- 1Institut de Recherches Cliniques de Montréal (IRCM), RNA Biology Department, Montreal, QC, Canada
- 2Département de Biochimie, Université de Montréal, Montreal, QC, Canada
- 3Divison of Experimental Medicine, McGill University, Montreal, QC, Canada
In the evolutionary arms race, symbionts have evolved means to modulate each other's physiology, oftentimes through the dissemination of biological signals. Beyond small molecules and proteins, recent evidence shows that small RNA molecules are transferred between organisms and transmit functional RNA interference signals across biological species. However, the mechanisms through which specific RNAs involved in cross-species communication are sorted for secretion and protected from degradation in the environment remain largely enigmatic. Over the last decade, extracellular vesicles have emerged as prominent vehicles of biological signals. They can stabilize specific RNA transcripts in biological fluids and selectively deliver them to recipient cells. Here, we review examples of small RNA transfers between plants and bacterial, fungal, and animal symbionts. We also discuss the transmission of RNA interference signals from intestinal cells to populations of the gut microbiota, along with its roles in intestinal homeostasis. We suggest that extracellular vesicles may contribute to inter-species crosstalk mediated by small RNA. We review the mechanisms of RNA sorting to extracellular vesicles and evaluate their relevance in cross-species communication by discussing conservation, stability, stoichiometry, and co-occurrence of vesicles with alternative communication vehicles.
Introduction
The “one gene, one enzyme” paradigm has long dominated our understanding of molecular biology. Although peptides are key effectors of cell physiology, strictly protein-centrist portraits of life have encountered early criticism and been deemed reductive since the 1950s (Mc, 1950). In the post-genomic era, it has become increasingly clear that the bulk of eukaryotic genomes—loci previously dubbed “junk DNA” or “dark matter”—undergo pervasive transcription, yielding thousands of non-coding (nc)RNAs, many of which are conserved and tissue-specific (Clark et al., 2011; Coffey et al., 2011; Derrien et al., 2012; Jalali et al., 2016). In particular, small non-coding (s)RNAs transcribed from intergenic, intronic, and repeated regions can exert RNA interference (RNAi) by guiding Argonaute ribonucleases (RNAses) to specific complementary targets (Hammond et al., 2001). The pivotal role of sRNA in a broad range of biological contexts is well-established: estimates suggest that up to 60% of mammalian mRNA is subjected to RNAi by sRNA (Lewis et al., 2005).
Symbiotic relationships favor the intricate proximity of multiple species in biological niches. To sustain the evolutionary arms race, symbionts have evolved means to influence each other via secreted signals. In line with the “one gene, one enzyme” paradigm, communication across species was long taught to strictly involve peptides and small metabolites. Over the last decade, however, reports of communication across species via transfers of RNA silencing signals have surged in diverse biological niches, prompting a re-evaluation of cross-species communication (Knip et al., 2014; Weiberg et al., 2015).
Naked RNA transcripts are rapidly degraded in the human systemic circulation (Tsui et al., 2002) but extracellular vesicles (EVs), ribonucleoprotein complexes (RNPs) and lipoproteins can stabilize transcripts and protect them from RNAse degradation (Valadi et al., 2007; Hunter et al., 2008; Lasser et al., 2011; Lefebvre et al., 2016). Microarray and deep-sequencing approaches have revealed that repertoires of secreted sRNA don't mirror cellular populations, suggesting the involvement of selective sorting mechanisms (Valadi et al., 2007; Hunter et al., 2008; Lasser et al., 2011; Lefebvre et al., 2016). Various proteins of the RNAi machinery have been found in mammalian EVs and can perform cell-independent sRNA maturation (Melo et al., 2014). In addition, RNA silencing activity can be transferred across tissues, with emerging implications in early development (da Silveira et al., 2015; Sharma et al., 2016), cancer biology (Melo et al., 2014; Dror et al., 2016), immunology (Montecalvo et al., 2012), regenerative medicine (Hergenreider et al., 2012), and gene therapy (Mizrak et al., 2013). Recent reports show that EVs enable inter-organismal and long-range transfers of functional RNA and proteins in C. elegans (Wang et al., 2014) and in mammals (Viss et al., 2003), suggesting that EVs may contribute to cross-species transfer of RNA silencing activity.
Here, we survey the properties of eukaryotic sRNA and review emerging evidence of inter-organismal RNAi across species and kingdoms, including crosstalk between plants, bacterial, fungal, and metazoan pathogens and host-microbiota interactions in the gut. We discuss the mechanisms of RNA sorting to mammalian EVs for secretion. We suggest that sRNA-loaded EVs may contribute to cross-species RNAi activity. To put this hypothesis in perspective, we discuss contrasting evidence challenging the efficiency of EV-mediated sRNA transfer in light of deficient stability and stoichiometry.
Overview of Gene Silencing by sRNA
Small RNAs have three defining features: (1) they are short (21–31 nucleotides), (2) don't encode peptides, and (3) can associate with RNAses of the Argonaute family (AGO) to modulate gene expression by targeting complementary transcripts. sRNA can impact gene expression through at least four distinct mechanisms: (1) AGO-dependent cleavage of target RNA, (2) destabilization of target mRNA through polyA tail shortening, (3) translational inhibition via polysomal protein interactions, and (4) transcriptional silencing through chromatin modifications (Volpe et al., 2002; Ghildiyal and Zamore, 2009; Rissland and Lai, 2011). The field of RNAi truly emerged in the late 1990s, after the discovery that double-stranded (ds) RNA can specifically silence complementary transcripts in C. elegans (Fire et al., 1998). An expanding repertoire of sRNA classes has since surfaced, including microRNA (miRNA), endogenous small interfering RNA (endo-siRNA), and Piwi-interacting RNA (piRNA). In addition, infrastructural non-coding RNA, such as transfer RNA (tRNA) and vaultRNA (vtRNA) can be processed and recognized by the RNAi machinery to silence diverse mRNA targets (Persson et al., 2009; Sharma et al., 2016). The protein machinery involved in sRNA nuclear export, maturation and RNAi is highly conserved and was likely present in the last common ancestor of eukaryotes (Shabalina and Koonin, 2008), emphasizing the potential functionality of RNAi in cross-species communication. The biogenesis of sRNA in mammals has been clarified over the last decade (Lee et al., 2004; Forstemann et al., 2005; Yeom et al., 2006) and reviewed in detail elsewhere (Mattick and Makunin, 2005; Collins and Cheng, 2006; Ghildiyal and Zamore, 2009; Rissland and Lai, 2011; Figure 1).
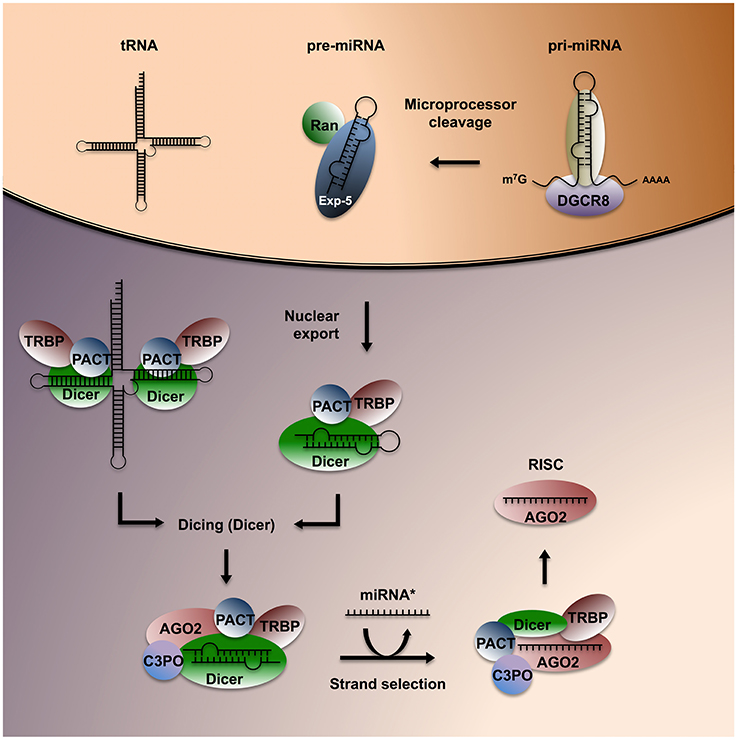
Figure 1. Overview of sRNA biogenesis in mammals. Pri-miRNA is cleaved into pre-miRNA by the microprocessor complex, consisting of two nuclear proteins, Drosha and its cofactor DGCR8. Pre-miRNA is exported to the cytoplasm through Exportin-5 (Exp-5), then bound and processed into short dsRNA sequences of ~22 nt by the RBP Dicer and its associated factors TRBP and PACT. Structured ncRNA encompassing stretches of paired nucleotides such as tRNAs can also be recognized and processed as Dicer substrates. Dicer recruits AGO2 and its cleavage yields two single-stranded RNA sequences, called the leading strand and the guide strand (or miRNA*). The leading strand is actively repositioned in the complex and loaded onto AGO2 to form a RISC, which can exert RNA silencing.
Most miRNAs are transcribed from intergenic or intronic regions, with a few examples derived from exons of protein-coding genes. Hairpins found on primary (pri)-miRNA transcripts (≈1,000 nt) bind to the nuclear microprocessor complex consisting of the RNAse III enzyme Drosha and the RBP DGCR8/Pasha. The microprocessor cleaves pri-miRNAs into precursor (pre)-miRNAs (≈70 nt), which undergo nuclear export via Exportin-5 (Yi et al., 2003). Pre-miRNA and long dsRNA sequences are recognized by Dicer and subsequently cleaved (“diced”) to generate sRNA duplexes (≈22 nt). Recent evidence suggests that structured dsRNA regions of tRNA and vtRNA can also be recognized and processed by Dicer, yielding small sequences similar to a mature miRNA (Persson et al., 2009). Argonaute 2 (AGO2) is recruited to the complex by the Dicer-binding protein TRBP, enabling the transfer of the leading (or guide) RNA strand to the PAZ domain of AGO2. TRBP, PACT and C3PO are involved in leading strand selection and re-positioning (Noland et al., 2011; Noland and Doudna, 2013). They also clear the remaining complementary single-stranded RNA copy, the passenger strand, or miRNA*, which is typically targeted for degradation (Liu et al., 2009). The resulting minimal RNA-induced silencing complex (RISC) consists of AGO2 bound to the mature miRNA.
RNAi in Crosstalk between Plants, Bacteria, Fungi, Insects and Nematodes
Plants rely on RNAi for various endogenous processes, including defense against viral parasites. The genome of Arabidopsis thaliana encodes over 10 different AGO proteins, presumably reflecting the emergence of new functions (Zhang et al., 2015). In 1990, Napoli et al. reported an unexpected block in anthocyanin biosynthesis upon the introduction of a chimeric chalcone synthase construct in petunia (Napoli et al., 1990). The resulting crop exhibited white and patterned flowers presenting pale non-clonal sectors on a pigmented wild type (WT) background. The mechanism involved was deemed unclear at the time and only became apparent after the realization that dsRNA expression leads to gene silencing (Fire et al., 1998). More recently, engineering of plant RNAi has been described as an effective and “eco-friendly” method to modulate crop phenotypes in the aim of increasing productivity (Younis et al., 2014). In particular, host gene silencing–hairpin RNAi (HGS-hpRNAi), wherein a transgenic sRNA-encoding hairpin is expressed in plants has emerged over the last 15 years to impact pathogen resistance (Viss et al., 2003). HGS-hpRNAi is reportedly effective against diverse pathogens, including bacteria (e.g., Agrobacterium), fungi (e.g., Fusarium), insects (e.g., Helicoverpa) and nematodes (e.g., Meloidogyne; Table 1). However, the mechanisms through which plant-encoded dsRNA and/or sRNA molecules are transferred to symbiotic neighbors remain largely unclear and could involve EVs, RNPs and/or lipoproteins.
The soil bacterium Agrobacterium tumefaciens causes crown gall disease through disruptions of host's auxin and cytokinin biosynthesis, leading to the formation of tumor in various species of Eudicotidae flowering plants (Hoekema et al., 1983). The pathogenesis of Agrobacterium crown gall disease is well- characterized and involves the bacterial Tumor-inducing (Ti) plasmid (McCullen and Binns, 2006). Ti ssDNA is trafficked from the bacterium to the host plant via a conjugation pilus (McCullen and Binns, 2006), which is a prevalent vehicle of nucleic acid exchange among bacteria. Whether the bacterial conjugation pilus can enable host-encoded sRNA populations to enter Agrobacterium remains unclear. Ti is integrated in the host genome by recombination and encodes opine synthesis oncogenes (iaaM and ipt). Escobar et al. reported resistance to crown gall tumorigenesis in transgenic Arabidopsis expressing self-complementary constructions designed to initiate HGS-hpRNAi against iaaM and ipt (Escobar et al., 2001). This finding was later confirmed in apple (Malus pumila) and walnut (Juglans regia) trees (Escobar et al., 2002; Viss et al., 2003).
Ascomycete pathogens of the Fusarium genus release mycotoxins and cause “root rot” and Fusarium head blight pathologies, leading annually to severe loss in cereal crop productions. Koch et al. showed that A. thaliana and Hordeum vulgare (barley) plants expressing dsRNA targeting the fungal gene CYP51 were completely immune to Fusarium graminearum (Koch et al., 2013; Figure 2). In transgenic plants, fungal growth was strongly restricted (≥99%) and only present in the vicinity of inoculation sites. The authors reported increased sporulation and altered morphology of Fusarium exposed to transgenic plants, consistent with compromised levels of cytochrome P450 lanosterol C-14α-demethylase, the enzyme encoded by CYP51. Similarly, Ghag et al. achieved effective resistance to Fusarium oxysporum cubense in transgenic bananas (Musa paradisiaca) expressing hairpins to target two vital fungal genes, velvet and Fusarium transcription factor 1 (Ghag et al., 2014). Additional demonstrations of HGS-hpRNAi from plants to fungi have involved the mutualistic mycorrihzal genus Glomus. Helber et al. characterized the Monosaccharide Transporter2 (MST2) gene in Glomus species and provided evidence of its requirement for mycorrhiza formation through a HGS-hpRNAi loss-of-function model (Helber et al., 2011). A similar approach was used by Vega-Arreguin et al. to demonstrate the role of the fungal gene Avr3a1 in the hypersensitive response exhibited by diverse species of the genus Nicotiana to the pathogenic oomycete Phytophthora capsici (Vega-Arreguin et al., 2014).
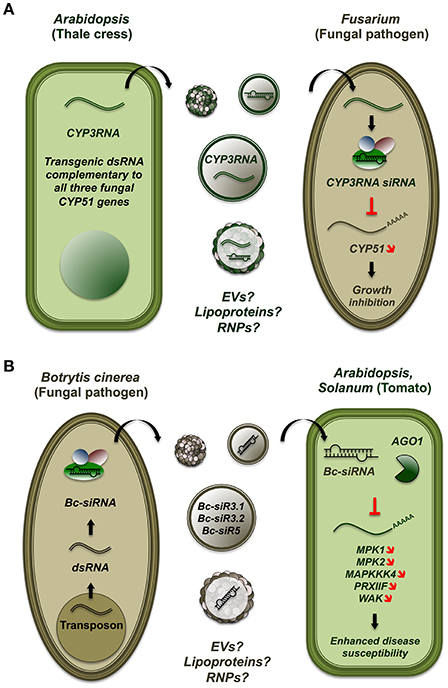
Figure 2. Host-induced hairpin RNA-mediated silencing confers resistance to the fungal pathogen Fusarium. (A) Host-induced hairpin RNA-mediated silencing enables plant to resist to the fungal pathogen Fusarium. In Arabidopsis, expression of a dsRNA construct complementary to fungal CYP51 transcripts can immunize transgenic plants to the pathogenic ascomycete Fusarium graminearum by inhibiting fungal growth (Koch et al., 2013). The vehicles through which transgenic dsRNA and/or sRNA is transferred are unknown and possibly include plant EVs, secreted RNPs and/or lipoproteins. (B) Botrytis cinerea sRNA populations hijack Arabidopsis RNAi pathways to suppress plant immunity. Populations of sRNA derived from a B. cinerea retrotransposon are shuttled to infected Arabidopsis and Solanum lycopersicum. In plants, fungal sRNA are loaded onto AGO1 and direct the silencing of diverse proteins, including Mitogen-activated kinases, which impact the host's immune response (Weiberg et al., 2013). The vehicles through which dsRNA and/or sRNA are transferred from fungus to plant are unknown and possibly include fungal EVs, secreted RNPs and/or lipoproteins.
Nematodes are appealing models to study RNAi and HGS-hpRNAi. In the nematode C. elegans, where RNAi was discovered (Fire et al., 1998), sRNA induces a systemic, amplified, and heritable response (Collins and Cheng, 2006). Strong evidence indicates that dsRNA expressed in E. coli fed to C. elegans can transmit systemic and heritable silencing activity upon ingestion (Liu et al., 2012). In C. elegans, the intestinal transmembrane protein SID-1 binds and imports dsRNA (Jose et al., 2009), while SID-2 is associated with cellular export of RNAi signals and required for systemic environmental RNAi (Winston et al., 2007). Parasitic nematodes of the Meloidogyne genus are soilborne root pathogens that feed on diverse plants, notably potato (Solanum tuberosum) and soybean (Glycine max). Meloidogyne damages roots and compromises the plant's ability to absorb water and nutrients, affecting crop productivity (Abad et al., 2008). Dinh et al. showed that Arabidopsis and potato plants expressing dsRNA constructs that target the nematode gene 16D10L develop resistance to Meloidogyne chitwoodi. Interestingly, RNAi against 16D10L was transferred to the progeny of worms feeding on transgenic roots, consistent with reports of heritable RNAi in nematodes (Dinh et al., 2014). In addition, Ibrahim et al. assessed the efficiency of HGS-hpRNAi at reducing galls formed by Meloidogyne incognita in soybean roots. The authors tested four potential targets in M. incognita and reported that HGS-hpRNAi against transcripts encoding Tyrosine Phosphatase (TP) and Mitochondrial Stress-70 Protein Pre-cursor (MSP) were highly efficient at reducing galls in infected plants (Ibrahim et al., 2011).
Like nematodes, several arthropods can internalize dietary dsRNA molecules (Khila and Grbic, 2007). Indeed, orthologs of the sid-1 gene have been identified in several insects, including Apis mellifera (honeybee), Bombyx mori (silkworm), and Tribolium castaneum (red flour beetle; Gramates, 2006). In light of these findings, Baum et al. provided a general assessment of HGS-hpRNAi usefulness for the control of coleopteran insects. Baum et al. showed that the western corn rootworm Diabrotica virgifera virgifera Leconte is sensitive to orally provided dsRNA, exhibiting dramatic suppression of 17 endogenous targets within 24 h of ingestion (Baum et al., 2007). The authors demonstrated the potential of oral dsRNA delivery for insect pest control by determining the lethal dose of sequences targeting diverse protein-coding genes. Among the 290 dsRNA tested, 125 showed significant larval mortality. In a HGS-hpRNAi assay, maize plants expressing dsRNA targeting coleopteran v-ATPse A were protected from Diabrotica feeding damage. Thakur et al. and Mutti et al. provided additional evidence that expression of dsRNA targeting insect genes can improve crop resistance in various models (Mutti et al., 2006; Thakur et al., 2014). Multiple plants release secondary metabolites or phytochemicals that promote resistance to parasites. For example, cotton plants (Gossypium genus) synthesize gossypol, a toxic sesquiterpene compound that detracts most herbivores (Mao et al., 2007). However, the cotton bollworm, Helicoverpa armigera, tolerates high concentrations of gossypol. Mao et al. showed that a cytochrome P450 gene, CYP6AE14, is induced by gossypol and required for insect tolerance to the compound (Mao et al., 2007). When fed Arabidposis or Nicotiana plant material expressing a dsRNA construct raised against CYP6AE14, the sensitivity of Helicoverpa larvae to gossypol was markedly increased.
Recent evidence suggests that fungal pathogens can transfer RNAi signals to modulate the immunity of the plants they parasite. Indeed, Weiberg et al. reported that Botyris cinerea, the causative agent of gray mold disease, encodes sRNA populations derived from retrotransposons which can silence Arabidopsis and Solanum genes involved in immunity (Weiberg et al., 2013; Figure 2). In this study, Weiberg et al. generated sRNA sequencing libraries from B. cinerea mycelia, conidiospores and total biomass. In parallel, they profiled leaves from B. cinerea-infected Arabidopsis and tomato plants (Solanum lycopersicum). Interestingly, a total of 832 B. cinerea-encoded sRNAs were tracked and overrepresented in infected plant extracts, 52 of which mapped to six different fungal long terminal repeat (LTR) retrotransposons. Among predicted Arabidopsis and Solanum targets, reporter assays confirmed mitogen activated protein kinases (MPK1, MPK2, MAPKKK4), peroxiredoxin (PRXIIF), and cell-wall associated kinase (WAK). Transgenic Arabidopsis plants ectopically expressing three B. cinerea sRNAs (Bc-siRNA3.1, Bc-siRNA3.2, Bc-siRNA5) displayed normal morphology but enhanced disease susceptibility upon pathogen challenge. A consistent phenotype was observed in mpk1 mpk2 double mutants, suggesting that these factors are involved in host immunity against B. cinerea. Immunoprecipitation of AGO1 in B. cinerea-infected Arabidopsis retrieved Bc-siRNA3.1, Bc-siRNA3.2 and Bc-siRNA5. Arabidopsis mutants of Ago1 (ago1-27) showed reduced disease susceptibility to B. cinerea, whereas the Dicer-like mutant dcl1-7 displayed enhanced disease phenotype. By contrast, knock out of B. cinerea dcl genes depleted sRNA pools and reduced virulence upon Arabidopsis and Solanum inoculation. Together, these results show that fungal Dicer and plant Ago1 are involved in Bc-siRNA3.1-, Bc-siRNA3.2-, and Bc-siRNA5-induced gene silencing in Arabidopsis. Weiberg et al. thus unraveled a mechanism whereby fungal sRNA populations hijack the plant host's RNAi machinery to subvert plant immunity and promote disease progression.
RNAi in Crosstalk between Intestinal Cells and the Gut Microbiota
With over 100 trillion organisms representing 1,000 species, the gut microbiota plays pivotal roles in human health and disease (Ley et al., 2006; Faith et al., 2013). Inflammatory bowel disease (Halfvarson et al., 2008), diabetes (Patterson et al., 2016), obesity (Tilg et al., 2009; Nehra et al., 2016), diverse malignancies (Louis et al., 2014; 2012), and neurological disorders (Moos et al., 2016) have been linked to disruptions in intestinal homeostasis. Several studies have reported increased parasite susceptibility in mice bearing a conditional Dicer deletion in intestinal epithelial cells (Dicer1Δgut), suggesting that RNAi is involved in mucosal immunity, possibly affecting communication with gut microorganisms.
Singh et al. identified 16 miRNA transcripts differentially expressed in caecum samples from germ-free and conventionally fed mice. Computational approaches pointed to over 2,000 putative mRNA targets, including factors involved in intestinal barrier function and immune regulation (Singh et al., 2012). The authors found a strong overlap between their list of target mRNA and a previous survey of factors deregulated in the mucosa of Dicer1Δgut mice. This observation suggests that the gut microbiota modulates miRNA expression in the host, impacting intestinal barrier integrity. Along with several other studies (Dalmasso et al., 2011; Dai et al., 2015; Runtsch et al., 2015), Singh et al. provides evidence that RNAi modulates crosstalk between gut epithelial cells and the microorganisms that surround them. However, the prevalence and relevance of direct transfers of sRNA molecules from host to gut microbiota remain unclear. In a recent study, Liu et al. contributes to bridge that gap, showing that commensal gut bacteria uptake host miRNAs secreted in feces and that host miRNAs can exert RNAi in E. coli and F. nucleatum (Liu et al., 2016; Figure 3). Liu et al. (2016) isolated and characterized miRNA populations contained within EVs in human and mice fecal samples. They identified over 180 miRNA in feces, which were differentially distributed in gut luminal content from the distal ileum and colon of mice. They show that intestinal epithelial cells, Paneth cells, and goblet cells all contribute miRNA transcripts that account for fecal populations.
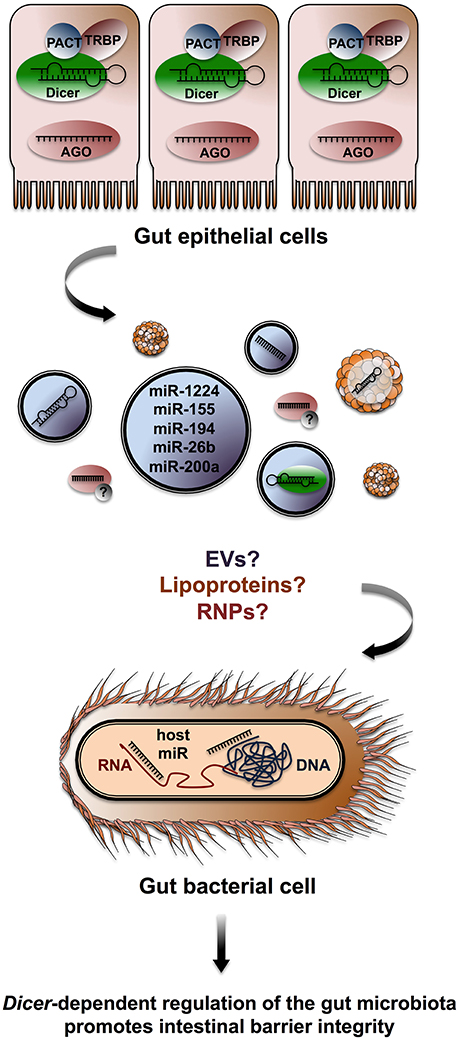
Figure 3. Host miRNA targets microbiota gene expression. Gut epithelial cells release miRNAs that can be recovered in murine and human fecal matter. Fecal miRNA populations are likely stabilized through EVs and possibly through lipoproteins or RNPs containing AGO2. Host miRNA enters E. coli and F. nucleatum where it co-localizes with bacterial nucleic acids and impacts bacterial growth by interacting with nucleic acids (Liu et al., 2016).
Liu et al. (2016) compared the gut microbiota in fecal matter from Dicer1Δgut and control (Dicer1fl/fl) mice by sequencing the V4 region of rRNA 16S. Several differences were noted: representation of the bacterial phyla Firmicutes and Proteobacteria was notably increased in Dicer1Δgut samples. Liu et al. (2016) then submitted seven abundant bacterial RNA sequences from E. coli and F. nucleatum to a miRBase analysis (Kozomara and Griffiths-Jones, 2014) and identified numerous putative base-pairing events with host miRNA. Synthesized miRNA mimics of hsa-miR-1226-5p promoted the growth of E. coli, while hsa-miR-515-5p favored F. nucleatum in vitro. Mutated controls preventing base pairing of miRNA mimics to bacterial targets had no impact. Fluorescent Cy3-conjugated miRNA entered E. coli and F. nucleatum, co-localized with nucleic acids and increased the 16S rRNA/23S rRNA ratio in F. nucleatum. In E. coli, RNAseP levels were increased by miR-4747-3p while the bacterial transcripts rutA and fucO levels were decreased by miR-1224-p and miR-623, respectively. Target levels where not affected by mice miRNA mimics bearing mutations in predicted base-pairing nucleotides. Fecal gavage of Dicer1Δgut mice with WT samples led to a restoration of WT microbiota populations after 7 days, as determined by 16S rRNA sequencing.
Next, Liu et al. (2016) investigated the phenotype of Dicer1Δgut mice and found evidence of reduced MHCII levels in intestinal lymphoid tissue inducer cells. Diverse cytokines were also decreased in the ileum and colon, including LT-β, IFN-γ, and TGF-β. The resistin-like molecules Relm-α and Relm-β, which are critical for maintenance of intestinal barrier integrity, were compromised in the ileum of Dicer1Δgut mice, along with Occludin-1, ZO-1, and Claudin-1, -2, and -5, echoing previous reports of Dicer1Δgut phenotypes (Braniste et al., 2014). Based on these findings, the authors suspected increased susceptibility to colitis in Dicer1Δgut mice, and tested the hypothesis by inducing the disease through oral administration of dextran sulfate sodium. As expected, Dicer1Δgut mice exhibited greater body weight loss, colon shortening, and colon infiltration in response to dextran sulfate than WT mice. However, gavage of Dicer1Δgut mice with fecal matter from WT mice prior to dextran sulfate treatment alleviated the severity of these phenotypes, suggesting that fecal miRNA can attenuate the colonic alterations seen in Dicer1Δgut mice.
Together, these observations strongly suggest that host miRNA can be internalized, exert RNAi, and mediate compositional changes in gut bacterial populations to promote intestinal homeostasis. Although Liu et al. (2016) does not directly demonstrate that host miRNA populations are transferred via EVs, the study shows that sequences enriched in fecal EVs are involved in cross-kingdom RNAi. Interestingly, in mice, EVs have been identified as a communication vehicle between intestinal epithelial cells and the immune system enabling MHCII protein transfers (Van Niel et al., 2003).
Diverse Subpopulations of Secreted Vesicles Contain sRNA
In mammalian cells, the release of membranous vesicles upon exocytosis of vesicular endosomes was first reported in 1983 by Harding et al. (1983). Long dismissed as cellular debris, EVs have emerged over the last decade as key vehicles of biological signals, notably sRNA. Transcripts enriched in EVs include specific mRNA and full-length and fragmented non-coding transcripts, such as ribosomal (r)RNA, long non-coding (lnc)RNA, transfer (t)RNA, vault (vt)RNA, Y RNA, small nuclear (sn)RNA, and small nucleolar (sno)RNA populations (Kalra et al., 2012; Nolte-'t Hoen et al., 2012; Xiao et al., 2012; Li et al., 2013; Lefebvre et al., 2016). Viral transcripts have been identified in EVs of cells infected with Epstein-Barr virus (Pegtel et al., 2010; Nanbo et al., 2013). Although specific miRNAs are overrepresented in EVs, diverse studies indicate that cumulative miRNA abundance is lower in EVs than in cells (Chevillet et al., 2014; Koppers-Lalic et al., 2014).
The EV field have largely focussed on mammalian systems. In humans, EVs have notably been described as promising sources of biomarkers for diverse diseases (Skog et al., 2013). EVs and EV-associated RNA populations have been identified and profiled by RNA-seq in multiple human biological fluids, including blood (Mitchell et al., 2008; Huang et al., 2013), milk (Chen et al., 2014), semen (Vojtech et al., 2014), saliva (Michael et al., 2010), cerebral spinal fluid (Baraniskin et al., 2011), urine (Nilsson et al., 2009), and ascitic fluids (Kahlert and Kalluri, 2013). The release of exosome-like vesicles carrying sRNA populations has also been described in the nematode Caenorhabditis elegans, the arthropod Drosophila melanogaster (Lefebvre et al., 2016) and the unicellular fungi Cryptococcus neoformans, Paracoccidioides brasiliensis, Candida albicans, and Saccharomyces cerevisae (Peres da Silva et al., 2015). Similarly, specific populations of sRNA have been defined in protozoans of the Leishmania (Lambertz et al., 2015) and Trypanosoma (Fernandez-Calero et al., 2015) genera. Outer membrane vesicles released by Gram-negative bacteria, notably Vibrio cholera, have been shown to contain specific RNA populations and suggested to function as an RNA delivery system during infection. Release of MVE-associated exosomes in plants has been hypothesized 40 years ago (Halperin and Jensen, 1967) and is consistent with electron microscopy evidence (Tanchak and Fowke, 1987).
“EV” is an umbrella term referring to diverse subpopulations of membrane-enclosed vesicles, often co-purified together in protocols that involve sequential ultracentrifugation of biological fluids (Hill et al., 2013). Exosomes are small EVs (40–120 nm) that originate in endosomes and are released in the extracellular space upon exocytosis of multivesicular endosomes (MVEs). Vesicles shed by the plasma membrane through an actin-dependent abscission are typically larger (50–1,000 nm) and have been called microvesicles, ectosomes, or microparticles (Akers et al., 2013). Apoptotic cells release small vesicles (50–500 nm) in addition to large apoptotic bodies containing organelles (Ihara et al., 1998; Elmore, 2007; 50–5,000 nm). Membrane-enclosed particles with retroviral-like composition and morphology (90–100 nm) have also been identified in cancer cell media (Muster et al., 2003) and in plasma samples of lymphoma patients (Contreras-Galindo et al., 2008). Beyond EVs, sRNA can be stably shuttled in biological fluids in association with lipoproteins and RNP complexes, some containing AGO2 and Nucleophosmin-1 (NPM1). At least two studies suggest that extracellular AGO2 and miRNA are more abundant in soluble complexes than within EVs (Arroyo et al., 2011; Turchinovich et al., 2011). In addition, “tunneling nanotubes” are actin-rich protrusions that can bridge eukaryotic cells and may provide an alternative route for nucleic acid transfers (Belting and Wittrup, 2008). First described in 2004 in cultures of rat pheochromocytoma cells (Rustom et al., 2004), tunneling nanotubes have since been shown to enable transmission of HIV particles between T cells and Jurkat cells (Sowinski et al., 2008).
It is technically challenging to separate subpopulations of EVs. Kowal et al. developed a tailored approach to separate EV subpopulations using continuous density gradients and immuno-isolation (Kowal et al., 2016). Mass spectrometry revealed distinct but partially overlapping protein profiles in human exosomes, microvesicles, and apoptotic bodies. Other studies reported divergent nucleic acid imprints in EV subpopulations (Crescitelli et al., 2013; Lazaro-Ibanez et al., 2014). Crescitelli et al. found that ribosomal RNA are more abundant in apoptotic bodies and in microvesicles than in exosomes, which reportedly contain more RNA than microvesicles. However, Kanada et al. showed that microvesicles could target reporter molecules to recipient cells more efficiently than exosomes, which appeared ineffective at delivering nucleic acids (Kanada et al., 2015).
Mechanisms of sRNA Sorting to EVs
Our understanding of EVs in health and disease has expanded rapidly over the last years and has been frequently reviewed (Stoorvogel et al., 2002; Raposo and Stoorvogel, 2013). In this section, we will focus on the mechanisms involved in sorting sRNA to mammalian EVs (Figure 4). Spatially resolved subcellular targeting of RNA molecules is often mediated by sequence or structure motifs found in the transcript. Called cis-acting elements, these sequences specifically interact with trans-acting factors, usually RBPs (Martin and Ephrussi, 2009; Cody et al., 2013). At the subcellular level, mRNA localization is a prevalent process with key functional contributions, notably in embryogenesis (MacDonald, 1990; Lecuyer et al., 2007; Cody et al., 2013) and synaptogenesis (Latham et al., 1994; Czaplinski and Singer, 2006; Du et al., 2007). Batagov et al. (2011) extended the rationale of subcellular localization and submitted a list of EV-targeted transcripts inferred from microarray datasets (Skog et al., 2008) to motif search algorithms. Although multiple alignments and position-specific scoring approaches failed to identify shared signatures among EV RNA, the authors found that EV-enriched transcripts display significantly shorter half-lives than cell-retained transcripts. By contrast, Bolukbasi et al. identified a 25 nt motif in the 3′UTR of mRNAs enriched in EVs from glioblastoma and melanoma cell lines (Bolukbasi et al., 2012). Mutagenesis and reporter assays confirmed the functionality of the sequence at targeting mRNA to EVs. Interestingly, the authors found that the motif encompasses the seed region for miR-1289 along with a core CUGCC sequence. Furthermore, altering the levels of miR-1289 was sufficient to modulate artificial target levels in EVs, suggesting a role for miRNA in sorting complementary mRNA to EVs. It should be noted that the studies discussed above used microarray data focussed on mRNA and long non-coding RNA. Other sources have since emphasized the enrichment of shorter sequences in EVs, including specific miRNA (Bellingham et al., 2012). Hung and Leonard showed that RNA length alone strongly modulates targeting efficiency (Hung and Leonard, 2016). They developed a tailored approach based on the MS2-GFP system and confirmed that long sequences (1.5 kb) are poorly loaded in EVs.
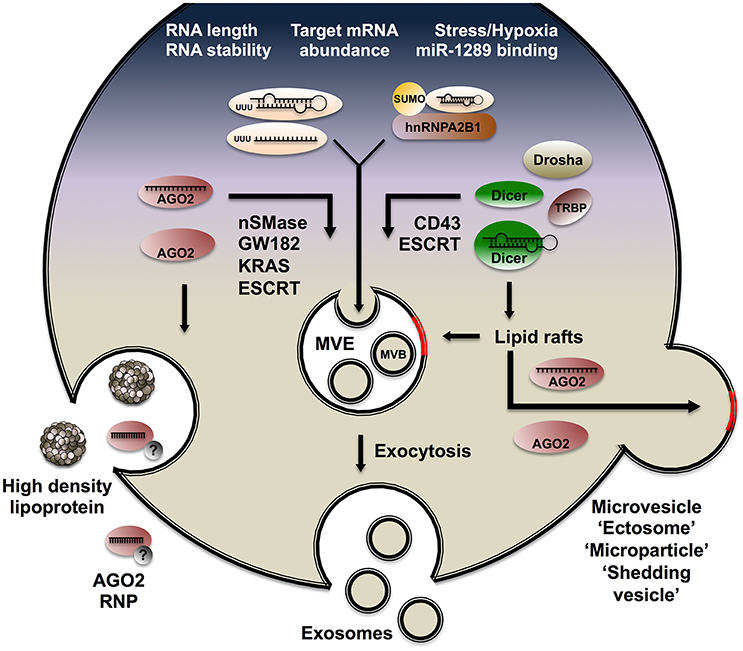
Figure 4. Mechanisms of sRNA loading to EVs. Schematic view of a mammalian cell releasing sRNA through lipoproteins and AGO2 RNPs (left), exosomes (center), and membrane-shed microvesicles (right). Properties broadly associated with RNA targeting to EVs are listed on top (white font). Mechanisms, lipid structures and RBPs involved in sorting RNA molecules to exosome and microvesicles are portrayed.
EV-associated miRNA repertoires exhibit considerable cell-type specificity and dramatic alterations in these populations have been observed upon cell fate commitment or changes in environmental status and stimuli. Hypoxic conditions have been shown to increase exosomal release and modulate associated miRNA in breast cancer cell lines, notably leading to a strong increase in miR-210 targeting (King et al., 2012). Interleukin treatment also promotes activation-dependent changes in miRNA populations released by macrophages through EVs (Squadrito et al., 2014). The miRNA repertoire of colon cancer cell EVs is profoundly affected by mutations in the transcription factor KRAS. Cha et al. profiled the transcriptome of EVs released by cell lines differing only in KRAS status and showed that levels of the pro-metastatic miR-100 are decreased in mutant KRAS EVs, whereas miR-10b abundance is increased in these samples (Cha et al., 2015).
Unlike EVs released by non-malignant cells, breast cancer exosomes contain the proteins Dicer, TRBP and AGO2, which can perform cell-independent miRNA processing within EVs (Melo et al., 2014). Several studies have identified populations of pre-miRNA, mature miRNA, miRNA* strands, hairpins loops, and pre-miRNA cleavage products in EVs (Chen et al., 2010; Melo et al., 2014). Over the course of 48 h, Melo et al. identified a sharp decrease in pre-miRNA abundance in previously purified exosomes, which coincided with a marked increase in corresponding mature miRNA levels. In addition, Melo et al. identified a role of the sialoglycoprotein CD43 in recruiting Dicer to cancer cell exosomes. Indeed, co-immunoprecipiation revealed an interaction between the two proteins and silencing of CD43 severely compromised the recruitment of Dicer to exosomes. Moreover, inhibiting Dicer activity in breast cancer exosomes significantly impaired growth in recipient malignant cells, providing evidence of its involvement in tumor progression.
Villarroya-Beltri et al. provided robust evidence of a sequence-specific mechanism involving the RBP hnRNPA2B1 in miRNA sorting to EVs (Villarroya-Beltri et al., 2013). The authors investigated activation-dependent changes in the miRNA repertoire of lymphoblasts and observed divergent trends in cells and EVs consistent with active, sequence-specific loading. Sequence alignments and targeted mutagenesis revealed a role of the GGAG motif in miRNA targeting to EVs. RNA pull-down experiments coupled to mass spectrometry identified three hnRNP factors specifically bound to EV-targeted miRNA. The author focused on hnRNPA2B1 and confirmed specific miRNA association by immunoprecipitation coupled to qPCR. They also showed that hnRNPA2B1 targeting to EVs is regulated by SUMO conjugation. Annexin A2 is a Ca2+-binding protein that contributes to link membrane-associated complexes to cytoskeletal components (Gerke et al., 2005). Annexin A2 exhibits sequence-specific RNA-binding activity and is involved in c-myc post-transcriptional regulation (Filipenko et al., 2004). Proteomic studies have revealed that Annexin A2 is among the most abundant proteins in EVs (Hagiwara et al., 2015). Hagiwara et al. provided evidence that Annexin A2 can bind miRNA in the presence of Ca2+ in diverse cancer cell lines (Hagiwara et al., 2015). The authors reported a global decrease in miRNA loading to cancer cell EVs upon Annexin A2 silencing.
Lipidomics studies based on mass spectrometry and nuclear magnetic resonance have unraveled profound differences in the composition of EV and plasma membranes (Choi et al., 2013). Lipid rafts are dynamic, detergent-resistant membrane microdomains enriched in sphingomyelin and depleted in phosphatidylcholine (de Gassart et al., 2003). Lipid rafts are overrepresented in EVs and have been involved in sorting proteins and RNAs to exosomes (de Gassart et al., 2003; Dubois et al., 2015). The sphingolipid ceramide is enriched in lipid rafts and implicated in membrane sorting during exosome budding (Megha and London, 2004; Trajkovic et al., 2008). Neutral sphingomyelinase (nSMase) is the rate-limiting enzyme in ceramide biogenesis and its inhibitor GW4869 has been used by several groups to restrict exosome release in vitro (Trajkovic et al., 2008; Yuyama et al., 2012; Essandoh et al., 2015). The “ceramide pathway” has emerged as an important route for miRNA loading to exosomes (Yuyama et al., 2012). Kosaka et al. provided evidence that metastatic cancer cells exert microenvironment remodeling of endothelial cells through exosome-associated miR-210 (Kosaka et al., 2013). This phenotype was abrogated by silencing nSMase in breast cancer cell lines, consistent with a role of the ceramide pathway in exosomal miRNA sorting.
Koppers-Lalic et al. reported that 3′ end uridylated miRNA isoforms are enriched in B cell exosomes, whereas 3′ end adenylated isoforms are poorly targeted and relatively enriched in cells (Koppers-Lalic et al., 2014). The authors extended their finding in EVs purified from human urine samples and concluded that non-templated terminal uridylation promotes miRNA sorting to EVs. Previous studies have shown that terminal adenylation increases transcript stability while uridylation has a destabilizing effect (Scott and Norbury, 2013), bridging the findings of Koppers-Lalic et al. to Batagov et al.'s conclusions that RNAs with short half-lives are enriched in EVs. Squadrito et al. investigated co-dependencies in miRNA and target mRNA levels in bone marrow-derived macrophages and corresponding EVs (Squadrito et al., 2014). The authors used IL-4 and genetic perturbations to alter the expression of miRNA and their target mRNA. Their observations suggest that the levels of endogenous target modulate miRNA sorting to EVs, likely through a relocation of RISC from P-bodies to MVEs. Indeed, several studies suggest that intracellular targeting of AGO2 complexes to endolysosomal compartments and exosomes reflects the dynamics of membrane-less organelles such as P-bodies and GW182-bodies (Siomi and Siomi, 2009).
Recruitment of AGO2-Bound miRNA to MVEs
Gibbings et al. investigated RISC subcellular distribution using cell fractionation, immunofluorescence and qPCR (Gibbings et al., 2009). They showed that “GW-bodies” containing AGO2 and its cofactor GW182 are distinct from canonical P-bodies and selectively congregate with MVEs. Immunogold labeling of monocoyte-derived exosomes revealed enrichments for GW182 but not DCP1A, a canonical P-body marker. Diverse miRNA and their target mRNA were enriched in the vicinity of GW182-positive MVEs. To understand how GW-182 bodies are recruited to MVEs, the authors silenced components of the endosomal sorting complexes required for transport (ESCRT), a highly conserved multisubunit machinery that localizes to MVEs and performs bending and scission of the membrane involved in protein and exosome release (Schmidt and Teis, 2012). They showed that depleting ESCRT components severely impairs miRNA silencing activity in the cell by monitoring let-7-a and miR-206 repression through reporter assays. Gibbings et al. thus established two key principles: (1) miRNA-loaded RISCs congregate at the site of exosome biogenesis and (2) ESCRT components regulate both exosome biogenesis and RNAi.
Independently of its involvement in exosome secretion, the ESCRT-II complex exhibits sequence-specific RNA-binding activity in metazoans. Irion et al. (Irion and St Johnston, 2007) focused on bicoid mRNA localization during Drosophila development. The study revealed that mutations in all three subunits of the ESCRT-II complex abolish the localization of bicoid mRNA at the anterior pole of the egg. The authors demonstrated a direct interaction between the N-terminal GLUE domain of VPS36 and stem-loop V in bicoid 3′UTR using UV-crosslinking and a yeast three-hybrid assay. They extended their finding in Xenopus, establishing conservation of the interaction in Vertebrates. ESCRT-II is thus at the crossroads of exosome biogenesis, RNAi and subcellular RNA localization, prompting speculations that the complex may contribute to sRNA sorting to exosomes. Kosaka et al. tested the hypothesis and depleted an ESCRT component, Alix, in HEK293 cells. In agreement with Gibbings et al. luciferase assays showed a reduction in intracellular silencing activity by miR-146. However, the amount of miR-146 in EVs was not altered by Alix depletion. EVs from Alix-depleted HEK293 cells contained miR-146 and silenced a reporter gene in recipient cells as efficiently as EVs released by untreated cells. Further efforts are required to elucidate the involvement of ESCRT components in sRNA sorting to EVs.
Recent work by McKenzie et al. provides an alternative mechanism of AGO2-miRNA relocation from P-bodies to MVEs (McKenzie et al., 2016). Echoing Cha et al.'s identification of KRAS signaling as a modulator of miRNA sorting to EVs, McKenzie et al. showed that KRAS-dependent activation of the MEK-ERK pathway inhibits AGO2 sorting to EVs. This work revisits a previously identified KRAS-dependent phosphorylation of serine residue 387 on AGO2 and demonstrates its implication in excluding AGO2-miRNA complexes from MVE association and exosome targeting. AGO2 targeting to exosomes thus reflects KRAS-MEK-ERK signaling status, which is impacted by environmental cues. These reconciliatory findings provide a possible explanation for discrepancies in previous reports regarding AGO2 levels in exosomes (Gibbings et al., 2009; Melo et al., 2014).
Contrasting and “EV Sceptic” Perspectives
We have reviewed examples of RNAi activity transfers across diverse species spanning the eukaryotic and prokaryotic domains of life. We then envisioned possible vehicles of sRNA transfer, including EVs, lipoproteins, soluble RNPs, and tunneling nanotubes. We emphasized emerging mechanisms of sRNA sorting to EVs in mammalian system, suggesting that these vesicles may contribute to cross-species sRNA transfers. EV association strongly enhances the stability of RNA molecules in the extracellular environment. In addition, examples of long-range transfers of biomolecules through EVs have been reported in diverse systems. In C. elegans, EVs transferred between worms contribute to the specification of male sexual behavior (Wang et al., 2014), while functional EV-associated transcripts encoding a Cre recombinase are shuttled across distant tumors in mice (Zomer et al., 2015). Transfers of EVs and delivery of molecular cargo from human to mouse cultured cells and from the protozoan pathogen Trypanosoma cruzi to human erythrocytes have been documented, suggesting that EVs can indeed serve as widespread mediators of interspecies RNA transfers (Valadi et al., 2007; Deolindo et al., 2013; Evans-Osses et al., 2015).
Numerous studies thus support the functionality of EV-associated sRNA populations in intercellular communication (Pegtel et al., 2010; Katsuda et al., 2014; Melo et al., 2014). However, contrasting reports resulting from careful quantitative assessments argue that EVs are poor vehicles for RNA transfers due to degradation upon recipient cell entry and/or insufficient cargo abundance. Kanada et al. examined the fate of nucleic acids contained in HEK293FT small exosome-like EVs and larger microvesicle-like EVs upon recipient cell entry (Kanada et al., 2015). They found that exosome-like EVs fail to transfer nucleic acids to murine 4TI recipient cells. Microvesicle-like EVs delivered reporter RNA, which was successfully amplified using a nested PCR approach 24 h after delivery. However, full-length and fragmented reporter RNA was undetectable 48 h after transfer assays, likely due to degradation in acidic lysosomal compartments. Plasmid-encoded Cre recombinase was efficiently loaded in microvesicle-like EVs as plasmidic (p)DNA, RNA and protein. Recombinase activity was stably transmitted to recipient cells, but exclusively through pDNA. These findings suggest that pDNA transfers may have confounded the conclusions of several studies using reporter constructions to investigate RNA transfers.
Chevillet et al. purified EVs from five human biological fluids and cell line media and used quantitative approaches to determine miRNA stoichiometric abundance in these samples (Chevillet et al., 2014). Regardless of the source, they found that EV samples contain low counts of individual miRNA, amounting at most to an average of (0.00825 ± 0.02) miRNA molecules per EV. While this result suggests that EVs are poor miRNA transfer vehicles in vivo, Chevillet et al. discussed diverse stoichiometric models to reconcile their assessments with reports of functional miRNA delivery. Indeed, population analyses are unable to determine the distributions of miRNA molecules in individual EVs. A low occupancy/high miRNA concentration model, wherein most EVs contain no miRNA molecule but a single EV carries several copies could be compatible with reports of functional transfers. Similarly, the kinetics of EV uptake could impact functionality in recipient cells, provided they internalize EVs at a high frequency. In the context of interspecies communication, it should be noted that organisms able to amplify systemic RNAi responses such as plants and nematodes may exhibit enhanced sensitivity to signals conveyed by a low number of initial miRNA copies.
Contrasting and skeptical reports are highly valuable to the EV field and should inform attentive methodological choices for future experiments. In transfer assays, reporter genes expressed from chromosomal insertions should be favored rather than plasmid-based approaches, effectively ruling out pDNA transfers. DNAse treatments should be used when specifically investigating the roles of EV-associated RNA. Imaging EV transfers through time-lapse analysis of high-resolution microscopy data could illuminate the kinetics of internalization. In addition, the fate of transferred biomolecules should be considered over the course of several days, documenting the association of transferred material with subcellular compartments of recipient cells that may impact stability. Dutiful application of these principles will reveal whether membrane vesicles can indeed spread silencing instructions across phylogenetic boundaries.
Author Contributions
FL wrote the manuscript and assembled the figures. EL provided oversight and revised the manuscript.
Funding
EV research in the Lécuyer lab has been supported by the Cancer Research Society of Canada (grant #20227).
Conflict of Interest Statement
The authors declare that the research was conducted in the absence of any commercial or financial relationships that could be construed as a potential conflict of interest.
Acknowledgments
FL is supported by a scholarship from the Canadian Institute of Health Research (CIHR).
References
(2012). Alterations in the intestinal microbiota promote colorectal cancer. Cancer Discov. 2:866. doi: 10.1158/2159-8290.CD-RW2012-138
Abad, P., Gouzy, J., Aury, J. M., Castagnone-Sereno, P., Danchin, E. G. J., Deleury, E., et al. (2008). Genome sequence of the metazoan plant-parasitic nematode Meloidogyne incognita. Nat. Biotechnol. 26, 909–915. doi: 10.1038/nbt.1482
Akers, J. C., Gonda, D., Kim, R., Carter, B. S., and Chen, C. C. (2013). Biogenesis of extracellular vesicles (EV): exosomes, microvesicles, retrovirus-like vesicles, and apoptotic bodies. J. Neurooncol. 113, 1–11. doi: 10.1007/s11060-013-1084-8
Arroyo, J. D., Chevillet, J. R., Kroh, E. M., Ruf, I. K., Pritchard, C. C., Gibson, D. F., et al. (2011). Argonaute2 complexes carry a population of circulating microRNAs independent of vesicles in human plasma. Proc. Natl. Acad. Sci. U.S.A. 108, 5003–5008. doi: 10.1073/pnas.1019055108
Baraniskin, A., Kuhnhenn, J., Schlegel, U., Chan, A., Deckert, M., Gold, R., et al. (2011). Identification of microRNAs in the cerebrospinal fluid as marker for primary diffuse large B-cell lymphoma of the central nervous system. Blood 117, 3140–3146. doi: 10.1182/blood-2010-09-308684
Batagov, A. O., Kuznetsov, V. A., and Kurochkin, I. V. (2011). Identification of nucleotide patterns enriched in secreted RNAs as putative cis-acting elements targeting them to exosome nano-vesicles. BMC Genomics 12(Suppl. 3):S18. doi: 10.1186/1471-2164-12-S3-S18
Baum, J. A., Bogaert, T., Clinton, W., Heck, G. R., Feldmann, P., Ilagan, O., et al. (2007). Control of coleopteran insect pests through RNA interference. Nat. Biotechnol. 25, 1322–1326. doi: 10.1038/nbt1359
Bellingham, S. A., Coleman, B. M., and Hill, A. F. (2012). Small RNA deep sequencing reveals a distinct miRNA signature released in exosomes from prion-infected neuronal cells. Nucleic Acids Res. 40, 10937–10949. doi: 10.1093/nar/gks832
Belting, M., and Wittrup, A. (2008). Nanotubes, exosomes, and nucleic acid-binding peptides provide novel mechanisms of intercellular communication in eukaryotic cells: implications in health and disease. J. Cell Biol. 183, 1187–1191. doi: 10.1083/jcb.200810038
Bolukbasi, M. F., Mizrak, A., Ozdener, G. B., Madlener, S., Strobel, T., Erkan, E. P., et al. (2012). miR-1289 and “Zipcode”-like Sequence enrich mRNAs in microvesicles. Mol. Ther. Nucl. Acids 1:e10. doi: 10.1038/mtna.2011.2
Braniste, V., Al-Asmakh, M., Kowal, C., Anuar, F., Abbaspour, A., Toth, M., et al. (2014). The gut microbiota influences blood-brain barrier permeability in mice. Sci. Transl. Med. 6:263ra158. doi: 10.1126/scitranslmed.3009759
Cha, D. J., Franklin, J. L., Dou, Y., Liu, Q., Higginbotham, J. N., Demory Beckler, M., et al. (2015). KRAS-dependent sorting of miRNA to exosomes. Elife 4:e07197. doi: 10.7554/eLife.07197
Chen, T. S., Lai, R. C., Lee, M. M., Choo, A. B., Lee, C. N., and Lim, S. K. (2010). Mesenchymal stem cell secretes microparticles enriched in pre-microRNAs. Nucleic Acids Res. 38, 215–224. doi: 10.1093/nar/gkp857
Chen, T., Xi, Q. Y., Ye, R. S., Cheng, X., Qi, Q. E., Wang, S. B., et al. (2014). Exploration of microRNAs in porcine milk exosomes. BMC Genomics 15:100. doi: 10.1186/1471-2164-15-100
Chevillet, J. R., Kang, Q., Ruf, I. K., Briggs, H. A., Vojtech, L. N., Hughes, S. M., et al. (2014). Quantitative and stoichiometric analysis of the microRNA content of exosomes. Proc. Natl. Acad. Sci. U.S.A. 111, 14888–14893. doi: 10.1073/pnas.1408301111
Choi, D. S., Kim, D. K., Kim, Y. K., and Gho, Y. S. (2013). Proteomics, transcriptomics and lipidomics of exosomes and ectosomes. Proteomics 13, 1554–1571. doi: 10.1002/pmic.201200329
Clark, M. B., Amaral, P. P., Schlesinger, F. J., Dinger, M. E., Taft, R. J., Rinn, J. L., et al. (2011). The reality of pervasive transcription. PLoS Biol. 9:e1000625. discussion:e1102. doi: 10.1371/journal.pbio.1001102
Cody, N. A., Iampietro, C., and Lécuyer, E. (2013). The many functions of mRNA localization during normal development and disease: from pillar to post. WIREs Dev. Biol. 2, 781–796. doi: 10.1002/wdev.113
Coffey, A. J., Kokocinski, F., Calafato, M. S., Scott, C. E., Palta, P., Drury, E., et al. (2011). The GENCODE exome: sequencing the complete human exome. Eur. J. Hum. Genet. 19, 827–831. doi: 10.1038/ejhg.2011.28
Collins, R. E., and Cheng, X. (2006). Structural and biochemical advances in mammalian RNAi. J. Cell Biochem. 99, 1251–1266. doi: 10.1002/jcb.21069
Contreras-Galindo, R., Kaplan, M. H., Leissner, P., Verjat, T., Ferlenghi, I., Bagnoli, F., et al. (2008). Human endogenous retrovirus K (HML-2) elements in the plasma of people with lymphoma and breast cancer. J. Virol. 82, 9329–9336. doi: 10.1128/JVI.00646-08
Crescitelli, R., Lasser, C., Szabo, T. G., Kittel, A., Eldh, M., Dianzani, I., et al. (2013). Distinct RNA profiles in subpopulations of extracellular vesicles: apoptotic bodies, microvesicles and exosomes. J. Extracell. Vesicles 2:e10. doi: 10.3402/jev.v2i0.20677
Czaplinski, K., and Singer, R. H. (2006). Pathways for mRNA localization in the cytoplasm. Trends Biochem Sci. 31, 687–693. doi: 10.1016/j.tibs.2006.10.007
da Silveira, J. C., de Andrade, G. M., Nogueira, M. F., Meirelles, F. V., and Perecin, F. (2015). Involvement of miRNAs and cell-secreted vesicles in mammalian ovarian antral follicle development. Reprod. Sci. 22, 1474–1483. doi: 10.1177/1933719115574344
Dai, X., Chen, X., Chen, Q., Shi, L., Liang, H. W., Zhou, Z., et al. (2015). MicroRNA-193a-3p Reduces intestinal inflammation in response to microbiota via down-regulation of colonic PepT1. J. Biol. Chem. 290, 16099–16115. doi: 10.1074/jbc.M115.659318
Dalmasso, G., Nguyen, H. T. T., Yan, Y., Laroui, H., Charania, M. A., Ayyadurai, S., et al. (2011). Microbiota modulates host gene expression via MicroRNAs. Gastroenterology 140:S663. doi: 10.1016/S0016-5085(11)62754-6
de Gassart, A., Geminard, C., Fevrier, B., Raposo, G., and Vidal, M. (2003). Lipid raft-associated protein sorting in exosomes. Blood 102, 4336–4344. doi: 10.1182/blood-2003-03-0871
Deolindo, P., Evans-Osses, I., and Ramirez, M. I. (2013). Microvesicles and exosomes as vehicles between protozoan and host cell communication. Biochem. Soc. Trans. 41, 252–257. doi: 10.1042/BST20120217
Derrien, T., Johnson, R., Bussotti, G., Tanzer, A., Djebali, S., Tilgner, H., et al. (2012). The GENCODE v7 catalog of human long noncoding RNAs: analysis of their gene structure, evolution, and expression. Genome Res. 22, 1775–1789. doi: 10.1101/gr.132159.111
Dinh, P. T. Y., Brown, C. R., and Elling, A. A. (2014). RNA interference of effector gene Mc16D10L confers resistance against Meloidogyne chitwoodi in Arabidopsis and potato. Phytopathology 104, 1098–1106. doi: 10.1094/PHYTO-03-14-0063-R
Dror, S., Sander, L., Schwartz, H., Sheinboim, D., Barzilai, A., Dishon, Y., et al. (2016). Melanoma miRNA trafficking controls tumour primary niche formation. Nat. Cell Biol. 18, 1006–1017. doi: 10.1038/ncb3399
Du, T. G., Schmid, M., and Jansen, R. P. (2007). Why cells move messages: the biological functions of mRNA localization. Semin. Cell Dev. Biol. 18, 171–177. doi: 10.1016/j.semcdb.2007.01.010
Dubois, L., Ronquist, K. K., Ek, B., Ronquist, G., and Larsson, A. (2015). Proteomic profiling of detergent resistant membranes (Lipid Rafts) of prostasomes. Mol. Cell. Proteomics 14, 3015–3022. doi: 10.1074/mcp.M114.047530
Elmore, S. (2007). Apoptosis: a review of programmed cell death. Toxicol. Pathol. 35, 495–516. doi: 10.1080/01926230701320337
Escobar, M. A., Civerolo, E. L., Summerfelt, K. R., and Dandekar, A. M. (2001). RNAi-mediated oncogene silencing confers resistance to crown gall tumorigenesis. Proc. Natl. Acad. Sci. U.S.A. 98, 13437–13442. doi: 10.1073/pnas.241276898
Escobar, M. A., Leslie, C. A., McGranahan, G. H., and Dandekar, A. M. (2002). Silencing crown gall disease in walnut (Juglans regia L.). Plant Sci. 163, 591–597. doi: 10.1016/S0168-9452(02)00164-4
Essandoh, K., Yang, L., Huang, W., Wang, X., Qin, D., Wang, Y., et al. (2015). Blockade of exosome release with Gw4869 dampens sepsis-induced inflammation and cardiac dysfunction. Shock 43:123.
Evans-Osses, I., Reichembach, L. H., and Ramirez, M. I. (2015). Exosomes or microvesicles? Two kinds of extracellular vesicles with different routes to modify protozoan-host cell interaction. Parasitol. Res. 114, 3567–3575. doi: 10.1007/s00436-015-4659-9
Faith, J. J., Guruge, J. L., Charbonneau, M., Subramanian, S., Seedorf, H., Goodman, A. L., et al. (2013). The long-term stability of the human gut microbiota. Science 341:44. doi: 10.1126/science.1237439
Fernandez-Calero, T., Garcia-Silva, R., Pena, A., Robello, C., Persson, H., Rovira, C., et al. (2015). Profiling of small RNA cargo of extracellular vesicles shed by Trypanosoma cruzi reveals a specific extracellular signature. Mol. Biochem. Parasit. 199, 19–28. doi: 10.1016/j.molbiopara.2015.03.003
Filipenko, N. R., MacLeod, T. J., Yoon, C. S., and Waisman, D. M. (2004). Annexin A2 is a novel RNA-binding protein. J. Biol. Chem. 279, 8723–8731. doi: 10.1074/jbc.M311951200
Fire, A., Xu, S., Montgomery, M. K., Kostas, S. A., Driver, S. E., and Mello, C. C. (1998). Potent and specific genetic interference by double-stranded RNA in Caenorhabditis elegans. Nature 391, 806–811. doi: 10.1038/35888
Fitzgerald, A., van Kan, J. A. L., and Plummer, K. M. (2004). Simultaneous silencing of multiple genes in the apple scab fungus, Venturia inaequalis, by expression of RNA with chimeric inverted repeats. Fungal Genet. Biol. 41, 963–971. doi: 10.1016/j.fgb.2004.06.006
Forstemann, K., Tomari, Y., Du, T., Vagin, V. V., Denli, A. M., Bratu, D. P., et al. (2005). Normal microRNA maturation and germ-line stem cell maintenance requires Loquacious, a double-stranded RNA-binding domain protein. PLoS Biol. 3:e236. doi: 10.1371/journal.pbio.0030236
Gerke, V., Creutz, C. E., and Moss, S. E. (2005). Annexins: linking Ca2+ signalling to membrane dynamics. Nat. Rev. Mol. Cell. Biol. 6, 449–461. doi: 10.1038/nrm1661
Ghag, S. B., Shekhawat, U. K. S., and Ganapathi, T. R. (2014). Host-induced post-transcriptional hairpin RNA-mediated gene silencing of vital fungal genes confers efficient resistance against Fusarium wilt in banana. Plant Biotechnol. J. 12, 541–553. doi: 10.1111/pbi.12158
Ghildiyal, M., and Zamore, P. D. (2009). Small silencing RNAs: an expanding universe. Nat. Rev. Genet. 10, 94–108. doi: 10.1038/nrg2504
Gibbings, D. J., Ciaudo, C., Erhardt, M., and Voinnet, O. (2009). Multivesicular bodies associate with components of miRNA effector complexes and modulate miRNA activity. Nat. Cell Biol. 11, 1143–1149. doi: 10.1038/ncb1929
Gramates, L. S. (2006). Insights into social insects from the genome of the honeybee Apis mellifera. Nature 2006 443, 931–949. doi: 10.1038/nature05400
Hagiwara, K., Katsuda, T., Gailhouste, L., Kosaka, N., and Ochiya, T. (2015). Commitment of Annexin A2 in recruitment of microRNAs into extracellular vesicles. FEBS Lett. 589, 4071–4078. doi: 10.1016/j.febslet.2015.11.036
Halfvarson, J., Dicksved, J., Rosenquist, M., Jarnerot, G., Tysk, C., Engstrand, L., et al. (2008). Molecular fingerprinting of the gut microbiota of twins reveals differences according to Crohn's disease. Gastroenterology 134:A650. doi: 10.1016/S0016-5085(08)63034-6
Halperin, W., and Jensen, W. A. (1967). Ultrastructural changes during growth and embryogenesis in carrot cell cultures. J. Ultrastruct. Res. 18, 428–443. doi: 10.1016/S0022-5320(67)80128-X
Hammond, S. M., Boettcher, S., Caudy, A. A., Kobayashi, R., and Hannon, G. J. (2001). Argonaute2, a link between genetic and biochemical analyses of RNAi. Science 293, 1146–1150. doi: 10.1126/science.1064023
Harding, C., Heuser, J., and Stahl, P. (1983). Receptor-mediated endocytosis of transferrin and recycling of the transferrin receptor in rat reticulocytes. J. Cell Biol. 97, 329–339. doi: 10.1083/jcb.97.2.329
Helber, N., Wippel, K., Sauer, N., Schaarschmidt, S., Hause, B., and Requena, N. (2011). A versatile monosaccharide transporter that operates in the arbuscular mycorrhizal fungus Glomus sp is crucial for the symbiotic relationship with plants. Plant Cell 23, 3812–3823. doi: 10.1105/tpc.111.089813
Hergenreider, E., Heydt, S., Treguer, K., Boettger, T., Horrevoets, A. J., Zeiher, A. M., et al. (2012). Atheroprotective communication between endothelial cells and smooth muscle cells through miRNAs. Nat. Cell Biol. 14, 249–256. doi: 10.1038/ncb2441
Hill, A. F., Pegtel, D. M., Lambertz, U., Leonardi, T., O'Driscoll, L., Pluchino, S., et al. (2013). ISEV position paper: extracellular vesicle RNA analysis and bioinformatics. J. Extracell. Vesicles 2:e8. doi: 10.3402/jev.v2i0.22859
Hoekema, A., Hirsch, P. R., Hooykaas, P. J. J., and Schilperoort, R. A. (1983). A binary plant vector strategy based on separation of vir-region and T-region of the Agrobacterium-tumefaciens Ti-plasmid. Nature 303, 179–180. doi: 10.1038/303179a0
Huang, X. Y., Yuan, T. Z., Tschannen, M., Sun, Z. F., Jacob, H., Du, M. J., et al. (2013). Characterization of human plasma-derived exosomal RNAs by deep sequencing. BMC Genomics 14:319. doi: 10.1186/1471-2164-14-319
Hung, M. E., and Leonard, J. N. (2016). A platform for actively loading cargo RNA to elucidate limiting steps in EV-mediated delivery. J. Extracell. Vesicles 5:e13. doi: 10.3402/jev.v5.31027
Hunter, M. P., Ismail, N., Zhang, X., Aguda, B. D., Lee, E. J., Yu, L., et al. (2008). Detection of microRNA expression in human peripheral blood microvesicles. PLoS ONE 3:e3694. doi: 10.1371/journal.pone.0003694
Ibrahim, H. M., Alkharouf, N. W., Meyer, S. L., Aly, M. A., El-Din, A. E. Y. G., Hussein, E. H. A., et al. (2011). Post-transcriptional gene silencing of root-knot nematode in transformed soybean roots. Exp. Parasitol. 127, 90–99. doi: 10.1016/j.exppara.2010.06.037
Ihara, T., Yamamoto, T., Sugamata, M., Okumura, H., and Ueno, Y. (1998). The process of ultrastructural changes from nuclei to apoptotic body. Virchows Arch. 433, 443–447. doi: 10.1007/s004280050272
Irion, U., and St Johnston, D. (2007). bicoid RNA localization requires specific binding of an endosomal sorting complex. Nature 445, 554–558. doi: 10.1038/nature05503
Jalali, S., Gandhi, S., and Scaria, V. (2016). Navigating the dynamic landscape of long noncoding RNA and protein-coding gene annotations in GENCODE. Hum Genomics 10:35. doi: 10.1186/s40246-016-0090-2
Jose, A. M., Smith, J. J., and Hunter, C. P. (2009). Export of RNA silencing from C-elegans tissues does not require the RNA channel SID-1. Proc. Natl. Acad. Sci. U.S.A. 106, 2283–2288. doi: 10.1073/pnas.0809760106
Kahlert, C., and Kalluri, R. (2013). Exosomes in tumor microenvironment influence cancer progression and metastasis. J. Mol. Med. 91, 431–437. doi: 10.1007/s00109-013-1020-6
Kalra, H., Simpson, R. J., Ji, H., Aikawa, E., Altevogt, P., Askenase, P., et al. (2012). Vesiclepedia: a compendium for extracellular vesicles with continuous community annotation. PLoS Biol. 10:e1001450. doi: 10.1371/journal.pbio.1001450
Kanada, M., Bachmann, M. H., Hardy, J. W., Frimannson, D. O., Bronsart, L., Wang, A., et al. (2015). Differential fates of biomolecules delivered to target cells via extracellular vesicles. Proc. Natl. Acad. Sci. U.S.A. 112, E1433–E1442. doi: 10.1073/pnas.1418401112
Katsuda, T., Ikeda, S., Yoshioka, Y., Kosaka, N., Kawamata, M., and Ochiya, T. (2014). Physiological and pathological relevance of secretory microRNAs and a perspective on their clinical application. Biol. Chem. 395, 365–373. doi: 10.1515/hsz-2013-0222
Khila, A., and Grbic, M. (2007). Gene silencing in the spider mite Tetranychus urticae: dsRNA and siRNA parental silencing of the Distal-less gene. Dev. Genes Evol. 217, 241–251. doi: 10.1007/s00427-007-0132-9
King, H. W., Michael, M. Z., and Gleadle, J. M. (2012). Hypoxic enhancement of exosome release by breast cancer cells. BMC Cancer 12:421. doi: 10.1186/1471-2407-12-421
Knip, M., Constantin, M. E., and Thordal-Christensen, H. (2014). Trans-kingdom cross-talk: small RNAs on the move. PLoS Genet. 10:e1004602. doi: 10.1371/journal.pgen.1004602
Koch, A., Kumar, N., Weber, L., Keller, H., Imani, J., and Kogel, K. H. (2013). Host-induced gene silencing of cytochrome P450 lanosterol C14alpha-demethylase-encoding genes confers strong resistance to Fusarium species. Proc. Natl. Acad. Sci. U.S.A. 110, 19324–19329. doi: 10.1073/pnas.1306373110
Koppers-Lalic, D., Hackenberg, M., Bijnsdorp, I. V., van Eijndhoven, M. A. J., Sadek, P., Sie, D., et al. (2014). Nontemplated nucleotide additions distinguish the small RNA composition in cells from exosomes. Cell Rep. 8, 1649–1658. doi: 10.1016/j.celrep.2014.08.027
Kosaka, N., Iguchi, H., Hagiwara, K., Yoshioka, Y., Takeshita, F., and Ochiya, T. (2013). Neutral sphingomyelinase 2 (nSMase2)-dependent exosomal transfer of angiogenic microRNAs regulate cancer cell metastasis. J. Biol. Chem. 288, 10849–10859. doi: 10.1074/jbc.M112.446831
Kowal, J., Arras, G., Colombo, M., Jouve, M., Morath, J. P., Primdal-Bengtson, B., et al. (2016). Proteomic comparison defines novel markers to characterize heterogeneous populations of extracellular vesicle subtypes. Proc. Natl. Acad. Sci. U.S.A. 113, E968–E977. doi: 10.1073/pnas.1521230113
Kozomara, A., and Griffiths-Jones, S. (2014). miRBase: annotating high confidence microRNAs using deep sequencing data. Nucleic Acids Res. 42, D68–D73. doi: 10.1093/nar/gkt1181
Lambertz, U., Ovando, M. E. O., Vasconcelos, E. J. R., Unrau, P. J., Myler, P. J., and Reiner, N. E. (2015). Small RNAs derived from tRNAs and rRNAs are highly enriched in exosomes from both old and new world Leishmania providing evidence for conserved exosomal RNA Packaging. BMC Genomics 16:151. doi: 10.1186/s12864-015-1260-7
Lasser, C., Alikhani, V. S., Ekstrom, K., Eldh, M., Paredes, P. T., Bossios, A., et al. (2011). Human saliva, plasma and breast milk exosomes contain RNA: uptake by macrophages. J. Transl. Med. 9:e10. doi: 10.1186/1479-5876-9-9
Latham, V. M. Jr., Kislauskis, E. H., Singer, R. H., and Ross, A. F. (1994). Beta-actin mRNA localization is regulated by signal transduction mechanisms. J. Cell Biol. 126, 1211–1219. doi: 10.1083/jcb.126.5.1211
Lazaro-Ibanez, E., Sanz-Garcia, A., Visakorpi, T., Escobedo-Lucea, C., Siljander, P., Ayuso-Sacido, A., et al. (2014). Different gDNA content in the subpopulations of prostate cancer extracellular vesicles: apoptotic bodies, microvesicles, and exosomes. Prostate 74, 1379–1390. doi: 10.1002/pros.22853
Lecuyer, E., Yoshida, H., Parthasarathy, N., Alm, C., Babak, T., Cerovina, T., et al. (2007). Global analysis of mRNA localization reveals a prominent role in organizing cellular architecture and function. Cell 131, 174–187. doi: 10.1016/j.cell.2007.08.003
Lee, Y. S., Nakahara, K., Pham, J. W., Kim, K., He, Z., Sontheimer, E. J., et al. (2004). Distinct roles for drosophila dicer-1 and dicer-2 in the siRNA/miRNA silencing pathways. Cell 117, 69–81. doi: 10.1016/S0092-8674(04)00261-2
Lefebvre, F. A., Benoit Bouvrette, L. P., Perras, L., Blanchet-Cohen, A., Garnier, D., Rak, J., et al. (2016). Comparative transcriptomic analysis of human and Drosophila extracellular vesicles. Sci Rep. 6:27680. doi: 10.1038/srep27680
Lewis, B. P., Burge, C. B., and Bartel, D. P. (2005). Conserved seed pairing, often flanked by adenosines, indicates that thousands of human genes are microRNA targets. Cell 120, 15–20. doi: 10.1016/j.cell.2004.12.035
Ley, R. E., Peterson, D. A., and Gordon, J. I. (2006). Ecological and evolutionary forces shaping microbial diversity in the human intestine. Cell 124, 837–848. doi: 10.1016/j.cell.2006.02.017
Li, C. C., Eaton, S. A., Young, P. E., Lee, M., Shuttleworth, R., Humphreys, D. T., et al. (2013). Glioma microvesicles carry selectively packaged coding and non-coding RNAs which alter gene expression in recipient cells. RNA Biol. 10, 1333–1344. doi: 10.4161/rna.25281
Liu, H., Wang, X., Wang, H. D., Wu, J., Ren, J., Meng, L., et al. (2012). Escherichia coli noncoding RNAs can affect gene expression and physiology of Caenorhabditis elegans. Nat. Commun. 3:1073. doi: 10.1038/ncomms2071
Liu, S. R., da Cunha, A. P., Rezende, R. M., Cialic, R., Wei, Z. Y., Bry, L., et al. (2016). The host shapes the gut microbiota via fecal microRNA. Cell Host Microbe 19, 32–43. doi: 10.1016/j.chom.2015.12.005
Liu, Y., Ye, X., Jiang, F., Liang, C., Chen, D., Peng, J., et al. (2009). C3PO, an endoribonuclease that promotes RNAi by facilitating RISC activation. Science 325, 750–753. doi: 10.1126/science.1176325
Louis, P., Hold, G. L., and Flint, H. J. (2014). The gut microbiota, bacterial metabolites and colorectal cancer. Nat. Rev. Microbiol. 12, 661–672. doi: 10.1038/nrmicro3344
MacDonald, P. M. (1990). bicoid mRNA localization signal: phylogenetic conservation of function and RNA secondary structure. Development 110, 161–171.
Mao, Y. B., Cai, W. J., Wang, J. W., Hong, G. J., Tao, X. Y., Wang, L. J., et al. (2007). Silencing a cotton bollworm P450 monooxygenase gene by plant-mediated RNAi impairs larval tolerance of gossypol. Nat. Biotechnol. 25, 1307–1313. doi: 10.1038/nbt1352
Martin, K. C., and Ephrussi, A. (2009). mRNA localization: gene expression in the spatial dimension. Cell 136, 719–730. doi: 10.1016/j.cell.2009.01.044
Mattick, J. S., and Makunin, I. V. (2005). Small regulatory RNAs in mammals. Hum Mol Genet. 14 Spec No 1, R121–R132. doi: 10.1093/hmg/ddi101
Mc, C. B. (1950). The origin and behavior of mutable loci in maize. Proc. Natl. Acad. Sci. U.S.A. 36, 344–355. doi: 10.1073/pnas.36.6.344
McCullen, C. A., and Binns, A. N. (2006). Agrobacterium tumefaciens and plant cell interactions and activities required for interkingdom macromolecular transfer. Annu. Rev. Cell Dev. Biol. 22, 101–127. doi: 10.1146/annurev.cellbio.22.011105.102022
McKenzie, A. J., Hoshino, D., Hong, N. H., Cha, D. J., Franklin, J. L., Coffey, R. J., et al. (2016). KRAS-MEK signaling controls Ago2 sorting into exosomes. Cell Rep. 15, 978–987. doi: 10.1016/j.celrep.2016.03.085
Megha, and London, E. (2004). Ceramide selectively displaces cholesterol from ordered lipid domains (rafts): implications for lipid raft structure and function. J. Biol. Chem. 279, 9997–10004. doi: 10.1074/jbc.M309992200
Melo, S. A., Sugimoto, H., O'Connell, J. T., Kato, N., Villanueva, A., Vidal, A., et al. (2014). Cancer exosomes perform cell-independent microRNA biogenesis and promote tumorigenesis. Cancer Cell. 26, 707–721. doi: 10.1016/j.ccell.2014.09.005
Michael, A., Bajracharya, S. D., Yuen, P. S. T., Zhou, H., Star, R. A., Illei, G. G., et al. (2010). Exosomes from human saliva as a source of microRNA biomarkers. Oral Dis. 16, 34–38. doi: 10.1111/j.1601-0825.2009.01604.x
Mitchell, P. S., Parkin, R. K., Kroh, E. M., Fritz, B. R., Wyman, S. K., Pogosova-Agadjanyan, E. L., et al. (2008). Circulating microRNAs as stable blood-based markers for cancer detection. Proc. Natl. Acad. Sci. U.S.A. 105, 10513–10518. doi: 10.1073/pnas.0804549105
Mizrak, A., Bolukbasi, M. F., Ozdener, G. B., Brenner, G. J., Madlener, S., Erkan, E. P., et al. (2013). Genetically engineered microvesicles carrying suicide mRNA/protein inhibit schwannoma tumor growth. Mol. Ther. 21, 101–108. doi: 10.1038/mt.2012.161
Montecalvo, A., Larregina, A. T., Shufesky, W. J., Stolz, D. B., Sullivan, M. L., Karlsson, J. M., et al. (2012). Mechanism of transfer of functional microRNAs between mouse dendritic cells via exosomes. Blood 119, 756–766. doi: 10.1182/blood-2011-02-338004
Moos, W. H., Faller, D. V., Harpp, D. N., Kanara, I., Pernokas, J., Powers, W. R., et al. (2016). Microbiota and neurological disorders: a gut feeling. Biores. Open Access 5, 137–145. doi: 10.1089/biores.2016.0010
Muster, T., Waltenberger, A., Grassauer, A., Hirschl, S., Caucig, P., Romirer, I., et al. (2003). An endogenous retrovirus derived from human melanoma cells. Cancer Res. 63, 8735–8741.
Mutti, N. S., Park, Y., Reese, J. C., and Reeck, G. R. (2006). RNAi knockdown of a salivary transcript leading to lethality in the pea aphid, Acyrthosiphon pisum. J. Insect Sci. 6:38. doi: 10.1673/031.006.3801
Nanbo, A., Kawanishi, E., Yoshida, R., and Yoshiyama, H. (2013). Exosomes derived from epstein-barr virus-infected cells are internalized via caveola-dependent endocytosis and promote phenotypic modulation in target cells. J. Virol. 87, 10334–10347. doi: 10.1128/JVI.01310-13
Napoli, C., Lemieux, C., and Jorgensen, R. (1990). Introduction of a chimeric chalcone synthase gene into petunia results in reversible co-suppression of homologous genes in trans. Plant Cell 2, 279–289. doi: 10.1105/tpc.2.4.279
Nehra, V., Allen, J. M., Mailing, L. J., Kashyap, P. C., and Woods, J. A. (2016). Gut Microbiota: modulation of host physiology in obesity. Physiology 31, 327–335. doi: 10.1152/physiol.00005.2016
Nilsson, J., Skog, J., Nordstrand, A., Baranov, V., Mincheva-Nilsson, L., Breakefield, X. O., et al. (2009). Prostate cancer-derived urine exosomes: a novel approach to biomarkers for prostate cancer. Brit. J. Cancer 100, 1603–1607. doi: 10.1038/sj.bjc.6605058
Noland, C. L., and Doudna, J. A. (2013). Multiple sensors ensure guide strand selection in human RNAi pathways. RNA 19, 639–648. doi: 10.1261/rna.037424.112
Noland, C. L., Ma, E., and Doudna, J. A. (2011). siRNA repositioning for guide strand selection by human Dicer complexes. Mol Cell. 43, 110–121. doi: 10.1016/j.molcel.2011.05.028
Nolte-'t Hoen, E. N., Buermans, H. P., Waasdorp, M., Stoorvogel, W., Wauben, M. H., and t Hoen, P. A. (2012). Deep sequencing of RNA from immune cell-derived vesicles uncovers the selective incorporation of small non-coding RNA biotypes with potential regulatory functions. Nucleic Acids Res. 40, 9272–9285. doi: 10.1093/nar/gks658
Patterson, E., Ryan, P. M., Cryan, J. F., Dinan, T. G., Ross, R. P., Fitzgerald, G. F., et al. (2016). Gut microbiota, obesity and diabetes. Postgrad. Med. J. 92, 286–300. doi: 10.1136/postgradmedj-2015-133285
Pegtel, D. M., Cosmopoulos, K., Thorley-Lawson, D. A., van Eijndhoven, M. A., Hopmans, E. S., Lindenberg, J. L., et al. (2010). Functional delivery of viral miRNAs via exosomes. Proc. Natl. Acad. Sci. U.S.A. 107, 6328–6333. doi: 10.1073/pnas.0914843107
Peres da Silva, R., Puccia, R., Rodrigues, M. L., Oliveira, D. L., Joffe, L. S., Cesar, G. V., et al. (2015). Extracellular vesicle-mediated export of fungal RNA. Sci Rep. 5:7763. doi: 10.1038/srep07763
Persson, H., Kvist, A., Vallon-Christersson, J., Medstrand, P., Borg, A., and Rovira, C. (2009). The non-coding RNA of the multidrug resistance-linked vault particle encodes multiple regulatory small RNAs. Nat. Cell Biol. 11, 1268–1271. doi: 10.1038/ncb1972
Pitino, M., Coleman, A. D., Maffei, M. E., Ridout, C. J., and Hogenhout, S. A. (2011). Silencing of aphid genes by dsRNA feeding from plants. PLoS ONE 6:e25709. doi: 10.1371/journal.pone.0025709
Raposo, G., and Stoorvogel, W. (2013). Extracellular vesicles: exosomes, microvesicles, and friends. J. Cell Biol. 200, 373–383. doi: 10.1083/jcb.201211138
Rissland, O. S., and Lai, E. C. (2011). RNA silencing in Monterey. Development 138, 3093–3102. doi: 10.1242/dev.065284
Runtsch, M. C., Hu, R. Z., Alexander, M., Wallace, J., Kagele, D., Petersen, C., et al. (2015). MicroRNA-146a constrains multiple parameters of intestinal immunity and increases susceptibility to DSS colitis. Oncotarget 6, 28556–28572. doi: 10.18632/oncotarget.5597
Rustom, A., Saffrich, R., Markovic, I., Walther, P., and Gerdes, H. H. (2004). Nanotubular highways for intercellular organelle transport. Science 303, 1007–1010. doi: 10.1126/science.1093133
Schmidt, O., and Teis, D. (2012). The ESCRT machinery. Curr. Biol. 2, R116–R120. doi: 10.1016/j.cub.2012.01.028
Scott, D. D., and Norbury, C. J. (2013). RNA decay via 3' uridylation. Biochim. Biophys. Acta 1829, 654–665. doi: 10.1016/j.bbagrm.2013.01.009
Shabalina, S. A., and Koonin, E. V. (2008). Origins and evolution of eukaryotic RNA interference. Trends Ecol. Evol. 23, 578–587. doi: 10.1016/j.tree.2008.06.005
Sharma, U., Conine, C. C., Shea, J. M., Boskovic, A., Derr, A. G., Bing, X. Y., et al. (2016). Biogenesis and function of tRNA fragments during sperm maturation and fertilization in mammals. Science 351, 391–396. doi: 10.1126/science.aad6780
Singh, N., Shirdel, E. A., Waldron, L., Zhang, R. H., Jurisica, I., and Comelli, E. M. (2012). The murine caecal microrna signature depends on the presence of the endogenous microbiota. Int. J. Biol. Sci. 8, 171–186. doi: 10.7150/ijbs.8.171
Siomi, H., and Siomi, M. C. (2009). RISC hitches onto endosome trafficking. Nat. Cell Biol. 11, 1049–1051. doi: 10.1038/ncb0909-1049
Skog, J., Noerholm, M., Bentink, S., Romain, C., Fishbeck, J., Sinclair, I., et al. (2013). Development of a urine microvesicle/exosome RNA biomarker panel to identify prostate cancer. Mol. Cancer Ther. 12:e8. doi: 10.1158/1535-7163.TARG-13-B34
Skog, J., Wurdinger, T., van Rijn, S., Meijer, D. H., Gainche, L., Sena-Esteves, M., et al. (2008). Glioblastoma microvesicles transport RNA and proteins that promote tumour growth and provide diagnostic biomarkers. Nat. Cell Biol. 10, 1470–1476. doi: 10.1038/ncb1800
Sowinski, S., Jolly, C., Berninghausen, O., Purbhoo, M. A., Chauveau, A., Kohler, K., et al. (2008). Membrane nanotubes physically connect T cells over long distances presenting a novel route for HIV-1 transmission. Nat. Cell Biol. 10, 211–219. doi: 10.1038/ncb1682
Squadrito, M. L., Baer, C., Burdet, F., Maderna, C., Gilfillan, G. D., Lyle, R., et al. (2014). Endogenous RNAs modulate microRNA sorting to exosomes and transfer to acceptor cells. Cell Rep. 8, 1432–1446. doi: 10.1016/j.celrep.2014.07.035
Stoorvogel, W., Kleijmeer, M. J., Geuze, H. J., and Raposo, G. (2002). The biogenesis and functions of exosomes. Traffic 3, 321–330. doi: 10.1034/j.1600-0854.2002.30502.x
Tanchak, M. A., and Fowke, L. C. (1987). The morphology of multivesicular bodies in soybean protoplasts and their role in endocytosis. Protoplasma 138, 173–182. doi: 10.1007/BF01281026
Thakur, N., Upadhyay, S. K., Verma, P. C., Chandrashekar, K., Tuli, R., and Singh, P. K. (2014). Enhanced whitefly resistance in transgenic tobacco plants expressing double stranded RNA of v-ATPase A gene. PLoS ONE 9:e87235. doi: 10.1371/journal.pone.0087235
Tilg, H., Moschen, A. R., and Kaser, A. (2009). Obesity and the microbiota. Gastroenterology 136, 1476–1483. doi: 10.1053/j.gastro.2009.03.030
Trajkovic, K., Hsu, C., Chiantia, S., Rajendran, L., Wenzel, D., Wieland, F., et al. (2008). Ceramide triggers budding of exosome vesicles into multivesicular Endosomes. Science 319, 1244–1247. doi: 10.1126/science.1153124
Tsui, N. B., Ng, E. K., and Lo, Y. M. (2002). Stability of endogenous and added RNA in blood specimens, serum, and plasma. Clin. Chem. 48, 1647–1653.
Turchinovich, A., Weiz, L., Langheinz, A., and Burwinkel, B. (2011). Characterization of extracellular circulating microRNA. Nucleic Acids Res. 39, 7223–7233. doi: 10.1093/nar/gkr254
Valadi, H., Ekstrom, K., Bossios, A., Sjostrand, M., Lee, J. J., and Lotvall, J. O. (2007). Exosome-mediated transfer of mRNAs and microRNAs is a novel mechanism of genetic exchange between cells. Nat. Cell Biol. 9, 654–659. doi: 10.1038/ncb1596
Van Niel, G., Mallegol, J., Bevilacqua, C., Candalh, C., Brugiere, S., Tomaskovic-Crook, E., et al. (2003). Intestinal epithelial exosomes carry MHC class II/peptides able to inform the immune system in mice. Gut 52, 1690–1697. doi: 10.1136/gut.52.12.1690
Vega-Arreguin, J. C., Jalloh, A., Bos, J. I., and Moffett, P. (2014). Recognition of an Avr3a homologue plays a major role in mediating nonhost resistance to Phytophthora capsici in Nicotiana species. Mol. Plant Microbe Interact. 27, 770–780. doi: 10.1094/MPMI-01-14-0014-R
Villarroya-Beltri, C., Gutierrez-Vazquez, C., Sanchez-Cabo, F., Perez-Hernandez, D., Vazquez, J., Martin-Cofreces, N., et al. (2013). Sumoylated hnRNPA2B1 controls the sorting of miRNAs into exosomes through binding to specific motifs. Nat. Commun. 4:2980 doi: 10.1038/ncomms3980
Viss, W. J., Pitrak, J., Humann, J., Cook, M., Driver, J., and Ream, W. (2003). Crown-gall-resistant transgenic apple trees that silence Agrobacterium tumefaciens oncogenes. Mol. Breed. 12, 283–295. doi: 10.1023/B:MOLB.0000006805.76717.08
Vojtech, L., Woo, S., Hughes, S., Levy, C., Ballweber, L., Sauteraud, R. P., et al. (2014). Exosomes in human semen carry a distinctive repertoire of small non-coding RNAs with potential regulatory functions. Nucleic Acids Res. 42, 7290–7304. doi: 10.1093/nar/gku347
Volpe, T. A., Kidner, C., Hall, I. M., Teng, G., Grewal, S. I., and Martienssen, R. A. (2002). Regulation of heterochromatic silencing and histone H3 lysine-9 methylation by RNAi. Science 297, 1833–1837. doi: 10.1126/science.1074973
Wang, J., Silva, M., Haas, L. A., Morsci, N. S., Nguyen, K. C., Hall, D. H., et al. (2014). C. elegans ciliated sensory neurons release extracellular vesicles that function in animal communication. Curr. Biol. 24, 519–525. doi: 10.1016/j.cub.2014.01.002
Weiberg, A., Bellinger, M., and Jin, H. L. (2015). Conversations between kingdoms: small RNAs. Curr. Opin. Biotechnol. 32, 207–215. doi: 10.1016/j.copbio.2014.12.025
Weiberg, A., Wang, M., Lin, F. M., Zhao, H., Zhang, Z., Kaloshian, I., et al. (2013). Fungal small RNAs suppress plant immunity by hijacking host RNA interference pathways. Science 342, 118–123. doi: 10.1126/science.1239705
Winston, W. M., Sutherlin, M., Wright, A. J., Feinberg, E. H., and Hunter, C. P. (2007). Caenorhabditis elegans SID-2 is required for environmental RNA interference. Proc. Natl. Acad. Sci. U.S.A. 104, 10565–10570. doi: 10.1073/pnas.0611282104
Xiao, D., Ohlendorf, J., Chen, Y., Taylor, D. D., Rai, S. N., Waigel, S., et al. (2012). Identifying mRNA, microRNA and protein profiles of melanoma exosomes. PLoS ONE 7:e46874. doi: 10.1371/journal.pone.0046874
Yeom, K. H., Lee, Y., Han, J., Suh, M. R., and Kim, V. N. (2006). Characterization of DGCR8/Pasha, the essential cofactor for Drosha in primary miRNA processing. Nucleic Acids Res. 34, 4622–4629. doi: 10.1093/nar/gkl458
Yi, R., Qin, Y., Macara, I. G., and Cullen, B. R. (2003). Exportin-5 mediates the nuclear export of pre-microRNAs and short hairpin RNAs. Genes Dev. 17, 3011–3016. doi: 10.1101/gad.1158803
Younis, A., Siddique, M. I., Kim, C. K., and Lim, K. B. (2014). RNA Interference (RNAi) Induced gene silencing: a promising approach of hi-tech plant breeding. Int. J. Biol. Sci. 10, 1150–1158. doi: 10.7150/ijbs.10452
Yuyama, K., Sun, H., Mitsutake, S., and Igarashi, Y. (2012). Sphingolipid-modulated exosome secretion promotes clearance of amyloid-beta by microglia. J. Biol. Chem. 287, 10977–10989. doi: 10.1074/jbc.M111.324616
Zha, W. J., Peng, X. X., Chen, R. Z., Du, B., Zhu, L. L., and He, G. C. (2011). Knockdown of midgut genes by dsRNA-transgenic plant-mediated RNA interference in the hemipteran insect Nilaparvata lugens. PLoS ONE 6:e20504. doi: 10.1371/journal.pone.0020504
Zhang, H., Xia, R., Meyers, B. C., and Walbot, V. (2015). Evolution, functions, and mysteries of plant ARGONAUTE proteins. Curr. Opin. Plant Biol. 27, 84–90. doi: 10.1016/j.pbi.2015.06.011
Zhu, J. Q., Liu, S. M., Ma, Y., Zhang, J. Q., Qi, H. S., Wei, Z. J., et al. (2012). Improvement of pest resistance in transgenic tobacco plants expressing dsRNA of an insect-associated gene EcR. PLoS ONE 7:e38572. doi: 10.1371/journal.pone.0038572
Keywords: extracellular vesicles, exosomes, miRNA, small RNA, communication, argonaute, gene silencing
Citation: Lefebvre FA and Lécuyer E (2017) Small Luggage for a Long Journey: Transfer of Vesicle-Enclosed Small RNA in Interspecies Communication. Front. Microbiol. 8:377. doi: 10.3389/fmicb.2017.00377
Received: 01 December 2016; Accepted: 23 February 2017;
Published: 16 March 2017.
Edited by:
Wolfgang R. Streit, University of Hamburg, GermanyReviewed by:
Hua Xie, Meharry Medical College, USADinesh Sriramulu, Shres Consultancy (Life Sciences), India
Copyright © 2017 Lefebvre and Lécuyer. This is an open-access article distributed under the terms of the Creative Commons Attribution License (CC BY). The use, distribution or reproduction in other forums is permitted, provided the original author(s) or licensor are credited and that the original publication in this journal is cited, in accordance with accepted academic practice. No use, distribution or reproduction is permitted which does not comply with these terms.
*Correspondence: Eric Lécuyer, RXJpYy5MZWN1eWVyQGlyY20ucWMuY2E=