; Ramteke and Gogate, 2016). In particular, the efficacy of Fenton processes is enhanced under acidic conditions (pH 2.5 ~ 3.5) (Mandal et al., 2010; Ramteke and Gogate, 2016). Considering the maintained survivability of Ec-PprM at high concentrations of H2O2 (up to 40 mM) (Figure 1A), in addition to its acid tolerance (Figure S3), it is possible to use this strain in FAOP and to develop a new hybrid FAOP using genetically engineered Ec-PprM.
Author Contributions
SL, YC, and IN contributed with experimental design and results interpretation of this study. SP and HS carried out all experiments, DA and JJ advised the cell survival assay, and SJ purified the PprM protein. SP and HS wrote the draft manuscript, and SL was in charge of writing the manuscript. All authors have made substantial contribution to the work and approved it for publication.
Funding
This research was supported by Nuclear R&D program of Ministry of Science, ICT & Future Planning (MSIP), Republic of Korea.
Conflict of Interest Statement
The authors declare that the research was conducted in the absence of any commercial or financial relationships that could be construed as a potential conflict of interest.
Supplementary Material
The Supplementary Material for this article can be found online at: http://journal.frontiersin.org/article/10.3389/fmicb.2016.02124/full#supplementary-material
References
Anjem, A., Varghese, S., and Imlay, J. A. (2009). Manganese import is a key element of the OxyR response to hydrogen peroxide in Escherichia coli. Mol. Microbiol. 72, 844–858. doi: 10.1111/j.1365-2958.2009.06699.x
Appukuttan, D., Singh, H., Park, S.-H., Jung, J.-H., Jeong, S., Seo, H. S., et al. (2016). Engineering synthetic multi-stress tolerance in Escherichia coli by using a deinococcal response regulator, DR1558. Appl. Environ. Microbiol. 82, 1154–1166. doi: 10.1128/AEM.03371-15
Basak, S., and Jiang, R. (2012). Enhancing E. coli tolerance towards oxidative stress via engineering its global regulator cAMP receptor protein (CRP). PLoS ONE 7:e51179. doi: 10.1371/journal.pone.0051179
Chiang, S. M., and Schellhorn, H. E. (2012). Regulators of oxidative stress response genes in Escherichia coli and their functional conservation in bacteria. Arch. Biochem. Biophys. 525, 161–169. doi: 10.1016/j.abb.2012.02.007
Chung, H. J., Bang, W., and Drake, M. A. (2006). Stress response of Escherichia coli. Compr. Rev. Food Sci. Food Saf. 5, 52–64. doi: 10.1111/j.1541-4337.2006.00002.x
Clomburg, J. M., and Gonzalez, R. (2010). Biofuel production in Escherichia coli: the role of metabolic engineering and synthetic biology. Appl. Microbiol. Biotechnol. 86, 419–434. doi: 10.1007/s00253-010-2446-1
Daly, M. J. (2009). A new perspective on radiation resistance based on Deinococcus radiodurans. Nat. Rev. Microbiol. 7, 237–245. doi: 10.1038/nrmicro2073
Daly, M. J., Gaidamakova, E. K., Matrosova, V. Y., Vasilenko, A., Zhai, M., Leapman, R. D., et al. (2007). Protein oxidation implicated as the primary determinant of bacterial radioresistance. PLoS Biol. 5:e92. doi: 10.1371/journal.pbio.0050092
Datsenko, K. A., and Wanner, B. L. (2000). One-step inactivation of chromosomal genes in Escherichia coli K-12 using PCR products. Proc. Natl. Acad. Sci. U.S.A. 97, 6640–6645. doi: 10.1073/pnas.120163297
Devigne, A., Mersaoui, S., Bouthier-de-la-Tour, C., Sommer, S., and Servant, P. (2013). The PprA protein is required for accurate cell division of γ-irradiated Deinococcus radiodurans bacteria. DNA Repair (Amst). 12, 265–272. doi: 10.1016/j.dnarep.2013.01.004
Faulkner, M. J., and Helmann, J. D. (2011). Peroxide stress elicits adaptive changes in bacterial metal ion homeostasis. Antioxid. Redox. Signal. 15, 175–189. doi: 10.1089/ars.2010.3682
Ferrer-Miralles, N., Domingo-Espín, J., Corchero, J. L., Vázquez, E., and Villaverde, A. (2009). Microbial factories for recombinant pharmaceuticals. Microb. Cell. Fact. 8:17. doi: 10.1186/1475-2859-8-17
Gao, G., Tian, B., Liu, L., Sheng, D., Shen, B., and Hua, Y. (2003). Expression of Deinococcus radiodurans PprI enhances the radioresistance of Escherichia coli. DNA Repair (Amst). 2, 1419–1427. doi: 10.1016/j.dnarep.2003.08.012
Gopal, G. J., and Kumar, A. (2013). Strategies for the production of recombinant protein in Escherichia coli. Protein J. 32, 419–425. doi: 10.1007/s10930-013-9502-5
Haiyan, S., and Baoming, T. (2010). Radioresistance analysis of Deinococcus radiodurans gene DR1709 in Escherichia coli. Afr. J. Microbiol. Res. 4, 1412–1418.
Han, M.-J., Yoon, S. S., and Lee, S. Y. (2001). Proteome analysis of metabolically engineered Escherichia coli producing poly(3-hydroxybutyrate). J. Bacteriol. 183, 301–308. doi: 10.1128/JB.183.1.301-308.2001
Hoffmann, F., and Rinas, U. (2004). Stress induced by recombinant protein production in Escherichia coli. Adv. Biochem. Eng. Biotechnol. 89, 73–92. doi: 10.1007/b93994
Horn, G., Hofweber, R., Kremer, W., and Kalbitzer, H. R. (2007). Structure and function of bacterial cold shock proteins. Cell. Mol. Life Sci. 64, 1457–1470. doi: 10.1007/s00018-007-6388-4
Hua, Y., Narumi, I., Gao, G., Tian, B., Satoh, K., Kitayama, S., et al. (2003). PprI: a general switch responsible for extreme radioresistance of Deinococcus radiodurans. Biochem. Biophys. Res. Commun. 306, 354–360. doi: 10.1016/S0006-291X(03)00965-3
Imlay, J. A. (2013). The molecular mechanisms and physiological consequences of oxidative stress: lessons from a model bacterium. Nat. Rev. Microbiol. 11, 443–454. doi: 10.1038/nrmicro3032
Ishino, Y., and Narumi, I. (2015). DNA repair in hyperthermophilic and hyperradioresistant microorganisms. Curr. Opin. Microbiol. 25, 103–112. doi: 10.1016/j.mib.2015.05.010
Jeong, S.-W., Jung, J.-H., Kim, M.-K., Seo, H. S., Lim, H.-M., and Lim, S. (2016a). The three catalases in Deinococcus radiodurans: only two show catalase activity. Biochem. Biophys. Res. Commun. 469, 443–448. doi: 10.1016/j.bbrc.2015.12.017
Jeong, S.-W., Seo, H. S., Kim, M.-K., Choi, J.-I., Lim, H.-M., and Lim, S. (2016b). PprM is necessary for up-regulation of katE1, encoding the major catalase of Deinococcus radiodurans, under unstressed culture conditions. J. Microbiol. 54, 426–431. doi: 10.1007/s12275-016-6175-8
Jia, H., Fan, Y., Feng, X., and Li, C. (2014). Enhancing stress-resistance for efficient microbial biotransformations by synthetic biology. Front. Bioeng. Biotechnol. 2:44. doi: 10.3389/fbioe.2014.00044
Khairnar, N. P., Misra, H. S., and Apte, S. K. (2003). Pyrroloquinoline-quinone synthesized in Escherichia coli by pyrroloquinoline-quinone synthase of Deinococcus radiodurans plays a role beyond mineral phosphate solubilization. Biochem. Biophys. Res. Commun. 312, 303–308. doi: 10.1016/j.bbrc.2003.10.121
Kota, S., Charaka, V. K., Ringgaard, S., Waldor, M. K., and Misra, H. S. (2014). PprA contributes to Deinococcus radiodurans resistance to nalidixic acid, genome maintenance after DNA damage and interacts with deinococcal topoisomerases. PLoS ONE 9:e85288. doi: 10.1371/journal.pone.0085288
Kota, S., and Misra, H. S. (2006). PprA: a protein implicated in radioresistance of Deinococcus radiodurans stimulates catalase activity in Escherichia coli. Appl. Microbiol. Biotechnol. 72, 790–796. doi: 10.1007/s00253-006-0340-7
Lee, J., Jayaraman, A., and Wood, T. K. (2007a). Indole is an inter-species biofilm signal mediated by SdiA. BMC Microbiol. 7:42. doi: 10.1186/1471-2180-7-42
Lee, J., Page, R., García-Contreras, R., Palermino, J.-M., Zhang, X.-S., Doshi, O., et al. (2007b). Structure and function of the Escherichia coli protein YmgB: a protein critical for biofilm formation and acid-resistance. J. Mol. Biol. 373, 11–26. doi: 10.1016/j.jmb.2007.07.037
Lee, J.-Y., Lee, H. J., Seo, J., Kim, E.-S., Lee, H.-S., and Kim, P. (2014). Artificial oxidative stress-tolerant Corynebacterium glutamicum. AMB Expr. 4, 15. doi: 10.1186/s13568-014-0015-1
Lee, M. H., Moo, Y. R., Chung, B. Y., Kim, J.-S., Lee, K.-S., Cho, J.-Y., et al. (2009). Practical use of chemical probes for reactive oxygen species produced in biological systems by γ-irradiation. Radiat. Phys. Chem. 78, 323–327. doi: 10.1016/j.radphyschem.2009.03.001
Lennen, R. M., and Herrgård, M. J. (2014). Combinatorial strategies for improving multiple-stress resistance in industrially relevant Escherichia coli strains. Appl. Environ. Microbiol. 80, 6223–6242. doi: 10.1128/AEM.01542-14
Li, Q., Harvey, L. M., and McNeil, B. (2009). Oxidative stress in industrial fungi. Crit. Rev. Biotechnol. 29, 199–213. doi: 10.1080/07388550903004795
Lin, Z., Zhang, Y., and Wang, J. (2013). Engineering of transcriptional regulators enhances microbial stress tolerance. Biotechnol. Adv. 31, 986–991. doi: 10.1016/j.biotechadv.2013.02.010
Lisher, J. P., and Giedroc, D. P. (2013). Manganese acquisition and homeostasis at the host-pathogen interface. Front. Cell. Infect. Microbiol. 3:91. doi: 10.3389/fcimb.2013.00091
Lu, H., Gao, G., Xu, G., Fan, L., Yin, L., Shen, B., et al. (2009). Deinococcus radiodurans PprI switches on DNA damage response and cellular survival networks after radiation damage. Mol. Cell. Proteomics 8, 481–494. doi: 10.1074/mcp.M800123-MCP200
Lu, J., and Holmgren, A. (2014). The thioredoxin antioxidant system. Free Radic. Biol. Med. 66, 75–87. doi: 10.1016/j.freeradbiomed.2013.07.036
Lund, P., Tramonti, A., and De Biase, D. (2014). Coping with low pH: molecular strategies in neutralophilic bacteria. FEMS Microbiol. Rev. 38, 1091–1125. doi: 10.1111/1574-6976.12076
Ma, R., Zhang, Y., Hong, H., Lu, W., Lin, M., Chen, M., et al. (2011). Improved osmotic tolerance and ethanol production of ethanologenic Escherichia coli by IrrE, a global regulator of radiation-resistance of Deinococcus radiodurans. Curr. Microbiol. 62, 659–664. doi: 10.1007/s00284-010-9759-2
Makino, T., Skretas, G., and Georgiou, G. (2011). Strain engineering for improved expression of recombinant proteins in bacteria. Microb. Cell. Fact. 10:32. doi: 10.1186/1475-2859-10-32
Mancini, S., and Imlay, J. A. (2015). The induction of two biosynthetic enzymes helps Escherichia coli sustain heme synthesis and activate catalase during hydrogen peroxide stress. Mol. Microbiol. 96, 744–763. doi: 10.1111/mmi.12967
Mandal, T., Maity, S., Dasgupta, D., and Datta, S. (2010). Advanced oxidation process and biotreatment: their roles in combined industrial wastewater treatment. Desalination 250, 87–94. doi: 10.1016/j.desal.2009.04.012
Meenatchisundaram, S., Devaraj, M., Rai, C. L., and Nadarajan, K. M. (2014). An integrated approach for enhanced textile dye degradation by pre-treatment combined biodegradation. Clean Techn. Environ. Policy 16, 501–511. doi: 10.1007/s10098-013-0649-8
Munteanu, A.-C., Uivarosi, V., and Andries, A. (2015). Recent progress in understanding the molecular mechanisms of radioresistance in Deinococcus bacteria. Extremophiles 19, 707–719. doi: 10.1007/s00792-015-0759-9
Narumi, I., Satoh, K., Cui, S., Funayama, T., Kitayama, S., and Watanabe, H. (2004). PprA: a novel protein from Deinococcus radiodurans that stimulates DNA ligation. Mol. Microbiol. 54, 278–285. doi: 10.1111/j.1365-2958.2004.04272.x
Ohba, H., Satoh, K., Sghaier, H., Yanagisawa, T., and Narumi, I. (2009). Identification of PprM: a modulator of the PprI-dependent DNA damage response in Deinococcus radiodurans. Extremophiles 13, 471–479. doi: 10.1007/s00792-009-0232-8
Pan, J., Wang, J., Zhou, Z., Yan, Y., Zhang, W., Lu, W., et al. (2009). IrrE, a global regulator of extreme radiation resistance in Deinococcus radiodurans, enhances salt tolerance in Escherichia coli and Brassica napus. PLoS ONE 4:e4422. doi: 10.1371/journal.pone.0004422
Patzer, S. I., and Hantke, K. (2001). Dual repression by Fe2+-Fur and Mn2+-MntR of the mntH gene, encoding an NRAMP-like Mn2+ transporter in Escherichia coli. J. Bacteriol. 183, 4806–4813. doi: 10.1128/JB.183.16.4806-4813.2001
Phadtare, S., Tadigotla, V., Shin, W. H., Sengupta, A., and Severinov, K. (2006). Analysis of Escherichia coli global gene expression profiles in response to overexpression and deletion of CspC and CspE. J. Bacteriol. 188, 2521–2527. doi: 10.1128/JB.188.7.2521-2527.2006
Pomposiello, P. J., and Demple, B. (2001). Redox-operated genetic switches: the SoxR and OxyR transcription factors. Trends Biotechnol. 19, 109–114. doi: 10.1016/S0167-7799(00)01542-0
Ramteke, L. P., and Gogate, P. R. (2016). Treatment of real industrial wastewater using the combined approach of advanced oxidation followed by aerobic oxidation. Envrion. Sci. Pollut. Res. 23, 9712–9729. doi: 10.1007/s11356-016-6156-9
Rutherford, B. J., Dahl, R. H., Price, R. E., Szmidt, H. L., Benke, P. I., Mukhopadhyay, A., et al. (2010). Functional genomic study of exogenous n-butanol stress in Escherichia coli. Appl. Environ. Microbiol. 76, 1935–1945. doi: 10.1128/AEM.02323-09
Selvam, K., Duncan, J. R., Tanaka, M., and Battista, J. R. (2013). DdrA, DdrD, and PprA: components of UV and mitomycin C resistance in Deinococcus radiodurans R1. PLoS ONE 8:e69007. doi: 10.1371/journal.pone.0069007
Seo, S. W., Kim, D., Latif, H., O'Brien, E. J., Szubin, R., and Palsson, B. O. (2014). Deciphering Fur transcriptional regulatory network highlights its complex role beyond iron metabolism in Escherichia coli. Nat. Commun. 5, 4910. doi: 10.1038/ncomms5910
Singh, H., Appukuttan, D., and Lim, S. (2014). Hsp20, a small heat shock protein of Deinococcus radiodurans, confers tolerance to hydrogen peroxide in Escherichia coli. J. Microbiol. Biotechnol. 24, 1118–1122. doi: 10.4014/jmb.1403.03006
Slade, D., and Radman, M. (2011). Oxidative stress resistance in Deinococcus radiodurans. Microbiol. Mol. Biol. Rev. 75, 133–191. doi: 10.1128/MMBR.00015-10
Son, G., Kim, D.-H., Lee, J. S., and Lee, H. (2016). Enhanced methylene blue oxidative removal by copper electrode-based plasma irradiation with the addition of hydrogen peroxide. Chemosphere 157, 271–275. doi: 10.1016/j.chemosphere.2016.05.005
Tschowri, N., Busse, S., and Hengge, R. (2009). The BLUF-EAL protein YcgF acts as a direct anti-repressor in a blue-light response of Escherichia coli. Genes Dev. 23, 522–534. doi: 10.1101/gad.499409
Tschowri, N., Lindenberg, S., and Hengge, R. (2012). Molecular function and potential evolution of the biofilm-modulating blue light-signalling pathway of Escherichia coli. Mol. Microbiol. 85, 893–906. doi: 10.1111/j.1365-2958.2012.08147.x
Weber, H., Polen, T., Heuveling, J., Wendisch, V. F., and Hengge, R. (2005). Genome-wide analysis of the general stress response network in Escherichia coli: σS-dependent genes, promoters, and sigma factor selectivity. J. Bacteriol. 187, 1591–1603. doi: 10.1128/JB.187.5.1591-1603.2005
Zhao, H., Li, J., Han, B., Li, X., and Chen, J. (2014). Improvement oxidative stress tolerance in Saccharomyces cerevisiae through global transcription machinery engineering. J. Ind. Microbiol. Biotechnol. 41, 869–878. doi: 10.1007/s10295-014-1421-8
Zheng, M., Wang, X., Templeton, L. J., Smulski, D. R., LaRossa, R. A., and Storz, G. (2001). DNA microarray-mediated transcriptional profiling of the Escherichia coli response to hydrogen peroxide. J. Bacteriol. 183, 4562–4570. doi: 10.1128/JB.183.15.4562-4570.2001
Zhu, L., Cai, Z., Zhang, Y., and Li, Y. (2014). Engineering stress tolerance of Escherichia coli by stress-induced mutagenesis (SIM)-based adaptive evolution. Biotech. J. 9, 120–127. doi: 10.1002/biot.201300277
Keywords: Deinococcus radiodurans, PprM, Escherichia coli, oxidative stress tolerance, MntH (proton-dependent Mn transporter), ycgA-ymgABC operon
Citation: Park S-H, Singh H, Appukuttan D, Jeong S, Choi YJ, Jung J-H, Narumi I and Lim S (2017) PprM, a Cold Shock Domain-Containing Protein from Deinococcus radiodurans, Confers Oxidative Stress Tolerance to Escherichia coli. Front. Microbiol. 7:2124. doi: 10.3389/fmicb.2016.02124
Received: 30 June 2016; Accepted: 15 December 2016;
Published: 10 January 2017.
Edited by:
Thomas E. Hanson, University of Delaware, USAReviewed by:
Steffen P. Graether, University of Guelph, CanadaJohn R. Battista, Louisiana State University, USA
Copyright © 2017 Park, Singh, Appukuttan, Jeong, Choi, Jung, Narumi and Lim. This is an open-access article distributed under the terms of the Creative Commons Attribution License (CC BY). The use, distribution or reproduction in other forums is permitted, provided the original author(s) or licensor are credited and that the original publication in this journal is cited, in accordance with accepted academic practice. No use, distribution or reproduction is permitted which does not comply with these terms.
*Correspondence: Sangyong Lim, c2F5bGltQGthZXJpLnJlLmty
†Present Address: Harinder Singh, Biological Sciences, SD School of Science, NMIMS University, Mumbai, India
‡These authors have contributed equally to this work.